- 1Department of Basic Medical Sciences, College of Medicine, University of Sharjah, Sharjah, United Arab Emirates
- 2Department of Clinical Sciences, College of Medicine, University of Sharjah, Sharjah, United Arab Emirates
- 3Meakins-Christie Laboratories, Research Institute of the McGill University Health Center, Montreal, QC, Canada
Colorectal cancer (CRC) is among the topmost malignancies for both genders. Despite the high incidence rate and advances in diagnostic tools, treatment in many cases is still ineffective. Most cancerous lesions in CRC begin as benign, followed by the development of invasive forms and metastases. The development of CRC has been linked to defects in autophagy, which plays both a pro-and anti-tumor role and is mainly context-dependent. Autophagy suppression could enhance apoptosis via p53 activation, or autophagy also promotes tumor progression by maintaining tumor growth and increasing resistance to chemotherapy. Autophagy promotes the invasion and metastasis of CRC cells via increased epithelial-mesenchymal transition (EMT). Moreover, dysbiosis of gut microbiota upregulated autophagy and metastasis markers. Autophagy responses may also modulate the tumor microenvironment (TME) via regulating the differentiation process of several innate immune cells. Treatments that promote tumor cell death by stimulating or inhibiting autophagy could be beneficial if used as an adjunct treatment, but the precise role of various autophagy-modulating drugs in CRC patients is needed to be explored. In this article, we present an overview of the autophagy process and its role in the pathogenesis and therapeutic resistance of CRC. Also, we focused on the current understanding of the role of the EMT and TME, including its relation to gut microbiota and immune cells, in autophagic manipulation of CRC. We believe that there is a potential link between autophagy, TME, EMT, and drug resistance, suggesting that further studies are needed to explore this aspect.
Introduction
Colorectal cancer (CRC) is one of the most common malignancies in men and women, and the rate of occurrence is on the rise among young adults (1). Despite the significant attention directed at developing therapeutic and screening strategies to prevent CRC, it remains a serious disease. CRC progression from adenoma to carcinoma is a multi-stage process in which many genomic pathways play a role. At least three pathways have been identified as contributing to CRC origin and progression: Chromosomal Instability (CIN); and CpG Island Methylator Phenotype (CIMP); Microsatellite Instability (MSI). The CIN pathway is the most common cause of CRC, accounting for 85% of all. CIN-associated tumors are characterized by mutations in the adenomatous polyposis coli (APC) gene and are considered one of the earliest genetic events in colorectal cancer (2). In nearly all cases of CIN-associated tumors, Wnt signaling is activated, a crucial regulator of intestinal epithelial proliferation. CIMP is another major pathway that contributes to CRC. About 20-30% of total CRCs are CIMP-positive cancers. Most of the CIMP-related tumors are found in the proximal colon, but only 3-12% are found in the distal colon, and they are predominantly found in women. A CIMP is characterized by hypermethylation of CpG island sites that result in the inactivation of multiple cancer-related genes (3). About 15% of all CRCs demonstrate high levels of MSI. Tumors associated with the MSI pathway have defects in the MMR system due to mutations or epigenetic silencing of MMR genes, such as MLH1, MSH2, MSH6, or PMS2. This results in DNA mismatch errors within microsatellite regions. Lynch syndrome, a form of inherited cancer that accounts for three percent of all CRCs, is also associated with a germline mutation in MMR genes (MLH1, MSH2, and MSH6, PMS2) (4, 5). MMR mutations cause a hallmark phenotype known as microsatellite instability (MSI), which is characterized by changes in the length of the tandem repeat genome (6). CRCs with MSI are characterized by distinct pathological features, such as the predominance of proximal colon and/or mucinous histology as well as an increased number of lymphocytes infiltrating the tumor (7, 8). Most CRC lesions begin as benign due to mutations followed by the development of invasive forms and metastases (9). In addition to preventing these lesions from turning malignant, early detection of such benign lesions allows clinicians to halt cancer progression through therapeutic interventions (10). Currently, metastatic CRC is incurable, and the new treatment regimens focus primarily on relieving symptoms and reducing the disease progression. Therapeutic modalities for CRC include surgery, radiotherapy, chemotherapy, targeted therapy, and immunotherapy (11). Despite many advances in the availability of new diagnostic and treatments for metastatic CRC, the median survival time remains below 30 months for most patients (12). Several diseases including CRC, have been linked to defects in autophagy since its role in tumor formation is vastly complex and depends on several biological aspects. Tumorigenic and anti-tumorigenic autophagy plays a dichotomous role in cancer development. Alternative therapies like targeting autophagy may prove helpful in this regard because they can stimulate or inhibit autophagy. Subsequently, upregulation of autophagy by cancer therapies can either promote tumor cell survival or oppositely cause tumor cell death. Given this, a growing body of studies focuses on the autophagy pathway and its potential role in the pathogenesis of CRC. In the present review, we focus on the role of autophagy in the pathogenesis and therapeutic resistance of CRC.
Introduction to Autophagy Process
Autophagy is a physiological process that maintains metabolism and cellular homeostasis by recycling damaged cellular components. Depending on the mode of cargo delivery into the lysosomes, autophagy can be classified into three types: chaperon-mediated autophagy (CMA), microautophagy, and macroautophagy. Interestingly all autophagic pathways end up in the lysosome, but each of them differs in the regulatory mechanism and the circumstances under which it is activated. CMA occurs when a specific protein having a “KFERQ” motif is recognized by heat shock cognate 71 kDa protein (HSC70), a cytoplasmic chaperone is delivered directly to the lysosome followed by lysosomal membrane translocation through interactions with lysosomal-associated membrane protein 2 (LAMP2). To date, CMA has only been associated with aging, neurodegenerative diseases, and glioblastoma (13), but its role in CRC was not reported. In microautophagy, the lysosome directly invaginates its membrane to engulf cytoplasmic material when the cell is under starvation conditions (14). Thirdly, macroautophagy is the best-studied form of autophagy and the changes associated with it have been extensively documented in cancer research (15). Macroautophagy is a multistep process including initiation, nucleation, elongation, maturation, fusion, and degradation. In the past few years, a wide array of inhibitors of the autophagy pathway have been developed. (Figure 1). A family of autophagy-related (ATG) genes regulates this form of autophagy (Table 1).
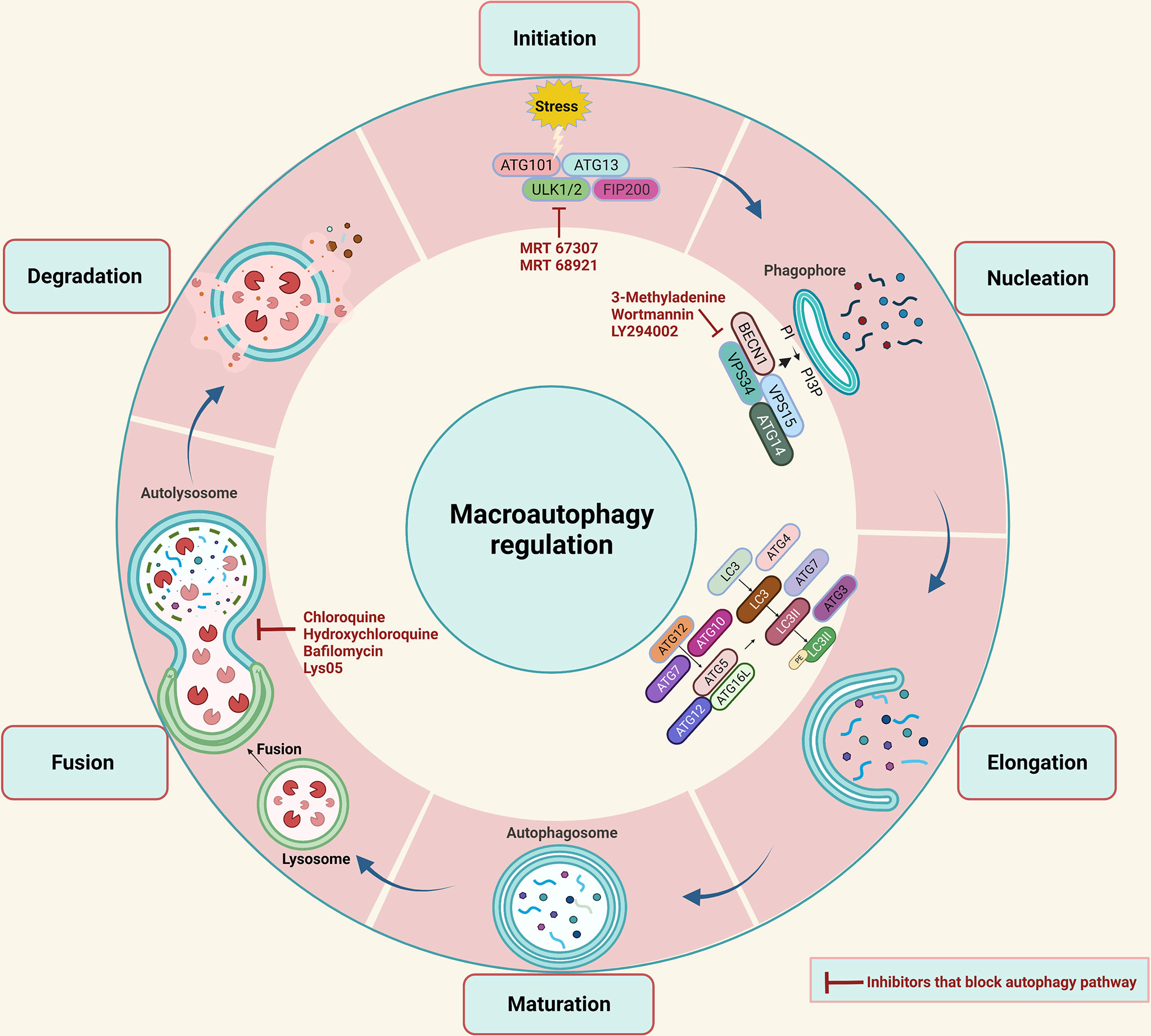
Figure 1 A diagrammatic representation of the macroautophagy (also known as autophagy) process is shown. A phagophore forms in autophagy that sequester cytoplasmic material in a vesicle that later matures into an autophagosome, which then fuses with lysosomes to form an autolysosome, where the sequestered material is degraded. The autophagy process takes place in several steps such as Initiation, Nucleation, Elongation, Maturation, Fusion, and Degradation. The autophagy process can be blocked at various steps with the help of autophagy inhibitors listed in the red color.
In this process, autophagy initiation requires the translocation of the ULK1 complex consisting of ULK1, FAK family kinase interacting protein of 200 kDa (FIP200), ATG13, and ATG101 to the site of phagophore initiation, where dephosphorylation will cause the activation of this complex (16). Subsequently, the activated ULK1 complex acts as a recruitment scaffold for the class III phosphatidylinositol 3-kinase (PI3K) complex, including vacuolar protein sorting 34 (VPS34), Beclin-1, VPS15, and ATG14L-like. In response, phospholipid 3-phosphate (PI3P) is produced and is accumulated on the phagophore membrane by activated class III PI3K. This is followed by the recruitment of ATG proteins such as WD repeat domain, phosphoinositide interacting 1 (WIPI1), and WIPI2. As the phagophore elongates into a double-membrane structure, autophagosomes are formed. Two pathways of ubiquitin-like conjugation, the ATG12 conjugation system, and the ATG8 conjugation system, are essentially required for the phagophore elongation step. The ATG12 conjugation mechanism relies on ATG7 and ATG10 to help conjugate ATG12 to ATG5, which then pairs with ATG16 to produce the ATG12-ATG5-ATG16 conjugate, which is necessary for the expanding phagophore’s curvature. The ATG12-ATG5-ATG16 complex dissociates from the membrane as the autophagosome is formed, making it a suitable marker for early autophagic events (17).
Meanwhile, the ATG8 conjugation system results in the LC3 proteolytic activation. When the autophagy is initiated, LC3B is cleaved by ATG4 to form LC3B-I, which interacts with ATG7 in an ATP-dependent manner to activate LC3-I. The latter conjugates with phosphatidylethanolamine (PE) phospholipid by ATG7 and ATG3 along with the ATG12-ATG5-ATG16 complex to form LC3-II. Consequently, the membrane of the phagophore is expanded and closed. ATG8 is released from autophagosomes following elongation and maturation through deconjugation by ATG4. Afterward, autophagosomes and lysosomes merge to form an autolysosome where acidic hydrolases and lipases degrade the sequestered material to recycle it for cellular metabolism and homeostasis (18).
Several studies have suggested a complex role of autophagy in cancer development and progression. It is believed that early-stage autophagy prevents cancer development. However, when cancer has progressed, the autophagy process increases tumor cells’ ability to grow and adapt to adverse environments (19–22).
Role of Autophagy in CRC
The autophagy pathway plays both a pro-and anti-tumor role in colon cancer and its function varies depending on biological factors such as genetic mutations, tumor type, and tumor stage (23–25). It is well known that autophagy processes can restore cellular homeostasis and prevent malignant transformation by clearing damaged organelles and foreign bodies. It also promotes tumor progression by maintaining cell stability, increasing resistance to unfavorable environments, and maintaining tumor growth (26). In CRC autophagy modulation has recently been investigated (27). Table 2 summarizes several autophagy regulatory factors that are implicated in CRC carcinogenesis and therapeutic resistance.
A recent study demonstrated that CRC cell lines have a diverse sensitivity to autophagy modulators (65). Studies indicate that autophagy contributes to the early stage of CRC development, suggesting an important role of autophagy in CRC (36). It is now becoming clear that autophagy is involved in CRC development and progression, leading to a new insight into CRC therapeutic strategies using autophagy as a target. A study in CRC patients found LC3B expression is significantly co-related to aggressive tumor phenotype suggesting autophagy involvement in tumor promotion (28). One study showed that 38% of CRC patients had high LC3B levels and that LC3B was also significantly correlated with lymphatic invasion and p53 mutations (29). In regards to the CRC subtypes, the level of LC3B-II expression was higher in microsatellite stable (MSS) than that in MSI carcinomas (66). Immunohistochemistry of 68 patients with CRC revealed a significant correlation between Beclin 1 levels and those of LC3 and 4E-BP1 and overexpression of LC3 in tumor tissues compared to normal tissues (30). In another study done on 155 CRC patients’ overexpression of Beclin 1, a scaffold for the formation of autophagosomes was linked to tumor aggressiveness. However, patients with extensive over-or under-expression of Beclin 1 had a significantly poorer overall survival compared with the other two groups suggesting a dual role of Beclin 1 in CRC (31). In regards to the CRC subtypes, Wang et al., found that the expression of Beclin 1 and LC3 was unrelated to all clinicopathological parameters and overall survival in the MSI-H-CRC subgroup (67). Patients with colon cancer treated with adjuvant 5-FU who overexpressed Beclin 1 had a reduced survival rate, indicating autophagy’s role in chemoresistance (32). The inhibition of autophagy through genetic ablation of ATG5 inhibits tumor growth in colon cancer-derived cells and CRC patient-derived enteroid models (33). In a study involving patients and patient samples, LAMP3, a member of the family of autophagy-related proteins, is found to be higher in colorectal adenocarcinoma cells than in non-cancerous cells which is related to the stage of cancer (34, 35). In colon cancer samples, miR-502 expression was downregulated, suggesting it could serve as a tumor suppressor. Moreover, miR-502 inhibited autophagy in colon cancer cells suggesting that the effect of miR-502 has on colon cancer cell growth is partially due to the disruption of autophagy (62). The deficiency of ATG7 induced metabolic defects, an AMPK-mediated cell-cycle arrest, and AMPK activation in tumor cells but not in normal tissue (36). It was also found that ATG7 was crucial for the viability of CRC cells, and its inhibition induced cell death via activating apoptosis. Further, it exerted synergistic effects when combined with conventional chemotherapy (37). Both the autophagy markers, ATG5 and ATG 7, are known for their complex roles in other cancers (19); however, we found strong evidences supporting their pro-tumorigenic roles in CRC and targeting these genes could lead to enhanced antitumor responses (33, 36).
Survival rates were significantly higher for patients without ATG10-expressing tumors than those with ATG10-expressing tumors. Additionally, tumor invasion and metastasis are associated with the expression level of autophagy-related protein ATG10 (44). As colonic epithelial carcinogenesis progresses, RACK1 (receptor of activated kinase 1) increases remarkably and is positively associated with aggressiveness and negatively associated with patient survival. Further mechanistic analysis revealed that induction of autophagy by RACK1 led to proliferation and inhibition of apoptosis in colon cancer cells (38). Mutations in KRAS are known to be one of the most common causes of CRC (68, 69). In regards to the CRC subtypes, KRAS is found mutated in a substantial number of cases in CRCs, with a particular increased prevalence in MSS, however this prevalence decreases if only MSI CRC cases are studied (66). Through the MEK/ERK pathway, KRAS activating mutations increase autophagy in CRC cells, contributing to their survival under starvation conditions (23). This suggests that colorectal cancers resulting from KRAS mutations may require autophagy to survive. FOXO3A (Forkhead box O3), a member of the forkhead family of transcription factors, has been shown to activate apoptosis via upregulation of apoptotic genes and downregulation of antiapoptotic genes (70). In the absence of FOXO3A, cells may be more resistant to apoptosis and cell-cycle progression (71). In CRC cells, an increase in FOXO3A due to autophagy impairment caused an increase in transcription of a proapoptotic gene such as BBC3/PUMA, which resulted in apoptosis sensitization (72). In colon cancer cells, autophagy suppression enhanced apoptosis through p53 and UPR activation, resulting in antitumor effects (73). In vitro and in vivo findings in CRC cells suggest that autophagy inhibitors can significantly improve sinoporphyrin sodium-mediated photodynamic therapy-mediated anticancer activity (74). Hypoxia-induced autophagy plays a crucial role in the initiation and progression of CRC (75). Autophagy was found to be a mechanism through which long non-coding RNA urothelial carcinoma-associated 1 (UCA1) suppressed CRC cell growth. Autophagy inhibition by UCA1 suppresses cell proliferation and promotes apoptosis (63).
On the other hand, autophagy has been shown to reduce tumor development, supporting its tumor-suppressing role in cancer. Increased Beclin 1 expression was higher in CRC tissues than in normal tissues, and in CRCs, it was shown to be an important prognostic factor for overall survival and disease-free survival (51). In another study involving CRC patients, a negative correlation was seen between Beclin 1 protein expression and liver, whereas in distant metastasis there was no correlation with age, sex, depth of invasion, lymphatic or venous invasion, lymph node metastasis, tumor-node-metastasis staging, or differentiation (52). An additional study showed LC3 was suppressed in colorectal cancers along with a reduction in the expression of Beclin 1. Furthermore, in vitro experiments showed that overexpression of Beclin 1 inhibited CRC cell growth and further enhanced the antitumor potency of rapamycin (53). Interestingly, loss of various autophagy-related proteins such as Beclin1, LC3B and ATG5 is associated with poor clinical outcomes in CRC, and the prognostic impact of these proteins does not seem to be dependent upon already established clinicopathological parameters (54). The effects of silencing LETM1 (Leucine zipper-EF-hand-containing transmembrane protein 1), a protein overexpressed in CRC tissues compared to normal tissues were studied and were found to suppress cancer stemness and proliferation. Analysis of the autophagic response in cells revealed an increase in Beclin1 expression and a higher ratio of MAP-LC3II/I, suggesting an enhanced autophagic response after silencing LETM1 (50). A study found a down-regulation of ATG5 in CRC patients, but its increased expression was associated with lymph node infiltration (76). Using ATG5-deficient and Beclin1 knockout CRC cells, it was observed that the potency of niclosamide, an inhibitor of Wnt signaling was dependent on autophagy. Niclosamide inhibited mTORC1 and ULK1 activity and stimulated autophagy, making CRC cells sensitive to treatment (77). Through ROS/JNK signaling, photodynamic therapy induces autophagy-mediated cell death in human CRC, and knockdown of ATG5 or ATG7 inhibited the apoptosis of CRC cells by inhibiting the autophagic response after photodynamic therapy (78). In addition, it has been demonstrated that ERK inhibitors induce autophagy in CRC cells by activating ROS/p53 and cell death simultaneously (79). In CRC cells, hindering activated ROS-mediated mitochondrial signaling and AMPK/mTOR signaling pathways causes apoptosis and autophagy (64).
Role of Autophagy in EMT and Metastasis
The epithelial-mesenchymal transformation (EMT) is a progressive process that allows epithelial cells to acquire mesenchymal characteristics. This gives epithelial cells properties such as motility and metastatic potential (80). Studies in recent years have shown that EMT plays a pivotal role in cancer cell invasion, metastasis, and drug resistance (81, 82). In this section, we will discuss recent evidence suggesting autophagy is involved in CRC cell plasticity and metastasis. EMT largely contributes to the pro-metastatic function of tumors, and studies indicate that there are several interactions between autophagy and EMT-associated signaling pathways. Consequently, inhibiting autophagy to control EMT development would be a promising strategy for CRC treatment (83). To date, the relationship between autophagy and EMT in tumors is not completely understood. Autophagy is involved in the metastasis of cancer cells as a double-edged sword. In the early stages, it inhibits cancer cells from spreading, but it promotes cancer cell survival and proliferation during advanced stages. During metastasis, cells must detach from the extracellular matrix, enter the bloodstream, and overcome environmental stresses such as hypoxia and nutrient deprivation. Due to the uncontrolled cell proliferation that outstrips the oxygen supply, tumors are gradually exposed to hypoxia, leading to tumor progression (84). It is well established that autophagy induction is one of the responses of cancer cells to hypoxia, and it is induced by hypoxia-inducible factor 1-alpha (HIF-1α). As hypoxia stimulates autophagy in cancer, blocking autophagy reduces the likelihood of metastatic and invasive progress (85). Hypoxia in CRC controls the EMT through various indirect mechanisms, for instance, gene associated with retinoid‐interferon‐induced mortality‐19 (GRIM‐19), a cell death regulatory protein known to act as a tumor suppressor (Figure 2) (86). GRIM‐19 expression was downregulated in CRC patients suggesting its favorable role in CRC carcinogenesis (57, 58). Also, it was shown to increase apoptosis, reduce invasion, and reduce migration in CRC (87). Recently GRIM‐19 was demonstrated to suppress EMT and the subsequent invasion through inhibition of hypoxia-dependent autophagy (59). However, it is not known if hypoxia-induced autophagy upregulates itself by degrading GRIM-19. Silent mating type information regulation 1 (SIRT1) an autophagy-related protein is associated with poor prognosis, tumor invasiveness, and metastasis in CRC patients (39–42). Recently, it was shown under hypoxic conditions SIRT1 modulates migration and invasion (88). Whether SIRT1-dependent autophagy has a role in CRC EMT is still not known.
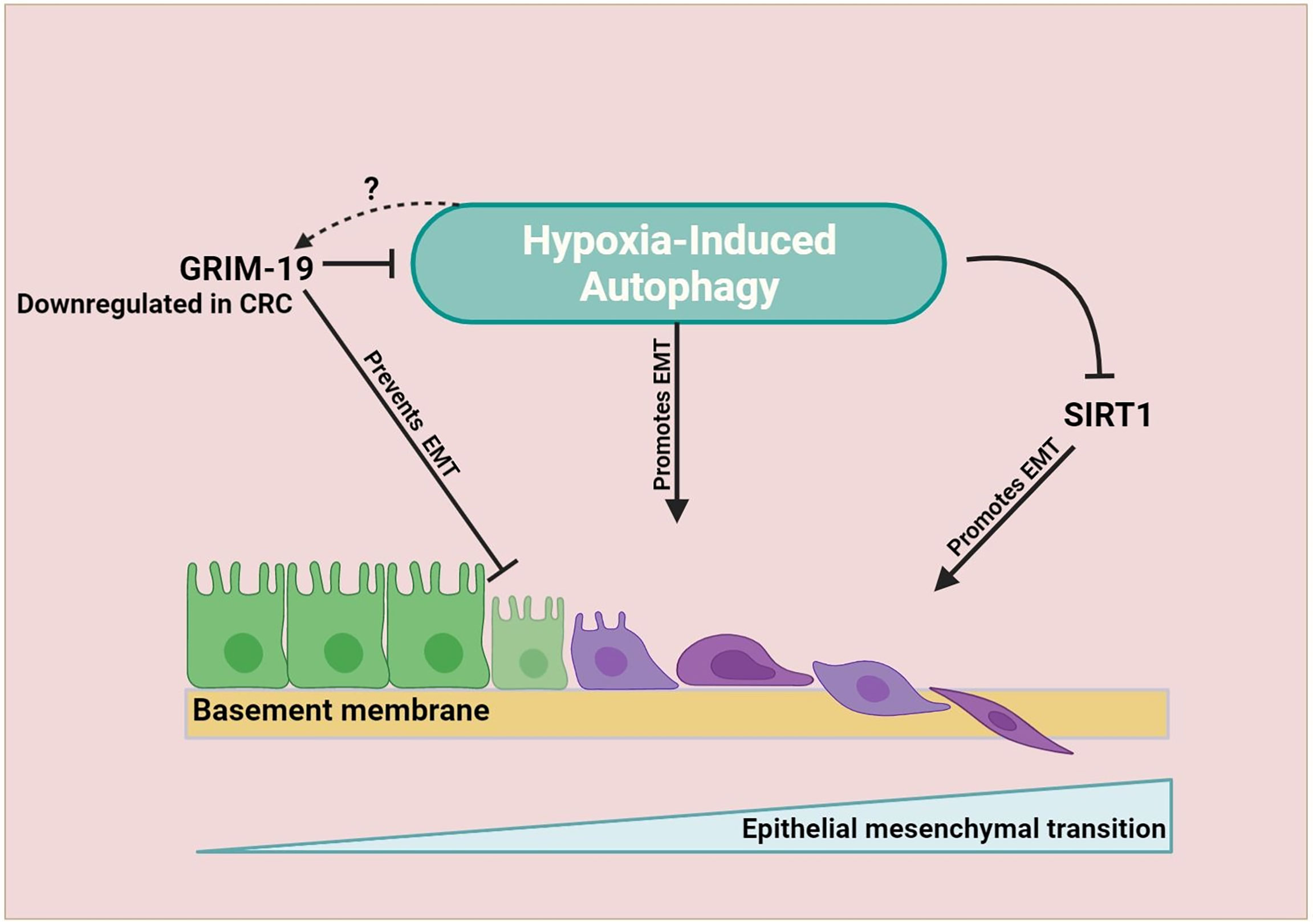
Figure 2 EMT is promoted by hypoxia-dependent autophagy in CRC. As the tumor progresses, hypoxia becomes more prevalent. A growing tumor triggers autophagy as an adaptive survival mechanism. Autophagy increases the plasticity of tumor cells, causing them to acquire mesenchymal characteristics. In CRC hypoxia-induced autophagy target SIRT1 promotes EMT process and hypoxia-induced autophagy inhibition by GRIM-19 prevents EMT. However, whether hypoxia-induced autophagy can upregulate itself by downregulating the expression of GRIM-19 is not known.
ATG10 is an E2-like enzyme that is important for autophagosome formation. Its overexpression is associated with lymphovascular invasion and lymph node metastasis in CRC (44). Sphingosine kinase 1 (SphK1) is an enzyme that phosphorylates sphingosine to sphingosine-1-phosphate (SIP), which regulates proliferation and survival (89). Earlier, a study showed SphK1 modulated EMT markers in CRC and its inhibition reduced cancer cell migration (90). In support of this, a study published recently demonstrated that SphK1 stimulates autophagy in CRC cells and that autophagy driven by SphK1 may promote the invasion and metastasis of CRC by promoting the expression of focal adhesion paxillin (45). In another finding, CRC cells were protected from EMT by inhibiting autophagy. Through the inhibition of Beclin 1, expression of Twist and Vimentin (a mesenchymal marker) were decreased (91). Sex-determining region Y-box2 (SOX2) a transcriptional factor is associated with multidrug resistance genes and accelerated CRC progression (92). Recently it was found to promote malignant CRC phenotype through upregulated transcriptional activation of Beclin 1 (46). MicroRNA has-miR-140-5p was reported to be weakly expressed in metastatic tissues compared to normal mucosa. There was evidence that the hsa-miR-140-5p reduced CRC progression and metastasis through suppression of autophagy (60). Dysbiosis of gut microbiota is often associated with cancer (93, 94). Certain microbes can induce inflammation and promote tumorigenesis, such as Fusobacterium nucleatum (F. nucleatum). Recently it was reported that F. nucleatum is highly present in patients with metastatic CRC. Further investigations in mice and cell lines models revealed F. nucleatum infection upregulated autophagy and metastasis markers such as LC3-II, Beclin1, and Vimentin and lower levels of P62 and E-cadherin. Autophagy inhibitor treatment reversed the effects showing that F. nucleatum could promote metastasis in CRC via upregulation of autophagy (95).
In contrast, various studies reported the advantage of autophagy in protecting CRC cells against EMT and metastasis. In one study, autophagy inhibition in CRC cells was found to promote invasion and metastasis, which was further confirmed by an increase in metastasis markers such as N-cadherin, Snail family transcriptional repressor (SNAIl), and Twist Family BHLH Transcription Factor 1 (Twist1) proteins (96, 97). Similarly, in Ras-mutated HCT116 CRC cell line, a knockdown of ATG 5 upregulated EMT markers such as zinc finger box binding homeobox 1 (ZEB1), and SNAI2 (56). In addition to this, treatment of CRC cell lines with autophagy promotor Torin1 increased LC3 levels and downregulated Twist1 expression. Treatment with autophagy inhibitor compound MHY1485 increased p62 levels and upregulated the expression of Twist1 (98). Beta-catenin has a central role in directing several developmental processes. Wnt/β-catenin signaling pathway is crucial for various cellular functions and is abnormally activated in most colorectal cancers. Through the degradation of crucial mediators of Wnt signaling such as β-catenin, autophagy blocks EMT (99). A study found long intergenic noncoding RNAs (linc-POU3F3) control the CRC cell apoptosis, migration, and invasion via autophagy manipulation. Linc-POU3F3 is highly expressed in CRC and is correlated with tumor aggressiveness. Furthermore, in vitro studies showed inhibition of linc-POU3F3 induced cell cycle arrest and simultaneously increased autophagy (47). The mitochondrial serine protease Lactamase Beta (LACTB) is expressed in the CRC patient samples weakly, compared to the non-malignant CRC patient samples. Further in vivo and in vitro investigations demonstrated that LACTB knockdown inhibits autophagy as evident by lower LC3-II/LC3-I ratio, and induction of proliferation, invasion, migration, and EMT, whereas overexpression of LACTB has the opposite effect (61).
Autophagy activation was found to attenuate the migration of CRC cells (100). Yet another study reported that Beclin 1 overexpression prevented invasion and migration of aggressive CRC cells suggesting autophagy activation may reverse the aggressive cancers (55). Pleckstrin homology-like domain family A member 2 (PHLDA2) promotes tumorigenesis in CRC. PHLDA2 knockdown in vivo inhibits tumor aggressiveness and EMT and subsequently, increases autophagy (48). Long non-coding RNA RAMS11 was highly expressed in CRC cell lines and was associated with cancer aggressiveness. Further investigation showed that silencing of RAMS11 prevented EMT and stimulated autophagy via the mTOR-dependent pathway (49)
Autophagy and Tumor Microenvironment
A solid tumor is highly heterogeneous at both cellular and acellular levels. The cellular components include mesenchymal cells, immune cells, and endothelial cells. On the other hand, the acellular components include extracellular matrix proteins, such as collagen, elastin, fibronectin, laminin, and secretory proteins, including cytokines, chemokines, proteases, growth factors, and metabolites (101). Cancer cells cause significant molecular, cellular, and physical changes in the surrounding tissues to promote tumor growth and progression. The TME is a complex and continuously evolving entity that differs between tumor types but is characterized by presence of immune cells, stromal cells, blood vessels, and extracellular matrix. It is proposed that the TME is not innocent during cancer progression but rather a decisive factor encouraging tumor growth. A potent complementarity emerges between cancer cells and cellular and acellular components of the TME during the early stages of tumor formation that aids tumor cell survival, invasion, and metastasis. Consequently, TME meshes a mechanism that promotes angiogenesis to restore oxygen and nutrient supply (102). In this section, we will discuss the main components of the TME, such as cancer-associated fibroblast cells, endothelial cells, and immune cells in the context of autophagy.
Cancer-associated fibroblasts (CAFs) are the most abundant types of mesenchymal cells in the tumor stroma that contribute to tumor initiation, immune evasion, and metastasis (103). According to a 2010 study, co-culturing CAFs with cancer cells activates hypoxia-induced autophagy in the CAFs, preventing apoptosis in cancer cells (104). Based on this, a model called “the autophagic tumor stroma model of cancer” was proposed. According to this model, autophagy activation in CAFs facilitates cancer cells’ survival and aggressiveness by providing them with recycled nutrients and building blocks. Nevertheless, another study showed that stromal fibroblast cells were essential for the metabolic reprogramming of CRC cells because they can induce oxidative stress in fibroblasts leading to changes in their metabolism. Oxidative stress and autophagy inhibitors could suppress the metabolic reprogramming between tumor cells and fibroblasts (105). Recently microRNA has been shown to play a pivotal role in cancer, exerting a regulatory effect on autophagy. In the development of CRC, miR-31 plays an important role in regulating autophagy by targeting various genes. Inhibition of miR-31 affects colon cancer by increasing autophagy in the CAFs of these cells, promoting proliferation, invasion, and metastasis in a co-culture system, as well as increasing cancer cells’ radiosensitivity (106).
Furthermore, loss of tumor protein p53, an oncogene, promotes the activation of surrounding fibroblasts into carcinoma-associated fibroblasts through the suppression of autophagy. It was found that exosome-derived miR-1434 from TP53-inactivated CRC cells interact with normal fibroblasts to suppress autophagy by targeting intracellular ATG2B, leading to fibroblast activation and induction of CRC cell proliferation (107). In contrast, another study demonstrated cancer-associated fibroblast-derived secretory mediator induced CRC cell proliferation, invasion, and migration by reducing autophagy (108). Several studies show that autophagy plays a role in tumor vasculature function. Endothelial cells in the adverse TME are deprived of nutrients resulting in hypoxia due to the restricted blood supply in solid tumors. This causes damage to tumor blood vessels, which become permeable and more fragile than normal blood vessels (109). There is mounting evidence suggesting that autophagy is important for endothelial cell homeostasis under physiological and pathological conditions, but whether autophagy plays a positive or negative role in the regulation of angiogenesis is still not clear (110, 111). It was observed that SIRT1 stimulates autophagy in tumor stroma under oxidative stress conditions, which aids endothelial cell survival (112). CRC-derived exosomes interfere with vascular endothelial barriers which stimulate angiogenesis and vascular permeability by transferring exosomes to the endothelial cells (113).
Microbiota Induces Autophagy in CRC
Several studies have demonstrated that microorganisms may also contribute to the initiation and progression of cancer (114). Different types of cancer including CRC, are associated with microbial infections (115–119). Despite this, the correlation between cancer and microbiota is still unclear. As part of intestinal homeostasis, autophagy ensures intracellular defenses against microbes, maintains the integrity of secretory granules in Paneth cells, and mediates antigen presentation (120). Furthermore, mice models of CRC have demonstrated that gut microbiota plays a vital role in cancer development. It was found that about 80% of CRC cases are caused by the APC regulator of Wnt signaling pathway gene mutation (121). One of the best-known CRC models is ApcMin/+ mice bearing a loss-of-function mutation in the Apc gene (122). When compared with ApcMin/+ mice bearing microbiota, germ-free ApcMin/+ mice displayed a lower rate of intestinal and colorectal tumors (123). Microbiota in the gut is associated with cancer, particularly F. nucleatum. F. nuclearum induces chemoresistance by activating autophagy in CRC cells. F. nucleatum appears to be a driver of colorectal carcinogenesis since it is associated with a poor prognosis in CRC patients. By utilizing innate immune signaling and microRNAs, F. nucleatum altered chemotherapeutic responses for CRC (124). The crosstalk among microbiota and autophagy needs to be explored further to map the correlation better and to determine how they lead to cancer progression and whether probiotics could have potential to be used as a therapy to treat CRC remains an open question.
Role of Autophagy in Immune Cells
As immune cells play a crucial role in the tumor microenvironment, an interesting paradox involves the relationship between immune cells and the tumor microenvironment, whereby immune cells can either suppress the growth of the tumor or promote it (125). Tumor response is regulated by both adaptive and innate immune cells. Innate immunity consists of non-specific responses involving macrophages, NK cells, neutrophils, and dendritic cells, while adaptive immunity is based on antigen-specific responses involving T and B cells. In the following subsections we will describe the effects of autophagy on innate and adaptive immune cells concerning CRC development and growth.
Effects on NK Cells
The autophagy process has recently been linked to tumor immune evasion in pancreatic ductal adenocarcinoma. Natural killer (NK) cells have been considered the first-line defense against CRC since they can respond to stimulation within hours of stimulation, even without prior immunization (126, 127). Peripheral blood lymphocytes with medium to high cytotoxic activity have a lower cancer risk than those with low activity (128). Additionally, decreased NK cell frequency was associated with higher cancer risk and poor clinical outcomes in multiple cancers, including CRC (129). Numerous studies have found few NK cells infiltrating cancer cells or have impaired function (130–132). Dysfunctional NK cells often result from an imbalance of stimulatory and inhibitory receptors (133). In patients with CRC, NK cells isolated from PBMCs have expressed significantly lower levels of the activating receptor NKG2D (134). The role of NK cells in the progression of CRC has been supported by the finding that patients with CRC post-operatively develop lipid accumulation, which impairs their functions and facilitates the spreading of cancer (135).
Autophagy is involved in the differentiation and memory of NK cells, but how it modulates NK cell function in the CRC TME is not well understood. A recent study showed the importance of autophagy in mature NK cells development by ablation of autophagosome machinery component ATG5 in NK cells. The same study showed that NK cell’s autophagy promotes survival by limiting cell-intrinsic apoptosis. Further, treatment with metformin, a drug known to induce autophagy, increased lymphocyte counts by activating autophagy in an ATG5-dependent manner (136). According to a recent study, autophagy contributes to NK cell’s viability by removing damaged mitochondria and intracellular ROS. Further, the study reported that phosphorylated FoxO1 of NK cells interact with ATG7 on phagophores, causing autophagy and that FoxO1 deficiency undermines autophagy initiation which may impede NK cells development. This indicates that FoxO1-mediated autophagy is required for NK cells development (137). In support of this, another study showed that autophagy regulates NK cells’ survival and immunological memory. The same study showed that pharmacological activation of autophagy increased the number of memory NK cells through a mechanism that involves ATG3. This autophagy mechanism is important during the transition from effector cells to memory NK cells (138). The effect of autophagy activation and inhibition on NK cells infiltration into tumor growth sites remains largely unknown. However, limited evidence suggests inhibition of the autophagy protein Beclin1 in melanoma cells stimulates the infiltration of effector NK cells into the tumor bed through CCL5-dependent mechanism leading to the suppression of the tumor (139). In vitro studies demonstrated that breast cancer cells under hypoxic conditions could evade NK cells killing by enzymatic degradation of granzyme B (140). In contrast, in normoxia, when p53 function was restored to breast cancer cells, NK cells cytotoxicity is enhanced by p53 mediated autophagic sequestration of anti-apoptotic proteins facilitating Granzyme B-mediated mitochondrial outer membrane permeabilization or NK cells induced cell death (141). It is crucial to determine the effect of autophagy on tumor cells’ susceptibility to NK cells cytotoxicity in vivo as differences in the TME could alter the ability of autophagy to inhibit and/or enhance NK cells-induced apoptosis in tumor cells.
Together, these studies suggest that autophagy may play a role in the proper development of NK cells and that autophagic responses may modulate NK cell-dependent anticancer immunity. However, whether autophagy should be activated or inhibited in cancer treatment is a topic of extensive debate, and further studies are needed to determine its role in NK cells dependent anticancer immunity.
Effects on Macrophages
Macrophages are an important member of the innate immune system since they phagocytose foreign substances and present them to the immune system. Activated macrophages are classified as M1 or M2 macrophages. M1 macrophages are primarily involved in pro-inflammatory responses, while M2 macrophages are predominantly anti-inflammatory (142). In tumors, M2 macrophages contribute to an immunosuppressive and tumor-promoting environment by secreting cytokines such as IL-10, IL-13, and TGF-β (143). The TME favors the M2 subset of macrophages through various mechanisms (144). It remains unclear what role tumor-associated macrophages (TAMs) play. Several studies have attempted to establish the association between macrophages density and CRC prognosis. Earlier research revealed a clear correlation between a high density of TAMs and a good prognosis in CRC (145–147). However, it is accepted that infiltration of M2 macrophages is associated with poor prognosis, metastasis, and tumor progression (148–151).
The results of one study demonstrated that autophagy was induced when monocytes were directed towards differentiation and that the induction of autophagy was crucial for monocytes’ differentiation into macrophages as well as for their survival (152). Additionally, inhibiting autophagy genetically or pharmacologically hinders differentiation, cytokine production, and induces apoptosis of monocytes, suggesting that autophagy is necessary for monocytes to macrophages differentiation (153). In addition, an in vivo and in vitro study demonstrated that pharmacological and genetic inhibition of autophagy prevented the differentiation of human monocytes into macrophages induced by colony-stimulating factor 1 (CSF-1) (153, 154). Yet another study involving ATG7 deficient macrophages reported that autophagy impairment caused reduced phagocytosis and respiratory burst activity. However, the same study found that macrophages could differentiate normally in the absence of autophagy (155). Another study reported that induction of mTOR-dependent autophagy was crucial for the proper differentiation of monocytes into macrophages (156).
The autophagy induction was also shown to be a critical regulator of the transition of TAMs to M2 phenotype. It was found that Chloroquine (CQ) treatment inhibits autophagy which in turn, impedes the transition of M1 into the M2 phenotype within TME (157). A study focused on the TME in colon cancer observed that co-culturing macrophages with colon cancer cells stimulated the release of epidermal growth factor (EGF) in CRC cells, which activated the EGFR/PI3K/AKT/mTOR signaling pathways, resulting in a polarization of TAMs into M2 phenotype (158). In a similar context, a study involving co-culturing macrophages with colon cancer cells demonstrated that autophagy manipulation in macrophages can affect colon cancer survival. The upregulation of autophagy in macrophages by autophagy-inducing treatments increased apoptosis in colon cancer cells and consequently, their sensitivity to radiotherapy (159).
Another study indicated that macrophages took up exosomes derived from CRC cells, which activated the PI3K/AKT pathway resulting in polarized M2 macrophages, and promoting liver metastasis (160). In addition, autophagy plays a crucial role in developing CRC in other innate immune cells, such as neutrophils. It has been found that tumor-associated neutrophils facilitate the progression of CRC, and that increasing neutrophil autophagy contributes to cancer cell migration (161, 162).
Effects on Adaptive Immune Cells
In response to antigen stimulation, T cells differentiate into effector T cells, such as Th cells (Th1, Th2, Th17), Tregs, and cytotoxic T cells. Pro-inflammatory cytokines are produced by Th1, Th2, and Th17 cells, and anti-inflammatory cytokines are produced by Tregs. Cytotoxic T cells (CTLs) kill tumor cells by releasing perforin and granzymes (163). Over the past few years, several studies have demonstrated the importance of autophagy for T-cell activation, differentiation, and homeostasis (163–171). In this regard, autophagy has a crucial role in antigen processing and presentation to T cells (172). Autophagy has been reported to enhance the adaptive immune response by facilitating MHC I or MHC II-restricted presentation and maintaining T cells survival, function, and homeostasis (173). In response to TNF-α activation, autophagy was shown to improve the processing and presentation of mitochondrial viral antigens (174).
Two decades ago, Naito et al. showed that the infiltration of tumors with CD8+ T cells positively impacts CRC prognosis. CD8+ T-cells are widely recognized for their positive prognostic role in CRC (175–177). It has been reported that autophagy enhances the adaptive immune response by improving antigen-presenting cells (APCs) recognition, presentation, and maintaining T cell homeostasis in colitis-associated CRC (178). A recent study showed CD8+ based immune activity was increased by induction of autophagy in the intestinal epithelial cells. Increased autophagy causes lysosomal membrane permeabilization, enhancing MHC I presentation and CD8+ T cell activation (179). Autophagy was also essential for Treg cell’s survival, activity, and stability (180). As a result of the ablation of autophagy genes in Treg cells, fewer cells were produced, which led to an increase in the number of effectors CD8+ and CD4+ T cells infiltrating the tumor, resulting in increased tumor resistance (181). Another study showed that autophagy inhibition of T cells suppresses tumor growth in mice challenged with MC38 colon cancer and B16 melanoma cells (182). According to one study, the infiltration of Treg cells into CRC tumors is inversely correlated with the expression of autophagy-related protein SQSTM1 (183).
Role of Autophagy in Resistance to Therapy
To prevent malignant transformation, autophagy ensures genome stability, removes abnormal organelles, and eliminates abnormal protein aggregation during the early stages of tumor formation. However, it also facilitates tumor progression by supporting tumor cell adaptation to the unwanted microenvironment. Upon chemotherapy radiotherapy or immunotherapy treatment, autophagy antagonizes, cooperates, or accompanies diverse cell death pathways.
Role of Autophagy in Therapeutic Resistance
A growing body of evidence indicates that autophagy plays an integral role in developing chemoresistance. Additionally, various studies demonstrate that inhibition of autophagy combined with anticancer drugs can enhance cancer cell cytotoxicity and reduce resistance. To date, 5-fluorouracil (5-FU), along with other drugs like oxaliplatin, remains a widely used chemotherapeutic drug for treating CRC. Recently, we reported that 5-FU treatment upregulated LC3-II, SIRT1, and other autophagy proteins, inducing nucleophagy (a form of autophagy) in microsatellite stable (MSS) CRC cell lines, leading to resistance to 5-FU treatment (43). Nevertheless, multiple studies have shown autophagy induction after 5-FU treatment in CRC cells. It has been observed that mitogen-activated protein kinase 14 (MAPK14)/p38α helps protect colon cancer cells against the cytotoxic effects of 5-FU and irinotecan by triggering cytoprotective autophagy (184, 185). After 5-FU treatment, the autophagy response typically manifests itself as a pro-survival response in 5-FU-resistant cells, associated with higher activation of autophagy proteins. Using colon cancer cells in xenografts, it was found that curcumin enhances 5-FU antitumor effects by suppressing autophagic activity via AMPK/ULK1 signaling (186). Similarly, EGFR overexpression was shown to induce cytoprotective autophagy in CRC cells in response to 5-FU treatment (187). Ubiquitin-specific protease 11 (USP11) was found to induce resistance to 5-FU by inducing autophagy through AMPK/AKT/mTOR pathway in CRC cell lines (188).
Furthermore, microRNAs have been shown to play a role in chemotherapy sensitivity in CRC. It has recently been demonstrated that miR-125b confers resistance to 5-FU in CRC through induction of autophagy both in vitro and in vivo (189). Overexpression of miR-34a or knockdown of NEAT1 reduced the growth of CRC cell lines and made them more sensitive to 5-FU. Further studies found that NEAT1 facilitates autophagy in CRC cells by downregulating miR‐34a (190). MiR-34a modulates oxaliplatin resistance in CRC by enhancing macroautophagy via the TGF-β/Smad4 pathway. When miR-34a is inhibited, OXA is more effective at combating resistant CRC cells (191). MiR-22 also inhibits autophagy in CRC cells and promotes 5-FU induced apoptosis, killing the cancer cells more efficiently (192).
Additionally, CQ significantly enhances the anti-tumor effects of 5-FU both in vitro and in vivo. In an in vivo mice study, 5-FU induced autophagy was blocked by CQ treatment, which resulted in chemosensitivity (193). Similarly, in HT29 murine xenografts, sensitivity to oxaliplatin was augmented by autophagy inhibition with CQ treatment or by genetic manipulation of autophagy genes such as Beclin 1 and ATG5 (194). Metastatic CRC patients treated with HCQ (Hydroxychloroquine) demonstrate an improved immunity and a suppressed autophagy system by boosting p62 accumulation and upregulating lysosomal cathepsin D expression (195). The inhibition of autophagy by CQ increases the sensitivity of CRC cells in vitro to concurrent treatments with 5-FU and radiotherapy (196). Over the past few years, targeted therapy and immunotherapy-based treatment options have gained increasing popularity. This approach involves targeting molecular pathways crucial to tumor growth and maintenance, while immunotherapy involves stimulating the patient’s immune cells to detect and attack cancer cells. Recently, autophagy has been identified as a mechanism associated with cancer therapy resistance. Additionally, autophagy may modulate cancer immunotherapy response by degrading immune checkpoint proteins, releasing pro-inflammatory cytokines, generating and degrading antigens (197).
Specific therapies are available for patients with stage IV metastatic colon cancer these therapies target epidermal growth factor receptors (EGFR) such as cetuximab, Panitumumab, and angiogenesis such as cabozantinib, bevacizumab, and regorafenib. These drugs show improvement, but their effects are short-lived due to a rapid build-up of resistance (198, 199). Cetuximab works by blocking EGFR, thus inhibiting downstream EGFR signaling, negatively impacting tumor cell proliferation, invasion, and angiogenesis. Lately, it has been observed in an in vitro study that cetuximab treatment in many cancer cell lines, including CRC, stimulates cytoprotective autophagy through the inhibition of EGFR/PI3K/mTOR signaling pathway (200). An EGFR inhibitor, panitumumab, also inhibited cell proliferation in the DLD-1 CRC cell line, along with upregulation of autophagy genes, but no effect was seen on apoptosis and cell cycle progression, indicating that autophagy induction is responsible for reduced proliferation (201). Cabozantinib is a receptor tyrosine kinase inhibitor (TKI) that suppresses many targets, including VEGFR2. Recently, a study reported decrease in levels of activation PI3K/AKT/mTOR axis in colorectal cancer explants after cabozantinib treatment. Further in vitro investigation included cabozantinib combined with autophagy inhibitor treatment in CRC cell lines which resulted in the increased anticancer effect of cabozantinib (202). It is interesting to note that in vitro and in vivo studies showed that bevacizumab induces autophagy in CRC cell lines, as determined by the presence of increased autophagic markers. Additionally, inhibition of autophagy increases the cytotoxic effects of bevacizumab (203). Brigatinib a next-generationtion TKI, recently a study showed that brigatinib combined treatment with CQ enhanced the anticancer activity in CRC cells (204). Regorafenib is a multi-kinase inhibitor, and its resistance in CRC has been reported however the exact mechanism remains to be elucidated. Recently a study on glioblastoma found regorafenib treatment caused cytotoxicity through autophagy arrest (205). However, it remains to be determined if regorafenib combined with autophagy inhibitors would reverse the resistance in CRC.
In addition, a subgroup of CRCs with microsatellite instability (MSI) accounts for approximately 15% of all CRCs (206). Programmed cell death 1 (PD1) blocking antibodies that enhance tumor immunity are currently under investigation in MSI high CRCs (207). Immune checkpoints serve to prevent autoimmunity by modulating the immune system, but their presence becomes an escape mechanism for tumors from the immune system. CRCs with MMR/MSI-H respond well to PD-1 blockade immunotherapy (208). Nevertheless, it is estimated that 45-70% of these tumors showed resistance to immune checkpoint blockades (209–211). In vitro study involving RKO CRC cell line with BRAFV600E and MSI-H phenotype treated with immune checkpoint inhibitors and anti-EGFR upregulated autophagy and PD-L1 levels via MEK/ERK signaling pathway. The inhibition of autophagy resulted in cells becoming more sensitive to immune checkpoint inhibitors and anti-EGFR treatments (212). Consequently, indicating autophagy induction as a mechanism of resistance to the immune checkpoint inhibitors. In addition, this suggests the need for in vivo studies to decipher how autophagy dysregulation might contribute to immune checkpoint inhibitor resistance in CRC patients.
Conclusions
Autophagy plays a complex and context-dependent role by limiting the cancer cell proliferation at the initial stages but facilitates cancer progression under stressful conditions in the later stages. Early retrospective studies using human CRC tissues have highlighted the link between autophagy and CRC. The majority of studies indicate that higher expression of autophagy proteins in CRC is associated with poor prognosis and metastasis. On the other hand, few studies have linked autophagy to a favorable prognosis in CRC. This discrepancy could be attributed to the different sizes of cohort and intra-tumoral genetic heterogeneity of the patients involved. Moreover, autophagy acts as an important switch in tumor progression and EMT. Hypoxia-dependent autophagy supports the cancer cell growth to support cancer cells in adverse conditions. Through regulation of autophagy, crosstalk among tumor microenvironment elements can influence tumor progression, EMT, and metastasis. To unravel mechanisms that control such a complex network, examining the autophagy network within the tumor microenvironment, including the CAF, immune cells, epithelial cells, and mesenchymal cells, should be advocated. Figure 3 summarizes the dichotomous role of autophagy.
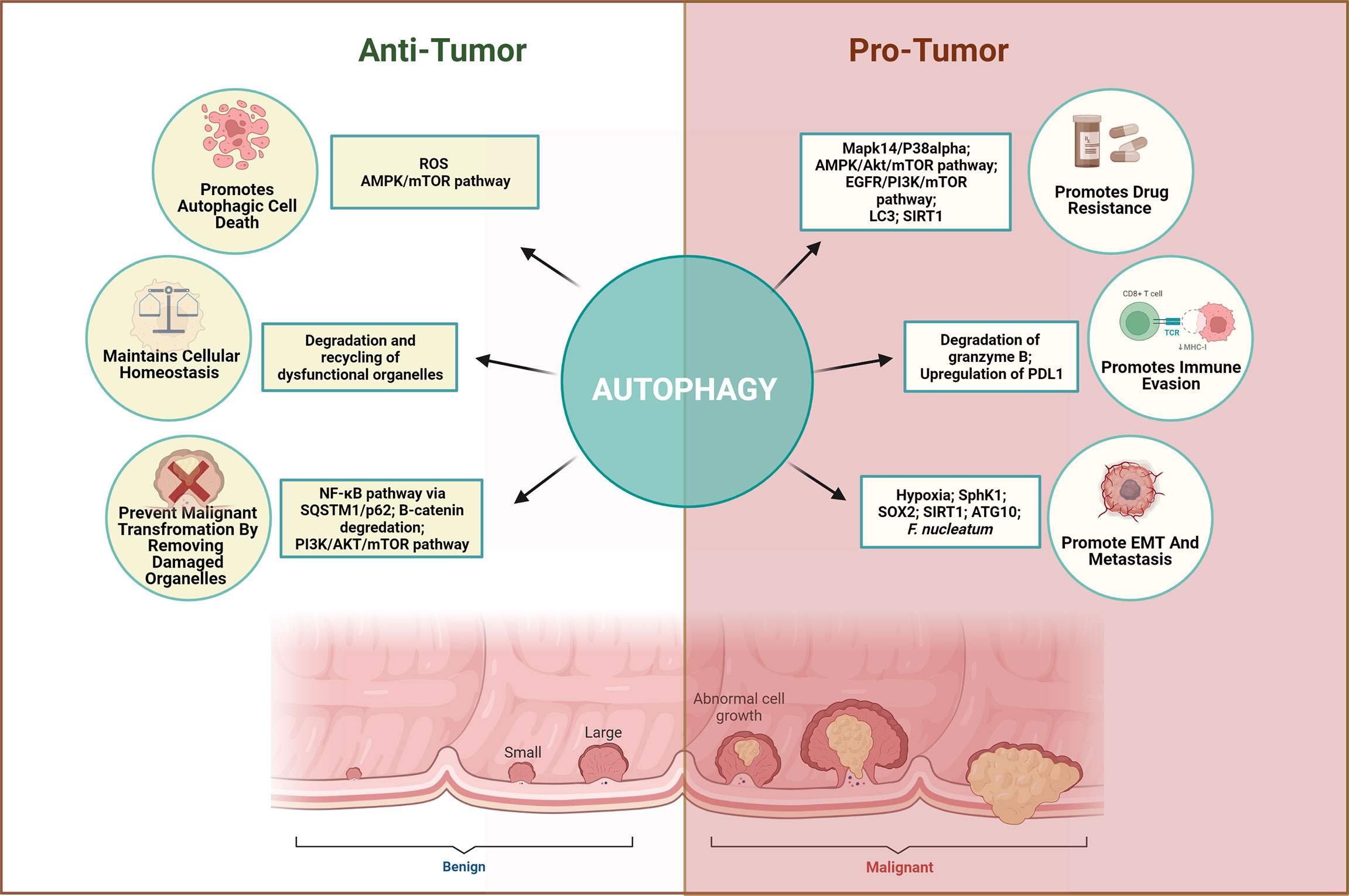
Figure 3 Dichotomous role of autophagy in CRC. Autophagy plays a complex and context-dependent role. On one hand, it can protect against abnormal survival by promoting autophagic death of tumor cells, maintain homeostasis and remove dysfunctional organelles in early stages, while on the other hand, it can promote tumor growth by favoring immune evasion, EMT, angiogenesis, and resisting the therapeutic effects when cancer has advanced.
Furthermore, most studies examining the mechanistic role of autophagy on CRC tumorigenesis used xenograft mice models and CRC cell lines. Many of these studies have targeted autophagy transiently either through pharmacological or genetic manipulations, which can affect the study in various ways. Improved gene-editing techniques such as CRISPR/Cas9 are required to be used in preclinical research to delineate the mechanistic role of autophagy in CRC. In clinical trials, patients with mismatch repair deficiency or microsatellite instability-high CRCs responded better to immune-checkpoint inhibitors than CRC patients with MSS. However, most patients developed resistance during treatments. Currently, many clinical trials are underway utilizing autophagy inhibitors as an adjunct drug or in combinational chemotherapy (213). These trials intend to capture the positive outcomes of using autophagy as a process of inducing programmed cell death in cancer cells. Such autophagic manipulation may lead to promising effects in CRC treatment. Although there is ample evidence available and was discussed in this review, there are still many questions unanswered, especially when it comes to solving the paradox of autophagy switching from an anti-cancer to a pro-cancer mechanism. We believe that a comprehensive understanding provided by this study should clarify the gaps required to be filled by performing studies to effectively target autophagic pathways in combination with the conventional therapies in the treatment of CRC.
Author Contributions
SM and JM were involved in the conception of the idea. SM wrote the initial draft prepared the tables and figures. QH, AM, and JM conducted supervision and critical review. SM, JM, AM, and QH revised and prepared the final version. All authors agreed to the final version of the manuscript.
Conflict of Interest
The authors declare that the research was conducted in the absence of any commercial or financial relationships that could be construed as a potential conflict of interest.
Publisher’s Note
All claims expressed in this article are solely those of the authors and do not necessarily represent those of their affiliated organizations, or those of the publisher, the editors and the reviewers. Any product that may be evaluated in this article, or claim that may be made by its manufacturer, is not guaranteed or endorsed by the publisher.
Acknowledgments
The work in the authors laboratories is supported by grants from the Terry Fox Foundation (MISC051) and the Sharjah Research Academy to AM, and by Research Institute of Medical and Health Sciences, University of Sharjah (1901090159) to JM.
Glossary
References
1. Siegel RL, Miller KD, Goding Sauer A, Fedewa SA, Butterly LF, Anderson JC, et al. Colorectal Cancer Statistics, 2020. CA Cancer J Clin (2020) 70(3):145–64. doi: 10.3322/caac.21601
2. Esteller M, Corn PG, Baylin SB. Herman Jg. A Gene Hypermethylation Profile Hum Cancer Cancer Res (2001) 61(8):3225–9.
3. Nazemalhosseini Mojarad E, Kuppen PJ, Aghdaei HA, Zali MR. The CPG Island Methylator Phenotype (CIMP) in Colorectal Cancer. Gastroenterol Hepatol Bed Bench (2013) 6(3):120–8.
4. Li K, Luo H, Huang L, Luo H, Zhu X. Microsatellite Instability: A Review of What the Oncologist Should Know. Cancer Cell Int (2020) 20:16. doi: 10.1186/s12935-019-1091-8
5. Sinicrope FA, Sargent DJ. Molecular Pathways: Microsatellite Instability in Colorectal Cancer: Prognostic, Predictive, and Therapeutic Implications. Clin Cancer Res (2012) 18(6):1506–12. doi: 10.1158/1078-0432.CCR-11-1469
6. Li SKH, Martin A. Mismatch Repair and Colon Cancer: Mechanisms and Therapies Explored. Trends Mol Med (2016) 22(4):274–89. doi: 10.1016/j.molmed.2016.02.003
7. Dekker E, Tanis PJ, Vleugels JLA, Kasi PM, Wallace MB. Colorectal Cancer. Lancet (2019) 394(10207):1467–80. doi: 10.1016/S0140-6736(19)32319-0
8. Hewish M, Lord CJ, Martin SA, Cunningham D, Ashworth A. Mismatch Repair Deficient Colorectal Cancer in the Era of Personalized Treatment. Nat Rev Clin Oncol (2010) 7(4):197–208. doi: 10.1038/nrclinonc.2010.18
9. Kuipers EJ, Grady WM, Lieberman D, Seufferlein T, Sung JJ, Boelens PG, et al. Colorectal Cancer. Nat Rev Dis Primers (2015) 1:15065. doi: 10.1038/nrdp.2015.65
10. Wilkins T, McMechan D, Talukder A. Colorectal Cancer Screening and Prevention. Am Fam Physician (2018) 97(10):658–65.
11. Xie YH, Chen YX, Fang JY. Comprehensive Review of Targeted Therapy for Colorectal Cancer. Signal Transduct Target Ther (2020) 5(1):22. doi: 10.1038/s41392-020-0116-z
12. Joachim C, Macni J, Drame M, Pomier A, Escarmant P, Veronique-Baudin J, et al. Overall Survival of Colorectal Cancer by Stage at Diagnosis: Data From the Martinique Cancer Registry. Med (Baltimore) (2019) 98(35):e16941. doi: 10.1097/MD.0000000000016941
13. Auzmendi-Iriarte J, Matheu A. Impact of Chaperone-Mediated Autophagy in Brain Aging: Neurodegenerative Diseases and Glioblastoma. Front Aging Neurosci (2020) 12:630743. doi: 10.3389/fnagi.2020.630743
14. Mijaljica D, Prescott M, Devenish RJ. Microautophagy in Mammalian Cells: Revisiting a 40-Year-Old Conundrum. Autophagy (2011) 7(7):673–82. doi: 10.4161/auto.7.7.14733
15. Parzych KR, Klionsky DJ. An Overview of Autophagy: Morphology, Mechanism, and Regulation. Antioxid Redox Signal (2014) 20(3):460–73. doi: 10.1089/ars.2013.5371
16. Zachari M, Ganley IG. The Mammalian ULK1 Complex and Autophagy Initiation. Essays Biochem (2017) 61(6):585–96. doi: 10.1042/EBC20170021
17. Shibutani ST, Yoshimori T. A Current Perspective of Autophagosome Biogenesis. Cell Res(2014) 24(1):58–68. doi: 10.1038/cr.2013.159
18. Li X, He S, Ma B. Autophagy and Autophagy-Related Proteins in Cancer. Mol Cancer (2020) 19(1):12. doi: 10.1186/s12943-020-1138-4
19. Amaravadi R, Kimmelman AC, White E. Recent Insights Into the Function of Autophagy in Cancer. Genes Dev (2016) 30(17):1913–30. doi: 10.1101/gad.287524.116
20. Talukdar S, Pradhan AK, Bhoopathi P, Shen XN, August LA, Windle JJ, et al. MDA-9/Syntenin Regulates Protective Autophagy in Anoikis-Resistant Glioma Stem Cells. Proc Natl Acad Sci U S A (2018) 115(22):5768–73. doi: 10.1073/pnas.1721650115
21. White E. Deconvoluting the Context-Dependent Role for Autophagy in Cancer. Nat Rev Cancer (2012) 12(6):401–10. doi: 10.1038/nrc3262
22. Yang Z, Sun Q, Guo J, Wang S, Song G, Liu W, et al. GRSF1-Mediated MIR-G-1 Promotes Malignant Behavior and Nuclear Autophagy by Directly Upregulating TMED5 and LMNB1 in Cervical Cancer Cells. Autophagy (2019) 15(4):668–85. doi: 10.1080/15548627.2018.1539590
23. Alves S, Castro L, Fernandes MS, Francisco R, Castro P, Priault M, et al. Colorectal Cancer-Related Mutant Kras Alleles Function as Positive Regulators of Autophagy. Oncotarget (2015) 6(31):30787–802. doi: 10.18632/oncotarget.5021
24. Dong Y, Wu Y, Zhao GL, Ye ZY, Xing CG, Yang XD. Inhibition of Autophagy by 3-MA Promotes Hypoxia-Induced Apoptosis in Human Colorectal Cancer Cells. Eur Rev Med Pharmacol Sci (2019) 23(3):1047–54. doi: 10.26355/eurrev_201902_16992
25. Singh SS, Vats S, Chia AY, Tan TZ, Deng S, Ong MS, et al. Dual Role of Autophagy in Hallmarks of Cancer. Oncogene (2018) 37(9):1142–58. doi: 10.1038/s41388-017-0046-6
26. Rybstein MD, Bravo-San Pedro JM, Kroemer G, Galluzzi L. The Autophagic Network and Cancer. Nat Cell Biol (2018) 20(3):243–51. doi: 10.1038/s41556-018-0042-2
27. Burada F, Nicoli ER, Ciurea ME, Uscatu DC, Ioana M, Gheonea DI. Autophagy in Colorectal Cancer: An Important Switch From Physiology to Pathology. World J Gastrointest Oncol (2015) 7(11):271–84. doi: 10.4251/wjgo.v7.i11.271
28. Zheng HY, Zhang XY, Wang XF, Sun BC. Autophagy Enhances the Aggressiveness of Human Colorectal Cancer Cells and Their Ability to Adapt to Apoptotic Stimulus. Cancer Biol Med (2012) 9(2):105–10. doi: 10.3969/j.issn.2095-3941.2012.02.004
29. Sakanashi F, Shintani M, Tsuneyoshi M, Ohsaki H, Kamoshida S. Apoptosis, Necroptosis and Autophagy in Colorectal Cancer: Associations With Tumor Aggressiveness and p53 Status. Pathol Res Pract (2019) 215(7):152425. doi: 10.1016/j.prp.2019.04.017
30. Guo GF, Wang YX, Zhang YJ, Chen XX, Lu JB, Wang HH, et al. Predictive and Prognostic Implications of 4e-BP1, Beclin-1, and LC3 for Cetuximab Treatment Combined With Chemotherapy in Advanced Colorectal Cancer With Wild-Type KRAS: Analysis From Real-World Data. World J Gastroenterol (2019) 25(15):1840–53. doi: 10.3748/wjg.v25.i15.1840
31. Koukourakis MI, Giatromanolaki A, Sivridis E, Pitiakoudis M, Gatter KC, Harris AL. Beclin 1 Over- and Underexpression in Colorectal Cancer: Distinct Patterns Relate to Prognosis and Tumour Hypoxia. Br J Cancer (2010) 103(8):1209–14. doi: 10.1038/sj.bjc.6605904
32. Park JM, Huang S, Wu TT, Foster NR, Sinicrope FA. Prognostic Impact of Beclin 1, P62/Sequestosome 1 and LC3 Protein Expression in Colon Carcinomas From Patients Receiving 5-Fluorouracil as Adjuvant Chemotherapy. Cancer Biol Ther (2013) 14(2):100–7. doi: 10.4161/cbt.22954
33. Devenport SN, Singhal R, Radyk MD, Taranto JG, Kerk SA, Chen B, et al. Colorectal Cancer Cells Utilize Autophagy to Maintain Mitochondrial Metabolism for Cell Proliferation Under Nutrient Stress. JCI Insight (2021) 6(14). doi: 10.1172/jci.insight.138835
34. Sun R, Wang X, Zhu H, Mei H, Wang W, Zhang S, et al. Prognostic Value of LAMP3 and TP53 Overexpression in Benign and Malignant Gastrointestinal Tissues. Oncotarget (2014) 5(23):12398–409. doi: 10.18632/oncotarget.2643
35. Bednarczyk M, Simka K, Muc-Wierzgoń M, Zmarzły N, Kruszniewska-Rajs C, Grabarek B, et al. The Transcriptional Activity of LAMP3 Gene Involved in Autophagocytosis in Colorectal Cancer LAMP3 Expression in Colorectal Cancer. J Biosci Medicines (2017) 5:24–36. doi: 10.4236/jbm.2017.512004
36. Levy J, Cacheux W, Bara MA, L'Hermitte A, Lepage P, Fraudeau M, et al. Intestinal Inhibition of Atg7 Prevents Tumour Initiation Through a Microbiome-Influenced Immune Response and Suppresses Tumour Growth. Nat Cell Biol (2015) 17(8):1062–73. doi: 10.1038/ncb3206
37. Scherr AL, Jassowicz A, Pato A, Elssner C, Ismail L, Schmitt N, et al. Knockdown of Atg7 Induces Nuclear-LC3 Dependent Apoptosis and Augments Chemotherapy in Colorectal Cancer Cells. Int J Mol Sci (2020) 21(3). doi: 10.3390/ijms21031099
38. Xiao T, Zhu W, Huang W, Lu SS, Li XH, Xiao ZQ, et al. RACK1 Promotes Tumorigenicity of Colon Cancer by Inducing Cell Autophagy. Cell Death Dis (2018) 9(12):1148. doi: 10.1038/s41419-018-1113-9
39. Chen X, Sun K, Jiao S, Cai N, Zhao X, Zou H, et al. High Levels of SIRT1 Expression Enhance Tumorigenesis and Associate With a Poor Prognosis of Colorectal Carcinoma Patients. Sci Rep (2014) 4:7481. doi: 10.1038/srep07481
40. Qiu G, Li X, Che X, Wei C, He S, Lu J, et al. SIRT1 Is a Regulator of Autophagy: Implications in Gastric Cancer Progression and Treatment. FEBS Lett (2015) 589(16):2034–42. doi: 10.1016/j.febslet.2015.05.042
41. Cheng F, Su L, Yao C, Liu L, Shen J, Liu C, et al. SIRT1 Promotes Epithelial-Mesenchymal Transition and Metastasis in Colorectal Cancer by Regulating Fra-1 Expression. Cancer Lett (2016) 375(2):274–83. doi: 10.1016/j.canlet.2016.03.010
42. Yu DF, Jiang SJ, Pan ZP, Cheng WD, Zhang WJ, Yao XK, et al. Expression and Clinical Significance of SIRT1 in Colorectal Cancer. Oncol Lett (2016) 11(2):1167–72. doi: 10.3892/ol.2015.3982
43. Manzoor S, Saber-Ayad M, Maghazachi AA, Hamid Q, Muhammad JS. MLH1 Mediates Cytoprotective Nucleophagy to Resist 5-Fluorouracil-Induced Cell Death in Colorectal Carcinoma. Neoplasia (2022) 24(2):76–85. doi: 10.1016/j.neo.2021.12.003
44. Jo YK, Kim SC, Park IJ, Park SJ, Jin DH, Hong SW, et al. Increased Expression of Atg10 in Colorectal Cancer Is Associated With Lymphovascular Invasion and Lymph Node Metastasis. PLoS One (2012) 7(12):e52705. doi: 10.1371/journal.pone.0052705
45. Wu JN, Lin L, Luo SB, Qiu XZ, Zhu LY, Chen D, et al. Sphk1-Driven Autophagy Potentiates Focal Adhesion Paxillin-Mediated Metastasis in Colorectal Cancer. Cancer Med (2021) 10(17):6010–21. doi: 10.1002/cam4.4129
46. Zhu Y, Huang S, Chen S, Chen J, Wang Z, Wang Y, et al. SOX2 Promotes Chemoresistance, Cancer Stem Cells Properties, and Epithelial-Mesenchymal Transition by Beta-Catenin and Beclin1/Autophagy Signaling in Colorectal Cancer. Cell Death Dis (2021) 12(5):449. doi: 10.1038/s41419-021-03733-5
47. Shan TD, Xu JH, Yu T, Li JY, Zhao LN, Ouyang H, et al. Knockdown of Linc-POU3f3 Suppresses the Proliferation, Apoptosis, and Migration Resistance of Colorectal Cancer. Oncotarget (2016) 7(1):961–75. doi: 10.18632/oncotarget.5830
48. Ma Z, Lou S, Jiang Z. PHLDA2 Regulates EMT and Autophagy in Colorectal Cancer Via the PI3K/AKT Signaling Pathway. Aging (Albany NY) (2020) 12(9):7985–8000. doi: 10.18632/aging.103117
49. Islam Khan MZ, Law HKW. RAMS11 Promotes Crc Through mTOR-Dependent Inhibition of Autophagy, Suppression of Apoptosis, and Promotion of Epithelial-Mesenchymal Transition. Cancer Cell Int (2021) 21(1):321. doi: 10.1186/s12935-021-02023-6
50. Che N, Yang Z, Liu X, Li M, Feng Y, Zhang C, et al. Suppression of LETM1 Inhibits the Proliferation and Stemness of Colorectal Cancer Cells Through Reactive Oxygen Species-Induced Autophagy. J Cell Mol Med (2021) 25(4):2110–20. doi: 10.1111/jcmm.16169
51. Yang Z, Ghoorun RA, Fan X, Wu P, Bai Y, Li J, et al. High Expression of Beclin-1 Predicts Favorable Prognosis for Patients With Colorectal Cancer. Clin Res Hepatol Gastroenterol (2015) 39(1):98–106. doi: 10.1016/j.clinre.2014.06.014
52. Zhang MY, Gou WF, Zhao S, Mao XY, Zheng ZH, Takano Y, et al. Beclin 1 Expression Is Closely Linked to Colorectal Carcinogenesis and Distant Metastasis of Colorectal Carcinoma. Int J Mol Sci (2014) 15(8):14372–85. doi: 10.3390/ijms150814372
53. Chen Z, Li Y, Zhang C, Yi H, Wu C, Wang J, et al. Downregulation of Beclin 1 and Impairment of Autophagy in a Small Population of Colorectal Cancer. Dig Dis Sci (2013) 58(10):2887–94. doi: 10.1007/s10620-013-2732-8
54. Choi JH, Cho YS, Ko YH, Hong SU, Park JH, Lee MA. Absence of Autophagy-Related Proteins Expression Is Associated With Poor Prognosis in Patients With Colorectal Adenocarcinoma. Gastroenterol Res Pract (2014) 2014:179586. doi: 10.1155/2014/179586
55. Zhang MY, Wang LY, Zhao S, Guo XC, Xu YQ, Zheng ZH, et al. Effects of Beclin 1 Overexpression on Aggressive Phenotypes of Colon Cancer Cells. Oncol Lett (2019) 17(2):2441–50. doi: 10.3892/ol.2018.9817
56. Wang Y, Xiong H, Liu D, Hill C, Ertay A, Li J, et al. Autophagy Inhibition Specifically Promotes Epithelial-Mesenchymal Transition and Invasion in RAS-Mutated Cancer Cells. Autophagy (2019) 15(5):886–99. doi: 10.1080/15548627.2019.1569912
57. Chen H, Wu Q. Expression of GW112 and GRIM-19 in Colorectal Cancer Tissues. J BUON (2015) 20(2):438–42.
58. Hao M, Shu Z, Sun H, Sun R, Wang Y, Liu T, et al. GRIM-19 Expression Is a Potent Prognostic Marker in Colorectal Cancer. Hum Pathol (2015) 46(12):1815–20. doi: 10.1016/j.humpath.2015.07.020
59. Zhang J, Chu D, Kawamura T, Tanaka K, He S. GRIM-19 Repressed Hypoxia-Induced Invasion and EMT of Colorectal Cancer by Repressing Autophagy Through Inactivation of STAT3/HIF-1alpha Signaling Axis. J Cell Physiol (2019) 234(8):12800–8. doi: 10.1002/jcp.27914
60. Zhai H, Fesler A, Ba Y, Wu S, Ju J. Inhibition of Colorectal Cancer Stem Cell Survival and Invasive Potential by hsa-miR-140-5p Mediated Suppression of Smad2 and Autophagy. Oncotarget (2015) 6(23):19735–46. doi: 10.18632/oncotarget.3771
61. Xu W, Yu M, Qin J, Luo Y, Zhong M. LACTB Regulates PIK3R3 to Promote Autophagy and Inhibit EMT and Proliferation Through the PI3K/AKT/mTOR Signaling Pathway in Colorectal Cancer. Cancer Manag Res (2020) 12:5181–200. doi: 10.2147/CMAR.S250661
62. Zhai H, Song B, Xu X, Zhu W, Ju J. Inhibition of Autophagy and Tumor Growth in Colon Cancer by miR-502. Oncogene (2013) 32(12):1570–9. doi: 10.1038/onc.2012.167
63. Song F, Li L, Liang D, Zhuo Y, Wang X, Dai H. Knockdown of Long Noncoding RNA Urothelial Carcinoma Associated 1 Inhibits Colorectal Cancer Cell Proliferation and Promotes Apoptosis Via Modulating Autophagy. J Cell Physiol (2019) 234(5):7420–34. doi: 10.1002/jcp.27500
64. Sun J, Feng Y, Wang Y, Ji Q, Cai G, Shi L, et al. Alpha-Hederin Induces Autophagic Cell Death in Colorectal Cancer Cells Through Reactive Oxygen Species Dependent AMPK/mTOR Signaling Pathway Activation. Int J Oncol (2019) 54(5):1601–12. doi: 10.3892/ijo.2019.4757
65. Lauzier A, Normandeau-Guimond J, Vaillancourt-Lavigueur V, Boivin V, Charbonneau M, Rivard N, et al. Colorectal Cancer Cells Respond Differentially to Autophagy Inhibition in Vivo. Sci Rep (2019) 9(1):11316. doi: 10.1038/s41598-019-47659-7
66. Sena P, Mariani F, Mancini S, Benincasa M, Magnani G, Pedroni M, et al. Autophagy Is Upregulated During Colorectal Carcinogenesis, and in DNA Microsatellite Stable Carcinomas. Oncol Rep (2015) 34(6):3222–30. doi: 10.3892/or.2015.4326
67. Wang Y, Zhao Z, Zhuang J, Wu X, Wang Z, Zhang B, et al. Prognostic Value of Autophagy, Microsatellite Instability, and KRAS Mutations in Colorectal Cancer. J Cancer (2021) 12(12):3515–28. doi: 10.7150/jca.51430
68. Zhu G, Pei L, Xia H, Tang Q, Bi F. Role of Oncogenic KRAS in the Prognosis, Diagnosis and Treatment of Colorectal Cancer. Mol Cancer (2021) 20(1):143. doi: 10.1186/s12943-021-01441-4
69. Lievre A, Blons H, Laurent-Puig P. Oncogenic Mutations as Predictive Factors in Colorectal Cancer. Oncogene (2010) 29(21):3033–43. doi: 10.1038/onc.2010.89
70. Ekoff M, Kaufmann T, Engstrom M, Motoyama N, Villunger A, Jonsson JI, et al. The BH3-Only Protein Puma Plays an Essential Role in Cytokine Deprivation Induced Apoptosis of Mast Cells. Blood (2007) 110(9):3209–17. doi: 10.1182/blood-2007-02-073957
71. Myatt SS, Lam EW. The Emerging Roles of Forkhead Box (FOX) Proteins in Cancer. Nat Rev Cancer (2007) 7(11):847–59. doi: 10.1038/nrc2223
72. Fitzwalter BE, Towers CG, Sullivan KD, Andrysik Z, Hoh M, Ludwig M, et al. Autophagy Inhibition Mediates Apoptosis Sensitization in Cancer Therapy by Relieving FOXO3a Turnover. Dev Cell (2018) 44(5):555–65 e3. doi: 10.1016/j.devcel.2018.02.014
73. Sakitani K, Hirata Y, Hikiba Y, Hayakawa Y, Ihara S, Suzuki H, et al. Inhibition of Autophagy Exerts Anti-Colon Cancer Effects Via Apoptosis Induced by p53 Activation and ER Stress. BMC Cancer (2015) 15:795. doi: 10.1186/s12885-015-1789-5
74. Xiong L, Liu Z, Ouyang G, Lin L, Huang H, Kang H, et al. Autophagy Inhibition Enhances Photocytotoxicity of Photosan-II in Human Colorectal Cancer Cells. Oncotarget (2017) 8(4):6419–32. doi: 10.18632/oncotarget.14117
75. Qureshi-Baig K, Kuhn D, Viry E, Pozdeev VI, Schmitz M, Rodriguez F, et al. Hypoxia-Induced Autophagy Drives Colorectal Cancer Initiation and Progression by Activating the PRKC/PKC-EZR (Ezrin) Pathway. Autophagy (2020) 16(8):1436–52. doi: 10.1080/15548627.2019.1687213
76. Cho DH, Jo YK, Kim SC, Park IJ, Kim JC. Down-Regulated Expression of Atg5 in Colorectal Cancer. Anticancer Res (2012) 32(9):4091–6.
77. Wang J, Ren XR, Piao H, Zhao S, Osada T, Premont RT, et al. Niclosamide-Induced Wnt Signaling Inhibition in Colorectal Cancer Is Mediated by Autophagy. Biochem J (2019) 476(3):535–46. doi: 10.1042/BCJ20180385
78. Song C, Xu W, Wu H, Wang X, Gong Q, Liu C, et al. Photodynamic Therapy Induces Autophagy-Mediated Cell Death in Human Colorectal Cancer Cells Via Activation of the ROS/JNK Signaling Pathway. Cell Death Dis (2020) 11(10):938. doi: 10.1038/s41419-020-03136-y
79. Mi W, Wang C, Luo G, Li J, Zhang Y, Jiang M, et al. Targeting ERK Induced Cell Death and p53/ROS-Dependent Protective Autophagy in Colorectal Cancer. Cell Death Discov (2021) 7(1):375. doi: 10.1038/s41420-021-00677-9
80. Wang K, Song K, Ma Z, Yao Y, Liu C, Yang J, et al. Identification of EMT-Related High-Risk stage II Colorectal Cancer and Characterisation of Metastasis-Related Genes. Br J Cancer (2020) 123(3):410–7. doi: 10.1038/s41416-020-0902-y
81. Li J, Yang B, Zhou Q, Wu Y, Shang D, Guo Y, et al. Autophagy Promotes Hepatocellular Carcinoma Cell Invasion Through Activation of Epithelial-Mesenchymal Transition. Carcinogenesis (2013) 34(6):1343–51. doi: 10.1093/carcin/bgt063
82. Choi JE, Bae JS, Kang MJ, Chung MJ, Jang KY, Park HS, et al. Expression of Epithelial-Mesenchymal Transition and Cancer Stem Cell Markers in Colorectal Adenocarcinoma: Clinicopathological Significance. Oncol Rep (2017) 38(3):1695–705. doi: 10.3892/or.2017.5790
83. Hill C, Wang Y. The Importance of Epithelial-Mesenchymal Transition and Autophagy in Cancer Drug Resistance. Cancer Drug Resist (2020) 3(1):38–47. doi: 10.20517/cdr.2019.75
84. Emami Nejad A, Najafgholian S, Rostami A, Sistani A, Shojaeifar S, Esparvarinha M, et al. The Role of Hypoxia in the Tumor Microenvironment and Development of Cancer Stem Cell: A Novel Approach to Developing Treatment. Cancer Cell Int (2021) 21(1):62. doi: 10.1186/s12935-020-01719-5
85. Zhang W, Yuan W, Song J, Wang S, Gu X. LncRNA CPS1-IT1 Suppresses EMT and Metastasis of Colorectal Cancer by Inhibiting Hypoxia-Induced Autophagy Through Inactivation of HIF-1alpha. Biochimie (2018) 144:21–7. doi: 10.1016/j.biochi.2017.10.002
86. Wu N, Hui H, Cui L, Yang F. GRIM-19 Represses the Proliferation and Invasion of Cutaneous Squamous Cell Carcinoma Cells Associated With Downregulation of STAT3 Signaling. BioMed Pharmacother (2017) 95:1169–76. doi: 10.1016/j.biopha.2017.09.055
87. Pang L, Xia Y, Wang D, Meng X. Antitumor Activity of iNGR-GRIM-19 in Colorectal Cancer. Jpn J Clin Oncol (2017) 47(9):795–808. doi: 10.1093/jjco/hyx090
88. Yu S, Zhou R, Yang T, Liu S, Cui Z, Qiao Q, et al. Hypoxia Promotes Colorectal Cancer Cell Migration and Invasion in a SIRT1-Dependent Manner. Cancer Cell Int (2019) 19:116. doi: 10.1186/s12935-019-0819-9
89. Pyne NJ, El Buri A, Adams DR, Pyne S. Sphingosine 1-Phosphate and Cancer. Adv Biol Regul (2018) 68:97–106. doi: 10.1016/j.jbior.2017.09.006
90. Xu CY, Liu SQ, Qin MB, Zhuge CF, Qin L, Qin N, et al. Sphk1 Modulates Cell Migration and EMT-Related Marker Expression by Regulating the Expression of p-FAK in Colorectal Cancer Cells. Int J Mol Med (2017) 39(5):1277–84. doi: 10.3892/ijmm.2017.2921
91. Shen H, Yin L, Deng G, Guo C, Han Y, Li Y, et al. Knockdown of Beclin-1 Impairs Epithelial-Mesenchymal Transition of Colon Cancer Cells. J Cell Biochem (2018) 119(8):7022–31. doi: 10.1002/jcb.26912
92. Zheng J, Xu L, Pan Y, Yu S, Wang H, Kennedy D, et al. SOX2 Modulates Motility and Enhances Progression of Colorectal Cancer Via the Rho-ROCK Signaling Pathway. Oncotarget (2017) 8(58):98635–45. doi: 10.18632/oncotarget.21709
93. Yang Y, Du L, Shi D, Kong C, Liu J, Liu G, et al. Dysbiosis of Human Gut Microbiome in Young-Onset Colorectal Cancer. Nat Commun (2021) 12(1):6757. doi: 10.1038/s41467-021-27112-y
94. Fan X, Jin Y, Chen G, Ma X, Zhang L. Gut Microbiota Dysbiosis Drives the Development of Colorectal Cancer. Digestion (2021) 102(4):508–15. doi: 10.1159/000508328
95. Chen Y, Chen Y, Zhang J, Cao P, Su W, Deng Y, et al. Fusobacterium nucleatum Promotes Metastasis in Colorectal Cancer by Activating Autophagy Signaling Via the Upregulation of CARD3 Expression. Theranostics (2020) 10(1):323–39. doi: 10.7150/thno.38870
96. Ju J, Huang C, Lan SH, Wang T, Lin P, Lee J, et al. Characterization of a Colorectal Cancer Migration and Autophagy-Related Microrna miR-338-5p and Its Target Gene PIK3C3. Biomarkers Genomic Med (2013) 5(3):74–8. doi: 10.1016/j.bgm.2013.07.006
97. Chu CA, Lee CT, Lee JC, Wang YW, Huang CT, Lan SH, et al. miR-338-5p Promotes Metastasis of Colorectal Cancer by Inhibition of Phosphatidylinositol 3-Kinase, Catalytic Subunit Type 3-Mediated Autophagy Pathway. EBioMedicine (2019) 43:270–81. doi: 10.1016/j.ebiom.2019.04.010
98. Wei R, Xiao Y, Song Y, Yuan H, Luo J, Xu W. FAT4 Regulates the EMT and Autophagy in Colorectal Cancer Cells in Part Via the PI3K-AKT Signaling Axis. J Exp Clin Cancer Res (2019) 38(1):112. doi: 10.1186/s13046-019-1043-0
99. Wu H, Lu XX, Wang JR, Yang TY, Li XM, He XS, et al. TRAF6 Inhibits Colorectal Cancer Metastasis Through Regulating Selective Autophagic CTNNB1/β-Catenin Degradation and Is Targeted for GSK3B/GSK3β-Mediated Phosphorylation and Degradation. Autophagy (2019) 15(9):1506–22. doi: 10.1080/15548627.2019.1586250
100. Islam Khan MZ, Law HKW. Cancer Susceptibility Candidate 9 (CASC9) Promotes Colorectal Cancer Carcinogenesis Via mTOR-Dependent Autophagy and Epithelial-Mesenchymal Transition Pathways. Front Mol Biosci (2021) 8:627022. doi: 10.3389/fmolb.2021.627022
101. Jin MZ, Jin WL. The Updated Landscape of Tumor Microenvironment and Drug Repurposing. Signal Transduct Target Ther (2020) 5(1):166. doi: 10.1038/s41392-020-00280-x
102. Ceelen W, Ramsay RG, Narasimhan V, Heriot AG, De Wever O. Targeting the Tumor Microenvironment in Colorectal Peritoneal Metastases. Trends Cancer (2020) 6(3):236–46. doi: 10.1016/j.trecan.2019.12.008
103. Kalluri R. The Biology and Function of Fibroblasts in Cancer. Nat Rev Cancer (2016) 16(9):582–98. doi: 10.1038/nrc.2016.73
104. Martinez-Outschoorn UE, Trimmer C, Lin Z, Whitaker-Menezes D, Chiavarina B, Zhou J, et al. Autophagy in Cancer Associated Fibroblasts Promotes Tumor Cell Survival: Role of Hypoxia, HIF1 Induction and NFκB Activation in the Tumor Stromal Microenvironment. Cell Cycle (2010) 9(17):3515–33. doi: 10.4161/cc.9.17.12928
105. Zhou W, Xu G, Wang Y, Xu Z, Liu X, Xu X, et al. Oxidative Stress Induced Autophagy in Cancer Associated Fibroblast Enhances Proliferation and Metabolism of Colorectal Cancer Cells. Cell Cycle (2017) 16(1):73–81. doi: 10.1080/15384101.2016.1252882
106. Yang X, Xu X, Zhu J, Zhang S, Wu Y, Wu Y, et al. miR-31 Affects Colorectal Cancer Cells by Inhibiting Autophagy in Cancer-Associated Fibroblasts. Oncotarget (2016) 7(48):79617–28. doi: 10.18632/oncotarget.12873
107. Inoue T, Hayashi Y, Tsujii Y, Yoshii S, Sakatani A, Kimura K, et al. Suppression of Autophagy Promotes Fibroblast Activation in p53-Deficient Colorectal Cancer Cells. Sci Rep (2021) 11(1):19524. doi: 10.1038/s41598-021-98865-1
108. Thongchot S, Singsuksawat E, Sumransub N, Pongpaibul A, Trakarnsanga A, Thuwajit P, et al. Periostin Regulates Autophagy Through Integrin α5β1 or α6β4 and an AKT-Dependent Pathway in Colorectal Cancer Cell Migration. J Cell Mol Med (2020) 24(21):12421–32. doi: 10.1111/jcmm.15756
109. Sun W. Angiogenesis in Metastatic Colorectal Cancer and the Benefits of Targeted Therapy. J Hematol Oncol (2012) 5:63. doi: 10.1186/1756-8722-5-63
110. Hassanpour M, Rezabakhsh A, Pezeshkian M, Rahbarghazi R, Nouri M. Distinct Role of Autophagy on Angiogenesis: Highlights on the Effect of Autophagy in Endothelial Lineage and Progenitor Cells. Stem Cell Res Ther (2018) 9(1):305. doi: 10.1186/s13287-018-1060-5
111. Kardideh B, Samimi Z, Norooznezhad F, Kiani S, Mansouri K. Autophagy, Cancer and Angiogenesis: Where Is the Link? Cell Biosci (2019) 9:65. doi: 10.1186/s13578-019-0327-6
112. Ou X, Lee MR, Huang X, Messina-Graham S, Broxmeyer HE. SIRT1 Positively Regulates Autophagy and Mitochondria Function in Embryonic Stem Cells Under Oxidative Stress. Stem Cells (2014) 32(5):1183–94. doi: 10.1002/stem.1641
113. Zeng Z, Li Y, Pan Y, Lan X, Song F, Sun J, et al. Cancer-Derived Exosomal miR-25-3p Promotes Pre-Metastatic Niche Formation by Inducing Vascular Permeability and Angiogenesis. Nat Commun (2018) 9(1):5395. doi: 10.1038/s41467-018-07810-w
114. Plummer M, de Martel C, Vignat J, Ferlay J, Bray F, Franceschi S. Global Burden of Cancers Attributable to Infections in 2012: A Synthetic Analysis. Lancet Glob Health (2016) 4(9):e609–16. doi: 10.1016/S2214-109X(16)30143-7
115. Muhammad JS, Nanjo S, Ando T, Yamashita S, Maekita T, Ushijima T, et al. Autophagy Impairment by Helicobacter Pylori-Induced Methylation Silencing of MAP1LC3AV1 Promotes Gastric Carcinogenesis. Int J Cancer (2017) 140(10):2272–83. doi: 10.1002/ijc.30657
116. Ferreira RM, Pereira-Marques J, Pinto-Ribeiro I, Costa JL, Carneiro F, Machado JC, et al. Gastric Microbial Community Profiling Reveals a Dysbiotic Cancer-Associated Microbiota. Gut (2018) 67(2):226–36. doi: 10.1136/gutjnl-2017-314205
117. Pushalkar S, Hundeyin M, Daley D, Zambirinis CP, Kurz E, Mishra A, et al. The Pancreatic Cancer Microbiome Promotes Oncogenesis by Induction of Innate and Adaptive Immune Suppression. Cancer Discov (2018) 8(4):403–16. doi: 10.1158/2159-8290.CD-17-1134
118. Cheng Y, Ling Z, Li L. The Intestinal Microbiota and Colorectal Cancer. Front Immunol (2020) 11:615056. doi: 10.3389/fimmu.2020.615056
119. Okumura S, Konishi Y, Narukawa M, Sugiura Y, Yoshimoto S, Arai Y, et al. Gut Bacteria Identified in Colorectal Cancer Patients Promote Tumourigenesis Via Butyrate Secretion. Nat Commun (2021) 12(1):5674. doi: 10.1038/s41467-021-25965-x
120. Gardet A, Xavier RJ. Common Alleles That Influence Autophagy and the Risk for Inflammatory Bowel Disease. Curr Opin Immunol (2012) 24(5):522–9. doi: 10.1016/j.coi.2012.08.001
121. Arvelo F, Sojo F, Cotte C. Biology of Colorectal Cancer. Ecancermedicalscience (2015) 9:520. doi: 10.3332/ecancer.2015.520
122. Washington K, Zemper AED. APC-Related Models of Intestinal Neoplasia: A Brief Review for Pathologists. Surg Exp Pathol (2019) 2:11. doi: 10.1186/s42047-019-0036-9
123. Li Y, Kundu P, Seow SW, de Matos CT, Aronsson L, Chin KC, et al. Gut Microbiota Accelerate Tumor Growth Via c-jun and STAT3 phosphorylation in APCMin/+ mice. Carcinogenesis (2012) 33(6):1231–8. doi: 10.1093/carcin/bgs137
124. Yu T, Guo F, Yu Y, Sun T, Ma D, Han J, et al. Fusobacterium nucleatum Promotes Chemoresistance to Colorectal Cancer by Modulating Autophagy. Cell (2017) 170(3):548–63 e16. doi: 10.1016/j.cell.2017.07.008
125. Rolin J, Maghazachi AA. Effects of Lysophospholipids on Tumor Microenvironment. Cancer Microenviron (2011) 4(3):393–403. doi: 10.1007/s12307-011-0088-1
126. Paul S, Lal G. The Molecular Mechanism of Natural Killer Cells Function and Its Importance in Cancer Immunotherapy. Front Immunol (2017) 8:1124. doi: 10.3389/fimmu.2017.01124
127. Elemam NM, Al-Jaderi Z, Hachim MY, Maghazachi AA. HCT-116 Colorectal Cancer Cells Secrete Chemokines Which Induce Chemoattraction and Intracellular Calcium Mobilization in NK92 Cells. Cancer Immunol Immunother (2019) 68(6):883–95. doi: 10.1007/s00262-019-02319-7
128. Imai K, Matsuyama S, Miyake S, Suga K, Nakachi K. Natural Cytotoxic Activity of Peripheral-Blood Lymphocytes and Cancer Incidence: An 11-Year Follow-Up Study of a General Population. Lancet (2000) 356(9244):1795–9. doi: 10.1016/S0140-6736(00)03231-1
129. Halama N, Braun M, Kahlert C, Spille A, Quack C, Rahbari N, et al. Natural Killer Cells Are Scarce in Colorectal Carcinoma Tissue Despite High Levels of Chemokines and Cytokines. Clin Cancer Res (2011) 17(4):678–89. doi: 10.1158/1078-0432.CCR-10-2173
130. Peng LS, Zhang JY, Teng YS, Zhao YL, Wang TT, Mao FY, et al. Tumor-Associated Monocytes/Macrophages Impair Nk-Cell Function Via TGFβ1 in Human Gastric Cancer. Cancer Immunol Res (2017) 5(3):248–56. doi: 10.1158/2326-6066.CIR-16-0152
131. Zheng X, Qian Y, Fu B, Jiao D, Jiang Y, Chen P, et al. Mitochondrial Fragmentation Limits Nk Cell-Based Tumor Immunosurveillance. Nat Immunol (2019) 20(12):1656–67. doi: 10.1038/s41590-019-0511-1
132. Cozar B, Greppi M, Carpentier S, Narni-Mancinelli E, Chiossone L, Vivier E. Tumor-Infiltrating Natural Killer Cells. Cancer Discov (2021) 11(1):34–44. doi: 10.1158/2159-8290.CD-20-0655
133. Maghazachi AA. Insights Into Seven and Single Transmembrane-Spanning Domain Receptors and Their Signaling Pathways in Human Natural Killer Cells. Pharmacol Rev (2005) 57(3):339–57. doi: 10.1124/pr.57.3.5
134. Gharagozloo M, Kalantari H, Rezaei A, Maracy MR, Salehi M, Bahador A, et al. The Decrease in NKG2D+ Natural Killer Cells in Peripheral Blood of Patients With Metastatic Colorectal Cancer. Bratisl Lek Listy (2015) 116(5):296–301. doi: 10.4149/bll_2015_056
135. Niavarani SR, Lawson C, Bakos O, Boudaud M, Batenchuk C, Rouleau S, et al. Lipid Accumulation Impairs Natural Killer Cell Cytotoxicity and Tumor Control in the Postoperative Period. BMC Cancer (2019) 19(1):823. doi: 10.1186/s12885-019-6045-y
136. O'Sullivan TE, Geary CD, Weizman OE, Geiger TL, Rapp M, Dorn GW. 2nd, Et al. Atg5 Is Essential for the Development and Survival of Innate Lymphocytes. Cell Rep (2016) 15(9):1910–9. doi: 10.1016/j.celrep.2016.04.082
137. Wang S, Xia P, Huang G, Zhu P, Liu J, Ye B, et al. FOXO1-Mediated Autophagy Is Required for NK Cell Development and Innate Immunity. Nat Commun (2016) 7:11023. doi: 10.1038/ncomms11023
138. O'Sullivan TE, Johnson LR, Kang HH, Sun JC. BNIP3- and BNIP3l-Mediated Mitophagy Promotes the Generation of Natural Killer Cell Memory. Immunity (2015) 43(2):331–42. doi: 10.1016/j.immuni.2015.07.012
139. Mgrditchian T, Arakelian T, Paggetti J, Noman MZ, Viry E, Moussay E, et al. Targeting Autophagy Inhibits Melanoma Growth by Enhancing NK Cells Infiltration in a CCL5-Dependent Manner. Proc Natl Acad Sci U S A (2017) 114(44):E9271–E9. doi: 10.1073/pnas.1703921114
140. Baginska J, Viry E, Berchem G, Poli A, Noman MZ, van Moer K, et al. Granzyme B Degradation by Autophagy Decreases Tumor Cell Susceptibility to Natural Killer-Mediated Lysis Under Hypoxia. Proc Natl Acad Sci U S A (2013) 110(43):17450–5. doi: 10.1073/pnas.1304790110
141. Chollat-Namy M, Ben Safta-Saadoun T, Haferssas D, Meurice G, Chouaib S, Thiery J. The Pharmalogical Reactivation of p53 Function Improves Breast Tumor Cell Lysis by Granzyme B and NK Cells Through Induction of Autophagy. Cell Death Dis (2019) 10(10):695. doi: 10.1038/s41419-019-1950-1
142. Duan Z, Luo Y. Targeting Macrophages in Cancer Immunotherapy. Signal Transduct Target Ther (2021) 6(1):127. doi: 10.1038/s41392-021-00506-6
143. Mantovani A, Marchesi F, Malesci A, Laghi L, Allavena P. Tumour-Associated Macrophages as Treatment Targets in Oncology. Nat Rev Clin Oncol (2017) 14(7):399–416. doi: 10.1038/nrclinonc.2016.217
144. Wang H, Tian T, Zhang J. Tumor-Associated Macrophages (TAM) in Colorectal Cancer (CRC): From Mechanism to Therapy and Prognosis. Int J Mol Sci (2021) 22(16):8470. doi: 10.3390/ijms22168470
145. Zhou Q, Peng RQ, Wu XJ, Xia Q, Hou JH, Ding Y, et al. The Density of Macrophages in the Invasive Front Is Inversely Correlated to Liver Metastasis in Colon Cancer. J Transl Med (2010) 8:13. doi: 10.1186/1479-5876-8-13
146. Algars A, Irjala H, Vaittinen S, Huhtinen H, Sundstrom J, Salmi M, et al. Type and Location of Tumor-Infiltrating Macrophages and Lymphatic Vessels Predict Survival of Colorectal Cancer Patients. Int J Cancer (2012) 131(4):864–73. doi: 10.1002/ijc.26457
147. Edin S, Wikberg ML, Dahlin AM, Rutegard J, Oberg A, Oldenborg PA, et al. The Distribution of Macrophages With a M1 or M2 Phenotype in Relation to Prognosis and the Molecular Characteristics of Colorectal Cancer. PLoS One (2012) 7(10):e47045. doi: 10.1371/journal.pone.0047045
148. Wei C, Yang C, Wang S, Shi D, Zhang C, Lin X, et al. Crosstalk Between Cancer Cells and Tumor Associated Macrophages Is Required for Mesenchymal Circulating Tumor Cell-Mediated Colorectal Cancer Metastasis. Mol Cancer (2019) 18(1):64. doi: 10.1186/s12943-019-0976-4
149. Yang C, Wei C, Wang S, Shi D, Zhang C, Lin X, et al. Elevated CD163(+)/CD68(+) Ratio at Tumor Invasive Front Is Closely Associated With Aggressive Phenotype and Poor Prognosis in Colorectal Cancer. Int J Biol Sci (2019) 15(5):984–98. doi: 10.7150/ijbs.29836
150. Yang Z, Zhang M, Peng R, Liu J, Wang F, Li Y, et al. The Prognostic and Clinicopathological Value of Tumor-Associated Macrophages in Patients With Colorectal Cancer: A Systematic Review and Meta-Analysis. Int J Colorectal Dis (2020) 35(9):1651–61. doi: 10.1007/s00384-020-03686-9
151. Inagaki K, Kunisho S, Takigawa H, Yuge R, Oka S, Tanaka S, et al. Role of Tumor-Associated Macrophages at the Invasive Front in Human Colorectal Cancer Progression. Cancer Sci (2021) 112(7):2692–704. doi: 10.1111/cas.14940
152. Zhang Y, Morgan MJ, Chen K, Choksi S, Liu ZG. Induction of Autophagy Is Essential for Monocyte-Macrophage Differentiation. Blood (2012) 119(12):2895–905. doi: 10.1182/blood-2011-08-372383
153. Jacquel A, Obba S, Boyer L, Dufies M, Robert G, Gounon P, et al. Autophagy Is Required for CSF-1-Induced Macrophagic Differentiation and Acquisition of Phagocytic Functions. Blood (2012) 119(19):4527–31. doi: 10.1182/blood-2011-11-392167
154. Jacquel A, Obba S, Solary E, Auberger P. Proper Macrophagic Differentiation Requires Both Autophagy and Caspase Activation. Autophagy (2012) 8(7):1141–3. doi: 10.4161/auto.20367
155. Stranks AJ, Hansen AL, Panse I, Mortensen M, Ferguson DJ, Puleston DJ, et al. Autophagy Controls Acquisition of Aging Features in Macrophages. J Innate Immun (2015) 7(4):375–91. doi: 10.1159/000370112
156. Bhattacharya A, Ghosh P, Singh A, Ghosh A, Bhowmick A, Sinha DK, et al. Delineating the Complex Mechanistic Interplay Between NF-κβ Driven mTOR Depedent Autophagy and Monocyte to Macrophage Differentiation: A Functional Perspective. Cell Signal (2021) 88:110150. doi: 10.1016/j.cellsig.2021.110150
157. Yang M, Liu J, Shao J, Qin Y, Ji Q, Zhang X, et al. Cathepsin S-Mediated Autophagic Flux in Tumor-Associated Macrophages Accelerate Tumor Development by Promoting M2 Polarization. Mol Cancer (2014) 13:43. doi: 10.1186/1476-4598-13-43
158. Lian G, Chen S, Ouyang M, Li F, Chen L, Yang J. Colon Cancer Cell Secretes Egf to Promote M2 Polarization of Tam Through Egfr/Pi3k/Akt/mTOR Pathway. Technol Cancer Res Treat (2019) 18:1533033819849068. doi: 10.1177/1533033819849068
159. Shao LN, Zhu BS, Xing CG, Yang XD, Young W, Cao JP. Effects of Autophagy Regulation of Tumor-Associated Macrophages on Radiosensitivity of Colorectal Cancer Cells. Mol Med Rep (2016) 13(3):2661–70. doi: 10.3892/mmr.2016.4820
160. Zhao S, Mi Y, Guan B, Zheng B, Wei P, Gu Y, et al. Tumor-Derived Exosomal miR-934 Induces Macrophage M2 Polarization to Promote Liver Metastasis of Colorectal Cancer. J Hematol Oncol (2020) 13(1):156. doi: 10.1186/s13045-020-00991-2
161. Shang K, Bai YP, Wang C, Wang Z, Gu HY, Du X, et al. Crucial Involvement of Tumor-Associated Neutrophils in the Regulation of Chronic Colitis-Associated Carcinogenesis in Mice. PloS One (2012) 7(12):e51848. doi: 10.1371/journal.pone.0051848
162. Li XF, Chen DP, Ouyang FZ, Chen MM, Wu Y, Kuang DM, et al. Increased Autophagy Sustains the Survival and Pro-Tumourigenic Effects of Neutrophils in Human Hepatocellular Carcinoma. J Hepatol (2015) 62(1):131–9. doi: 10.1016/j.jhep.2014.08.023
163. Kaiko GE, Horvat JC, Beagley KW, Hansbro PM. Immunological Decision-Making: How Does the Immune System Decide to Mount a Helper T-Cell Response? Immunology (2008) 123(3):326–38. doi: 10.1111/j.1365-2567.2007.02719.x
164. Jia W, Pua HH, Li QJ, He YW. Autophagy Regulates Endoplasmic Reticulum Homeostasis and Calcium Mobilization in T Lymphocytes. J Immunol (2011) 186(3):1564–74. doi: 10.4049/jimmunol.1001822
165. Kovacs JR, Li C, Yang Q, Li G, Garcia IG, Ju S, et al. Autophagy Promotes T-Cell Survival Through Degradation of Proteins of the Cell Death Machinery. Cell Death Differ (2012) 19(1):144–52. doi: 10.1038/cdd.2011.78
166. Willinger T, Flavell RA. Canonical Autophagy Dependent on the Class III Phosphoinositide-3 Kinase Vps34 Is Required for Naive T-Cell Homeostasis. Proc Natl Acad Sci U S A (2012) 109(22):8670–5. doi: 10.1073/pnas.1205305109
167. Parekh VV, Wu L, Boyd KL, Williams JA, Gaddy JA, Olivares-Villagomez D, et al. Impaired Autophagy, Defective T Cell Homeostasis, and a Wasting Syndrome in Mice With a T Cell-Specific Deletion of Vps34. J Immunol (2013) 190(10):5086–101. doi: 10.4049/jimmunol.1202071
168. Xu X, Araki K, Li S, Han JH, Ye L, Tan WG, et al. Autophagy Is Essential for Effector Cd8(+) T Cell Survival and Memory Formation. Nat Immunol (2014) 15(12):1152–61. doi: 10.1038/ni.3025
169. Murera D, Arbogast F, Arnold J, Bouis D, Muller S, Gros F. CD4 T Cell Autophagy Is Integral to Memory Maintenance. Sci Rep (2018) 8(1):5951. doi: 10.1038/s41598-018-23993-0
170. Clarke AJ, Simon AK. Autophagy in the Renewal, Differentiation and Homeostasis of Immune Cells. Nat Rev Immunol (2019) 19(3):170–83. doi: 10.1038/s41577-018-0095-2
171. Vodnala SK, Eil R, Kishton RJ, Sukumar M, Yamamoto TN, Ha NH, et al. T Cell Stemness and Dysfunction in Tumors Are Triggered by a Common Mechanism. Science (2019) 363(6434):eaau0135. doi: 10.1126/science.aau0135
172. Crotzer VL, Blum JS. Autophagy and Its Role in MHC-Mediated Antigen Presentation. J Immunol (2009) 182(6):3335–41. doi: 10.4049/jimmunol.0803458
173. Shibutani ST, Saitoh T, Nowag H, Munz C, Yoshimori T. Autophagy and Autophagy-Related Proteins in the Immune System. Nat Immunol (2015) 16(10):1014–24. doi: 10.1038/ni.3273
174. Bell C, English L, Boulais J, Chemali M, Caron-Lizotte O, Desjardins M, et al. Quantitative Proteomics Reveals the Induction of Mitophagy in Tumor Necrosis Factor-Alpha-Activated (TNFα) Macrophages. Mol Cell Proteomics (2013) 12(9):2394–407. doi: 10.1074/mcp.M112.025775
175. Pages F, Berger A, Camus M, Sanchez-Cabo F, Costes A, Molidor R, et al. Effector Memory T Cells, Early Metastasis, and Survival in Colorectal Cancer. N Engl J Med (2005) 353(25):2654–66. doi: 10.1056/NEJMoa051424
176. Fridman WH, Pages F, Sautes-Fridman C, Galon J. The Immune Contexture in Human Tumours: Impact on Clinical Outcome. Nat Rev Cancer (2012) 12(4):298–306. doi: 10.1038/nrc3245
177. Galon J, Mlecnik B, Bindea G, Angell HK, Berger A, Lagorce C, et al. Towards the Introduction of the 'Immunoscore' in the Classification of Malignant Tumours. J Pathol (2014) 232(2):199–209. doi: 10.1002/path.4287
178. Wu Y, Yao J, Xie J, Liu Z, Zhou Y, Pan H, et al. The Role of Autophagy in Colitis-Associated Colorectal Cancer. Signal Transduct Target Ther (2018) 3:31. doi: 10.1038/s41392-018-0031-8
179. Ziegler PK, Bollrath J, Pallangyo CK, Matsutani T, Canli O, De Oliveira T, et al. Mitophagy in Intestinal Epithelial Cells Triggers Adaptive Immunity During Tumorigenesis. Cell (2018) 174(1):88–101 e16. doi: 10.1016/j.cell.2018.05.028
180. Zhang J, Chen L, Xiong F, Zhang S, Huang K, Zhang Z, et al. Autophagy in Regulatory T Cells: A Double-Edged Sword in Disease Settings. Mol Immunol (2019) 109:43–50. doi: 10.1016/j.molimm.2019.02.004
181. Wei J, Long L, Yang K, Guy C, Shrestha S, Chen Z, et al. Autophagy Enforces Functional Integrity of Regulatory T Cells by Coupling Environmental Cues and Metabolic Homeostasis. Nat Immunol (2016) 17(3):277–85. doi: 10.1038/ni.3365
182. Rivera Vargas T, Cai Z, Shen Y, Dosset M, Benoit-Lizon I, Martin T, et al. Selective Degradation of PU.1 During Autophagy Represses the Differentiation and Antitumour Activity of Th9 Cells. Nat Commun (2017) 8(1):559. doi: 10.1038/s41467-017-00468-w
183. Kosumi K, Masugi Y, Yang J, Qian ZR, Kim SA, Li W, et al. Tumor SQSTM1 (P62) Expression and T Cells in Colorectal Cancer. Oncoimmunology (2017) 6(3):e1284720. doi: 10.1080/2162402X.2017.1284720
184. De la Cruz-Morcillo MA, Valero ML, Callejas-Valera JL, Arias-Gonzalez L, Melgar-Rojas P, Galan-Moya EM, et al. P38MAPK Is a Major Determinant of the Balance Between Apoptosis and Autophagy Triggered by 5-Fluorouracil: Implication in Resistance. Oncogene (2012) 31(9):1073–85. doi: 10.1038/onc.2011.321
185. Paillas S, Causse A, Marzi L, de Medina P, Poirot M, Denis V, et al. MAPK14/p38α Confers Irinotecan Resistance to Tp53-Defective Cells by Inducing Survival Autophagy. Autophagy (2012) 8(7):1098–112. doi: 10.4161/auto.20268
186. Zhang P, Lai ZL, Chen HF, Zhang M, Wang A, Jia T, et al. Curcumin Synergizes With 5-Fluorouracil by Impairing AMPK/ULK1-Dependent Autophagy, AKT Activity and Enhancing Apoptosis in Colon Cancer Cells With Tumor Growth Inhibition in Xenograft Mice. J Exp Clin Cancer Res (2017) 36(1):190. doi: 10.1186/s13046-017-0661-7
187. Gu XY, Jiang Y, Li MQ, Han P, Liu YL, Cui BB. Over-Expression of EGFR Regulated by RARA Contributes to 5-FU Resistance in Colon Cancer. Aging (Albany NY) (2020) 12(1):156–77. doi: 10.18632/aging.102607
188. Sun H, Wang R, Liu Y, Mei H, Liu X, Peng Z. USP11 Induce Resistance to 5-Fluorouracil in Colorectal Cancer Through Activating Autophagy by Stabilizing VCP. J Cancer (2021) 12(8):2317–25. doi: 10.7150/jca.52158
189. Yu X, Shi W, Zhang Y, Wang X, Sun S, Song Z, et al. CXCL12/CXCR4 Axis Induced miR-125b Promotes Invasion and Confers 5-Fluorouracil Resistance Through Enhancing Autophagy in Colorectal Cancer. Sci Rep (2017) 7:42226. doi: 10.1038/srep42226
190. Liu F, Ai FY, Zhang DC, Tian L, Yang ZY, Liu SJ. Lncrna Neat1 Knockdown Attenuates Autophagy to Elevate 5-Fu Sensitivity in Colorectal Cancer Via Targeting miR-34a. Cancer Med (2020) 9(3):1079–91. doi: 10.1002/cam4.2746
191. Sun C, Wang FJ, Zhang HG, Xu XZ, Jia RC, Yao L, et al. miR-34a Mediates Oxaliplatin Resistance of Colorectal Cancer Cells by Inhibiting Macroautophagy Via Transforming Growth Factor-β/Smad4 Pathway. World J Gastroenterol (2017) 23(10):1816–27. doi: 10.3748/wjg.v23.i10.1816
192. Zhang H, Tang J, Li C, Kong J, Wang J, Wu Y, et al. miR-22 Regulates 5-FU Sensitivity by Inhibiting Autophagy and Promoting Apoptosis in Colorectal Cancer Cells. Cancer Lett (2015) 356(2 Pt B):781–90. doi: 10.1016/j.canlet.2014.10.029
193. Sasaki K, Tsuno NH, Sunami E, Kawai K, Hongo K, Hiyoshi M, et al. Resistance of Colon Cancer to 5-Fluorouracil May Be Overcome by Combination With Chloroquine, an in Vivo Study. Anticancer Drugs (2012) 23(7):675–82. doi: 10.1097/CAD.0b013e328353f8c7
194. Selvakumaran M, Amaravadi RK, Vasilevskaya IA, O'Dwyer PJ. Autophagy Inhibition Sensitizes Colon Cancer Cells to Antiangiogenic and Cytotoxic Therapy. Clin Cancer Res (2013) 19(11):2995–3007. doi: 10.1158/1078-0432.CCR-12-1542
195. Patel S, Hurez V, Nawrocki ST, Goros M, Michalek J, Sarantopoulos J, et al. Vorinostat and Hydroxychloroquine Improve Immunity and Inhibit Autophagy in Metastatic Colorectal Cancer. Oncotarget (2016) 7(37):59087–97. doi: 10.18632/oncotarget.10824
196. Schonewolf CA, Mehta M, Schiff D, Wu H, Haffty BG, Karantza V, et al. Autophagy Inhibition by Chloroquine Sensitizes HT-29 Colorectal Cancer Cells to Concurrent Chemoradiation. World J Gastrointest Oncol (2014) 6(3):74–82. doi: 10.4251/wjgo.v6.i3.74
197. Yamamoto K, Venida A, Yano J, Biancur DE, Kakiuchi M, Gupta S, et al. Autophagy Promotes Immune Evasion of Pancreatic Cancer by Degrading MHC-I. Nature (2020) 581(7806):100–5. doi: 10.1038/s41586-020-2229-5
198. Van Cutsem E, Kohne CH, Hitre E, Zaluski J, Chang Chien CR, Makhson A, et al. Cetuximab and Chemotherapy as Initial Treatment for Metastatic Colorectal Cancer. N Engl J Med (2009) 360(14):1408–17. doi: 10.1056/NEJMoa0805019
199. Van der Jeught K, Xu HC, Li YJ, Lu XB, Ji G. Drug Resistance and New Therapies in Colorectal Cancer. World J Gastroenterol (2018) 24(34):3834–48. doi: 10.3748/wjg.v24.i34.3834
200. Li X, Fan Z. The Epidermal Growth Factor Receptor Antibody Cetuximab Induces Autophagy in Cancer Cells by Downregulating HIF-1alpha and Bcl-2 and activating the Beclin 1/HVps34 complex. Cancer Res (2010) 70(14):5942–52. doi: 10.1158/0008-5472.CAN-10-0157
201. Giannopoulou E, Antonacopoulou A, Matsouka P, Kalofonos HP. Autophagy: Novel Action of Panitumumab in Colon Cancer. Anticancer Res (2009) 29(12):5077–82.
202. Scott AJ, Arcaroli JJ, Bagby SM, Yahn R, Huber KM, Serkova NJ, et al. Cabozantinib Exhibits Potent Antitumor Activity in Colorectal Cancer Patient-Derived Tumor Xenograft Models Via Autophagy and Signaling Mechanisms. Mol Cancer Ther (2018) 17(10):2112–22. doi: 10.1158/1535-7163.MCT-17-0131
203. Zhao Z, Xia G, Li N, Su R, Chen X, Zhong L. Autophagy Inhibition Promotes Bevacizumab-Induced Apoptosis and Proliferation Inhibition in Colorectal Cancer Cells. J Cancer (2018) 9(18):3407–16. doi: 10.7150/jca.24201
204. Zhang Z, Gao W, Zhou L, Chen Y, Qin S, Zhang L, et al. Repurposing Brigatinib for the Treatment of Colorectal Cancer Based on Inhibition of ER-Phagy. Theranostics (2019) 9(17):4878–92. doi: 10.7150/thno.36254
205. Jiang J, Zhang L, Chen H, Lei Y, Zhang T, Wang Y, et al. Regorafenib Induces Lethal Autophagy Arrest by Stabilizing PSAT1 in Glioblastoma. Autophagy (2020) 16(1):106–22. doi: 10.1080/15548627.2019.1598752
206. Gatalica Z, Vranic S, Xiu J, Swensen J, Reddy S. High Microsatellite Instability (MSI-H) Colorectal Carcinoma: A Brief Review of Predictive Biomarkers in the Era of Personalized Medicine. Fam Cancer (2016) 15(3):405–12. doi: 10.1007/s10689-016-9884-6
207. Ganesh K, Stadler ZK, Cercek A, Mendelsohn RB, Shia J, Segal NH, et al. Immunotherapy in Colorectal Cancer: Rationale, Challenges and Potential. Nat Rev Gastroenterol Hepatol (2019) 16(6):361–75. doi: 10.1038/s41575-019-0126-x
208. Le DT, Uram JN, Wang H, Bartlett BR, Kemberling H, Eyring AD, et al. PD-1 Blockade in Tumors With Mismatch-Repair Deficiency. N Engl J Med (2015) 372(26):2509–20. doi: 10.1056/NEJMoa1500596
209. Overman MJ, McDermott R, Leach JL, Lonardi S, Lenz HJ, Morse MA, et al. Nivolumab in Patients With Metastatic DNA Mismatch Repair-Deficient or Microsatellite Instability-High Colorectal Cancer (Checkmate 142): An Open-Label, Multicentre, Phase 2 Study. Lancet Oncol (2017) 18(9):1182–91. doi: 10.1016/S1470-2045(17)30422-9
210. Overman MJ, Lonardi S, Wong KYM, Lenz HJ, Gelsomino F, Aglietta M, et al. Durable Clinical Benefit With Nivolumab Plus Ipilimumab in DNA Mismatch Repair-Deficient/Microsatellite Instability-High Metastatic Colorectal Cancer. J Clin Oncol (2018) 36(8):773–9. doi: 10.1200/JCO.2017.76.9901
211. Le DT, Kim TW, Van Cutsem E, Geva R, Jager D, Hara H, et al. Phase II Open-Label Study of Pembrolizumab in Treatment-Refractory, Microsatellite Instability-High/Mismatch Repair-Deficient Metastatic Colorectal Cancer: Keynote-164. J Clin Oncol (2020) 38(1):11–9. doi: 10.1200/JCO.19.02107
212. Koustas E, Papavassiliou AG, Karamouzis MV. The Role of Autophagy in the Treatment of BRAF Mutant Colorectal Carcinomas Differs Based on Microsatellite Instability Status. PLoS One (2018) 13(11):e0207227. doi: 10.1371/journal.pone.0207227
Keywords: colorectal cancer, autophagy, epithelial-mesenchymal transition, metastasis, tumor microenvironment, therapeutic resistance
Citation: Manzoor S, Muhammad JS, Maghazachi AA and Hamid Q (2022) Autophagy: A Versatile Player in the Progression of Colorectal Cancer and Drug Resistance. Front. Oncol. 12:924290. doi: 10.3389/fonc.2022.924290
Received: 20 April 2022; Accepted: 06 June 2022;
Published: 14 July 2022.
Edited by:
Yanhong Deng, The Sixth Affiliated Hospital of Sun Yat-sen University, ChinaReviewed by:
Yunping Hu, Third Military Medical University, ChinaZongsheng He, Army Medical University, China
Copyright © 2022 Manzoor, Muhammad, Maghazachi and Hamid. This is an open-access article distributed under the terms of the Creative Commons Attribution License (CC BY). The use, distribution or reproduction in other forums is permitted, provided the original author(s) and the copyright owner(s) are credited and that the original publication in this journal is cited, in accordance with accepted academic practice. No use, distribution or reproduction is permitted which does not comply with these terms.
*Correspondence: Qutayba Hamid, cXV0YXliYS5oYW1pZEBtY2dpbGwuY2E=