- 1The Second Clinical Medical College of Lanzhou University, Lanzhou University, Lanzhou, China
- 2Key Laboratory of Digestive System Tumors of Gansu Province, Second Hospital of Lanzhou University, Lanzhou, China
The tumor microenvironment restricts the function and survival of various immune cells by up-regulating inhibitory immune checkpoints, and participates in the immune escape of tumors. The development of immunotherapies targeting immune checkpoints, such as programmed cell death receptor 1 antibody and anti-cytotoxic T lymphocyte-associated antigen 4 antibody, has provided many options for cancer treatment. The efficacy of other immune checkpoint inhibitors is also under development and research. Among them, T cell immunoreceptor with Ig and ITIM domains (TIGIT) has shown excellent clinical application prospects. Correspondingly, poliovirus receptor (PVR, CD155), one of the main ligands of TIGIT, is mainly expressed in various human malignant tumors and myeloid cells. CD155 interacts with TIGIT on natural killer cells and T cells, mediating inhibitory immunomodulatory regulation. This study summarized the mechanism of CD155/TIGIT in regulating immune cells and its role in the occurrence and development of digestive system tumors, aiming to provide a new perspective for immunotherapy of digestive cancers.
Introduction
Immune checkpoints are stimulatory and inhibitory receptors of the immune system. The inhibitory immune checkpoints are involved in regulating immune activation, preventing excessive activation of immune responses, and maintaining immune tolerance. Tumor cells can achieve immune escape by hijacking inhibitory immune checkpoints, such as programmed cell death-1 protein/ligand (PD-1/PD-L1), cytotoxic T lymphocyte antigen 4 (CTLA4), T cell immunoglobulin and mucin domain-containing protein 3 (TIM-3), which regulates the function and survival of immune cells and facilitates immune suppressive microenvironment (1, 2). At present, immune checkpoint inhibitors (ICIs) have been developed for clinical application, which block the interaction between tumor cells expressing inhibitory immune checkpoints and immune cells and reinvigorate anti-tumor immune responses. Nivolumab and pembrolizumab, ICIs that target PD-1, showed promising anti-tumor activity in patients with non-small cell lung carcinoma, with an objective response rate of 56% and 45.2%, respectively (3, 4). In patients with advanced cutaneous squamous-cell carcinoma, the response to PD-1 inhibitors was 47% (5). Melanoma patients treated with CTLA-4 inhibitors exhibited improved survival (6). However, multiple clinical trials revealed that various types of tumors, such as pancreatic ductal adenocarcinoma (7), esophageal adenocarcinoma (8) and prostate cancer (9), exhibited poor responses to ICIs. Furthermore, the clinical benefits of ICIs are also accompanied by severe immune toxicity, including cardiotoxicity (10), pneumonia (11), hepatitis,colitis (12), pancreatitis (13) and endocrine dysfunction (14). The excessive immune cell responses caused by ICIs, including the activation of T cells and macrophages, produce many proinflammatory cytokines and induce a life-threatening inflammatory response (15, 16). Therefore, it might be promising to develop new immune checkpoints targets to optimize treatment regimens and reduce cytotoxicity.
Natural killer (NK) cells and T cells represent the central part of the immune system (17, 18), and inhibitory immune checkpoints expressed on their surfaces are gradually being explored. T cell immunoreceptor with Ig and ITIM domains (TIGIT) is considered to be an inhibitory receptor in activated T cells, NK cells, and Treg cells (18–20), and has become a promising target for immunotherapy. Poliovirus receptor (PVR, CD155) is the ligand with the highest binding affinity to TIGIT, which plays a critical role in cell adhesion, migration, proliferation and regulation of immune responses (21). The interaction between TIGIT and CD155 can mediate the functional exhaustion and hyporesponsiveness of lymphocytes, and induce the immune escape of tumor cells. This emerging immune checkpoint is expected to become a novel target for tumor immunotherapy (22–24). This review summarizes the biological function and mechanism of TIGIT/CD155 in immune cells and the latest research progress in digestive system tumors.
Expression and Function of CD155/TIGIT
TIGIT is a member of the immunoglobulin superfamily with a molecular structure consisting of extracellular Ig variable region, type I transmembrane domain, intracellular immunoreceptor tyrosine inhibitory motif (ITIM) and immunoglobulin tail tyrosine (ITT)-like motif (25) (Figure 1). Current studies reveal that TIGIT is expressed on activated CD8+ T cells, CD4+T cells, regulatory T (Treg) cells and NK cells, and can inhibit the anti-tumor responses mediated by immune cells (26). The latest research has shown that TIGIT is also expressed on B cells, which binds to CD155 expressed on dendritic cells (DC), leading to decreased expression of IL-12, IL-16 and CCR7, thereby inhibiting the maturation and proinflammatory response of DC (27).
CD155, one of the main ligands of TIGIT, exerts functions via different mechanisms in tumor and immunology. CD155 is found to be up-regulated in a variety of human malignancies and plays a carcinogenic role by affecting biological functions such as cell adhesion, migration, invasion and proliferation (28). The elevated level of CD155 in human glioma cells modulates the Src/FAK/Paxillin/p130Cas signaling pathway induced by adhesion, promoting cancer cell migration, which is one of the ways for tumor cells to acquire aggressiveness (29). Besides, CD155 deletion can synergize with doxorubicin to induce apoptosis of breast cancer cells and suppress cell growth (30). In immunology, CD155 regulates the functions of immune cells by binding to costimulatory immune receptor CD226 (also named DNAM-1) and inhibitory checkpoint receptors TIGIT and CD96 (28, 31). TIGIT competes with CD226 to bind CD155 and inhibits CD226 signal by disrupting CD226 homodimerization (32). It has been reported that CD155, widely expressed in human and mouse tumor-infiltrating myeloid cells, promotes tumor cell growth and metastasis by down-regulating the expression of CD226 and inhibiting the effects of CD8+T and NK cells (33). Like TIGIT, CD96 is an inhibitory receptor binding to CD155, which can induce NK cell exhaustion and mediate the adhesion function of immune cells (34). Additionally, CD96 and CD226 competitively bind to CD155, impairing the function of NK cells (31).
The immune inhibitory receptors share a common ITIM in their cytoplasmic regions, responsible for transmitting inhibitory signals. TIGIT binds to CD155 with a higher affinity than CD226 and CD96 (20, 28, 35). TIGIT binding to CD155 could suppress the activity and function of lymphocytes. The mechanisms mediated by TIGIT/CD155 signaling axis mainly include the following four aspects: 1) TIGIT interacts with CD155 expressed on DC, inducing IL-10 and suppressing IL-12 production, which directly inhibits DC maturation and indirectly reduces the proliferation and function of T cells (20). 2) They directly exert the inherent inhibitory effect of T cells through the recruitment of SHIP1 and SHP2 phosphatases (36). 3) Direct inhibition of NK cell cytotoxicity and cytokine release (37). 4) The lack of CD226 can enhance the TIGIT/CD155 signal in the inflammatory environment, thereby inhibiting the AKT-mTORC1 pathway, stabilizing Foxp3 and maintaining the immunosuppressive function of Treg cells (38). Currently, CD155/TIGIT has shown potential targeted therapeutic effects in a variety of malignancies, such as high-grade serous ovarian cancer (39) and head and neck squamous cell carcinoma (40). In the sepsis model of tumor-bearing mice, TIGIT was overexpressed on Treg and NK cells, binding to CD155 and mediating immunosuppression. Targeting TIGIT significantly improved the survival of mice and the reduction of T cell apoptosis (41). Based on the studies of CD155/TIGIT in tumor and immunology, it is suggested that TIGIT/CD155 is a promising target.
Regulation of CD155/TIGIT in Immune Cells
NK Cells
NK cells are innate immune cells, which mainly play a role in early infections and inhibiting tumor growth and metastasis. TIGIT, as an inhibitory receptor expressed by all NK cells, recognizes CD155 expressed on cells derived from normal epithelium to protect normal cells from NK cell destruction (37). CD155/TIGIT has been proven to limit the toxic effect and cytokine release function of NK cells in vitro. When CD155/TIGIT signal is initiated, the ITT-like motif in the cytoplasmic tail of TIGIT is phosphorylated at Tyr225 and combined with Grb2. Then SHIP1 is recruited to terminate PI3K and MAPK signals prematurely, resulting in the inhibition of NK cell function (Figure 2). SHIP1 deletion can significantly reverse the immunosuppression mediated by TIGIT and CD155 (42).
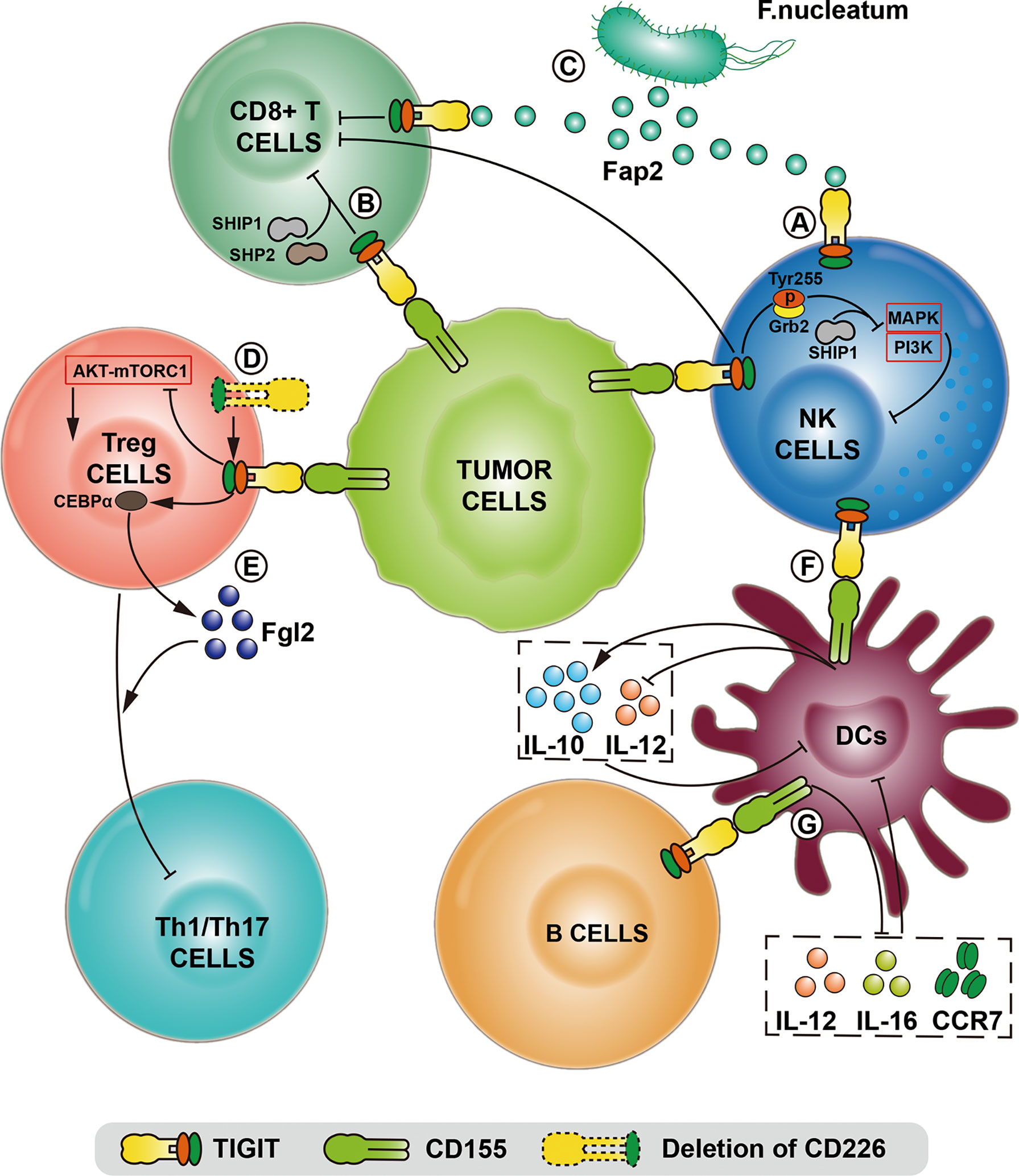
Figure 2 The comprehensive mechanisms of TIGIT/CD155 axis mediated in the immune system in various models and biological contexts. (A) After TIGIT expressed on NK cells binds to CD155, the intracellular immunoreceptor (ITT)-like motif of TIGIT is phosphorylated at Tyr255 and interacts with Grb2, which terminates MAPK and PI3K signaling via recruiting SHIIP1, leading to the inhibition of NK cell function. (B) TIGIT/CD155 axis directly induces CD8+T cell exhaustion by recruiting SHIP1 and SHP2 phosphatase. (C) Tumor cells restrict NK and CD8+T cell activity using the Fap2 protein of F.nucleatum bacteria to interact with TIGIT. (D) The deletion of CD226 enhances TIGIT/CD155 signaling, thereby maintaining the immunosuppressive function of Tregs with the inhibition of the AKT-mTORC1 pathway. (E) After TIGIT/CD155 ligation, the transcription factor CEBPα promotes the transcription of the soluble effector molecule fibrinogen-like protein 2 (Fgl2), thereby the hypersecretion of Fgl2 in Tregs facilitates the suppression of pro-inflammatory Th1 and Th17 cells. (F) The binding of CD155 to TIGIT on dendritic cells (DC) induces the production of IL-10 and inhibits the production of IL-12, which directly leads to the DC maturation and indirectly reduces the proliferation and function of T cells. (G) TIGIT expressed on specific B cell subsets binds to CD155 expressed on DC, leading to the decreased expression of IL-12, IL-16 and CCR7, thereby inhibiting the maturation and pro-inflammatory response of DC.
IL-15 signaling plays a critical role in NK survival and differential and can enhance the cytotoxicity mediated by NK cells. One study showed that the combination of IL-15 and anti-TIGIT antibodies improved the immune function of NK cells and T cells (43). Another study confirmed that the combination of IL-15 and TIGIT blockade activated the killing effect of tumor-infiltrating NK cells (44). The blockade of TIGIT in NK cells requires consideration of multiple factors. Previous studies have supported that dual the blockade of PD-1 and TIGIT mainly exerted effects on NK cells, thereby enhancing the anti-tumor activity of CD8+T cells. However, blocking TIGIT alone cannot reverse the cytotoxic effect of NK cells infiltrated in melanoma, while activated NK cells can induce TIGIT expression (31). The anti-tumor effect of blocking TIGIT may also need to consider the activation status of the CD226. CD155 expressed on membrane leads to increased internalization and degradation CD226 on NK cells and promotes NK cell dysfunction. It has been reported that NK cells from malignant ovarian cancer up-regulate TIGIT and down-regulate CD226 expression. Subsequent in vitro and in vivo experiments have shown that targeting TIGIT can achieve superior efficacy based on up-regulation of CD226 expression (45).
NK cells rely on many receptor combinations to initiate effector function, among which inhibitory receptors recognize MHC-I proteins. Thus, cells expressing MHC-I proteins could be protected from NK cell-mediated lysis. Since CD8+T cells mediate immune destruction of MHC-I presenting tumor cells, NK cells are effective against MHC-I deficient tumors. Simultaneous activation of NK cells and CD8+T cells can be considered an anti-tumor therapy strategy. Therefore, targeting TIGIT/CD155 signaling axis may simultaneously enhance the effector function of CD8+T cells and NK cells, which could be a potential treatment option.
CD8+T Cells
As the primary anti-tumor effector cells, CD8+ T cells have become a hot spot for immune checkpoint blocking therapy in recent years. In the malignant tumor microenvironment, the infiltrating proportion of CD8+ T cells and the expression pattern of related immune checkpoints affect the prognosis of patients and the response to immunotherapy (46, 47). In mice and humans, including bladder cancer (48), melanoma (49), gastric cancer (50), colorectal cancer (51) and other tumors (52), the expression of TIGIT on infiltrating CD8+ T cells is related to patient survival.
CD155 and TIGIT affect function and survival of CD8+T cells via multiple mechanisms. TIGIT can inhibit the NK cell-mediated immune response by binding to CD155, thereby suppressing the anti-tumor effect of CD8+T cells (53). The infiltrating CD8+ T cells in metastatic melanoma have increased expression of TIGIT, which induced the downregulation of costimulatory factor CD226. The corresponding ligand CD155 was elevated in melanoma cells and involved in the malignant progression of the tumor (49).
TIGIT can also mediate tumor immune escape in a bacteria-dependent manner, in which tumor cells directly interact with TIGIT to restrict NK and T cell activity using the Fap2 protein of Fusobacterium nucleatum (54). In a combined analysis of 155 patients with metastatic melanoma treated with ICIs, it was found that poor response to immunotherapy was associated with high expression of CD155 (55). CD155 deletion enhanced tumor sensitivity to PD-1 blockade, which was observed in a vivo non-small cell lung cancer study. It may be attributed to the tumor-infiltrating immune cells, especially the more frequent expression of PD-1 in Tregs. Secondly, CD155 deletion increases the anti-tumor activity of CD8+T cells and NK cells in tumors (56). This feature targets CD155/TIGIT combined with PD-1 blocking therapy to improve the immune response. A chimeric antigen receptor (CAR) T cell targeting CD19 that integrates PD-1 and TIGIT has shown effective anti-tumor activity in relapsed or refractory large B-cell lymphoma, and significantly enhanced CAR-T persistence cells in vivo (57).
Treg Cells
Treg cells are a subset of CD4+T cells characterized by the expression of Foxp3, CD25 and CD4, which play an essential role in regulating immune response and maintaining immune tolerance. Treg cells expressing TIGIT can serve as a subset of cells with activating phenotypic function. TIGIT signal in this cell subset promotes the transcription of the soluble effector molecule fibrinogen-like protein 2 (Fgl2) by inducing the transcription factor CEBPα, thereby the hypersecretion of Fgl2 facilitates the suppression of pro-inflammatory Th1 and Th17 cells mediated by Treg cells (58). Activated Treg cells express TIGIT infiltrating in the tumor microenvironment, which is related to poor survival (59). γδTregs, a new subset of Tregs, has been reported that higher TIGIT+ γδTregs contributed to the poorer overall survival in patients with acute myeloid leukemia, suggesting that TIGIT may be involved in regulating Treg cells in malignancies (60). Besides, highly infiltrated TIGIT+ Treg cells and high levels of CD155 jointly mediate the enhanced inhibitory function of Treg cells in the tumor microenvironment of melanoma (61). The co-stimulatory receptor CD226 competes with TIGIT, and the deletion of CD226 can also enhance Treg cell function mediated by TIGIT (38).
Role of CD155/TIGIT in Digestive System Cancers
Gastric Cancer
Gastric cancer is one of the most malignant tumors of the digestive system, and the five-year survival rate remains low (62). The immune microenvironment of gastric cancer is characterized by high heterogeneity and complexity (63), and there is also a high incidence of somatic mutations (64), suggesting that gastric cancer may benefit from immunotherapy. Therefore, identifying novel immune-related therapeutic targets may improve the immune microenvironment and the survival of gastric cancer patients. A study based on clinical samples showed that the elevated expression of TIGIT and CD155 in gastric cancer tissues was closely associated with poor prognosis of patients and functional exhaustion of highly infiltrated CD8+T cells (65). Thus TIGIT/CD155 may be a potential therapeutic target and prognostic marker for gastric cancer.
In human tissues, there is a soluble form of CD155 (sCD155) (66). Membrane binding protein is encoded by CD155α and CD155δ, while sCD155 is encoded by CD155γ and CD155β, and lacks a transmembrane region. However, their significance varies in gastric cancer. The increased expression of sCD155 in the serum of patients with gastric cancer was related to the degree of tumor differentiation (67). sCD155 may serve as a serum marker for the early diagnosis of gastric cancer. It has been reported that sCD155 preferentially binds to CD226 and suppresses CD226-mediated NK cell cytotoxic activity, promoting melanoma metastasis (68). However, it is still unclear whether sCD155 has a similar effect to membrane-expressed CD155 and can combine with TIGIT to generate the immunosuppressive signal.
Chemotherapy is one of the indispensable treatment options for patients with gastric cancer, but it can lead to immunosuppression (69). The high expression of TIGIT on CD8+T cells in patients undergoing chemotherapy is closely associated with the recurrence of gastric cancer, and blocking TIGIT could enhance the proliferation capacity of CD8+T cells and induce the secretion of IFN-γ (70). A recent study demonstrated that the infiltrated CD8+ T cells up-regulated the level of TIGIT in gastric cancer, exhibiting exhausted and decreased metabolic activity. Additionally, these cancer cells suppressed CD8+T cell metabolism via CD155/TIGIT signaling, mediated by down-regulation of the AKT/mTOR signaling pathway (50). What needs to be considered is that CD155/TIGIT may mediate cellular immune function in different cell populations including T cells, NK cells and DC cells. TIGIT and PD-1 exerted inhabitation function on CD8+T cell growth, proliferation, and cytokine secretion in gastric cancer patients receiving WT1-targeted DC vaccine. The combination of targeting TIGIT and PD-1 restored DC vaccine-induced activity of T cells (71).
Liver Cancer
Currently, the FDA approved ICIs for advanced liver cancer include Nivolumab, Durvalumab, and ipilimumab, but only a minority of patients showed a favorable response. Therefore, there is an urgent need to develop new immunotherapies. TIGIT is elevated on lymphocytes infiltrating liver cancer tissue and may participate in the tumor progression (72, 73). Transcriptome analysis of the mouse liver cancer model demonstrated that TIGIT served as a marker of T cell depletion compared with PD-1 (74). Correspondingly, CD155 is up-regulated in liver cancer cells and contributes to the poor prognosis of patients with liver cancer. Besides, high expression of CD155 can induce the expression of TIGIT in CD8+T cells, further suppressing the activation of PI3K, MAPK and NF-κB signaling pathways, thereby reducing the secretion of IFN-γ and TNF-α by CD8+ T cells. Blocking TIGIT can reverse the effector function of CD8+T cells (75). In addition, bacteria are also involved in the interaction of the immune microenvironment of liver cancer. It has been reported that TIGIT and E. multilocularis infection in the liver can induce NK cell depletion and immune escape in tumor microenvironment based on clinical samples and mouse models (76).
Another study indicated that the high expression of CD155 in liver cancer could induce NK cells to up-regulate CD96, leading to impaired NK cell function and inhibition of survival, which is associated with poor clinical prognosis of patients with liver cancer. Blocking the interaction between CD96 and CD155 restored the anti-tumor activities of NK cells (34). Given the recent findings that TIGIT is a significantly expressed immune checkpoint in patients receiving anti-PD-1 treatment, the role of CD155/TIGIT should be noted in the regulation of immune responses. It has been reported that PVRL1 stabilized CD155 expression on the surface of liver cancer cells by reducing the endocytosis of CD155, and then interacted with TIGIT on the surface of CD8+ T cells (77). The anti-PD-1 and TIGIT therapy combination also reversed the proliferation and killing capacities of CD8+ T cells infiltrated by liver cancer, which was observed in another study (73). Based on the treatment of oncolytic viruses targeting PD-1, TIGIT blockade can increase the anti-tumor efficacy of oncolytic viruses (78). The combination therapy may improve the outcome of patients with liver cancer in the future. Therefore, CD155/TIGIT is expected to become a novel target for liver cancer immunotherapy.
At present, therapies targeting CD155/TIGIT are gradually being developed. In a liver cancer ascites model, the construction of the oncolytic virus expressing the soluble extracellular domain of CD155 increased the infiltration of CD8+ T cells and the secretion of IFN-γ, which exhibited the durable tumor-specific immune surveillance. The underlying mechanism was that oncolytic viruses blocked the CD155/TIGIT signal axis and activated CD226 (79). Wang. et al. designed a nanoparticle that blocked the binding of TIGIT and CD155 and targeted LncRNA ANRIL, which exhibited a significant anti-tumor effect and increased the proportion of NK cells and T cells in an in vivo model of liver cancer (80). The whole-cell vaccine for liver cancer targeting STAT3 reversed the depletion of T cells and NK cells in the liver cancer microenvironment, which may be attributed to the low expression of PD-1 and TIGIT (81).
Hepatitis B virus (HBV), a risk factors for liver cancer, could induce chronic inflammation, cirrhosis, and ultimately process cancer. The proportion of infiltrated PD-1+ TIGIT+ CD8+ T cells was increased and in a hypo-responsive state in liver cancer patients infected with HBV, especially at advanced stages, which was significantly associated with the poor clinical prognosis (82). This finding indicated that TIGIT might play an essential role in the progression of HBV-related liver cancer. Single-cell sequencing analysis of HBV-related liver cancer showed that tumor-associated macrophages facilitated the formation of an inhibitory immune microenvironment in cancer through the interaction between TIGIT and CD112 (83). Another study confirmed that TIGIT was highly expressed on CD8+ T cells in the HBV immune tolerance mouse model analysis. However, TIGIT blockade or deletion reversed the tolerance of CD8+T cells to viral antigen, which caused chronic hepatitis in mice and eventually led to the development of liver cancer (84). The treatment targeting TIGIT may increase the risk of chronic hepatitis and liver cancer progression. Current studies have shown that antiviral therapy can eliminate the activity of histological necrotic inflammation and improve the prognosis of HBV-associated liver cancer patients (85, 86). We infer that the combination of drugs that improve liver function and antiviral drugs may improve the excessive inflammation caused by the promotion of immunotherapy when immunotherapy targeting CD155/TIGIT is applied to HBV-related liver cancer. Therefore, the protection of liver function and the management of chronic hepatitis should be taken into account in the immunotherapy for liver cancer.
Pancreatic Cancer
Pancreatic cancer is a highly invasive and malignant tumor. Most patients were non-responders after receiving immune-based treatment strategies, which was attributed to the pathological types of pancreatic cancer were microsatellite stable (87, 88). In recent years, neoantigens have been discovered to be tumor specificity and recognized by the immune system. Previous studies have shown that pancreatic cancer has high-affinity MHC class I-restricted neo-epitopes, and the CD155/TIGIT signal axis is sufficient to facilitate immune evasion mediated by pancreatic cancer expressing neo-epitopes (24). Besides, the infiltrating proportion of T cells is related to the prognosis of pancreatic cancer. Based on single-cell sequencing analysis, the immune microenvironment of pancreatic cancer is characterized by the presence of a large number of immunosuppressive cell populations and functionally exhausted CD8+ T cells that express TIGIT (89). The above findings suggested that the CD155/TIGIT signal axis could serve as the target for pancreatic cancer immunotherapy, especially in patients who do not respond to anti-PD-1 therapy, which is expected to improve the suppressive immune system microenvironment.
A recent study demonstrated that the abundance of CD155 expression in pancreatic cancer tissues was an independent prognostic factor for patients and negatively correlated with the proportion of tumor infiltrating immune cells (90). In the pancreatic cancer microenvironment, NK cells down-regulate the activating receptor molecule CD226 and induce the increased expression of TIGIT (91). Correspondingly, CD155 presents high expression in tumor cells (92). Consistent with this finding, CD8+T cells with elevated expression of CD226 tend to have a more significant response to TIGIT blockade, which indicated that CD226 could be used as a marker for anti-TIGIT therapy (93). Therefore, CD155 may be involved in the progression through immune or non-immune mechanisms, and is expected to become a therapeutic target for pancreatic cancer.
A previous study revealed that the number of NK cells was positively correlated with overall survival of pancreatic cancer patients (94). An in-vivo study demonstrated that the increasing infiltration of NK cells in pancreatic cancer could improve prognosis (95). Recently, NK cells and T cells have gained much attention for immunotherapy in pancreatic cancer (96, 97). Current treatment strategies are focused on activating T cells and NK cells after gemcitabine treatment to prevent a recurrence. It has been reported that the application of neoadjuvant administration of PD-1 antagonist combined with gemcitabine improved the viability of NK cells and T cells and inhibited the local recurrence of pancreatic cancer. On this basis, further blocking the immune checkpoint CD96 expressed on NK cells, which bound to CD155, could significantly prevent distant metastasis of pancreatic cancer (98). Based on the above researches, CD155/TIGIT plays an essential role in the unique microenvironment of pancreatic cancer, and targeting this signal axis may become a treatment strategy for pancreatic cancer.
Colorectal Cancer
Previous studies have demonstrated that TIGIT and CD155 were elevated in colorectal cancer compared with normal tissue (99). The density of CD8+T cells in the tumor margin increased, and expression of PD-1 and TIGIT was up-regulated, compared with that in the tumor center of colorectal cancer (100). Besides, the overexpression of TIGIT in infiltrating lymphocytes exhibited functional failure and hyporesponsive state, which was associated with the advanced stage of tumor (51). High expression of TIGT could lead to impaired glucose metabolism of T cells, and blocking TIGIT restored T cell activity and function (101). Furthermore, the interaction between TIGIT and CD155 participated in regulating CD8+ T cell effector via the activation of the NF-κB signaling pathway (102). Thus, TIGIT may serve as an immunotherapy target for colorectal cancer. Interestingly, TIGIT was also expressed on cancer cells of colorectal cancer, but this expression pattern did not affect cell proliferation in vitro (103). Given that CD155 is expressed on NK cells and T cells, TIGIT on tumor cells may transmit inhibitory signals to immune cells via the interaction with CD155.
Intestinal microorganisms are involved in the symbiotic interaction of the human mucosal immune system. There is a potential connection with the initiation and development of colorectal cancer. Accumulating evidence demonstrated that Fusobacterium nucleatum is enriched in colon cancer and identified as the pro-inflammatory bacteria, contributing to the poor prognosis and low infiltrating T cells in colon cancer (104, 105). A bacteria-dependent immune escape is mediated by cancer cells in the tumor microenvironment. Fusobacterium nucleatum suppressed immune cells cytotoxicity and viability to protect tumor cells from being killed by immune cells through the interaction between Fap2 and TIGIT on NK cells and T cells. The binding site was different from TIGIT and CD155 (54). Fusobacterium nucleatum can also inhibit the activity of T cells and NK cells by binding to the inhibitory receptor CEACAM1 (106). In the future, combinatorial therapy can be considered to treat tumors colonized by Fusobacterium nucleatum.
Furthermore, chronic inflammation mediated by intestinal flora is also a risk factor for colorectal cancer. As a classic non-steroidal anti-inflammatory drug, Aspirin inhibits colorectal cancer cell proliferation and induces tumor cell apoptosis through TIGIT/CD155 signaling axis (107). Previous studies have shown that specific intestinal flora can affect patients’ response to ICIs and contribute to the occurrence of colitis after receiving immune checkpoint treatment (108, 109). In addition, targeting CD155/TIGIT may induce excessive activation of infiltrating CD8+T cells in tumors, leading to excessive inflammation. Therefore, targeted therapies need to consider the effect of intestinal flora or enhance the anti-tumor efficacy by regulating intestinal flora or combining anti-inflammatory drugs.
Colorectal cancer often displays DNA mismatch repair deficiency or microsatellite instability-high, so that tumor cells often express many neoantigens, which are easily recognized by the immune system. Previous studies indicated that immune checkpoint blocking therapy had shown significant efficacy in colorectal cancer patients with DNA mismatch repair deficiency or microsatellite instability-high (110). Immunotherapy shows excellent promise in specific colon cancer genotypes. It has shown that the expression of PD-1 and TIGIT is elevated in colorectal cancers that are defective in mismatch repair (111). When used in combination with PD-1 blockade, blocking TIGT enhances the efficacy of radiotherapy for colorectal cancer (112). CD155 was significantly enriched in colorectal cancer with KRAS mutations, and down-regulated CD155 inhibited the growth of tumor cells (113). However, it is worth noting that 85% of patients with colorectal cancers are mismatch-repair proficient or microsatellite instability-low, displaying no response to immune checkpoint inhibitor therapy (114). Given these, it is necessary to explore new immune checkpoints and deeply investigate the resistance mechanism of immunotherapy in specific genotypes of colon cancer. The efficacy of targeting the TIGIT/CD155 signal axis may depend on further genotyping in colorectal cancer.
Other Digestive Cancers
Consistent with that in gastric cancer, sCD155 in serum can be used as a biomarker for esophageal cancer. The expression level of sCD155 is closely correlated with the response and prognosis of patients with esophageal cancer after receiving chemotherapy (115). The regulatory mechanism of CD155/TIGIT in esophageal cancer still needs further study. As a malignant tumor with a poor prognosis, the expression of CD155 is elevated in gallbladder cancer, which contributes to the unfavorable prognosis of patients. TIGIT has been observed increased expression in immune cells infiltrated in cancer (116). Thus, CD155/TIGT might become a novel target for immunotherapy of gallbladder cancer. Cholangiocarcinoma and head and neck squamous cell carcinoma, including oral cancer, pharyngeal cancer and laryngeal cancer, exhibit an inhibitory immune microenvironment, in which TIGIT is positively expressed (117, 118), suggesting that targeting TIGIT may be with a promising application prospect.
Conclusions and Perspectives
The current evidence shows that CD155 and TIGIT are highly expressed in various malignant tumors and can be used as potential biomarkers and therapeutic targets. This signal axis mainly suppresses the survival activity and anti-tumor responses of NK and CD8+ T cells through multiple mechanisms and mediates the immune escape of tumor cells. Recent studies indicate that TIGIT is also expressed on tumor cells and involved in the regulation of B cells, and the role of TIGIT and the underlying mechanism remains to be further investigated. CD155/TIGIT is expected to become a new target for immunotherapy of digestive cancers. The treatment strategy for targeting CD155/TIGIT is still at the preclinical research stage, mainly presented as monoclonal antibody therapy. Considering that emerging immunotherapies include ICIs, DC vaccines, adoptive cell therapy, they can be applied to target CD155/TIGIT in the future to achieve better clinical efficacy. Previous studies have supported the combination of blocking TIGIT and PD-1 can further enhance the response of immunotherapy. Therefore, combining ICIs may be one of the potential strategies for treating malignant digestive cancers. Given the differences between individuals of digestive system tumors, it is necessary to comprehensively consider the effect of tumor genotyping, intestinal flora and inflammation on treatment efficacy in future clinical practice.
Author Contributions
All authors conceptualized and wrote the manuscript. YL conceived and modified the structure of this review. DW and YG additionally performed literature analysis and figure preparation. All authors contributed to the article and approved the submitted version.
Funding
This work was supported by the National Natural Science Foundation of China under Grant NO.31770537, the Natural Science Foundation of Gansu Province under Grant NO.20JR10RA740, and the Natural Science Foundation of Gansu Province under Grant NO.20JR5RA345.
Conflict of Interest
The authors declare that the research was conducted in the absence of any commercial or financial relationships that could be construed as a potential conflict of interest.
Publisher’s Note
All claims expressed in this article are solely those of the authors and do not necessarily represent those of their affiliated organizations, or those of the publisher, the editors and the reviewers. Any product that may be evaluated in this article, or claim that may be made by its manufacturer, is not guaranteed or endorsed by the publisher.
References
1. Pardoll DM. The Blockade of Immune Checkpoints in Cancer Immunotherapy. Nat Rev Cancer (2012) 12(4):252–64. doi: 10.1038/nrc3239
2. Qin S, Xu L, Yi M, Yu S, Wu K, Luo S. Novel Immune Checkpoint Targets: Moving Beyond PD-1 and CTLA-4. Mol Cancer (2019) 18(1):155. doi: 10.1186/s12943-019-1091-2
3. Borghaei H, Paz-Ares L, Horn L, Spigel DR, Steins M, Ready NE, et al. Nivolumab Versus Docetaxel in Advanced Nonsquamous Non-Small-Cell Lung Cancer. N Engl J Med (2015) 373(17):1627–39. doi: 10.1056/NEJMoa1507643
4. Garon EB, Rizvi NA, Hui R, Leighl N, Balmanoukian AS, Eder JP, et al. Pembrolizumab for the Treatment of non-Small-Cell Lung Cancer. N Engl J Med (2015) 372(21):2018–28. doi: 10.1056/NEJMoa1501824
5. Migden MR, Rischin D, Schmults CD, Guminski A, Hauschild A, Lewis KD, et al. PD-1 Blockade With Cemiplimab in Advanced Cutaneous Squamous-Cell Carcinoma. N Engl J Med (2018) 379(4):341–51. doi: 10.1056/NEJMoa1805131
6. Hodi FS, O'Day SJ, McDermott DF, Weber RW, Sosman JA, Haanen JB, et al. Improved Survival With Ipilimumab in Patients With Metastatic Melanoma. N Engl J Med (2010) 363(8):711–23. doi: 10.1056/NEJMoa1003466
7. Royal RE, Levy C, Turner K, Mathur A, Hughes M, Kammula US, et al. Phase 2 Trial of Single Agent Ipilimumab (Anti-CTLA-4) for Locally Advanced or Metastatic Pancreatic Adenocarcinoma. J Immunother (Hagerstown Md 1997) (2010) 33(8):828–33. doi: 10.1097/CJI.0b013e3181eec14c
8. Ralph C, Elkord E, Burt DJ, O'Dwyer JF, Austin EB, Stern PL, et al. Modulation of Lymphocyte Regulation for Cancer Therapy: A Phase II Trial of Tremelimumab in Advanced Gastric and Esophageal Adenocarcinoma. Clin Cancer Res (2010) 16(5):1662–72. doi: 10.1158/1078-0432.CCR-09-2870
9. Beer TM, Kwon ED, Drake CG, Fizazi K, Logothetis C, Gravis G, et al. Randomized, Double-Blind, Phase III Trial of Ipilimumab Versus Placebo in Asymptomatic or Minimally Symptomatic Patients With Metastatic Chemotherapy-Naive Castration-Resistant Prostate Cancer. J Clin Oncol (2017) 35(1):40–7. doi: 10.1200/JCO.2016.69.1584
10. Patel RP, Parikh R, Gunturu KS, Tariq RZ, Dani SS, Ganatra S, et al. Cardiotoxicity of Immune Checkpoint Inhibitors. Curr Oncol Rep (2021) 23(7):79. doi: 10.1007/s11912-021-01070-6
11. Kalisz KR, Ramaiya NH, Laukamp KR, Gupta A. Immune Checkpoint Inhibitor Therapy-Related Pneumonitis: Patterns and Management. Radiographics Rev Publ Radiol Soc North Am Inc (2019) 39(7):1923–37. doi: 10.1148/rg.2019190036
12. Reddy HG, Schneider BJ, Tai AW. Immune Checkpoint Inhibitor-Associated Colitis and Hepatitis. Clin Trans Gastroenterol (2018) 9(9):180. doi: 10.1038/s41424-018-0049-9
13. Abu-Sbeih H, Tang T, Lu Y, Thirumurthi S, Altan M, Jazaeri AA, et al. Clinical Characteristics and Outcomes of Immune Checkpoint Inhibitor-Induced Pancreatic Injury. J Immunother Cancer (2019) 7(1):31. doi: 10.1186/s40425-019-0502-7
14. Barroso-Sousa R, Barry WT, Garrido-Castro AC, Hodi FS, Min L, Krop IE, et al. Incidence of Endocrine Dysfunction Following the Use of Different Immune Checkpoint Inhibitor Regimens: A Systematic Review and Meta-Analysis. JAMA Oncol (2018) 4(2):173–82. doi: 10.1001/jamaoncol.2017.3064
15. Johnson DB, Chandra S, Sosman JA. Immune Checkpoint Inhibitor Toxicity in 2018. JAMA (2018) 320(16):1702–3. doi: 10.1001/jama.2018.13995
16. Özdemir BC, Latifyan S, Perreau M, Fenwick C, Alberio L, Waeber G, et al. Cytokine-Directed Therapy With Tocilizumab for Immune Checkpoint Inhibitor-Related Hemophagocytic Lymphohistiocytosis. Ann Oncol (2020) 31(12):1775–8. doi: 10.1016/j.annonc.2020.08.2101
17. Sun JC, Lanier LL. NK Cell Development, Homeostasis and Function: Parallels With CD8+ T Cells. Nat Rev Immunol (2011) 11(10):645–57. doi: 10.1038/nri3044
18. Walsh SR, Simovic B, Chen L, Bastin D, Nguyen A, Stephenson K, et al. Endogenous T Cells Prevent Tumor Immune Escape Following Adoptive T Cell Therapy. J Clin Invest (2019) 129(12):5400–10. doi: 10.1172/JCI126199
19. Boles KS, Vermi W, Facchetti F, Fuchs A, Wilson TJ, Diacovo TG, et al. A Novel Molecular Interaction for the Adhesion of Follicular CD4 T Cells to Follicular DC. Eur J Immunol (2009) 39(3):695–703. doi: 10.1002/eji.200839116
20. Yu X, Harden K, Gonzalez LC, Francesco M, Chiang E, Irving B, et al. The Surface Protein TIGIT Suppresses T Cell Activation by Promoting the Generation of Mature Immunoregulatory Dendritic Cells. Nat Immunol (2009) 10(1):48–57. doi: 10.1038/ni.1674
21. Xu Z, Jin B. A Novel Interface Consisting of Homologous Immunoglobulin Superfamily Members With Multiple Functions. Cell Mol Immunol (2010) 7(1):11–9. doi: 10.1038/cmi.2009.108
22. Mao L, Xiao Y, Yang QC, Yang SC, Yang LL, Sun ZJ. TIGIT/CD155 Blockade Enhances Anti-PD-L1 Therapy in Head and Neck Squamous Cell Carcinoma by Targeting Myeloid-Derived Suppressor Cells. Oral Oncol (2021) 121:105472. doi: 10.1016/j.oraloncology.2021.105472
23. Kawashima S, Inozume T, Kawazu M, Ueno T, Nagasaki J, Tanji E, et al. TIGIT/CD155 Axis Mediates Resistance to Immunotherapy in Patients With Melanoma With the Inflamed Tumor Microenvironment. J Immunother Cancer (2021) 9(11):e003134. doi: 10.1136/jitc-2021-003134
24. Freed-Pastor WA, Lambert LJ, Ely ZA, Pattada NB, Bhutkar A, Eng G, et al. The CD155/TIGIT Axis Promotes and Maintains Immune Evasion in Neoantigen-Expressing Pancreatic Cancer. Cancer Cell (2021) 39(10):1342–60.e14. doi: 10.1016/j.ccell.2021.07.007
25. Chauvin JM, Zarour HM. TIGIT in Cancer Immunotherapy. J Immunother Cancer (2020) 8(2):e000957. doi: 10.1136/jitc-2020-000957
26. Anderson AC, Joller N, Kuchroo VK. Lag-3, Tim-3, and TIGIT: Co-Inhibitory Receptors With Specialized Functions in Immune Regulation. Immunity (2016) 44(5):989–1004. doi: 10.1016/j.immuni.2016.05.001
27. Hasan MM, Nair SS, O'Leary JG, Thompson-Snipes L, Nyarige V, Wang J, et al. Implication of TIGIT(+) Human Memory B Cells in Immune Regulation. Nat Commun (2021) 12(1):1534. doi: 10.1038/s41467-021-21413-y
28. O'Donnell JS, Madore J, Li XY, Smyth MJ. Tumor Intrinsic and Extrinsic Immune Functions of CD155. Semin Cancer Biol (2020) 65:189–96. doi: 10.1016/j.semcancer.2019.11.013
29. Sloan KE, Stewart JK, Treloar AF, Matthews RT, Jay DG. CD155/PVR Enhances Glioma Cell Dispersal by Regulating Adhesion Signaling and Focal Adhesion Dynamics. Cancer Res (2005) 65(23):10930–7. doi: 10.1158/0008-5472.CAN-05-1890
30. Gao J, Zheng Q, Shao Y, Wang W, Zhao C. CD155 Downregulation Synergizes With Adriamycin to Induce Breast Cancer Cell Apoptosis. Apoptosis Int J Prog Cell Death (2018) 23(9-10):512–20. doi: 10.1007/s10495-018-1473-8
31. Chan CJ, Martinet L, Gilfillan S, Souza-Fonseca-Guimaraes F, Chow MT, Town L, et al. The Receptors CD96 and CD226 Oppose Each Other in the Regulation of Natural Killer Cell Functions. Nat Immunol (2014) 15(5):431–8. doi: 10.1038/ni.2850
32. Johnston RJ, Comps-Agrar L, Hackney J, Yu X, Huseni M, Yang Y, et al. The Immunoreceptor TIGIT Regulates Antitumor and Antiviral CD8(+) T Cell Effector Function. Cancer Cell (2014) 26(6):923–37. doi: 10.1016/j.ccell.2014.10.018
33. Li X-Y, Das I, Lepletier A, Addala V, Bald T, Stannard K, et al. CD155 Loss Enhances Tumor Suppression via Combined Host and Tumor-Intrinsic Mechanisms. J Clin Invest (2018) 128(6):2613–25. doi: 10.1172/JCI98769
34. Sun H, Huang Q, Huang M, Wen H, Lin R, Zheng M, et al. Human CD96 Correlates to Natural Killer Cell Exhaustion and Predicts the Prognosis of Human Hepatocellular Carcinoma. Hepatol (Baltimore Md) (2019) 70(1):168–83. doi: 10.1002/hep.30347
35. Deuss FA, Watson GM, Fu Z, Rossjohn J, Berry R. Structural Basis for CD96 Immune Receptor Recognition of Nectin-Like Protein-5, Cd155. Struct (Lond Engl 1993) (2019) 27(2):219–28.e3. doi: 10.1016/j.str.2018.10.023
36. Levin SD, Taft DW, Brandt CS, Bucher C, Howard ED, Chadwick EM, et al. Vstm3 is a Member of the CD28 Family and an Important Modulator of T-Cell Function. Eur J Immunol (2011) 41(4):902–15. doi: 10.1002/eji.201041136
37. Stanietsky N, Simic H, Arapovic J, Toporik A, Levy O, Novik A, et al. The Interaction of TIGIT With PVR and PVRL2 Inhibits Human NK Cell Cytotoxicity. Proc Natl Acad Sci USA (2009) 106(42):17858–63. doi: 10.1073/pnas.0903474106
38. Sato K, Yamashita-Kanemaru Y, Abe F, Murata R, Nakamura-Shinya Y, Kanemaru K, et al. DNAM-1 Regulates Foxp3 Expression in Regulatory T Cells by Interfering With TIGIT Under Inflammatory Conditions. Proc Natl Acad Sci USA (2021) 118(21):e2021309118. doi: 10.1073/pnas.2021309118
39. Smazynski J, Hamilton PT, Thornton S, Milne K, Wouters MCA, Webb JR, et al. The Immune Suppressive Factors CD155 and PD-L1 Show Contrasting Expression Patterns and Immune Correlates in Ovarian and Other Cancers. Gynecol Oncol (2020) 158(1):167–77. doi: 10.1016/j.ygyno.2020.04.689
40. Wu L, Mao L, Liu JF, Chen L, Yu GT, Yang LL, et al. Blockade of TIGIT/CD155 Signaling Reverses T-Cell Exhaustion and Enhances Antitumor Capability in Head and Neck Squamous Cell Carcinoma. Cancer Immunol Res (2019) 7(10):1700–13. doi: 10.1158/2326-6066.CIR-18-0725
41. Zhang W, Anyalebechi JC, Ramonell KM, Chen CW, Xie J, Liang Z, et al. TIGIT Modulates Sepsis-Induced Immune Dysregulation in Mice With Preexisting Malignancy. JCI Insight (2021) 6(11):e139823. doi: 10.1172/jci.insight.139823
42. Liu S, Zhang H, Li M, Hu D, Li C, Ge B, et al. Recruitment of Grb2 and SHIP1 by the ITT-Like Motif of TIGIT Suppresses Granule Polarization and Cytotoxicity of NK Cells. Cell Death Differ (2013) 20(3):456–64. doi: 10.1038/cdd.2012.141
43. Judge SJ, Darrow MA, Thorpe SW, Gingrich AA, O'Donnell EF, Bellini AR, et al. Analysis of Tumor-Infiltrating NK and T Cells Highlights IL-15 Stimulation and TIGIT Blockade as a Combination Immunotherapy Strategy for Soft Tissue Sarcomas. J Immunother Cancer (2020) 8(2):e001355. doi: 10.1136/jitc-2020-001355
44. Chauvin JM, Ka M, Pagliano O, Menna C, Ding Q, DeBlasio R, et al. IL15 Stimulation With TIGIT Blockade Reverses CD155-Mediated NK-Cell Dysfunction in Melanoma. Clin Cancer Res (2020) 26(20):5520–33. doi: 10.1158/1078-0432.CCR-20-0575
45. Maas RJ, Hoogstad-van Evert JS, van der Meer JM, Mekers V, Rezaeifard S, Korman AJ, et al. TIGIT Blockade Enhances Functionality of Peritoneal NK Cells With Altered Expression of DNAM-1/TIGIT/CD96 Checkpoint Molecules in Ovarian Cancer. Oncoimmunology (2020) 9(1):1843247. doi: 10.1080/2162402X.2020.1843247
46. Kumagai S, Togashi Y, Kamada T, Sugiyama E, Nishinakamura H, Takeuchi Y, et al. The PD-1 Expression Balance Between Effector and Regulatory T Cells Predicts the Clinical Efficacy of PD-1 Blockade Therapies. Nat Immunol (2020) 21(11):1346–58. doi: 10.1038/s41590-020-0769-3
47. Fairfax BP, Taylor CA, Watson RA, Nassiri I, Danielli S, Fang H, et al. Peripheral CD8(+) T Cell Characteristics Associated With Durable Responses to Immune Checkpoint Blockade in Patients With Metastatic Melanoma. Nat Med (2020) 26(2):193–9. doi: 10.1038/s41591-019-0734-6
48. Liu Z, Zhou Q, Wang Z, Zhang H, Zeng H, Huang Q, et al. Intratumoral TIGIT(+) CD8(+) T-Cell Infiltration Determines Poor Prognosis and Immune Evasion in Patients With Muscle-Invasive Bladder Cancer. J Immunother Cancer (2020) 8(2):e000978. doi: 10.1136/jitc-2020-000978
49. Chauvin JM, Pagliano O, Fourcade J, Sun Z, Wang H, Sander C, et al. TIGIT and PD-1 Impair Tumor Antigen-Specific CD8+ T Cells in Melanoma Patients. J Clin Invest (2015) 125(5):2046–58. doi: 10.1172/JCI80445
50. He W, Zhang H, Han F, Chen X, Lin R, Wang W, et al. CD155T/TIGIT Signaling Regulates CD8(+) T-Cell Metabolism and Promotes Tumor Progression in Human Gastric Cancer. Cancer Res (2017) 77(22):6375–88. doi: 10.1158/0008-5472.CAN-17-0381
51. Liang R, Zhu X, Lan T, Ding D, Zheng Z, Chen T, et al. TIGIT Promotes CD8(+)T Cells Exhaustion and Predicts Poor Prognosis of Colorectal Cancer. Cancer Immunol Immunother CII (2021) 70(10):2781–93. doi: 10.1007/s00262-021-02886-8
52. Valhondo I, Hassouneh F, Lopez-Sejas N, Pera A, Sanchez-Correa B, Guerrero B, et al. Characterization of the DNAM-1, TIGIT and TACTILE Axis on Circulating NK, NKT-Like and T Cell Subsets in Patients With Acute Myeloid Leukemia. Cancers (2020) 12(8):2171. doi: 10.3390/cancers12082171
53. Zhang Q, Bi J, Zheng X, Chen Y, Wang H, Wu W, et al. Blockade of the Checkpoint Receptor TIGIT Prevents NK Cell Exhaustion and Elicits Potent Anti-Tumor Immunity. Nat Immunol (2018) 19(7):723–32. doi: 10.1038/s41590-018-0132-0
54. Gur C, Ibrahim Y, Isaacson B, Yamin R, Abed J, Gamliel M, et al. Binding of the Fap2 Protein of Fusobacterium Nucleatum to Human Inhibitory Receptor TIGIT Protects Tumors From Immune Cell Attack. Immunity (2015) 42(2):344–55. doi: 10.1016/j.immuni.2015.01.010
55. Lepletier A, Madore J, O'Donnell JS, Johnston RL, Li XY, McDonald E, et al. Tumor CD155 Expression Is Associated With Resistance to Anti-PD1 Immunotherapy in Metastatic Melanoma. Clin Cancer Res (2020) 26(14):3671–81. doi: 10.1158/1078-0432.CCR-19-3925
56. Lee BR, Chae S, Moon J, Kim MJ, Lee H, Ko HW, et al. Combination of PD-L1 and PVR Determines Sensitivity to PD-1 Blockade. JCI Insight (2020) 5(14):e128633. doi: 10.1172/jci.insight.128633
57. Lee YH, Lee HJ, Kim HC, Lee Y, Nam SK, Hupperetz C, et al. PD-1 and TIGIT Downregulation Distinctly Affect the Effector and Early Memory Phenotypes of CD19-Targeting CAR T Cells. Mol Ther J Am Soc Gene Ther (2021) 30(2):579–92. doi: 10.1016/j.ymthe.2021.10.004
58. Joller N, Lozano E, Burkett PR, Patel B, Xiao S, Zhu C, et al. Treg Cells Expressing the Coinhibitory Molecule TIGIT Selectively Inhibit Proinflammatory Th1 and Th17 Cell Responses. Immunity (2014) 40(4):569–81. doi: 10.1016/j.immuni.2014.02.012
59. Yang ZZ, Kim HJ, Wu H, Jalali S, Tang X, Krull JE, et al. TIGIT Expression Is Associated With T-Cell Suppression and Exhaustion and Predicts Clinical Outcome and Anti-PD-1 Response in Follicular Lymphoma. Clin Cancer Res (2020) 26(19):5217–31. doi: 10.1158/1078-0432.CCR-20-0558
60. Jin Z, Ye W, Lan T, Zhao Y, Liu X, Chen J, et al. Characteristic of TIGIT and DNAM-1 Expression on Foxp3+ γδ T Cells in AML Patients. BioMed Res Int (2020) 2020:4612952. doi: 10.1155/2020/4612952
61. Fourcade J, Sun Z, Chauvin JM, Ka M, Davar D, Pagliano O, et al. CD226 Opposes TIGIT to Disrupt Tregs in Melanoma. JCI Insight (2018) 3(14):e121157. doi: 10.1172/jci.insight.121157
62. Smyth EC, Nilsson M, Grabsch HI, van Grieken NC, Lordick F. Gastric Cancer. Lancet (Lond Engl) (2020) 396(10251):635–48. doi: 10.1016/S0140-6736(20)31288-5
63. Derks S, de Klerk LK, Xu X, Fleitas T, Liu KX, Liu Y, et al. Characterizing Diversity in the Tumor-Immune Microenvironment of Distinct Subclasses of Gastroesophageal Adenocarcinomas. Ann Oncol (2020) 31(8):1011–20. doi: 10.1016/j.annonc.2020.04.011
64. Fan Y, Ying H, Wu X, Chen H, Hu Y, Zhang H, et al. The Mutational Pattern of Homologous Recombination (HR)-Associated Genes and its Relevance to the Immunotherapeutic Response in Gastric Cancer. Cancer Biol Med (2020) 17(4):1002–13. doi: 10.20892/j.issn.2095-3941.2020.0089
65. Xu D, Zhao E, Zhu C, Zhao W, Wang C, Zhang Z, et al. TIGIT and PD-1 may Serve as Potential Prognostic Biomarkers for Gastric Cancer. Immunobiology (2020) 225(3):151915. doi: 10.1016/j.imbio.2020.151915
66. Baury B, Masson D, McDermott BM Jr., Jarry A, Blottière HM, Blanchardie P, et al. Identification of Secreted CD155 Isoforms. Biochem Biophys Res Commun (2003) 309(1):175–82. doi: 10.1016/S0006-291X(03)01560-2
67. Iguchi-Manaka A, Okumura G, Kojima H, Cho Y, Hirochika R, Bando H, et al. Increased Soluble CD155 in the Serum of Cancer Patients. PLoS One (2016) 11(4):e0152982. doi: 10.1371/journal.pone.0152982
68. Okumura G, Iguchi-Manaka A, Murata R, Yamashita-Kanemaru Y, Shibuya A, Shibuya K. Tumor-Derived Soluble CD155 Inhibits DNAM-1-Mediated Antitumor Activity of Natural Killer Cells. J Exp Med (2020) 217(4):1. doi: 10.1084/jem.20191290
69. Zhang M, Fan Y, Che X, Hou K, Zhang C, Li C, et al. 5-FU-Induced Upregulation of Exosomal PD-L1 Causes Immunosuppression in Advanced Gastric Cancer Patients. Front Oncol (2020) 10:492. doi: 10.3389/fonc.2020.00492
70. Tang W, Pan X, Han D, Rong D, Zhang M, Yang L, et al. Clinical Significance of CD8(+) T Cell Immunoreceptor With Ig and ITIM Domains(+) in Locally Advanced Gastric Cancer Treated With SOX Regimen After D2 Gastrectomy. Oncoimmunology (2019) 8(6):e1593807. doi: 10.1080/2162402X.2019.1593807
71. Lu X, Liu J, Cui P, Liu T, Piao C, Xu X, et al. Co-Inhibition of TIGIT, PD1, and Tim3 Reverses Dysfunction of Wilms Tumor Protein-1 (WT1)-Specific CD8+ T Lymphocytes After Dendritic Cell Vaccination in Gastric Cancer. Am J Cancer Res (2018) 8(8):1564–75.
72. Duan X, Liu J, Cui J, Ma B, Zhou Q, Yang X, et al. Expression of TIGIT/CD155 and Correlations With Clinical Pathological Features in Human Hepatocellular Carcinoma. Mol Med Rep (2019) 20(4):3773–81. doi: 10.3892/mmr.2019.10641
73. Ge Z, Zhou G, Campos Carrascosa L, Gausvik E, Boor PPC, Noordam L, et al. TIGIT and PD1 Co-Blockade Restores Ex Vivo Functions of Human Tumor-Infiltrating CD8(+) T Cells in Hepatocellular Carcinoma. Cell Mol Gastroenterol Hepatol (2021) 12(2):443–64. doi: 10.1016/j.jcmgh.2021.03.003
74. Ostroumov D, Duong S, Wingerath J, Woller N, Manns MP, Timrott K, et al. Transcriptome Profiling Identifies TIGIT as a Marker of T-Cell Exhaustion in Liver Cancer. Hepatol (Baltimore Md) (2021) 73(4):1399–418. doi: 10.1002/hep.31466
75. Zhang C, Wang Y, Xun X, Wang S, Xiang X, Hu S, et al. TIGIT Can Exert Immunosuppressive Effects on CD8+ T Cells by the CD155/TIGIT Signaling Pathway for Hepatocellular Carcinoma In Vitro. J immunother (Hagerstown Md 1997) (2020) 43(8):236–43. doi: 10.1097/CJI.0000000000000330
76. Chuanshan Z, Wang H, Jing L, Hou X, Li L, Wang W, et al. Involvement of TIGIT in NK Cell Exhaustion and Immune Escape in Patients and Mouse Model With Liver E. Multilocularis Infection. Hepatol (Baltimore Md) (2021) 74(6):3376–93. doi: 10.1002/hep.32035
77. Chiu DK, Yuen VW, Cheu JW, Wei LL, Ting V, Fehlings M, et al. Hepatocellular Carcinoma Cells Up-Regulate PVRL1, Stabilizing PVR and Inhibiting the Cytotoxic T-Cell Response via TIGIT to Mediate Tumor Resistance to PD1 Inhibitors in Mice. Gastroenterology (2020) 159(2):609–23. doi: 10.1053/j.gastro.2020.03.074
78. Lin C, Ren W, Luo Y, Li S, Chang Y, Li L, et al. Intratumoral Delivery of a PD-1-Blocking scFv Encoded in Oncolytic HSV-1 Promotes Antitumor Immunity and Synergizes With TIGIT Blockade. Cancer Immunol Res (2020) 8(5):632–47. doi: 10.1158/2326-6066.CIR-19-0628
79. Zhang H, Zhang Y, Dong J, Li B, Xu C, Wei M, et al. Recombinant Oncolytic Adenovirus Expressing a Soluble PVR Elicits Long-Term Antitumor Immune Surveillance. Mol Ther Oncol (2021) 20:12–22. doi: 10.1016/j.omto.2020.11.001
80. Wang T, Li P, Wan T, Tu B, Li J, Huang F. TIGIT/PVR and LncRNA ANRIL Dual-Targetable PAMAM Polymeric Nanoparticles Efficiently Inhibited the Hepatoma Carcinoma by Combination of Immunotherapy and Gene Therapy. J Drug Target (2021) 29(7):783–91. doi: 10.1080/1061186X.2021.1879088
81. Han Q, Wang Y, Pang M, Zhang J. STAT3-Blocked Whole-Cell Hepatoma Vaccine Induces Cellular and Humoral Immune Response Against HCC. J Exp Clin Cancer Res CR (2017) 36(1):156. doi: 10.1186/s13046-017-0623-0
82. Liu X, Li M, Wang X, Dang Z, Jiang Y, Wang X, et al. PD-1(+) TIGIT(+) CD8(+) T Cells Are Associated With Pathogenesis and Progression of Patients With Hepatitis B Virus-Related Hepatocellular Carcinoma. Cancer Immunol Immunother CII (2019) 68(12):2041–54. doi: 10.1007/s00262-019-02426-5
83. Ho DW, Tsui YM, Chan LK, Sze KM, Zhang X, Cheu JW, et al. Single-Cell RNA Sequencing Shows the Immunosuppressive Landscape and Tumor Heterogeneity of HBV-Associated Hepatocellular Carcinoma. Nat Commun (2021) 12(1):3684. doi: 10.1038/s41467-021-24010-1
84. Zong L, Peng H, Sun C, Li F, Zheng M, Chen Y, et al. Breakdown of Adaptive Immunotolerance Induces Hepatocellular Carcinoma in HBsAg-Tg Mice. Nat Commun (2019) 10(1):221. doi: 10.1038/s41467-018-08096-8
85. Choi J, Jo C, Lim YS. Tenofovir Versus Entecavir on Recurrence of Hepatitis B Virus-Related Hepatocellular Carcinoma After Surgical Resection. Hepatol (Baltimore Md) (2021) 73(2):661–73. doi: 10.1002/hep.31289
86. Nguyen MH, Wong G, Gane E, Kao JH, Dusheiko G. Hepatitis B Virus: Advances in Prevention, Diagnosis, and Therapy. Clin Microbiol Rev (2020) 33(2):e00046–19. doi: 10.1128/CMR.00046-19
87. Valero C, Lee M, Hoen D, Zehir A, Berger MF, Seshan VE, et al. Response Rates to Anti-PD-1 Immunotherapy in Microsatellite-Stable Solid Tumors With 10 or More Mutations Per Megabase. JAMA Oncol (2021) 7(5):739–43. doi: 10.1001/jamaoncol.2020.7684
88. Biasci D, Smoragiewicz M, Connell CM, Wang Z, Gao Y, Thaventhiran JED, et al. CXCR4 Inhibition in Human Pancreatic and Colorectal Cancers Induces an Integrated Immune Response. Proc Natl Acad Sci USA (2020) 117(46):28960–70. doi: 10.1073/pnas.2013644117
89. Sivakumar S, Abu-Shah E, Ahern DJ, Arbe-Barnes EH, Jainarayanan AK, Mangal N, et al. Activated Regulatory T-Cells, Dysfunctional and Senescent T-Cells Hinder the Immunity in Pancreatic Cancer. Cancers (2021) 13(8):1776. doi: 10.3390/cancers13081776
90. Nishiwada S, Sho M, Yasuda S, Shimada K, Yamato I, Akahori T, et al. Clinical Significance of CD155 Expression in Human Pancreatic Cancer. Anticancer Res (2015) 35(4):2287–97.
91. Lim SA, Kim J, Jeon S, Shin MH, Kwon J, Kim TJ, et al. Defective Localization With Impaired Tumor Cytotoxicity Contributes to the Immune Escape of NK Cells in Pancreatic Cancer Patients. Front Immunol (2019) 10:496. doi: 10.3389/fimmu.2019.00496
92. Marcon F, Zuo J, Pearce H, Nicol S, Margielewska-Davies S, Farhat M, et al. NK Cells in Pancreatic Cancer Demonstrate Impaired Cytotoxicity and a Regulatory IL-10 Phenotype. Oncoimmunology (2020) 9(1):1845424. doi: 10.1080/2162402X.2020.1845424
93. Jin HS, Ko M, Choi DS, Kim JH, Lee DH, Kang SH, et al. CD226(hi)CD8(+) T Cells Are a Prerequisite for Anti-TIGIT Immunotherapy. Cancer Immunol Res (2020) 8(7):912–25. doi: 10.1158/2326-6066.CIR-19-0877
94. Davis M, Conlon K, Bohac GC, Barcenas J, Leslie W, Watkins L, et al. Effect of Pemetrexed on Innate Immune Killer Cells and Adaptive Immune T Cells in Subjects With Adenocarcinoma of the Pancreas. J Immunother (Hagerstown Md 1997) (2012) 35(8):629–40. doi: 10.1097/CJI.0b013e31826c8a4f
95. Lee J, Kang TH, Yoo W, Choi H, Jo S, Kong K, et al. An Antibody Designed to Improve Adoptive NK-Cell Therapy Inhibits Pancreatic Cancer Progression in a Murine Model. Cancer Immunol Res (2019) 7(2):219–29. doi: 10.1158/2326-6066.CIR-18-0317
96. Van Audenaerde JRM, Roeyen G, Darcy PK, Kershaw MH, Peeters M, Smits ELJ. Natural Killer Cells and Their Therapeutic Role in Pancreatic Cancer: A Systematic Review. Pharmacol Ther (2018) 189:31–44. doi: 10.1016/j.pharmthera.2018.04.003
97. Bear AS, Vonderheide RH, O'Hara MH. Challenges and Opportunities for Pancreatic Cancer Immunotherapy. Cancer Cell (2020) 38(6):788–802. doi: 10.1016/j.ccell.2020.08.004
98. Brooks J, Fleischmann-Mundt B, Woller N, Niemann J, Ribback S, Peters K, et al. Perioperative, Spatiotemporally Coordinated Activation of T and NK Cells Prevents Recurrence of Pancreatic Cancer. Cancer Res (2018) 78(2):475–88. doi: 10.1158/0008-5472.CAN-17-2415
99. Masson D, Jarry A, Baury B, Blanchardie P, Laboisse C, Lustenberger P, et al. Overexpression of the CD155 Gene in Human Colorectal Carcinoma. Gut (2001) 49(2):236–40. doi: 10.1136/gut.49.2.236
100. Blessin NC, Simon R, Kluth M, Fischer K, Hube-Magg C, Li W, et al. Patterns of TIGIT Expression in Lymphatic Tissue, Inflammation, and Cancer. Dis Markers (2019) 2019:5160565. doi: 10.1155/2019/5160565
101. Shao Q, Wang L, Yuan M, Jin X, Chen Z, Wu C. TIGIT Induces (CD3+) T Cell Dysfunction in Colorectal Cancer by Inhibiting Glucose Metabolism. Front Immunol (2021) 12:688961. doi: 10.3389/fimmu.2021.688961
102. Li S, Ding J, Wang Y, Wang X, Lv L. CD155/TIGIT Signaling Regulates the Effector Function of Tumor-Infiltrating CD8(+) T Cell by NF-κb Pathway in Colorectal Cancer. J Gastroenterol Hepatol (2021) 37(1):154–63. doi: 10.1111/jgh.15730
103. Zhou XM, Li WQ, Wu YH, Han L, Cao XG, Yang XM, et al. Intrinsic Expression of Immune Checkpoint Molecule TIGIT Could Help Tumor Growth In Vivo by Suppressing the Function of NK and CD8(+) T Cells. Front Immunol (2018) 9:2821. doi: 10.3389/fimmu.2018.02821
104. Bashir A, Miskeen AY, Hazari YM, Asrafuzzaman S, Fazili KM. Fusobacterium Nucleatum, Inflammation, and Immunity: The Fire Within Human Gut. Tumour Biol J Int Soc Oncodev Biol Med (2016) 37(3):2805–10. doi: 10.1007/s13277-015-4724-0
105. Mima K, Nishihara R, Qian ZR, Cao Y, Sukawa Y, Nowak JA, et al. Fusobacterium Nucleatum in Colorectal Carcinoma Tissue and Patient Prognosis. Gut (2016) 65(12):1973–80. doi: 10.1136/gutjnl-2015-310101
106. Gur C, Maalouf N, Shhadeh A, Berhani O, Singer BB, Bachrach G, et al. Fusobacterium Nucleatum Supresses Anti-Tumor Immunity by Activating CEACAM1. Oncoimmunology (2019) 8(6):e1581531. doi: 10.1080/2162402X.2019.1581531
107. Ma B, Duan X, Zhou Q, Liu J, Yang X, Zhang D, et al. Use of Aspirin in the Prevention of Colorectal Cancer Through TIGIT-CD155 Pathway. J Cell Mol Med (2019) 23(7):4514–22. doi: 10.1111/jcmm.14332
108. Matson V, Fessler J, Bao R, Chongsuwat T, Zha Y, Alegre ML, et al. The Commensal Microbiome Is Associated With Anti-PD-1 Efficacy in Metastatic Melanoma Patients. Sci (New York NY) (2018) 359(6371):104–8. doi: 10.1126/science.aao3290
109. Dubin K, Callahan MK, Ren B, Khanin R, Viale A, Ling L, et al. Intestinal Microbiome Analyses Identify Melanoma Patients at Risk for Checkpoint-Blockade-Induced Colitis. Nat Commun (2016) 7:10391. doi: 10.1038/ncomms10391
110. Chalabi M, Fanchi LF, Dijkstra KK, Van den Berg JG, Aalbers AG, Sikorska K, et al. Neoadjuvant Immunotherapy Leads to Pathological Responses in MMR-Proficient and MMR-Deficient Early-Stage Colon Cancers. Nat Med (2020) 26(4):566–76. doi: 10.1038/s41591-020-0805-8
111. Zhou X, Ding X, Li H, Yang C, Ma Z, Xu G, et al. Upregulation of TIGIT and PD-1 in Colorectal Cancer With Mismatch-Repair Deficiency. Immunol Invest (2021) 50(4):338–55. doi: 10.1080/08820139.2020.1758130
112. Grapin M, Richard C, Limagne E, Boidot R, Morgand V, Bertaut A, et al. Optimized Fractionated Radiotherapy With Anti-PD-L1 and Anti-TIGIT: A Promising New Combination. J Immunother Cancer (2019) 7(1):160. doi: 10.1186/s40425-019-0634-9
113. Nishi K, Ishikura S, Umebayashi M, Morisaki T, Inozume T, Kinugasa T, et al. Mutant KRAS Promotes NKG2D(+) T Cell Infiltration and CD155 Dependent Immune Evasion. Anticancer Res (2020) 40(8):4663–74. doi: 10.21873/anticanres.14465
114. Fathi M, Pustokhina I, Kuznetsov SV, Khayrullin M, Hojjat-Farsangi M, Karpisheh V, et al. T-Cell Immunoglobulin and ITIM Domain, as a Potential Immune Checkpoint Target for Immunotherapy of Colorectal Cancer. IUBMB Life (2021) 73(5):726–38. doi: 10.1002/iub.2461
115. Yoshida J, Ishikawa T, Doi T, Ota T, Yasuda T, Okayama T, et al. Clinical Significance of Soluble Forms of Immune Checkpoint Molecules in Advanced Esophageal Cancer. Med Oncol (Northwood Lond Engl) (2019) 36(7):60. doi: 10.1007/s12032-019-1285-x
116. Albrecht T, Brinkmann F, Albrecht M, Lonsdorf AS, Mehrabi A, Hoffmann K, et al. Programmed Death Ligand-1 (PD-L1) Is an Independent Negative Prognosticator in Western-World Gallbladder Cancer. Cancers (2021) 13(7):1682. doi: 10.3390/cancers13071682
117. Sato Y, Tanaka S, Kinoshita M, Takemura S, Shinkawa H, Kokudo T, et al. Immunosuppressive Tumor Microenvironment in Occupational Cholangiocarcinoma: Supportive Evidence for the Efficacy of Immune Checkpoint Inhibitor Therapy. J Hepatobiliary Pancreat Sci (2020) 27(11):860–9. doi: 10.1002/jhbp.788
Keywords: TIGIT, CD155, T cells, NK cells, gastric cancer, liver cancer, pancreatic cancer, colorectal cancer
Citation: Wang D, Gu Y, Yan X, Huo C, Wang G, Zhao Y, Teng M and Li Y (2022) Role of CD155/TIGIT in Digestive Cancers: Promising Cancer Target for Immunotherapy. Front. Oncol. 12:844260. doi: 10.3389/fonc.2022.844260
Received: 11 January 2022; Accepted: 04 March 2022;
Published: 30 March 2022.
Edited by:
Sandro Matosevic, Purdue University, United StatesReviewed by:
Songguang Ju, Soochow University, ChinaAna Hennino, Institut National de la Santé et de la Recherche Médicale (INSERM), France
Copyright © 2022 Wang, Gu, Yan, Huo, Wang, Zhao, Teng and Li. This is an open-access article distributed under the terms of the Creative Commons Attribution License (CC BY). The use, distribution or reproduction in other forums is permitted, provided the original author(s) and the copyright owner(s) are credited and that the original publication in this journal is cited, in accordance with accepted academic practice. No use, distribution or reproduction is permitted which does not comply with these terms.
*Correspondence: Yumin Li, bGl5bUBsenUuZWR1LmNu; Muzhou Teng, dGVuZ216QGxldS5lZHUuY24=; Yang Zhao, ZXJ5X3poYW95YW5nQGx6dS5lZHUuY24=
†These authors have contributed equally to this work and share first authorship