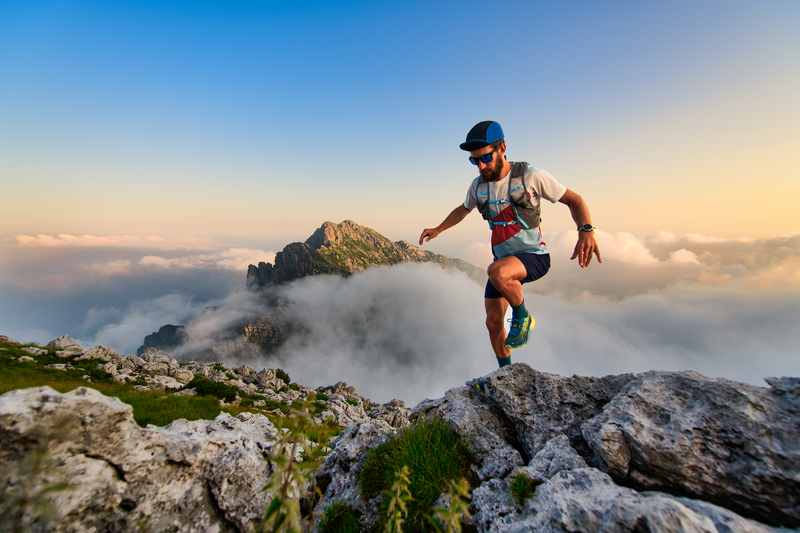
94% of researchers rate our articles as excellent or good
Learn more about the work of our research integrity team to safeguard the quality of each article we publish.
Find out more
PERSPECTIVE article
Front. Oncol. , 21 April 2022
Sec. Breast Cancer
Volume 12 - 2022 | https://doi.org/10.3389/fonc.2022.877384
This article is part of the Research Topic Cancer Stem Cells as Attractive Targets for Breast Cancer Therapy View all 5 articles
There is substantial evidence to suggest that complete tumor eradication relies on the effective elimination of cancer stem cells (CSCs). CSCs have been widely described as mediators of resistance to conventional therapies, including chemo- and radiotherapy, as well as of tumor metastasization and relapse in different tumor types, including breast cancer. However, the resistant phenotype of CSCs makes their targeting a tough task, and immunotherapy may therefore be an interesting option. Nevertheless, although immunotherapeutic approaches to cancer treatment have generated great enthusiasm due to recent success in clinics, breast cancer treatment mostly relies on standard approaches. In this context, we review the existing literature on the immunological properties of breast CSC and immunotherapeutic approaches to them. We will thus attempt to clarify whether there is room for the immunotargeting of breast CSCs in the current landscape of breast cancer therapies. Finally, we will provide our opinion on the CSC-targeting immunotherapeutic strategies that could prospectively be attempted.
Breast cancer (BC) is the most common cancer in women (1), and is still the first cause of cancer-associated mortality in women in several countries due to the development of therapy resistance and subsequent local or distant relapses (2). High inter-tumor and intra-tumor heterogeneity, leading to cell populations with different sensitivities to therapy (3), is a major challenge in BC treatment. The heterogeneity observed during BC progression can be attributed to both genetic and environmental factors, and the presence of Cancer Stem Cells (CSCs) (4, 5). CSCs are a small population of cancer cells with stem-like features that can be functionally defined by their capability to self-renew, differentiate, give rise to all the cell lineages that compose the tumor bulk and seed new tumors (6, 7). CSCs are endowed with plasticity and can generate cells with different functional, phenotypic and metabolic features, inducing tumor heterogeneity. The breast CSC (BCSC) niche contains various non-malignant cells that communicate with CSCs through direct contact and soluble factors to promote self-renewal, radio- and chemotherapy resistance and metastasis generation (8–10). BCSCs were identified (11) as a rare population of cancer cells of a CD44+CD24−/lowLin− phenotype that possessed higher tumor initiation capacity than BC cells of a different phenotype. Although the presence of BCSCs has since been demonstrated by several research groups, many biological features, including their phenotypic markers and ontology, are still controversial (12). CSCs can originate from a normal adult stem or progenitor cell undergoing neoplastic transformation, non-stem cells that gain self-renewal capabilities upon transformation, and from the de-differentiation of cancer cells without further genomic alterations (13–16). BCSCs are plastic and dynamic and can acquire different features following interactions with the tumor microenvironment and the BCSC niche. Two distinct states of BCSCs have been identified: mesenchymal-like BCSCs, characterized by the CD44+CD24−/low phenotype; and epithelial-like BCSCs, which express high levels of the aldehyde dehydrogenase (ALDH)1 enzyme (17). Mesenchymal BCSCs are quiescent, highly migratory, localized in hypoxic areas and at the invasive tumor front, and principally responsible for metastatic dissemination. Conversely, epithelial BCSCs are located in the inner tumor region and are highly proliferative and chemoresistant (18). These two phenotypes can dynamically convert one into the other.
The presence of high CSC levels in a tumor is associated with poor prognosis (19), and CSCs can induce cancer relapse (20, 21), as demonstrated by an enrichment in the CSC-related transcriptional signature observed in the tumor tissue remaining after endocrine therapy and chemotherapy in BC patients (22). Therefore, CSCs are an ideal therapeutic target as their eradication would eliminate the source of tumor progression, heterogeneity and relapse. However, BCSCs activate molecular pathways that render them resistant to current therapies, such as the increased functionality of DNA-repair mechanisms, the overexpression of detoxifying enzymes and efflux pumps, enhanced anti-oxidant capabilities and resistance to apoptosis and other cell-death forms (4, 23). Overall, these features make BCSCs resistant to conventional anti-cancer treatments. The emergence of immunotherapy as a valuable anti-cancer strategy potentially makes it an effective approach to targeting BCSCs, although many open questions remain (15). We herein summarize current knowledge on the interplay between BCSCs and the immune system, review the immunotargeting approaches developed for BCSCs in preclinical and clinical settings (listed in Table 1), and discuss the influence that the presence of BCSCs may exert on the immunotherapy outcome.
It has been reported that BCSCs employ several mechanisms to evade the immune response. Firstly, they possess defective antigen-presentation machinery, which is the result of the downregulation of major histocompatibility complex class I (MHC-I) molecules and of molecules involved in the peptide-loading process. This protects them from recognition by CD8+ T cells (25). Nevertheless, the absence of MHC-I may render BCSCs a target for Natural Killer (NK) cells (25). Indeed, the upregulation of NK ligands means that NK cells preferentially kill BCSCs, whether CD44+/CD24- (26) or ALDH1bright (27), compared to differentiated cells. Moreover, radiotherapy increases the expression of stress ligands on surviving CSCs, causing increased susceptibility to NK killing (28). However, others have reported BCSCs having reduced susceptibility to NK-mediated killing, due to the downregulation of NK-activating NKG2D ligands, meaning they selectively escape from NK-mediated killing and trastuzumab-induced antibody-dependent-cell-cytotoxicity (ADCC) (29–31). Moreover, BCSCs overexpress many immunosuppressive molecules, including several immune checkpoint (IC) ligands that weaken the activity of NK and T cells. Indeed, BCSCs express high levels of Programmed death-ligand (PD-L)1 and sometimes PD-L2 (32, 33), which, besides inhibiting NK and effector T-cell function by binding to PD1, also directly promote stemness by inducing the AKT-dependent expression of NANOG, Octamer-binding transcription factor (OCT)-4A and B lymphoma Mo-MLV insertion region 1 homolog (BMI1) (34). BCSCs also overexpress CD276 and CD155, two IC ligands that can inhibit T and NK cell killing (35), and CD200 (36), which induces a switch from an antitumor T helper (Th)1 to an immunosuppressive Th2 phenotype (37). Similarly, CD47 is overexpressed on BCSCs and, besides inhibiting their phagocytosis by macrophages via binding to the signal regulatory protein alpha (SIRPα) receptor and acting as a “Don’t eat me” signal, it directly protects CSCs from apoptosis (38).
Additionally, CSCs secrete immunosuppressive cytokines and other molecules that convert immune cells into tumor allies (4, 39), such as transforming growth factor (TGF)-β, interleukin (IL)-6, IL-8 and vascular endothelial growth factor (VEGF) (40, 41). We have previously demonstrated that BCSCs produce these cytokines as a consequence of an autocrine loop mediated by the overexpression of Toll-like receptor 2 and its engagement by high-mobility group box (HMGB)1 (42, 43). TGF-β plays a complex role in BC progression as it induces epithelial-to-mesenchymal-transition (EMT), and thus acts as a source of stem-like cells and promotes metastases. Along with VEGF, it promotes angiogenesis and induces immunosuppression by favoring T regulatory cells (Tregs) and myeloid-derived suppressor cell (MDSC) infiltration into tumors (44). IL-6 promotes the production of immunosuppressive cytokines IL-10 and IL-21 by Tregs, potentiating their activity (45), and reduces the expression of MHC-II and costimulatory molecules on dendritic cells (DC), thus impairing their ability to activate anti-cancer T cells (46). Whether secreted by tumor or immune cells, IL-6 further sustains BC’s stem-like qualities and progression by stimulating the Signal Transducer and Activator of Transcription (STAT)3-dependent expression of genes involved in stemness, tumorigenesis, migration and metastases (44, 47, 48). The inhibition of MDSC-derived IL-6 extends survival in BC mouse models (49). IL-8 is another cytokine that increases CSC frequency, and its receptor chemokine C-X-C motif receptor (CXCR)1 is more highly expressed in ALDH1+ than in ALDH1– BC cells (50). Furthermore, chemotherapy-induced cytotoxicity may increase local IL-8 levels, thus boosting CSC proportions. Indeed, high levels of IL-6 and IL-8 in advanced BC patient sera have been correlated with metastasis development and low therapeutic efficacy (51).
DC vaccines are common experimental vaccines in BC treatment, despite minimal evidence of clinical activity as a single agent (52). Preclinical studies have demonstrated that DC, pulsed with human BCSC-lysates, prolonged the survival of tumor-bearing mice with humanized immune systems, although no comparison with non-CSC lysates was performed (53). While DC-based vaccines specifically directed against BCSCs are under evaluation in clinics, trial outcome is currently unknown (NCT01782274, NCT02063893).
Besides DC-based vaccines, other vaccine formulations and antigen sources have been exploited to target BCSCs in preclinical studies. Vaccines consisting of irradiated induced pluripotent stem cells (iPSC) resulted in reductions in tumor growth due to the shared antigens in iPSCs and CSCs (54, 55). Our group has tested several vaccine platforms (DNA, oncolytic virus, virus-like particles) to target the CSC-antigen xCT (56–60). These, together with DNA vaccines that target Cripto-1 (61) and peptide-based vaccines targeting Hypoxia-Inducible Factor (HIF)-1α (62), have all demonstrated their efficacy against BCSCs. One DNA-vector-based vaccine, designed to target defined BCSC antigens (CD105/Yb-1/SOX2/CDH3/MDM2), is currently on trial (NCT02157051).
Besides vaccines, CSC-primed T cells and chimeric antigen receptor (CAR)-T cells have been developed to generate CSC-targeted immune responses. CSC-primed T cells are generated by stimulating donor CD8+ T cells in vitro with autologous DC pulsed with CSC-derived peptides, and are then infused back into the host. For instance, the in-vitro stimulation of human CD8+ T cells with autologous DC, pulsed with an ALDH1 peptide, triggered ALDH1-specific CD8+ T cells that restrained BC metastasis and improved survival when transferred into tumor-bearing immunodeficient mice (63).
However, the frequent downregulation of MHC-I molecules and antigen presentation in CSCs (37) can negatively affect the perspective use of T-cell adoptive transfer against CSCs. This hurdle can be overcome by CAR-T cells. These demonstrated activity in patients, leading to the FDA approval of CAR-T cells targeting CD19 in non-Hodgkin lymphomas and pediatric acute lymphoblastic leukemia (64). In preclinical models of BC, CAR-T cells targeting the BCSC-associated antigen GD2 efficiently impaired tumor growth and prevented metastasis formation, despite GD2 only being expressed on a small minority of cells (65). Similar observations were made in CAR-T that target the CSC-associated antigen Tumor endothelial marker 8 (66). CAR-T cells that target BCSC markers, in particular Human Epidermal Growth Factor Receptor (HER)2, GD2 and CD44v6 (NCT04430595), CD44v6 (NCT04427449), Epithelial Cell Adhesion Molecule (EpCam, NCT02915445) and CD133 (NCT02541370), are currently undergoing clinical studies involving BC patients.
The administration of γδ T lymphocytes, which are non-MHC-restricted and bear an invariant γδ TCR, is another approach that can bypass MHC-I downregulation. Clinical testing in BC patients revealed that the adoptive transfer of γδ T cells is safe and feasible, although proof of efficacy is lacking (67). Moving on, the innate effector properties of NK cells make them suitable candidates for immunotherapy. However, the controversial susceptibility of CSCs to NK cell killing, discussed in Section 2, means that there is no consensus on the efficacy of using NK to target BCSCs. Clinical trials using NK cell infusion to treat advanced solid tumors, including BC (NCT03319459), have been completed, although no results have yet been reported.
Monoclonal antibodies (mAb), including mAbs with direct inhibitory effects and the capacity to elicit ADCC in cancer cells, mAb-drug conjugates that deliver cytotoxic agents and mAb that target ICs, have proven to be a feasible strategy for cancer treatment.
Antibody-based therapies targeting CSC-maintaining pathways, such as the Wingless-related integration site (Wnt) pathway, have reached clinics. Vantictumab is a human mAb that suppresses the induction of the canonical Wnt signaling pathway via binding to the frizzled (Fzd) 1, 2, 5, 7 and 8 receptors. This agent reduced the frequency of CSCs and tumor growth in patient-derived xenografts (PDX) models of various tumor types, including BC, and exhibited synergistic activity with standard-of-care chemotherapy (68). In a phase Ib study of vantictumab with paclitaxel involving patients with metastatic HER2- BC, 33% of patients had a partial response and 29% had stable disease (69). Cirmtuzumab is a mAb that targets Receptor-tyrosine-kinase-like-orphan-receptor1, a receptor for Wnt5 found on BCSCs. Treating BC PDX mice with cirmtuzumab repressed the expression of genes associated with stemness and impaired cancer cell capacity to metastasize and regraft. Combination with paclitaxel further improved single-agent efficacy (70). A phase I study of cirmtuzumab plus paclitaxel in BC patients is ongoing (NCT02776917). mAbs against the CSC marker CD44 have been tested in preclinical models of BC (71), and the mAb-drug conjugate bivatuzumab mertansine, targeting CD44v6, has been tested in BC patients (NCT02254005, NCT02254031). However, no recent news is available on the development of this agent.
mAbs that target ICs, so-called IC inhibitors (ICIs), are currently used to treat different cancer types, alone and in combination with other treatments. The anti-PD-L1 atezolizumab has been approved for use in combination with nab-paclitaxel to treat metastatic Triple Negative Breast Cancer (TNBC) (72). As the expression levels of PD-L1 are higher in BCSCs than in non-CSCs (73–75), and as it has a role in promoting both their immune-evading phenotype and stemness (34), targeting PD-L1 may decrease the BCSC pool and improve the efficacy of combined treatments. For example, anti-PD-L1 treatment, in combination with an anti-ALDH1 vaccine, exerted potent antitumor efficacy and prolonged survival in multiple cancer murine models, including BC (76). Anti-PD-L1 treatment might also potentiate adoptive cell therapy. Indeed, increased levels of PD-L1 in CSCs render the γδ T-cells hypo-responsive. CSC sensitivity to γδ-mediated killing can thus be restored via PD-1 blockade (77, 78).
Another promising approach for targeting CSCs is to enhance phagocytosis using anti-CD47 mAbs (38). The anti-CD47 antibody Hu5F9-G4 displayed a manageable safety profile in a phase I trial on patients suffering from advanced solid tumors, including BC (79). To avoid the phagocytosis of normal cells, as CD47 is ubiquitous, an HER2 mAb was fused with SIRPα to generate a bispecific mAb-Trap antibody-receptor fusion protein that will be tested in patients with HER2-expressing advanced solid tumors (NCT05076591).
mAbs can be used to revert the immunosuppressive tumor microenvironment and hinder CSC self-renewal by targeting cytokines such as IL-6 and IL-8 (48, 80, 81). Tocilizumab, a mAb that targets the IL-6 receptor, suppresses the stem-like properties of BCSCs and their metastatic ability, potentiating the cytotoxicity of chemotherapy in TNBC (82). The FDA has approved this drug for rheumatoid arthritis and it is now under study for BC treatment (NCT03135171, NCT03424005).
Besides mAb, small molecules have also been used to target the CSC/immune-microenvironment interaction and have reached the clinic; for example, ruxolitinib inhibits the IL-6/JAK/STAT pathway (NCT02066532, NCT02041429, NCT02876302, NCT02120417, NCT01562873, NCT01594216, NCT03012230) with evidence of clinical activity (24), while reparixin targets the IL-8 receptor CXCR1, selectively depletes CSCs in vitro and reduces tumor growth and metastasization in vivo (81). Reparixin has moved to clinical trials (83), giving promising results in terms of safety (84) and efficacy in reducing BCSC frequency (85, 86). Other trials involving reparixin to target CSCs have been recently completed (NCT02370238, NCT02001974, NCT01861054).
Although many immunological approaches for targeting CSCs have given promising results in clinical trials, no CSC-targeting immunotherapy has yet been approved. Moreover, the CSC concept has rarely been implemented in clinical practice, possibly because of the elusive phenotype and exiguous number of CSCs.
The CSC theory is often seen as opposing the clonal evolution theory (87), which is still the mainstream explanation for observed intra-tumor heterogeneity. There may therefore be less interest in assessing the clinical efficacy of CSC-targeted therapies than in those targeting altered pathways and molecules expressed by the tumor bulk. However, the high expression of putative CSC markers is consistently associated with poorer overall survival in BC patients (88), and the presence of residual ALDH1+ cells after neoadjuvant therapy is associated with increased metastasization risk (89), indicating that targeting CSCs would have a beneficial impact on patient life expectancy. Nevertheless, evaluating the efficacy of any therapy on the CSC subset is difficult as they are only a minor fraction of cells and are hard to detect with routine tumor-biopsy methods, i.e., IHC, IF, FISH. Currently, no standardized approaches to assess and quantify CSCs within tumor biopsies exist. Moreover, commonly employed clinical endpoints, such as tumor shrinkage, would not reveal any therapeutic effect on CSCs, which may remain as a quiescent residual population before reactivation and relapse.
The lack of a unique marker associated to stemness that can be exploited for CSC detection is another hurdle. Indeed, CSC markers may vary according to the mammary cancer subtype, and different BCSC populations, with different properties (and markers), may even exist within the same tumor. As mentioned, mesenchymal-like and epithelial-like BCSCs co-exist in different proportions according to BC subtype (89). Furthermore, BCSC phenotype is not stable, and different BCSC populations can switch after endogenous (i.e., microenvironmental) and exogenous (i.e., therapeutic) pressure. Similarly, a CSC can differentiate towards a bulk cell, which can conversely de-differentiate to CSC status. CSCs are therefore a moving target, and difficulties in their ontology consequently bring difficulties in their treatment.
The switch between one CSC phenotype and another is accompanied by a switch in associated markers and, thus, targetable antigens. Effective therapy should therefore not rely on one target antigen, but include a set of CSC-associated antigens. However, not all the antigens for the many CSC phenotypes are known, thus limiting the window of intervention for antigen-targeted strategies. CSC-derived lysates have therefore been efficiently used as an (unselected) antigen source for DC loading, in preclinical studies involving squamous cell carcinoma and melanoma (90). CSC-loaded DCs were more potent in inducing an anti-tumor immune response than unselected cancer cells, suggesting that CSCs express a different and underrepresented set of antigens, and that therapeutic efficacy can only be achieved by eliminating CSCs. This proof-of-concept is extremely relevant in the context of the reported difficulty in treating BC with immunotherapy. BC carries a lower load of mutations, and thus of neoantigens, than melanoma and lung cancer. This has a negative impact on the generation of spontaneous anti-tumor T-cell responses and has been associated to the poor efficacy of ICIs in BC (91). Nevertheless, preclinical proof that BCSC-associated antigens represent an activatable vulnerability in BC treatment may pave the way for the use of vaccine platforms in which BCSC-antigen-specific T-cell responses can be triggered, thus improving ICI efficacy.
A further complication is the ability of CSCs to evade immune system recognition, which raises doubt in the applicability of some immunotherapeutic approaches to CSC targeting. Moreover, CSCs contribute to the induction of an immunosuppressive microenvironment, which is a reported cause of immunotherapy’s low efficacy in BC (91). This highlights the importance of using agents that block such immunosuppressive pathways (Figure 1).
Figure 1 Immunotherapies targeting CSCs and CSC immunoescaping mechanism. Schematic representation of the main immunoevasive mechanisms adopted by BCSCs and how these can be addressed using different immunotherapeutic strategies.
It is clear that mono(immune)therapy is not an ideal solution for BCSCs. ICIs and microenvironment-modulating agents provide the opportunity to revert the immunoevasive properties of BCSCs, while vaccine formulations that are not restrained to known BCSC antigens, such as using peptides or lysates from BCSC-enriched populations to pulse autologous DC, would allow an immune response to be mounted against all BCSC subsets. Other interesting approaches include targeting membrane molecules with a role in BCSC function, the so called “oncoantigens” (92): among others, CD44, xCT, Chondroitin Sulfate Proteoglycan 4 and Cripto1 (58, 61, 71, 93) that, when inhibited, would compromise the stem-like properties of cancer cells, making them sensitive to standard therapies. This, in combination with ICIs and bulk-shrinking therapies, would be more effective than single approaches in preventing phenotype switch, de-differentiation and eventually relapse.
The original contributions presented in the study are included in the article/supplementary material. Further inquiries can be directed to the corresponding author. The clinical trials described in this review can be found on the website ClinicalTrials.gov (https://clinicaltrials.gov/).
RR and LC wrote the first draft of the manuscript, FC critically revised its content and form, ADL performed the clinical trial search and produced tables and figures. All authors contributed to manuscript revision, read, and approved the submitted version
This review article was supported by grants from the Italian Association for Cancer Research to FC (grant number IG 21468) and to LC (grant number IG 25766), from the University of Turin and from the Fondazione Ricerca Molinette. RR was supported by Fondazione Umberto Veronesi.
The authors declare that the article was written in the absence of any commercial or financial relationships that could be construed as a potential conflict of interest.
All claims expressed in this article are solely those of the authors and do not necessarily represent those of their affiliated organizations, or those of the publisher, the editors and the reviewers. Any product that may be evaluated in this article, or claim that may be made by its manufacturer, is not guaranteed or endorsed by the publisher.
We thank Dale Lawson for the manuscript English language editing. Figures were created with BioRender.com.
1. Sung H, Ferlay J, Siegel RL, Laversanne M, Soerjomataram I, Jemal A, et al. Global Cancer Statistics 2020: GLOBOCAN Estimates of Incidence and Mortality Worldwide for 36 Cancers in 185 Countries. CA Cancer J Clin (2021) 71:209–49. doi: 10.3322/caac.21660
2. Ayala de la Pena F, Andres R, Garcia-Saenz JA, Manso L, Margeli M, Dalmau E, et al. SEOM Clinical Guidelines in Early Stage Breast Cancer (2018). Clin Transl Oncol (2019) 21:18–30. doi: 10.1007/s12094-018-1973-6
3. Dagogo-Jack I, Shaw AT. Tumour Heterogeneity and Resistance to Cancer Therapies. Nat Rev Clin Oncol (2018) 15:81–94. doi: 10.1038/nrclinonc.2017.166
4. Ruiu R, Tarone L, Rolih V, Barutello G, Bolli E, Riccardo F, et al. Cancer Stem Cell Immunology and Immunotherapy: Harnessing the Immune System Against Cancer’s Source. Prog Mol Biol Transl Sci (2019) 164:119–88. doi: 10.1016/bs.pmbts.2019.03.008
5. Karaayvaz M, Cristea S, Gillespie SM, Patel AP, Mylvaganam R, Luo CC, et al. Unravelling Subclonal Heterogeneity and Aggressive Disease States in TNBC Through Single-Cell RNA-Seq. Nat Commun (2018) 9:3588. doi: 10.1038/s41467-018-06052-0
6. Owens TW, Naylor MJ. Breast Cancer Stem Cells. Front Physiol (2013) 4:225. doi: 10.3389/fphys.2013.00225
7. Clarke MF, Dick JE, Dirks PB, Eaves CJ, Jamieson CHM, Jones DL, et al. Cancer Stem Cells - Perspectives on Current Status and Future Directions: AACR Workshop on Cancer Stem Cells. Cancer Res (2006) 66:9339–44. doi: 10.1158/0008-5472.CAN-06-3126
8. Conti L, Ruiu R, Barutello G, Macagno M, Bandini S, Cavallo F, et al. Microenvironment, Oncoantigens, and Antitumor Vaccination: Lessons Learned From BALB-neuT Mice. BioMed Res Int (2014) 2014:534969. doi: 10.1155/2014/534969
9. Badve S, Nakshatri H. Breast-Cancer Stem Cells-Beyond Semantics. Lancet Oncol (2012) 13:e43–8. doi: 10.1016/S1470-2045(11)70191-7
10. De Angelis ML, Francescangeli F, Zeuner A. Breast Cancer Stem Cells as Drivers of Tumor Chemoresistance, Dormancy and Relapse: New Challenges and Therapeutic Opportunities. Cancers (Basel) (2019) 11:1569. doi: 10.3390/cancers11101569
11. Al-Hajj M, Wicha MS, Benito-Hernandez A, Morrison SJ, Clarke MF. Prospective Identification of Tumorigenic Breast Cancer Cells. PNAS (2003) 100:3983–8. doi: 10.1073/pnas.0530291100
12. Wang T, Shigdar S, Gantier MP, Hou Y, Wang L, Li Y, et al. Cancer Stem Cell Targeted Therapy: Progress Amid Controversies. Oncotarget (2015) 6:44191–206. doi: 10.18632/oncotarget.6176
13. Pece S, Tosoni D, Confalonieri S, Mazzarol G, Vecchi M, Ronzoni S, et al. Biological and Molecular Heterogeneity of Breast Cancers Correlates With Their Cancer Stem Cell Content. Cell (2010) 140:62–73. doi: 10.1016/j.cell.2009.12.007
14. Lloyd-Lewis B, Harris OB, Watson CJ, Davis FM. Mammary Stem Cells: Premise, Properties, and Perspectives. Trends Cell Biol (2017) 27:556–67. doi: 10.1016/j.tcb.2017.04.001
15. Quaglino E, Conti L, Cavallo F. Breast Cancer Stem Cell Antigens as Targets for Immunotherapy. Semin Immunol (2020) 47:101386. doi: 10.1016/j.smim.2020.101386
16. Bai X, Ni J, Beretov J, Graham P, Li Y. Cancer Stem Cell in Breast Cancer Therapeutic Resistance. Cancer Treat Rev (2018) 69:152–63. doi: 10.1016/j.ctrv.2018.07.004
17. Brooks MD, Wicha MS. Tumor Twitter: Cellular Communication in the Breast Cancer Stem Cell Niche. Cancer Discov (2015) 5:469–71. doi: 10.1158/2159-8290.CD-15-0327
18. Brooks MD, Burness ML, Wicha MS. Therapeutic Implications of Cellular Heterogeneity and Plasticity in Breast Cancer. Cell Stem Cell (2015) 17:260–71. doi: 10.1016/j.stem.2015.08.014
19. Pece S, Disalvatore D, Tosoni D, Vecchi M, Confalonieri S, Bertalot G, et al. Identification and Clinical Validation of a Multigene Assay That Interrogates the Biology of Cancer Stem Cells and Predicts Metastasis in Breast Cancer: A Retrospective Consecutive Study. EBioMedicine (2019) 42:352–62. doi: 10.1016/j.ebiom.2019.02.036
20. Cojoc M, Mäbert K, Muders MH, Dubrovska A. A Role for Cancer Stem Cells in Therapy Resistance: Cellular and Molecular Mechanisms. Semin Cancer Biol (2015) 31:16–27. doi: 10.1016/j.semcancer.2014.06.004
21. Quaglino E, Cavallo F, Conti L. Cancer Stem Cell Antigens as Targets for New Combined Anti-Cancer Therapies. Int J Biochem Cell Biol (2020) 129:105861. doi: 10.1016/j.biocel.2020.105861
22. Creighton CJ, Li X, Landis M, Dixon JM, Neumeister VM, Sjolund A, et al. Residual Breast Cancers After Conventional Therapy Display Mesenchymal as Well as Tumor-Initiating Features. Proc Natl Acad Sci (2009) 106:13820–5. doi: 10.1073/pnas.0905718106
23. Magri J, Gasparetto A, Conti L, Calautti E, Cossu C, Ruiu R, et al. Tumor-Associated Antigen xCT and Mutant-P53 as Molecular Targets for New Combinatorial Antitumor Strategies. Cells (2021) 10:108. doi: 10.3390/cells10010108
24. Lynce F, Williams JT, Regan MM, Bunnell CA, Freedman RA, Tolaney SM, et al. Phase I Study of JAK1/2 Inhibitor Ruxolitinib With Weekly Paclitaxel for the Treatment of HER2-Negative Metastatic Breast Cancer. Cancer Chemother Pharmacol (2021) 87:673–9. doi: 10.1007/s00280-021-04245-x
25. Tallerico R, Conti L, Lanzardo S, Sottile R, Wagner AK, Johansson MH, et al. NK Cells Control Breast Cancer and Related Cancer Stem Cell Hematological Spread. Oncoimmunology (2017) 6:1–11. doi: 10.1080/2162402X.2017.1284718
26. Yin T, Wang G, He S, Liu Q, Sun J, Wang Y. Human Cancer Cells With Stem Cell-Like Phenotype Exhibit Enhanced Sensitivity to the Cytotoxicity of IL-2 and IL-15 Activated Natural Killer Cells. Cell Immunol (2016) 300:41–5. doi: 10.1016/j.cellimm.2015.11.009
27. Ames E, Canter RJ, Grossenbacher SK, Mac S, Chen M, Smith RC, et al. NK Cells Preferentially Target Tumor Cells With a Cancer Stem Cell Phenotype. J Immunol (2015) 195:4010–9. doi: 10.4049/jimmunol.1500447
28. Ames E, Canter RJ, Grossenbacher SK, Mac S, Smith RC, Monjazeb AM, et al. Enhanced Targeting of Stem-Like Solid Tumor Cells With Radiation and Natural Killer Cells. Oncoimmunology (2015) 4:e1036212. doi: 10.1080/2162402X.2015.1036212
29. Reim F, Dombrowski Y, Ritter C, Buttmann M, Hausler S, Ossadnik M, et al. Immunoselection of Breast and Ovarian Cancer Cells With Trastuzumab and Natural Killer Cells: Selective Escape of CD44high/CD24low/HER2low Breast Cancer Stem Cells. Cancer Res (2009) 69:8058–66. doi: 10.1158/0008-5472.CAN-09-0834
30. Wang B, Wang Q, Wang Z, Jiang J, Yu SC, Ping YF, et al. Metastatic Consequences of Immune Escape From NK Cell Cytotoxicity by Human Breast Cancer Stem Cells. Cancer Res (2014) 74:5746–57. doi: 10.1158/0008-5472.CAN-13-2563
31. Jin H, Kim HJ. NK Cells Lose Their Cytotoxicity Function Against Cancer Stem Cell-Rich Radiotherapy-Resistant Breast Cancer Cell Populations. Int J Mol Sci (2021) 22:9639. doi: 10.3390/ijms22179639
32. Mediratta K, El-Sahli S, D’Costa V, Wang L. Current Progresses and Challenges of Immunotherapy in Triple-Negative Breast Cancer. Cancers (Basel) (2020) 12:3529. doi: 10.3390/cancers12123529
33. Castagnoli L, Cancila V, Cordoba-Romero SL, Faraci S, Talarico G, Belmonte B, et al. WNT Signaling Modulates PD-L1 Expression in the Stem Cell Compartment of Triple-Negative Breast Cancer. Oncogene (2019) 38:4047. doi: 10.1038/s41388-019-0700-2
34. Almozyan S, Colak D, Mansour F, Alaiya A, Al-Harazi O, Qattan A, et al. PD-L1 Promotes OCT4 and Nanog Expression in Breast Cancer Stem Cells by Sustaining PI3K/AKT Pathway Activation. Int J Cancer (2017) 141:1402–12. doi: 10.1002/ijc.30834
35. Miranda A, Hamilton PT, Zhang AW, Pattnaik S, Becht E, Mezheyeuski A, et al. Cancer Stemness, Intratumoral Heterogeneity, and Immune Response Across Cancers. Proc Natl Acad Sci USA (2019) 116:9020–9. doi: 10.1073/pnas.1818210116
36. Kawasaki BT, Mistree T, Hurt EM, Kalathur M, Farrar WL. Co-Expression of the Toleragenic Glycoprotein, CD200, With Markers for Cancer Stem Cells. Biochem Biophys Res Commun (2007) 364:778–82. doi: 10.1016/j.bbrc.2007.10.067
37. Kretz-Rommel A, Qin F, Dakappagari N, Ravey EP, McWhirter J, Oltean D, et al. CD200 Expression on Tumor Cells Suppresses Antitumor Immunity: New Approaches to Cancer Immunotherapy. J Immunol (2007) 178:5595–605. doi: 10.4049/jimmunol.178.9.5595
38. Zhang H, Lu H, Xiang L, Bullen JW, Zhang C, Samanta D, et al. HIF-1 Regulates CD47 Expression in Breast Cancer Cells to Promote Evasion of Phagocytosis and Maintenance of Cancer Stem Cells. Proc Natl Acad Sci USA (2015) 112:E6215–23. doi: 10.1073/pnas.1520032112
39. Galassi C, Musella M, Manduca N, Maccafeo E, Sistigu A. The Immune Privilege of Cancer Stem Cells: A Key to Understanding Tumor Immune Escape and Therapy Failure. Cells (2021) 10:2361. doi: 10.3390/cells10092361
40. Korkaya H, Liu S, Wicha MS. Regulation of Cancer Stem Cells by Cytokine Networks : Attacking Cancer’s Inflammatory Roots. Clin Cancer Res (2011) 17:6125–30. doi: 10.1158/1078-0432.CCR-10-2743
41. Hartman ZC, Poage GM, Den Hollander P, Tsimelzon A, Hill J, Panupinthu N, et al. Growth of Triple-Negative Breast Cancer Cells Relies Upon Coordinate Autocrine Expression of the Proinflammatory Cytokines IL-6 and IL-8. Cancer Res (2013) 73:3470–80. doi: 10.1158/0008-5472.CAN-12-4524-T
42. Di Lorenzo A, Bolli E, Tarone L, Cavallo F, Conti L. Toll-Like Receptor 2 at the Crossroad Between Cancer Cells, the Immune System, and the Microbiota. Int J Mol Sci (2020) 21:9418. doi: 10.3390/ijms21249418
43. Conti L, Lanzardo S, Arigoni M, Antonazzo R, Radaelli E, Cantarella D, et al. The Noninflammatory Role of High Mobility Group Box 1/Toll-Like Receptor 2 Axis in the Self-Renewal of Mammary Cancer Stem Cells. FASEB J (2013) 27:4731–44. doi: 10.1096/fj.13-230201
44. Salemme V, Centonze G, Cavallo F, Defilippi P, Conti L. The Crosstalk Between Tumor Cells and the Immune Microenvironment in Breast Cancer: Implications for Immunotherapy. Front Oncol (2021) 11:610303. doi: 10.3389/fonc.2021.610303
45. Chen YQ, Li PC, Pan N, Gao R, Wen ZF, Zhang TY, et al. Tumor-Released Autophagosomes Induces CD4(+) T Cell-Mediated Immunosuppression via a TLR2-IL-6 Cascade. J Immunother Cancer (2019) 7:178. doi: 10.1186/s40425-019-0646-5
46. Tang M, Diao J, Gu H, Khatri I, Zhao J, Cattral MS. Toll-Like Receptor 2 Activation Promotes Tumor Dendritic Cell Dysfunction by Regulating IL-6 and IL-10 Receptor Signaling. Cell Rep (2015) 13:2851–64. doi: 10.1016/j.celrep.2015.11.053
47. Sansone P, Storci G, Tavolari S, Guarnieri T, Giovannini C, Taffurelli M, et al. IL-6 Triggers Malignant Features in Mammospheres From Human Ductal Breast Carcinoma and Normal Mammary Gland. J Clin Invest (2007) 117:3988–4002. doi: 10.1172/JCI32533
48. Kim SY, Kang JW, Song X, Kim BK, Yoo YD, Kwon YT, et al. Role of the IL-6-JAK1-STAT3-Oct-4 Pathway in the Conversion of Non-Stem Cancer Cells Into Cancer Stem-Like Cells. Cell Signal (2013) 25:961–9. doi: 10.1016/j.cellsig.2013.01.007
49. Peng D, Tanikawa T, Li W, Zhao L, Vatan L, Szeliga W, et al. Myeloid-Derived Suppressor Cells Endow Stem-Like Qualities to Breast Cancer Cells Through IL6/STAT3 and NO/NOTCH Cross-Talk Signaling. Cancer Res (2016) 76:3156–65. doi: 10.1158/0008-5472.CAN-15-2528
50. Charafe-Jauffret E, Ginestier C, Iovino F, Wicinski J, Cervera N, Finetti P, et al. Breast Cancer Cell Lines Contain Functional Cancer Stem Sells With Metastatic Capacity and a Distinct Molecular Signature. Cancer Res (2009) 69:1302–13. doi: 10.1158/0008-5472.CAN-08-2741
51. Liu S, Wicha MS. Targeting Breast Cancer Stem Cells. J Clin Oncol (2010) 28:4006. doi: 10.1200/JCO.2009.27.5388
52. Adams S, Gatti-Mays ME, Kalinsky K, Korde LA, Sharon E, Amiri-Kordestani L, et al. Current Landscape of Immunotherapy in Breast Cancer: A Review. JAMA Oncol (2019) 5:1205–14. doi: 10.1001/jamaoncol.2018.7147
53. Van Pham P, Le HT, Vu BT, Pham VQ, Le PM, Phan NLC, et al. Targeting Breast Cancer Stem Cells by Dendritic Cell Vaccination in Humanized Mice With Breast Tumor: Preliminary Results. Onco Targets Ther (2016) 9:4441–51. doi: 10.2147/OTT.S105239
54. Kishi M, Asgarova A, Desterke C, Chaker D, Artus J, Turhan AG, et al. Evidence of Antitumor and Antimetastatic Potential of Induced Pluripotent Stem Cell-Based Vaccines in Cancer Immunotherapy. Front Med (2021) 0:2532. doi: 10.3389/fmed.2021.729018
55. Kooreman NG, Kim Y, de Almeida PE, Termglinchan V, Diecke S, Shao NY, et al. Autologous iPSC-Based Vaccines Elicit Anti-Tumor Responses. In Vivo Cell Stem Cell (2018) 22:501–13.e7. doi: 10.1016/j.stem.2018.01.016
56. Conti L, Bolli E, Di Lorenzo A, Franceschi V, Macchi F, Riccardo F, et al. Immunotargeting of the xCT Cystine/Glutamate Antiporter Potentiates the Efficacy of HER2-Targeted Immunotherapies in Breast Cancer. Cancer Immunol Res (2020) 8:1039–53. doi: 10.1158/2326-6066.CIR-20-0082
57. Donofrio G, Tebaldi G, Lanzardo S, Ruiu R, Bolli E, Ballatore A, et al. Bovine Herpesvirus 4-Based Vector Delivering the Full Length xCT DNA Efficiently Protects Mice From Mammary Cancer Metastases by Targeting Cancer Stem Cells. Oncoimmunology (2018) 00:1–16. doi: 10.1080/2162402X.2018.1494108
58. Ruiu R, Rolih V, Bolli E, Barutello G, Riccardo F, Quaglino E, et al. Fighting Breast Cancer Stem Cells Through the Immune-Targeting of the xCT Cystine–Glutamate Antiporter. Cancer Immunol Immunother (2019) 68:131–41. doi: 10.1007/s00262-018-2185-1
59. Lanzardo S, Conti L, Rooke R, Ruiu R, Accart N, Bolli E, et al. Immunotargeting of Antigen xCT Attenuates Stem-Like Cell Behavior and Metastatic Progression in Breast Cancer. Cancer Res (2016) 76:62–72. doi: 10.1158/0008-5472.CAN-15-1208
60. Bolli E, O’Rourke JP, Conti L, Lanzardo S, Rolih V, Christen JM, et al. A Virus-Like-Particle Immunotherapy Targeting Epitope-Specific anti-xCT Expressed on Cancer Stem Cell Inhibits the Progression of Metastatic Cancer In Vivo. Oncoimmunology (2018) 7:e1408746. doi: 10.1080/2162402X.2017.1408746
61. Witt K, Ligtenberg MA, Conti L, Lanzardo S, Ruiu R, Wallmann T, et al. Cripto-1 Plasmid DNA Vaccination Targets Metastasis and Cancer Stem Cells in Murine Mammary Carcinoma. Cancer Immunol Res (2018) 1:1417–25. doi: 10.1158/2326-6066.CIR-17-0572
62. Cecil DL, Slota M, O’Meara MM, Curtis BC, Gad E, Dang Y, et al. Immunization Against HIF-1α Inhibits the Growth of Basal Mammary Tumors and Targets Mammary Stem Cells In Vivo. Clin Cancer Res (2017) 23:3396–404. doi: 10.1158/1078-0432.CCR-16-1678
63. Visus C, Wang Y, Lozano-Leon A, Ferris RL, Silver S, Szczepanski MJ, et al. Targeting ALDH Bright Human Carcinoma-Initiating Cells With ALDH1A1-Specific CD8 + T Cells. Clin Cancer Res (2011) 17:6174–84. doi: 10.1158/1078-0432.CCR-11-1111.
64. Mullard A. FDA Approves Fourth CAR-T Cell Therapy. Nat Rev Drug Discov (2021) 20:166. doi: 10.1038/d41573-021-00031-9
65. Seitz CM, Schroeder S, Knopf P, Krahl AC, Hau J, Schleicher S, et al. GD2-Targeted Chimeric Antigen Receptor T Cells Prevent Metastasis Formation by Elimination of Breast Cancer Stem-Like Cells. Oncoimmunology (2020) 9:1683345. doi: 10.1080/2162402X.2019.1683345
66. Byrd TT, Fousek K, Pignata A, Szot C, Samaha H, Seaman S, et al. TEM8/ANTXR1-Specific CAR T Cells as a Targeted Therapy for Triple-Negative Breast Cancer. Cancer Res (2018) 78:489–500. doi: 10.1158/0008-5472.CAN-16-1911
67. Meraviglia S, Eberl M, Vermijlen D, Todaro M, Buccheri S, Cicero G, et al. In Vivo Manipulation of Vgamma9Vdelta2 T Cells With Zoledronate and Low-Dose Interleukin-2 for Immunotherapy of Advanced Breast Cancer Patients. Clin Exp Immunol (2010) 161:290–7. doi: 10.1111/j.1365-2249.2010.04167.x
68. Gurney A, Axelrod F, Bond CJ, Cain J, Chartier C, Donigan L, et al. Wnt Pathway Inhibition via the Targeting of Frizzled Receptors Results in Decreased Growth and Tumorigenicity of Human Tumors. Proc Natl Acad Sci USA (2012) 109:11717–22. doi: 10.1073/pnas.1120068109
69. Mita MM, Becerra C, Richards DA, Mita AC, Shagisultanova E, Osborne CRC, et al. Phase 1b Study of WNT Inhibitor Vantictumab (VAN, Human Monoclonal Antibody) With Paclitaxel (P) in Patients (Pts) With 1st- to 3rd-Line Metastatic HER2-Negative Breast Cancer (BC). J Clin Oncol (2016) 34:2516–6. doi: 10.1200/JCO.2016.34.15_suppl.2516
70. Zhang S, Zhang H, Ghia EM, Huang J, Wu L, Zhang J, et al. Inhibition of Chemotherapy Resistant Breast Cancer Stem Cells by a ROR1 Specific Antibody. Proc Natl Acad Sci USA (2019) 116:1370–7. doi: 10.1073/pnas.1816262116
71. Ghatak S, Misra S, Toole BP. Hyaluronan Oligosaccharides Inhibit Anchorage-Independent Growth of Tumor Cells by Suppressing the Phosphoinositide 3-Kinase/Akt Cell Survival Pathway. J Biol Chem (2002) 277:38013–20. doi: 10.1074/jbc.M202404200
72. Schmid P, Adams S, Rugo HS, Schneeweiss A, Barrios CH, Iwata H, et al. Atezolizumab and Nab-Paclitaxel in Advanced Triple-Negative Breast Cancer. N Engl J Med (2018) 379:2108–21. doi: 10.1056/NEJMoa1809615
73. Wu Y, Chen M, Wu P, Chen C, Xu ZP, Gu W. Increased PD-L1 Expression in Breast and Colon Cancer Stem Cells. Clin Exp Pharmacol Physiol (2017) 44:602–4. doi: 10.1111/1440-1681.12732
74. Darvin P, Sasidharan Nair V, Elkord E. PD-L1 Expression in Human Breast Cancer Stem Cells Is Epigenetically Regulated Through Posttranslational Histone Modifications. J Oncol (2019) 2019:3958908. doi: 10.1155/2019/3958908
75. Hsu JM, Xia W, Hsu YH, Chan LC, Yu WH, Cha JH, et al. STT3-Dependent PD-L1 Accumulation on Cancer Stem Cells Promotes Immune Evasion. Nat Commun (2018) 9:1908. doi: 10.1038/s41467-018-04313-6
76. Hassani Najafabadi A, Zhang J, Aikins ME, Najaf Abadi ZI, Liao F, Qin Y, et al. Cancer Immunotherapy via Targeting Cancer Stem Cells Using Vaccine Nanodiscs. Nano Lett (2020) 20:7783–92. doi: 10.1021/acs.nanolett.0c03414
77. Dutta I, Dieters-Castator D, Papatzimas JW, Medina A, Schueler J, Derksen DJ, et al. ADAM Protease Inhibition Overcomes Resistance of Breast Cancer Stem-Like Cells to γδ T Cell Immunotherapy. Cancer Lett (2021) 496:156–68. doi: 10.1016/j.canlet.2020.10.013
78. Chen HC, Joalland N, Bridgeman JS, Alchami FS, Jarry U, Khan MWA, et al. Synergistic Targeting of Breast Cancer Stem-Like Cells by Human γδ T Cells and CD8 + T Cells. Immunol Cell Biol (2017) 95:620–9. doi: 10.1038/icb.2017.21
79. Sikic BI, Lakhani N, Patnaik A, Shah SA, Chandana SR, Rasco D, et al. First-In-Human, First-In-Class Phase I Trial of the Anti-CD47 Antibody Hu5F9-G4 in Patients With Advanced Cancers. J Clin Oncol (2019) 37:946. doi: 10.1200/JCO.18.02018
80. Jia D, Li L, Andrew S, Allan D, Li X, Lee J, et al. An Autocrine Inflammatory Forward-Feedback Loop After Chemotherapy Withdrawal Facilitates the Repopulation of Drug-Resistant Breast Cancer Cells. Cell Death Dis (2017) 8:e2932. doi: 10.1038/cddis.2017.319
81. Ginestier C, Liu S, Diebel ME, Korkaya H, Luo M, Brown M, et al. CXCR1 Blockade Selectively Targets Human Breast Cancer Stem Cells In Vitro and in Xenografts. J Clin Invest (2010) 120:485–97. doi: 10.1172/JCI39397
82. Alraouji NN, Al-Mohanna FH, Ghebeh H, Arafah M, Almeer R, Al-Tweigeri T, et al. Tocilizumab Potentiates Cisplatin Cytotoxicity and Targets Cancer Stem Cells in Triple-Negative Breast Cancer. Mol Carcinog (2020) 59:1041–51. doi: 10.1002/mc.23234
83. Ruffini PA. The CXCL8-CXCR1/2 Axis as a Therapeutic Target in Breast Cancer Stem-Like Cells. Front Oncol (2019) 9:40. doi: 10.3389/fonc.2019.00040
84. Schott AF, Goldstein LJ, Cristofanilli M, Ruffini PA, McCanna S, Reuben JM, et al. Phase Ib Pilot Study to Evaluate Reparixin in Combination With Weekly Paclitaxel in Patients With HER-2–Negative Metastatic Breast Cancer. Clin Cancer Res (2017) 23:5358–65. doi: 10.1158/1078-0432.CCR-16-2748
85. Goldstein LJ, Perez RP, Yardley D, Han LK, Reuben JM, Gao H, et al. A Window-of-Opportunity Trial of the CXCR1/2 Inhibitor Reparixin in Operable HER-2-Negative Breast Cancer. Breast Cancer Res (2020) 22:4. doi: 10.1186/s13058-019-1243-8.
86. Goldstein LJ, Mansutti M, Levy C, Chang JC, Henry S, Fernandez-Perez I, et al. A Randomized, Placebo-Controlled Phase 2 Study of Paclitaxel in Combination With Reparixin Compared to Paclitaxel Alone as Front-Line Therapy for Metastatic Triple-Negative Breast Cancer (Frida). Breast Cancer Res Treat (2021) 190:265. doi: 10.1007/s10549-021-06367-5
87. Shackleton M, Quintana E, Fearon ER, Morrison SJ. Heterogeneity in Cancer: Cancer Stem Cells Versus Clonal Evolution. Cell (2009) 138:822–9. doi: 10.1016/j.cell.2009.08.017
88. Lathia J, Liu H, Matei D. The Clinical Impact of Cancer Stem Cells. Oncologist (2020) 25:123–31. doi: 10.1634/theoncologist.2019-0517
89. Brooks MD, Burness ML, Wicha MS. Therapeutic Implications of Cellular Heterogeneity and Plasticity in Breast Cancer. Cell Stem Cell (2015) 17:260–71. doi: 10.1016/j.stem.2015.08.014
90. Ning N, Pan Q, Zheng F, Teitz-tennenbaum S, Egenti M, Yet J, et al. Cancer Stem Cell Vaccination Confers Significant Antitumor Immunity. Cancer Res (2012) 72:1853–65. doi: 10.1158/0008-5472.CAN-11-1400
91. Vonderheide RH, Domchek SM, Clark AS. Immunotherapy for Breast Cancer: What Are We Missing? Clin Cancer Res (2017) 23:2640–6. doi: 10.1158/1078-0432.CCR-16-2569
92. Iezzi M, Quaglino E, Amici A, Lollini P-L, Forni G, Cavallo F. DNA Vaccination Against Oncoantigens: A Promise. Oncoimmunology (2012) 1:316–25. doi: 10.4161/onci.19127
Keywords: breast cancer, cancer stem cells, immunotherapy, CAR-T, vaccination, monoclonal antibody, clinical trial
Citation: Ruiu R, Di Lorenzo A, Cavallo F and Conti L (2022) Are Cancer Stem Cells a Suitable Target for Breast Cancer Immunotherapy? Front. Oncol. 12:877384. doi: 10.3389/fonc.2022.877384
Received: 16 February 2022; Accepted: 21 March 2022;
Published: 21 April 2022.
Edited by:
Martin Götte, University of Münster, GermanyReviewed by:
Giorgio Stassi, University of Palermo, ItalyCopyright © 2022 Ruiu, Di Lorenzo, Cavallo and Conti. This is an open-access article distributed under the terms of the Creative Commons Attribution License (CC BY). The use, distribution or reproduction in other forums is permitted, provided the original author(s) and the copyright owner(s) are credited and that the original publication in this journal is cited, in accordance with accepted academic practice. No use, distribution or reproduction is permitted which does not comply with these terms.
*Correspondence: Federica Cavallo, ZmVkZXJpY2EuY2F2YWxsb0B1bml0by5pdA==
Disclaimer: All claims expressed in this article are solely those of the authors and do not necessarily represent those of their affiliated organizations, or those of the publisher, the editors and the reviewers. Any product that may be evaluated in this article or claim that may be made by its manufacturer is not guaranteed or endorsed by the publisher.
Research integrity at Frontiers
Learn more about the work of our research integrity team to safeguard the quality of each article we publish.