- Department of Radiation Oncology, University of Pennsylvania, Philadelphia, PA, United States
Radiation therapy (RT) is an important modality in cancer treatment with >50% of cancer patients undergoing RT for curative or palliative intent. In patients with breast, lung, and esophageal cancer, as well as mediastinal malignancies, incidental RT dose to heart or vascular structures has been linked to the development of Radiation-Induced Heart Disease (RIHD) which manifests as ischemic heart disease, cardiomyopathy, cardiac dysfunction, and heart failure. Despite the remarkable progress in the delivery of radiotherapy treatment, off-target cardiac toxicities are unavoidable. One of the best-studied pathological consequences of incidental exposure of the heart to RT is collagen deposition and fibrosis, leading to the development of radiation-induced myocardial fibrosis (RIMF). However, the pathogenesis of RIMF is still largely unknown. Moreover, there are no available clinical approaches to reverse RIMF once it occurs and it continues to impair the quality of life of long-term cancer survivors. Hence, there is an increasing need for more clinically relevant preclinical models to elucidate the molecular and cellular mechanisms involved in the development of RIMF. This review offers an insight into the existing preclinical models to study RIHD and the suggested mechanisms of RIMF, as well as available multi-modality treatments and outcomes. Moreover, we summarize the valuable detection methods of RIHD/RIMF, and the clinical use of sensitive radiographic and circulating biomarkers.
Introduction
Current approaches to oncologic care rely heavily on multidisciplinary therapies delivered concurrently, sequentially, or in a response-adapted stepwise fashion. This strategy along with the use of novel therapies have contributed to significant increases in treatment response rates and long-term survival of cancer patients, leading to a population of cancer survivors of approximately 17 million in the United States alone, and 19.3 million new cancer diagnoses in 2020 worldwide (1, 2). However, as cancer patients are overcoming their diagnoses at increasingly convincing rates, they are also presenting clinicians with a new set of challenges involving the management of morbid and quality-of-life-limiting sequelae of their treatments. In particular, treatment-related cardiovascular mortality has become a major contributor to the global burden of cardiovascular disease, motivating a paradigm shift in the way we approach cardiovascular screening, risk stratification, diagnosis, treatment, and surveillance in cancer patients.
Cancer therapy-induced cardiovascular toxicity can manifest as a variety of clinical pathologies. The European Society of Cardiology guidelines have broadly categorized cardiotoxicity into several disease groups, including cardiomyopathy, myocarditis, pericardial disease, arrhythmias, myocardial ischemia, hypertension, vascular events, and valvular disease (3). The large spectrum of cardiovascular pathologies that have been observed is in part due to the large number of cardiotoxic agents increasingly used in oncologic care, including chemotherapies such as anthracyclines, targeted therapies such as tyrosine kinase inhibitors, immunotherapies such as immune checkpoint inhibitors, hormone therapies, and thoracic radiotherapy. This clinical heterogeneity is also dependent on multiple patient-specific factors, including age at therapy, sex, baseline cardiovascular risk, and overall health comorbidities (4). Moreover, throughout their disease course, many patients are increasingly receiving more than one cardiotoxic agent, further complicating the underlying disease mechanisms involved in causative pathophysiology.
Radiation therapy is one treatment modality with dose-dependent cardiotoxic potential (5–7). Radiation exploits a therapeutic index between normal tissue and tumor cells, causing differential cell death due to DNA damage and associated free radical formation, immune cell infiltration, and cytokine production. Despite vast improvements in radiation technologies and treatment delivery, such as Intensity-Modulated Radiotherapy (IMRT), Stereotactic Body Radiotherapy (SBRT) and proton therapy, incidental dose delivery to the heart is still a limiting factor in thoracic radiation therapy (8, 9). A recent large retrospective study reported that compared with non-irradiated patients, chest radiation therapy patients have a 2% higher absolute risk of cardiac morbidity and death at 5 years, and a 23% increased absolute risk at 20 years post-treatment (10). Furthermore, several large studies have demonstrated significant cardiovascular consequences of radiation therapy in breast cancer, lung cancer, and mediastinal lymphoma patient populations (10–13).
While recent research has provided significant insight, there remains a critical knowledge gap in pathophysiologic mechanisms of cardiovascular toxicity and optimal strategies for risk stratification, monitoring, and treating patients with cardiovascular dysfunction. This review describes the mechanisms by which thoracic radiation can cause therapy-induced myocardial fibrosis and discusses innovative preclinical models for studying such pathophysiology. We include an overview of the current landscape of radiographic and plasma biomarkers for therapy-induced toxicity, the beneficial effects and risks of multi-modality treatments and end with a discussion of future directions for the field.
Preclinical models to study RIHD
Preclinical animal models of mice, rats, rabbits, dogs, pigs, and non-human primates have long been used in studies of RIHD (14–21). Favorable characteristics such as the low maintenance and housing costs, reduced gestational times and lifespan, as well as the convenience for genetic modifications and selections have rendered the rodents an excellent model to perform mechanistic studies.
Many manifestations of RIHD have been successfully modeled in rodents yielding a wealth of information regarding the molecular pathways that participate in the development of the radiation-induced cardiotoxicity (22–29). Several genetically engineered rodent models developed to be prone to cardiac pathologies, such as atherosclerosis, have been studied following radiation delivery (30–36). For instance, it has been demonstrated that inflammatory and thrombotic responses are accelerated post ionizing irradiation on atherosclerosis-prone ApoE(-/-) mice but not on wildtype C57BL/6J mice (32, 37, 38). Moreover, endothelial-specific knock-out mouse models of p53 and p21 have contributed to the delineation of the role of endothelial cells in cardiac damage post-radiation (39, 40). Therefore, it is of high importance the selection of the right rodent genetic background to study any aspect of RIHD (41). In addition, Cre-loxP technology has offered the opportunity to create more pronounced or rapidly developed phenotypes of cardiac toxicity (42–44). Cardiac radiotherapy has also benefitted from rodent models of partial heart irradiation which aid in the identification of molecular signaling pathways regulating the RIHD milieu (42, 45–47). However, rodent models pose some limitations such as their phylogenetic distance from humans, and their physiological responses to treatment schemes (48–50), thus there is an obvious need for larger animal models of RIHD.
Rabbits were introduced as another animal model to study the RIHD to avoid the limitations experienced by the rodent models. Due to several commonalities in cardiac physiology with humans (51–53), rabbits serve as an excellent model to study heart failure, ischemic heart disease, and electrophysiological phenomena caused by radiation and as an alternative choice to larger animals (51–55). Nevertheless, there are marked differences in the physical size of the rabbit heart, the heart rates, and body weight, and these facts ought to be taken into consideration especially in studies of arrhythmia as a side effect of radiotherapy (53, 56). The extensive use of rabbits in cardiac radiation research since 1968 (New Zealand white rabbits) (57) has resulted in a plethora of available transgenic rabbit models of cardiovascular disease and rabbit-specific antibodies, facilitating their extended use (53, 58–60).
On the contrary, significantly fewer canine models have been chosen in heart radiation studies (16, 61–64) despite the fact that canine models present many similarities on both the organ and cellular levels with humans. Moreover, it has been demonstrated that canine coronary circulation presents similarities to ischemic hearts of elderly people (65–67). This can be attributed to the fact that more strict regulations and regional approval procedures may apply to the use of dogs as well as to the very expensive housing and maintenance. Furthermore, another obvious obstacle is the human-canine bond developed by the society which provokes criticism for the canine studies (68–70).
Manifestations of RIHD are significantly common between pigs and non-human primates with humans (65–67) but these species have also been used by only a few groups as they carry significantly higher costs than other models (19–21, 71).
Although the choice of species, strain, and genotype needs extra attention, other parameters that need to be carefully chosen when designing studies to mimic a clinical scenario of RIHD include the animal age, size, and gender, as they can greatly influence the experimental findings (72, 73). Detailed reporting of these variables will allow for unbiased comparisons between studies.
Radiation-induced myocardial fibrosis
Radiation-induced myocardial fibrosis (RIMF) is considered the final stage of RIHD (74–78) and is characterized by the excess collagen deposition in the damaged cardiac tissue (79–81); nevertheless, the molecular mechanisms remain unclear. RIMF increases the stiffness of the myocardium and decreases the systolic and diastolic functions, leading to the development of a myocardial electrical physiological disorder, arrhythmias, deficient heart function, or death (81). The etiology of the myocardial stiffness (23, 82, 83) lies in the increased presence of cytoplasmic actin stress fibers, on myofibroblasts producing collagen (84, 85) and on an activated inflammatory milieu (86, 87). Diverse mechanisms link RIMF with downstream pathologies, such as arrhythmia, cardiomyopathy, and myocardial ischemia. Fibrosis affecting the heart’s conduction system or cardiomyocyte conduction could interfere with the transmission of electrophysiological signals, causing arrhythmias like AV-nodal bradycardia or heart block (88). Similarly, microvascular damage may result in ischemia affecting these systems to cause these conduction abnormalities. Such microvascular damage is also mechanistically linked to cardiomyopathy following radiotherapy. The reduction of capillaries supplying cardiomyocytes results in hypoxia and death of myocardial tissue with progressive fibrosis. This reduces ejection fraction and increases left ventricular end-diastolic volume. One study of patients with left-sided breast cancer associated Tc-99m sestamibi perfusion deficits with wall motion abnormalities after radiation (89).
The induction of oxidative stress (OS) and the triggering of an early pro-inflammatory environment by irradiation are considered major factors contributing to the initiation and development of RIMF. The inflammatory cascade is initiated by the vascular injury and endothelial dysfunction at approximately six hours post-irradiation (90, 91), which is followed by neutrophil adherence to the endothelium and migration to the damaged heart (92, 93). Various cytokines and growth factors, including Transforming Growth Factor β (TGF-β) (94–96), Tumor Necrosis Factor α (TNF-α) (97–99), Interleukin-1 (IL-1) (100, 101), Interleukin-11 (IL-11) (102–104), Connective Tissue Growth Factor (CTGF) (105), Platelet-derived Growth Factors (PDGFs) (106, 107), Vascular Endothelial Growth Factor (VEGF) and Fibroblast Growth Factor (FGF) (108, 109) flood the damaged heart a few hours after irradiation resulting in higher numbers of infiltrated neutrophils and lymphocytes (110). Additionally, monocytes differentiate into the M2 subtype of macrophages to start secreting TGF-β. In turn, TGF-β differentiates the resident fibroblasts and bone marrow progenitors (111) into myofibroblasts and this event is considered a hallmark of RIHD-related fibrosis (74, 112–114). Subsequently, activated myofibroblasts intensify the synthesis of extracellular matrix (ECM) which consists of a complex combination of collagens, glycoproteins, proteoglycans and matricellular proteins (109, 115). The excessive synthesis of ECM is accompanied by the increased expression of integrins, the tissue inhibitors of matrix metalloproteinases (MMP) (109, 112, 115). Also, the decreased cardiac contraction which has been associated with the development of arrhythmias and mortality can be triggered by the damage to the mechano-electric coupling of the cardiomyocytes caused by the excess ECM. Moreover, deprivation of oxygen and nutrients due to inflammation and fibrosis can further aggravate the adverse cardiac remodeling (116) and impair elasticity and distensibility, promoting ejection fraction and cardiac failure (110). Besides the inflammatory pathways, the proto-oncogenes c-Jun and c-Myc may contribute to the emergence of late fibrosis (74, 117). In addition to the inflammatory signaling, DNA damage response, chronic oxidative stress, chronic hypoxia, epigenetic regulation, and telomere extension have also been inculpated in the radiation-induced fibrosis and the related heart pathologies (74, 118). Another potential mechanism is that the radiation-induced DNA damage which cannot be efficiently repaired in the cardiac tissue (119) can eventually upregulate BAX and downregulate BCL2 in cardiomyocytes, contributing to apoptosis and subsequent development of fibrosis (120, 121) and the progression of the RIHD (39). The above-described mechanisms of radiation-induced myocardial fibrosis are depicted in Figure 1.
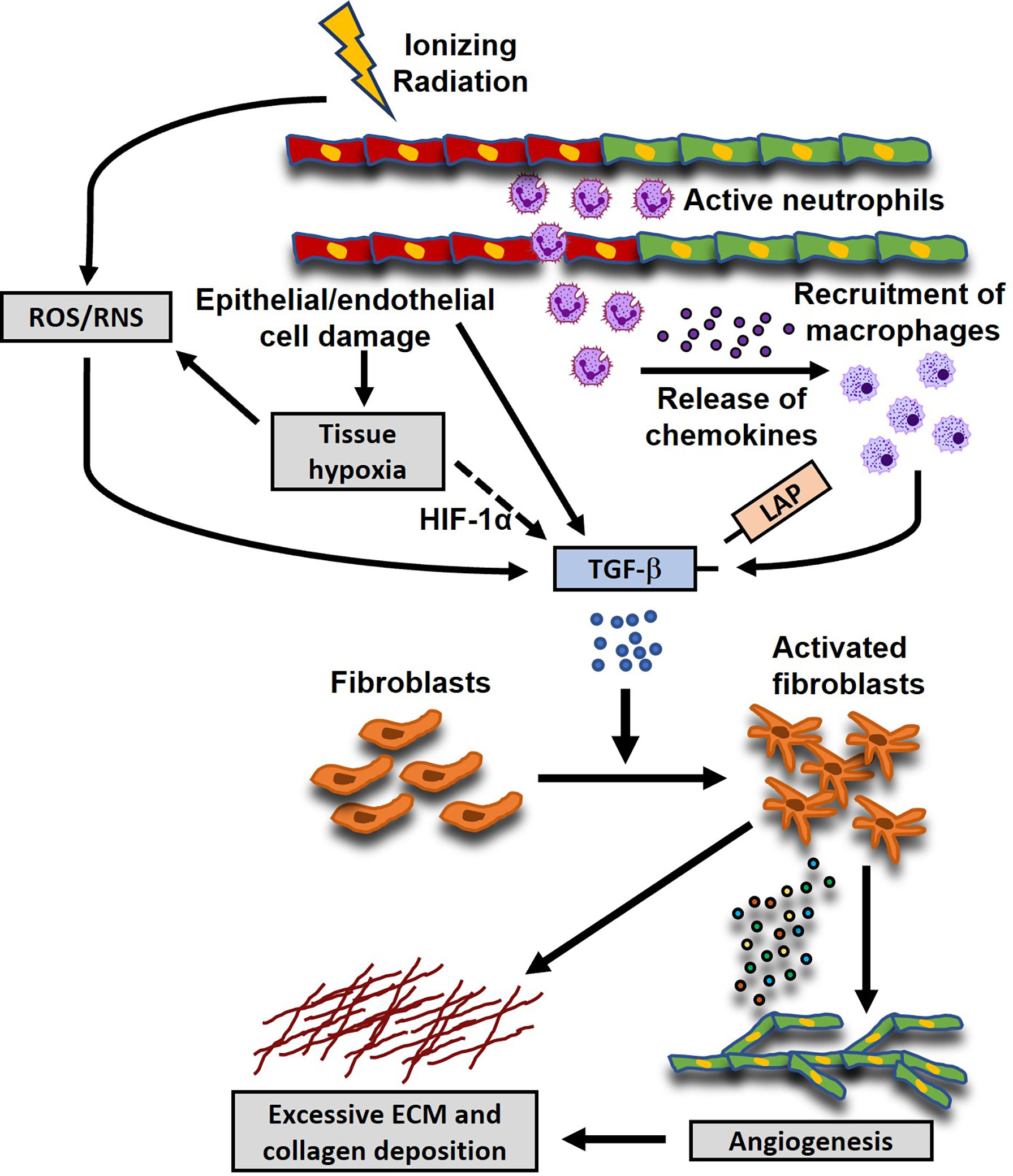
Figure 1 Proposed mechanisms of radiation-induced myocardial fibrosis (RIMF). Ionizing radiation damages epithelial and endothelial (shown here; red- and green-colored cells are damaged and healthy cells, respectively) cells which in turn induce a coordinated cellular response that also involves the secretion of pro-fibrotic cytokines, such as Transforming Growth Factor b (TGF-β). Moreover, the damaged endothelial cells secrete inflammatory chemokines to recruit neutrophils and macrophages to the injured sites, which in turn release pro-fibrotic cytokines like Platelet-Derived Growth Factor (PDGF), basic Fibroblast Growth Factor (FGF), TGF-β, etc., leading to a chronic inflammatory environment. Additionally, radiation causes perturbations of the cellular homeostasis by the excessive production of reactive oxygen and nitrogen species (ROS and RNS), which can also lead to the activation of TGF-β through the dissociation of the Latency Associated Peptide (LAP) from the active mature form of TGF-β. Another potential mechanism is that the damaged vasculature and the uncontrolled tissue remodeling can initiate the TGF-β signaling pathway through the ROS/RNS imbalance or the up-regulation of the expression of Hypoxia-Inducible Factor 1α (HIF-1α). The activated TGF-β signaling pathway leads to the differentiation and activation of the residual and migrated fibroblasts, characterized by the increased secretion of extracellular matrix (ECM) and collagen deposition.
Detection methods for RIHD/RIMF and biomarkers
The current paradigm for cardiovascular care of oncology patients involves a number of steps once a cancer diagnosis is established. Standard practice involves baseline cardiovascular risk assessment based on medical and family history, age, obesity, smoking, and diabetes, cardiotoxicity monitoring during treatment, and long-term cardiovascular surveillance most commonly with serial echocardiography. However, cardiovascular disease is often diagnosed when damage is irreversible and is typically treated in a non-targeted fashion with routine cardioprotective medications such as beta-blockers or statins (122, 123). The identification and use of sensitive radiographic and circulating biomarkers to risk stratify or diagnose cardiotoxicity before tissue damage becomes irreversible is therefore an active area of research, with an overarching goal of informing more targeted and effective therapeutic intervention (Figure 2).
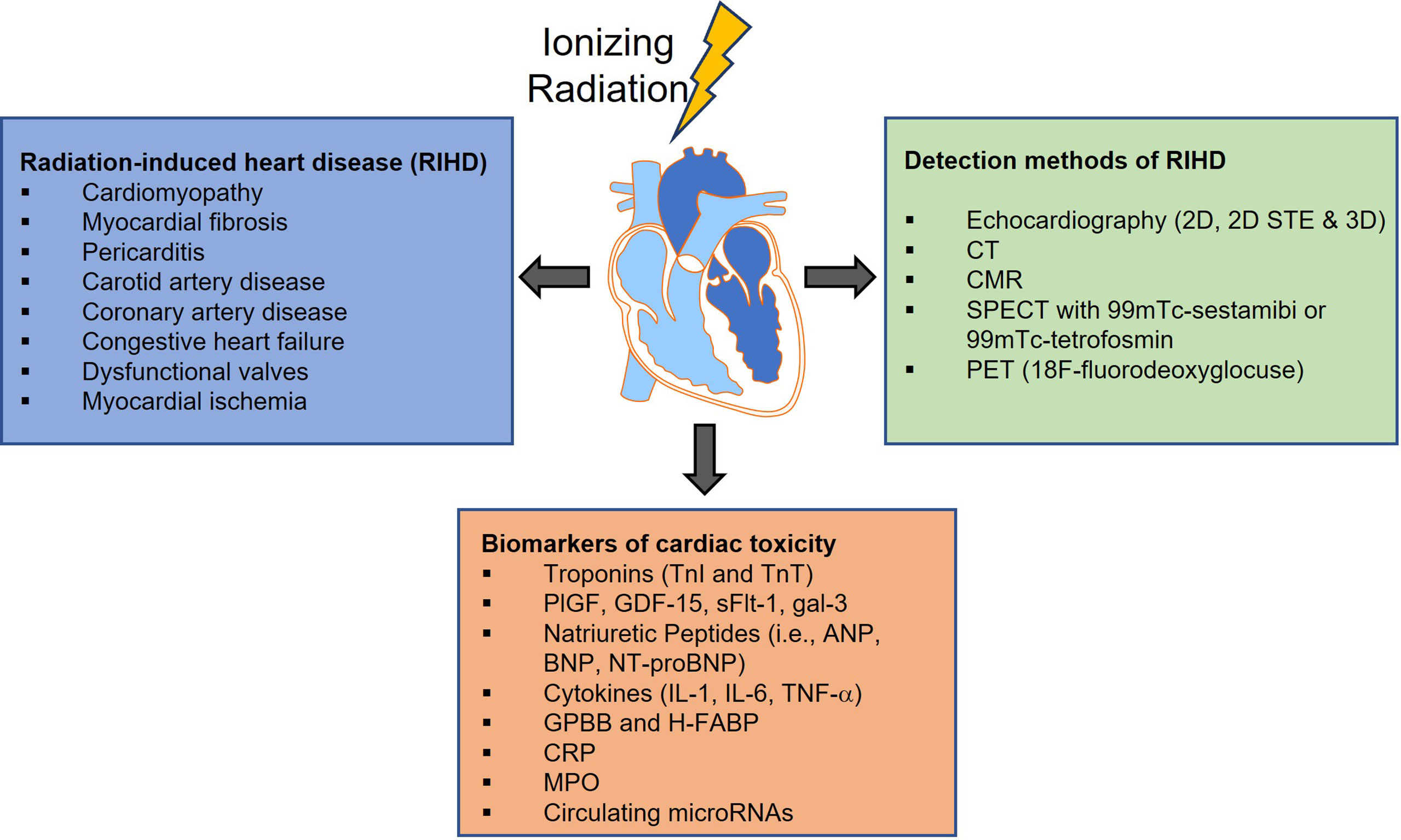
Figure 2 Radiation-induced cardiovascular toxicity can manifest as a variety of clinical pathologies. Although the implementation of technological improvements to the radiotherapy (RT) treatment, has led to more precise targeting and has allowed modest increases in RT dose to the tumor, incidental RT to the heart and development of RIHD is still unavoidable. Over the past decades, numerous methods have been developed for cancer therapy-related cardiovascular disease screening and diagnosis, and more particularly for diagnosing radiation-induced injury. However, cardiovascular disease is often diagnosed when damage is irreversible thus the identification and use of sensitive radiographic and circulating biomarkers to risk stratify or diagnose cardiotoxicity are urgently needed. 2D STE, Two-Dimensional Speckle Tracking Echocardiography; CT, Computed Tomography; CMR, Cardiac Magnetic Resonance imaging; SPECT, Single Photon Emission Computerized Echocardiography; PET, Positron Emission Tomography; PlGF, Placenta Growth Factor; GDF-15, Growth Differentiation Factor-15; sFlt-1, soluble fms-like tyrosine kinase receptor-1; gal-3, galectin-3; ANP, Atrial Natriuretic Peptide; BNP and NT-proBNP, Brain Natriuretic Peptide and its amino-terminal component; IL-1, interleukin-1; IL-6, interleukin-6, TNF-a, Tumor Necrosis Factor-alpha; GPBB, Glycogen Phosphorylase isoenzyme BB; H-FABP, Heart-type Fatty Acid-Binding Protein; CRP, C-Reactive Protein; MPO, Myeloperoxidase.
Echocardiography
2D transthoracic echocardiography has been the most widely used imaging modality for screening baseline cardiovascular risk and for diagnosing cardiac injury, largely due to its widespread availability, non-invasiveness, cost-effectiveness, and validation in diagnosing non-oncologic cardiac disease. Clinicians have relied heavily on >10% decreases in left ventricular ejection fraction (LVEF) to diagnose myocardial dysfunction, and many clinical studies and national guidelines include LVEF changes by echocardiography as a primary or secondary outcome measure (124, 125). Still, 2D echocardiography-derived LVEF has its limitations, including operator variability and nontrivial false-negative rates. The use of more advanced techniques such as 3D echocardiography or ultrasound-based contrast agents has therefore been proposed as appealing alternatives due to their improved image acquisition.
Still, it is now widely accepted that measuring LVEF alone does not typically provide a sufficient assessment of a patient’s overall cardiac function. LVEF provides limited insight into underlying pathophysiology, for example, in discerning whether decreased LVEF is due to intrinsic myocardial injury or vascular damage-causing ischemia. Additionally, even though recovery of LVEF is possible if a diagnosis of the cardiac injury is made early enough, decreases in LVEF are often only detectable once tissue injury is irreparable. As such, LVEF measurements have been increasingly analyzed in conjunction with additional radiographic markers of cardiac mechanics, such as myocardial strain, torsion, and vascular stiffness (126). The global longitudinal strain has emerged as an attractive radiographic biomarker with higher sensitivity in diagnosing subclinical cardiotoxicity, largely due to the fact that changes in strain are usually seen prior to changes in LVEF (127, 128). The ongoing SUCCOUR study is prospectively investigating the initiation of cardioprotective medications following a decrease in global longitudinal strain compared to a decrease in LVEF (129). However, such biomarkers often require more advanced imaging techniques such as speckle-tracking echocardiography (130), which is not always routinely available, and further validation in large studies will be required prior to widespread implementation in the clinic.
Cardiac magnetic resonance imaging
Cardiac magnetic resonance imaging (CMR) is a useful imaging modality that has the potential to address some of the limitations of echocardiography. For one, the high topographic resolution and reproducibility of CMR allows not only for sensitive diagnosis but also aids in phenotyping underlying pathology while sparing patients from repeated radiation exposure. Both T1 and T2 sequences that exploit differential water content and exchange of healthy and diseased cardiac tissue have been widely validated in non-oncologic populations. Changes in tissue structure are easily detectable with increases in T1 and T2, particularly for edema, inflammation, and fibrosis, all of which have been implicated in cancer therapy-related cardiac disease.
CMR also offers a mild but real improvement in measuring myocardial strain, and several studies have reported a decrease in myocardial strain measurements after anthracyclines (131–133). Following radiation therapy, several studies have reported changes in LVEF and left ventricular mass on CMR just 6 months after treatment (134, 135). Several “tagging” sequences, such as harmonic phase (HARP) or displacement encoding (DENSE), have also demonstrated utility in measuring myocardial movement during the cardiac cycle, which can detect subclinical cardiotoxicity early. Nevertheless, CMR also has its disadvantages, including high cost, long image-acquisition time, and limited availability. Until these challenges can be addressed, the use of CMR in cardio-oncologic populations will likely remain limited to those in whom echocardiographic assessments are of poor quality or inconclusive.
Nuclear imaging
While echocardiography and CMR have been the most frequently used imaging modalities for cancer therapy-related cardiovascular disease screening and diagnosis, the role of nuclear imaging has received increasing interest, particularly for diagnosing radiation-induced injury. Recent research has demonstrated that radiation-induced cardiovascular damage is driven at least in large part by inflammatory cell activation and plaque formation along with direct vascular cell damage. The most basic consequence of these events is decreased coronary circulation and myocardial perfusion deficits. Single-photon emission computerized echocardiography (SPECT) with 99mTc-sestamibi or 99mTc-tetrofosmin is a widely recognized imaging modality for the detection of myocardial perfusion deficits secondary to myocardial fibrosis and/or endothelial cell dysfunction. To date, multiple studies have investigated the use of SPECT imaging in thoracic radiation patients and have found convincing associations between perfusion deficits and volume and location of the irradiated field, supporting a volume and dose-dependent relationship between radiation and cardiovascular damage (136–140). However, SPECT imaging is limited by its relatively low spatial resolution and can therefore fail to reveal microvascular dysfunction or perfusion deficits in less easily detectable locations. Additionally, SPECT involves radiation exposure and can be relatively time-consuming for patients due to the long half-life of SPECT isotopes.
Positron emission tomography (PET) imaging for cardiac disease is another imaging modality that has been increasingly implemented, with the potential to identify a variety of distinct cardiac pathologies depending on the radiotracer used (141, 142). For example, 18F-fluorodeoxyglucose (18F-FDG) is a well-known marker of inflammation in the body, and in the case of cardiac PET, 18F-FDG can identify inflammation in the cardiac vasculature which often precedes atherosclerotic plaque progression and rupture (143, 144). Patient preparation for cardiac PET does involve a fasting period of 12-18 hours with or without a diet low in carbohydrates for 12-24 hours to suppress basal myocardial glucose update. Nevertheless, a major advantage of FDG-PET imaging is its ability to detect early metabolic changes in the myocardium that occur prior to decreases in LVEF.
It is also worth mentioning a number of novel molecular tracers that have become more widely available in clinical care in recent years. For example, tracers such as Rubidium-82 and [13N]Ammonia are particularly useful for measuring myocardial blood flow in the microvasculature, offering higher resolution than SPECT (145). Additionally, 99mTc-labeled annexin V, a plasma protein that can detect cellular apoptosis in unstable plaques, has already been used to reveal dose-dependent cell death prior to changes in echocardiography (146). Investigation in large cohorts is warranted and will be necessary to establish the role of these radiotracers in diagnosing and monitoring subclinical cardiotoxicity in cancer patients.
Plasma biomarkers
Identifying and monitoring cardiovascular toxicity during and after treatment with blood-based biomarkers is an attractive clinical approach that can complement radiographic assessments. Circulating biomarkers are typically reproducible and easily obtainable measures that do not rely on operator skill. Additionally, circulating biomarkers have the added benefit of reflecting underlying disease pathophysiology. All biomarkers that mentioned in this section and are related to radiation-induced heart disease as well as the putative biomarkers of cardiac toxicity associated with cancer treatment are summarized in Tables 1 and 2, respectively.
Cardiac troponins and natriuretic peptides have been at the center of cardiovascular disease diagnosis both in oncologic and non-oncologic patient populations. Similar to well-known markers of myocardial stress and heart failure, increases in troponin and B-type natriuretic peptide (BNP) have been used to signal cardiac injury at various stages in a patient’s cancer treatment, including in baseline risk assessment, monitoring during therapy, and surveillance after therapy (158, 159). To date, multiple studies have evaluated increases in troponin and BNP following cardiotoxic therapy administration with some promising but mixed results (148–155, 160, 161). A recent meta-analysis of 61 trials including 5691 cancer patients reported that anticancer therapy was associated with increases in the levels of troponin was in turn associated with a higher risk for cardiac dysfunction (160). Nevertheless, mixed study results have highlighted the need for more sensitive and specific biochemical markers of cardiovascular damage (162).
Several novel circulating biomarkers that have been proposed include markers of inflammation, oxidative stress, fibrosis, angiogenesis, and vascular remodeling, including cytokines, interleukins, myeloperoxidase, C-reactive protein, galectin-3, placental growth factor, and growth differentiation factor-15 (Figure 2) (157, 163). For example, one study involving 54 women with human epidermal growth factor receptor 2 (HER2)-positive early-stage breast cancer treated with trastuzumab showed that peak levels of high-sensitivity C-reactive protein (hs-CRP) were detected after a median of 78 days before decreases in LVEF were observed (156). Moreover, biomarkers analysis in a multicenter study of 78 patients with breast cancer demonstrated early increases in myeloperoxidase and Troponin I (TnI) levels following treatment with doxorubicin and trastuzumab (155). Additionally, microRNAs which mediate cardiac hypertrophy and fibrosis, and genome-wide association studies represent relatively untapped resources in the field of cardio-oncology (147, 164–167). Their role in diagnosing cardiovascular dysfunction remains to be determined in this patient population.
Preclinical endpoints for RIHD
Preclinical studies benefit from many of the same techniques used to assess cardiovascular dysfunction in patients, but additionally see more frequent use of the gold standard for detecting RIMF: histologic analysis of cardiac tissue. Colorimetric stains, such as Masson’s trichrome (47) and picrosirius red (168), have been used to detect and quantify extracellular matrix components in preclinical models of RIHD. Similarly, more advanced microscopy techniques like second-harmonic generation (169) or atomic force microscopy (170) have been used to assess fibrillar collagen structure and tissue stiffness in other cardiovascular diseases. These techniques may provide insight into the supramolecular assembly of collagen and how it limits contractility in RIHD. The spatial dimension of cardiac histology allows for the assessment of cell-cell interactions, immune cell infiltration, and patterns of gene expression. Especially with the advent of novel spatial transcriptomic (171), tissue-based analysis can provide additional insight into the genetic programs induced in and around regions of RIMF.
Meanwhile, transthoracic echocardiography (TTE), CMR, or nuclear medicine studies allows for functional analysis in mouse models of RIHD. Small animal TTE platforms may be utilized to assess LVEF, ventricular wall motion abnormalities, valvular disease, and other pathologies in rodent RIHD studies (172), while clinical ultrasound machines are sufficient for larger animal studies. Cardiac MRI using high-resolution small animal MRI scanners offer similar endpoints as TTE for use in studies of RIHD (173). Similarly, Tc-99m-Sestamibi SPECT has been utilized to detect perfusion deficits in studies of RIHD (47) and myocardial infarction (174). Furthermore, animal models excel in biomarker detection because serial blood sampling for analysis of serum proteins may be correlated with imaging and/or histologic findings in the same animal.
Synergistic cardiotoxicities with chemoradiation or radioimmunotherapy
Multiple studies have shown the clinical and therapeutic implications of combining radiotherapy with established chemotherapy and/or immunotherapy in the treatment of solid tumors (175–177). However, these potent and intense anti-tumor combined therapies have been associated with considerable tissue toxicities, most notably cardiotoxicities, which may adversely affect the quality associated with the improved outcome. These toxicities have been well characterized and remain a focus area for improving the therapeutic index of thoracic tumor treatment. Therefore, oncologists and cardiologists are called to design a multimodality treatment approach by integrating the latest clinical findings in cardiotoxicity, to achieve the best overall care for cancer patients undergoing chemoradiotherapy and/or immunoradiotherapy.
Chemotherapy and radiation
The use of concurrent chemotherapy and radiotherapy (cCRT) against a variety of solid tumors, such as esophageal, breast, and non-small cell lung cancer (NSCLC) has been a promising strategy for the synergistic enhancement of local and distal tumor control, which in many cases has been translated into survival benefits (178–182). Moreover, numerous preclinical studies have also described that the combination of chemotherapeutic agents with radiotherapy could be a promising strategy for synergistic enhancement of treatment efficacy (183, 184). However, this multi-modality treatment has also been shown to increase risks for adverse cardiac events such as ischemic heart disease, myocardial infarction, heart failure, and cardiac dysfunction (185–187). These side effects can vary among individuals and are often correlated with the cumulative dose of the treatment.
In a retrospective analysis of a large cohort of older patients with NSCLC, patients who received radiotherapy or chemoradiotherapy treatment in the left lung had a significantly increased risk of ischemic heart disease compared to those who received either radiation-only (HR = 1.18, 95% CI 1.05–1.34) or chemoradiation (HR = 1.29, 95% CI 1.09–1.52) treatment in the right lung (187). Similar findings have been reported in other studies showing that radiotherapy to the left chest has been linked to a higher risk of cardiac toxicity and subsequent risk of developing chronic cardiac disorders compared to the right chest radiotherapy (188–190). Additionally, in another retrospective analysis of NSCLC patients, there was an increased risk of major adverse cardiac events (cardiac death, unstable angina, myocardial infarction, heart failure hospitalization, or coronary bypass grafting) in patients receiving thoracic chemoradiotherapy (adjusted hazard ratio: 1.05/Gy) (191). Chemoradiotherapy is also an effective treatment in the management of superficial esophageal cancer. In a retrospective analysis of 80 patients with submucosal invasive non-metastatic esophageal cancer treated with 5-fluorouracil or cisplatin and 60 Gy in 30 fractions, 13 patients developed severe cardiac events and the 5-year cumulative cardiac event occurrence rate was 16.3% (192). In the same study, they also showed that the level of the heart’s exposure to radiation was a major prognostic factor for these occurrences. Patients exposed to radiation with more than 280 mL of V5000 cGy had a 16.8 times more likely chance of developing these cardiac events than those who received a smaller volume (192). In a prospective study of patients with non-metastatic esophageal cancer, the authors showed that 30% of the patients developed myocardial fibrosis and/or reversible ischemia as early as 3 months following neoadjuvant chemoradiation (193). Furthermore, several large-scale studies have demonstrated similar findings that chemoradiotherapy increases the risk of cardiotoxicity in lymphoma (194, 195) and esophageal cancer treatment (196) as compared to radiotherapy alone. The design of comprehensive treatment strategies will allow patients to receive potentially curative benefits from multi-modality treatments and minimize the cardiac-related toxicities.
Immunotherapy and radiation
The discovery of the immune-checkpoint inhibitors [(ipilimumab, an anti-cytotoxic T lymphocyte-associated protein 4 (CTLA-4) antibody, programmed death 1 (PD-1) and programmed death-ligand 1 (PD-L1)], has placed immunotherapy to the front-line of cancer treatment, especially for advanced NSCLC (197, 198). Furthermore, in some types of cancer, immunotherapy has proven more effective (as a monotherapy) than the standard care treatment. This success also encouraged researchers to combine immunotherapy with other conventional therapies, i.e., radiotherapy, to improve the effectiveness. As in chemoradiotherapy, the combination of immunotherapy with radiotherapy has shown synergistic effects in both local and systemic tumor control (199–201), possibly due to the synergistic immune activation. For instance, in a phase III randomized-controlled, double-blinded trial with stage III non-small-cell lung cancer patients undergoing chemoradiotherapy, the addition of durvalumab (anti-PDL1 antibody) showed a significant increase in progression-free survival (198). Interestingly, an abscopal effect has also been reported post combined radiotherapy with immune checkpoint inhibitors (202, 203). Moreover, multiple preclinical studies have also demonstrated synergistic effects of combined immunotherapy with radiotherapy on treatment efficacy (202, 204–206). Although these studies support the beneficial effects of immunoradiotherapy in tumor control mainly through increased immune-mediated antitumor activity, they also resulted in increased rates of cumulative cardiotoxicity (207). In a preclinical study by Du et al., the authors reported radiation-induced cardiac dysfunction and acute fibrosis which was exacerbated by the concurrent treatment with PD-1 blockade (208). In another in vivo study, the combination of thoracic irradiation with anti-PD-1 antibody significantly decreased the survival of the C57Bl/6 mice compared to radiation alone and that was positively correlated with the T cell infiltration into the lung and heart tissues (209).
Although the clinical use of immune checkpoint inhibitors in cancer treatment is exponentially increased in the last decade, the pathology and treatment of the immune-related toxicities are complex and still not well known. Therefore, more screening procedures and extensive research on the pathophysiology of cardiotoxicity are required. Additionally, the optimization of the radiation treatment planning (dose, timing, etc.) and the identification of sensitive biomarkers of cardiotoxicity will give further access to the promising treatment strategy of immunoradiotherapy.
Targeted therapies and radiation
Similar to immunotherapy, targeted therapies are now commonly used in the comprehensive care of cancer patients who also often receive chemotherapy or radiation. However, there is little data regarding the combined impact of various targeted therapies and radiotherapy on cardiotoxicity. A few studies have assessed the combination of radiotherapy and Trastuzumab, a monoclonal antibody used in the treatment of HER2 receptor positive breast cancer. Three studies found no increase in acute cardiotoxicity in breast cancer patients treated with chemoradiotherapy and trastuzumab compared to chemoradiation alone, suggesting this combination therapy is clinically feasible (210–212). Yet, these studies are potentially confounded by the cardiotoxicities associated with anthracycline-containing chemotherapy regimens which limits our understanding of the toxicities induced by the combination of radiotherapy and trastuzumab. A clinical trial which assessed left-sided radiotherapy with concurrent trastuzumab compared to radiotherapy alone found a non-significant increase in the incidence of LVEF dysfunction (7.8% vs 4.1%, respectively), though treatment was generally well-tolerated. They also noted that the sequencing of RT and trastuzumab was an independent risk factor for the development of cardiotoxicity (213). This suggests that further study is warranted to understand the adverse effects of this combination. More generally, potential additive or synergistic toxicities of other targeted therapies used in combination with radiotherapy (and the associated incidental heart irradiation) should be investigated in the context of RIHD due to the limited clinical datasets available. For example, tyrosine kinase inhibitors, such as Osimertinib which is used in lung cancer, or anti-angiogenic agents, like bevacizumab, have been found to increase the risk of adverse events in patients receiving thoracic radiotherapy (214, 215). However, cardiotoxicity has not been specifically investigated in these settings. This is an area where the use of well-controlled preclinical models may prove useful, given how many potential combinations exist between targeted therapies and thoracic radiotherapy.
Conclusions
Despite the advances in the delivery of thoracic radiation therapy, radiation-induced heart disease constitutes a growing clinical issue that severely compromises the quality of life of cancer survivors treated with thoracic RT alone or in combination with genotoxic chemotherapy or/and immunotherapy. Currently, as the multi-modality treatments have been considered successful approaches for cancer therapy, we need to reconsider the potential synergistic cardiac-related adverse effects. Although there is considerable progress in delineating the molecular and pathophysiological mechanisms behind the RIHD, the underlying development of RIHD still remains not thoroughly understood, therefore, new strategies need to be developed that can ameliorate or even reverse the course of RIHD. Therefore, there is an increasing need for more clinically relevant preclinical models to identify biomarkers and targetable mediators of RIHD which will lead to the development of prognostic screening techniques and new treatment options.
Methods
Methods: A literature review of publications describing the preclinical models of RIHD/RIMF, the radiographic and circulating biomarkers, synergistic heart toxicities seen in radiotherapy combined with chemo/immunotherapy was performed. Specific databases utilized included PubMed and clinicaltrials.gov. Additionally, specific keywords have been used such as “Radiation-Induced heart disease AND rodents”, “Radiation-Induced heart disease AND rabbits”, “Radiation-Induced heart disease AND canine”, “Radiation-Induced heart disease AND pigs”, “Radiation-Induced heart disease AND non-human primates”, “Radiation-Induced myocardial fibrosis AND mechanisms AND rodents”, “Radiation-Induced myocardial fibrosis AND factors”, “Radiation-Induced myocardial fibrosis AND biomarkers”, “Radiation-Induced myocardial fibrosis AND radiographic”, “Radiation-Induced heart disease AND preclinical endpoints”, “cardiac toxicity AND radiation AND chemotherapy”, “cardiac toxicity AND radiation AND immunotherapy”, and other closely related terms.
Author contributions
AD, AV, HA, BB, and IV conceptualized, wrote, and reviewed the manuscript. All authors contributed to the article and approved the submitted version.
Funding
This work was partially supported by the National Center for Advancing Translational Sciences of the National Institutes of Health under Award Number UL1TR001878 and the Institute for Translational Medicine and Therapeutics’ (ITMAT) Transdisciplinary Program in Translational Medicine and Therapeutics to IV. The content is solely the responsibility of the authors and does not necessarily represent the official views of the NIH.
Conflict of interest
The authors declare that the research was conducted in the absence of any commercial or financial relationships that could be construed as a potential conflict of interest.
Publisher’s note
All claims expressed in this article are solely those of the authors and do not necessarily represent those of their affiliated organizations, or those of the publisher, the editors and the reviewers. Any product that may be evaluated in this article, or claim that may be made by its manufacturer, is not guaranteed or endorsed by the publisher.
References
1. Miller KD, Nogueira L, Mariotto AB, Rowland JH, Yabroff KR, Alfano CM, et al. Cancer treatment and survivorship statistic. CA Cancer J Clin (2019) 69:363–85. doi: 10.3322/caac.21565
2. Sung H, Ferlay J, Siegel RL, Laversanne M, Soerjomataram I, Jemal A, et al. Global cancer statistics 2020: GLOBOCAN estimates of incidence and mortality worldwide for 36 cancers in 185 countries. CA Cancer J Clin (2021) 71:209–49. doi: 10.3322/caac.21660
3. Zamorano JL, Gottfridsson C, Asteggiano R, Atar D, Badimon L, Bax JJ, et al. The cancer patient and cardiology. Eur J Heart Fail (2020) 22:2290–309. doi: 10.1002/ejhf.1985
4. Wilcox NS, Rotz SJ, Mullen M, Song EJ, Ky Hamilton B, Moslehi J, et al. Sex-specific cardiovascular risks of cancer and its therapies. Circ Res (2022) 130:632–51. doi: 10.1161/CIRCRESAHA.121.319901
5. Bhakta N, Liu Q, Yeo F, Baassiri M, Ehrhardt MJ, Srivastava DK, et al. Cumulative burden of cardiovascular morbidity in paediatric, adolescent, and young adult survivors of hodgkin's lymphoma: an analysis from the St Jude lifetime cohort study. Lancet Oncol (2016) 17:1325–34. doi: 10.1016/S1470-2045(16)30215-7
6. Speirs CK, Dewees TA, Rehman S, Molotievschi A, Velez MA, Mullen D, et al. Heart dose is an independent dosimetric predictor of overall survival in locally advanced non-small cell lung cancer. J Thorac Oncol (2017) 12:293–301. doi: 10.1016/j.jtho.2016.09.134
7. Wang K, Eblan MJ, Deal AM, Lipner M, Zagar TM, Wang Y, et al. Cardiac toxicity after radiotherapy for stage III non-Small-Cell lung cancer: Pooled analysis of dose-escalation trials delivering 70 to 90 gy. J Clin Oncol (2017) 35:1387–94. doi: 10.1200/JCO.2016.70.0229
8. Jumeau R, Ozsahin M, Schwitter J, Elicin O, Reichlin T, Roten L, et al. Stereotactic radiotherapy for the management of refractory ventricular tachycardia: Promise and future directions. Front Cardiovasc Med (2020) 7:108. doi: 10.3389/fcvm.2020.00108
9. Ramella S, D'angelillo RM. Proton beam or photon beam radiotherapy in the treatment of non-small-cell lung cancer. Lancet Oncol (2020) 21:873–5. doi: 10.1016/S1470-2045(20)30246-1
10. Galper SL, Yu JB, Mauch PM, Strasser JF, Silver B, Lacasce A, et al. Clinically significant cardiac disease in patients with Hodgkin lymphoma treated with mediastinal irradiation. Blood (2011) 117:412–8. doi: 10.1182/blood-2010-06-291328
11. Darby SC, Ewertz M, Hall P. Ischemic heart disease after breast cancer radiotherapy. N Engl J Med (2013) 368: 987–98. doi: 10.1056/NEJMoa1209825
12. Eaton BR, Pugh SL, Bradley JD, Masters G, Kavadi VS, Narayan S, et al. Institutional enrollment and survival among NSCLC patients receiving chemoradiation: NRG oncology radiation therapy oncology group (RTOG) 0617. J Natl Cancer Inst (2016) 108. doi: 10.1093/jnci/djw034
13. Atkins KM, Chaunzwa TL, Lamba N, Bitterman DS, Rawal B, Bredfeldt J, et al. Association of left anterior descending coronary artery radiation dose with major adverse cardiac events and mortality in patients with non-small cell lung cancer. JAMA Oncol (2021) 7:206–19. doi: 10.1001/jamaoncol.2020.6332
14. Eltringham JR, Fajardo LF, Stewart JR. Adriamycin cardiomyopathy: enhanced cardiac damage in rabbits with combined drug and cardiac irradiation. Radiology (1975) 115:471–2. doi: 10.1148/115.2.471
15. Yang VV, Stearner SP, Tyler SA. Radiation-induced changes in the fine structure of the heart: comparison of fission neutrons and 60Co gamma rays in the mouse. Radiat Res (1976) 67:344–60. doi: 10.2307/3574423
16. Mcchesney SL, Gillette EL, Powers BE. Radiation-induced cardiomyopathy in the dog. Radiat Res (1988) 113:120–32. doi: 10.2307/3577185
17. Schultz-Hector S, Balz K. Radiation-induced loss of endothelial alkaline phosphatase activity and development of myocardial degeneration. an ultrastructural study. Lab Invest (1994) 71:252–60.
18. Labudova O, Hardmeier R, Rink H, Lubec G. The transcription of the XRCC1 gene in the heart of radiation-resistant and radiation-sensitive mice after ionizing irradiation. Pediatr Res (1997) 41:435–9. doi: 10.1203/00006450-199703000-00022
19. Virmani R, Farb A, Carter AJ, Jones RM. Comparative pathology: radiation-induced coronary artery disease in man and animals. Semin Interv Cardiol (1998) 3:163–72.
20. Virmani R, Farb A, Carter AJ, Jones RM. Pathology of radiation-induced coronary artery disease in human and pig. Cardiovasc Radiat Med (1999) 1:98–101. doi: 10.1016/S1522-1865(98)00010-9
21. Debo RJ, Lees CJ, Dugan GO, Caudell DL, Michalson KT, Hanbury DB, et al. Late effects of total-body gamma irradiation on cardiac structure and function in Male rhesus macaques. Radiat Res (2016) 186:55–64. doi: 10.1667/RR14357.1
22. Lauk S, Trott KR. Radiation induced heart disease in hypertensive rats. Int J Radiat Oncol Biol Phys (1988) 14:109–14. doi: 10.1016/0360-3016(88)90058-2
23. Boerma M, Roberto KA, Hauer-Jensen M. Prevention and treatment of functional and structural radiation injury in the rat heart by pentoxifylline and alpha-tocopherol. Int J Radiat Oncol Biol Phys (2008) 72:170–7. doi: 10.1016/j.ijrobp.2008.04.042
24. Boerma M, Hauer-Jensen M. Preclinical research into basic mechanisms of radiation-induced heart disease. Cardiol Res Pract (2010) 2011. doi: 10.4061/2011/858262
25. Boerma M. Experimental radiation-induced heart disease: past, present, and future. Radiat Res (2012) 178:1–6. doi: 10.1667/RR2933.1
26. Gurses I, Ozeren M, Serin M, Yucel N, Erkal HS. Histopathological evaluation of melatonin as a protective agent in heart injury induced by radiation in a rat model. Pathol Res Pract (2014) 210:863–71. doi: 10.1016/j.prp.2014.08.006
27. He W, Tang Y, Li C, Zhang X, Huang S, Tan B, et al. Exercise enhanced cardiac function in mice with radiation-induced heart disease via the FNDC5/Irisin-dependent mitochondrial turnover pathway. Front Physiol (2021) 12:739485. doi: 10.3389/fphys.2021.739485
28. Li L, Nie X, Zhang P, Huang Y, Ma L, Li F, et al. Dexrazoxane ameliorates radiation-induced heart disease in a rat model. Aging (Albany NY) (2021) 13:3699–711. doi: 10.18632/aging.202332
29. Ortiz De Choudens S, Sparapani R, Narayanan J, Lohr N, Gao F, Fish BL, et al. Lisinopril mitigates radiation-induced mitochondrial defects in rat heart and blood cells. Front Oncol (2022) 12:828177. doi: 10.3389/fonc.2022.828177
30. Zaragoza C, Gomez-Guerrero C, Martin-Ventura JL, Blanco-Colio L, Lavin B, Mallavia B, et al. Animal models of cardiovascular diseases. J BioMed Biotechnol (2011) 2011:497841. doi: 10.1155/2011/497841
31. Gabriels K, Hoving S, Seemann I, Visser NL, Gijbels MJ, Pol JF, et al. Local heart irradiation of ApoE(-/-) mice induces microvascular and endocardial damage and accelerates coronary atherosclerosis. Radiother Oncol (2012) 105:358–64. doi: 10.1016/j.radonc.2012.08.002
32. Hoving S, Heeneman S, Gijbels MJ, Te Poele JA, Visser N, Cleutjens J, et al. Irradiation induces different inflammatory and thrombotic responses in carotid arteries of wildtype C57BL/6J and atherosclerosis-prone ApoE(-/-) mice. Radiother Oncol (2012) 105:365–70. doi: 10.1016/j.radonc.2012.11.001
33. Gabriels K, Hoving S, Gijbels MJ, Pol JF, Te Poele JA, Biessen EA, et al. Irradiation of existing atherosclerotic lesions increased inflammation by favoring pro-inflammatory macrophages. Radiother Oncol (2014) 110:455–60. doi: 10.1016/j.radonc.2014.01.006
34. Patties I, Haagen J, Dörr W, Hildebrandt G, Glasow A. Late inflammatory and thrombotic changes in irradiated hearts of C57BL/6 wild-type and atherosclerosis-prone ApoE-deficient mice. Strahlenther Onkol (2015) 191:172–9. doi: 10.1007/s00066-014-0745-7
35. Oppi S, Luscher TF, Stein S. Mouse models for atherosclerosis research-which is my line? Front Cardiovasc Med (2019) 6:46. doi: 10.3389/fcvm.2019.00046
36. Schlaak RA, Frei A, Schottstaedt AM, Tsaih SW, Fish BL, Harmann L, et al. Mapping genetic modifiers of radiation-induced cardiotoxicity to rat chromosome 3. Am J Physiol Heart Circ Physiol (2019) 316:H1267–80. doi: 10.1152/ajpheart.00482.2018
37. Stewart FA, Heeneman S, Te Poele J, Kruse J, Russell NS, Gijbels M, et al. Ionizing radiation accelerates the development of atherosclerotic lesions in ApoE-/- mice and predisposes to an inflammatory plaque phenotype prone to hemorrhage. Am J Pathol (2006) 168:649–58. doi: 10.2353/ajpath.2006.050409
38. Yamamoto Y, Minami M, Yoshida K, Nagata M, Miyata T, Yang T, et al. Irradiation accelerates plaque formation and cellular senescence in flow-altered carotid arteries of apolipoprotein e knock-out mice. J Am Heart Assoc (2021) 10:e020712. doi: 10.1161/JAHA.120.020712
39. Lee CL, Moding EJ, Cuneo KC, Li Y, Sullivan JM, Mao L, et al. p53 functions in endothelial cells to prevent radiation-induced myocardial injury in mice. Sci Signal (2012) 5:ra52. doi: 10.1126/scisignal.2002918
40. Mitchel RE, Hasu M, Bugden M, Wyatt H, Hildebrandt G, Chen YX, et al. Low-dose radiation exposure and protection against atherosclerosis in ApoE(-/-) mice: the influence of P53 heterozygosity. Radiat Res (2013) 179:190–9. doi: 10.1667/RR3140.1
41. Schlaak RA, Frei A, Senthilkumar G, Tsaih SW, Wells C, Mishra J, et al. Differences in expression of mitochondrial complexes due to genetic variants may alter sensitivity to radiation-induced cardiac dysfunction. Front Cardiovasc Med (2020) 7:23. doi: 10.3389/fcvm.2020.00023
42. Castle KD, Chen M, Wisdom AJ, Kirsch DG. Genetically engineered mouse models for studying radiation biology. Transl Cancer Res (2017) 6:S900–s913. doi: 10.21037/tcr.2017.06.19
43. Jarrett KE, Lee CM, Yeh YH, Hsu RH, Gupta R, Zhang M, et al. Somatic genome editing with CRISPR/Cas9 generates and corrects a metabolic disease. Sci Rep (2017) 7:44624. doi: 10.1038/srep44624
44. Wang X, Huang R, Zhang L, Li S, Luo J, Gu Y, et al. A severe atherosclerosis mouse model on the resistant NOD background. Dis Model Mech (2018) 11. doi: 10.1242/dmm.033852
45. Lee CL, Min H, Befera N, Clark D, Qi Y, Das S, et al. Assessing cardiac injury in mice with dual energy-microCT, 4D-microCT, and microSPECT imaging after partial heart irradiation. Int J Radiat Oncol Biol Phys (2014) 88:686–93. doi: 10.1016/j.ijrobp.2013.11.238
46. Sievert W, Stangl S, Steiger K, Multhoff G. Improved overall survival of mice by reducing lung side effects after high-precision heart irradiation using a small animal radiation research platform. Int J Radiat Oncol Biol Phys (2018) 101:671–9. doi: 10.1016/j.ijrobp.2018.02.017
47. Dreyfuss AD, Goia D, Shoniyozov K, Shewale SV, Velalopoulou A, Mazzoni S, et al. A novel mouse model of radiation-induced cardiac injury reveals biological and radiological biomarkers of cardiac dysfunction with potential clinical relevance. Clin Cancer Res (2021) 27 (8):2266–2276. doi: 10.1158/1078-0432.CCR-20-3882
48. Mayosi BM, Kardos A, Davies CH, Gumedze F, Hovnanian A, Burge S, et al. Heterozygous disruption of SERCA2a is not associated with impairment of cardiac performance in humans: implications for SERCA2a as a therapeutic target in heart failure. Heart (2006) 92:105–9. doi: 10.1136/hrt.2004.051037
49. Camacho P, Fan H, Liu Z, He JQ. Small mammalian animal models of heart disease. Am J Cardiovasc Dis (2016) 6:70–80.
50. Vegter EL, Ovchinnikova ES, Silljé HHW, Meems LMG, van der Pol A, van der Velde AR, et al. Rodent heart failure models do not reflect the human circulating microRNA signature in heart failure. PloS One (2017) 12:e0177242. doi: 10.1371/journal.pone.0177242
51. Pogwizd SM, Bers DM. Cellular basis of triggered arrhythmias in heart failure. Trends Cardiovasc Med (2004) 14:61–6. doi: 10.1016/j.tcm.2003.12.002
52. Bers DM. Altered cardiac myocyte Ca regulation in heart failure. Physiol (Bethesda) (2006) 21:380–7. doi: 10.1152/physiol.00019.2006
53. Pogwizd SM, Bers DM. Rabbit models of heart disease. Drug Discov Today Dis Models (2008) 5:185–93. doi: 10.1016/j.ddmod.2009.02.001
54. Ponnaluri AV, Perotti LE, Liu M, Qu Z, Weiss JN, Ennis DB, et al. Electrophysiology of heart failure using a rabbit model: From the failing myocyte to ventricular fibrillation. PloS Comput Biol (2016) 12:e1004968. doi: 10.1371/journal.pcbi.1004968
55. Quinn TA, Kohl P. Rabbit models of cardiac mechano-electric and mechano-mechanical coupling. Prog Biophys Mol Biol (2016) 121:110–22. doi: 10.1016/j.pbiomolbio.2016.05.003
56. Robinson CG, Samson PP, Moore KMS, Hugo GD, Knutson N, Mutic S, et al. Phase I/II trial of electrophysiology-guided noninvasive cardiac radioablation for ventricular tachycardia. Circulation (2019) 139:313–21. doi: 10.1161/CIRCULATIONAHA.118.038261
57. Tewart JR, Fujardo LF, Cohn KE, Page V. Experimental radiation-induced heart disease in rabbits. Radiology (1968) 91:814–7. doi: 10.1148/91.4.814
58. Peng X. Transgenic rabbit models for studying human cardiovascular diseases. Comp Med (2012) 62:472–9.
59. Lu R, Yuan T, Wang Y, Zhang T, Yuan Y, Wu D, et al. Spontaneous severe hypercholesterolemia and atherosclerosis lesions in rabbits with deficiency of low-density lipoprotein receptor (LDLR) on exon 7. EBioMedicine (2018) 36:29–38. doi: 10.1016/j.ebiom.2018.09.020
60. Hornyik T, Rieder M, Castiglione A, Major P, Baczko I, Brunner M, et al. Transgenic rabbit models for cardiac disease research. Br J Pharmacol (2022) 179:938–57. doi: 10.1111/bph.15484
61. Barnes M, Pass H, Deluca A, Tochner Z, Potter D, Terrill R, et al. Response of the mediastinal and thoracic viscera of the dog to intraoperative radiation therapy (IORT). Int J Radiat Oncol Biol Phys (1987) 13:371–8. doi: 10.1016/0360-3016(87)90011-3
62. Yan R, Song J, Wu Z, Guo M, Liu J, Li J, et al. Detection of myocardial metabolic abnormalities by 18F-FDG PET/CT and corresponding pathological changes in beagles with local heart irradiation. Korean J Radiol (2015) 16:919–28. doi: 10.3348/kjr.2015.16.4.919
63. Song J, Yan R, Wu Z, Li J, Yan M, Hao X, et al. (13)N-ammonia PET/CT detection of myocardial perfusion abnormalities in beagle dogs after local heart irradiation. J Nucl Med (2017) 58:605–10. doi: 10.2967/jnumed.116.179697
64. El-Sherif O, Xhaferllari I, Sykes J, Butler J, Dekemp RA, Renaud J, et al. [(18)F]FDG cardiac PET imaging in a canine model of radiation-induced cardiovascular disease associated with breast cancer radiotherapy. Am J Physiol Heart Circ Physiol (2019) 316:H586–h595. doi: 10.1152/ajpheart.00273.2018
65. Hearse DJ, Sutherland FJ. Experimental models for the study of cardiovascular function and disease. Pharmacol Res (2000) 41:597–603. doi: 10.1006/phrs.1999.0651
66. Recchia FA, Lionetti V. Animal models of dilated cardiomyopathy for translational research. Vet Res Commun (2007) 31 Suppl 1:35–41. doi: 10.1007/s11259-007-0005-8
67. Camacho P, Fan H, Liu Z, He JQ. Large Mammalian animal models of heart disease. J Cardiovasc Dev Dis (2016) 3. doi: 10.3390/jcdd3040030
68. Hasiwa N, Bailey J, Clausing P, Daneshian M, Eileraas M, Farkas S, et al. Critical evaluation of the use of dogs in biomedical research and testing in Europe. Altex (2011) 28:326–40. doi: 10.14573/altex.2011.4.326
69. Milani-Nejad N, Janssen PM. Small and large animal models in cardiac contraction research: advantages and disadvantages. Pharmacol Ther (2014) 141:235–49. doi: 10.1016/j.pharmthera.2013.10.007
70. Spannbauer A, Traxler D, Zlabinger K, Gugerell A, Winkler J, Mester-Tonczar J, et al. Large Animal models of heart failure with reduced ejection fraction (HFrEF). Front Cardiovasc Med (2019) 6:117. doi: 10.3389/fcvm.2019.00117
71. Zalesak-Kravec S, Huang W, Wang P, Yu J, Liu T, Defnet AE, et al. Multi-omic analysis of non-human primate heart after partial-body radiation with minimal bone marrow sparing. Health Phys (2021) 121:352–71. doi: 10.1097/HP.0000000000001478
72. Medhora M, Gao F, Gasperetti T, Narayanan J, Khan AH, Jacobs ER, et al. Delayed effects of acute radiation exposure (Deare) in juvenile and old rats: Mitigation by lisinopril. Health Phys (2019) 116:529–45. doi: 10.1097/HP.0000000000000920
73. Schlaak RA, Senthilkumar G, Boerma M, Bergom C. Advances in preclinical research models of radiation-induced cardiac toxicity. Cancers (Basel) (2020) 12. doi: 10.3390/cancers12020415
74. Taunk NK, Haffty BG, Kostis JB, Goyal S. Radiation-induced heart disease: pathologic abnormalities and putative mechanisms. Front Oncol (2015) 5:39. doi: 10.3389/fonc.2015.00039
75. Gujral DM, Lloyd G, Bhattacharyya S. Radiation-induced valvular heart disease. Heart (2016) 102:269–76. doi: 10.1136/heartjnl-2015-308765
76. Tapio S. Pathology and biology of radiation-induced cardiac disease. J Radiat Res (2016) 57:439–48. doi: 10.1093/jrr/rrw064
77. Raghunathan D, Khilji MI, Hassan SA, Yusuf SW. Radiation-induced cardiovascular disease. Curr Atheroscler Rep (2017) 19:22. doi: 10.1007/s11883-017-0658-x
78. De Groot C, Beukema JC, Langendijk JA, van der Laan HP, Van Luijk P, Van Melle JP, et al. Radiation-induced myocardial fibrosis in long-term esophageal cancer survivors. Int J Radiat Oncol Biol Phys (2021) 110:1013–21. doi: 10.1016/j.ijrobp.2021.02.007
79. Fajardo LF, Stewart JR. Experimental radiation-induced heart disease. i. light microscopic studies. Am J Pathol (1970) 59:299–316.
80. Kong P, Christia P, Frangogiannis NG. The pathogenesis of cardiac fibrosis. Cell Mol Life Sci (2014) 71:549–74. doi: 10.1007/s00018-013-1349-6
81. Wang B, Wang H, Zhang M, Ji R, Wei J, Xin Y, et al. Radiation-induced myocardial fibrosis: Mechanisms underlying its pathogenesis and therapeutic strategies. J Cell Mol Med (2020) 24:7717–29. doi: 10.1111/jcmm.15479
82. Krüse JJ, Zurcher C, Strootman EG, Bart CI, Schlagwein N, Leer JW, et al. Structural changes in the auricles of the rat heart after local ionizing irradiation. Radiother Oncol (2001) 58:303–11. doi: 10.1016/S0167-8140(00)00327-3
83. Seemann I, Gabriels K, Visser NL, Hoving S, Te Poele JA, Pol JF, et al. Irradiation induced modest changes in murine cardiac function despite progressive structural damage to the myocardium and microvasculature. Radiother Oncol (2012) 103:143–50. doi: 10.1016/j.radonc.2011.10.011
84. Hinz B, Mastrangelo D, Iselin CE, Chaponnier C, Gabbiani G. Mechanical tension controls granulation tissue contractile activity and myofibroblast differentiation. Am J Pathol (2001) 159:1009–20. doi: 10.1016/S0002-9440(10)61776-2
85. Hinz B, Gabbiani G. Mechanisms of force generation and transmission by myofibroblasts. Curr Opin Biotechnol (2003) 14:538–46. doi: 10.1016/j.copbio.2003.08.006
86. Simms MG, Walley KR. Activated macrophages decrease rat cardiac myocyte contractility: importance of ICAM-1-dependent adhesion. Am J Physiol (1999) 277:H253–260. doi: 10.1152/ajpheart.1999.277.1.H253
87. Boyd JH, Kan B, Roberts H, Wang Y, Walley KR. S100A8 and S100A9 mediate endotoxin-induced cardiomyocyte dysfunction via the receptor for advanced glycation end products. Circ Res (2008) 102:1239–46. doi: 10.1161/CIRCRESAHA.107.167544
88. Slama MS, Le Guludec D, Sebag C, Leenhardt AR, Davy JM, Pellerin DE, et al. Complete atrioventricular block following mediastinal irradiation: a report of six cases. Pacing Clin Electrophysiol (1991) 14:1112–8. doi: 10.1111/j.1540-8159.1991.tb02842.x
89. Marks LB, Yu X, Prosnitz RG, Zhou SM, Hardenbergh PH, Blazing M, et al. The incidence and functional consequences of RT-associated cardiac perfusion defects. Int J Radiat Oncol Biol Phys (2005) 63:214–23. doi: 10.1016/j.ijrobp.2005.01.029
90. Venkatesulu BP, Mahadevan LS, Aliru ML, Yang X, Bodd MH, Singh PK, et al. Radiation-induced endothelial vascular injury: A review of possible mechanisms. JACC Basic Transl Sci (2018) 3:563–72. doi: 10.1016/j.jacbts.2018.01.014
91. Baselet B, Sonveaux P, Baatout S, Aerts A. Pathological effects of ionizing radiation: endothelial activation and dysfunction. Cell Mol Life Sci (2019) 76:699–728. doi: 10.1007/s00018-018-2956-z
92. Deanfield JE, Halcox JP, Rabelink TJ. Endothelial function and dysfunction: testing and clinical relevance. Circulation (2007) 115:1285–95. doi: 10.1161/CIRCULATIONAHA.106.652859
93. Guipaud O, Jaillet C, Clement-Colmou K, Francois A, Supiot S, Milliat F. The importance of the vascular endothelial barrier in the immune-inflammatory response induced by radiotherapy. Br J Radiol (2018) 91:20170762. doi: 10.1259/bjr.20170762
94. Krüse JJ, Bart CI, Visser A, Wondergem J. Changes in transforming growth factor-beta (TGF-beta 1), procollagen types I and II mRNA in the rat heart after irradiation. Int J Radiat Biol (1999) 75:1429–36. doi: 10.1080/095530099139296
95. Rosenkranz S, Flesch M, Amann K, Haeuseler C, Kilter H, Seeland U, et al. Alterations of beta-adrenergic signaling and cardiac hypertrophy in transgenic mice overexpressing TGF-beta(1). Am J Physiol Heart Circ Physiol (2002) 283:H1253–1262. doi: 10.1152/ajpheart.00578.2001
96. Khan R, Sheppard R. Fibrosis in heart disease: understanding the role of transforming growth factor-beta in cardiomyopathy, valvular disease and arrhythmia. Immunology (2006) 118:10–24. doi: 10.1111/j.1365-2567.2006.02336.x
97. Kubota T, Mctiernan CF, Frye CS, Demetris AJ, Feldman AM. Cardiac-specific overexpression of tumor necrosis factor-alpha causes lethal myocarditis in transgenic mice. J Card Fail (1997) 3:117–24. doi: 10.1016/S1071-9164(97)90045-2
98. Bryant D, Becker L, Richardson J, Shelton J, Franco F, Peshock R, et al. Cardiac failure in transgenic mice with myocardial expression of tumor necrosis factor-alpha. Circulation (1998) 97:1375–81. doi: 10.1161/01.CIR.97.14.1375
99. Li X, Moody MR, Engel D, Walker S, Clubb FJ Jr., Sivasubramanian N, et al. Cardiac-specific overexpression of tumor necrosis factor-alpha causes oxidative stress and contractile dysfunction in mouse diaphragm. Circulation (2000) 102:1690–6. doi: 10.1161/01.CIR.102.14.1690
100. Isoda K, Kamezawa Y, Tada N, Sato M, Ohsuzu F. Myocardial hypertrophy in transgenic mice overexpressing human interleukin 1alpha. J Card Fail (2001) 7:355–64. doi: 10.1054/jcaf.2001.28221
101. Mezzaroma E, Mikkelsen RB, Toldo S, Mauro AG, Sharma K, Marchetti C, et al. Role of interleukin-1 in radiation-induced cardiomyopathy. Mol Med (2015) 21:210–8. doi: 10.2119/molmed.2014.00243
102. Obana M, Maeda M, Takeda K, Hayama A, Mohri T, Yamashita T, et al. Therapeutic activation of signal transducer and activator of transcription 3 by interleukin-11 ameliorates cardiac fibrosis after myocardial infarction. Circulation (2010) 121:684–91. doi: 10.1161/CIRCULATIONAHA.109.893677
103. Schafer S, Viswanathan S, Widjaja AA, Lim WW, Moreno-Moral A, Delaughter DM, et al. IL-11 is a crucial determinant of cardiovascular fibrosis. Nature (2017) 552:110–5. doi: 10.1038/nature24676
104. Widjaja AA, Singh BK, Adami E, Viswanathan S, Dong J, D'agostino GA, et al. Inhibiting interleukin 11 signaling reduces hepatocyte death and liver fibrosis, inflammation, and steatosis in mouse models of nonalcoholic steatohepatitis. Gastroenterology (2019) 157:777–792.e714. doi: 10.1053/j.gastro.2019.05.002
105. Matsui Y, Sadoshima J. Rapid upregulation of CTGF in cardiac myocytes by hypertrophic stimuli: implication for cardiac fibrosis and hypertrophy. J Mol Cell Cardiol (2004) 37:477–81. doi: 10.1016/j.yjmcc.2004.05.012
106. Pontén A, Li X, Thorén P, Aase K, Sjöblom T, Ostman A, et al. Transgenic overexpression of platelet-derived growth factor-c in the mouse heart induces cardiac fibrosis, hypertrophy, and dilated cardiomyopathy. Am J Pathol (2003) 163:673–82. doi: 10.1016/S0002-9440(10)63694-2
107. Pontén A, Folestad EB, Pietras K, Eriksson U. Platelet-derived growth factor d induces cardiac fibrosis and proliferation of vascular smooth muscle cells in heart-specific transgenic mice. Circ Res (2005) 97:1036–45. doi: 10.1161/01.RES.0000190590.31545.d4
108. Gao M, Shirato H, Miyasaka K, Kuwabara M, Koyama T. Induction of growth factors in rat cardiac tissue by X irradiation. Radiat Res (2000) 153:540–7. doi: 10.1667/0033-7587(2000)153[0540:IOGFIR]2.0.CO;2
109. Frangogiannis NG. Cardiac fibrosis: Cell biological mechanisms, molecular pathways and therapeutic opportunities. Mol Aspects Med (2019) 65:70–99. doi: 10.1016/j.mam.2018.07.001
110. Madan R, Benson R, Sharma DN, Julka PK, Rath GK. Radiation induced heart disease: Pathogenesis, management and review literature. J Egypt Natl Canc Inst (2015) 27:187–93. doi: 10.1016/j.jnci.2015.07.005
111. Khalil H, Kanisicak O, Prasad V, Correll RN, Fu X, Schips T, et al. Fibroblast-specific TGF-β-Smad2/3 signaling underlies cardiac fibrosis. J Clin Invest (2017) 127:3770–83. doi: 10.1172/JCI94753
112. Czubryt MP. Cardiac fibroblast to myofibroblast phenotype conversion-an unexploited therapeutic target. J Cardiovasc Dev Dis (2019) 6. doi: 10.3390/jcdd6030028
113. Ejaz A, Greenberger JS, Rubin PJ. Understanding the mechanism of radiation induced fibrosis and therapy options. Pharmacol Ther (2019) 204:107399. doi: 10.1016/j.pharmthera.2019.107399
114. Ma C-X, Zhao X-K, Li Y-D. New therapeutic insights into radiation-induced myocardial fibrosis. Ther Adv Chronic Dis (2019) 10. doi: 10.1177/2040622319868383
115. Gourdie RG, Dimmeler S, Kohl P. Novel therapeutic strategies targeting fibroblasts and fibrosis in heart disease. Nat Rev Drug Discov (2016) 15:620–38. doi: 10.1038/nrd.2016.89
116. Kai H, Mori T, Tokuda K, Takayama N, Tahara N, Takemiya K, et al. Pressure overload-induced transient oxidative stress mediates perivascular inflammation and cardiac fibrosis through angiotensin II. Hypertens Res (2006) 29:711–8. doi: 10.1291/hypres.29.711
117. Sherman ML, Datta R, Hallahan DE, Weichselbaum RR, Kufe DW. Ionizing radiation regulates expression of the c-jun protooncogene. Proc Natl Acad Sci U.S.A. (1990) 87:5663–6. doi: 10.1073/pnas.87.15.5663
118. Bhattacharya S, Asaithamby A. Ionizing radiation and heart risks. Semin Cell Dev Biol (2016) 58:14–25. doi: 10.1016/j.semcdb.2016.01.045
119. Firsanov D, Vasilishina A, Kropotov A, Mikhailov V. Dynamics of gammaH2AX formation and elimination in mammalian cells after X-irradiation. Biochimie (2012) 94:2416–22. doi: 10.1016/j.biochi.2012.06.019
120. Sarosiek KA, Chi X, Bachman JA, Sims JJ, Montero J, Patel L, et al. BID preferentially activates BAK while BIM preferentially activates BAX, affecting chemotherapy response. Mol Cell (2013) 51:751–65. doi: 10.1016/j.molcel.2013.08.048
121. Salata C, Ferreira-Machado SC, De Andrade CB, Mencalha AL, Mandarim-De-Lacerda CA, De Almeida CE. Apoptosis induction of cardiomyocytes and subsequent fibrosis after irradiation and neoadjuvant chemotherapy. Int J Radiat Biol (2014) 90:284–90. doi: 10.3109/09553002.2014.887869
122. Huang S, Zhao Q, Yang ZG, Diao KY, He Y, Shi K, et al. Protective role of beta-blockers in chemotherapy-induced cardiotoxicity-a systematic review and meta-analysis of carvedilol. Heart Fail Rev (2019) 24:325–33. doi: 10.1007/s10741-018-9755-3
123. Abdel-Qadir H, Bobrowski D, Zhou L, Austin PC, Calvillo-Argüelles O, Amir E, et al. Statin exposure and risk of heart failure after anthracycline- or trastuzumab-based chemotherapy for early breast cancer: A propensity Score–Matched cohort study. J Am Heart Assoc (2021) 10:e018393. doi: 10.1161/JAHA.119.018393
124. Zamorano JL, Lancellotti P, Rodriguez Munoz D, Aboyans V, Asteggiano R, Galderisi M, et al. 2016 ESC position paper on cancer treatments and cardiovascular toxicity developed under the auspices of the ESC committee for practice guidelines: The task force for cancer treatments and cardiovascular toxicity of the European society of cardiology (ESC). Eur J Heart Fail (2017) 19:9–42. doi: 10.1002/ejhf.654
125. Demissei BG, Freedman G, Feigenberg SJ, Plastaras JP, Maity A, Smith AM, et al. Early changes in cardiovascular biomarkers with contemporary thoracic radiation therapy for breast cancer, lung cancer, and lymphoma. Int J Radiat Oncol Biol Phys (2019) 103:851–60. doi: 10.1016/j.ijrobp.2018.11.013
126. Negishi K, Negishi T, Hare JL, Haluska BA, Plana JC, Marwick TH. Independent and incremental value of deformation indices for prediction of trastuzumab-induced cardiotoxicity. J Am Soc Echocardiogr (2013) 26:493–8. doi: 10.1016/j.echo.2013.02.008
127. Thavendiranathan P, Poulin F, Lim KD, Plana JC, Woo A, Marwick TH. Use of myocardial strain imaging by echocardiography for the early detection of cardiotoxicity in patients during and after cancer chemotherapy: a systematic review. J Am Coll Cardiol (2014) 63:2751–68. doi: 10.1016/j.jacc.2014.01.073
128. Charbonnel C, Convers-Domart R, Rigaudeau S, Taksin AL, Baron N, Lambert J, et al. Assessment of global longitudinal strain at low-dose anthracycline-based chemotherapy, for the prediction of subsequent cardiotoxicity. Eur Heart J Cardiovasc Imaging (2017) 18:392–401. doi: 10.1093/ehjci/jew223
129. Negishi T, Thavendiranathan P, Negishi K, Marwick TH, Investigators S. Rationale and design of the strain surveillance of chemotherapy for improving cardiovascular outcomes: The SUCCOUR trial. JACC Cardiovasc Imaging (2018) 11:1098–105. doi: 10.1016/j.jcmg.2018.03.019
130. Zhu D, Li T, Zhuang H, Cui M. Early detection of cardiac damage by two-dimensional speckle tracking echocardiography after thoracic radiation therapy: Study protocol for a prospective cohort study. Front Cardiovasc Med (2021) 8:735265. doi: 10.3389/fcvm.2021.735265
131. Drafts BC, Twomley KM, D'agostino R Jr., Lawrence J, Avis N, Ellis LR, et al. Low to moderate dose anthracycline-based chemotherapy is associated with early noninvasive imaging evidence of subclinical cardiovascular disease. JACC Cardiovasc Imaging (2013) 6:877–85. doi: 10.1016/j.jcmg.2012.11.017
132. Nakano S, Takahashi M, Kimura F, Senoo T, Saeki T, Ueda S, et al. Cardiac magnetic resonance imaging-based myocardial strain study for evaluation of cardiotoxicity in breast cancer patients treated with trastuzumab: A pilot study to evaluate the feasibility of the method. Cardiol J (2016) 23:270–80. doi: 10.5603/CJ.a2016.0023
133. Haslbauer JD, Lindner S, Valbuena-Lopez S, Zainal H, Zhou H, D'angelo T, et al. CMR imaging biosignature of cardiac involvement due to cancer-related treatment by T1 and T2 mapping. Int J Cardiol (2019) 275:179–86. doi: 10.1016/j.ijcard.2018.10.023
134. Neilan TG, Coelho-Filho OR, Pena-Herrera D, Shah RV, Jerosch-Herold M, Francis SA, et al. Left ventricular mass in patients with a cardiomyopathy after treatment with anthracyclines. Am J Cardiol (2012) 110:1679–86. doi: 10.1016/j.amjcard.2012.07.040
135. Ylanen K, Poutanen T, Savikurki-Heikkila P, Rinta-Kiikka I, Eerola A, Vettenranta K. Cardiac magnetic resonance imaging in the evaluation of the late effects of anthracyclines among long-term survivors of childhood cancer. J Am Coll Cardiol (2013) 61:1539–47. doi: 10.1016/j.jacc.2013.01.019
136. Seddon B, Cook A, Gothard L, Salmon E, Latus K, Underwood SR, et al. Detection of defects in myocardial perfusion imaging in patients with early breast cancer treated with radiotherapy. Radiother Oncol (2002) 64:53–63. doi: 10.1016/S0167-8140(02)00133-0
137. Prosnitz RG, Hubbs JL, Evans ES, Zhou SM, Yu X, Blazing MA, et al. Prospective assessment of radiotherapy-associated cardiac toxicity in breast cancer patients: analysis of data 3 to 6 years after treatment. Cancer (2007) 110:1840–50. doi: 10.1002/cncr.22965
138. Correa CR, Das IJ, Litt HI, Ferrari V, Hwang WT, Solin LJ, et al. Association between tangential beam treatment parameters and cardiac abnormalities after definitive radiation treatment for left-sided breast cancer. Int J Radiat Oncol Biol Phys (2008) 72:508–16. doi: 10.1016/j.ijrobp.2007.12.037
139. Zellars R, Bravo PE, Tryggestad E, Hopfer K, Myers L, Tahari A, et al. SPECT analysis of cardiac perfusion changes after whole-breast/chest wall radiation therapy with or without active breathing coordinator: results of a randomized phase 3 trial. Int J Radiat Oncol Biol Phys (2014) 88:778–85. doi: 10.1016/j.ijrobp.2013.12.035
140. Kaidar-Person O, Zagar TM, Oldan JD, Matney J, Jones EL, Das S, et al. Early cardiac perfusion defects after left-sided radiation therapy for breast cancer: is there a volume response? Breast Cancer Res Treat (2017) 164:253–62. doi: 10.1007/s10549-017-4248-y
141. Kajander S, Joutsiniemi E, Saraste M, Pietilä M, Ukkonen H, Saraste A, et al. Cardiac positron emission tomography/computed tomography imaging accurately detects anatomically and functionally significant coronary artery disease. Circulation (2010) 122:603–13. doi: 10.1161/CIRCULATIONAHA.109.915009
142. Balogh V, Macaskill MG, Hadoke PWF, Gray GA. Positron emission tomography techniques to measure active inflammation, fibrosis and angiogenesis: Potential for non-invasive imaging of hypertensive heart failure. Front Cardiovasc Med (2021) 8:719031. doi: 10.3389/fcvm.2021.719031
143. Mayer M, Borja AJ, Hancin EC, Auslander T, Revheim ME, Moghbel MC, et al. Imaging atherosclerosis by PET, with emphasis on the role of FDG and NaF as potential biomarkers for this disorder. Front Physiol (2020) 11:511391. doi: 10.3389/fphys.2020.511391
144. Ravikanth R. Role of (18)F-FDG positron emission tomography in carotid atherosclerotic plaque imaging: A systematic review. World J Nucl Med (2020) 19:327–35. doi: 10.4103/wjnm.WJNM_26_20
145. Juneau D, Erthal F, Ohira H, Mc Ardle B, Hessian R, Dekemp RA, et al. Clinical PET myocardial perfusion imaging and flow quantification. Cardiol Clin (2016) 34:69–85. doi: 10.1016/j.ccl.2015.07.013
146. Gabrielson KL, Mok GS, Nimmagadda S, Bedja D, Pin S, Tsao A, et al. Detection of dose response in chronic doxorubicin-mediated cell death with cardiac technetium 99m annexin V single-photon emission computed tomography. Mol Imaging (2008) 7:132–8. doi: 10.2310/7290.2008.00015
147. Hawkins PG, Sun Y, Dess RT, Jackson WC, Sun G, Bi N, et al. Circulating microRNAs as biomarkers of radiation-induced cardiac toxicity in non-small-cell lung cancer. J Cancer Res Clin Oncol (2019) 145:1635–43. doi: 10.1007/s00432-019-02903-5
148. Palumbo I, Palumbo B, Fravolini ML, Marcantonini M, Perrucci E, Latini ME, et al. Brain natriuretic peptide as a cardiac marker of transient radiotherapy-related damage in left-sided breast cancer patients: A prospective study. Breast (2016) 25:45–50. doi: 10.1016/j.breast.2015.10.004
149. Serrano NA, Mikkelsen R, Canada J, Mezzaroma E, Weiss E, Abbate A. Biomarkers of cardiac injury in patients undergoing thoracic radiation therapy. Int J Cardiol (2016) 223:507–9. doi: 10.1016/j.ijcard.2016.08.263
150. Gomez DR, Yusuf SW, Munsell MF, Welsh JW, Liao Z, Lin SH, et al. Prospective exploratory analysis of cardiac biomarkers and electrocardiogram abnormalities in patients receiving thoracic radiation therapy with high-dose heart exposure. J Thorac Oncol (2014) 9:1554–60. doi: 10.1097/JTO.0000000000000306
151. D'errico MP, Grimaldi L, Petruzzelli MF, Gianicolo EA, Tramacere F, Monetti A, et al. N-terminal pro-b-type natriuretic peptide plasma levels as a potential biomarker for cardiac damage after radiotherapy in patients with left-sided breast cancer. Int J Radiat Oncol Biol Phys (2012) 82:e239–246. doi: 10.1016/j.ijrobp.2011.03.058
152. Nellessen U, Zingel M, Hecker H, Bahnsen J, Borschke D. Effects of radiation therapy on myocardial cell integrity and pump function: which role for cardiac biomarkers? Chemotherapy (2010) 56:147–52. doi: 10.1159/000313528
153. Kozak KR, Hong TS, Sluss PM, Lewandrowski EL, Aleryani SL, Macdonald SM, et al. Cardiac blood biomarkers in patients receiving thoracic (chemo)radiation. Lung Cancer (2008) 62:351–5. doi: 10.1016/j.lungcan.2008.03.024
154. Perik PJ, De Vries EG, Boomsma F, van der Graaf WT, Sleijfer DT, Van Veldhuisen DJ, et al. Use of natriuretic peptides for detecting cardiac dysfunction in long-term disease-free breast cancer survivors. Anticancer Res (2005) 25:3651–7.
155. Ky B, Putt M, Sawaya H, French B, Januzzi JL Jr., Sebag IA, et al. Early increases in multiple biomarkers predict subsequent cardiotoxicity in patients with breast cancer treated with doxorubicin, taxanes, and trastuzumab. J Am Coll Cardiol (2014) 63:809–16. doi: 10.1016/j.jacc.2013.10.061
156. Onitilo AA, Engel JM, Stankowski RV, Liang H, Berg RL, Doi SA. High-sensitivity c-reactive protein (hs-CRP) as a biomarker for trastuzumab-induced cardiotoxicity in HER2-positive early-stage breast cancer: a pilot study. Breast Cancer Res Treat (2012) 134:291–8. doi: 10.1007/s10549-012-2039-z
157. Elghandour AH, El Sorady M, Azab S, Elrahman M. Human heart-type fatty acid-binding protein as an early diagnostic marker of doxorubicin cardiac toxicity. Hematol Rev (2009) 1:e6. doi: 10.4081/hr.2009.e6
158. Troughton R, Michael Felker G. Natriuretic peptide-guided heart failure management. Eur Heart J (2014) 35:16–24. doi: 10.1093/eurheartj/eht463
159. Chow SL, Maisel AS, Anand I, Bozkurt B, De Boer RA, Felker GM, et al. Role of biomarkers for the prevention, assessment, and management of heart failure: A scientific statement from the American heart association. Circulation (2017) 135:e1054–91. doi: 10.1161/CIR.0000000000000490
160. Michel L, Mincu RI, Mahabadi AA, Settelmeier S, Al-Rashid F, Rassaf T, et al. Troponins and brain natriuretic peptides for the prediction of cardiotoxicity in cancer patients: a meta-analysis. Eur J Heart Fail (2020) 22:350–61. doi: 10.1002/ejhf.1631
161. Ruger AM, Schneeweiss A, Seiler S, Tesch H, Van Mackelenbergh M, Marme F, et al. Cardiotoxicity and cardiovascular biomarkers in patients with breast cancer: Data from the GeparOcto-GBG 84 trial. J Am Heart Assoc (2020) 9:e018143. doi: 10.1161/JAHA.120.018143
162. Kuo AH, Ancukiewicz M, Kozak KR, Yock TI, Padera TP. Cardiac and inflammatory biomarkers do not correlate with volume of heart or lung receiving radiation. Radiat Oncol (2015) 10:5. doi: 10.1186/s13014-014-0324-1
163. Horacek JM, Jebavy L, Ulrychova M, Tichy M, Pudil R, Zak P, et al. Glycogen phosphorylase BB could be a new biomarker for detection of cardiac toxicity during hematopoietic cell transplantation for hematological malignancies. Bone Marrow Transplant (2010) 45:1123–4. doi: 10.1038/bmt.2009.306
164. Wang J, Liew OW, Richards AM, Chen YT. Overview of MicroRNAs in cardiac hypertrophy, fibrosis, and apoptosis. Int J Mol Sci (2016) 17. doi: 10.3390/ijms18010001
165. Wehbe N, Nasser SA, Pintus G, Badran A, Eid AH, Baydoun E. MicroRNAs in cardiac hypertrophy. Int J Mol Sci (2019) 20. doi: 10.3390/ijms20194714
166. Glinge C, Lahrouchi N, Jabbari R, Tfelt-Hansen J, Bezzina CR. Genome-wide association studies of cardiac electrical phenotypes. Cardiovasc Res (2020) 116:1620–34. doi: 10.1093/cvr/cvaa144
167. Scholman KT, Meijborg VMF, Galvez-Monton C, Lodder EM, Boukens BJ. From genome-wide association studies to cardiac electrophysiology: Through the maze of biological complexity. Front Physiol (2020) 11:557. doi: 10.3389/fphys.2020.00557
168. Kiscsatari L, Sarkozy M, Kovari B, Varga Z, Gomori K, Morvay N, et al. High-dose radiation induced heart damage in a rat model. In Vivo (2016) 30:623–31.
169. Nicolas JD, Khan A, Markus A, Mohamed BA, Toischer K, Alves F, et al. X-Ray diffraction and second harmonic imaging reveal new insights into structural alterations caused by pressure-overload in murine hearts. Sci Rep (2020) 10:19317. doi: 10.1038/s41598-020-76163-6
170. Collier P, Watson CJ, Van Es MH, Phelan D, Mcgorrian C, Tolan M, et al. Getting to the heart of cardiac remodeling; how collagen subtypes may contribute to phenotype. J Mol Cell Cardiol (2012) 52:148–53. doi: 10.1016/j.yjmcc.2011.10.002
171. Moses L, Pachter L. Museum of spatial transcriptomics. Nat Methods (2022) 19:534–46. doi: 10.1038/s41592-022-01409-2
172. Mezzaroma E, Di X, Graves P, Toldo S, Van Tassell BW, Kannan H, et al. A mouse model of radiation-induced cardiomyopathy. Int J Cardiol (2012) 156:231–3. doi: 10.1016/j.ijcard.2012.01.038
173. Ibrahim EH, Baruah D, Croisille P, Stojanovska J, Rubenstein JC, Frei A, et al. Cardiac magnetic resonance for early detection of radiation therapy-induced cardiotoxicity in a small animal model. JACC CardioOncol (2021) 3:113–30. doi: 10.1016/j.jaccao.2020.12.006
174. Roelants V, Delgaudine M, Walrand S, Lhommel R, Beguin Y, Jamar F, et al. Myocardial infarct size quantification in mice by SPECT using a novel algorithm independent of a normal perfusion database. EJNMMI Res (2012) 2:64. doi: 10.1186/2191-219X-2-64
175. Antonia SJ, Villegas A, Daniel D, Vicente D, Murakami S, Hui R, et al. Overall survival with durvalumab after chemoradiotherapy in stage III NSCLC. N Engl J Med (2018) 379:2342–50. doi: 10.1056/NEJMoa1809697
176. Kasmann L, Taugner J, Manapov F. Chemo-/immuno-/radiotherapy combination in treatment of solid cancer. Oncotarget (2019) 10:5387–8. doi: 10.18632/oncotarget.27141
177. Faivre-Finn C, Vicente D, Kurata T, Planchard D, Paz-Ares L, Vansteenkiste JF, et al. Four-year survival with durvalumab after chemoradiotherapy in stage III NSCLC-an update from the PACIFIC trial. J Thorac Oncol (2021) 16:860–7. doi: 10.1016/j.jtho.2020.12.015
178. Uy KL, Darling G, Xu W, Yi QL, De Perrot M, Pierre AF, et al. Improved results of induction chemoradiation before surgical intervention for selected patients with stage IIIA-N2 non-small cell lung cancer. J Thorac Cardiovasc Surg (2007) 134:188–93. doi: 10.1016/j.jtcvs.2007.01.078
179. Lv J, Cao XF, Zhu B, Ji L, Tao L, Wang DD. Effect of neoadjuvant chemoradiotherapy on prognosis and surgery for esophageal carcinoma. World J Gastroenterol (2009) 15:4962–8. doi: 10.3748/wjg.15.4962
180. O'rourke N, Roque IFM, Farre Bernado N, Macbeth F. Concurrent chemoradiotherapy in non-small cell lung cancer. Cochrane Database Syst Rev (2010) 15, CD002140. doi: 10.1002/14651858.CD002140.pub3
181. Stinchcombe TE, Gore EM. Limited-stage small cell lung cancer: current chemoradiotherapy treatment paradigms. Oncologist (2010) 15:187–95. doi: 10.1634/theoncologist.2009-0298
182. Mandilaras V, Bouganim N, Spayne J, Dent R, Arnaout A, Boileau JF, et al. Concurrent chemoradiotherapy for locally advanced breast cancer-time for a new paradigm? Curr Oncol (2015) 22:25–32. doi: 10.3747/co.21.2043
183. Reboul FL. Radiotherapy and chemotherapy in locally advanced non-small cell lung cancer: preclinical and early clinical data. Hematol Oncol Clin North Am (2004) 18:41–53. doi: 10.1016/S0889-8588(03)00138-2
184. Elbanna M, Chowdhury NN, Rhome R, Fishel ML. Clinical and preclinical outcomes of combining targeted therapy with radiotherapy. Front Oncol (2021) 11:749496. doi: 10.3389/fonc.2021.749496
185. Stinchcombe TE, Morris DE, Lee CB, Moore DT, Hayes DN, Halle JS, et al. Induction chemotherapy with carboplatin, irinotecan, and paclitaxel followed by high dose three-dimension conformal thoracic radiotherapy (74 gy) with concurrent carboplatin, paclitaxel, and gefitinib in unresectable stage IIIA and stage IIIB non-small cell lung cancer. J Thorac Oncol (2008) 3:250–7. doi: 10.1097/JTO.0b013e3181653cf4
186. Lee CB, Stinchcombe TE, Moore DT, Morris DE, Hayes DN, Halle J, et al. Late complications of high-dose (>/=66 gy) thoracic conformal radiation therapy in combined modality trials in unresectable stage III non-small cell lung cancer. J Thorac Oncol (2009) 4:74–9. doi: 10.1097/JTO.0b013e3181915028
187. Hardy D, Liu CC, Cormier JN, Xia R, Du XL. Cardiac toxicity in association with chemotherapy and radiation therapy in a large cohort of older patients with non-small-cell lung cancer. Ann Oncol (2010) 21:1825–33. doi: 10.1093/annonc/mdq042
188. Hicks GL Jr. Coronary artery operation in radiation-associated atherosclerosis: long-term follow-up. Ann Thorac Surg (1992) 53:670–4. doi: 10.1016/0003-4975(92)90331-W
189. Schulman HE, Korr KS, Myers TJ. Left internal thoracic artery graft occlusion following mediastinal radiation therapy. Chest (1994) 105:1881–2. doi: 10.1378/chest.105.6.1881
190. Darby SC, Mcgale P, Taylor CW, Peto R. Long-term mortality from heart disease and lung cancer after radiotherapy for early breast cancer: prospective cohort study of about 300,000 women in US SEER cancer registries. Lancet Oncol (2005) 6:557–65. doi: 10.1016/S1470-2045(05)70251-5
191. Atkins KM, Rawal B, Chaunzwa TL, Lamba N, Bitterman DS, Williams CL, et al. Cardiac radiation dose, cardiac disease, and mortality in patients with lung cancer. J Am Coll Cardiol (2019) 73:2976–87. doi: 10.1016/j.jacc.2019.03.500
192. Hayashi Y, Iijima H, Isohashi F, Tsujii Y, Fujinaga T, Nagai K, et al. The heart's exposure to radiation increases the risk of cardiac toxicity after chemoradiotherapy for superficial esophageal cancer: a retrospective cohort study. BMC Cancer (2019) 19:195. doi: 10.1186/s12885-019-5421-y
193. Burke AM, Yeh C, Kim S, Bergquist P, Krishnan P, Barac A, et al. A prospective study of early radiation associated cardiac toxicity following neoadjuvant chemoradiation for distal esophageal cancer. Front Oncol (2020) 10: 1169. doi: 10.3389/fonc.2020.01169
194. Aleman BM, Van Den Belt-Dusebout AW, De Bruin ML, Van 'T Veer MB, Baaijens MH, De Boer JP, et al. Late cardiotoxicity after treatment for Hodgkin lymphoma. Blood (2007) 109:1878–86. doi: 10.1182/blood-2006-07-034405
195. Van Nimwegen FA, Schaapveld M, Janus CP, Krol AD, Petersen EJ, Raemaekers JM, et al. Cardiovascular disease after Hodgkin lymphoma treatment: 40-year disease risk. JAMA Intern Med (2015) 175:1007–17. doi: 10.1001/jamainternmed.2015.1180
196. Wei X, Liu HH, Tucker SL, Wang S, Mohan R, Cox JD, et al. Risk factors for pericardial effusion in inoperable esophageal cancer patients treated with definitive chemoradiation therapy. Int J Radiat Oncol Biol Phys (2008) 70:707–14. doi: 10.1016/j.ijrobp.2007.10.056
197. Brahmer J, Reckamp KL, Baas P, Crino L, Eberhardt WE, Poddubskaya E, et al. Nivolumab versus docetaxel in advanced squamous-cell non-Small-Cell lung cancer. N Engl J Med (2015) 373:123–35. doi: 10.1056/NEJMoa1504627
198. Antonia SJ, Villegas A, Daniel D, Vicente D, Murakami S, Hui R, et al. Durvalumab after chemoradiotherapy in stage III non-Small-Cell lung cancer. N Engl J Med (2017) 377:1919–29. doi: 10.1056/NEJMoa1709937
199. Jiang W, Chan CK, Weissman IL, Kim BYS, Hahn SM. Immune priming of the tumor microenvironment by radiation. Trends Cancer (2016) 2:638–45. doi: 10.1016/j.trecan.2016.09.007
200. Frey B, Ruckert M, Deloch L, Ruhle PF, Derer A, Fietkau R, et al. Immunomodulation by ionizing radiation-impact for design of radio-immunotherapies and for treatment of inflammatory diseases. Immunol Rev (2017) 280:231–48. doi: 10.1111/imr.12572
201. Son CH, Fleming GF, Moroney JW. Potential role of radiation therapy in augmenting the activity of immunotherapy for gynecologic cancers. Cancer Manag Res (2017) 9:553–63. doi: 10.2147/CMAR.S116683
202. Twyman-Saint Victor C, Rech AJ, Maity A, Rengan R, Pauken KE, Stelekati E, et al. Radiation and dual checkpoint blockade activate non-redundant immune mechanisms in cancer. Nature (2015) 520:373–7. doi: 10.1038/nature14292
203. Shaverdian N, Lisberg AE, Bornazyan K, Veruttipong D, Goldman JW, Formenti SC, et al. Previous radiotherapy and the clinical activity and toxicity of pembrolizumab in the treatment of non-small-cell lung cancer: a secondary analysis of the KEYNOTE-001 phase 1 trial. Lancet Oncol (2017) 18:895–903. doi: 10.1016/S1470-2045(17)30380-7
204. Dewan MZ, Galloway AE, Kawashima N, Dewyngaert JK, Babb JS, Formenti SC, et al. Fractionated but not single-dose radiotherapy induces an immune-mediated abscopal effect when combined with anti-CTLA-4 antibody. Clin Cancer Res (2009) 15:5379–88. doi: 10.1158/1078-0432.CCR-09-0265
205. Deng L, Liang H, Burnette B, Beckett M, Darga T, Weichselbaum RR, et al. Irradiation and anti-PD-L1 treatment synergistically promote antitumor immunity in mice. J Clin Invest (2014) 124:687–95. doi: 10.1172/JCI67313
206. Dovedi SJ, Adlard AL, Lipowska-Bhalla G, Mckenna C, Jones S, Cheadle EJ, et al. Acquired resistance to fractionated radiotherapy can be overcome by concurrent PD-L1 blockade. Cancer Res (2014) 74:5458–68. doi: 10.1158/0008-5472.CAN-14-1258
207. Xu S, Sharma UC, Tuttle C, Pokharel S. Immune checkpoint inhibitors: Cardiotoxicity in pre-clinical models and clinical studies. Front Cardiovasc Med (2021) 8:619650. doi: 10.3389/fcvm.2021.619650
208. Du S, Zhou L, Alexander GS, Park K, Yang L, Wang N, et al. PD-1 modulates radiation-induced cardiac toxicity through cytotoxic T lymphocytes. J Thorac Oncol (2018) 13:510–20. doi: 10.1016/j.jtho.2017.12.002
209. Myers CJ, Lu B. Decreased survival after combining thoracic irradiation and an anti-PD-1 antibody correlated with increased T-cell infiltration into cardiac and lung tissues. Int J Radiat Oncol Biol Phys (2017) 99:1129–36. doi: 10.1016/j.ijrobp.2017.06.2452
210. Belkacemi Y, Gligorov J, Ozsahin M, Marsiglia H, De Lafontan B, Laharie-Mineur H, et al. Concurrent trastuzumab with adjuvant radiotherapy in HER2-positive breast cancer patients: acute toxicity analyses from the French multicentric study. Ann Oncol (2008) 19:1110–6. doi: 10.1093/annonc/mdn029
211. Halyard MY, Pisansky TM, Dueck AC, Suman V, Pierce L, Solin L, et al. Radiotherapy and adjuvant trastuzumab in operable breast cancer: tolerability and adverse event data from the NCCTG phase III trial N9831. J Clin Oncol (2009) 27:2638–44. doi: 10.1200/JCO.2008.17.9549
212. Shaffer R, Tyldesley S, Rolles M, Chia S, Mohamed I. Acute cardiotoxicity with concurrent trastuzumab and radiotherapy including internal mammary chain nodes: a retrospective single-institution study. Radiother Oncol (2009) 90:122–6. doi: 10.1016/j.radonc.2008.09.003
213. Cao L, Cai G, Chang C, Yang ZZ, Feng Y, Yu XL, et al. Early cardiac toxicity following adjuvant radiotherapy of left-sided breast cancer with or without concurrent trastuzumab. Oncotarget (2016) 7:1042–54. doi: 10.18632/oncotarget.6053
214. Lind JS, Senan S, Smit EF. Pulmonary toxicity after bevacizumab and concurrent thoracic radiotherapy observed in a phase I study for inoperable stage III non-small-cell lung cancer. J Clin Oncol (2012) 30:e104–108. doi: 10.1200/JCO.2011.38.4552
Keywords: radiation therapy, radiation-induced heart disease (RIHD), cardiovascular toxicity, biomarkers, radiation-induced myocardial fibrosis, preclinical model, chemoradiotherapy, immunoradiotherapy
Citation: Dreyfuss AD, Velalopoulou A, Avgousti H, Bell BI and Verginadis II (2022) Preclinical models of radiation-induced cardiac toxicity: Potential mechanisms and biomarkers. Front. Oncol. 12:920867. doi: 10.3389/fonc.2022.920867
Received: 15 April 2022; Accepted: 12 September 2022;
Published: 12 October 2022.
Edited by:
Henry Soo-Min Park, Yale University, United StatesReviewed by:
Arya Amini, City of Hope National Medical Center, United StatesSerena Monti, National Research Council (CNR), Italy
Copyright © 2022 Dreyfuss, Velalopoulou, Avgousti, Bell and Verginadis. This is an open-access article distributed under the terms of the Creative Commons Attribution License (CC BY). The use, distribution or reproduction in other forums is permitted, provided the original author(s) and the copyright owner(s) are credited and that the original publication in this journal is cited, in accordance with accepted academic practice. No use, distribution or reproduction is permitted which does not comply with these terms.
*Correspondence: Ioannis I. Verginadis, dmlvYW5uaXNAcGVubm1lZGljaW5lLnVwZW5uLmVkdQ==