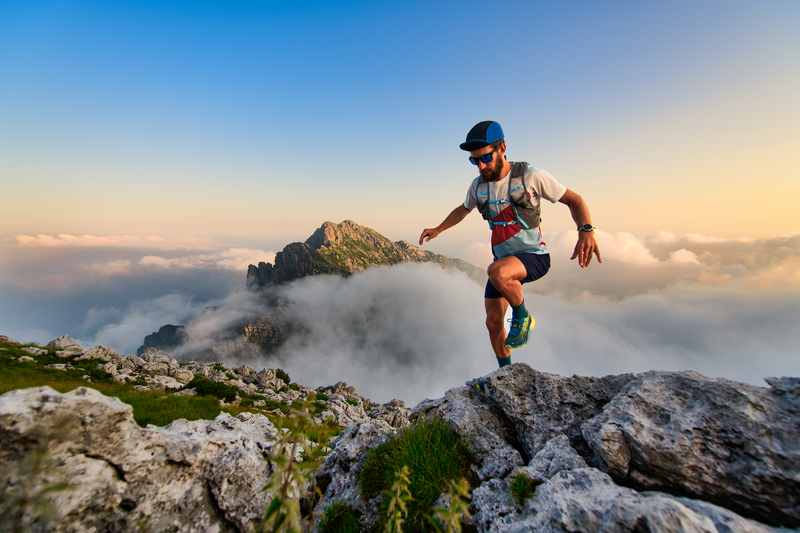
94% of researchers rate our articles as excellent or good
Learn more about the work of our research integrity team to safeguard the quality of each article we publish.
Find out more
ORIGINAL RESEARCH article
Front. Oncol. , 30 August 2022
Sec. Molecular and Cellular Oncology
Volume 12 - 2022 | https://doi.org/10.3389/fonc.2022.897207
This article is part of the Research Topic Mitochondria in Tumorigenesis, Cancer Progression and Metastasis View all 6 articles
Remodeling of mitochondrial energy metabolism is essential for the survival of tumor cells in limited nutrient availability and hypoxic conditions. Defects in oxidative phosphorylation (OXPHOS) and mitochondrial biogenesis also cause a switch in energy metabolism from oxidative to aerobic glycolysis contributing to the tumor heterogeneity in cancer. Specifically, the aberrant expressions of mitochondrial translation components such as ribosomal proteins (MRPs) and translation factors have been increasingly associated with many different cancers including breast cancer. The mitochondrial translation is responsible for the synthesis 13 of mitochondrial-encoded OXPHOS subunits of complexes. In this study, we investigated the contribution of mitochondrial translation in the remodeling of oxidative energy metabolism through altered expression of OXPHOS subunits in 26 ER/PR(+) breast tumors. We observed a significant correlation between the changes in the expression of mitochondrial translation-related proteins and OXPHOS subunits in the majority of the ER/PR(+) breast tumors and breast cancer cell lines. The reduced expression of OXPHOS and mitochondrial translation components also correlated well with the changes in epithelial-mesenchymal transition (EMT) markers, E-cadherin (CHD1), and vimentin (VIM) in the ER/PR(+) tumor biopsies. Data mining analysis of the Clinical Proteomic Tumor Analysis Consortium (CPTAC) breast cancer proteome further supported the correlation between the reduced OXPHOS subunit expression and increased EMT and metastatic marker expression in the majority of the ER/PR(+) tumors. Therefore, understanding the role of MRPs in the remodeling of energy metabolism will be essential in the characterization of heterogeneity at the molecular level and serve as diagnostic and prognostic markers in breast cancer.
Breast cancer is one of the common causes of cancer-related deaths in the world due to high rates of invasiveness, metastasis, and recurrence. Since the recognition of energy metabolism as one of the major hallmarks of cancer, many studies revealed the contribution of oxidative phosphorylation (OXPHOS) and mitochondrial metabolites to the heterogeneity of tumor cell metabolism in cancer (1–5). OXPHOS complexes, I-V, consists of mitochondrial and nuclear-encoded subunits localized to the inner mitochondrial membrane. Mitochondrial genome encodes for the 13 mRNAs, two rRNAs (12S and 16S), and 22 tRNAs to support OXPHOS in humans. These 13 mitochondrial (mt)-encoded essential subunits form the integral core of the OXPHOS complexes I, III, IV, and V (ATP synthase). mtDNA mutations, low copy numbers, and reduced mitochondrial transcript levels are associated with increased metastasis and poor prognosis in breast cancer (6–10). A subset of genetic variations in complex I and IV genes, MT-ND2 and MT-COII, has recently been related to breast cancer risk of European Americans in 2,723 breast cancer cases and 3,260 controls from a Multiethnic Cohort Study (11).
In addition to the changes in mt-encoded genes affecting OXPHOS capacity, nuclear-encoded factors supporting biogenesis of OXPHOS subunits such as mitochondrial transcription and translation machineries are also implicated in remodeling energy metabolism (12–17). Mitochondrial translation machinery is composed of 55S ribosomes and translation factors and its components are completely different from their cytosolic counterparts (18–24). In the initial proteomics studies of 55S ribosomes, we identified over 80 mitochondrial ribosomal proteins (MRPs) which are solely responsible for the synthesis of 13 essential OXPHOS subunits (20, 21, 25–27). Some MRPs have acquired additional roles in health and various diseases (28–31). For example, two previously identified pro-apoptotic MRPs, DAP3 and PDCD9 (also known as MRPS29 and MRPS30), are the bona fide components of the mitochondrial ribosome involved in apoptosis (32–38).
Altered expression of MRP genes is mostly detected in various carcinomas, and their possible roles in mitochondrial translation have been elegantly and systematically reviewed in several publications (30, 31, 39). Studies from multiple laboratories have shown the remodeling of the tumor metabolism by changes in expression of MRP transcripts while driving heterogeneity, invasive capabilities, and recurrence in breast cancer (40–47). In agreement with these reports, reduced DAP3 expression levels have been associated with local recurrence, distant metastasis, and mortality in breast tumors (48–50). A single nucleotide polymorphism (SNP) in chromosome 5p12 breast cancer susceptibility locus affects MRPS30 expression in estrogen receptor positive (ER(+)) tumors (43, 51–54). Long noncoding (LncRNA) MRPS30-divergent transcript (DT) is overexpressed in breast carcinoma and its knockdown significantly reduced cell proliferation and invasion in breast cancer cell lines (55). In two independent studies, overexpression of the MRPL13 gene is proposed as a possible prognostic biomarker and correlated with unfavorable clinical outcomes of breast cancer patients (56, 57). Similarly, the overexpression of the MRPS6 and MRPS23 genes affected tumorigenic cellular processes in breast cancer and their knockdown decreased proliferation, expression of select mesenchymal marker and increased expression of tumor suppressor genes (58–60). On the other hand, the reduced stability of MRPS23 by its methylation at arginine R21 has been shown to promote breast cancer metastasis through inhibiting OXPHOS subunit expression (61).
As summarized above, the altered gene expression for MRPs such as, DAP3, MRPS6, MRPS18A, MRPS18B, and MRPS23 has been detected in highly proliferative and aggressive forms of breast cancers (40, 45, 46, 48, 50, 60); however, their steady-state protein expressions have not been studied in relation to neither OXPHOS subunit nor the mitochondrial translation component expressions in the majority of these studies. Quantitative analyses of protein expression in breast cancer and its subtypes in an unbiased manner have been greatly facilitated by mass spectrometry (MS)-based proteogenomic studies (62–65).
In this study, we have used 26 ER/PR(+) biopsies from breast cancer patients and shown that the altered MRP expression was correlated with reduced mitochondrial translation and OXPHOS subunit expression. The mining of the breast cancer proteomics data published by the Clinical Proteomic Tumor Analysis Consortium (CPTAC) also supported our findings on the heterogeneity of OXPHOS subunit and MRP expression in breast cancer. These observations suggest that defects in mitochondrial translation components play an important role in the remodeling of the energy metabolism by altering OXPHOS subunit expression, and possibly, mitochondrial apoptotic pathways in ER/PR(+) breast cancer. The alterations in mitochondrial translation and its components could serve as diagnostic markers in determining heterogeneity of ER/PR(+) subtypes and predicting disease prognosis.
Twenty-six treatment-naïve de-identified ER/PR(+) tumor (invasive ductal carcinoma) and normal breast tissue biopsies were removed by surgical excision from patients treated at the Marshall University Edwards Comprehensive Cancer Center, Huntington, WV. Tumor characteristics of biopsy samples are given in Table S1. Breast cancer subtypes were determined by immunohistochemistry, immunofluorescence, and fluorescence in situ hybridization techniques by the Edwards Comprehensive Cancer. Tissue protein lysates were prepared by resuspension and sonication of biopsies in RIPA buffer containing 1% SDS and NP40. Protein concentration was determined by the bicinchoninic acid (BCA) assay (Pierce, Rockford, USA).
Human breast cell lines MCF10A, MCF7, and MDA-MB-231 cell lines were purchased from the American Type Culture Collection (ATCC). The MCF 10A cell line was cultured in Dulbecco’s Modified Eagle Medium/Nutrient Mixture F-12 (DMEM/F12 (1:1)) as recommended by ATCC. Monolayer cultures of MCF7 and MDA-MB-231 cell lines were maintained in DMEM (HyClone, Thermo-Scientific, Waltham, MA) containing 10% fetal bovine serum (FBS) (Rocky Mountain Biologicals, Missoula, MT), 4 mM glutamine, 1 mM pyruvate, and 1% penicillin/streptomycin (Corning Cellgro, Manassas, VA). The cells were grown in a humidified incubator at 37°C with 5% CO2.
The expression of the 13 mt-encoded subunits of OXPHOS complexes was determined by 35S-Met pulse labeling described previously (66). Pulse labeling experiments were performed with breast cancer cell lines grown to 60-70% confluency. After arresting cytosolic protein synthesis by emetine, cells were incubated in 0.2 mCi/mL of [35S]-methionine (Perkin Elmer) containing media for 2h. Cells were lysed in RIPA buffer supplemented with protease and phosphatase inhibitors (Sigma-Aldrich). Whole cell lysates (30 μg) were electrophoresed through 13% SDS-PAGE. The gels were dried on 3MM chromatography paper (Whatman), and the signal intensities of the bands were quantified by ImageJ.
Tissue lysates obtained from biopsies and cell lines were either diluted further or lysed in RIPA buffer containing 50 mM Tris-HCl (pH 7.6), 150 mM NaCl, 1 mM EDTA, 1 mM EGTA, 1% NP40, 0.1% SDS, 0.5% deoxycholate, and protease and phosphatase inhibitor cocktails. Protein concentrations were determined using BCA assays (Pierce, Rockford, USA). The protein lysates (usually 15-30 µg) were then separated on 12% SDS-PAGE, transferred to nitrocellulose membranes (Amersham, GE Healthcare, Pittsburg, PA), stained with Ponceau S to ensure equal protein loading. The antibodies were commercially obtained as follows: Human OXPHOS antibody cocktail and SDHA from Abcam (Eugene, OR); MRPS30, MRPL11, and MRPS18B antibodies from Sigma Aldrich (St. Louis, MO); DAP3 (or MRPS29) antibody from BD Biosciences (San Jose, CA); mitochondrial Citrate synthase (CS), DAR2, TSFM, TUFM and TFAM; antibodies from Santa Cruz (Dallas, TX); and the loading control antibody GAPDH from Fitzgerald (Acton, MA). The secondary anti-rabbit and mouse HRP-conjugate antibodies from (Pierce, Rockford, USA). The protein immunoreactivity was detected using the ECL Western blotting kit from Amersham (GE Healthcare, UK) and the membranes were developed per the manufacturer’s protocol. Immunoblotting signal intensities were quantified by ImageJ (67) and UN-Scan-It (Silk Scientific, Inc, Orem, UT) and normalized to Ponceau S stained membranes. Relative protein expression detected by immunoblotting analyses was normalized to protein loading adjusted by Ponceau S staining and the average of protein expression detected by the same antibody in all biopsies. Normalizing the expression of a protein in each tumor biopsy to the average of the expression of the same protein in tumor biopsies was also used in proteomics studies of breast cancer by CPTAC (65). Due to the differences in breast tumor and normal tissue albumin expression, relative protein expression was only compared in ER/PR(+) tumor biopsies to prevent unequal protein loading (Supplementary Figures 1, 2).
Breast tissue biopsies and breast cancer cell lines were homogenized by sonication in Complex IV assay buffer (10 mM Tris-HCl, pH 7.0, 120 mM KCl, 250 mM sucrose, 1 mM n-Dodecyl-β-D maltoside). Protein concentration was determined by BCA assay. Complex IV activity was measured kinetically by oxidation of reduced ferrocytochrome c at 550 nm as previously described (68). Specific activities were calculated from the linear region of the kinetic measurements and normalized to the protein amounts using the Ponceau S staining (Supplementary Figure 3). The data are expressed as the mean ± SD of at least three independent experiments of triplicates.
Total RNA was extracted from breast tumor biopsies in the presence of TRIzol (Invitrogen, Carlsbad, CA) and converted to cDNA with the High-Capacity cDNA reverse transcription kit using random primers (Applied Biosystems, Inc., Foster City, CA). Quantitative real-time PCR (qRT-PCR) was carried out using the PowerUp SYBR green master mix (Applied Biosystems, Inc.), and samples were run on an Applied Biosystems Step One Plus instrument. The relative expression values were calculated using the ΔΔCt method for both biological and technical replicates (33). Relative mRNA expression levels were calculated using GAPDH as endogenous control and MCF10A values as calibrator. The following forward and reverse primers were used for quantitative real-time PCR.
GAPDH5' GTCTTCACCACCATGGAGAAGG 3' (FW)
5' ATGAGGTCCACCACCCTGTTGC 3' (REV)
ND65' CTCACCAAGACCTCAACCCC 3' (FW)
5' ATTGTTAGCGGTGTGGTCGG 3' (REV)
COI5' TTCGCCGACCGTTGACTATT 3' (FW)
5' GGGGGCACCGATTATTAGGG 3'(REV)
DAP35' AATCCCACTCAGTCAGAGCC 3' (FW)
5' CCAGTGGATGGAGTTGCCTT 3'(REV)
MRPL115' AAGCAGAGGGGGTTAGTGGT 3' (FW)
5' TGGGGGTTGTCTAGGATGGT 3' (REV)
MRPS18B 5' TCCTGACGTTACATTGTCCCC 3' (FW)
5' AGTCAGAGCCACCAGGTACA 3'(REV)
Statistical and graphical analyses were performed using Excel and GraphPad Prism 9.3. All the values were in triplicates wherever possible and expressed as mean ± SD, unless otherwise described.
Increased energy metabolism, specifically OXPHOS, is implicated in the progression and metastasis of breast tumors and is suggested to cause resistance to endocrine treatment (47, 69). Studies revealing the heterogeneity of OXPHOS and the underlying factors can be essential to develop alternative treatment options and prognostic markers for ER/PR(+) breast cancer. To evaluate the modulation of mitochondrial energy metabolism and biogenesis, we obtained 26 treatment-naïve ER/PR(+) invasive ductal carcinoma biopsies. The tumor characteristics and stages of invasive ductal carcinoma biopsies are given in the Table S1 and the majority of these biopsies were classified as ER/PR (+) subtype.
One of the convenient and effective ways to determine the changes in expression of proteins involved in OXPHOS is to detect steady-state expression of OXPHOS subunits by immunoblotting using an antibody cocktail that recognizes at least one subunit from each complex. The cocktail is a mixture of five antibodies generated against four of the nuclear-encoded subunits of complex V (ATP5A1), III (UQCRC2), II (SDHB), and I (NDUFB8) and a mt-encoded subunit of complex IV (MT-COII). We first compared OXPHOS subunit expression using equal protein loading in tumor and adjacent normal tissue biopsies for each patient. Due to the high albumin content detected by the Ponceau S staining in normal tissue biopsies, equal protein loading was not sufficient to demonstrate the changes in OXPHOS subunit expression in tumor biopsies relative to the normal tissue lysates. Clearly, the OXPHOS subunit expression was at least 2-3-fold higher in tumor biopsies (Supplementary Figure 1). This observation supports the increase in epithelial cell content in breast tumors and makes the interpretation of the increased mitochondrial energy metabolism in breast tumors relative to normal tissue more challenging.
To evaluate the changes in mitochondrial energy metabolism in tumor biopsies, equal amounts of tumor lysates were separated on 12% SDS-PAGE and the same membrane blot was probed with OXPHOS, SDHA, and GAPDH antibodies (Figure 1A and Supplementary Figure 2). Relative expression of each OXPHOS subunit was quantified by normalizing protein loading to Ponceau S staining and the average signal detected by the corresponding antibody in all the tissues. Using this analysis, we were able to quantify OXPHOS subunit expression in ER/PR(+) tumor biopsies (Figure 1B). The change in expression of complex I and IV subunits, NDUFS8 and MT-COII, respectively, were more drastic than that of complex V, III, and II subunits (shown by arrows in Figure 1A). Although the NDUFB8 is a nuclear-encoded complex I subunit, its expression is highly sensitive to the reduced expression of seven mt-encoded complex I subunits which are shown to be critical for defining an aggressive phenotype in breast cancer (70).
Figure 1 OXPHOS subunit expression heterogeneity in ER/PR(+) breast cancer. (A) The expression of OXPHOS subunits, including ATP5A1 (Complex V), UQCRC2 (Complex III), SDHA and SDHB (Complex II), MT-COII (Complex IV), and NDUFB8 (Complex I),were detected by immunoblotting in ER/PR(+) invasive ductal carcinoma biopsy lysates. Approximately, 20 μg of protein lysates obtained from tumor tissues was separated by 12% SDS-PAGE, and equal protein loading was evaluated by GAPDH antibody and Ponceau S staining (see Figure S2). (B) Quantitation of OXPHOS subunit expression in patient tumor biopsies by normalizing average signal intensities for each antibody to protein loading. AU; arbitrary units. (C) Complex IV specific activity was determined by measuring the rate of cytochrome c oxidation at 550 nm using equal amounts (~20 μg) of biopsy lysates selected from patients expressing high, medium, and low levels of MT-COII as shown in panel A. Results represent the mean ± SD of at least three experiments. (D) Heatmap representation of log2 ratios of OXPHOS subunit expression in luminal A and B subtypes of breast cancer detected by MS-based proteomics published by Krug et al. (65) (Table S2). Only the ER/PR(+) luminal A and B subtypes analyzed by the CPTAC used in the figure. The expression of OXPHOS subunits is ranked from high (red) to low (blue) MT-COII log2 ratios.
The changes in MT-COII expression imply a defect in mitochondrial biogenesis as it is one of the three mt-encoded subunits of complex IV. To correlate the changes in MT-COII protein expression to complex IV activity, assays were performed using biopsies chosen from groups expressing varying steady-state levels of MT-COII (Figure 1A). Here, the complex IV activity assay serves as a measure of defects caused by MT-COI, MT-COII, and MT-COIII and other nuclear-encoded subunits of complex IV. The complex IV subunit expression and activity is determined to be the rate-controlling step in breast cancer (71). Not to our surprise, we also observed a direct correlation between the subunit expression (shown in Figure 1A) and the complex IV activities when biopsy samples from the same patient were compared (Figure 1C). Hence, the decrease detected in MT-COII expression is directly proportional to complex IV activities in these biopsies. Taken together, these observations suggest that the ER/PR(+) breast cancer can be further sub-classified into tumors with high, medium, and low OXPHOS activities in ER/PR(+) subtypes.
Recent proteogenomic studies by the CPTAC have provided MS-based quantitation of proteins expressed in various breast cancer subtypes (64, 65). The data mining studies of these proteomics data repositories allowed us to compare the expression of the same nuclear- and mt-encoded OXPHOS subunits in breast cancer luminal A and B subtypes which mainly correspond to the ER/PR(+) subtype that we analyzed in this study. Additionally, the expression of nuclear-encoded subunits SDHA, ATP5A1, UCQRC2, and SDHB displayed more variability while the mt-encoded subunit MT-COII and NDUFB8 expressions were lower in more than half of the tumor tissues analyzed by the CPTAC (Figure 1D and Table S2). The agreement between the data from the breast cancer CPTAC proteomics analyses and our immunoblotting studies imply that the reduced steady-state expression of complex I and IV subunits, NDUFB8 and MT-COII, respectively, contributes to the heterogeneity of energy metabolism in ER/PR(+) breast cancer.
Based on our studies of mitochondrial translation over the decades, one of the most obvious causes for the reduced expression of mt-encoded subunits of complex IV such as MT-COI and MT-COII is a defect in the translation machinery including ribosomes and translation factors. For this reason, we carried out immunoblotting analyses of several MRPs associated with breast cancer as well as mitochondrial translation and transcription factors in tumor biopsies for their impacts on OXPHOS subunit expression. As shown in Figure 2A, the changes in the small (28S) and the large (39S) ribosomal subunit proteins, DAP3 (MRPS29), MRPS18B, MRPS30, and MRPL11, reflected the changes observed in MT-COII and NDUFB8 expressions obtained for the same patient (Figures 1A, 2A). The other translation-related proteins mitochondrial translation elongation factor Ts (TSFM) and mitochondrial aspartyl-tRNA synthetase (DARS2) also followed a relatively similar pattern to MRP, MT-COII, and NDUFB8 expressions when compared to the same patient. This observation allowed us to correlate MRP expressions to OXPHOS subunit expression and group their expressions as high, medium, and low in 26 ER/PR(+) biopsies (Figures 1B, 2B and Supplementary Figure 3). On the other hand, neither nuclear and mitochondrial transcription factors, PGC1a and TFAM, respectively, nor VDAC and CS protein expressions could be correlated to OXPHOS subunit expression in tumor biopsies (Figure 2A). Therefore, this led us to suggest that mitochondrial translation is one of the major determinants of the energy metabolism in a significant number of ER/PR(+) biopsies analyzed by immunoblotting in our studies.
Figure 2 Mitochondrial translation and transcription related protein expression in ER/PR(+) breast cancer. (A) Relative expression of the mitochondrial small (28S) and large (39S) subunit proteins DAP3, MRPS18B, MRPS30, and MRPL11 were detected by immunoblotting analyses. TSFM and DARS2 are the two other mitochondrial translation related factors. Mitochondrial transcription related proteins, TFAM and PGC1a, as well as VDAC are also detected by immunoblotting of tumor lysates. Approximately 20 μg of lysates were separated by 12% SDS-PAGE and equal protein loading was evaluated by probing membranes with Ponceau S staining and GAPDH (data not shown). (B) Expression of various MRPs in the CPTAC data (65) set as described in Figure 1D legend. DAP3 expression in luminal A and B breast tumors is ranked from high (red) to low(blue) expression to show the correlation between the MRPs and the translation related factors DARS2 and TSFM.
The correlation observed between the MRP and mitochondrial translation factor expressions in ER/PR(+) tumor biopsies was also assessed using the CPTAC breast cancer proteomics data published by Krug et al. (65). The heat map analysis of MRPs and various mitochondrial translation-related proteins factors also verified the reduced expression of these proteins in the majority of the ER/PR(+) luminal A and B subtypes (Figure 2B and Table S2). Moreover, the majority of the small and large subunit MRP expressions followed a consistent pattern patient to patient in all the breast cancer subtypes (Figure S4). Another significant observation was the reduced expression of MRPs seen in over 70% of the ER/PR (+) luminal A subtype. The CPTAC proteomics data and our results suggest that the MRP and/or mitochondrial translation-related protein expression can be used as prognostic markers of tumor metabolism in ER/PR(+) breast cancer and its subtypes.
Epithelial to mesenchymal transition (EMT) is associated with metastatic characteristics of tumors as well as their resistance to therapy. In many cases, this transition occurs through a hybrid state identified by the expression of many different markers for the epithelial, hybrid, or mesenchymal states (72). The best predictors for these states are determined by vimentin (VIM) and E-cadherin (CHD1) gene expression ratio combined with claudin 7 (CLDN7) expression using the TCGA breast cancer datasets (73) as well as the correlation of MMP2 expression to the progression of breast cancer to bone metastasis (74). Overexpression of the two mitochondrial proteins, citrate synthase (CS) and complex V subunit, ATP5C1 (or ATP5F1C), are described as markers for aggressiveness in triple negative breast cancer (75, 76).
The EMT status of the ER/PR(+) breast tumor biopsies was evaluated by immunoblotting analyses of VIM, CHD1, and CS expressions. As anticipated, the majority of the biopsies expressed high levels of VIM while half of the samples expressed both VIM and CHD1 (Figure 3A). Expression of both VIM and CHD1 is interpreted as the hybrid state as often observed in the TCGA breast tumor data sets (73). There was no correlation between the tumor stages and high VIM and CS expressions; however, some of the biopsies still preserved the CHD1 expression (Figure 3A). When the expression of EMT markers was compared to OXPHOS subunit expression for the same patient, the patients with high to moderate OXPHOS subunit expression, specifically the MT-COII, with the CHD1 expression suggesting a hybrid EMT state for the patient (Figure 3A).
Figure 3 Expression of epithelial-mesenchymal transition markers in ER/PR(+) breast cancer. (A) Expressions of vimentin (VIM), E-cadherin (CHD1), and citrate synthase (CS) were detected by immunoblotting using 20 μg of patient biopsies as described in Figures 1A and 2A. Relative VIM, CHD1, and CS expressions were quantified by normalizing antibody signal intensities to protein loading. (B) Expression of VIM, metalloprotease-2 (MMP2), CHD1, and claudin-7 (CLDN7) was compared to MT-COII and NDUFB8 log2 expression ratios to demonstrate the changes in EMT and metastatic markers using the CPTAC data set (65) (see text for the discussion). VIM expression is ranked from high (red) to low (blue). Increased VIM and MMP2 expression in majority of the luminal A and B biopsies correspond to the decreased OXPHOS subunit expression (MT-COII and NDUFB8) and epithelial markers (CHD1 and CLDN-7).
Although immunoblotting analyses were not directly comparable due to different signal intensities, ER/PR(+) breast tumor proteomics data allowed us to correlate mitochondrial energy metabolism and translation to EMT status further. The log2 protein expression values for OXPHOS subunits and mitochondrial translation components used in Figures 1D, 2B, respectively, were compared to VIM, CLDN7, and MMP2 expression going from high to low VIM expression (Figure 3B and Table S2). The VIM and MMP2 expression trends were in agreement while CLDN7 expression increased as VIM and MMP2 decreased. In the majority of the luminal A and B biopsies with high VIM and MMP2 expression, both MT-COII and NDUFB8 decreased (Figure 3B). This tendency was also observed in mitochondrial translation components (Figure S5). As seen in immunoblotting analyses of ER/PR(+) biopsies, the OXPHOS subunits, specifically the MT-COII and NDUFB8, expressions were proportional to the changes in mitochondrial translation components rather than the mitochondrial transcription related factors TFAM and PGC1a (Figures 1A, 2A). Moreover, other mitochondrial proteins involved in breast cancer aggressiveness, such as ATP5C1 and CS did not show any specific tendency or change with increased VIM and MMP2 expressions in luminal A and B breast cancer subtypes (Figure 3B). Therefore, the modulation of mitochondrial translation and/or expression of its components could be significant in remodeling of energy metabolism and EMT transition in ER/PR (+) breast cancer.
Previously, mtDNA mutations, low copy numbers, and reduced mitochondrial transcript levels were associated with increased metastasis and poor prognosis in breast cancer (6–9). The results presented in Figures 1, 2 also clearly demonstrated that the ER/PR(+) breast tumor biopsies have a high degree of OXPHOS heterogeneity supported by the changes in MRP expression or reduced protein synthesis in mitochondria. The majority of the biopsies had medium or low OXPHOS levels, while OXPHOS and MRP expression levels were significantly increased in the remainder. It is then highly likely that the low OXPHOS levels are due to the defects in mitochondrial biogenesis including mitochondrial translation and transcription in the ER/PR(+) biopsies. To recapitulate these observations, we first determined the OXPHOS subunit expression in a non-tumorigenic epithelial breast cell line MCF10A, ER/PR(+) MCF7, and triple-negative MDA-MB-231 cell lines by immunoblotting (Figure 4A). Similar to the patient biopsies, complex I and IV subunit expressions were more variable than the complex II, III, and V subunits specifically in MCF7 and MDA-MB-231 cell lines (shown by arrows in Figure 4A). The variation at the steady-state nuclear and mitochondrial-encoded protein OXPHOS subunit expression was also reflected in OXPHOS activities in these cell lines (4).
Figure 4 Altered OXPHOS subunit expression and mitochondrial translation in breast cancer cell lines. (A) The OXPHOS subunit expression was detected by immunoblotting of approximately 30 μg lysates obtained from breast cancer cell lines MCF10A (10A), MCF7 (MCF7), and MDA-MB-23 (MDA) as described in Figure 1A. The relative quantitation of OXPHOS subunit expression represent the mean ± SD of at least three experiments with MCF10A subunit expression adjusted to 100%. (B) Mitochondrial translation is determined by 35S-Met pulse labeling of 13 mt-encoded OXPHOS subunits in breast cancer cell lines. The 13 mt-encoded complex I subunits ND1-ND6; complex III subunit cyt b; complex IV subunits COI-COIII; and complex V subunits ATP6 and ATP8 are labeled on the autoradiography of the 13% SDS-PAGE. Total protein loading (30 μg) is visualized by Coomassie Blue staining of the 13% gel. Relative quantitation of mitochondrial translation in MCF10A, MCF7, and MDA-MB-231 cells represents the mean ± SD of the pulse labeling of 13 mt-encoded proteins. (C) The complex IV specific activity was determined using (~20 μg) of MCF7 and MDA-MB-231 cell lysates as described in Figure 1C. (D) Immunoblotting analyses of MRPs (DAP3, MRPS18B, MRPS30, and MRPL11) and mitochondrial translation (TUFM) and transcription (TFAM) related proteins in breast cancer cell lines. Equal protein loading was determined by probing membranes with GAPDH and Ponceau S staining (data not shown). Results represent the mean ± SD of at least three experiments. (E) Relative changes in mt-encoded ribosomal large subunit rRNA, 16S rRNA, and the MRP mRNAs, DAP3, MRPS18B, and MRPL11 were determined by quantitative RT-PCR in the MCF10A, MCF7, and MDA-MB-231 cell lines. Results represent the mean ± SD of at least three experiments adjusted to MCF10A mRNA expression as the 100%.
We next investigated the role of mitochondrial translation on reduced OXPHOS subunit expression and complex IV activities in breast cancer cell lines. For this purpose, [35S]-Met pulse-labeling of de novo synthesized mt-encoded proteins was performed in the presence of emetine, an inhibitor of cytosolic protein synthesis (66). In this analysis, [35S]-Met is only incorporated into the 13 mt-encoded OXPHOS subunits by the mitochondrial translation machinery (Figure 4B). The de novo protein synthesis relative to that of the MCF10A non-tumorigenic epithelial breast cell line was reduced by about 50% and 70% in MCF7 and MDA-MB-231 cell lines, respectively (Figures 4B). The effect of reduced OXPHOS subunit expression shown in Figures 4A, B also was observed in complex IV assays performed using MCF7 and MDA-MB-231 cell lysates. The de novo mitochondrial protein synthesis in MDA-MB-231 cells was approximately 50% of the protein synthesis in MCF7 cells (Figure 4B). This reduction was in agreement with the complex IV activities determined in MCF7 and MDA-MB-231 cells (Figure 4C).
The defect observed in mitochondrial translation could be due to defect(s) in the expression of mitochondrial translation components or their transcription. In order to assess their effect on OXPHOS subunit expression, we performed immunoblotting and qRT-PCR analyses in these cell lines. Quantitation of immunoblots showed a clear reduction in several MRPs, such as DAP3 and MRPS30, as well as mitochondrial transcription factor TFAM in ER/PR(+) MCF7 cell lines (Figure 4D). A clear reduction at the steady-state expression of MRPs was not observed in our analyses of MDA-MB-231 cells; however, decreased expression of MRPS23, one of the 30 mitochondrial small subunit ribosomal proteins, and as well as its methylation at Arg and Lys residues have shown to inhibit OXPHOS and promote the aggressive and metastatic types of breast cancer (61). At the transcription level, 16S rRNA and DAP3 transcripts were reduced in both MCF7 and MDA-MB-231 cell lines (Figure 4E). The other MRP transcripts on the other hand did either not significantly change or increased 2-3 folds possibly to compensate for the reduced synthesis of MRPs, TFAM, or TUFM in these cell lines (Figure 4E). The 50% decrease observed in de novo protein synthesis in MCF7 cell lines could be caused by defects in mitochondrial translation and transcription (Figure 4B). The only factor that decreased in the MDA-MB-231 cell line was the mitochondrial translation factor TUFM. The four-fold reduction in mitochondrial translation cannot only be explained by the 35% reduction in TUFM expression in MDA-MB-231 (Figures 4B, D). MDA-MB-231 has relatively high mitochondrial heteroplasmy associated with higher invasion and metastatic capabilities supported by the glycolytic energy metabolism rather than OXPHOS compared to the MCF7 cell lines (77). In fact, the MS-based proteomics studies performed in our laboratory clearly show that the expression of glycolytic enzymes increased significantly in MDA-MB-231 cells relative to that of MCF7 cell lines (Table S3). The changes determined in the de novo protein synthesis and in expression of mitochondrial translation components in these cell lines could also be correlated to alterations observed in 26 ER/PR(+) breast tumor biopsies.
Evidence suggests that the mutations and defects in mtDNA and the proteins supporting OXPHOS and apoptosis alter mitochondrial function in disease, specifically in cancer. Undeniably, mitochondria play a key role in these processes; however, we are far from understanding the cellular and molecular mechanisms maintaining this balance. In this study, we investigated the changes in the expression of OXPHOS subunits and MRPs responsible for the synthesis of 13 mt-encoded subunits in 26 ER/PR(+) biopsies. Alterations in the expression of MRPs and mitochondrial translation-related proteins directly influenced the expression of complex I and IV subunits, NDUFB8 and MT-COII, in the majority of the biopsies. The strong agreement between the OXPHOS subunit expression analysis by immunoblotting and the MS-based proteogenomic studies by the CPTAC have provided confidence in our hypothesis given above. Although we were not able to associate tumor stages and OXPHOS subunit expression, the reduced OXPHOS and MRP expression in the majority of the ER/PR(+) biopsies is noteworthy.
With the metabolic heterogeneity of tumor cells in mind, one of the controversies that need to be resolved is the contribution of OXPHOS to aggressiveness and development of chemo-resistance and recurrence in breast cancer. At large, defects and mutations in mtDNA and other OXPHOS components are known to induce invasiveness and recurrence in breast cancer (12, 13, 47). In several histopathological and patient-derived primary cell studies, the increased mitochondrial mass has been shown to develop cancer stem-like cells, which are more chemo-resistant, resulting in tumor recurrence, and distant metastasis (12, 16, 42). In conclusion, further elucidation of mitochondrial transcription and translation machineries supporting OXPHOS is necessary to identify the mechanism(s) fueling breast cancer invasiveness, metastasis, and chemo-resistance.
The datasets presented in this study can be found in online repositories. The names of the repository/repositories and accession number(s) can be found in the article/Supplementary Material.
EK and HK designed the study. EK, FCK, and FK prepared biopsies and breast cancer cell lines for immunoblotting analyses and activity assays. EK performed 35S-Met pulse labeling assays. FCK and EK performed the data mining analysis of the CPTAC proteome and prepared the figures. EK, MT, and HK involved in writing or revising the manuscript and the grant proposal that partially funded this work. All authors contributed to the article and approved the submitted version.
This research was partially funded by Edwards Comprehensive Cancer Center to EK, HK, and MT and The Scientific and Technological Research Council (TUBITAK) of Turkey to FK. The tissue procurement center at the Marshall University Edwards Comprehensive Cancer Center was supported by a grant (2U54GM104942) from the National Institute for General Medical Sciences (NIGMS) awarded to the West Virginia Clinical and Translational Science Institute (WV-CTSI).
The authors would like to thank Tissue Procurement Center at the Edwards Comprehensive Cancer Center at the Marshall University for providing tumor and normal breast biopsies. We also gratefully acknowledge Biomedical Sciences Department at Joan C. Edwards School of Medicine, Marshall University for its support. The authors also appreciate the discussions regarding data analysis with Drs. Vincent Sollars and James Denvir.
The authors declare that the research was conducted in the absence of any commercial or financial relationships that could be construed as a potential conflict of interest.
All claims expressed in this article are solely those of the authors and do not necessarily represent those of their affiliated organizations, or those of the publisher, the editors and the reviewers. Any product that may be evaluated in this article, or claim that may be made by its manufacturer, is not guaranteed or endorsed by the publisher.
The Supplementary Material for this article can be found online at: https://www.frontiersin.org/articles/10.3389/fonc.2022.897207/full#supplementary-material
Data Sheet 1 - Supplementary Figures.
1. Hanahan D, Weinberg RA. Hallmarks of cancer: the next generation. Cell (2011) 144(5):646–74. doi: 10.1016/j.cell.2011.02.013
3. Choudhury AR, Singh KK. Mitochondrial determinants of cancer health disparities. Semin Cancer Biol (2017) 47:125–46. doi: 10.1016/j.semcancer.2017.05.001
4. Owens KM, Kulawiec M, Desouki MM, Vanniarajan A, Singh KK. Impaired OXPHOS complex III in breast cancer. PloS One (2011) 6(8):e23846. doi: 10.1371/journal.pone.0023846
5. Plak K, Czarnecka AM, Krawczyk T, Golik P, Bartnik E. Breast cancer as a mitochondrial disorder (Review). Oncol Rep (2009) 21(4):845–51. doi: 10.3892/or_00000293
6. Tseng LM, Yin PH, Chi CW, Hsu CY, Wu CW, Lee LM, et al. Mitochondrial DNA mutations and mitochondrial DNA depletion in breast cancer. Genes Chromosomes Cancer (2006) 45(7):629–38. doi: 10.1002/gcc.20326
7. Yadav N, Chandra D. Mitochondrial DNA mutations and breast tumorigenesis. Biochim Biophys Acta (2013) 1836(2):336–44. doi: 10.1016/j.bbcan.2013.10.002
8. Guha M, Srinivasan S, Ruthel G, Kashina AK, Carstens RP, Mendoza A, et al. Mitochondrial retrograde signaling induces epithelial-mesenchymal transition and generates breast cancer stem cells. Oncogene (2014) 33(45):5238–50. doi: 10.1038/onc.2013.467
9. Guha M, Srinivasan S, Raman P, Jiang Y, Kaufman BA, Taylor D, et al. Aggressive triple negative breast cancers have unique molecular signature on the basis of mitochondrial genetic and functional defects. Biochim Biophys Acta Mol Basis Dis (2018) 1864(4 Pt A):1060–71. doi: 10.1016/j.bbadis.2018.01.002
10. Peng S, Lu B, Ruan W, Zhu Y, Sheng H, Lai M. Genetic polymorphisms and breast cancer risk: evidence from meta-analyses, pooled analyses, and genome-wide association studies. Breast Cancer Res Treat (2011) 127(2):309–24. doi: 10.1007/s10549-011-1459-5
11. Li Y, Giorgi EE, Beckman KB, Caberto C, Kazma R, Lum-Jones A, et al. Association between mitochondrial genetic variation and breast cancer risk: The multiethnic cohort. PloS One (2019) 14(10):e0222284. doi: 10.1371/journal.pone.0222284
12. Peiris-Pages M, Ozsvári B, Sotgia F, Lisanti MP. Mitochondrial and ribosomal biogenesis are new hallmarks of stemness, oncometabolism and biomass accumulation in cancer: Mito-stemness and ribo-stemness features. Aging (Albany NY) (2019) 11(14):4801–35. doi: 10.18632/aging.102054
13. Lunetti P, Di Giacomo M, Vergara D, De Domenico S, Maffia M, Zara V, et al. Metabolic reprogramming in breast cancer results in distinct mitochondrial bioenergetics between luminal and basal subtypes. FEBS J (2019) 286(4):688–709. doi: 10.1111/febs.14756
14. Gao W, Wu M, Wang N, Zhang Y, Hua J, Tang G, et al. Increased expression of mitochondrial transcription factor a and nuclear respiratory factor-1 predicts a poor clinical outcome of breast cancer. Oncol Lett (2018) 15(2):1449–58.
15. Brandon M, Baldi P, Wallace DC. Mitochondrial mutations in cancer. Oncogene (2006) 25(34):4647–62. doi: 10.1038/sj.onc.1209607
16. Salem AF, Whitaker-Menezes D, Howell A, Sotgia F, Lisanti MP. Mitochondrial biogenesis in epithelial cancer cells promotes breast cancer tumor growth and confers autophagy resistance. Cell Cycle (2012) 11(22):4174–80. doi: 10.4161/cc.22376
17. D'Souza AR, Minczuk M. Mitochondrial transcription and translation: overview. Essays Biochem (2018) 62(3):309–20.
18. Koc EC, Spremulli LL. Identification of mammalian mitochondrial translational initiation factor 3 and examination of its role in initiation complex formation with natural mRNAs. J Biol Chem (2002) 277(38):35541–9. doi: 10.1074/jbc.M202498200
19. Koc EC, Cimen H, Kumcuoglu B, Abu N, Akpinar G, Haque ME, et al. Identification and characterization of CHCHD1, AURKAIP1, and CRIF1 as new members of the mammalian mitochondrial ribosome. Front Physiol (2013) 4:183. doi: 10.3389/fphys.2013.00183
20. Koc EC, Burkhart W, Blackburn K, Moyer MB, Schlatzer DM, Moseley A, et al. The large subunit of the mammalian mitochondrial ribosome. analysis of the complement of ribosomal proteins present. J Biol Chem (2001) 276(47):43958–69. doi: 10.1074/jbc.M106510200
21. Koc EC, Burkhart W, Blackburn K, Moseley A, Spremulli LL. The small subunit of the mammalian mitochondrial ribosome: Identification of the full complement of ribosomal proteins present. J Biol Chem (2001) 276:19363–74. doi: 10.1074/jbc.M100727200
22. Amunts A, Brown A, Toots J, Scheres SHW, Ramakrishnan V. Ribosome. the structure of the human mitochondrial ribosome. Science (2015) 348(6230):95–8.
23. Brown A, Amunts A, Bai XC, Sugimoto Y, Edwards PC, Murshudov G, et al. Structure of the large ribosomal subunit from human mitochondria. Science (2014) 346(6210):718–22. doi: 10.1126/science.1258026
24. Suzuki T, Terasaki M, Takemoto-Hori C, Hanada T, Ueda T, Wada A, et al. Structural compensation for the deficit of rRNA with proteins in the mammalian mitochondrial ribosome. systematic analysis of protein components of the large ribosomal subunit from mammalian mitochondria. J Biol Chem (2001) 276(24):21724–36. doi: 10.1074/jbc.M100432200
25. Koc EC, Haque ME, Spremulli LL. Current views of the structure of the mammalian mitochondrial ribosome. Israel J Chem (2010) 50(1):45–59. doi: 10.1002/ijch.201000002
26. Koc EC, Burkhart W, Blackburn K, Koc H, Moseley A, Spremulli LL. Identification of four proteins from the small subunit of the mammalian mitochondrial ribosome using a proteomics approach. Prot. Sci. (2001) 10:471–81. doi: 10.1110/ps.35301
27. Koc EC, Burkhart W, Blackburn K, Moseley A, Koc H, Spremulli LL.. A proteomics approach to the identification of mammalian mitochondrial small subunit ribosomal proteins. J Biol Chem (2000) 275:32585–91. doi: 10.1074/jbc.M003596200
28. Kenmochi N, Suzuki T, Uechi T, Magoori M, Kuniba M, Higa S, et al. The human mitochondrial ribosomal protein genes: mapping of 54 genes to the chromosomes and implications for human disorders. Genomics (2001) 77(1/2):65–70. doi: 10.1006/geno.2001.6622
29. O'Brien T W, O'Brien B J, Norman RA. Nuclear MRP genes and mitochondrial disease. Gene (2005) 354:147–51. doi: 10.1016/j.gene.2005.03.026
30. Huang G, Li H, Zhang H. Abnormal expression of mitochondrial ribosomal proteins and their encoding genes with cell apoptosis and diseases. Int J Mol Sci (2020) 21(22):8879–98. doi: 10.3390/ijms21228879
31. Gopisetty G, Thangarajan R. Mammalian mitochondrial ribosomal small subunit (MRPS) genes: A putative role in human disease. Gene (2016) 589(1):27–35. doi: 10.1016/j.gene.2016.05.008
32. Koc EC, Ranasinghe A, Burkhart W, Blackburn K, Koc H, Moseley A, et al. A new face on apoptosis: Death-associated protein 3 and PDCD9 are mitochondrial ribosomal proteins. FEBS Lett (2001) 492:166–70. doi: 10.1016/S0014-5793(01)02250-5
33. Han MJ, Chiu DT, Koc EC. Regulation of mitochondrial ribosomal protein S29 (MRPS29) expression by a 5'-upstream open reading frame. Mitochondrion (2010) 10(3):274–83. doi: 10.1016/j.mito.2009.12.150
34. Miller JL, Koc H, Koc EC. Identification of phosphorylation sites in mammalian mitochondrial ribosomal protein DAP3. Protein Sci (2008) 17(2):251–60. doi: 10.1110/ps.073185608
35. Miyazaki T, Shen M, Fujikura D, Tosa N, Kim HR, Kon S, et al. Functional role of death associated protein 3 (DAP3) in anoikis. J Biol Chem (2004) 279(43):44667–72. doi: 10.1074/jbc.M408101200
36. Takeda S, Iwai A, Nakashima M, Fujikura D, Chiba S, Li HM, et al. LKB1 is crucial for TRAIL-mediated apoptosis induction in osteosarcoma. Anticancer Res (2007) 27(2):761–8.
37. Kim HR, Chae HJ, Thomas M, Miyazaki T, Monosov A, Monosov E, et al. Mammalian dap3 is an essential gene required for mitochondrial homeostasis in vivo and contributing to the extrinsic pathway for apoptosis. FASEB J (2007) 21(1):188–96. doi: 10.1096/fj.06-6283com
38. Kissil JL, Cohen O, Raveh T, Kimchi A. Structure-function analysis of an evolutionary conserved protein, DAP3, which mediates TNF-alpha- and fas-induced cell death. EMBO J (1999) 18(2):353–62. doi: 10.1093/emboj/18.2.353
39. Kim HJ, Maiti P, Barrientos A. Mitochondrial ribosomes in cancer. Semin Cancer Biol (2017) 47:67–81. doi: 10.1016/j.semcancer.2017.04.004
40. Kashuba E, Yurchenko M, Yenamandra SP, Snopok B, Isaguliants M, Szekely L, et al. EBV-encoded EBNA-6 binds and targets MRS18-2 to the nucleus, resulting in the disruption of pRb-E2F1 complexes. Proc Natl Acad Sci U.S.A. (2008) 105(14):5489–94. doi: 10.1073/pnas.0801053105
41. Huang Y, Ballinger DG, Dai JY, Peters U, Hinds DA, Cox DR, et al. Genetic variants in the MRPS30 region and postmenopausal breast cancer risk. Genome Med (2011) 3(6):42. doi: 10.1186/gm258
42. Sotgia F, Whitaker-Menezes D, Martinez-Outschoorn UE, Salem AF, Tsirigos A, Lamb R, et al. Mitochondria "fuel" breast cancer metabolism: fifteen markers of mitochondrial biogenesis label epithelial cancer cells, but are excluded from adjacent stromal cells. Cell Cycle (2012) 11(23):4390–401. doi: 10.4161/cc.22777
43. Ghoussaini M, French JD, Michailidou K, Nord S, Beesley J, Canisus S, et al. Evidence that the 5p12 variant rs10941679 confers susceptibility to estrogen-Receptor-Positive breast cancer through FGF10 and MRPS30 regulation. Am J Hum Genet (2016) 99(4):903–11. doi: 10.1016/j.ajhg.2016.07.017
44. Kim TW, Kim B, Kim JH, Kang S, Park SB, Jeong G, et al. Nuclear-encoded mitochondrial MTO1 and MRPL41 are regulated in an opposite epigenetic mode based on estrogen receptor status in breast cancer. BMC Cancer (2013) 13:502. doi: 10.1186/1471-2407-13-502
45. Sorensen KM, Meldgaard T, Melchjorsen CJ, Fridriksdottir AJ, Pedersen H, Petersen OW, et al. Upregulation of Mrps18a in breast cancer identified by selecting phage antibody libraries on breast tissue sections. BMC Cancer (2017) 17(1):19. doi: 10.1186/s12885-016-2987-5
46. Buchynska LG, Iurchenko NP, Kashuba EV, Brieieva OV, Glushchenko NM, Mints M, et al. Overexpression of the mitochondrial ribosomal protein S18-2 in the invasive breast carcinomas. Exp Oncol (2018) 40(4):303–8. doi: 10.31768/2312-8852.2018.40(4):303-308
47. Sotgia F, Fiorillo M, Lisanti MP. Mitochondrial markers predict recurrence, metastasis and tamoxifen-resistance in breast cancer patients: Early detection of treatment failure with companion diagnostics. Oncotarget (2017) 8(40):68730–45. doi: 10.18632/oncotarget.19612
48. Wazir U, Jiang WG, Sharma AK, Mokbel K. The mRNA expression of DAP3 in human breast cancer: correlation with clinicopathological parameters. Anticancer Res (2012) 32(2):671–4.
49. Wazir U, Orakzai MM, Khanzada ZS, Jiang WG, Sharma AK, Kasem A, et al. The role of death-associated protein 3 in apoptosis, anoikis and human cancer. Cancer Cell Int (2015) 15:39. doi: 10.1186/s12935-015-0187-z
50. Wazir U, Sanders AJ, Wazir AM, Ye L, Jiang WG, Ster I, et al. Effects of the knockdown of death-associated protein 3 expression on cell adhesion, growth and migration in breast cancer cells. Oncol Rep (2015) 33(5):2575–82. doi: 10.3892/or.2015.3825
51. Stacey SN, Manolescu A, Sulem P, Thorlacius S, Gudjonsson SA, Jonsson GF, et al. Common variants on chromosome 5p12 confer susceptibility to estrogen receptor-positive breast cancer. Nat Genet (2008) 40(6):703–6. doi: 10.1038/ng.131
52. Bhatti P, Doody MM, Rajaraman P, Alexander BH, Yeager M, Hutchinson A, et al. Novel breast cancer risk alleles and interaction with ionizing radiation among U.S. radiologic technologists. Radiat Res (2010) 173(2):214–24.
53. Woolcott CG, Maskarinec G, Haiman CA, Verheus M, Pagano IS, Le Marchand L, et al. Association between breast cancer susceptibility loci and mammographic density: the multiethnic cohort. Breast Cancer Res (2009) 11(1):R10. doi: 10.1186/bcr2229
54. Quigley DA, Fiorito E, Nord S, Van Loo P, Alnaes GG, Fleischer T, et al. The 5p12 breast cancer susceptibility locus affects MRPS30 expression in estrogen-receptor positive tumors. Mol Oncol (2014) 8(2):273–84. doi: 10.1016/j.molonc.2013.11.008
55. Wu B, Pan Y, Liu G, Yang T, Jin Y, Zhou F, et al. MRPS30-DT knockdown inhibits breast cancer progression by targeting Jab1/Cops5. Front Oncol (2019) 9:1170. doi: 10.3389/fonc.2019.01170
56. Cai M, Li H, Chen R, Zhou X.. MRPL13 promotes tumor cell proliferation, migration and EMT process in breast cancer through the PI3K-AKT-mTOR pathway. Cancer Manag Res (2021) 13:2009–24. doi: 10.2147/CMAR.S296038
57. Tao Z, Suo H, Zhang L, Jin Z, Wang Z, Wang D, et al. MRPL13 is a prognostic cancer biomarker and correlates with immune infiltrates in breast cancer. Onco Targets Ther (2020) 13:12255–68. doi: 10.2147/OTT.S263998
58. Gao Y, Li F, Zhou H, Yang Y, Wu R, Chen Y, et al. Down-regulation of MRPS23 inhibits rat breast cancer proliferation and metastasis. Oncotarget (2017) 8(42):71772–81. doi: 10.18632/oncotarget.17888
59. Klaestad E, Opdahl S, Engstrom MJ, Ytterhus B, Wik E, Bofin AM, et al. MRPS23 amplification and gene expression in breast cancer; association with proliferation and the non-basal subtypes. Breast Cancer Res Treat (2020) 180(1):73–86. doi: 10.1007/s10549-020-05532-6
60. Oviya RP, Gopal G, Shirley SS, Sridevi V, Jayavelu S, Rajkumar T. Mitochondrial ribosomal small subunit proteins (MRPS) MRPS6 and MRPS23 show dysregulation in breast cancer affecting tumorigenic cellular processes. Gene (2021) 790:145697. doi: 10.1016/j.gene.2021.145697
61. Liu L, Zhang X, Ding H, Liu X, Cao D, Liu Y, et al. Arginine and lysine methylation of MRPS23 promotes breast cancer metastasis through regulating OXPHOS. Oncogene (2021) 40(20):3548–63. doi: 10.1038/s41388-021-01785-7
62. Geiger T, Madden SF, Gallagher WM, Cox J, Mann M. Proteomic portrait of human breast cancer progression identifies novel prognostic markers. Cancer Res (2012) 72(9):2428–39. doi: 10.1158/0008-5472.CAN-11-3711
63. Tyanova S, Albrechtsen R, Kronqvist P, Cox J, Mann M, Geiger T. Proteomic maps of breast cancer subtypes. Nat Commun (2016) 7:10259. doi: 10.1038/ncomms10259
64. Mertins P, Mani DR, Ruggles KV, Gillette MA, Clauser KR, Wang P, et al. Proteogenomics connects somatic mutations to signalling in breast cancer. Nature (2016) 534(7605):55–62. doi: 10.1038/nature18003
65. Krug K, Jaehnig EJ, Satpathy S, Blumenberg L, Karpova A, Anurag M, et al. Proteogenomic landscape of breast cancer tumorigenesis and targeted therapy. Cell (2020) 183(5):1436–1456 e31. doi: 10.1016/j.cell.2020.10.036
66. Chomyn A. In vivo labeling and analysis of human mitochondrial translation products. Methods Enzymol (1996) 264:197–211. doi: 10.1016/S0076-6879(96)64020-8
67. Schneider CA, Rasband WS, Eliceiri KW. NIH Image to ImageJ: 25 years of image analysis. Nat Methods (2012) 9(7):671–5. doi: 10.1038/nmeth.2089
68. Birch-Machin MA, Turnbull DM. Assaying mitochondrial respiratory complex activity in mitochondria isolated from human cells and tissues. Methods Cell Biol (2001) 65:97–117. doi: 10.1016/S0091-679X(01)65006-4
69. Farnie G, Sotgia F, Lisanti MP. High mitochondrial mass identifies a sub-population of stem-like cancer cells that are chemo-resistant. Oncotarget (2015) 6(31):30472–86. doi: 10.18632/oncotarget.5401
70. Santidrian AF, Matsuno-Yagi A, Ritland M, Seo BB, LeBoeuf SE, Gay LJ, et al. Mitochondrial complex I activity and NAD+/NADH balance regulate breast cancer progression. J Clin Invest (2013) 123(3):1068–81. doi: 10.1172/JCI64264
71. Koit A, Shevchuk I, Ounpuu L, Klepinin A, Chekulayev V, Timohhina N, et al. Mitochondrial respiration in human colorectal and breast cancer clinical material is regulated differently. Oxid Med Cell Longev (2017) p:1372640. doi: 10.1155/2017/1372640
72. Pastushenko I, Brisebarre A, Sifrim A, Fioramonti M, Revenco T, Boumahdi S, et al. Identification of the tumour transition states occurring during EMT. Nature (2018) 556(7702):463–8. doi: 10.1038/s41586-018-0040-3
73. George JT, Jolly MK, Xu S, Somarelli JA, Levine H. Survival outcomes in cancer patients predicted by a partial EMT gene expression scoring metric. Cancer Res (2017) 77(22):6415–28. doi: 10.1158/0008-5472.CAN-16-3521
74. Tauro M, Lynch CC. Cutting to the chase: How matrix metalloproteinase-2 activity controls breast-Cancer-to-Bone metastasis. Cancers (Basel) (2018) 10(6). doi: 10.3390/cancers10060185
75. Koit A, Timohhina N, Truu L, Chekulayev V, Gudlawar S, Shevchuk I, et al. Metabolic and OXPHOS activities quantified by temporal ex vivo analysis display patient-specific metabolic vulnerabilities in human breast cancers. Front Oncol (2020) 10:1053. doi: 10.3389/fonc.2020.01053
76. Fiorillo M, Scatena C, Naccarato AG, Sotgia F, Lisanti MP. Bedaquiline, an FDA-approved drug, inhibits mitochondrial ATP production and metastasis in vivo, by targeting the gamma subunit (ATP5F1C) of the ATP synthase. Cell Death Differ (2021) 28(9):2797–817. doi: 10.1038/s41418-021-00788-x
Keywords: mitochondrial translation, mitochondrial ribosomal proteins (MRPs), oxidative phosphorylation (OXPHOS), breast cancer, ER/PR(+), luminal A
Citation: Koc EC, Koc FC, Kartal F, Tirona M and Koc H (2022) Role of mitochondrial translation in remodeling of energy metabolism in ER/PR(+) breast cancer. Front. Oncol. 12:897207. doi: 10.3389/fonc.2022.897207
Received: 15 March 2022; Accepted: 25 July 2022;
Published: 30 August 2022.
Edited by:
Bela Ozsvari, University of Salford, United KingdomReviewed by:
Juan Carlos Gallardo, Instituto Nacional de Cardiologia Ignacio Chavez, MexicoCopyright © 2022 Koc, Koc, Kartal, Tirona and Koc. This is an open-access article distributed under the terms of the Creative Commons Attribution License (CC BY). The use, distribution or reproduction in other forums is permitted, provided the original author(s) and the copyright owner(s) are credited and that the original publication in this journal is cited, in accordance with accepted academic practice. No use, distribution or reproduction is permitted which does not comply with these terms.
*Correspondence: Emine C. Koc, a29jZUBtYXJzaGFsbC5lZHU=; Hasan Koc, a29jaGFAbWFyc2hhbGwuZWR1
†These authors have contributed equally to this work
‡Present address: Funda Kartal, Department of Biochemistry, Science of Faculty, Ege University, Bornova, Izmir, Turkey
Disclaimer: All claims expressed in this article are solely those of the authors and do not necessarily represent those of their affiliated organizations, or those of the publisher, the editors and the reviewers. Any product that may be evaluated in this article or claim that may be made by its manufacturer is not guaranteed or endorsed by the publisher.
Research integrity at Frontiers
Learn more about the work of our research integrity team to safeguard the quality of each article we publish.