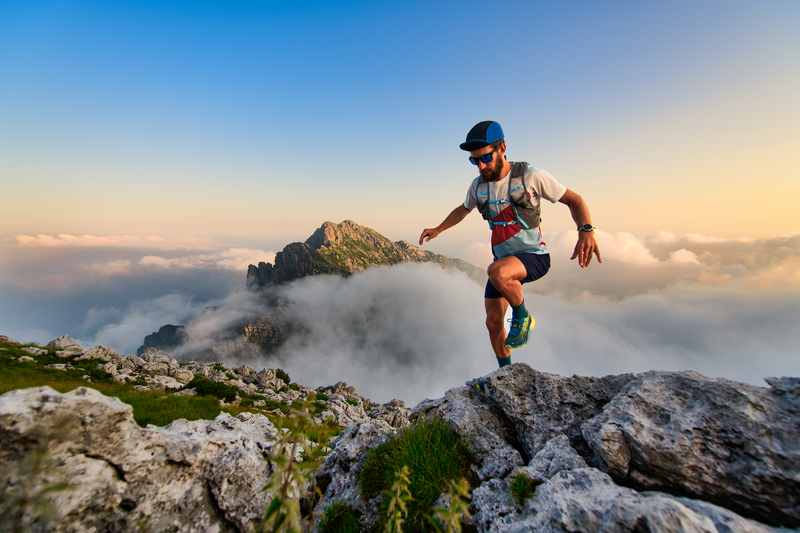
95% of researchers rate our articles as excellent or good
Learn more about the work of our research integrity team to safeguard the quality of each article we publish.
Find out more
REVIEW article
Front. Oncol. , 22 March 2022
Sec. Pharmacology of Anti-Cancer Drugs
Volume 12 - 2022 | https://doi.org/10.3389/fonc.2022.855019
This article is part of the Research Topic Nanomaterials and Multimodal Tumor Therapy View all 45 articles
The complexity of the tumor microenvironment presents significant challenges to cancer therapy, while providing opportunities for targeted drug delivery. Using characteristic signals of the tumor microenvironment, various stimuli-responsive drug delivery systems can be constructed for targeted drug delivery to tumor sites. Among these, the pH is frequently utilized, owing to the pH of the tumor microenvironment being lower than that of blood and healthy tissues. pH-responsive polymer carriers can improve the efficiency of drug delivery in vivo, allow targeted drug delivery, and reduce adverse drug reactions, enabling multifunctional and personalized treatment. pH-responsive polymers have gained increasing interest due to their advantageous properties and potential for applicability in tumor therapy. In this review, recent advances in, and common applications of, pH-responsive polymer nanomaterials for drug delivery in cancer therapy are summarized, with a focus on the different types of pH-responsive polymers. Moreover, the challenges and future applications in this field are prospected.
Cancer is a major cause of morbidity and mortality, greatly impacts quality of life, and is a serious threat to human health; the number of diagnosed patients has increased gradually in recent years (1–4). For example, recently, there were 18 million new cancer cases and 9.5 million cancer deaths worldwide in 2018 (1). Many scientists worldwide are committed to researching the diagnosis and treatment of cancerous tumors (5–10). Although this research has achieved some results, to some extent, the complex tumor microenvironment remains a barrier to novel tumor therapy programs. Chemotherapeutic therapy, referred to as chemotherapy, is still one of the main methods of tumor treatment (11–13). Some chemical drugs, such as oxaliplatin, doxorubicin (DOX), and paclitaxel (PTX), are applied to kill tumor cells and shrink or destroy the tumor (14–18). Because drug delivery is generally given systemically, the body itself will also be affected and damaged to some extent, causing side effects, complications, and even death in some serious cases (19–22). Therefore, the development of target-specific or stimuli-sensitive drugs that can identify tumor cells, to achieve the purpose of tumor treatment with no or very little impact on normal tissue cells, is the current focus of research.
During tumor growth, cell proliferation and angiogenesis are uncontrolled and dysfunctional, resulting in a complex tumor microenvironment, comprising severe hypoxia, a slightly acidic pH, and excessive ROS (22–24). Owning to the severely hypoxic microenvironment, a large number of acid metabolites are produced through glycolysis in tumor cells, leading to a weakly acidic microenvironment (pH < 6.5) around tumor tissues, which is lower than the pH value of blood and healthy tissues (pH 7.4) (25–27). In addition, after endocytosis, the rapid acidification due to proton influx decreases the pH values of intracellular and suborganelle structures (pH 5.0–6.0) and lysosomes (pH 4.0–5.0) (28, 29).
This complex tumor microenvironment can be exploited by researchers to obtain specific responses; for example, pH-responsive stimuli were found to respond to the difference in pH between normal and tumor tissues (30, 31). pH-responsive stimulation is a powerful tool that can be used to regulate the properties of materials (32). Researchers have designed pH-responsive polymer nanomaterials (10–100 nm) (33) that can be engineered to suit the need of the therapy and target area. These materials are relatively stable in the blood and normal tissue but are unstable in the tumor area (acidic pH), leading to rapid changes in the degree of ionization in pH-responsive groups, causing material changes that release the anti-tumor drugs, achieving the goal of targeted tumor therapy (34, 35). Researchers have performed sustainable research on these pH-responsive polymer nanomaterials (36, 37). This review will focus on pH-responsive polymer nanomaterials in tumor therapy and comprises a brief introduction to anti-tumor drug loading methods, a clarification of the mechanism and application of pH-responsive polymer nanomaterials in tumor therapy, an outlook addressing the great recent challenges faced, and suggestions for applications and future research directions.
Theoretically, pH-responsive polymer nanomaterials can carry anti-tumor drugs, which are transported into the tumor tissue and are then released to produce therapeutic effects on tumors. pH-responsive nanomaterials are classified into organic nanomaterials (liposomes, polymer micelles, polymer capsules, nano-gels, dendrimers, etc.), inorganic nanomaterials [carbon nanoparticles (NPs), silica NPs, gold NPs, etc.], and composite nanomaterials [metal–organic frameworks (MOFs) and metal-phenolic networks, etc.] (Table 1). They exhibit many different structures: linear (homopolymer, biblock copolymers, multiblock copolymers, and organic/inorganic hybrid polymers), non-linear branched (branched or hyperbranched polymers), and grid. Linear homopolymers and amphiphilic and amphiphilic block copolymers the most common types, and mainly form micelles, vesicles, dendritic macromolecules, and nanogels. Owing to the different properties of different drug molecules, it is necessary to select the appropriate nanomaterial according to the properties of the particular drug; drugs and nanomaterials can be combined in different ways. The anti-tumor drug loading methods for pH-responsive polymer nanomaterials can be classified into three categories: physical encapsulation, intermolecular force, and chemical bonding, via the connection between the pH-responsive polymer nanomaterial and anti-tumor drug (Figure 1).
Figure 1 Schematic diagram of pH-responsive polymer nanomaterials loaded with anti-tumor drugs and entering the tumor environment.
Physical encapsulation is the earliest and most effective drug loading method for nanomaterials. It can be used in organic, inorganic, and composite nanomaterials; the most common nanomaterials discussed for its use in the literature are liposomes, polymer micelles, polymer capsules, mesoporous silica NPs, MOFs, and metal–polyphenol nano-networks.
Liposomes were the earliest kinds of nano-drug. They mainly consisted of a bilayer of phospholipid molecules, which could be used to encapsulate the anti-tumor drugs in a hydrophobic phospholipid bilayer or a central hydrophilic cavity; their drug piggybacking was achieved through the physical encapsulation route. Hong et al. (38) designed lipid–polymer hybrid NPs with poly(lactic-co-glycolic acid) as the hydrophobic core to encapsulate afatinib and/or miR-139 encapsulated in an amphiphilic shell of polyethylene glycol (PEG) liposomes, and further modified the shell layer with ligand arginine and pH-sensitive cell-penetrating peptide histidine to enhance the targeting and penetration of these multifunctional NPs in the acidic microenvironment of colorectal cancer cells. Similar to iposomes, physical encapsulation is the main method of drug loading polymer capsules; the polymeric capsules encapsulate hydrophobic drug molecules within the capsule wall and hydrophilic drug molecules in the cavity (39). Zhong et al. (40) synthesized a pH-responsive block polymer, which comprised methoxy PEG (mPEG) and poly(asparagyl diisopropylethylenediamine-co-phenylalanine), self-assembled into nano-microcapsules as a drug carrier, and encapsulated the hydrophilic hypoxia-activated prodrug tirapamine and hydrophobic photosensitizer dihydroporphyrin [chlorin e6 (Ce6)] to treat breast cancer effectively. Polymeric micelles are the most common carriers of high-molecular-weight polymers. They are thermodynamically stable colloidal solutions formed by the self-assembly of amphiphilic block copolymers in water, and their “core-shell” structure can encapsulate hydrophobic drugs in hydrophobic cores. Xu et al. (41) encapsulated the reactive oxygen species (ROS) generator vitamin K3 with pH/ROS dual-sensitive polymer prodrug PEG-b-P[(LL-g-TK-PTX)-(LL-g-DMA)] micellar NPs to prepare polymeric prodrug-based drug delivery systems (DDSs) with pH-induced surface charge inversion and ROS amplification for ROS-triggered self-accelerated drug release. Mesoporous silica NPs (MSNs) contain a large number of pore structures, which can significantly increase the drug loading capacity and can be blocked by blocking agents, such as surface films or pH-sensitive ZnO quantum dots (QDs), to prevent the drug from leaking at non-targeted positions during transportation in vivo. The inorganic material on the walls of MSNs can prevent mechanical, thermal, and biological degradation of the encapsulated drugs to provide safe and effective drug delivery (42). Yan et al. (43) developed a biodegradable pH-responsive hollow MSN for the co-delivery of pheophorbide a and DOX. The NP possessed controllable particle size and a large inner hollow core, giving it excellent encapsulation capacities. MOFs are crystalline materials consisting of organic bridging ligands linked to metal ions (clusters) employing coordination bonds to form an infinitely extended network-like structure, which can physically embed drugs. He et al. (44) loaded cisplatin precursor drugs into UiO nanoscale MOFs with hexagonal-plate morphologies by encapsulation and loaded multiple drug resistance gene-silencing siRNAs by the surface ligand to enhance the therapeutic efficacy against drug-resistant ovarian cancer cells. Liposomes and nanocapsules can carry drugs only by physical encapsulation, unlike other carriers, which can carry drugs through physical encapsulation, using intermolecular forces, or via chemical bonding.
The use of intermolecular forces is also a common non-chemical bonding method of attaching drugs to carriers. Such forces include electrostatic attraction, hydrogen bonding, π–π interaction, van der Waals forces, and hydrophobic interactions. For water-soluble polymers, drugs can be loaded onto nanomaterials by intermolecular interaction to increase their circulation time and limit their toxicity to normal tissues (45–48). Polymer micelles, nanogels, carbon NPs, and dendritic macromolecules can be combined with drugs via one or more intermolecular forces. Polymeric micelles are capable of carrying drug molecules in their cores using hydrophobic forces and hydrogen bonding forces. Wu et al. (49) wrapped hydrophobic DOX in the core of core/shell PEGylated poly(glycerol sebacate) (PEGS)/hydroxyapatite hybrid nanomicelles using hydrogen bonding and fabricated spherical NPs with a high drug loading capacity and pH-sensitive response release by optimizing the coordination of PEG nanomicelles with the hydroxyapatite mineralization-responsive release of spherical NPs. In addition, multi-intermolecular forces can stabilize the bond between carriers and drugs. Zhao et al. fabricated co-loaded NPs using supramolecular assembly, which consisted of a block copolymer of mPEG and polycarbonates, DOX, and Ce6. DOX and Ce6 were mainly dispersed in the hydrophobic core. The hydrophobic polycarbonate chain segments and cationic polycarbonate chain segments generated strong intermolecular interactions between the polymer and cargo, including hydrophobic interactions, hydrogen bonding interactions, and electrostatic interactions. The strong interactions between Ce6 and DOX, including electrostatic interactions, π–π stacking interactions, and hydrophobic interactions, favored the formation of NPs (50). Nanogels with an internal cross-linked structure often adsorb drug molecules through hydrophobic interactions and can use their swelling and anti-swelling characteristics to achieve the stimulus-responsive release of drug molecules. Howaili et al. (51) synthesized a plasmonic nanogel loaded with curcumin (AuNP@Ng/Cur) as a stimuli-responsive nanocarrier, using an incubation method at temperatures above the lower critical solution temperature to promote hydrophobic interactions between the Cur and nanogel to achieve loading. Dendrimers are well-defined spherical structures of multi-branched polymers, characterized by central cores, branches of repetitive units, and an outer layer of multivalent functional groups. Their hydrophobic core can encapsulate uncharged non-polar small molecular drugs, and their functional groups can electrostatically interact with charged polar molecules or chemically bind with immunotherapeutic agents such as therapeutic antibodies. Shi et al. (52) developed a unique polymeric hybrid NP nanoplatform for multi-stage siRNA delivery, which was formed by electrostatic interactions between the copolymer mPEG–poly(l-histidine)-poly(sulfadimethoxine), the dendrimer poly-l-lysine, and PLK1siRNA to efficiently deliver siRNA.
Researchers often combine drugs with carriers through chemical bonds, such as coordination bonds and acid-unstable bonds, and common nanomaterials, including composite nanomaterials (MOFs), organic nanomaterials (dendrimers), and inorganic nanomaterials (quantum dot nanomaterials).
MOFs are composite nanomaterials with intramolecular pores formed by the self-assembly of organic ligands and metal ions or clusters through coordination bonds (53). Shen et al. (54) prepared a core-shell nanocomposite composed of zeolite imidazole skeleton-90 (ZIF-90) coated with spermine-modified acetal dextran (SAD), in which hydrophilic DOX was covalently bonded to the ZIF-90 skeleton and the hydrophobic photosensitizer IR780 was loaded into the SAD shell, thus allowing combined photodynamic therapy and chemotherapy. Duan et al. (55) fabricated a novel pH-responsive MOF antigen-delivery system to solve the problems in the delivery strategy for tumor-associated antigens (TAAs). Through the one-pot synthesis process, TAAs were loaded into MOFs, which were released into the tumor site and disintegrated due to hydrolysis caused by the slightly acidic tumor environment, thus enhancing the anti-tumor effect. In dendrimer polymers, such as PAMAM, functional groups can be chemically linked with immunotherapeutic agents. Xia et al. (56) linked DOX to the terminal amino group of PAMAM through a pH-sensitive hydrazone bond [PEG-PAMAM-DOX (PPD) conjugate] and designed a PAMAM-based NP (LMWH/PPD/CpG) loaded with DOX and immunoadjuvant cytosine–phosphate–guanine oligonucleotides (CpGODNs) for the combined chemotherapy and immunotherapy of metastatic melanoma. QDs in inorganic nanocarriers can also combine drugs with carriers through special coordination. Cai et al. (57) synthesized the first acid-decomposable, luminescent aminated ZnO QDs as nanocarriers with ultra-small size, modified with dicarboxyl-terminated PEG and hyaluronic acid (HA), and finally, constructed a ZnO QD-based pH-responsive drug delivery platform, in which DOX molecules were successfully loaded to PEG-functionalized ZnO QDs via the formation of a metal–DOX complex and covalent interactions. After the ZnO QDs are taken up by cancer cells, the metal–drug complexes dissociate in the acidic environment of the endosomes or lysosomes, releasing Zn2+ and DOX, achieving synergistic therapy.
In terms of drug loading rate and accurate controlled drug release, the performance of physical encapsulation is poorer than that of the other two drug-loading methods, which can not only reduce the non-specific release of drugs during circulation, but can also increase drug loading by adjusting the ratio of reactants and have obvious advantages in clinical production and application. These benefits may be related to the molecular weight, surface and internal structures of the material, whether the surface is charged, and whether the surface is hydrophobic. Owing to the advantages and disadvantages of these three different loading methods and the complexity of the tumor microenvironment, researchers have constructed nanomaterials with a diverse combination of different drug-loading methods and achieved satisfactory results in drug delivery and tumor treatment. Two pH-sensitive conjugates, pluronic P123-mono–hydrazone bond-P123-single-hydrazone–docetaxel and pluronic P123-double–hydrazone bond–docetaxel, prepared by Su et al. (58) were used as carrier materials to encapsulate docetaxel physically. These polymer micelles, which were prepared using chemical combination and physical encapsulation, can increase the drug loading capacity and drug release control compared to those created by chemical combination or physical encapsulation.
pH-responsive polymer nanomaterials carrying anti-tumor drugs can change their structures or properties with the pH reduction in the tumor microenvironment, thus providing accurate targeted tumor therapy. The common pH-sensitive structures mainly include chemical bonds, which are relatively stable in a neutral or alkaline environment but unstable in an acidic environment and are hydrolyzed or broken; polymers that can change their charge properties according to the change in pH (59); and other special pH-responsive polymers. Since these special pH-responsive structures will change their structures or properties according to changes in pH in the environment, the structure of nano-drug carriers containing these structures will also change (rearrangement, expansion, or degradation) (60). The aim of this is to release the loaded therapeutic drugs, remove the protective layer, cause disintegration close to targeted molecules in areas of pH change, and overall, achieve improved drug delivery efficiency and therapeutic effects (61; 62).
Previously, we have discussed the drug loading methods for nanomaterials. Although the special spatial structure of some nanomaterials allows for the physical loading of drug molecules, combining the drugs and polymer carrier through chemical bonds can better control the distribution and release of drugs, can make the nano-drug system more stable in vivo, and only specifically degrade and release drugs in the tumor tissue environment (63–66). At present, we already know that many chemical bonds are unstable and easy to break in a strongly acidic or strongly alkaline environment. We can utilize these unstable chemical bonds in an acidic environment and introduce them into nano-drug carriers to construct a pH-responsive polymer DDS that can specifically release drugs in the slightly acidic tumor microenvironment. The common pH-sensitive bonds mainly include hydrazone/acyl hydrazone, imine, acetal/ketal, oxime, amide, ester/orthoester, β-thiopropionatent, and borate ester (59, 60, 67–69). The general structures of these bonds and the corresponding hydrolyzed products are summarized in Figure 2.
Hydrazone/acyl hydrazone bonds are formed by the condensation reaction of hydrazine groups (-NH-NH2) or hydrazide groups (-CONH-NH2) with ketones or aldehydes. This reaction is relatively stable at pH 7.4, and thus, the hydrolysis rate is very slow but will be accelerated at lower pH levels (70). Based on this, hydrazone/acyl hydrazone bonds are widely used in drug delivery (71).
In the early 1990s, Greenfield et al. (72) and Kaneko et al. (73) had studied various hydrazone bonds formed by the condensation of the ketone groups of DOX with hydrazide groups. The sensitivity of the hydrazone bond to the acidic environment has since been deeply and extensively studied, and it has been applied to various structures, including linear (73), star-shaped (74), and cross-linked (75) molecules. Recently, Long et al. (76) reported a novel strategy to endow cellulose nanocrystals (CNCs) with pH responsiveness and drug loading through the formation of hydrazone bonds between PEG-modified CNCs and the aldehyde group of DOX. DOX was loaded on PEG-modified CNCs and could be specifically released from pH-responsive drug polymer carriers at the tumor site. The results of anti-tumor experiments both in vivo and in vitro showed that the drug carrier had obvious cytotoxicity and a good anticancer effect.
The most common method of introducing hydrazone bonds into nano-drug carriers is the condensation of hydrazide groups in various polymers with ketones or aldehyde group drugs. Therefore, the existence of ketone or aldehyde functional groups in drug molecules is the main challenge hindering the application of hydrazone bonds (77). Although many researchers have synthesized drug derivatives to obtain ketones or aldehyde groups, such as PTX synthesized by Alani et al. (78), cisplatin synthesized by Aryal et al. (79), and Cur derivatives synthesized by Li et al. (80) and Wang et al. (81) in clinical application, the product development and regulatory approval of these drug derivatives are difficult in practice (77). At the same time, the cationic polymer residues that may be formed after the hydrazone bond breaks in an acidic environment will produce cytotoxicity to a certain extent (82). These limitations in the application of nano-polymers containing hydrazone bonds to tumor therapy require improvement through further research.
The structural formula of imine bonds is -C=N-, which is widely known as a pH-sensitive dynamic covalent bond. Its carbon–nitrogen double bond structure is obtained by the condensation reaction of aldehydes or ketones with primary amines, i.e., the “Schiff base reaction”. Therefore, the Schiff base structure mainly refers to a class of compounds containing C=N groups. Although it has been proven that the structure can exist stably in an alkaline or neutral environment and accelerate the hydrolysis/breaking rate in an acidic environment (83, 84), some researchers agree that imide bonds are not stable enough under physiological conditions (i.e., pH 7.4 and 37°C) (85, 86). Thus, it has been suggested that increasing the stability by conjugating with the π–π bond to form benzoic–imide bonds could be a solution to this (87).
The synthesis of benzoic–imide bonds involves amines and benzaldehydes, which exist naturally in many raw materials and are easy to obtain and synthesize. They are very suitable for constructing pH-responsive polymer nanomaterials and have been widely used in anti-tumor DDSs. For example, Liao et al. (88) prepared pH-responsive benzoic-imide polymer nanogels as carriers of indocyanine green (ICG) by crosslinking poly(ethylenimine)-g-mPEG (PEI-g-mPEG) copolymers with hydrophobic terephthalaldehyde molecules in an aqueous solution with pH 7.4. The nanogel loaded with ICG not only significantly improved the photostability of ICG in phosphate buffer, but also clearly slowed down the leakage of ICG at pH 7.8, and when the pH was decreased from 7.8 to 6.4, the benzoic–imide bonds in the nanogel broke and released ICG, showing targeted release.
The structural formula of acetals/ketals allows for two alkoxy groups to connect on the same carbon; the difference between them is that ketals have one more central carbon substituent than acetals. Under acidic conditions, one of the oxygen atoms is protonated, thus activating the adjacent carbon atoms and leading to the hydrolytic fracture of bonds. Previous studies have shown that polymers containing acetals/ketals can be degraded into soluble monomers in the acidic pH of a tumor microenvironment (89, 90).
Acetal/ketal bonds are also widely used in pH-responsive polymer nano-DDSs (NDDSs). Zhao et al. (91) polymerized cinnamaldehyde (CA) directly with glucose through acid-unstable acetal bonds and encapsulated 10-hydroxy camptothecin (HCPT), and then, synthesized a novel type of pH-responsive DDS comprising HCPT–CA-loaded NPs (PCH). In an acidic environment, both HCPT and CA could be rapidly released by PCH. The results of anti-tumor experiments both in vivo and in vitro showed that PCH could not only effectively prolong the drug circulation, increasing the drug accumulation in the tumor site and promoting the intake of drugs, but also induced the death of cancer cells by producing intracellular ROS, showing excellent therapeutic performance and improved biosafety.
The structural formula of oxime bonds is -C=N-O-. These bonds are reversible dynamic covalent chemical bonds, similar to imine bonds. The common method for preparing oxime bonds is a condensation of aldehydes or ketones with hydroxylamine groups, which can be hydrolyzed into aldehydes, ketones, and hydroxylamines under acidic conditions (92, 93). Studies have shown that oxime bonds are reversible, have dynamic pH sensitivity, and can form a new type of pH-responsive polymer (94). Jin et al. (94) successfully synthesized a pH-responsive triblock copolymer (PEG-OPCL-PEG) by ligating aminooxy terminals of OPCL with aldehyde-terminated PEG (PEG-CHO), which consisted of hydrophilic PEG and hydrophobic oxime-tethered polycaprolactone (OPCL). DOX was selected as an anti-tumor model drug, which was encapsulated in PEG-OPCL-PEG and self-assembled into micelles in an aqueous solution. The drug release studies showed that the release rate of DOX in micelles at pH 5.0 was significantly faster than that at pH 7.4, which indicated that the oxime bond DDS had the characteristic of pH response. The results of anti-tumor experiments both in vitro and in vivo also showed that DOX-loaded PEG-OPCL-PEG micelles had a high anti-cancer effect.
Amides are an important part of pharmaceutical chemistry, and more than 25% of drugs in the database of pharmaceutical chemistry contain amide bonds (95). The structural formula of amide bonds is (-C=O-N-). The synthesis method involves initial preparation using active carboxylic acid derivatives, such as acyl chloride, anhydride, ester, and azide, followed by development into an acylated preparation using substrates such as alcohols, aldehydes, and alkynes, catalyzed by new catalysts and amines (96, 97). Because some amide bonds with special side chains are hydrolyzed and broken under weakly acidic conditions (98, 99), such as maleic acid (MA) (60), cis-aconitine acid (100), and dimethyl maleic anhydride (DMMA) (101), amide bonds are also widely used in pH-responsive polymer NDDSs. Luo et al. (102) linked stearic acid (SA) to the main chain of carboxymethyl chitosan (CMC) through amide bonds, which then self-assembled into an amphiphilic stearic acid-O-carboxymethyl chitosan conjugate (SA-CMC), and then loaded the anti-tumor drug PTX onto the conjugate to form PTX-SA-CMC NPs. In the acidic environment of the tumor, the NPs disintegrated rapidly and released drugs specifically, owing to the pH sensitivity or acid instability of amide bonds. Both in vivo and in vitro experimental results showed that compared to those in the free PTX group, the drug uptake rate of tumor cells and killing effect in the PTX-SA-CMC NP group were significantly enhanced, which not only reduced the systemic toxicity but also achieved a noticeable anti-tumor effect.
The structure of the ester bonds or orthoester bonds is similar, in which one carbon atom is connected with two or three oxygen atoms. They are also pH-sensitive and degrade faster than acetals, ketals, or hydrazone bonds in an acidic environment (103, 104). At present, ester bonds or orthoester bonds have been widely studied and applied in pH-responsive polymers. Thambi et al. (105) prepared pH-sensitive amphiphilic block copolymers with orthoester bonds using hydrophilic PEG and hydrophobic poly(γ-benzyl-L-glutamate) (PBLG) as carriers and loaded DOX into them. In vitro release studies showed that DOX was released slowly from the carrier in the physiological buffer with pH 7.4, whereas the release rate of DOX increased significantly at pH 5.0. In vitro cytotoxicity tests showed that the toxicity of these DOX-loaded nanocarriers to SCC7 cancer cells was higher than that of DOX carriers without orthoester bonds. These results indicated that this pH-responsive copolymer NDDS with orthoester bonds has great potential in tumor therapy.
Owing to the inductive effect of the S atom in the β-thiopropionate bond, the hydrolysis of the bond is driven by the positive charge on the carbonyl carbon of the ester. Compared with other pH-resbeponsive chemical bonds, such as hydrazone, imide, and acetal/ketal bonds, which are very sensitive to pH changes, and thus, have a high hydrolysis rate in an acidic environment, β-thiopropionate bonds can be hydrolyzed at a relatively slow rate in a moderately acidic environment (for example, pH 5.5) (106–108).
β-thiopropionate bonds can be synthesized by simple Michael addition reaction of mercaptan methacrylate (106, 107). Samanta et al. (69) copolymerized 2-hydroxyethyl methacrylate and a coumarin-based methacrylate monomer containing a β-thiopropionate moiety via reversible addition–fragmentation chain transfer (RAFT) polymerization. The copolymer self-assembled to form vesicular nanoaggregates at physiological pH conditions, and then, photochemical crosslinking was performed via coumarin (2π + 2π) cycloaddition reaction to further stabilize the vesicles. In the acidic environment of tumor cells, the slow hydrolysis of β-thiopropionic acid led to the disintegration of the polymers, resulting in the sustainable release of the loaded drugs. The results of the in vivo and in vitro anti-tumor experiments showed that compared with free DOX, the 50% inhibiting concentration (IC50) of DOX-loaded nanomaterials toward the MG63 cancer cell line decreased significantly, showing excellent anti-tumor effect and good biosafety.
Borate ester bonds, formed by the condensation of phenylboronic acid and its derivatives with cis-diol or compounds containing catechol groups, are also reversible dynamic covalent bonds. At acidic pH levels, borate ester bonds can be reversibly hydrolyzed and broken, which gives them broad application prospects in tumor DDSs (109–111). Fang et al. (112) prepared a mitochondrial-targeted polymer, 3,4-dihydroxyphenyl propionic acid-chitosan oligosaccharide-dithiodipropionic acid-berberine (DHPA-CDB), which was used to load Cur and self-assemble into cationic nano-micelles (DHPA-CDB/CUR). Negatively charged oligomeric hyaluronic acid-3-carboxyphenylboric acid (OHA-PBA) was added to the prepared DHPA-CDB/CUR as the ligand of sialic acid and CD44, to shield the positive charge and prolong the circulation time in vivo. The OHA-PBA@DHPA-CDB/CUR covalent polymer was formed by OHA-PBA and DHPA-CDB/CUR through pH-sensitive borate ester bonds between PBA and DHPA. The low pH in tumor tissue promoted the degradation of borate ester bonds and the exposure of cationic micelles, which led to the reversal of the polymer surface charge, thus promoting internalization and mitochondrial localization. All experimental results showed that OHA-PBA@DHPA-CDB/CUR had mitochondrial targeting and tumor environmental charge-reversal capabilities, as well as great potential in targeted drug delivery.
It is well known that nano-drug carriers reach the target position mainly through blood circulation in the human body. If there is a positive charge on the surface of the drug carrier when it encounters the many negatively charged proteins in human plasma, they will attract each other strongly; this makes it very easy for the carriers to be swallowed and cleared by phagocytes in the human body (113, 114), resulting in reduced drug delivery efficiency. The drug uptake efficiency will be reduced due to electrostatic repulsion when a drug carrier with a neutral or electronegative surface encounters the electronegative cell membrane (115, 116), whereas electropositive nano-drug carriers can better target endothelial cells, have higher vascular permeability (117), and are more likely to be swallowed by tumor cells (118–120). However, the liver can act as a charge-selective filtration barrier, which can cause the accumulation of electronegative nano-drug carriers in the liver with consequential potential side-effects. Thus, the long-cycle ability of neutral polymers or amphoteric ionic polymers is a better option (121).
In addition to attaching pH-sensitive acid-unstable bonds to the polymer backbone or side chains, there is another way to make the nano-drug carriers pH-responsive: introduce a chemical group into the nanomaterial that can lead to a change in surface charge properties (protonation or deprotonation) with the change in environmental pH. Such groups include amino, phosphoric acid, and carboxyl groups. These groups have different chemical structures and pKa values, can accept or provide protons, and undergo changes in physical or chemical properties related to pH, such as swelling or solubility, resulting in drug release (59, 60, 122). A nano-drug carrier has been designed, which has neutral or amphoteric ionic when it circulates in the human body, as this allows an improved circulation time for the nano-drug carrier in the body. When the nano-drug carrier reaches the tumor site, the surface charge of the nano-drug carrier is converted to positive, owing to the decreased pH in the tumor microenvironment, which promotes the uptake of tumor cells, thus greatly improving drug delivery efficiency (116, 123). For example, Fang et al. (124) coupled berberine (Ber) and vitamin B6 with oligomeric hyaluronic acid (OHA), creating self-assembled spherical NPs in an aqueous solution to prepare pH-responsive mitochondria-targeting NPs (B6-OHASS-Ber). In the acidic microenvironment of the tumor tissue, the pyridine group of B6 was protonated, and the surface of the carrier changed from negatively to positively charged, which promoted the internalization of NPs. After lysosome escape, it actively targeted mitochondria and released the drugs. The in vivo anticancer activity experiment results showed that B6-OHA-SS-Ber/Cur had an obvious inhibitory effect on tumor growth. As shown in Figure 3, the micelles were modified by B6 to give the NPs the ability to convert charges, Ber was induced to focus on mitochondria preferentially by drugs and carriers, and OHA was used to target CD44 receptors (125, 126).
Figure 3 (A) Schematic structure of B6-OHA-SS-Ber self-assembly into micelles; (B) the micelles entered into tumor cells and concentrated on mitochondria by active targeting and released the drug to tumor cells (124).
In addition, there also exists a pH-responsive polymer that changes the hydrophilicity or solubility of nano-drug carriers after protonation with a decrease in pH, resulting in the change in carrier structure and drug release (127). For example, some polymers synthesized from acidic monomers, such as poly(methacrylic acid) (128, 129), poly(aspartic acid) (PAsp) (130), and sulfonamide-based polymers (131), change from hydrophilic to hydrophobic when the pH is decreased. Kang and Bae (132) studied oligomeric sulfonamides (OSASs), which could effectively release polymers or transport nucleic acids. Owing to the different pKa values of different sulfonamide groups, OSASs change from hydrophilic to hydrophobic with a decrease in the surrounding pH and show different proton buffering capacities. Kang and Bae (132) combined an OSAS with DNA and then complexed it with poly(L-lysine) (PLL) to form an OSA polymer. The experimental results showed that there was no significant difference in DNA uptake between the OSA polymer and PLL/DNA control group; however, the OSA polymer induced a more extensive distribution of DNA in cells and significantly enhanced the transfection rate of DNA.
We have previously discussed the two main mechanisms of action of pH-responsive polymers, namely, unstable chemical bonds with pH sensitivity and groups or polymers with charge transfer or water solubility changes. However, these pH-responsive polymers should remain stable when the physiological pH is 7.4, and it is challenging to ensure sufficient drug release rate or cell uptake rate with a decrease of 1 pH unit (60). At present, many preclinical studies are being conducted at two pH values (7.4 and 5.0). Nevertheless, if there is only a small difference between the targeted position of the nano-drug carriers and the physiological pH, the drug release time of polymers that solely depend on the pH-responsive mechanism, especially the nano-drug carriers that rely on covalent bond cleavage, will be very slow (60). Hence, compared to single stimulus-responsive nano-polymers, dual or multiple stimuli-responsive polymer NDDSs have higher physiological or biological system correlations, can better adapt to the interaction in the real biological system (133), and greatly improve the stability of drug delivery and the sensitivity of controlled release, giving excellent anti-tumor effects and broader application prospects (134).
Compared with normal tissues, tumor tissues show significantly different physiological characteristics, such as differences in pH, redox enzyme levels, ROS levels, owing to their uncontrolled growth and abnormal gene expression. We can select or combine the corresponding stimulus-response factors for tumor-targeted therapy according to specific changes in the tumor microenvironment. In addition, some polymers will change their morphological structure, physical, or chemical properties when exposed to external stimuli (temperature, light, magnetic field, and ultrasound), which can be combined with pH stimulation response for tumor treatment to improve the therapeutic effect (135–139).
To reduce the problem of non-specific drug release in oral DDSs, Song et al. (140) studied the effect of pH response combined with enzyme response (double stimuli) on precisely targeted drug delivery. They anchored polyacrylic acid (PAA) and chitosan (CS) onto Gd3+-doped mesoporous hydroxyapatite NPs (Gd-MHAPNPs) to realize programmed drug release and magnetic resonance imaging at tumor sites. Among them, the grafted PAA acted as a pH-responsive switch to control the release of drugs into the colon. In addition, CS was functionally modified into enzyme-sensitive parts and could be degraded by β-glucosidase in the colon. Chemotherapeutic drugs (5-fluorouracil) and targeted therapy drugs (gefitinib) were encapsulated in Gd-MHAP NPs for synergistic therapy (Figure 4). The experimental results showed that CS and PAA protected drug-loaded NPs from various physiological conditions in the gastrointestinal tract, in order for the drug to reach the colon tumor site, prevent premature drug release, and control drug release via the dual stimulus-response mechanism (pH and enzyme), which increased the drug load at the colon tumor site and improved the therapeutic effect.
Figure 4 Schematic illustration of the preparation of Gd-MHAPNPs and the mechanism of drug release in different physiological environments in the GIT (140).
Owing to the high reducibility in the tumor environment, such as the increased glutathione (GSH) content, Kim et al. (141) designed pH- and GSH-responsive fluorescent polymer dots (L-PDs) containing PTX. The core part of the L-PD nano-hybrid system used disulfide bonds as the redox response site, which could not only maintain the stability of the internal structure but could also avoid the leakage of PTX during the cycle. The nano-hybrid system showed fluorescence quenching behavior, with less than 2% of the PTX being released under physiological pH conditions. However, in the tumor microenvironment, fluorescence was restored under the acidic pH, and the PTX release was about 100%, which significantly improved the effectiveness of killing tumor cells. In addition, the experimental results showed that there were differences in the release of PTX under different GSH concentrations, and the explosive release of PTX was detected under a high GSH concentration.
In addition, because of the high levels of reactive oxygen species (ROS) and low pH levels in the tumor microenvironment, Jäger et al. (142) designed functionalized “AND gate” multi-responsive (MR) block amphiphilic copolymers with dual stimulus-response to ROS and pH for cancer drug delivery therapy. The hydrophobic part of the polymer contained masked pH reaction side chains, which were exclusively exposed in response to the high ROS in the tumor microenvironment. These researchers synthesized two kinds of pH-responsive polymers from MR-ethyl and MR-isopropyl and then prepared DOX-loaded nano-drug carriers (multi-responsive nanoparticles, MRPs) and control-free DOX carriers using microfluidic self-assembly technology. The experimental results showed that compared to the control-free DOX carriers, the drug release rate of the MRP group was significantly faster under the influence of the dual ROS and pH response.
In addition to taking the different biochemical indexes of tumor tissue as stimulus-response factors, some polymer components undergo volume phase transition when the external environment, such as temperature, changes, thereby triggering the selective release of drugs. For example, Wang et al. (143) synthesized the temperature and pH double stimuli-responsive NPs consisting of deoxycholic acid and hydroxybutyl-decorated chitosan (DAHBCNPs) and designed a series of DAHBCs with different lower critical solution temperatures (LCSTs, all below 37°C) to load Cur for the oral treatment of colorectal cancer. Owing to the mutual offset of the size change between the polymer expansion after pH response and the polymer contraction after temperature response, the DAHBC NPs were relatively stable under simulated gastric conditions (pH 1.2, 37°C). However, in simulated intestines (pH 7.0–7.4, 37°C), DAHBC NPs promoted the continuous release of Cur into the intestinal tract on account of the single effect of polymer contraction after temperature response. Compared with free Cur, DAHBC NPs at 27°C (DAHBC27) were better absorbed by Caco-2 cells through transcellular and paracellular transport pathways, and the intestinal absorption efficiency of Cur was greatly improved by paracellular transport pathways. In addition, Chen et al. (144) Feng et al. (145), and Lin et al. (146) reported on multifunctional nano-drug carriers with dual pH and temperature-stimuli responses (photothermal therapy). For example, Chen et al. (144) prepared a multifunctional NDDS with pH and temperature response based on an ordered mesoporous silica-sandwiched black phosphorus nanosheet (BP@MS) with vertical pore coating. The special structure of the drug carrier not only enhanced the dispersity of the BP nanosheet and improved the drug loading efficiency (DOX), but also facilitated post-modification, such as the PEGlyation and conjugation of the ligand targeting TKD peptide. The results of in vivo and in vitro anti-tumor experiments showed that the DOX-loaded BP@MS system (BSPTD) manifested heat-stimulative, pH-responsive, and sustained release behaviors. Moreover, the BSPTD could also significantly inhibit the lung metastasis of tumors, owing to the secondary administration promoted by photothermal degradation.
In addition to dual stimuli, the combined application of multiple stimuli in tumor therapy has been widely studied. Don et al. (147) synthesized a poly(AA-b-NIPAAm) copolymer (PAA-b-PNIPAAm) via reversible addition–fragmentation chain transfer polymerization, consisting of PAA and poly(N-isopropylacrylamide) (PNIPAAm), which self-assembled to form nanogels with cationic protein (protamine). Chemical characterization showed that the nanogel exhibited a change in protamine conformation, PAA-b-PNIPAAm disaggregation, and protein enzymatic hydrolysis when stimulated by temperature, pH, and enzyme activity level changes. Changing the pH from 7.4 to 5.0-6.5 increased the mean particle size and converted the surface charge from negative to positive. After cold shock treatment, DOX was rapidly released in the cells. Furthermore, the nanogel could also carry a rose bengal photosensitizer and cause significant damage to cancer cells under irradiation.
In addition to the two basic principles introduced above, another pH response mechanism has been gradually discovered and studied, the principle of which is that bicarbonate reacts with an acid to produce CO2 and H2O. Therefore, polymer nanomaterials containing bicarbonate structures may also release drugs specifically in an acidic environment, in what is known as a gas-generating pH-sensitive nano-system (59). Kim et al. (148) encapsulated poly(lactide-co-glycolide) (PLGA) NPs with sodium bicarbonate, which could be decomposed to produce CO2 under acidic conditions, and imidazoquinoline-based synthetic toll-like receptor 7/8 agonist (TLR7/8, also known as “522”). The addition of sodium bicarbonate not only significantly increased the loading dose of 522, but also decomposed and released CO2 through pH response after the NPs were internalized by tumor cells and phagocytized by lysosomes, resulting in the release of 522 into tumor cells. Finally, the experimental results also showed that the new pH-sensitive NPs had a better anti-tumor effect than traditional PLGA NPs.
At present, there are many effective treatment approaches for tumors, from surgical resection, chemotherapy, and radiotherapy to landmark molecular targeted therapy, immunotherapy, gene therapy, photodynamic therapy, photothermal therapy, and chemodynamic therapy, all of which have made great progress and development throughout the years. As is well known, the traditional administration of chemotherapy leads to the poor targeting of drugs to tumors, low drug utilization, and many other challenges. Therefore, scholars have proposed DDSs in which chemotherapeutic drugs are loaded and transported on carriers (149). To improve the efficiency of DDSs, it became a hot spot (150, 151) in tumor therapy research to design nano-drug carriers that can respond to the abnormal biochemical indicators in the tumor microenvironment (152) to release drugs directly into the tumor site. With the development of nanomaterials, nano-systems can enhance the permeability and retention of drugs in solid tumors (59) by introducing different stimulus-responsive structures into DDSs (153), among which pH-responsive polymers have been widely used in stimulus-responsive NDDSs (59, 100). The common applications of pH-responsive polymer nanomaterials in tumor therapy are summarized in Figure 5.
Figure 5 Application of pH-responsive polymer nanomaterials in tumor monotherapy (left) and application of pH-responsive polymer nanomaterials in tumor synergistic treatment (right).
Single drug therapy will produce serious drug resistance in tumor treatment, but combinations of different drugs have been proven to be very effective in overcoming multidrug resistance (154, 155). Moreover, the combination of two or more drugs can generally reduce the drug dose and toxicity (156). Thus, constructing a multifunctional pH-responsive NDDS provides an effective strategy for chemotherapy. Wang et al. (157) synthesized multifunctional NPs (FTDCAG NPs), using a pH-responsive prodrug (PEG2K-NH-N-DOX), a GSH-responsive prodrug (PEG2K-S-S-CPT), folate-receptor targeting polymers (FA-PEG2K-L8, FA-PEG2K-TOS), and T1-enhanced magnetic resonance imaging contrast agents (Gd-DTPA-N16-16) used to encapsulate combrestatin A4 (CA4). These FTDCAG NPs contained three drugs, namely, CA4, DOX, and CPT. At pH 6.5, the drug release rate of CA4 increased by 63.4%. After release, CA4 destroyed angiogenesis in the tumor microenvironment and promoted the uptake of the remaining FTDCG NPs by tumor cells. After entering tumor cells, the FTDCG NPs disintegrated, owing to the increase in intracellular GSH concentration and the decrease in pH levels in the endosomes or lysosomes, releasing CPT and DOX. The results showed that FTDCAG NPs had a significant anti-tumor effect, and controlled drug release in time and space, which could maximize the effect and accuracy of cancer chemotherapy.
Radiotherapy is the most effective method to treat malignant brain tumors, but it is a challenge to ensure minimum damage to the surrounding normal tissue, while providing the best therapeutic dose to the tumor tissue (158). Therefore, one of the basic treatments for glioblastoma is radiotherapy combined with radiosensitizers. Shirvalilou et al. (159) used 5-iodo-2-deoxyuridine (IUdR) as a radiosensitizer for glioblastoma and magnetic graphene oxide NPs coated with PLGA polymer as drug carriers for IUdR (MNPs). The results of in vivo and in vitro experiments showed that MNPs not only prolonged the circulation time in vivo but also targeted glioma through an external magnetic field after intravenous injection. Moreover, the experiment results illustrated a higher IUdR release rate at pH 5.6 than at pH 7.4, where 82% and 56% of the IUdR was released within 24<n,bsp/>h respectively. The drug release rate was significantly increased when the NPs reached the tumor site due to the degradation of PLGA polymers as a result of charge transfer under acidic pH conditions, revealing the controlled drug release abilities of the MNPs in the acidic environment of the tumor. Under high voltage X-ray irradiation, compared with the control group, IUdR/MNPs showed a stronger radiation sensitization effect and increased apoptosis induced by radiation, which improved the therapeutic effect.
Compared with chemotherapy, immunotherapy has more advantages in terms of avoiding toxicity and side effects, inhibiting tumor metastasis, and preventing tumor recurrence; it is recognized as the next generation of tumor therapy (160, 161). The complement system plays an important role in promoting antigen-specific immune responses (162). Polymers carrying nucleophilic groups, such as hydroxyl or amino groups, can activate the complement system, resulting in the maturation of dendritic cells and induction of enhanced immune responses (163–165). Li et al. (166) prepared a multifunctional pH-responsive micelle vaccine platform (COOH-NPs) based on a carboxyl-modified deblock copolymer of poly(2-ethyl-2-oxazoline)–poly(D, L-lactide) (COOH-PEOz-PLA). After injection, the vaccine showed good targeting, and the tertiary amine groups in the PEOz backbone became protonated under acidic conditions, imparting the micelle payloads with endosomal escape via the “proton sponge” effect (167, 168). Compared with the control group, the nano-vaccine exhibited good laminin targeting, antigen presentation ability of cytotoxic T lymphocyte immune response, and complement activation to promote dendritic cell maturation, which significantly inhibited tumor growth and prolonged survival time.
Traditional gene therapy generally adopts the method of enhancing host anticancer immunity, antagonizing antioncogenes, expressing suicide genes, or gene modification combined with high-dose chemotherapy. While achieving certain curative effects, it also has some disadvantages, such as low gene vector introduction efficiency and poor accuracy and controllability of foreign gene expression in vivo. With the development of genomics and genetic engineering research, recent new technologies, such as targeted gene-viro-therapy (169), RNAi technology (170), and microRNA (171), provide new bases or evidence for the accurate treatment of cancer. In gene therapy, hyperbranched PEI-25k and its derivatives have been widely developed and applied, owing to its high density of positive charges, which can bind closely to DNA and promote its escape from endosomes (172, 173). Chen et al. (174) synthesized a novel pH-responsive polymer shielding system (PAMT) via click reaction between poly(γ-allyl-L-glutamate) and thioglycolic acid or 2-(Boc-amino) ethanethiol. PAMT was electrostatically attached to the surface of the positively charged PEI/pDNA complex to form a ternary complex. When the pH in the external environment decreased from 7.4 to 6.8, the PAMT released gene therapy drugs through rapid charge conversion. The in vivo anti-tumor experiment showed that the transfection efficiency of the PAMT/PEI/shVEGF group was higher and the inhibitory effect on tumor growth was more obvious, compared to the control group.
In recent years, photodynamic therapy (PDT) has been widely used in tumor therapy. Earlier, Kelly et al. (175) successfully used hematoporphyrin derivatives as photosensitizers in the treatment of bladder cancer, creating a precedent for the application of PDT on tumors. The traditional DDSs relying on photosensitizers have limited application for the PDT of tumors, owing to their poor pharmacokinetics (176, 177) and long-term biological safety issues (176–178). For example, MoS2 DDSs, the biotoxicity of which is caused by quantum size effects and surface effects, may have adverse effects on the human reproductive system (178). Previous studies have shown that nanomaterial drug carriers have a higher uptake rate of tumor cells and fewer side effects (177), and the specific response of drugs to the tumor can be further enhanced by designing stimulus-responsive nano-drug carriers.
To overcome the shortcomings of the non-specific tumor therapy associated with PDT, Li et al. (179) and He et al. (180) applied nanomaterials with stimulus-response to PDT, which made the therapeutic drugs release specifically at the tumor site, reducing the non-specific phototoxicity of PDT and improving the anti-tumor effect. Ma et al. (181) combined chidamide as a histone deacetylase inhibitor (HADCi) with pH-responsive block polymer folate PEG-b-poly(aspartic acid) (PEG-b-PAsp)-grafted folate (FA-PEG-b-PAsp) to obtain block polymer folate PEG-b-poly(asparaginyl-chidamide) [FA-PEG-b-PAsp-chidamide (FPPC)]. Then the model photosensitizer pyropheophorbide a (Pha) was coated with FPPC and polymer micelles (Pha@FPPC) were formed in PBS. After the uptake of Pha@FPPC by tumor cells through FA-receptor-mediated endocytosis, PAsp changed from hydrophilic to hydrophobic in the lysosome due to the decrease in pH, which led to the directional release of Pha molecules. The results showed that Pha@FPPC had low dark cytotoxicity in vitro and a good therapeutic index, owing to its high dark cytotoxicity:photocytotoxicity ratio. Furthermore, compared with free Pha, Pha@FPPC not only significantly inhibited the growth of the implanted tumor and prolonged the survival time of melanoma-bearing mice, but also remarkably prevented the pulmonary metastasis of mice melanoma through folate-mediated and selective accumulation.
Similar to PDT, photothermal therapy (PTT) has become an effective method to treat cancer with high specificity, good anti-tumor effects, controlled drug release, and few side effects (154, 182, 183). As an NIR-absorbing organic photosensitizer, ICG is an amphiphilic tricarbocyanine dye that has been approved by the United States Food and Drug Administration for medical diagnosis and imaging. Although ICG has great potential in PTT applications, it also has many defects (184, 185). To overcome these shortcomings, pH-responsive polymer micelles or NPs have been used to design tumor-targeting ICG delivery methods. Ting et al. (186) co-assembled hybrid polymer NPs (PbCTPNs) comprising hydrophobic PLGA segments, ICG molecules, amphiphilic tocopherol PEG succinate (TPGS), and pH-responsive mPEG-benzoic imine-1-octadecylamine (mPEG-b-C18) segments. PbCTPNs maintained sufficient stability under physiological pH conditions, effectively inhibiting the self-aggregation and leakage of ICG. However, when the pH decreased to 5.5, the unstable imine bond in mPEG-b-C18 was hydrolyzed, which accelerated the release of ICG molecules in combination with near-infrared radiation, significantly killing MCF-7 cells and improving the anticancer efficacy of ICG-mediated PTT.
Previously, we discussed the application of pH-responsive polymers in tumor monotherapy, such as PDT, PPT, immunotherapy, and gene therapy; although they are still in the early clinical stages, they have shown good efficacy and clinical results. However, studies and clinical experiences have shown that it is difficult for monotherapy to eliminate tumors and control their metastasis; some cell subsets in unevenly distributed tumor tissues are resistant to monotherapy (187, 188). For example, in chemotherapy, long-term use of the same anticancer drug will induce tumor multidrug resistance, resulting in a decline in efficacy (189). Thanks to the rapid development of nanotechnology, we can combine two or more therapies and design multifunctional nano-drug carriers for the targeted delivery of multiple drugs, which can not only improve the curative effects, but can also reduce the side effects caused by high doses of a single therapy and make up for the defects and deficiencies of various other treatments (190, 191).
Photothermal therapeutic agents are also excellent photosensitizers, and the combination of PDT and PTT may have a synergistic effect on phototherapy. Bejjanki et al. (192) studied the efficacy of PTT combined with PDT in treating tumors. They combined ICG, Fe3O4, and palmitoyl ascorbic acid (PA) with pH-responsive PEG-b-PAEMA-PDMA polymers to form nano-drug carriers. The carriers remained stable under physiological pH and the cycle time was prolonged, while drug loading was released via charge conversion and tumor cell uptake was promoted in the acidic tumor environment. The results of in vivo and in vitro tumor cell experiments showed that the nano-drug carrier showed a synergistic PDT/PTT effect, had a high apoptosis rate, and significantly inhibited tumor growth.
Compared with photodynamic therapy, chemodynamic therapy is a new treatment strategy (193). It can kill tumor cells by converting overexpressed hydrogen peroxide into highly toxic hydroxyl free radicals through Fenton and Fenton-like reactions. It has a high tumor selectivity (194, 195). Wang et al. (196) reported a pH and ROS dual-response nano-drug (PtkDOX-NM), which accumulates in the tumor site through the enhanced permeability and retention effect. In the acidic endosomal environment, the poly(2-[diisopropylamino] ethylmethacrylate) segment of the drug changes from hydrophobic to hydrophilic, resulting in β-lapachone (Lap) dissociation and rapid release. The Lap promoted the production of hydrogen peroxide, and a Fenton reaction occurred in the presence of iron ions, which significantly increased the ROS content in the tumor environment, thus promoting the effective cascade release of antineoplastic drugs in response to ROS and enhancing the curative effect through the combination of chemodynamic therapy and chemotherapy.
To verify the synergistic effect between chemotherapy and PDT therapy, Li et al. (197) combined anti-tumor drug GNA002-mediated chemotherapy with PDT therapy through an NDDS, which improved the efficacy of anticancer therapy. They used 5-(4-carboxyphenyl)-10,15,20-triphenylporphyrin as the photosensitizer and GNA002 as the hydrophobic core and cyclic RGD peptide (cRGD)-modified PEG (cRGD-PEG) connected to cell-penetrating peptide hexa-arginine (R6) through an unstable hydrazone bond as a hydrophilic shell to synthesize a nucleus-targeted pH cascade-responsive micelle nano-platform (Figure 6). The NPs were accumulated at the tumor site, and then actively internalized into tumor cells through the cRGD ligands. In cells, when the NPs were swallowed by lysosomes, the hydrazone bond was hydrolyzed and the shell fell off due to the change in pH. Then nucleus-targeted drug delivery mediated by R6 was treated with chemotherapy and PDT under laser irradiation.
Figure 6 Schematic illustration of a pH cascade-responsive nucleus-targeted nanoplatform for synergistic chemo-photodynamic therapy (197).
In addition, Tang et al. (198) studied whether there was a synergistic effect between chemotherapy and PTT. They designed a biosensor with a MOF coated with multifunctional polydopamine (PDA) for the detection of miRNA-122 with Zn2+-triggered aggregation-induced enhancement (AIE) and synergistic chemotherapy/PTT. They used ZIF-8 NPs as DOX carriers and modified them with polydopamine to improve the pH response and biocompatibility of the nano-drug carriers. When the nano-drug carrier reached the tumor site, the acidic environment led to a change in the carrier structure and accelerated the release of Zn2+ and DOX. The experimental results not only showed that the nano-drug carrier accurately detected miRNA-122, but also verified the synergistic effect of chemotherapy and PTT on tumor therapy.
To solve the multidrug resistance of chemotherapeutic drugs, the combination of chemotherapy and other therapeutic methods for treating tumors has been widely studied and explored. For instance, Wang et al. (199) studied whether there was a synergy between chemotherapy and tumor immunotherapy. They employed a unique cationic switchable lipid as a pH-responsive drug carrier, which encapsulated the anti-tumor drug sorafenib and was loaded with anti-miRNA27a-loaded anti-GPC3 antibody to form targeted lipid NPs (G-S27LN), enabling targeted release the sorafenib and antibodies in the acidic environment of the tumor. Compared to the control group, the survival rate of tumor cells decreased significantly, and the proportion of apoptotic cells was remarkably higher than that in the control group, showing a synergistic therapeutic effect.
pH-responsive polymer nanomaterials can stably carry anti-tumor drugs through the body and release them into tumors through acid-unstable bond fracture or charge conversion for certain targeted therapeutic effects. Compared with conventional tumor chemotherapy, treatment with pH-responsive polymer nanomaterials exhibits improved therapeutic efficiency with minimal invasiveness to the host organism, which highlights its great potential in clinical trials or preclinical studies. Despite the notable progress made in the field of pH-responsive polymer nanomaterials, there are many critical issues that remain to be solved for better clinical application. Therefore, the potential focuses and challenges of pH-responsive polymer nanomaterials have been discussed below.
(1) Few pH-responsive polymer nanomaterials are currently available for clinical use, owing to the enhanced permeability and retention effect, as well as other delivery difficulties (200–204). These polymer nanomaterials are delivered through five stages in tumor therapy: being injected intravenously into the bloodstream, accumulating specifically around the tumor tissue, entering into the solid tumor, cellular internalization, and “CAPIR”, i.e., “circulation, accumulation, penetration, internalization, drug release.” In the current research stage, it is impossible to consider these five stages, and it is still impossible to achieve the complete treatment of tumors (205–207). This must be the main focus of future research.
(2) Further research is needed to achieve the precise targeting of pH-responsive polymer nanomaterials, to realize an accurate combination of pH-response stimuli and other response stimuli, to optimize pH-responsive stimulation as a tumor therapy, as well as to realize the multifunctional platform function of pH-response stimulation for tumor treatment.
(3) There are some crucial issues associated with pH-responsive polymer nanomaterials, especially those containing synthetic drugs or their derivatives, including manufacturing technique problems, safe application problems, tumor treatment evaluation criteria accuracy problems, and institutional problems, which should be resolved as soon as possible to favor the successful clinical application of treatments after fundamental research is completed. More attention should be paid to establishing the entire standard treatment process, systematic evaluation, and long-term observation.
(4) Finally, the acute short-term toxicity and chronic long-term toxicity of pH-responsive polymer nanomaterials must be considered for the biological safety of clinical application in the future. These nanomaterials should be deeply studied to meet safety standards. At present, some suggested polymer nanomaterials are poisonous to the cells, causing side effects and other disease complications. For example, as mentioned earlier, the polymer nanomaterials containing hydrazone bonds may produce cationic polymer residues after hydrolysis in vivo, which are toxic to normal human tissues to some extent. Accordingly, new pH-responsive polymer nanomaterials, which are biodegradable and harmless to the body, should be developed to avoid such side effects. Sustainable materials, such as CO2 copolymers, should be further studied, with focus on ensuring that these materials undergo degradation through the Krebs cycle, metabolize into CO2 and water, and be excreted in a harmless manner via exhalation and through the action of kidneys.
In conclusion, pH-responsive polymer nanomaterials possess great potential for tumor therapy, and we believe that their beneficial effects will be recognized on a greater scale with the development of new technology and ongoing research.
FG contributed to the research retrieval and outline drafting. SC, XS and YT contributed to the research retrieval and drafting and critically revised the manuscript. SC critically revised the manuscript. Every author gave final approval and agreed to be accountable for all aspects of the work. All authors have read and agreed to the published version of the manuscript.
Financial support from the Projects directly under Jilin Provincial Department of Finance, China (JCSZ2019378-23), and the Scientific Research Project of Jilin Provincial Department of Education, China (JJKH20211215KJ)
Authors are thankful to their respective departments/institutes/universities for providing space and other necessary facilities, which helped to draft this manuscript.
The authors declare that the research was conducted in the absence of any commercial or financial relationships that could be construed as a potential conflict of interest.
All claims expressed in this article are solely those of the authors and do not necessarily represent those of their affiliated organizations, or those of the publisher, the editors and the reviewers. Any product that may be evaluated in this article, or claim that may be made by its manufacturer, is not guaranteed or endorsed by the publisher.
1. Bray F, Ferlay J, Soerjomataram I, Siegel RL, Torre LA, Jemal A. Global Cancer Statistics 2018: GLOBOCAN Estimates of Incidence and Mortality Worldwide for 36 Cancers in 185 Countries. CA Cancer J Clin (2018) 68(6):394–424. doi: 10.3322/caac.21492
2. Ryder-Burbidge C, Diaz RL, Barr RD, Gupta S, Nathan PC, McKillop SJ, et al. The Burden of Late Effects and Related Risk Factors in Adolescent and Young Adult Cancer Survivors: A Scoping Review. Cancers (Basel) (2021) 13(19):4870. doi: 10.3390/cancers13194870
3. Seebacher NA, Stacy AE, Porter GM, Merlot AM. Clinical Development of Targeted and Immune Based Anti-Cancer Therapies. J Exp Clin Cancer Res (2019) 38(1):156. doi: 10.1186/s13046-019-1094-2
4. Sung H, Ferlay J, Siegel RL, Laversanne M, Soerjomataram I, Jemal A, et al. Global Cancer Statistics 2020: GLOBOCAN Estimates of Incidence and Mortality Worldwide for 36 Cancers in 185 Countries. CA Cancer J Clin (2021) 71(3):209–49. doi: 10.3322/caac.21660
5. Naito Y, Aburatani H, Amano T, Baba E, Furukawa T, Hayashida T, et al. Clinical Practice Guidance for Next-Generation Sequencing in Cancer Diagnosis and Treatment (Edition 2.1). Int J Clin Oncol (2021) 26(2):233–83. doi: 10.1007/s10147-020-01831-6
6. Xiong H, Veedu RN, Diermeier SD. Recent Advances in Oligonucleotide Therapeutics in Oncology. Int J Mol Sci (2021) 22(7):3295. doi: 10.3390/ijms22073295
7. Atout S, Shurrab S, Loveridge C. Evaluation of the Suitability of RNAscope as a Technique to Measure Gene Expression in Clinical Diagnostics: A Systematic Review. Mol Diagn Ther (2021) 26:1–19. doi: 10.1007/s40291-021-00570-2
8. Jayabal P, Ma X, Shiio Y. EZH2 Suppresses Endogenous Retroviruses and an Interferon Response in Cancers. Genes Cancer (2021) 12:96–105. doi: 10.18632/genesandcancer.218
9. Bethge WA, Eyrich M, Mielke S, Meisel R, Niederwieser D, Schlegel PG, et al. Results of a Multicenter Phase I/II Trial of Tcrαβ and CD19-Depleted Haploidentical Hematopoietic Stem Cell Transplantation for Adult and Pediatric Patients. Bone Marrow Transplant (2021) 24:1–8. doi: 10.1038/s41409-021-01551-z
10. Bandini M, Ahmed M, Basile G, Watkin N, Master V, Zhu Y, et al. A Global Approach to Improving Penile Cancer Care. Nat Rev Urol (2021) 22:1–9. doi: 10.1038/s41585-021-00557-y
11. Wang CI, Chu PM, Chen YL, Lin YH, Chen CY. Chemotherapeutic Drug-Regulated Cytokines Might Influence Therapeutic Efficacy in HCC. Int J Mo l Sci (2021) 22(24):13627. doi: 10.3390/ijms222413627
12. Zhou H, Alhaskawi A, Sun Q, Dong Y, Kota VG, Hasan Abdulla MHA, et al. Desmoplastic Small Round Cell Tumor in a Pregnant Woman: A Case Report and Literature Review. Yale J Biol Med (2021) 94(4):613–22.
13. Volkov I, Radchenko G, Tchernykh A. Digital Twins, Internet of Things and Mobile Medicine: A Review of Current Platforms to Support Smart Healthcare. Program Comput Soft (2021) 47(8):578–90. doi: 10.1134/S0361768821080284
14. Abd-Alla HI, Souguir D, Radwan MO. Genus Sophora: A Comprehensive Review on Secondary Chemical Metabolites and Their Biological Aspects From Past Achievements to Future Perspectives. Arch Pharm Res (2021) 44(11):903–86. doi: 10.1007/s12272-021-01354-2
15. Rodríguez M, Alonso-Alonso R, Tomás-Roca L, Rodríguez-Pinilla SM, Manso-Alonso R, Cereceda L, et al. Peripheral T-Cell Lymphoma: Molecular Profiling Recognizes Subclasses and Identifies Prognostic Markers. Blood Adv (2021) 5(24):5588–98. doi: 10.1182/bloodadvances.2021005171
16. Mohammed Saleh MF, Kotb A, Abdallah GEM, Muhsen IN, El Fakih R, Aljurf M. Recent Advances in Diagnosis and Therapy of Angioimmunoblastic T Cell Lymphoma. Curr Oncol (2021) 28(6):5480–98. doi: 10.3390/curroncol28060456
17. Gopal V, Dubashi B, Kayal S, Penumadu P, Rajaram M, Karunanithi G, et al. Challenges in the Management of Lung Cancer: Real-World Experience From a Tertiary Center in South India. South Asian J Cancer (2021) n10(3):175–82. doi: 10.1055/s-0041-1733312
18. Ding Y, Lv B, Zheng J, Lu C, Liu J, Lei Y, et al. RBC-Hitchhiking Chitosan Nanoparticles Loading Methylprednisolone for Lung-Targeting Delivery. J Control Release (2021) 341:702–15. doi: 10.1016/j.jconrel.2021.12.018
19. Masini C, Gallegati D, Gentili N, Massa I, Ciucci R, Altini M. The Challenge of Sustainability of High-Cost Oncological Drugs: A Budgeting Model in an Italian Cancer Center. Int J Environ Res Public Health (2021) 18(24):13413. doi: 10.3390/ijerph182413413
20. Nkune NW, Abrahamse H. Nanoparticle-Based Drug Delivery Systems for Photodynamic Therapy of Metastatic Melanoma: A Review. Int J Mol Sci (2021) 22(22):12549. doi: 10.3390/ijms222212549
21. Ftouh M, Kalboussi N, Abid N, Sfar S, Mignet N, Bahloul B. Contribution of Nanotechnologies to Vaccine Development and Drug Delivery Against Respiratory Viruses. PPAR Res (2021) 2021:6741290. doi: 10.1155/2021/6741290
22. Brasseur K, Gévry N, Asselin E. Chemoresistance and Targeted Therapies in Ovarian and Endometrial Cancers. Oncotarget (2017) 8(3):4008–42. doi: 10.18632/oncotarget.14021
23. Patel A, Sant S. Hypoxic Tumor Microenvironment: Opportunities to Develop Targeted Therapies. Biotechnol Adv (2016) 34(5):803–12. doi: 10.1016/j.biotechadv.2016.04.005
24. Liu JJ, Chen Q, Feng L, Liu Z. Nanomedicine for Tumor Microenvironment Modulation and Cancer Treatment Enhancement. Nano Today (2018) 21:55–73. doi: 10.1016/j.nantod.2018.06.008
25. Estrella V, Chen T, Lloyd M, Wojtkowiak J, Cornnell HH, Ibrahim-Hashim A, et al. Acidity Generated by the Tumor Microenvironment Drives Local Invasion. Cancer Res (2013) 73(5):1524–35. doi: 10.1158/0008-5472.CAN-12-2796
26. Li X, Li H, Zhang ZY. Research Progress of Stimuli-Responsive Polymers in Tumor Immunotherapy. Prog Pharm Sci (2021) 45(5):325–36.
27. Manchun S, Dass CR, Sriamornsak P. Targeted Therapy for Cancer Using pH-Responsive Nanocarrier Systems. Life Sci (2012) 90(11-12):381–7. doi: 10.1016/j.lfs.2012.01.008
28. McMahon HT, Boucrot E. Molecular Mechanism and Physiological Functions of Clathrin-Mediated Endocytosis. Nat Rev Mol Cell Biol (2011) 12(8):517–33. doi: 10.1038/nrm3151
29. Qiu L, Qiao M, Chen Q, Tian C, Long M, Wang M, et al. Enhanced Effect of pH-Sensitive Mixed Copolymer Micelles for Overcoming Multidrug Resistance of Doxorubicin. Biomaterials (2014) 35(37):9877–87. doi: 10.1016/j.biomaterials.2014.08.008
30. Liu FH, Cong Y, Qi GB, Ji L, Qiao ZY, Wang H. Near-Infrared Laser-Driven In Situ Self-Assembly as a General Strategy for Deep Tumor Therapy. Nano Lett (2018) 18(10):6577–84. doi: 10.1021/acs.nanolett.8b03174
31. Alsehli M. Polymeric Nanocarriers as Stimuli-Responsive Systems for Targeted Tumor (Cancer) Therapy: Recent Advances in Drug Delivery. Saudi Pharm J (2020) 28(3):255–65. doi: 10.1016/j.jsps.2020.01.004
32. Ramírez-García PD, Retamal JS, Shenoy P, Imlach W, Sykes M, Truong N, et al. A pH-Responsive Nanoparticle Targets the Neurokinin 1 Receptor in Endosomes to Prevent Chronic Pain. Nat Nanotechnol (2019) 14(12):1150–9. doi: 10.1038/s41565-019-0568-x
33. Wang J, Byrne JD, Napier ME, DeSimone JM. More Effective Nanomedicines Through Particle Design. Small (2011) 7(14):1919–31. doi: 10.1002/smll.201100442
34. Asad MI, Khan D, Rehman AU, Elaissari A, Ahmed N. Development and In Vitro/In Vivo Evaluation of pH-Sensitive Polymeric Nanoparticles Loaded Hydrogel for the Management of Psoriasis. Nanomater (Basel) (2021) 11(12):3433. doi: 10.3390/nano11123433
35. Real DA, Bolaños K, Priotti J, Yutronic N, Kogan MJ, Sierpe R, et al. Cyclodextrin-Modified Nanomaterials for Drug Delivery: Classification and Advances in Controlled Release and Bioavailability. Pharmaceutics (2021) 13(12):2131. doi: 10.3390/pharmaceutics13122131
36. Kanamala M, Wilson WR, Yang M, Palmer BD, Wu Z. Mechanisms and Biomaterials in pH-Responsive Tumor-Targeted Drug Delivery: A Review. Biomaterials (2016) 85:152–67. doi: 10.1016/j.biomaterials.2016.01.061
37. Zhang W, Wang F, Wang Y, Wang J, Yu Y, Guo S, et al. pH and Near-Infrared Light Dual-Stimuli Responsive Drug Delivery Using DNA-Conjugated Gold Nanorods for Effective Treatment of Multidrug Resistant Cancer Cells. J Control Release (2016) 232:9–19. doi: 10.1016/j.jconrel.2016.04.001
38. Hong ST, Lin H, Wang CS, Chang CH, Lin AM, Yang JC, et al. Improving the Anticancer Effect of Afatinib and microRNA by Using Lipid Polymeric Nanoparticles Conjugated With Dual pH-Responsive and Targeting Peptides. J Nanobiotech (2019) 17(1):89. doi: 10.1186/s12951-019-0519-6
39. Ke W, Li J, Mohammed F, Wang Y, Tou K, Liu X, et al. Therapeutic Polymersome Nanoreactors With Tumor-Specific Activable Cascade Reactions for Cooperative Cancer Therapy. ACS Nano (2019) 13(2):2357–69. doi: 10.1021/acsnano.8b09082
40. Zhong Y, Huang S, Zheng C, Huang J, Li B, Han S, et al. A Light and Hypoxia-Activated Nanodrug for Cascade Photodynamic-Chemo Cancer Therapy. Biomater Sci (2021) 9(15):5218–26. doi: 10.1039/d1bm00660f
41. Xu C, Song R, Lu P, Chen J, Zhou Y, Shen G, et al. A pH-Responsive Charge-Reversal Drug Delivery System With Tumor-Specific Drug Release and ROS Generation for Cancer Therapy. Int J Nanomed (2020) 15:65–80. doi: 10.2147/IJN.S230237
42. Mendiratta S, Hussein M, Nasser HA, Ali AAA. Multidisciplinary Role of Mesoporous Silica Nanoparticles in Brain Regeneration and Cancers: From Crossing the Blood–Brain Barrier to Treatment. Part Part Syst Charact (2019) 36:1900195. doi: 10.1002/ppsc.201900195
43. Yan T, He J, Liu R, Liu Z, Cheng J. Chitosan Capped pH-Responsive Hollow Mesoporous Silica Nanoparticles for Targeted Chemo-Photo Combination Therapy. Carbohydr Polym (2020) 231:115706. doi: 10.1016/j.carbpol.2019.115706
44. He C, Lu K, Liu D, Lin W. Nanoscale Metal-Organic Frameworks for the Co-Delivery of Cisplatin and Pooled siRNAs to Enhance Therapeutic Efficacy in Drug-Resistant Ovarian Cancer Cells. J Am Chem Soc (2014) 136(14):5181–4. doi: 10.1021/ja4098862
45. Hu CM, Zhang L. Nanoparticle-Based Combination Therapy Toward Overcoming Drug Resistance in Cancer. Biochem Pharmacol (2012) 83(8):1104–11. doi: 10.1016/j.bcp.2012.01.008
46. Mattheolabakis G, Rigas B, Constantinides PP. Nanodelivery Strategies in Cancer Chemotherapy: Biological Rationale and Pharmaceutical Perspectives. Nanomedicine (Lond) (2012) 7(10):1577–90. doi: 10.2217/nnm.12.128
47. Cho K, Wang X, Nie S, Chen ZG, Shin DM. Therapeutic Nanoparticles for Drug Delivery in Cancer. Clin Cancer Res (2008) 14(5):1310–6. doi: 10.1158/1078-0432
48. Minko T. Soluble Polymer Conjugates for Drug Delivery. Drug Discov Today Technol (2005) 2(1):15–20. doi: 10.1016/j.ddtec.2005.05.005
49. Wu Z, Ma X, Ma Y, Yang Z, Yuan Y, Liu C. Core/Shell PEGS/HA Hybrid Nanoparticle Via Micelle-Coordinated Mineralization for Tumor-Specific Therapy. ACS Appl Mater Interf (2020) 12(10):12109–19. doi: 10.1021/acsami.0c00068
50. Zhao M, Wan S, Peng X, Zhang B, Pan Q, Li S, et al. Leveraging a Polycationic Polymer to Direct Tunable Loading of an Anticancer Agent and Photosensitizer With Opposite Charges for Chemo-Photodynamic Therapy. J Mater Chem B (2020) 8(6):1235–44. doi: 10.1039/c9tb02400j
51. Howaili F, Özliseli E, Küçüktürkmen B, Razavi SM, Sadeghizadeh M, Rosenholm JM. Stimuli-Responsive, Plasmonic Nanogel for Dual Delivery of Curcumin and Photothermal Therapy for Cancer Treatment. Front Chem (2021) 8:602941:602941. doi: 10.3389/fchem.2020.602941
52. Shi M, Zhang J, Huang Z, Chen Y, Pan S, Hu H, et al. Stimuli-Responsive Release and Efficient siRNA Delivery in Non-Small Cell Lung Cancer by a Poly(L-Histidine)-Based Multifunctional Nanoplatform. J Mater Chem B (2020) 8(8):1616–28. doi: 10.1039/c9tb02764e
53. Batten SR, Champness NR, Chen XM, Garcia-Martinez J, Kitagawa S, Ohrstrom L, et al. Terminology of Metal-Organic Frameworks and Coordination Polymers (IUPAC Recommendations 2013). Pure Appl Chermistry (2013) 85(8):1715–24. doi: 10.1351/PAC-REC-12-11-20
54. Shen J, Ma M, Zhang H, Yu H, Xue F, Hao N, et al. Microfluidics-Assisted Surface Trifunctionalization of a Zeolitic Imidazolate Framework Nanocarrier for Targeted and Controllable Multitherapies of Tumors. ACS. Appl Mater Interfaces (2020) 12(41):45838–49. doi: 10.1021/acsami.0c14021
55. Duan F, Feng X, Yang X, Sun W, Jin Y, Liu H, et al. A Simple and Powerful Co-Delivery System Based on pH-Responsive Metal-Organic Frameworks for Enhanced Cancer Immunotherapy. Biomaterials (2017) 122:23–33. doi: 10.1016/j.biomaterials.2017.01.017
56. Xia C, Yin S, Xu S, Ran G, Deng M, Mei L, et al. Low Molecular Weight Heparin-Coated and Dendrimer-Based Core-Shell Nanoplatform With Enhanced Immune Activation and Multiple Anti-Metastatic Effects for Melanoma Treatment. Theranostics (2019) 9(2):337–54. doi: 10.7150/thno.29026
57. Cai X, Luo Y, Zhang W, Du D, Lin Y. pH-Sensitive ZnO Quantum Dots-Doxorubicin Nanoparticles for Lung Cancer Targeted Drug Delivery. ACS. Appl Mater Interfaces (2016) 8(34):22442–50. doi: 10.1021/acsami.6b04933
58. Su Z, Liang Y, Yao Y, Wang T, Zhang N. Polymeric Complex Micelles Based on the Double-Hydrazone Linkage and Dual Drug-Loading Strategy for pH-Sensitive Docetaxel Delivery. J Mater Chem B (2016) 4(6):1122–33. doi: 10.1039/c5tb02188j
59. Liu J, Huang Y, Kumar A, Tan A, Jin S, Mozhi A, et al. PH-Sensitive Nano-Systems for Drug Delivery in Cancer Therapy. Biotechnol Adv (2014) 32(4):693–710. doi: 10.1016/j.biotechadv.2013.11.009
60. Deirram N, Zhang C, Kermaniyan SS, Johnston APR, Such GK. PH-Responsive Polymer Nanoparticles for Drug Delivery. Macromol Rapid Commun (2019) 40(10):e1800917. doi: 10.1002/marc.201800917
61. Koren E, Apte A, Jani A, Torchilin VP. Multifunctional PEGylated 2C5-Immunoliposomes Containing pH-Sensitive Bonds and TAT Peptide for Enhanced Tumor Cell Internalization and Cytotoxicity. J Control Release (2012) 160(2):264–73. doi: 10.1016/j.jconrel.2011.12.002
62. Yu Y, Zhang X, Qiu. The Anti-Tumor Efficacy of Curcumin When Delivered by Size/Charge-Changing Multistage Polymeric Micelles Based on Amphiphilic Poly (β-Amino Ester) Derivates. Biomaterials (2014) 35(10):3467–79. doi: 10.1016/j.biomaterials.2013.12.096
63. Yhaya F, Binauld S, Kim Y, Stenzel MH. Shell Cross-Linking of Cyclodextrin-Based Micelles via Supramolecular Chemistry for the Delivery of Drugs. Macromol Rapid Commun (2012) 33(21):1868–74. doi: 10.1002/marc.201200473
64. Pang X, Jiang Y, Xiao Q, Leung AW, Hua H, Xu C. PH-Responsive Polymer-Drug Conjugates: Design and Progress. J Control Release (2016) 28(222):116–29. doi: 10.1016/j.jconrel.2015.12.024
65. Kakizawa Y, Kataoka K. Block Copolymer Micelles for Delivery of Gene and Related Compounds. Adv Drug Deliv Rev (2002) 54(2):203–22. doi: 10.1016/s0169-409x(02)00017-0
66. Wu D, Pusuluri A, Vogus D, Krishnan V, Shields CW, Kim J, et al. Design Principles of Drug Combinations for Chemotherapy. J Control Release (2020) 10(323):36–46. doi: 10.1016/j.jconrel.2020.04.018
67. Zheng T, Wang W, Wu F, Zhang M, Shen J, Sun Y. Zwitterionic Polymer-Gated Au@TiO2 Core-Shell Nanoparticles for Imaging-Guided Combined Cancer Therapy. Theranostics (2019) 9(17):5035–48. doi: 10.7150/thno.35418
68. Wu WX, Wang N, Liu BY, Deng QF, Yu XQ. Lipase-Catalyzed Synthesis of Azido-Functionalized Aliphatic Polyesters Towards Acid-Degradable Amphiphilic Graft Copolymers. Soft Matter (2014) 10(8):1199–213. doi: 10.1039/c3sm52496e
69. Samanta P, Kapat K, Maiti S, Biswas G, Dhara S, Dhara D. pH-Labile and Photochemically Cross-Linkable Polymer Vesicles From Coumarin Based Random Copolymer for Cancer Therapy. J Colloid Interface Sci (2019) 555:132–44. doi: 10.1016/j.jcis.2019.07.069
70. Algar WR, Prasuhn DE, Stewart MH, Jennings TL, Blanco-Canosa JB, Dawson PE, et al. The Controlled Display of Biomolecules on Nanoparticles: A Challenge Suited to Bioorthogonal Chemistry. Bioconjug Chem (2011) 22(5):825–58. doi: 10.1021/bc200065z
71. Yoshida T, Lai TC, Kwon GS, Sako K. pH- and Ion-Sensitive Polymers for Drug Delivery. Expert Opin Drug Deliv (2013) 10(11):1497–513. doi: 10.1517/17425247.2013.821978
72. Greenfield RS, Kaneko T, Daues A, Edson MA, Fitzgerald KA, Olech LJ, et al. Evaluation In Vitro of Adriamycin Immunoconjugates Synthesized Using an Acid-Sensitive Hydrazone Linker. Cancer Res (1990) 50(20):6600–7.
73. Kaneko T, Willner D, Monkovíc I, Knipe JO, Braslawsky GR, Greenfield RS, et al. New Hydrazone Derivatives of Adriamycin and Their Immunoconjugates-a Correlation Between Acid Stability and Cytotoxicity. Bioconjug Chem (1991) 2(3):133–41. doi: 10.1021/bc00009a001
74. Etrych T, Kovář L, Strohalm J, Chytil P, Ríhová B, Ulbrich K. Biodegradable Star HPMA Polymer-Drug Conjugates: Biodegradability, Distribution and Anti-Tumor Efficacy. J Control Release (2011) 154(3):241–8. doi: 10.1016/j.jconrel.2011.06.015
75. Ríhová B, Etrych T, Sírová M, Kovár L, Hovorka O, Kovár M, et al. Synergistic Action of Doxorubicin Bound to the Polymeric Carrier Based on N-(2-Hydroxypropyl) Methacrylamide Copolymers Through an Amide or Hydrazone Bond. Mol Pharm (2010) 7(4):1027–40. doi: 10.1021/mp100121g
76. Long W, Ouyang H, Zhou C, Wan W, Yu S, Qian K, et al. Simultaneous Surface Functionalization and Drug Loading: A Novel Method for Fabrication of Cellulose Nanocrystals-Based pH-Responsive Drug Delivery System. Int J Biol Macromol (2021) 1(182):2066–75. doi: 10.1016/j.ijbiomac.2021.05.193
77. Sonawane SJ, Kalhapure RS, Govender T. Hydrazone Linkages in pH-Responsive Drug Delivery Systems. Eur J Pharm Sci (2017) 1(99):45–65. doi: 10.1016/j.ejps.2016.12.011
78. Alani AW, Bae Y, Rao DA, Kwon GS. Polymeric Micelles for the pH-Dependent Controlled, Continuous Low Dose Release of Paclitaxel. Biomaterials (2010) 31(7):1765–72. doi: 10.1016/j.biomaterials.2009.11.038
79. Aryal S, Hu CM, Zhang L. Polymer-Cisplatin Conjugate Nanoparticles for Acid-Responsive Drug Delivery. ACS Nano (2010) 4(1):251–8. doi: 10.1021/nn9014032
80. Li H, Li M, Chen C, Fan A, Kong D, Wang Z, et al. On-Demand Combinational Delivery of Curcumin and Doxorubicin via a pH-Labile Micellar Nanocarrier. Int J Pharm (2015) 495(1):572–8. doi: 10.1016/j.ijpharm.2015.09.022
81. Wang Z, Chen C, Zhang Q, Gao M, Zhang J, Kong D, et al. Tuning the Architecture of Polymeric Conjugate to Mediate Intracellular Delivery of Pleiotropic Curcumin. Eur J Pharm Biopharm (2015) 90:53–62. doi: 10.1016/j.ejpb.2014.11.002
82. Zhang P, Kong J. Doxorubicin-Tethered Fluorescent Silica Nanoparticles for pH-Responsive Anticancer Drug Delivery. Talanta (2015) 134:501–7. doi: 10.1016/j.talanta.2014.09.041
83. Tauk L, Schröder AP, Decher G, Giuseppone N. Hierarchical Functional Gradients of pH-Responsive Self-Assembled Monolayers Using Dynamic Covalent Chemistry on Surfaces. Nat Chem (2009) 1(8):649–56. doi: 10.1038/nchem.400
84. Rowan SJ, Cantrill SJ, Cousins GR, Sanders JK, Stoddart JF. Dynamic Covalent Chemistry. Angew Chem Int Ed Engl (2002) 41(6):898–952. doi: 10.1002/1521-3773(20020315)41:6<898::aid-anie898>3.0.co;2-e
85. Kurita K. Controlled Functionalization of the Polysaccharide Chitin. Prog In Polymer Sci (2001) 26(9):1921–71. doi: 10.1016/S0079-6700(01)00007-7
86. Masson C, Garinot M, Mignet N, Wetzer B, Mailhe P, Scherman D, et al. pH-Sensitive PEG Lipids Containing Orthoester Linkers: New Potential Tools for Nonviral Gene Delivery. J Control Release (2004) 99(3):423–34. doi: 10.1016/j.jconrel.2004.07.016
87. Engel AK, Yoden T, Sanui K, Ogata N. Synthesis of Aromatic Schiff Base Oligomers at the Air/Water Interface. J Am Chem Society (1985) 107(26):8308–10. doi: 10.1021/ja00312a108
88. Liao SC, Ting CW, Chiang WH. Functionalized Polymeric Nanogels With pH-Sensitive Benzoic-Imine Cross-Linkages Designed as Vehicles for Indocyanine Green Delivery. J Colloid Interface Sci (2020) 561:11–22. doi: 10.1016/j.jcis.2019.11.109
89. Binauld S, Stenzel MH. Acid-Degradable Polymers for Drug Delivery: A Decade of Innovation. Chem Commun (Camb) (2013) 49(21):2082–102. doi: 10.1039/c2cc36589h
90. Nuhn L, Van Herck S, Best A, Deswarte K, Kokkinopoulou M, Lieberwirth I, et al. FRET Monitoring of Intracellular Ketal Hydrolysis in Synthetic Nanoparticles. Angew Chem Int Ed Engl (2018) 57(33):10760–4. doi: 10.1002/anie.201803847
91. Zhao C, Cao W, Zheng H, Xiao Z, Hu J, Yang L, et al. Acid-Responsive Nanoparticles as a Novel Oxidative Stress-Inducing Anticancer Therapeutic Agent for Colon Cancer. Int J Nanomed (2019) 14:1597–618. doi: 10.2147/IJN.S189923
92. Szabó I, Manea M, Orbán E, Csámpai A, Bosze S, Szabó R, et al. Development of an Oxime Bond Containing Daunorubicin-Gonadotropin-Releasing Hormone-III Conjugate as a Potential Anticancer Drug. Bioconjug Chem (2009) 20(4):656–65. doi: 10.1021/bc800542u
93. Kölmel DK, Kool ET. Oximes and Hydrazones in Bioconjugation: Mechanism and Catalysis. Chem Rev (2017) 117(15):10358–76. doi: 10.1021/acs.chemrev.7b00090
94. Jin Y, Song L, Su Y, Zhu L, Pang Y, Qiu F, et al. Oxime Linkage: A Robust Tool for the Design of pH-Sensitive Polymeric Drug Carriers. Biomacromolecules (2011) 12(10):3460–8. doi: 10.1021/bm200956u
95. Singh GS. Recent Progress in the Synthesis and Chemistry of Azetidinones. Tetrahedron (2003) 59(39):7631–49. doi: 10.1016/S0040-4020(03)01099-8
96. Curtius TH. Synthetische Versuche Mit Hippurazid. Berichte der deutschen chemischen Gesellschaft (1902) 35(3):3226–8. doi: 10.1002/cber.190203503125
97. Chan WK, Ho CM, Wong MK, Che CM. Oxidative Amide Synthesis and N-Terminal Alpha-Amino Group Ligation of Peptides in Aqueous Medium. J Am Chem Soc (2006) 128(46):14796–7. doi: 10.1021/ja064479s
98. Shao WY, Liu CR, Ma HJ, Hong Z, Xie QL, Lu YH. Fabrication of pH-Sensitive Thin-Film Nanocomposite Nanofiltration Membranes With Enhanced Performance by Incorporating Amine-Functionalized Graphene Oxide. Appl Surface Sci (2019) 487:1209–21. doi: 10.1016/j.apsusc.2019.05.157
99. Cai S, Thati S, Bagby TR, Diab HM, Davies NM, Cohen MS, et al. Localized Doxorubicin Chemotherapy With a Biopolymeric Nanocarrier Improves Survival and Reduces Toxicity in Xenografts of Human Breast Cancer. J Control Release (2010) 146(2):212–8. doi: 10.1016/j.jconrel.2010.04.006
100. Ofridam F, Tarhini M, Lebaz N, Gagniere E, Mangin D, Elaissari A. pH-Sensitive Polymers: Classification and Some Fine Potential Applications. Polymers Advanced Technologies (2021) 32(4):1455–84. doi: 10.1002/pat.5230
101. Huang X, Cao J, Zhang Y, Liu T, Yan H. Polyethylenimine Modified With 2,3-Dimethylmaleic Anhydride Potentiates the Antitumor Efficacy of Conventional Chemotherapy. Mater Sci Eng C Mater Biol Appl (2019) 102:558–68. doi: 10.1016/j.msec.2019.04.081
102. Luo F, Fan Z, Yin W, Yang L, Li T, Zhong L, et al. pH-Responsive Stearic Acid-O-Carboxymethyl Chitosan Assemblies as Carriers Delivering Small Molecular Drug for Chemotherapy. Mater Sci Eng C Mater Biol Appl (2019) 105:110107. doi: 10.1016/j.msec.2019.110107
103. Heller J, Barr J, Ng SY, Abdellauoi KS, Gurny R. Poly (Orthoesters), Synthesis, Characterization, Properties, and Uses. Adv Drug Deliv Rev (2002) 54(7):1015–39. doi: 10.1016/s0169-409x(02)00055-8
104. Tang R, Ji W, Wang C. Amphiphilic Block Copolymers Bearing Ortho Ester Side-Chains: pH-Dependent Hydrolysis and Self-Assembly in Water. Macromol Biosci (2010) 10(2):192–201. doi: 10.1002/mabi.200900229
105. Thambi T, Deepagan VG, Yoo CK, Park JH. Synthesis and Physicochemical Characterization of Amphiphilic Block Copolymers Bearing Acid-Sensitive Orthoester Linkage as the Drug Carrier. POLYMER (2011) 52(21):4753–9. doi: 10.1016/j.polymer.2011.08.024
106. Dan K, Pan R, Ghosh S. Aggregation and pH-Responsive Disassembly of a New Acid-Labile Surfactant Synthesized by Thiol-Acrylate Michael Addition Reaction. Langmuir (2011) 27(2):612–7. doi: 10.1021/la104445h
107. Dan K, Ghosh S. One-Pot Synthesis of an Acid-Labile Amphiphilic Triblock Copolymer and its pH-Responsive Vesicular Assembly. Angew Chem Int Ed Engl (2013) 52(28):7300–5. doi: 10.1002/anie.201302722
108. Molla MR, Marcinko T, Prasad P, Deming D, Garman SC, Thayumanavan S. Unlocking a Caged Lysosomal Protein From a Polymeric Nanogel With a pH Trigger. Biomacromolecules (2014) 15(11):4046–53. doi: 10.1021/bm501091p
109. Nishiyabu R, Kubo Y, James TD, Fossey JS. Boronic Acid Building Blocks: Tools for Self-Assembly. Chem Commun (Camb) (2011) 47(4):1124–50. doi: 10.1039/c0cc02921a
110. He L, Fullenkamp DE, Rivera JG, Messersmith PB. pH-Responsive Self-Healing Hydrogels Formed by Boronate-Catechol Complexation. Chem Commun (Camb) (2011) 47(26):7497–9. doi: 10.1039/c1cc11928a
111. Liang E, Zhou H, Ding X, Zheng Z, Peng Y. Fabrication of a Rhythmic Assembly System Based on Reversible Formation of Dynamic Covalent Bonds in a Chemical Oscillator. Chem Commun (Camb) (2013) 49(47):5384–6. doi: 10.1039/c3cc42533a
112. Fang L, Zhang W, Wang Z, Fan X, Cheng Z, Hou X, et al. Novel Mitochondrial Targeting Charge-Reversal Polysaccharide Hybrid Shell/Core Nanoparticles for Prolonged Systemic Circulation and Antitumor Drug Delivery. Drug Deliv (2019) 26(1):1125–39. doi: 10.1080/10717544.2019.1687614
113. Page DB, Postow MA, Callahan MK, Allison JP, Wolchok JD. Immune Modulation in Cancer With Antibodies. Annu Rev Med (2013) 2014(65):185–202. doi: 10.1146/annurev-med-092012-112807
114. Oupicky D, Ogris M, Howard KA, Dash PR, Ulbrich K, Seymour LW. Importance of Lateral and Steric Stabilization of Polyelectrolyte Gene Delivery Vectors for Extended Systemic Circulation. Mol Ther (2002) 5(4):463–72. doi: 10.1006/mthe.2002.0568
115. He C, Hu Y, Yin L, Tang C, Yin C. Effects of Particle Size and Surface Charge on Cellular Uptake and Biodistribution of Polymeric Nanoparticles. Biomaterials (2010) 31(13):3657–66. doi: 10.1016/j.biomaterials.2010.01.065
116. Shen Y, Zhou Z, Sui M, Tang J, Xu P, Van Kirk EA, et al. Charge-Reversal Polyamidoamine Dendrimer for Cascade Nuclear Drug Delivery. Nanomedicine (Lond) (2010) 5(8):1205–17. doi: 10.2217/nnm.10.86
117. Ji T, Zhao Y, Ding Y, Nie G. Using Functional Nanomaterials to Target and Regulate the Tumor Microenvironment: Diagnostic and Therapeutic Applications. Adv Mater (2013) 25(26):3508–25. doi: 10.1002/adma.201300299
118. Zarrin A, Foroozesh M, Hamidi M. Carrier Erythrocytes: Recent Advances, Present Status, Current Trends, and Future Horizons. Expert Opin Drug Deliv (2014) 11(3):433–47. doi: 10.1517/17425247.2014.880422
119. Arvizo RR, Miranda OR, Thompson MA, Pabelick CM, Bhattacharya R, Robertson JD, et al. Effect of Nanoparticle Surface Charge at the Plasma Membrane and Beyond. Nano Lett (2010) 10(7):2543–8. doi: 10.1021/nl101140t
120. Cho EC, Xie J, Wurm PA, Xia Y. Understanding the Role of Surface Charges in Cellular Adsorption Versus Internalization by Selectively Removing Gold Nanoparticles on the Cell Surface With a I2/KI Etchant. Nano Lett (2009) 9(3):1080–4. doi: 10.1021/nl803487r
121. Guo S, Lin CM, Xu Z, Miao L, Wang Y, Huang L. Co-Delivery of Cisplatin and Rapamycin for Enhanced Anticancer Therapy Through Synergistic Effects and Microenvironment Modulation. ACS. Nano (2014) 8(5):4996–5009. doi: 10.1021/nn5010815
122. Wong AS, Mann SK, Czuba E, Sahut A, Liu H, Suekama TC, et al. Self-Assembling Dual Component Nanoparticles With Endosomal Escape Capability. Soft Matter (2015) 11(15):2993–3002. doi: 10.1039/c5sm00082c
123. Huang Y, Tang Z, Zhang X, Yu H, Sun H, Pang X, et al. pH-Triggered Charge-Reversal Polypeptide Nanoparticles for Cisplatin Delivery: Preparation and In Vitro Evaluation. Biomacromolecules (2013) 14(6):2023–32. doi: 10.1021/bm400358z
124. Fang L, Fan H, Guo C, Cui L, Zhang P, Mu H, et al. Novel Mitochondrial Targeting Multifunctional Surface Charge-Reversal Polymeric Nanoparticles for Cancer Treatment. J BioMed Nanotechnol (2019) 15(11):2151–63. doi: 10.1166/jbn.2019.2854
125. Chen DQ, Song XY, Wang KL, Guo CJ, Yu YM, Fan HY, et al. Design and Evaluation of Dual CD44 Receptor and Folate Receptor-Targeting Double-Smart pH-Response Multifunctional Nanocarrier. J Nanoparticle Res (2017) 19(12):400. doi: 10.1007/s11051-017-4076-3
126. Liang G, Zhu Y, Jing A, Wang J, Hu F, Feng W, et al. Cationic microRNA-Delivering Nanocarriers for Efficient Treatment of Colon Carcinoma in Xenograft Model. Gene Ther (2016) 23(12):829–38. doi: 10.1038/gt.2016.60
127. Han K, Zhang J, Zhang W, Wang S, Xu L, Zhang C, et al. Tumor-Triggered Geometrical Shape Switch of Chimeric Peptide for Enhanced In Vivo Tumor Internalization and Photodynamic Therapy. ACS. Nano (2017) 11(3):3178–88. doi: 10.1021/acsnano.7b00216
128. Luo YL, Yu W, Xu F. pH-Responsive PMAA-B-PEG-B-PMAA Triblock Copolymer Micelles for Prednisone Drug Release and Release Kinetics. Polymer Bulletin (2012) 69(5):597–620. doi: 10.1007/s00289-012-0774-2
129. Abbasian M, Roudi MM, Mahmoodzadeh F, Eskandani M, Jaymand M. Chitosan-Grafted-Poly (Methacrylic Acid)/Graphene Oxide Nanocomposite as a pH-Responsive De Novo Cancer Chemotherapy Nanosystem. Int J Biol Macromol (2018) 118:1871–9. doi: 10.1016/j.ijbiomac.2018.07.036
130. Zhao Y, Su HJ, Fang L, Tan TW. Superabsorbent Hydrogels From Poly (Aspartic Acid) With Salt-, Temperature- and pH-Responsiveness Properties. Polymer (2005) 46(14):5368–76. doi: 10.1016/j.polymer.2005.04.015
131. Abel BA, Sims MB, McCormick CL. Tunable pH- and CO2-Responsive Sulfonamide-Containing Polymers by RAFT Polymerization. Macromolecules (2015) 48(16):5487–95. doi: 10.1021/acs.macromol.5b01453
132. Kang HC, Bae YH. pH-Tunable Endosomolytic Oligomers for Enhanced Nucleic Acid Delivery. Advanced Funct Materials (2007) 17(8):1263–72. doi: 10.1002/adfm.200601188
133. Fu X, Hosta-Rigau L, Chandrawati R, Cui JW. Multi-Stimuli-Responsive Polymer Particles, Films, and Hydrogels for Drug Delivery. Chem (2018) 4(9):2084–107. doi: 10.1016/j.chempr.2018.07.00
134. Cao ZQ, Wang GJ. Multi-Stimuli-Responsive Polymer Materials: Particles, Films, and Bulk Gels. Chem Rec (2016) 16(3):1398–435. doi: 10.1002/tcr.201500281
135. Cheng R, Meng FH, Deng C, Zhong ZY. Bioresponsive Polymeric Nanotherapeutics for Targeted Cancer Chemotherapy. Nano Today (2015) 10(5):656–70. doi: 10.1016/j.nantod.2015.09.005
136. Gribble FM, Loussouarn G, Tucker SJ, Zhao C, Nichols CG, Ashcroft FM. A Novel Method for Measurement of Submembrane ATP Concentration. J Biol Chem (2000) 275(39):30046–9. doi: 10.1074/jbc.M001010200
137. Gorman MW, Feigl EO, Buffington CW. Human Plasma ATP Concentration. Clin Chem (2007) 53(2):318–25. doi: 10.1373/clinchem.2006.076364
138. Deng B, Ma P, Xie Y. Reduction-Sensitive Polymeric Nanocarriers in Cancer Therapy: A Comprehensive Review. Nanoscale (2015) 7(30):12773–95. doi: 10.1039/c5nr02878g
139. Ganta S, Devalapally H, Shahiwala A, Amiji MA. Review of Stimuli-Responsive Nanocarriers for Drug and Gene Delivery. J Control Release (2008) 126(3):187–204. doi: 10.1016/j.jconrel.2007.12.017
140. Song Q, Jia J, Niu X, Zheng C, Zhao H, Sun L, et al. An Oral Drug Delivery System With Programmed Drug Release and Imaging Properties for Orthotopic Colon Cancer Therapy. Nanoscale (2019) 11(34):15958–70. doi: 10.1039/c9nr03802g
141. Kim SG, Ryplida B, Phuong PTM, Won HJ, Lee G, Bhang SH, et al. Reduction-Triggered Paclitaxel Release Nano-Hybrid System Based on Core-Crosslinked Polymer Dots With a pH-Responsive Shell-Cleavable Colorimetric Biosensor. Int J Mol Sci (2019) 20(21):5368. doi: 10.3390/ijms20215368
142. Jäger E, Humajová J, Dölen Y, Kučka J, Jäger A, Konefał R, et al. Enhanced Antitumor Efficacy Through an "AND Gate" Reactive Oxygen-Species-Dependent pH-Responsive Nanomedicine Approach. Adv Healthc Mater (2021) 10(13):e2100304. doi: 10.1002/adhm.202100304
143. Wang T, Wang F, Sun M, Tian M, Mu Y, Chen X, et al. Gastric Environment-Stable Oral Nanocarriers for In Situ Colorectal Cancer Therapy. Int J Biol Macromol (2019) 139:1035–45. doi: 10.1016/j.ijbiomac.2019.08.088
144. Chen L, Qian M, Jiang H, Zhou Y, Du Y, Yang Y, et al. Multifunctional Mesoporous Black Phosphorus-Based Nanosheet for Enhanced Tumor-Targeted Combined Therapy With Biodegradation-Mediated Metastasis Inhibition. Biomaterials (2020) 236:119770. doi: 10.1016/j.biomaterials.2020.119770
145. Feng Y, Cheng Y, Chang Y, Jian H, Zheng R, Wu X, et al. Time-Staggered Delivery of Erlotinib and Doxorubicin by Gold Nanocages With Two Smart Polymers for Reprogrammable Release and Synergistic With Photothermal Therapy. Biomaterials (2019) 217:119327. doi: 10.1016/j.biomaterials.2019.119327
146. Lin W, Zhang W, Liu S, Li Z, Hu X, Xie Z, et al. Engineering pH-Responsive BODIPY Nanoparticles for Tumor Selective Multimodal Imaging and Phototherapy. ACS. Appl Mater Interfaces (2019) 11(47):43928–35. doi: 10.1021/acsami.9b16403
147. Don TM, Lu KY, Lin LJ, Hsu CH, Wu JY, Mi FL. Temperature/pH/Enzyme Triple-Responsive Cationic Protein/PAA-B-PNIPAAm Nanogels for Controlled Anticancer Drug and Photosensitizer Delivery Against Multidrug Resistant Breast Cancer Cells. Mol Pharm (2017) 14(12):4648–60. doi: 10.1021/acs.molpharmaceut.7b00737
148. Kim H, Sehgal D, Kucaba TA, Ferguson DM, Griffith TS, Panyam J. Acidic pH-Responsive Polymer Nanoparticles as a TLR7/8 Agonist Delivery Platform for Cancer Immunotherapy. Nanoscale (2018) 10(44):20851–62. doi: 10.1039/c8nr07201a
149. Sun Q, Ma X, Zhang B, Zhou Z, Jin E, Shen Y, et al. Fabrication of Dendrimer-Releasing Lipidic Nanoassembly for Cancer Drug Delivery. Biomater Sci (2016) 4(6):958–69. doi: 10.1039/c6bm00189k
150. Langer R, Folkman J. Polymers for the Sustained Release of Proteins and Other Macromolecules. Nature (1976) 263(5580):797–800. doi: 10.1038/263797a0
151. Fischel-Ghodsian F, Brown L, Mathiowitz E, Brandenburg D, Langer R. Enzymatically Controlled Drug Delivery. Proc Natl Acad Sci USA (1988) 85(7):2403–6. doi: 10.1073/pnas.85.7.2403
152. Mo R, Gu Z. Tumor Microenvironment and Intracellular Signal-Activated Nanomaterials for Anticancer Drug Delivery. Materials Today (2016) 19(5):274–83. doi: 10.1016/j.mattod.2015.11.025
153. Traitel T, Goldbart R, Kost J. Smart Polymers for Responsive Drug-Delivery Systems. J Biomater Sci Polym Ed (2008) 19(6):755–67. doi: 10.1163/156856208784522065
154. Li Z, Yin Q, Chen B, Wang Z, Yan Y, Qi T, et al. Ultra-pH-Sensitive Indocyanine Green-Conjugated Nanoprobes for Fluorescence Imaging-Guided Photothermal Cancer Therapy. Nanomedicine (2019) 17:287–96. doi: 10.1016/j.nano.2019.02.001
155. Li S, Saw PE, Lin C, Nie Y, Tao W, Farokhzad OC, et al. Redox-Responsive Polyprodrug Nanoparticles for Targeted siRNA Delivery and Synergistic Liver Cancer Therapy. Biomaterials (2020) 234:119760. doi: 10.1016/j.biomaterials.2020.119760
156. Camacho KM, Kumar S, Menegatti S, Vogus DR, Anselmo AC, Mitragotri S. Synergistic Antitumor Activity of Camptothecin-Doxorubicin Combinations and Their Conjugates With Hyaluronic Acid. J Control Release (2015) 210:198–207. doi: 10.1016/j.jconrel.2015.04.031
157. Wang N, Liu C, Yao W, Zhou H, Yu S, Chen H, et al. A Traceable, GSH/pH Dual-Responsive Nanoparticles With Spatiotemporally Controlled Multiple Drugs Release Ability to Enhance Antitumor Efficacy. Colloids Surf. B Biointerfaces (2021) 205:111866. doi: 10.1016/j.colsurfb.2021.111866
158. Saucier-Sawyer JK, Seo YE, Gaudin A, Quijano E, Song E, Sawyer AJ, et al. Distribution of Polymer Nanoparticles by Convection-Enhanced Delivery to Brain Tumors. J Control Release (2016) 232:103–12. doi: 10.1016/j.jconrel.2016.04.006
159. Shirvalilou S, Khoei S, Khoee S, Mahdavi SR, Raoufi NJ, Motevalian M, et al. Enhancement Radiation-Induced Apoptosis in C6 Glioma Tumor-Bearing Rats via pH-Responsive Magnetic Graphene Oxide Nanocarrier. J Photochem Photobiol B (2020) 205:111827. doi: 10.1016/j.jphotobiol.2020.111827
160. Song W, Musetti SN, Huang L. Nanomaterials for Cancer Immunotherapy. Biomaterials (2017) 148:16–30. doi: 10.1016/j.biomaterials.2017.09.017
161. Liang R, Xie J, Li J, Wang K, Liu L, Gao Y, et al. Liposomes-Coated Gold Nanocages With Antigens and Adjuvants Targeted Delivery to Dendritic Cells for Enhancing Antitumor Immune Response. Biomaterials (2017) 149:41–50. doi: 10.1016/j.biomaterials.2017.09.029
162. Liu XY, Wang XY, Li RY, Jia SC, Sun P, Zhao M, et al. Recent Progress in the Understanding of Complement Activation and Its Role in Tumor Growth and Anti-Tumor Therapy. BioMed Pharmacother (2017) 91:446–56. doi: 10.1016/j.biopha.2017.04.101
163. Silva AL, Peres C, Conniot J, Matos AI, Moura L, Carreira B, et al. Nanoparticle Impact on Innate Immune Cell Pattern-Recognition Receptors and Inflammasomes Activation. Semin Immunol (2017) 34:3–24. doi: 10.1016/j.smim.2017.09.003
164. Moghimi SM, Simberg D. Complement Activation Turnover on Surfaces of Nanoparticles. Nano Today (2017) 15:8–10. doi: 10.1016/j.nantod.2017.03.001
165. Wells LA, Guo H, Emili A, Sefton MV. The Profile of Adsorbed Plasma and Serum Proteins on Methacrylic Acid Copolymer Beads: Effect on Complement Activation. Biomaterials (2017) 118:74–83. doi: 10.1016/j.biomaterials.2016.11.036
166. Li C, Lu Y, Chen Q, Hu H, Zhao X, Qiao M, et al. Tailored Polymers With Complement Activation Ability to Improve Antitumor Immunity. Mol Pharm (2019) 16(6):2648–60. doi: 10.1021/acs.molpharmaceut.9b00195
167. Zhao Y, Zhou Y, Wang D, Gao Y, Li J, Ma S, et al. pH-Responsive Polymeric Micelles Based on Poly(2-Ethyl-2-Oxazoline)-Poly(D,L-Lactide) for Tumor-Targeting and Controlled Delivery of Doxorubicin and P-Glycoprotein Inhibitor. Acta Biomater (2015) 17:182–92. doi: 10.1016/j.actbio.2015.01.010
168. Gao Y, Zhou Y, Zhao L, Zhang C, Li Y, Li J, et al. Enhanced Antitumor Efficacy by Cyclic RGDyK-Conjugated and Paclitaxel-Loaded pH-Responsive Polymeric Micelles. Acta Biomater (2015) 23:127–35. doi: 10.1016/j.actbio.2015.05.021
169. Zhang ZL, Zou WG, Luo CX, Li BH, Wang JH, Sun LY, et al. An Armed Oncolytic Adenovirus System, ZD55-Gene, Demonstrating Potent Antitumoral Efficacy. Cell Res (2003) 13(6):481–9. doi: 10.1038/sj.cr.7290191
170. Masiero M, Nardo G, Indraccolo S, Favaro E. RNA Interference: Implications for Cancer Treatment. Mol Aspects Med (2007) 28(1):143–66. doi: 10.1016/j.mam.2006.12.004
171. Zhang L, Huang J, Yang N, Greshock J, Megraw MS, Giannakakis A, et al. microRNAs Exhibit High Frequency Genomic Alterations in Human Cancer. Proc Natl Acad Sci USA (2006) 103(24):9136–41. doi: 10.1073/pnas.0508889103
172. Tian H, Lin L, Jiao Z, Guo Z, Chen J, Gao S, et al. Polylysine-Modified Polyethylenimine Inducing Tumor Apoptosis as an Efficient Gene Carrier. J Control Release (2013) 172(2):410–8. doi: 10.1016/j.jconrel.2013.06.026
173. Liang L, Chen Y, Chen XM, Zhang Y, Liu Y. Cyclodextrin/polyethylenimine-Based Supramolecular Nanoparticles for Loading and Sustained Release of ATP. Chem Letters (2018) 29(6):989–91. doi: 10.1016/j.cclet.2017.12.022
174. Chen J, Guo Z, Jiao Z, Lin L, Xu C, Tian H, et al. Poly (L-Glutamic Acid)-Based Zwitterionic Polymer in a Charge Conversional Shielding System for Gene Therapy of Malignant Tumors. ACS. Appl Mater Interfaces (2020) 12(17):19295–306. doi: 10.1021/acsami.0c02769
175. Kelly JF, Snell ME, Berenbaum MC. Photodynamic Destruction of Human Bladder Carcinoma. Br J Cancer (1975) 31(2):237–44. doi: 10.1038/bjc.1975.30
176. Li C, Luo Z, Yang L, Chen J, Cheng K, Xue Y, et al. Self-Assembled Porphyrin Polymer Nanoparticles With NIR-II Emission and Highly Efficient Photothermal Performance in Cancer Therapy. Mater Today Bio (2021) 13:100198. doi: 10.1016/j.mtbio.2021.100198
177. Li X, Yuan HJ, Tian XM, Tang J, Liu LF, Liu FY. Biocompatible Copper Sulfide-Based Nanocomposites for Artery Interventional Chemo-Photothermal Therapy of Orthotropic Hepatocellular Carcinoma. Mater Today Bio (2021) 12:100128. doi: 10.1016/j.mtbio.2021.100128
178. Wang JL, Sui LH, Huang J, Miao L, Nie Y, Wang KS, et al. MoS2-Based Nanocomposites for Cancer Diagnosis and Therapy. Bioactive Materials (2021) 6(11):4209–42. doi: 10.1016/j.bioactmat.2021.04.021
179. Li Z, Liu Y, Chen L, Hu X, Xie Z. A Glutathione-Activatable Photodynamic and Fluorescent Imaging Monochromatic Photosensitizer. J Mater Chem B (2017) 5(22):4239–45. doi: 10.1039/c7tb00724h
180. He DG, Hai L, He X, Yang X, Li HW. Glutathione-Activatable and O-2/Mn2+-Evolving Nanocomposite for Highly Efficient and Selective Photodynamic and Gene-Silencing Dual Therapy. Funct Materials (2017) 27(46):1704089. doi: 10.1002/adfm.201704089
181. Ma Z, Hu P, Guo C, Wang D, Zhang X, Chen M, et al. Folate-Mediated and pH-Responsive Chidamide-Bound Micelles Encapsulating Photosensitizers for Tumor-Targeting Photodynamic Therapy. Int J Nanomed (2019) 14:5527–40. doi: 10.2147/IJN.S208649
182. Chen Q, Xu L, Liang C, Wang C, Peng R, Liu Z. Photothermal Therapy With Immune-Adjuvant Nanoparticles Together With Checkpoint Blockade for Effective Cancer Immunotherapy. Nat Commun (2016) 7:13193. doi: 10.1038/ncomms13193
183. Zhu L, Li P, Gao D, Liu J, Liu Y, Sun C, et al. pH-Sensitive Loaded Retinal/Indocyanine Green Micelles as an "All-in-One" Theranostic Agent for Multi-Modal Imaging In Vivo Guided Cellular Senescence-Photothermal Synergistic Therapy. Chem Commun (Camb) (2019) 55(44):6209–12. doi: 10.1039/c9cc02567g
184. Yoon HJ, Lee HS, Lim JY, Park JH. Liposomal Indocyanine Green for Enhanced Photothermal Therapy. ACS. Appl Mater Interfaces (2017) 9(7):5683–91. doi: 10.1021/acsami.6b16801
185. Liu R, Tang J, Xu Y, Zhou Y, Dai Z. Nano-Sized Indocyanine Green J-Aggregate as a One-Component Theranostic Agent. Nanotheranostics (2017) 1(4):430–9. doi: 10.7150/ntno.19935
186. Ting CW, Chou YH, Huang SY, Chiang WH. Indocyanine Green-Carrying Polymeric Nanoparticles With Acid-Triggered Detachable PEG Coating and Drug Release for Boosting Cancer Photothermal Therapy. Colloids Surf B Biointerfaces (2021) 208:112048. doi: 10.1016/j.colsurfb.2021.112048
187. Du JZ, Du XJ, Mao CQ, Wang J. Tailor-Made Dual pH-Sensitive Polymer-Doxorubicin Nanoparticles for Efficient Anticancer Drug Delivery. J Am Chem Soc (2011) 133(44):17560–3. doi: 10.1021/ja207150n
188. Danquah MK, Zhang XA, Mahato RI. Extravasation of Polymeric Nanomedicines Across Tumor Vasculature. Adv Drug Deliv Rev (2011) 63(8):623–39. doi: 10.1016/j.addr.2010.11.005
189. Karve S, Werner ME, Sukumar R, Cummings ND, Copp JA, Wang EC, et al. Revival of the Abandoned Therapeutic Wortmannin by Nanoparticle Drug Delivery. Proc Natl Acad Sci USA (2012) 109(21):8230–5. doi: 10.1073/pnas.1120508109
190. Yoon HY, Koo H, Choi KY, Lee SJ, Kim K, Kwon IC, et al. Tumor-Targeting Hyaluronic Acid Nanoparticles for Photodynamic Imaging and Therapy. Biomaterials (2012) 33(15):3980–9. doi: 10.1016/j.biomaterials.2012.02.016
191. Mauceri HJ, Hanna NN, Beckett MA, Gorski DH, Staba MJ, Stellato KA, et al. Combined Effects of Angiostatin and Ionizing Radiation in Antitumour Therapy. Nature (1998) 394(6690):287–91. doi: 10.1038/28412
192. Bejjanki NK, Zhong Y, Liu J, Li Q, Xu H, Shen H, et al. Surface Charge Transition Nano-Theranostics Based on Ultra-Small Fe3O4 Nanoparticles for Enhanced Photodynamic and Photothermal Therapy Against Nasopharyngeal Carcinoma. Biochem Biophys Res Commun (2021) 557:240–6. doi: 10.1016/j.bbrc.2021.03.168
193. Gong NQ, Ma XW, Ye XX, Zhou QF, Chen XA, Tan XL, et al. Carbon-Dot-Supported Atomically Dispersed Gold as a Mitochondrial Oxidative Stress Amplifier for Cancer Treatment. Nat Nanotechnol (2019) 14(4):379–87. doi: 10.1038/s41565-019-0373-6
194. Ding YL, Liu FT, Jiang QH, Du B, Sun HD. 12-Hydrothermal Synthesis and Characterization of Fe3O4 Nanorods. J Inorg Organ P (2013) 23(2):379–84. doi: 10.1007/s10904-012-9789-2
195. Lacroix LM, Huls NF, Ho D, Sun X, Cheng K, Sun S. Stable Single-Crystalline Body Centered Cubic Fe Nanoparticles. Nano Lett (2011) 11(4):1641–5. doi: 10.1021/nl200110t
196. Wang S, Yu G, Wang Z, Jacobson O, Lin LS, Yang W, et al. Enhanced Antitumor Efficacy by a Cascade of Reactive Oxygen Species Generation and Drug Release. Angew Chem Int Ed Engl (2019) 58(41):14758–63. doi: 10.1002/anie.201908997
197. Li F, Liang Y, Wang M, Xu X, Zhao F, Wang X, et al. Multifunctional Nanoplatforms as Cascade-Responsive Drug-Delivery Carriers for Effective Synergistic Chemo-Photodynamic Cancer Treatment. J Nanobiotech (2021) 19(1):140. doi: 10.1186/s12951-021-00876-7
198. Tang K, Wang W, Song Z, Luo X. Multifunctional Nano-Biosensor Based on Metal-Organic Framework for Enhanced Fluorescence Imaging of Intracellular miRNA-122 and Synergistic Chemo-Photothermal Therapy of Tumor Cells. Anal Chim Acta (2021) 1176:338779. doi: 10.1016/j.aca.2021.338779
199. Wang Z, Zhao K, Zhang Y, Duan X, Zhao Y. Anti-GPC3 Antibody Tagged Cationic Switchable Lipid-Based Nanoparticles for the Co-Delivery of Anti-Mirna27a And Sorafenib in Liver Cancers. Pharm Res (2019) 36(10):145. doi: 10.1007/s11095-019-2669-5
200. Wang S, Liu Q, Li L, Urban MW. Recent Advances in Stimuli-Responsive Commodity Polymers. Macromol Rapid Commun (2021) 42(18):e2100054. doi: 10.1002/marc.202100054
201. Guan GJ, Wu MD, Han MY. Stimuli-Responsive Hybridized Nanostructures. Adv Funct (2020) 30:1903439–1903465. doi: 10.1002/adfm.201903439
202. Liao J, Jia Y, Wu Y, Shi K, Yang D, Li P, et al. Physical-, Chemical-, and Biological-Responsive Nanomedicine for Cancer Therapy. Wiley Interdiscip Rev Nanomed Nanobiotechnol (2020) 12(1):e1581. doi: 10.1002/wnan.1581
203. Ji T, Zhao Y, Wang J, Zheng X, Tian Y, Zhao Y, et al. Tumor Fibroblast Specific Activation of a Hybrid Ferritin Nanocage-Based Optical Probe for Tumor Microenvironment Imaging. Small (2013) 9(14):2427–31. doi: 10.1002/smll.201300600
204. Qin X, Li Y. Strategies To Design and Synthesize Polymer-Based Stimuli-Responsive Drug-Delivery Nanosystems. Chembiochem (2020) 21(9):1236–53. doi: 10.1002/cbic.201900550
205. Li HJ, Du JZ, Du XJ, Xu CF, Sun CY, Wang HX, et al. Stimuli-Responsive Clustered Nanoparticles for Improved Tumor Penetration and Therapeutic Efficacy. Proc Natl Acad Sci USA (2016) 113(15):4164–9. doi: 10.1073/pnas.1522080113
206. Li HJ, Du JZ, Liu J, Du XJ, Shen S, Zhu YH, et al. Smart Superstructures With Ultrahigh pH-Sensitivity for Targeting Acidic Tumor Microenvironment: Instantaneous Size Switching and Improved Tumor Penetration. ACS Nano (2016) 10(7):6753–61. doi: 10.1021/acsnano.6b02326
Keywords: tumor, pH-responsive polymer, nanomaterials, tumor microenvironment, drug delivery
Citation: Chu S, Shi X, Tian Y and Gao F (2022) pH-Responsive Polymer Nanomaterials for Tumor Therapy. Front. Oncol. 12:855019. doi: 10.3389/fonc.2022.855019
Received: 14 January 2022; Accepted: 24 February 2022;
Published: 22 March 2022.
Edited by:
Kelong Ai, Central South University, ChinaReviewed by:
Jia Huang, Central South University, ChinaCopyright © 2022 Chu, Shi, Tian and Gao. This is an open-access article distributed under the terms of the Creative Commons Attribution License (CC BY). The use, distribution or reproduction in other forums is permitted, provided the original author(s) and the copyright owner(s) are credited and that the original publication in this journal is cited, in accordance with accepted academic practice. No use, distribution or reproduction is permitted which does not comply with these terms.
*Correspondence: Fengxiang Gao, Z2Z4MjZAY2lhYy5hYy5jbg==
†These authors contributed to the work equally and should be regarded as co-second authors
Disclaimer: All claims expressed in this article are solely those of the authors and do not necessarily represent those of their affiliated organizations, or those of the publisher, the editors and the reviewers. Any product that may be evaluated in this article or claim that may be made by its manufacturer is not guaranteed or endorsed by the publisher.
Research integrity at Frontiers
Learn more about the work of our research integrity team to safeguard the quality of each article we publish.