- 1National Cancer Institute, Pediatric Oncology Branch, National Institutes of Health, Bethesda, MD, United States
- 2Seattle Children’s Research Institute, Seattle, WA, United States
- 3Department of Neurology, University of Washington, Seattle, WA, United States
Novel immunotherapies are increasingly being employed in pediatric oncology, both in the upfront and relapsed/refractory settings. Through various mechanisms of action, engagement and activation of the immune system can cause both generalized and disease site-specific inflammation, leading to immune-related adverse events (irAEs). One of the most worrisome irAEs is that of neurotoxicity. This can present as a large spectrum of neurological toxicities, including confusion, aphasia, neuropathies, seizures, and/or death, with variable onset and severity. Earlier identification and treatment, generally with corticosteroids, remains the mainstay of neurotoxicity management to optimize patient outcomes. The pathophysiology of neurotoxicity varies across the different therapeutic strategies and remains to be elucidated in most cases. Furthermore, little is known about long-term neurologic sequelae. This review will focus on neurotoxicity seen with the most common immunotherapies used in pediatric oncology, including CAR T cell therapy, alternative forms of adoptive cell therapy, antibody therapies, immune checkpoint inhibitors, and tumor vaccines. Herein we will discuss the incidence, pathophysiology, symptomatology, diagnosis, and management strategies currently being utilized for immunotherapy-associated neurotoxicity with a focus on pediatric specific considerations.
Introduction
The rapid emergence of novel immunotherapies has dramatically shifted the treatment paradigm in oncology, particularly in those with relapsed/refractory disease where standard therapies have failed. These novel therapies strive to overcome chemotherapeutic and radiotherapy resistance by harnessing the immune system to treat malignancies. Approaches include direct engagement of the immune system, indirect activation of the intrinsic immune response, or targeted delivery of anti-cancer therapeutics. We also include select non-immunotherapy based targeted antibody therapies in this review, such as bevacizumab, alongside other immunotherapeutic agents, given the side effect profiles and indications for use significantly overlaps with that of immunotherapies. With novel mechanisms of action, a host of unique adverse events have also materialized. Generally categorized as immune-related adverse events (irAEs), the constellation of toxicities is broad and includes inflammation-mediated manifestations, components of autoimmunity, and/or on-target/off-tumor effects amongst other etiologies (Table 1) (1, 2). Presenting as generalized and/or disease site-specific toxicities, irAEs may include localized inflammation at the disease site or multi-organ system involvement (2–4). Neurotoxicities are amongst the irAEs which are often the most worrisome and least understood (5–10). Neurologic irAEs can be grouped into 4 mechanistic categories (Figure 1): direct targeting of the nervous system due to immune dysregulation, off-tumor on-target toxicity, immune related pseudoprogression, or neurologic symptoms attributable to systemic inflammatory states. The exact mechanism of specific toxicities is often not completely clear, and there may be combination and overlap syndromes.
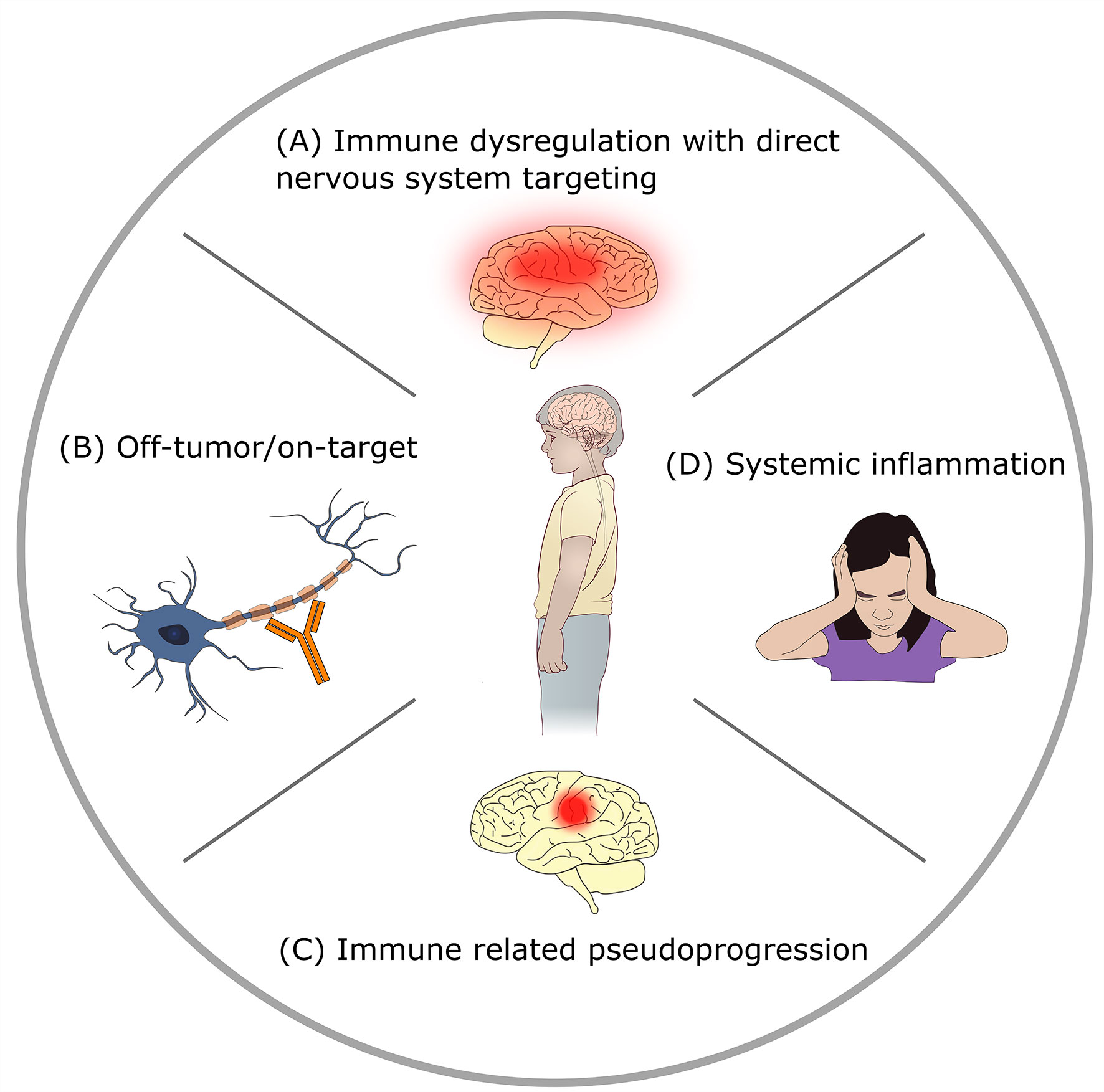
Figure 1 Mechanisms of neurotoxicity in immunotherapy. (A) Direct targeting of the nervous system by immune dysregulation: immunotherapy provokes autoimmunity or other immune-mediated CNS injury. Examples: checkpoint-blockade related encephalitis, immune effector cell associated neurotoxicity syndrome (ICANS). (B) Off-tumor on-target toxicity: immunotherapy agent targets normal tissue. Example: GD2 antibody mediated peripheral neuropathy. (C) Immune related pseudoprogression: targeting of tumor by immunotherapy causes peritumoral inflammation with increased edema. Example: tumor inflammation associated neurotoxicity (TIAN) related to GD2-CAR T therapy for brain tumors. (D) Systemic inflammation related neurologic signs and symptoms: neurologic symptoms in the setting of elevated systemic inflammatory states with absence of direct CNS injury. Example: headache after anti-tumor vaccination.
Utilization of immunotherapy in pediatric oncology continues to expand, necessitating both an enhanced understanding of how irAEs may manifest and how management strategies may differ in younger children. While generalized irAE or immune effector cell related toxicity management guidelines are increasingly available (11–14), experiences are predominantly based on treatment of adults. In the specific context of neurotoxicity, both the evaluation for and presentation of neurotoxicity may be quite disparate between children and adults, particularly in very young children. Treating clinicians must have both the knowledge of the neurotoxicities associated with specific immunotherapies and carry a high index of suspicion in the evaluation of patients with a new neurologic finding. In this review, we will focus on providing an overview of the neurotoxicity profile of the most used immunotherapeutic approaches in children and young adults and how they may manifest across the spectrum of pediatric cancer diagnoses.
Pediatric Specific Considerations for Evaluation of Immunotherapy Associated Neurotoxicity
The most important clinical consideration in children is the difference in approach toward recognition of neurotoxicity. Some tools that are used for detecting neurotoxicity in adults rely largely on language function and cannot be used for young children (15). Mild or early neurotoxicity may manifest in subtle behavioral changes that are only recognized by caregivers who know the child well. Therefore, prospective monitoring systems must be in place to catch early signs and symptoms. One excellent tool, the Cornell Assessment of Pediatric Delirium (CAPD), was validated for recognizing delirium in children hospitalized in intensive care units (16). This tool has been adopted into the guidelines for neurotoxicity monitoring after CAR T cell therapy and is now commonly used in settings where it has not yet been validated, such as acute care units and even outpatient care (15). A custom caregiver checklist was also developed to monitor pediatric and young adult patients for neurotoxicity in the acute setting post-CAR T cell therapy (17).
Apart from behavioral changes, several other signs and symptoms deserve special consideration in the pediatric population. Headache alone may or may not be considered a true neurotoxicity, yet it can be a harbinger of more serious CNS pathology. Headache can be difficult to ascertain in very young children, who may present instead with irritability. Changes in motor strength often go unrecognized in ill young children because of their limited ability to participate in a direct confrontational exam. Other unique considerations in pediatrics include the type of workup required for neurotoxicity, as children may require sedation for MRI, which can delay diagnosis, and may not be able to tolerate prolonged electroencephalography (EEG) monitoring or nerve conduction studies, hampering our ability to detect subtle toxicities which may be underrecognized.
Involvement of neurology consult services should occur prospectively, particularly in patients who have preexisting neurologic comorbidities, those with neurodevelopmental differences (18), or those receiving therapy at high risk of causing neurotoxicity. This is particularly important in younger children who require specialized neurologic exam techniques to account for developmental age. Baseline neurologic examination including detailed neurologic past medical history should be performed prior to therapy, or as soon as suspicion for neurotoxicity is raised.
There is no evidence currently to suggest differences in the mechanism of neurotoxicity-associated with immunotherapy between children and adults. Indeed, toxicities of CD19-directed CAR T cell therapy have been well studied in both children and adults with ALL, with similar rates of neurotoxicity and CRS (19). Nonetheless, for some agents there are differences in the reported incidence of specific subcategories of neurotoxicity between children and adults (Table 2).
Neurotoxicity Considerations by Oncologic Diagnosis
Hematologic Malignancies
Hematologic malignancies in pediatrics are the most diagnosed pediatric cancers and include acute and chronic leukemias, and both Hodgkin and Non-Hodgkin lymphomas (20). In general, pediatric patients with hematologic malignancies have a good prognosis, with 5-year overall survival rates ranging from 70-95% based on diagnosis (21–34). Improvement in outcomes have been noted over the last several decades in part due to cooperative group trials that incorporated multi-drug chemotherapy, early risk stratification with intensification or de-intensification therapy, implementation of CNS prophylaxis, and improvement in supportive care measures.
Despite the remarkable success rates in treating pediatric hematologic malignancies, these patients are at high risk of neurologic sequelae as a result of multi-modal therapy with systemic chemotherapy, repetitive intrathecal therapy, radiation therapy (either with total body irradiation or CNS directed therapy), and hematopoietic stem cell transplantation. Neurocognitive deficits are common, especially if therapy is administered early in childhood (35, 36). Additionally, these neurocognitive adverse events (nAEs) may manifest years after completion of therapy.
Given that immunotherapy is currently used in relapsed/refractory disease, the impact of prior therapy is important to note, as patients may already have neurologic comorbidities that may impact the patients’ ability to tolerate additional neurologic insults or leave them more vulnerable to neurotoxicity from additional therapy. As timing of immunotherapy continues to evolve with more trials moving it into the upfront treatment setting, immunotherapy may represent an attractive opportunity to decrease neurologic sequelae in this patient population.
Solid Tumors
Pediatric solid tumors located outside the central nervous system (CNS) comprise 20% of all newly diagnosed pediatric cancers. This broad category is composed of sarcomas, neuroblastoma, Wilms tumor, hepatoblastoma, germ cell tumors and other rare malignancies (37). While the overall cure rates for childhood cancer have improved significantly over the last 40 years with the use of multimodal therapy including surgery, chemotherapy, and radiation, improvements in outcome for children with solid tumors have lagged behind progress seen in hematologic malignancies (38). Children with high-risk, metastatic or recurrent disease face high mortality rates and new treatment modalities, including immunotherapy, are being actively evaluated. Nearly all children and young adults who receive chemotherapy and radiation will endure late sequelae of current curative treatments. Specifically, neurologic toxicity from therapy can include epilepsy, peripheral neuropathies, and impairment of vision and hearing (39–41). Novel treatment approaches, including immunotherapy, may ultimately impact survival and can reduce late effects of current treatment methods. Early phase clinical trials conducted by cooperative groups like Children’s Oncology Group facilitate the study of novel treatments in relatively rare patient populations. One notable example of how immunotherapy has changed the treatment paradigm for a high-risk pediatric solid tumor is the success of the anti-GD2 antibody, dinutuximab, which is FDA approved as part of first line therapy for high-risk neuroblastoma patients (42). The growing field of immunotherapy for solid tumors include T cell checkpoint inhibitors, monoclonal antibodies, antibody-drug conjugates, genetically engineered immune cells and vaccines. Unique neurotoxicity considerations for patients with solid tumors are largely attributed to the immunotherapy itself as opposed to the site or extent of disease.
Central Nervous System Tumors
Primary tumors of the CNS are the second most diagnosed pediatric malignancy, and the leading cause of cancer death in the pediatric population (43, 44). Approximately 550 children die from brain tumors annually in the US, the majority from high grade gliomas (HGG), medulloblastomas, and atypical teratoid rhabdoid tumors (44, 45). Treatment for CNS tumors typically consists of chemotherapy, radiation, and/or surgery, which commonly leaves survivors with debilitating morbidity and devastating neurologic sequelae including neurocognitive delays, neuroendocrine abnormalities, sensory disturbances, hearing loss, and seizure disorders (46–48).
Immunotherapy for pediatric brain tumors is evolving rapidly as a promising new treatment that can potentially improve both morbidity and neurocognitive outcomes in survivors (49–51). Importantly however, several key considerations including route of delivery and toxicity profile for CNS-targeted immunotherapies need to be investigated to optimize immunotherapy for childhood CNS tumors. In contrast to solid and hematologic malignancies, where systemic delivery of therapy has demonstrated the ability of treatment to reach sites of disease, the unique immune privilege of the CNS and the role of the blood-brain-barrier (BBB) need to be investigated to determine the ideal route of administering novel therapies. Additionally, tumor-targeting immune activity is likely to induce focal inflammation and edema in the CNS, which can cause new or worsening neurologic signs and symptoms such as weakness or focal seizures.
Another major challenge in CNS tumor immunotherapy is how to distinguish irAEs (also known as immune-related pseudoprogression) from tumor progression, which is important for ongoing medical treatment management. There are currently no imaging techniques that can reliably distinguish immune-related pseudoprogression from tumor growth in the acute setting (52). In 2015, immunotherapy Response Assessment for Neuro-Oncology (iRANO) MRI criteria were developed to account for possible immune-related pseudoprogression (53). However, the iRANO criteria have not yet been extensively validated, and will likely require further refinement (54, 55). When immune-related pseudoprogression is suspected, steroids are typically the first-line therapy, but clinical responses to steroids are similar for treatment-related and tumor-related edema and inflammation.
In addition to primary CNS tumors, brain metastases from non-CNS primary solid tumors and hematologic malignancies also cause significant morbidity. Brain metastases occur in approximately 1.4-4.9% of children with solid tumors (56–60), and approximately 10-20% of children with leukemia/lymphoma (61). Despite their high potential for morbidity, brain metastases are particularly poorly studied in the field of pediatric cancer immunotherapy. Toxicities from immunotherapies would be expected to be similar as for primary CNS tumors (local immune activation within the CNS), with possible overlap of neurotoxicity related to systemic immune activation.
Neurotoxicity Manifestations by Immunotherapeutic Treatment Approach
Adoptive Cell Therapy
General
Adoptive cell therapy uses immune cells to target cancer epitopes, either by selecting tumor-targeting immune cell subsets, or by genetically modifying them. The current most successful modality, chimeric antigen receptor (CAR) T cells, uses the latter approach. CARs are synthetic molecules that combine the antibody recognition properties (non-MHC restricted) of a B cell and the effector functions of a T cell. They consist of two domains, an extracellular binder (typically a single chain variable fragment) against a specific cell surface tumor epitope, and an intracellular signaling domain that induces target killing and T cell proliferation (62–66). The CAR transgene is introduced into autologous or allogeneic T cells, enabling them to identify and eliminate any cells that express the target antigen. This includes tumor cells (on-target/on-tumor activity) and normal cells expressing the target epitope (on-target/off-tumor activity). In addition, CAR T cell activity and expansion is typically accompanied by significant systemic cytokine release (62–66). Other types of adoptive cell therapy that have been tested in children include genetically modified T cell receptors (TCRs) and Natural Killer (NK) cell therapy. Bispecific antibodies, which serve as a linker between immune cells and cancer targets, are covered in the antibodies section of this review.
Hematologic Malignancies
CAR T cell therapy has revolutionized cancer treatment by inducing durable remissions in pediatric patients with relapsed/refractory B cell leukemia (B-ALL). Several studies have been published using CAR T cells for treatment of pediatric patients with relapsed/refractory B cell hematologic malignancies and have demonstrated impressive complete remission rates of 60-90% (67–72). The pivotal phase II global registration trial for CD19 targeted CAR T cells led to the historic, first pediatric FDA approved CAR product, tisagenlecleucel, for children and young adults up to age 25 with relapsed/refractory B-ALL (68, 73). Common toxicities (incidence > 20%) that occur post-CAR therapy in pediatric patients with B-ALL include CRS, neurotoxicity, infection, hypogammaglobulinemia, and fatigue (67–72, 74).
Neurotoxicity Experience in CAR T Cells
Neurotoxicity, now known as immune effector cell-associated neurotoxicity syndrome (ICANS) (15), is a common adverse event associated with CAR T cell therapy, with variable incidence of 7%-72% in published trials using CD19, CD22, or CD19/22 CAR T cells in children and young adults with hematologic malignancies (67–72, 74–77). In a recently published donor-derived CD7 CAR T cell trial for T cell ALL (T-ALL), incidence of ICANS was 15%, and all cases were grade 1 and associated with CNS disease (78). More severe cases of ICANS (≥ grade 3) have been described in up to 20% of pediatric patients treated with CAR T cell therapy. Clinical manifestations of ICANS vary, with most common presentations including depressed levels of consciousness, confusion, headache, tremors, and language disturbances (67–72, 74–77). More severe presentations of encephalopathy, seizures, and coma have occurred in up to 20% of patients, with rare cases of fatal cerebral edema (77, 79, 80). The incidence of seizures also varied significantly across studies, 0-20%, with most seizures being generalized tonic-clonic (67–72, 74–77). ICANS is often preceded by CRS onset, typically presenting within 7 days post-CAR infusion.
Acute neurocognitive functioning and patient reported outcomes measures have also been evaluated in pediatric patients receiving CAR therapy. Data collection in this patient population is feasible, with stable neurocognitive function and clinically meaningful health-related quality of life improvements demonstrated over time (17, 81).
As the application for CAR T cells continue to broaden, so too may the toxicity profile. Several hematologic CAR T cell trials in pediatrics are ongoing including those targeting CD5 and CD7 in T-ALL, and CD33 and CD123 in AML. Additionally, combinatorial CAR constructs with CD19, CD22, and/or CD20 are currently in clinical trials.
Pathophysiology of ICANS
The pathophysiology of ICANS post-CAR therapy remains under active investigation. Endothelial cell activation, vascular and blood brain barrier disruption, vascular leak, systemic inflammatory response with CRS, and off-tumor/on-target toxicity are all possible mechanisms by which neurotoxicity can occur in pediatric patients (10, 76, 77, 82, 83). Indeed, the highest risk of ICANS is typically seen during the acute CAR T cell expansion, which suggests that the systemic inflammatory surge drives cytokine mediated neurotoxicity, rather than a local CNS cytokine production (77). Mouse models have suggested possible roles for IL-1 and GM-CSF signaling underlying the development of neurotoxicity (84, 85).Additionally, this systemic inflammation can also lead to endothelial activation, astrocyte injury, and increased permeability of the blood brain barrier (76, 77). However, an association of CAR T neurotoxicity with elevated Angiopoietin-2 levels, indicative of endothelial activation, was shown in adults (10) but not children (77, 86). Increased CSF glial fibrillary acidic protein (GFAP), and S100 calcium-binding protein (S100b), were found to be elevated in the acute setting post-CAR, indicating astrocyte injury and activation, which could lead to osmotic dysregulation in the brain potentially causing or contributing to cerebral edema (77, 83). In a mouse model, blockade of brain capillaries by circulating leukocytes was associated with the development of neurotoxicity (87).CAR T cell trafficking to the CNS has been demonstrated in several pivotal pediatric trials, but presence or quantity of CAR T cells in the CSF does not predict whether ICANS will occur (67, 68, 70, 88, 89). In fact, CAR T cells are detected in the CSF of patients with and without ICANS. Recently, a study demonstrated CD19+ mural cells surrounding the endothelium of the brain, suggesting that off-tumor/on-target toxicity of CD19 CAR T cells can contribute to neurotoxicity (90). However, this finding needs to be cautiously interpreted as ICANS is seen with CAR T cells targeting other antigens (B cell maturation antigen, or CD22) and not all patients who receive CD19 directed CAR T cells develop neurotoxicity.
Risk Factors for CAR T Cell ICANS
The presence and severity of cytokine release syndrome is the strongest and most consistent risk factor for ICANS in pediatric CAR T cell patients (68, 76, 77, 91). There may also be additional risk conferred by pre-existing neurologic comorbidities (76, 91), including abnormal baseline brain MRI (77). Differences in demographics, CNS disease status, treatment history including prior brain radiation and bone marrow transplant, bone marrow disease burden at the time of CAR infusion, lymphodepletion regimen, or CAR T cell dose was not associated with development of ICANS in pediatric patients (70, 77, 91). It is important to note that disease burden, lymphodepletion regimen, and CAR T cell dose are associated with the development of ICANS in adult patients with hematologic malignancies, which likely affect the incidence and severity of CRS (10, 92, 93).
Evaluation and Management of CAR T Cell Related ICANS
The American Society for Transplantation and Cellular Therapy (ASTCT) grading criteria are now used to grade ICANS (15). ICANS grading incorporates a 10-point encephalopathy score for children >12 years and adults (ICE) or the CAPD score for children <12 years, as well as additional neurologic domains including level of consciousness, seizures, motor symptoms, and signs of increased intracranial pressure (ICP) or cerebral edema. Grading is typically based on the most severe symptoms present in each category. Treating medical teams need to perform detailed past medical histories including evaluation of any prior treatment-related neurologic toxicities and neurologic risk factor comorbidities (e.g., seizure disorder) in each patient prior to receipt of CAR T cells. Baseline neurologic examination should also include ICE or CAPD scores. In patients with a history of neurologic adverse events, CNS disease, or focal findings on exam, baseline neuroimaging should be considered. Additionally, for those deemed high-risk (e.g., those who have a prior history of seizures), or patients receiving CAR products that have high neurotoxicity incidence, anti-seizure prophylaxis prior to receipt of CAR T cells should be initiated. Post-CAR T cell infusion, patients should be monitored for the development of neurotoxicity with routine neurologic exams inclusive of ICE or CAPD scores, especially once cytokine release syndrome has started. In patients who develop ICANS, work up should be performed based on the severity of symptoms present, with routine labs, neurology consult, neuroimaging, EEG, and evaluation for infectious etiologies, with consideration for a lumbar puncture if deemed safe (13).
Based on the clinical data that has emerged from CD19-directed therapy in pediatric and young adult patients receiving CAR T cells, management of ICANS remains largely supportive, with administration of anti-pyretics and/or antiseizure medications. Although tocilizumab has demonstrated remarkable results in abrogating CRS severity, it has not proven beneficial in treatment of ICANS (10, 67, 68, 77, 92, 94). Based on ASTCT grading criteria, use of corticosteroids for ICANS should be considered in patients with grade 2 ICANS, and is recommended for patients who have grade 3 or grade 4 ICANS (13), with consideration of intrathecal hydrocortisone administration in cases of severe ICANS (95). Additionally, in patients with seizures, levetiracetam is recommended (96). When there is suspicion for worsening ICANS or signs of increased ICP, patients should be transferred to the ICU for close monitoring and initiation of urgent management as clinically indicated. In a majority of pediatric ICANS cases, symptoms typically resolve by day 28, however, rare chronic neurologic sequelae and fatal cases have been reported (77).
Solid Tumors
There are many ongoing adoptive cell therapy trials for solid tumors utilizing a patient’s own T cells or NK cells to directly target tumor cells. There are currently published results from clinical trials of CAR modified T cells or NK cells targeting CD171/L1CAM, GD2, and HER2 in pediatric patients with solid tumors (97–99, 102). All trials thus far show feasibility and safety of infusing CAR modified T cells or NK cells, with no obvious neurotoxicity. GD2 and CD171/L1CAM are both proteins known to be expressed by cells in the central nervous system and peripheral nerves, but no overt toxicities have been observed in clinical trials targeting these proteins (96–101). There is one published study of T cells genetically engineered with an NY-ESO-1 reactive T cell receptor (TCR) that includes young adults with synovial sarcoma, where antitumor efficacy was demonstrated and no neurotoxicity was seen (103). Of note, Guillain-Barre syndrome has been observed in adults treated with NY-ESO-1 directed T cell receptor therapy (104).
CNS Tumors
Multiple pediatric CNS tumor CAR T cell trials are underway, as well as trials using tumor specific T cells, but toxicity data is just beginning to emerge (105). Common to all studies is that focal neurologic symptoms were frequently seen after CAR T cell treatment for brain tumors, but typically the authors were not able to definitively distinguish progressive disease from immune-mediated toxicity. Results from two HER2-targeting trials showed a good safety profile. In a mixed age trial of HER2-CAR transduced virus specific T cells for glioblastoma that included 7 children, one child had a neurologic adverse event. This was one of the two patients among the 17 total patients who developed seizures and/or headaches (106). In a study of intracranially delivered HER2 CAR T cells, 3 of 3 patients developed headache and/or back pain. One patient had transient worsening of baseline neurologic deficits after the first CAR T cell dose, which was accompanied by increased peritumoral edema and contrast enhancement on imaging. Although this constituted a possible immune response, the patient had confirmed progressive disease 2 months after initiating treatment and did not meet iRANO criteria for pseudoprogression (107). A trial of GD2-targeting CAR T cells for diffuse intrinsic pontine gliomas and spinal cord diffuse midline gliomas showed evidence of efficacy, and evidence of intratumoral inflammation which the authors termed Tumor Inflammation-Associated Neurotoxicity (TIAN) (105, 108). Additional trials targeting HER2, GD2, B7H3, EGFR, and IL13Rα2 are ongoing.
Immune Checkpoint Inhibitors
General
Immune checkpoint proteins are a part of the adaptive immune system and their role is to prevent the immune system from over-engaging and destroying healthy cells (109). Under normal conditions, inhibitory checkpoint proteins work by engaging and binding to partner proteins and often initiate signaling pathways that suppress T cell function. Sometimes, however, cancer cells can evade detection by simulating immune checkpoint proteins thereby inhibiting T cell mechanics. Drugs that block the inhibitory checkpoint proteins, known as immune checkpoint inhibitors (ICIs), target immune checkpoints and can overcome tumor-mediated inhibition of T cell function. This effectively restores immune system function and often leads to T cell activation and tumor killing. ICIs have revolutionized cancer treatment paradigms and are approved for the treatment of multiple adult and pediatric malignancies (110, 111). With disruption of the checkpoint pathway, effectively taking the brakes off the immune system, ICIs can lead to a spectrum of inflammatory side effects including gastrointestinal, dermatologic, and endocrine toxicities (112). In the adult population, the incidence of neurologic complications ranges from 2-4% (1). In large pharmacovigilance studies, the most commonly reported neurologic syndromes were inflammatory myopathies, myasthenia gravis, noninfectious encephalitis or meningitis, and peripheral neuropathy (113, 114). Relapses of preexisting autoimmune neurologic conditions such as multiple sclerosis can occur (115, 116).
Neurotoxicity Experience in Immune Checkpoint Inhibitors
Hematologic and Solid Tumor Malignancies
Use of checkpoint inhibitors in pediatric clinical trials often includes patients with different oncologic diagnoses including hematologic (Hodgkin’s and Non-Hodgkin’s lymphoma) and solid tumor malignancies, with few cases reported in leukemia (117–121).
The overall reported incidence of neurotoxicity across trials ranges from 20-53%, with headaches being the most common neurologic symptom reported (118, 120, 121). Other neurologic side effects that occurred less commonly included blurred vision, seizures, and hemiparesis, with reports of severe toxicity such as stroke, blindness, encephalitis, and spinal cord compression occurring at approximately 1% incidence (120, 121). (See Table 3 for neurotoxicity related to individual agents in the larger pediatric clinical trials). Additionally, hypophysitis, which is a common irAE (122), can develop in patients treated with ICIs and often presents with concurrent neurologic symptomatology. Thus, assessment of endocrine function should always be part of the evaluation of neurologic complications of checkpoint blockade.
Central Nervous System Tumors
With ICIs, two types of neurologic toxicities can be expected: systemic immune-mediated neurologic disorders, which can occur with ICI use for any indication, and local immune activation at the tumor site, which is unique to brain tumors. Although these presentations are typically easy to distinguish, there may be overlap, and both will likely respond to steroids.
No randomized controlled trial results have yet been published on the use of ICI for treatment of pediatric brain tumors. Efficacy against malignant gliomas in pediatric patients with congenital mismatch repair deficiency (CMMRD) has been described in case reports, with clinical trials ongoing. Two siblings with CMMRD and glioblastoma responded to nivolumab, with their courses complicated by focal seizures and reversible radiographic pseudoprogression (123). In children without CMMRD, transient radiographic responses were seen in 3 of 10 patients with a variety of malignant CNS tumors, and no neurologic toxicities were reported (124). After treatment with 3 doses of nivolumab, a 10-year-old with glioblastoma developed successively worsening severe peritumoral edema; the first time it was reversible with steroids, the second time she required hemicraniectomy, and the third time she died (125). In this case, it was difficult to discern whether this death was from immune response or from tumor progression.
Pathophysiology, Risk Factors, Evaluation, and Management of ICI-Related Neurotoxicity
The mechanisms of checkpoint-blockade induced neurotoxicity are incompletely understood. Since most ICI toxicities have a high degree of overlap with known autoimmune or inflammatory conditions, failure of self-tolerance (1) and contributions from B cell and T cell mediated mechanisms are suspected (126). Indeed, increased clonal T cell expansion is associated with higher risk of ICI toxicity (127). Autoantibodies are frequently found in patients with ICI-related nAEs, with profiles distinct from but overlapping with typical paraneoplastic antibody profiles (128, 129). Thus, patients with preexisting autoimmune neurologic conditions such as multiple sclerosis or myasthenia gravis are at risk of relapse during ICI treatment (115, 116, 130).
Work up for neurotoxicity may include neuro-imaging, CSF studies, ophthalmologic exam, neurophysiologic testing for peripheral nerve pathologies, and antibody titers (131, 132). An endocrine workup should also be considered given the high incidence of endocrine complications during ICI treatment, particularly with ipilimumab (133).
Treatment of neurologic conditions related to ICI treatment is generally similar to interventions for when the same syndromes occur without ICI use. There is no evidence beyond expert opinion to support this approach. Depending on the severity of irAEs, immunotherapy may need to be paused or discontinued. If this is not sufficient, immunomodulators, primarily corticosteroids, may be indicated, as laid out in the Society for Immunotherapy of Cancer (SITC) and American Society of Clinical Oncology (ASCO) consensus guidelines of care for ICIs (11, 12, 134). For nAEs that are proven or suspected to be autoantibody-mediated, such as Guillain-Barré syndrome, IVIG or plasmapheresis are recommended (131).
Antibody-Based Therapy
General
Antibody-based therapy in cancer therapeutics utilizes the recognition properties of an antibody to bind to a specific antigen on a malignant cell. Upon binding, the immune system triggers downstream processes that promote cell destruction and apoptosis either through direct cytotoxicity, non-restricted activation of cytotoxic T cells, and/or Fc-mediated immune effector engagement (135). The toxicity profile of the antibody-based therapy will be dependent on whether an antibody is conjugated (bound to another substrate such as an enzyme, toxin, or inorganic compound) or unconjugated, as conjugation itself can alter the side effect profile. This section will focus on three categories, bispecific T cell engagers, unconjugated antibodies, and antibody-drug conjugates.
Hematologic Malignancies
Bispecific T Cell Engager
Blinatumomab
Antibody-based therapy in pediatric hematologic malignancies has demonstrated great clinical success in treating patients with relapsed/refractory acute lymphoblastic leukemia. Blinatumomab is a 55 kD-fusion protein that is a bispecific T cell engager that binds to and activates CD3+ T cells and links these T cells to the CD19 surface antigen on leukemia cells. Blinatumomab creates a synapse between the T cells and CD19+ cells, which results in upregulation of cell adhesion molecules, production of cytolytic proteins, release of inflammatory cytokines, and causes proliferation of T cells which results in lysis of CD19+ cells (136). Several studies have been published demonstrating complete remission rates of 30-50% in patients with relapsed/refractory ALL, with improved disease-free survival as compared to chemotherapy cohorts alone (137–139). Cytokine release syndrome and neurotoxicity are serious and common side effects that can occur with blinatumomab (136).
Neurotoxicity Experience in Blinatumomab
In pediatric patients with ALL receiving blinatumomab, neurologic toxicities have occurred in approximately 32% of patients in published reports (137–148). Most events occur early within the first treatment cycle and are usually associated with the start of the infusion or during the dose escalation phase. The most common neurologic manifestations in pediatric ALL patients are headache and tremor. Grade 3 or higher neurologic toxicities following initiation of blinatumomab occur in approximately 5% of pediatric patients and included seizures, encephalopathy, tremor, neuralgia, depressed level of consciousness, dysarthria, agitation, cranial nerve disorder, somnolence, and headaches (137–148). It is important to note that there is extremely limited data (case reports) of patients with active central nervous system ALL receiving blinatumomab as these patients were excluded from clinical trials, thus the incidence of neurotoxicity in this population is unknown.
Pathophysiology of Blinatumomab-Related Neurotoxicity
The pathophysiology of neurotoxicity post-blinatumomab is elusive and remains an active area of research. T cell adhesion to blood vessel endothelium, subsequent endothelial activation, and T cell migration into the perivascular space has been described as a potential mechanism for neurotoxicity (149). Additionally, histopathologic examinations and analyses of CSF in patients treated with blinatumomab demonstrated T cells at the brain microvascular endothelium and in the CSF. Interestingly, blinatumomab was detectable in the CSF, including in those patients with no to mild disturbance of their blood-CSF barrier (measured by CSF albumin concentration) (149). However, no direct relationship with CSF levels of blinatumomab and neurotoxicity have been seen.
Risk Factors for Blinatumomab Neurotoxicity
Risk factors in pediatric patients for development of neurotoxicity with blinatumomab treatment are not well defined, however higher doses of blinatumomab have correlated with higher incidence of neurotoxicity (150). In adult patients, a lower B:T cell ratio has been demonstrated to increase incidence of nAEs (149), however this has not been validated in pediatric patients.
Evaluation and Management of Blinatumomab-Related Neurotoxicity
In patients who develop treatment-associated neurotoxicity, work up should be performed based on symptoms present and severity, with neuroimaging and EEG performed in those with suspected seizures or severe nAEs. The majority of events resolve with a short interruption in the infusion, dose de-escalation, or complete discontinuation of therapy, and corticosteroids can be used in the presence of severe neurotoxicity. Very few fatalities in pediatric and young adult patients have occurred post blinatumomab (148, 149).
Monoclonal, Unconjugated, and Conjugated Antibodies
Targeted therapy using monoclonal, conjugated, and unconjugated antibodies has been utilized in early phase clinical trials, with some drugs now included in upfront treatment in hematologic malignancies. These include antibodies targeting CD20 (e.g., rituximab), CD22 (e.g., inotuzumab, epratuzumab), and CD30 (e.g., brentuximab vedotin) with specific clinical trials discussed in Table 3. Broadly, use of these antibodies in pediatric hematologic malignancies has shown an incidence of neurotoxicity of up to 30% in published trials, with the most common neurologic adverse events being pain, peripheral neuropathy, and headache (151–154). Other more rare and serious adverse events seen include chorioretinitis, cerebellar syndrome, cognitive disturbances, encephalopathy, intracranial hemorrhage, and meningitis (155, 156).
Solid Tumors
Bispecific T Cell Engager
Clinical trials with BITEs in pediatric solid tumor are ongoing with no toxicity data available.
Monoclonal Antibodies
Many unmodified and modified monoclonal antibodies have been evaluated for high-risk, relapsed, and refractory solid tumors. Reported neurotoxicity from published clinical trials on specific agents is summarized in Table 3. In clinical trials evaluating multiple agents, it is not possible to determine if neurotoxicity is directly caused by the monoclonal antibody. The disialoganglioside GD2 is a common monoclonal antibody target in pediatric oncology. Neuropathic pain is a well-described toxicity of anti-GD2 antibody therapy due to on-target binding of pain fibers and activation of the complement system (157). Co-administration of nonsteroidal anti-inflammatory drugs, gabapentinoids, and analgesic drugs, as well as temporarily stopping and slowing the infusion are primary ways to mitigate pain in patients. Common sites of pain are the abdomen, chest, back, and extremities. Pain is usually observed shortly after the start of the infusion, is most frequent during the first cycle of anti-GD2 therapy, and typically decreases in frequency during subsequent cycles (158).
Adie’s pupil is an ocular abnormality associated with anti-GD2 antibody therapy. It is characterized by mydriasis and accommodation deficits and is thought to be due to binding of antibody to neural structures in the ciliary and sphincter muscles of the eye. Most vision abnormalities can be corrected with prescription glasses and symptoms often improve or completely resolve after completion of therapy (159).
Posterior reversible encephalopathy syndrome (PRES) is also associated with GD2 targeting antibody therapy and can present with hypertension, seizures, headache, visual disturbance, and/or altered mentation. Characteristic radiographic findings of PRES on MRI of the brain are edematous changes most often seen in the parietal and occipital lobes. Prior brain irradiation is significantly associated with incidence of PRES in patients who received the anti-GD2 antibody, 3F8. Most patients have complete resolution of symptoms over weeks (160).
CNS Tumors
Bispecific T-Cell Engagers
Bispecific T cell engagers are in clinical trials for pediatric CNS tumors however toxicity data is not yet available.
Monoclonal, Unconjugated, and Conjugated Antibodies
Bevacizumab is the most frequently used antibody-drug in brain tumors (161, 162). It acts by blocking angiogenesis via neutralizing free circulating VEGF-A. Since it reduces contrast enhancement of tumors, interpretation of tumor response by imaging criteria alone is problematic. In pediatric trials, bevacizumab has been used together with chemotherapy and/or radiation. While safety profiles are favorable, efficacy has not been well established due to small trial size. Serious nAEs are rare; the most common of these are intracranial hemorrhages (ICH), which affect 1-4% of patients and are typically intratumoral (163–166). Importantly however, control groups not receiving bevacizumab have similar rates of ICH, which has been seen in primary brain tumors in children and adults, as well as in those with brain metastases (167–170). Other rare but serious reported adverse events include CNS ischemia (Table 3) (165).
EGFR is frequently expressed by pediatric brain tumors making EGFR a suitable epitope for tumor-targeting immunotherapies (171). The antibody drugs cetuximab, nimotuzumab, and depatuxizumab (172) have been tested in children, and generally have been well tolerated (173, 174), with rare cases of headaches and ICH seen. Depatuxizumab, which targets a tumor specific EGFR epitope, has been shown to be safe in adult studies (175). However, the drug-antibody conjugate depatuxizumab mafodotin has caused corneal keratopathy in 33-81% of adult patients with GBM (175, 176). Pediatric trials of depatuxizumab mafodotin for HGG have been completed but not yet been published.
Multiple radiolabeled antibody conjugates are in clinical trials for pediatric brain tumors but no toxicity data is available yet.
Vaccine Based Therapy
General
Vaccines for oncologic therapeutics contain tumor-associated antigens that work by engaging the immune system to recognize and react to these antigens and destroy cancer cells that contain them. The goal of therapeutic cancer vaccines is to induce tumor regression, eradicate minimal residual disease, and establish lasting anti-tumor memory (177). Neurologic toxicities have only been described in vaccine trials for brain tumors, where distinction between tumor progression and irAE is extremely challenging. Although it is plausible that a tumor-directed immune response could cause peritumoral inflammation, this has not been conclusively demonstrated. Thus, there is currently no evidence for a specifically immune-related neurologic syndrome associated with tumor vaccine.
Hematologic Malignancies
In pediatric hematologic malignancies, there is limited experience utilizing vaccines for therapeutic interventions. Wilms tumor gene, WT1, vaccine has been used with limited clinical data in patients with acute leukemias, either in the setting of minimal residual disease post hematopoietic stem cell transplant or in those with relapsed disease, without any reported neurotoxicity (178, 179).
Solid Tumors
There are a limited number of published clinical trials utilizing tumor antigen-derived peptide and dendritic cell vaccines in pediatric solid tumors. One study evaluating the NCCV Cocktail-1 vaccine, a combination of peptides derived from the KOC1, FOXM1, and KIF20A cancer antigens, described mild headache as a toxicity (16.7%) (180). Some vaccine studies reported injection site pain (180–183). No neurotoxicity, including complaints of pain, were noted in all other published vaccine trials for pediatric patients with solid tumors (184–186). All published vaccine studies in pediatric patients thus far have shown safety, feasibility, and some anti-tumor activity.
CNS Tumors
Tumor vaccines have been the most extensively studied immunotherapy in the pediatric brain tumor population (187). Peptide vaccines to sensitize against tumor-specific antigens have been safe, but few antitumor responses have been described despite successful sensitization. Injection-site reactions and mild flu-like symptoms including fever and headache occur in most patients. When nAEs occurred, they were focal in nature (e.g., gait disturbances, weakness, cranial nerve palsies) and attributable to the primary tumor site, with a differential diagnosis of tumor progression versus immune-related pseudoprogression (188–190). (See Table 3 for more vaccine and trial specific details).
Summary
Pediatric cancer immunotherapy comprises a diverse set of approaches for heterogeneous diseases, with distinct biology and mechanism of action of immunotherapy approaches. This makes it inherently challenging to draw conclusions on risks and benefits given small sample sizes. Current efforts are focused primarily on creation of novel immunotherapies to target tumors more effectively, and limit broad, more systemic toxicities seen with chemotherapy and radiation therapy. However, the emergence of neurotoxicity as a key adverse event in immune cell engaging therapies for hematologic malignancies has invigorated interest in this topic in the pediatric population.
Compared with the ability to create novel targeted therapies and our level of understanding on efficacy and target choice of immunotherapies, the mechanistic understanding of these novel toxicity profiles is lagging, at least in part due to lack of suitable in vitro and in vivo models to help evaluate these toxicities. Although the mechanisms of neurotoxicity remain unsolved, efforts at understanding the pathophysiology of this toxicity must be boosted to engineer safer novel therapies and inform diagnostic and therapeutic toxicity guidelines. For instance, based on pre-clinical models noting increased IL-1 in xenograft models post-CAR therapy, several clinical trials are now investigating use of anakinra as a therapeutic strategy to mitigate neurotoxicity. Future directions should focus on collaborative larger scale trials to enhance data sharing and to develop algorithms for early detection and effective treatment strategies for neurotoxicity.
Additionally, through multicenter trials, prospective evaluation of biomarkers and ancillary studies such as long-term neurocognitive assessments can be performed and may help validate biomarkers and enhance clinicians ability to identify patients at risk of developing acute and long-term neurotoxicity after novel immunotherapies.
Author Contributions
All authors planned and wrote the manuscript and contributed to the article and approved of the submitted version.
Funding
This work was supported in part by the Intramural Research Program, Center of Cancer Research, National Cancer Institute and NIH Clinical Center, National Institutes of Health (ZIA BC 011823, N.N.S), and the National Institute of Neurological Disorders and Stroke Mentored Clinical Scientist Research Career Development Award (1K08NS118138-01, JG).
Author Disclaimer
The content of this publication does not necessarily reflect the views of policies of the Department of Health and Human Services, nor does mention of trade names, commercial products, or organizations imply endorsement by the U.S. Government.
Conflict of Interest
The authors declare that the research was conducted in the absence of any commercial or financial relationships that could be construed as a potential conflict of interest.
Publisher’s Note
All claims expressed in this article are solely those of the authors and do not necessarily represent those of their affiliated organizations, or those of the publisher, the editors and the reviewers. Any product that may be evaluated in this article, or claim that may be made by its manufacturer, is not guaranteed or endorsed by the publisher.
Acknowledgments
We thank Lila Faulhaber with assistance in creating artwork for this manuscript.
References
1. Martins F, Sofiya L, Sykiotis GP, Lamine F, Maillard M, Fraga M, et al. Adverse Effects of Immune-Checkpoint Inhibitors: Epidemiology, Management and Surveillance. Nat Rev Clin Oncol (2019) 16(9):563–80. doi: 10.1038/s41571-019-0218-0
2. Morris EC, Neelapu SS, Giavridis T, Sadelain M. Cytokine Release Syndrome and Associated Neurotoxicity in Cancer Immunotherapy. Nat Rev Immunol (2022) 22:85–96. doi: 10.1038/s41577-021-00547-6
3. Fan Y, Xie W, Huang H, Wang Y, Li G, Ging Y, et al. Association of Immune Related Adverse Events With Efficacy of Immune Checkpoint Inhibitors and Overall Survival in Cancers: A Systemic Review and Meta-Analysis. Front Oncol (2021) 11:633032. doi: 10.3389/fonc.2021.633032
4. Jacob JB, Jacob MK, Parajuli P. Review of Immune Checkpoint Inhibitors in Immuno-Oncology. Adv Pharmacol (2021) 91:111–39. doi: 10.1016/bs.apha.2021.01.002
5. Wesley SF, Haggiagi A, Thakur KT, De Jager PL. Neurological Immunotoxicity From Cancer Treatment. Int J Mol Sci (2021) 22(13) 22(13):6716. doi: 10.3390/ijms22136716
6. Diamanti L, Picca A, Bini P, Gastaldi M, Alfonsi E, Pichiecchio A, et al. Characterization and Management of Neurological Adverse Events During Immune-Checkpoint Inhibitors Treatment: An Italian Multicentric Experience. Neurol Sci (2021). doi: 10.1007/s10072-021-05561-z
7. Rajendram P, Torbic H, Duggal A, Campbell J, Hovden M, Dhawan V, et al. Critically Ill Patients With Severe Immune Checkpoint Inhibitor Related Neurotoxicity: A Multi-Center Case Series. J Crit Care (2021) 65:126–32. doi: 10.1016/j.jcrc.2021.05.020
8. Duong SL, Barbiero FJ, Nowak RJ, Baehring JM. Neurotoxicities Associated With Immune Checkpoint Inhibitor Therapy. J Neurooncol (2021) 152(2):265–77. doi: 10.1007/s11060-021-03695-w
9. Gust J, Taraseviciute A, Turtle CJ. Neurotoxicity Associated With CD19-Targeted CAR-T Cell Therapies. CNS Drugs (2018) 32(12):1091–101. doi: 10.1007/s40263-018-0582-9
10. Gust J, Hay KA, Hanafi LA, Li D, Myerson D, Gonzalez-Cuyar LF, et al. Endothelial Activation and Blood-Brain Barrier Disruption in Neurotoxicity After Adoptive Immunotherapy With CD19 CAR-T Cells. Cancer Discov (2017) 7(12):1404–19. doi: 10.1158/2159-8290.CD-17-0698
11. Brahmer JR, Lacchetti C, Schneider BJ, Atkins MB, Brassil KJ, Caterino JM, et al. Management of Immune-Related Adverse Events in Patients Treated With Immune Checkpoint Inhibitor Therapy: American Society of Clinical Oncology Clinical Practice Guideline. J Clin Oncol (2018) 36(17):1714–68. doi: 10.1200/JCO.2017.77.6385
12. Brahmer JR, Abu-Sbeih H, Ascierto PA, Brufsky J, Cappelli LC, Cortazar FB, et al. Society for Immunotherapy of Cancer (SITC) Clinical Practice Guideline on Immune Checkpoint Inhibitor-Related Adverse Events. J Immunother Cancer (2021) 9(6):e002435. doi: 10.1136/jitc-2021-002435
13. Maus MV, Alexander S, Bishop MR, Brudno JN, Callahan C, Davila ML, et al. Society for Immunotherapy of Cancer (SITC) Clinical Practice Guideline on Immune Effector Cell-Related Adverse Events. J Immunother Cancer (2020) 8(2):e001511. doi: 10.1136/jitc-2020-001511
14. Santomasso BD, Nastoupil LJ, Adkins S, Lacchetti S, Schneider S, Anadkat S, et al. Management of Immune-Related Adverse Events in Patients Treated With Chimeric Antigen Receptor T-Cell Therapy: ASCO Guideline. J Clin Oncol (2021) 2021:JCO2101992. doi: 10.1200/JCO.21.01992
15. Lee DW, Santomasso BD, Locke FL, Ghobadi A, Turtle CJ, Brudno JN, et al. ASTCT Consensus Grading for Cytokine Release Syndrome and Neurologic Toxicity Associated With Immune Effector Cells. Biol Blood Marrow Transplant (2019) 25(4):625–38. doi: 10.1016/j.bbmt.2018.12.758
16. Traube C, Silver G, Kearney J, Patel A, Atkinson TM, Yoon MJ, et al. Cornell Assessment of Pediatric Delirium: A Valid, Rapid, Observational Tool for Screening Delirium in the PICU. Crit Care Med (2014) 42(3):656–63. doi: 10.1097/CCM.0b013e3182a66b76
17. Shalabi H, Wolters PL, Martin S, Toledo-Tamula MA, Roderick MC, Streumph K, et al. Systematic Evaluation of Neurotoxicity in Children and Young Adults Undergoing CD22 Chimeric Antigen Receptor T-Cell Therapy. J Immunother (2018) 41(7):350–8. doi: 10.1097/CJI.0000000000000241
18. Ripperger T, Bielack SS, Borkhardt A, Brecht IB, Burkhardt B, Calaminus G, et al. Childhood Cancer Predisposition Syndromes-A Concise Review and Recommendations by the Cancer Predisposition Working Group of the Society for Pediatric Oncology and Hematology. Am J Med Genet A (2017) 173(4):1017–37. doi: 10.1002/ajmg.a.38142
19. Neuro-Oncology Training for the Child Neurology Resident - Fatema Malbari, Sonia Partap, Juliane Gust, Elizabeth Duke, Aimee Sato, Yasmin Khakoo, Nicole J. Ullrich, 2021.
20. Noone AM, Cronin KA, Altekruse SF, Howlader N, Lewis DR, Petkov VI, et al. Cancer Incidence and Survival Trends by Subtype Using Data From the Surveillance Epidemiology and End Results Program, 1992-2013. Cancer Epidemiol Biomarkers Prev (2016) 26(4):632–41. doi: 10.1158/1055-9965.EPI-16-0520
21. Raetz EA. Teachey DT. T-Cell Acute Lymphoblastic Leukemia. Hematol Am Soc Hematol Educ Program (2016) 2016(1):580–8. doi: 10.1182/asheducation-2016.1.580
22. Moricke A, Zimmermann M, Valsecchi MG, Stanulla M, Biondi A, Mann G, et al. Dexamethasone vs Prednisone in Induction Treatment of Pediatric ALL: Results of the Randomized Trial AIEOP-BFM ALL 2000. Blood (2016) 127(17):2101–12. doi: 10.1182/blood-2015-09-670729
23. Larsen EC, Devidas M, Chen S, Salzer WL, Raetz EA, Loh ML, et al. Dexamethasone and High-Dose Methotrexate Improve Outcome for Children and Young Adults With High-Risk B-Acute Lymphoblastic Leukemia: A Report From Children’s Oncology Group Study Aall0232. J Clin Oncol (2016) 34(20):2380–8. doi: 10.1200/JCO.2015.62.4544
24. Maloney KW, Devidas M, Wang C, Mattano LA, Friedmann AM, Buckley P, et al. Outcome in Children With Standard-Risk B-Cell Acute Lymphoblastic Leukemia: Results of Children’s Oncology Group Trial Aall0331. J Clin Oncol (2020) 38(6):602–12. doi: 10.1200/JCO.19.01086
25. Winter SS, Dunsmore KP, Devidas M, Wood BL, Esiashvili N, Chen Z, et al. Improved Survival for Children and Young Adults With T-Lineage Acute Lymphoblastic Leukemia: Results From the Children’s Oncology Group AALL0434 Methotrexate Randomization. J Clin Oncol (2018) 36(29):2926–34. doi: 10.1200/JCO.2018.77.7250
26. Pieters R, de Groot-Kruseman H, van der Velden V, Fiocco M, van den Berg H, de Bont E, et al. Successful Therapy Reduction and Intensification for Childhood Acute Lymphoblastic Leukemia Based on Minimal Residual Disease Monitoring: Study ALL10 From the Dutch Childhood Oncology Group. J Clin Oncol (2016) 34(22):2591–601. doi: 10.1200/JCO.2015.64.6364
27. Vora A, Goulden N, Mitchell C, Hancock J, Hough R, Rowntree C, et al. Augmented Post-Remission Therapy for a Minimal Residual Disease-Defined High-Risk Subgroup of Children and Young People With Clinical Standard-Risk and Intermediate-Risk Acute Lymphoblastic Leukaemia (UKALL 2003): A Randomised Controlled Trial. Lancet Oncol (2014) 15(8):809–18. doi: 10.1016/S1470-2045(14)70243-8
28. Toft N, Birgens H, Abrahamsson J, Griskevicius L, Hallbook H, Heyman M, et al. Results of NOPHO ALL2008 Treatment for Patients Aged 1-45 Years With Acute Lymphoblastic Leukemia. Leukemia (2018) 32(3):606–15. doi: 10.1038/leu.2017.265
29. Jeha S, Pei D, Choi J, Cheng C, Sandlund JT, Coustan-Smith E, et al. Improved CNS Control of Childhood Acute Lymphoblastic Leukemia Without Cranial Irradiation: St Jude Total Therapy Study 16. J Clin Oncol (2019) 37(35):3377–91. doi: 10.1200/JCO.19.01692
30. Woods WG. Curing Childhood Acute Myeloid Leukemia (AML) at the Half-Way Point: Promises to Keep and Miles to Go Before We Sleep. Pediatr Blood Cancer (2006) 46(5):565–9. doi: 10.1002/pbc.20646
31. Pui CH, Pei D, Campana D, Bowman WP, Sandlund JT, Kaste SC, et al. Improved Prognosis for Older Adolescents With Acute Lymphoblastic Leukemia. J Clin Oncol (2011) 29(4):386–91. doi: 10.1200/JCO.2010.32.0325
32. Elgarten CW, Aplenc R. Pediatric Acute Myeloid Leukemia: Updates on Biology, Risk Stratification, and Therapy. Curr Opin Pediatr (2020) 32(1):57–66. doi: 10.1097/MOP.0000000000000855
33. Allen CE, Kelly KM, Bollard CM. Pediatric Lymphomas and Histiocytic Disorders of Childhood. Pediatr Clin North Am (2015) 62(1):139–65. doi: 10.1016/j.pcl.2014.09.010
34. Moore AS, Kearns PR, Knapper S, Pearson AD, Zwaan CM. Novel Therapies for Children With Acute Myeloid Leukaemia. Leukemia (2013) 27(7):1451–60. doi: 10.1038/leu.2013.106
35. Cheung YT, Krull KR. Neurocognitive Outcomes in Long-Term Survivors of Childhood Acute Lymphoblastic Leukemia Treated on Contemporary Treatment Protocols: A Systematic Review. Neurosci Biobehav Rev (2015) 53:108–20. doi: 10.1016/j.neubiorev.2015.03.016
36. Cheung YT, Sabin ND, Reddick WE, Bhojwani D, Liu W, Brinkman TM, et al. Leukoencephalopathy and Long-Term Neurobehavioral, Neurocognitive and Brain Imaging Outcomes in Survivors of Childhood Acute Lymphoblastic Leukemia Treated With Chemotherapy: A Longitudinal Analysis. Lancet Haematol (2016) 3(10):e456–66. doi: 10.1016/S2352-3026(16)30110-7
37. Nicholls L, Kopp LM. Immunotherapy for Pediatric Solid Tumors. In: Rezaei N, editor. Cancer Immunology: Cancer Immunotherapy for Organ-Specific Tumors. Cham: Springer International Publishing (2020). p. 49–73. doi: 10.1007/978-3-030-57949-4_2
38. Kopp LM, Katsanis E. Targeted Immunotherapy for Pediatric Solid Tumors. OncoImmunology (2016) 5(3):e1087637. doi: 10.1080/2162402X.2015.1087637
39. Robison LL, Hudson MM. Survivors of Childhood and Adolescent Cancer: Life-Long Risks and Responsibilities. Nat Rev Cancer (2014) 14(1):61–70. doi: 10.1038/nrc3634
40. Norsker FN, Rechnitzer C, Andersen EW, Linnet KM, Kenborg L, Holmqvist AS, et al. Neurologic Disorders in Long-Term Survivors of Neuroblastoma - A Population-Based Cohort Study Within the Adult Life After Childhood Cancer in Scandinavia (ALiCCS) Research Program. Acta Oncol (2020) 59(2):134–40. doi: 10.1080/0284186X.2019.1672892
41. Antunes NL. The Spectrum of Neurologic Disease in Children With Systemic Cancer. Pediatr Neurol (2001) 25(3):227–35. doi: 10.1016/S0887-8994(01)00313-7
42. Yu AL, Gilman AL, Özkaynak MF, London WB, Kreissman SG, Chen HX, et al. Anti-GD2 Antibody With GM-CSF, Interleukin-2, and Isotretinoin for Neuroblastoma. N Engl J Med (2010) 363(14):1324–34. doi: 10.1056/NEJMoa0911123
43. Bettio M, Negrao De Carvalho R, Dimitrova N, Dyba T, Giusti F, Martos Jimenez M, et al. Dataset Collection: European Cancer Information System. (2018).
44. Ostrom QT, de Blank PM, Kruchko C, Petersen CM, Liao P, Finlay JL, et al. Alex’s Lemonade Stand Foundation Infant and Childhood Primary Brain and Central Nervous System Tumors Diagnosed in the United States in 2007-2011. Neuro-Oncology (2015) 16:x1–x36. doi: 10.1093/neuonc/nou327
45. Ostrom QT, Gittleman H, Xu J, Kromer C, Wolinsy Y, Kruchko C, et al. CBTRUS Statistical Report: Primary Brain and Other Central Nervous System Tumors Diagnosed in the United States in 2009–2013. Neuro-Oncology (2016) 18(suppl_5):v1–v75. doi: 10.1093/neuonc/now207
46. Palmer JD, Tsang DS, Tinkle CL, Olch AJ, Kremer LC, Ronckers CM, et al. Late Effects of Radiation Therapy in Pediatric Patients and Survivorship. Pediatr Blood Cancer (2021) 68(S2):e28349. doi: 10.1002/pbc.28349
47. Kline CN, Mueller S. Neurocognitive Outcomes in Children With Brain Tumors. Semin Neurol (2020) 40(3):315–21. doi: 10.1055/s-0040-1708867
48. Roddy E, Mueller S. Late Effects of Treatment of Pediatric Central Nervous System Tumors. J Child Neurol (2016) 31(2):237–54. doi: 10.1177/0883073815587944
49. Farber SH, Elsamadicy AA, Atik F, Suryadevara CM, Chongsathidkiet P, Fecci PE, et al. The Safety of Available Immunotherapy for the Treatment of Glioblastoma. Expert Opin Drug Saf (2017) 16(3):277–87. doi: 10.1080/14740338.2017.1273898
50. Finch EA, Duke E, Hwang EI, Packer RJ. Immunotherapy Approaches for Pediatric CNS Tumors and Associated Neurotoxicity. Pediatr Neurol (2020) 107:7–15. doi: 10.1016/j.pediatrneurol.2020.01.004
51. Sampson JH, Maus MV, June CH. Immunotherapy for Brain Tumors. J Clin Oncol (2017) 35(21):2450–6. doi: 10.1200/JCO.2017.72.8089
52. Thust SC, van den Bent MJ, Smits M. Pseudoprogression of Brain Tumors. J Magn Reson Imaging (2018) 48(3):571–89. doi: 10.1002/jmri.26171
53. Okada H, Weller M, Huang R, Finocchiaro G, Gilbert MR, Wick W, et al. Immunotherapy Response Assessment in Neuro-Oncology: A Report of the RANO Working Group. Lancet Oncol (2015) 16(15):e534–42. doi: 10.1016/S1470-2045(15)00088-1
54. Chukwueke UN, Wen PY. Use of the Response Assessment in Neuro-Oncology (RANO) Criteria in Clinical Trials and Clinical Practice. CNS Oncol (2019) 8(1):CNS28. doi: 10.2217/cns-2018-0007
55. Chen X, Lim-Fat MJ, Qin L, Li A, Bryant A, Bay CP, et al. A Comparative Retrospective Study of Immunotherapy RANO Versus Standard RANO Criteria in Glioblastoma Patients Receiving Immune Checkpoint Inhibitor Therapy. Front Oncol (2021) 11:2444. doi: 10.3389/fonc.2021.679331
56. Suki D, Khoury Abdulla R, Ding M, Khatua S, Sawaya R. Brain Metastases in Patients Diagnosed With a Solid Primary Cancer During Childhood: Experience From a Single Referral Cancer Center. J Neurosurg Pediatr (2014) 14(4):372–85. doi: 10.3171/2014.7.PEDS13318
57. Paulino AC, Nguyen TX, Barker JL Jr. Brain Metastasis in Children With Sarcoma, Neuroblastoma, and Wilms’ Tumor. Int J Radiat Oncol Biol Phys (2003) 57(1):177–83. doi: 10.1016/S0360-3016(03)00502-9
58. Bekiesinska-Figatowska M, Duczkowska A, Duczkowski M, Bragoszewska H, Romaniuk-Doroszewska A, Iwanowska B, et al. CNS Metastases From Bone and Soft Tissue Sarcomas in Children, Adolescents, and Young Adults: Are They Really So Rare? BioMed Res Int (2017) 2017:1456473. doi: 10.1155/2017/1456473
59. Aguilar C, Toro V, Medina R. 66. Clinical Characteristics and Results of Pediatric Solid Tumors With Brain Metastases: Experience From a Single Referral Cancer Center. Neuro-Oncol Adv (2020) 2(Supplement_2):ii14. doi: 10.1093/noajnl/vdaa073.053
60. Suki D, Abdulla RK, Ding M, Khatua S, Sawaya R. Brain Metastases in Patients Diagnosed With a Solid Primary Cancer During Childhood: Experience From a Single Referral Cancer Center: Clinical Article. J Neurosurg: Pediatr (2014) 14(4):372–85. doi: 10.3171/2014.7.PEDS13318
61. Laningham FH, Kun LE, Reddick WE, Ogg RJ, Morris EB, Pui CH. Childhood Central Nervous System Leukemia: Historical Perspectives, Current Therapy, and Acute Neurological Sequelae. Neuroradiology (2007) 49(11):873–88. doi: 10.1007/s00234-007-0300-7
62. Grupp SA, Kalos M, Barrett D, Aplenc R, Porter DL, Rheingold SR, et al. Chimeric Antigen Receptor-Modified T Cells for Acute Lymphoid Leukemia. N Engl J Med (2013) 368(16):1509–18. doi: 10.1056/NEJMoa1215134
63. Maus MV, Grupp SA, Porter DL, June CH. Antibody-Modified T Cells: CARs Take the Front Seat for Hematologic Malignancies. Blood (2014) 123(17):2625–35. doi: 10.1182/blood-2013-11-492231
64. Fry TJ. Mackall CL. T-Cell Adoptive Immunotherapy for Acute Lymphoblastic Leukemia. Hematol Am Soc Hematol Educ Program (2013) 2013:348–53. doi: 10.1182/asheducation-2013.1.348
65. Mackall CL. Enhancing the Efficacy of CAR T Cells. Blood (2017) 130 (Suppl_1):SCI-15-SCI-15. doi: 10.1182/blood.V130.Suppl_1.SCI-15.SCI-15
66. Mackall CL, Merchant MS, Fry TJ. Immune-Based Therapies for Childhood Cancer. Nat Rev Clin Oncol (2014) 11(12):693–703. doi: 10.1038/nrclinonc.2014.177
67. Maude SL, Frey N, Shaw PA, Aplenc R, Barrett DM, Bunin NJ, et al. Chimeric Antigen Receptor T Cells for Sustained Remissions in Leukemia. N Engl J Med (2014) 371(16):1507–17. doi: 10.1056/NEJMoa1407222
68. Maude SL, Laetsch TW, Buechner J, Rives S, Boyer M, Bittencourt H, et al. Tisagenlecleucel in Children and Young Adults With B-Cell Lymphoblastic Leukemia. N Engl J Med (2018) 378(5):439–48. doi: 10.1056/NEJMoa1709866
69. Lee DW, Kochenderfer JN, Stetler-Stevenson M, Cui YK, Delbrook C, Feldman SA, et al. T Cells Expressing CD19 Chimeric Antigen Receptors for Acute Lymphoblastic Leukaemia in Children and Young Adults: A Phase 1 Dose-Escalation Trial. Lancet (2015) 385(9967):517–28. doi: 10.1016/S0140-6736(14)61403-3
70. Gardner RA, Finney O, Annesley C, Brakke H, Summers C, Leger K, et al. Intent-To-Treat Leukemia Remission by CD19 CAR T Cells of Defined Formulation and Dose in Children and Young Adults. Blood (2017) 129(25):3322–31. doi: 10.1182/blood-2017-02-769208
71. Fry TJ, Shah NN, Orentas RJ, Stetler-Stevenson M, Yuan CM, Ramakrishna S, et al. CD22-Targeted CAR T Cells Induce Remission in B-ALL That Is Naive or Resistant to CD19-Targeted CAR Immunotherapy. Nat Med (2018) 24(1):20–8. doi: 10.1038/nm.4441
72. Shah NN, Highfill SL, Shalabi H, Yates B, Jin J, Wolters PL, et al. CD4/CD8 T-Cell Selection Affects Chimeric Antigen Receptor (CAR) T-Cell Potency and Toxicity: Updated Results From a Phase I Anti-CD22 CAR T-Cell Trial. J Clin Oncol (2020) 38(17):1938–50. doi: 10.1200/JCO.19.03279
73. Kymriah (tisagenlecleucel) [package insert]. U.S Food and Drug Administration. Approval 2017; Revised 2020.
74. Shalabi H, Gust J, Taraseviciute A, Wolters PL, Leahy AB, Sandi C, et al. Beyond the Storm — Subacute Toxicities and Late Effects in Children Receiving CAR T Cells. Nat Rev Clin Oncol (2021) 2021:1–16. doi: 10.1038/s41571-020-00456-y
75. Gust J, Ceppi F, Turtle CJ. Chapter 7 - Neurotoxicities After CAR T-Cell Immunotherapy. In: Lee DW, Shah NN, editors. Chimeric Antigen Receptor T-Cell Therapies for Cancer. Amsterdam, Netherlands: Elsevier (2020). p. 83–105. doi: 10.1016/B978-0-323-66181-2.00007-X
76. Gofshteyn JS, Shaw PA, Teachey DT, Grupp SA, Maude S, Banwell B, et al. Neurotoxicity After CTL019 in a Pediatric and Young Adult Cohort. Ann Neurol (2018) 84(4):537–46. doi: 10.1002/ana.25315
77. Gust J, Finney OC, Li D, Brakke HM, Hicks RM, Futrell RB, et al. Glial Injury in Neurotoxicity After Pediatric CD19-Directed Chimeric Antigen Receptor T Cell Therapy. Ann Neurol (2019) 86(1):42–54. doi: 10.1002/ana.25502
78. Pan J, Tan Y, Wang G, Deng B, Ling Z, Song W, et al. Donor-Derived CD7 Chimeric Antigen Receptor T Cells for T-Cell Acute Lymphoblastic Leukemia: First-In-Human, Phase I Trial. J Clin Oncol (2021) 39(30):3340–51. doi: 10.1200/JCO.21.00389
79. Torre M, Solomon IH, Sutherland CL, Nikiforow S, DeAngelo DJ, Stone RM, et al. Neuropathology of a Case With Fatal CAR T-Cell-Associated Cerebral Edema. J Neuropathol Exp Neurol (2018) 77(10):877–82. doi: 10.1093/jnen/nly064
80. Gu R, Liu F, Zou D, Xu Y, Lu Y, Liu B, et al. Efficacy and Safety of CD19 CAR T Constructed With a New Anti-CD19 Chimeric Antigen Receptor in Relapsed or Refractory Acute Lymphoblastic Leukemia. J Hematol Oncol (2020) 13(1):122. doi: 10.1186/s13045-020-00953-8
81. Laetsch TW, Myers GD, Baruchel A, Dietz AC, Pulsipher MA, Bittencourt H, et al. Patient-Reported Quality of Life After Tisagenlecleucel Infusion in Children and Young Adults With Relapsed or Refractory B-Cell Acute Lymphoblastic Leukaemia: A Global, Single-Arm, Phase 2 Trial. Lancet Oncol (2019) 20(12):1710–8. doi: 10.1016/S1470-2045(19)30493-0
82. Gust J, Annesley CE, Gardner RA, Bozarth X. EEG Correlates of Delirium in Children and Young Adults With CD19-Directed CAR T Cell Treatment-Related Neurotoxicity. J Clin Neurophysiol (2021) 38(2):135–42. doi: 10.1097/WNP.0000000000000669
83. Gust J, Ponce R, Liles WC, Garden GA, Turtle CJ. Cytokines in CAR T Cell-Associated Neurotoxicity. Front Immunol (2020) 11:577027. doi: 10.3389/fimmu.2020.577027
84. Norelli M, Camisa B, Barbiera G, Falcone L, Purevdorj A, Genua M, et al. Monocyte-Derived IL-1 and IL-6 are Differentially Required for Cytokine-Release Syndrome and Neurotoxicity Due to CAR T Cells. Nat Med (2018) 24(6):739–48. doi: 10.1038/s41591-018-0036-4
85. Sterner RM, Sakemura R, Cox MJ, Yang N, Khadka RH, Forsman CL, et al. GM-CSF Inhibition Reduces Cytokine Release Syndrome and Neuroinflammation But Enhances CAR-T Cell Function in Xenografts. Blood (2019) 133(7):697–709. doi: 10.1182/blood-2018-10-881722
86. Gust J, Annesley CE, Gardner RA, Bozarth X. EEG Correlates of Delirium in Children and Young Adults With CD19-Directed CAR T Cell Treatment-Related Neurotoxicity. J Clin Neurophysiol (2019) 38(2):135–42. Publish Ahead of Print. doi: 10.1097/WNP.0000000000000669
87. Faulhaber LD, Phuong AQ, Hartsuyker KJ, Cho Y, Mand KK, Harper SD, et al. Brain Capillary Obstruction During Neurotoxicity in a Mouse Model of Anti-CD19 Chimeric Antigen Receptor T-cell Therapy. Brain Communications (2022) 4(1):fcab309. doi: 10.1093/braincomms/fcab309
88. Ahn SJ, Anrather J, Nishimura N, Schaffer C. Diverse Inflammatory Response After Cerebral Microbleeds Includes Coordinated Microglial Migration and Proliferation. Stroke (2018) 49:STROKEAHA.117.020461. doi: 10.1161/STROKEAHA.117.020461
89. Lee DW, Gardner R, Porter DL, Louis CU, Ahmed N, Jensen M, et al. Current Concepts in the Diagnosis and Management of Cytokine Release Syndrome. Blood (2014) 124(2):188–95. doi: 10.1182/blood-2014-05-552729
90. Parker KR, Migliorini D, Perkey E, Yost KE, Bhaduri A, Bagga P, et al. Single-Cell Analyses Identify Brain Mural Cells Expressing CD19 as Potential Off-Tumor Targets for CAR-T Immunotherapies. Cell (2020) 183(1):126–142 e117. doi: 10.1016/j.cell.2020.08.022
91. Leahy AB, Newman H, Li Y, Liu H, Myers R, DiNofia A, et al. CD19-Targeted Chimeric Antigen Receptor T-Cell Therapy for CNS Relapsed or Refractory Acute Lymphocytic Leukaemia: A Post-Hoc Analysis of Pooled Data From Five Clinical Trials. Lancet Haematol (2021) 8(10):e711–22. doi: 10.1016/S2352-3026(21)00238-6
92. Santomasso B, Park JH, Riviere I, Mead E, Halton E, Diamonte C, et al. Biomarkers Associated With Neurotoxicity in Adult Patients With Relapsed or Refractory B-ALL (R/R B-ALL) Treated With CD19 CAR T Cells. J Clin Oncol (2017) 35(15_suppl):3019–9. doi: 10.1200/JCO.2017.35.15_suppl.3019
93. Locke FL, Ghobadi A, Jacobson CA, Miklos DB, Lekakis LJ, Oluwole OO, et al. Long-Term Safety and Activity of Axicabtagene Ciloleucel in Refractory Large B-Cell Lymphoma (ZUMA-1): A Single-Arm, Multicentre, Phase 1–2 Trial. Lancet Oncol (2019) 20(1):31–42. doi: 10.1016/S1470-2045(18)30864-7
94. Hay KA. Cytokine Release Syndrome and Neurotoxicity After CD19 Chimeric Antigen Receptor-Modified (CAR-) T Cell Therapy. Br J Haematol (2018) 183(3):364–74. doi: 10.1111/bjh.15644
95. Shah NN, Johnson BD, Fenske TS, Raj RV, Hari P. Intrathecal Chemotherapy for Management of Steroid-Refractory CAR T-Cell–Associated Neurotoxicity Syndrome. Blood Adv (2020) 4(10):2119–22. doi: 10.1182/bloodadvances.2020001626
96. 32nd Annual Meeting and Pre-Conference Programs of the Society for Immunotherapy of Cancer (SITC 2017): Part One. J ImmunoTher Cancer (2017) 5(2):86. doi: 10.1186/s40425-017-0289-3
97. Park JR, Digiusto DL, Slovak M, Wright C, Naranjo A, Wagner J, et al. Adoptive Transfer of Chimeric Antigen Receptor Re-Directed Cytolytic T Lymphocyte Clones in Patients With Neuroblastoma. Mol Ther (2007) 15(4):825–33. doi: 10.1038/sj.mt.6300104
98. Heczey A, Courtney AN, Montalbano A, Robinson S, Liu K, Li M, et al. Anti-GD2 CAR-NKT Cells in Patients With Relapsed or Refractory Neuroblastoma: An Interim Analysis. Nat Med (2020) 26(11):1686–90. doi: 10.1038/s41591-020-1074-2
99. Louis CU, Savoldo B, Dotti G, Pule M, Yvon E, Myers GD, et al. Antitumor Activity and Long-Term Fate of Chimeric Antigen Receptor-Positive T Cells in Patients With Neuroblastoma. Blood (2011) 118(23):6050–6. doi: 10.1182/blood-2011-05-354449
100. Yu L, Huang L, Lin D, Lai X, Wu L, Liao X, et al. GD2-Specific Chimeric Antigen Receptor-Modified T Cells for the Treatment of Refractory and/or Recurrent Neuroblastoma in Pediatric Patients. J Cancer Res Clin Oncol (2021). doi: 10.1007/s00432-021-03839-5189
101. Straathof K, Flutter B, Wallace R, Jain N, Loka T, Depani S, et al. Antitumor Activity Without On-Target Off-Tumor Toxicity of GD2-Chimeric Antigen Receptor T Cells in Patients With Neuroblastoma. Sci Transl Med (2020) 12(571):eabd6169. doi: 10.1126/scitranslmed.abd6169
102. Ahmed N, Brawley VS, Hegde M, Robertson C, Ghazi A, Gerken C, et al. Human Epidermal Growth Factor Receptor 2 (HER2) -Specific Chimeric Antigen Receptor-Modified T Cells for the Immunotherapy of HER2-Positive Sarcoma. J Clin Oncol (2015) 33(15):1688–96. doi: 10.1200/JCO.2014.58.0225
103. D’Angelo SP, Melchiori L, Merchant MS, Bernstein D, Glod J, Kaplan R, et al. Antitumor Activity Associated With Prolonged Persistence of Adoptively Transferred NY-ESO-1 (C259)T Cells in Synovial Sarcoma. Cancer Discov (2018) 8(8):944–57. doi: 10.1158/2159-8290.CD-17-1417
104. Joseph J, Nathenson MJ, Trinh VA, Malik K, Nowell E, Carter K, et al. Guillain-Barre Syndrome Observed With Adoptive Transfer of Lymphocytes Genetically Engineered With an NY-ESO-1 Reactive T-Cell Receptor. J Immunother Cancer (2019) 7(1):296. doi: 10.1186/s40425-019-0759-x
105. Mount CW, Majzner RG, Sundaresh S, Arnold EP, Kadapakkam M, Haile S, et al. Potent Antitumor Efficacy of Anti-GD2 CAR T-Cells in H3K27M+ Diffuse Midline Gliomas. Nat Med (2018) 24(5):572–9. doi: 10.1038/s41591-018-0006-x
106. Ahmed N, Brawley V, Hegde M, Bielamowicz K, Kalra M, Landi D, et al. HER2-Specific Chimeric Antigen Receptor-Modified Virus-Specific T Cells for Progressive Glioblastoma: A Phase 1 Dose-Escalation Trial. JAMA Oncol (2017) 3(8):1094–101. doi: 10.1001/jamaoncol.2017.0184
107. Vitanza NA, Johnson AJ, Wilson AL, Brown C, Yokoyama JK, Künkele A, et al. Locoregional Infusion of HER2-Specific CAR T Cells in Children and Young Adults With Recurrent or Refractory CNS Tumors: An Interim Analysis. Nat Med (2021) 2021:1–9. doi: 10.1038/s41591-021-01404-8
108. Majzner RG, Ramakrishna S, Yeom KW, Patel S, Chinnasamy H, Schultz LM, et al. GD2-CAR T Cell Therapy for H3K27M-Mutated Diffuse Midline Gliomas. Nature (2022). doi: 10.1038/s41586-022-04489-4
109. Wei SC, Duffy CR, Allison JP. Fundamental Mechanisms of Immune Checkpoint Blockade Therapy. Cancer Discov (2018) 8(9):1069–86. doi: 10.1158/2159-8290.CD-18-0367
110. Perrinjaquet C, Desbaillets N, Hottinger AF. Neurotoxicity Associated With Cancer Immunotherapy: Immune Checkpoint Inhibitors and Chimeric Antigen Receptor T-Cell Therapy. Curr Opin Neurol (2019) 32(3):500–10. doi: 10.1097/WCO.0000000000000686
111. Vaddepally RK, Kharel P, Pandey R, Garje R, Chandra AB. Review of Indications of FDA-Approved Immune Checkpoint Inhibitors Per NCCN Guidelines With the Level of Evidence. Cancers (Basel) (2020) 12(3):738. doi: 10.3390/cancers12030738
112. Kennedy LB. Salama AKS. A Review of Cancer Immunotherapy Toxicity. CA Cancer J Clin (2020) 70(2):86–104. doi: 10.3322/caac.21596
113. Johnson DB, Manouchehri A, Haugh AM, et al. Neurologic Toxicity Associated With Immune Checkpoint Inhibitors: A Pharmacovigilance Study. J ImmunoTher Cancer (2019) 7(1):134. doi: 10.1186/s40425-019-0617-x
114. Sato K, Mano T, Iwata A, Toda T. Neurological and Related Adverse Events in Immune Checkpoint Inhibitors: A Pharmacovigilance Study From the Japanese Adverse Drug Event Report Database. J Neurooncol (2019) 145(1):1–9. doi: 10.1007/s11060-019-03273-1
115. Cao Y, Nylander A, Ramanan S, Goods BA, Ponath G, Zabad R, et al. CNS Demyelination and Enhanced Myelin-Reactive Responses After Ipilimumab Treatment. Neurology (2016) 86(16):1553–6. doi: 10.1212/WNL.0000000000002594
116. Gerdes LA, Held K, Beltrán E, Berking C, Prinz JC, Junker A, et al. CTLA4 as Immunological Checkpoint in the Development of Multiple Sclerosis. Ann Neurol (2016) 80(2):294–300. doi: 10.1002/ana.24715
117. Davis KL, Agarwal AM, Verma AR. Checkpoint Inhibition in Pediatric Hematologic Malignancies. Pediatr Hematol Oncol (2017) 34(6-7):379–94. doi: 10.1080/08880018.2017.1383542
118. Davis KL, Fox E, Merchant MS, Reid JM, Kudgus RA, Liu X, et al. Nivolumab in Children and Young Adults With Relapsed or Refractory Solid Tumours or Lymphoma (ADVL1412): A Multicentre, Open-Label, Single-Arm, Phase 1-2 Trial. Lancet Oncol (2020) 21(4):541–50. doi: 10.1016/S1470-2045(20)30023-1
119. Shad AT, Huo JS, Darcy C, Abu-Ghosh A, Esposito G, Holuba MJ, et al. Tolerance and Effectiveness of Nivolumab After Pediatric T-Cell Replete, Haploidentical, Bone Marrow Transplantation: A Case Report. Pediatr Blood Cancer (2017) 64(3):26257. doi: 10.1002/pbc.26257
120. Geoerger B, Kang HJ, Yalon-Oren M, Marshall HJ, Vezina HJ, Pappo HJ, et al. Pembrolizumab in Paediatric Patients With Advanced Melanoma or a PD-L1-Positive, Advanced, Relapsed, or Refractory Solid Tumour or Lymphoma (KEYNOTE-051): Interim Analysis of an Open-Label, Single-Arm, Phase 1-2 Trial. Lancet Oncol (2020) 21(1):121–33. doi: 10.1016/S1470-2045(19)30671-0
121. Geoerger B, Zwaan CM, Marshall LV, Michon J, Bourdeaut F, Casanova M, et al. Atezolizumab for Children and Young Adults With Previously Treated Solid Tumours, non-Hodgkin Lymphoma, and Hodgkin Lymphoma (iMATRIX): A Multicentre Phase 1-2 Study. Lancet Oncol (2020) 21(1):134–44. doi: 10.1016/S1470-2045(19)30693-X
122. Merchant MS, Wright M, Baird K, Wexler LH, Rodriguez-Galindo C, Bernstein D, et al. Phase I Clinical Trial of Ipilimumab in Pediatric Patients With Advanced Solid Tumors. Clin Cancer Res (2016) 22(6):1364–70. doi: 10.1158/1078-0432.CCR-15-0491
123. Bouffet E, Larouche V, Campbell BB, Merico D, de Borja R, Aronson M, et al. Immune Checkpoint Inhibition for Hypermutant Glioblastoma Multiforme Resulting From Germline Biallelic Mismatch Repair Deficiency. J Clin Oncol (2016) 34(19):2206–11. doi: 10.1200/JCO.2016.66.6552
124. Gorsi HS, Malicki DM, Barsan V, Tumblin M, Yeh-Nayre L, Milburn M, et al. Nivolumab in the Treatment of Recurrent or Refractory Pediatric Brain Tumors: A Single Institutional Experience. J Pediatr Hematol Oncol (2019) 41(4):e235–41. doi: 10.1097/MPH.0000000000001339
125. Zhu X, McDowell MM, Newman WC, Mason GE, Greene S, Tamber MS. Severe Cerebral Edema Following Nivolumab Treatment for Pediatric Glioblastoma: Case Report. J Neurosurg Pediatr (2017) 19(2):249–53. doi: 10.3171/2016.8.PEDS16326
126. Sullivan RJ, Weber JS. Immune-Related Toxicities of Checkpoint Inhibitors: Mechanisms and Mitigation Strategies. Nat Rev Drug Discov (2021) 2021:1–14. doi: 10.1038/s41573-021-00259-5
127. Subudhi SK, Aparicio A, Gao J, Zurita AJ, Araujo JC, Logothetis CJ, et al. Clonal Expansion of CD8 T Cells in the Systemic Circulation Precedes Development of Ipilimumab-Induced Toxicities. Proc Natl Acad Sci USA (2016) 113(42):11919–24. doi: 10.1073/pnas.1611421113
128. Sechi E, Markovic SN, McKeon A, Dubey D, Liewluck T, Lennon VA, et al. Neurologic Autoimmunity and Immune Checkpoint Inhibitors: Autoantibody Profiles and Outcomes. Neurology (2020) 95(17):e2442–52. doi: 10.1212/WNL.0000000000010632
129. Pruss H. Autoantibodies in Neurological Disease. Nat Rev Immunol (2021) 21(12):798–813. doi: 10.1038/s41577-021-00543-w
130. Safa H, Johnson DH, Trinh VA, Rodgers TE, Lin H, Suarez-Almazor ME, et al. Immune Checkpoint Inhibitor Related Myasthenia Gravis: Single Center Experience and Systematic Review of the Literature. J ImmunoTher Cancer (2019) 7(1):319. doi: 10.1186/s40425-019-0774-y
131. Reynolds KL, Guidon AC. Diagnosis and Management of Immune Checkpoint Inhibitor-Associated Neurologic Toxicity: Illustrative Case and Review of the Literature. Oncol (2019) 24(4):435–43. doi: 10.1634/theoncologist.2018-0359
132. Guidon AC, Burton LB, Chwalisz BK, Hillis J, Schaller TH, Amato AA, et al. Consensus Disease Definitions for Neurologic Immune-Related Adverse Events of Immune Checkpoint Inhibitors. J ImmunoTher Cancer (2021) 9(7):e002890. doi: 10.1136/jitc-2021-002890corr1
133. Barroso-Sousa R, Barry WT, Garrido-Castro AC, Hodi FS, Min L, Krop IE, et al. Incidence of Endocrine Dysfunction Following the Use of Different Immune Checkpoint Inhibitor Regimens: A Systematic Review and Meta-Analysis. JAMA Oncol (2018) 4(2):173–82. doi: 10.1001/jamaoncol.2017.3064
134. Puzanov I, Diab A, Abdallah K, Bingham CO 3rd, Brogdon C, Dadu R, et al. Managing Toxicities Associated With Immune Checkpoint Inhibitors: Consensus Recommendations From the Society for Immunotherapy of Cancer (SITC) Toxicity Management Working Group. J ImmunoTher Cancer (2017) 5(1):95. doi: 10.1186/s40425-017-0300-z
135. Weiner LM, Murray JC, Shuptrine CW. Antibody-Based Immunotherapy of Cancer. Cell (2012) 148(6):1081–4. doi: 10.1016/j.cell.2012.02.034
136. Blincyto (Blinatumomab) [Package Insert]. U.S Food and Drug Administration. Approval 2014; Revised 2018.
137. Brown PA, Ji L, Xu X, Devidas M, Hogan LE, Borowitz MJ, et al. Effect of Postreinduction Therapy Consolidation With Blinatumomab vs Chemotherapy on Disease-Free Survival in Children, Adolescents, and Young Adults With First Relapse of B-Cell Acute Lymphoblastic Leukemia: A Randomized Clinical Trial. JAMA (2021) 325(9):833–42. doi: 10.1001/jama.2021.0669
138. Locatelli F, Whitlock JA, Peters C, Chen-Santel C, Chia V, Dennis RM, et al. Blinatumomab Versus Historical Standard Therapy in Pediatric Patients With Relapsed/Refractory Ph-Negative B-Cell Precursor Acute Lymphoblastic Leukemia. Leukemia (2020) 34(9):2473–8. doi: 10.1038/s41375-020-0770-8
139. von Stackelberg A, Locatelli F, Zugmaier G, Handgretinger R, Trippett TM, Rizzari C, et al. Phase I/Phase II Study of Blinatumomab in Pediatric Patients With Relapsed/Refractory Acute Lymphoblastic Leukemia. J Clin Oncol (2016) 34(36):4381–9. doi: 10.1200/JCO.2016.67.3301
140. Locatelli F, Zugmaier G, Mergen N, Bader P, Jeha S, Schlegel PG, et al. Blinatumomab in Pediatric Patients With Relapsed/Refractory Acute Lymphoblastic Leukemia: Results of the RIALTO Trial, an Expanded Access Study. Blood Cancer J (2020) 10(7):77. doi: 10.1038/s41408-020-00342-x
141. Sutton R, Pozza LD, Khaw SL, Fraser C, Revesz T, Chamberlain J, et al. Outcomes for Australian Children With Relapsed/Refractory Acute Lymphoblastic Leukaemia Treated With Blinatumomab. Pediatr Blood Cancer (2021) 68(5):e28922. doi: 10.1002/pbc.28922
142. Contreras CF, Higham CS, Behnert A, Kim K, Stieglitz E, Tasian SK. Clinical Utilization of Blinatumomab and Inotuzumab Immunotherapy in Children With Relapsed or Refractory B-Acute Lymphoblastic Leukemia. Pediatr Blood Cancer (2021) 68(1):e28718. doi: 10.1002/pbc.28718
143. Ampatzidou M, Kattamis A, Baka M, Paterakis G, Anastasiou T, Tzanoudaki M, et al. Insights From the Greek Experience of the Use of Blinatumomab in Pediatric Relapsed and Refractory Acute Lymphoblastic Leukemia Patients. Neoplasma (2020) 67(6):1424–30. doi: 10.4149/neo_2020_200128N93
144. Horibe K, Morris JD, Tuglus CA, Dos Santos C, Kalabus J, Anderson A, et al. A Phase 1b Study of Blinatumomab in Japanese Children With Relapsed/Refractory B-Cell Precursor Acute Lymphoblastic Leukemia. Int J Hematol (2020) 112(2):223–33. doi: 10.1007/s12185-020-02907-9
145. Elitzur S, Arad-Cohen N, Barzilai-Birenboim S, Ben-Harush M, Bielorai B, Elhasid R, et al. Blinatumomab as a Bridge to Further Therapy in Cases of Overwhelming Toxicity in Pediatric B-Cell Precursor Acute Lymphoblastic Leukemia: Report From the Israeli Study Group of Childhood Leukemia. Pediatr Blood Cancer (2019) 66(10):e27898. doi: 10.1002/pbc.27898
146. Queudeville M, Schlegel P, Heinz AT, Lenz T, Döring M, Holzer U, et al. Blinatumomab in Pediatric Patients With Relapsed/Refractory B-Cell Precursor Acute Lymphoblastic Leukemia. Eur J Haematol (2021) 106(4):473–83. doi: 10.1111/ejh.13569
147. Fuster JL, Molinos-Quintana A, Fuentes C, Fernández JM, Velasco P, Pascual T, et al. Blinatumomab and Inotuzumab for B Cell Precursor Acute Lymphoblastic Leukaemia in Children: A Retrospective Study From the Leukemia Working Group of the Spanish Society of Pediatric Hematology and Oncology (SEHOP). Br J Haematol (2020) 190(5):764–71. doi: 10.1111/bjh.16647
148. Filippidou M, Avgerinou G, Katsibardi K, Gavra M, Pons R, Kattamis A. Delayed-Onset Severe Neurotoxicity Related to Blinatumomab in an Adolescent Patient With Refractory Acute Lymphoblastic Leukemia. Pediatr Blood Cancer (2021) 68(7):e29040. doi: 10.1002/pbc.29040
149. Klinger M, Zugmaier G, Nägele V, Goebeler ME, Brandl C, Stelljes M, et al. Adhesion of T Cells to Endothelial Cells Facilitates Blinatumomab-Associated Neurologic Adverse Events. Cancer Res (2020) 80(1):91–101. doi: 10.1158/0008-5472.CAN-19-1131
150. Goebeler ME, Knop S, Viardot A, Kufer P, Topp MS, Einsele H, et al. Bispecific T-Cell Engager (BiTE) Antibody Construct Blinatumomab for the Treatment of Patients With Relapsed/Refractory Non-Hodgkin Lymphoma: Final Results From a Phase I Study. J Clin Oncol (2016) 34(10):1104–11. doi: 10.1200/JCO.2014.59.1586
151. Bhojwani D, Sposto R, Shah NN, Rodriguez V, Yuan C, Stetler-Stevenson M, et al. Inotuzumab Ozogamicin in Pediatric Patients With Relapsed/Refractory Acute Lymphoblastic Leukemia. Leukemia (2019) 33(4):884–92. doi: 10.1038/s41375-018-0265-z
152. Meinhardt A, Burkhardt B, Zimmermann M, Borkhardt A, Kontny U, Klingebiel T, et al. Phase II Window Study on Rituximab in Newly Diagnosed Pediatric Mature B-Cell non-Hodgkin’s Lymphoma and Burkitt Leukemia. J Clin Oncol (2010) 28(19):3115–21. doi: 10.1200/JCO.2009.26.6791
153. Locatelli F, Mauz-Koerholz C, Neville K, Llort A, Beishuizen A, Daw S, et al. Brentuximab Vedotin for Paediatric Relapsed or Refractory Hodgkin’s Lymphoma and Anaplastic Large-Cell Lymphoma: A Multicentre, Open-Label, Phase 1/2 Study. Lancet Haematol (2018) 5(10):e450–61. doi: 10.1016/S2352-3026(18)30153-4
154. Brivio E, Locatelli F, Lopez-Yurda M, Malone A, Díaz-de-Heredia C, Bielorai B, et al. A Phase 1 Study of Inotuzumab Ozogamicin in Pediatric Relapsed/Refractory Acute Lymphoblastic Leukemia (ITCC-059 Study). Blood (2021) 137(12):1582–90. doi: 10.1182/blood.2020007848
155. Minard-Colin V, Auperin A, Pillon M, Burke GAA, Barkauskas DA, Wheatley K, et al. Rituximab for High-Risk, Mature B-Cell Non-Hodgkin’s Lymphoma in Children. N Engl J Med (2020) 382(23):2207–19. doi: 10.1056/NEJMoa1915315
156. Raetz EA, Cairo MS, Borowitz MJ, Lu X, Devidas M, Reid JM, et al. Re-Induction Chemoimmunotherapy With Epratuzumab in Relapsed Acute Lymphoblastic Leukemia (ALL): Phase II Results From Children’s Oncology Group (COG) Study ADVL04P2. Pediatr Blood Cancer (2015) 62(7):1171–5. doi: 10.1002/pbc.25454
157. Ladenstein R, Potschger U, Valteau-Couanet D, Luksch R, Castel V, Yaniv I, et al. Interleukin 2 With Anti-GD2 Antibody Ch14.18/CHO (Dinutuximab Beta) in Patients With High-Risk Neuroblastoma (HR-NBL1/SIOPEN): A Multicentre, Randomised, Phase 3 Trial. Lancet Oncol (2018) 19(12):1617–29. doi: 10.1016/S1470-2045(18)30578-3
158. Talleur AC, Triplett BM, Federico S, Mamcarz E, Janssen W, Wu J, et al. Consolidation Therapy for Newly Diagnosed Pediatric Patients With High-Risk Neuroblastoma Using Busulfan/Melphalan, Autologous Hematopoietic Cell Transplantation, Anti-GD2 Antibody, Granulocyte-Macrophage Colony-Stimulating Factor, Interleukin-2, and Haploidentical Natural Killer Cells. Biol Blood Marrow Transplant (2017) 23(11):1910–7. doi: 10.1016/j.bbmt.2017.07.011
159. Navid F, Sondel PM, Barfield R, Shulkin BL, Kaufman RA, Allay JA, et al. Phase I Trial of a Novel Anti-GD2 Monoclonal Antibody, Hu14.18K322A, Designed to Decrease Toxicity in Children With Refractory or Recurrent Neuroblastoma. J Clin Oncol (2014) 32(14):1445–52. doi: 10.1200/JCO.2013.50.4423
160. Kushner BH, Modak S, Basu EM, Roberts SS, Kramer K, Cheung NK. Posterior Reversible Encephalopathy Syndrome in Neuroblastoma Patients Receiving Anti-GD2 3F8 Monoclonal Antibody. Cancer (2013) 119(15):2789–95. doi: 10.1002/cncr.28137
161. Lampson LA. Monoclonal Antibodies in Neuro-Oncology: Getting Past the Blood-Brain Barrier. MAbs (2011) 3(2):153–60. doi: 10.4161/mabs.3.2.14239
162. Grothey A, Galanis E. Targeting Angiogenesis: Progress With Anti-VEGF Treatment With Large Molecules. Nat Rev Clin Oncol (2009) 6(9):507–18. doi: 10.1038/nrclinonc.2009.110
163. Gururangan S, Fangusaro J, Poussaint TY, McLendon RE, Onar-Thomas A, Wu S, et al. Efficacy of Bevacizumab Plus Irinotecan in Children With Recurrent Low-Grade Gliomas–a Pediatric Brain Tumor Consortium Study. Neuro Oncol (2014) 16(2):310–7. doi: 10.1093/neuonc/not154
164. Su JM-F, Murray JC, McNall-Knapp RY, Bowers DC, Shah S, Adesina AM, et al. A Phase 2 Study of Valproic Acid and Radiation, Followed by Maintenance Valproic Acid and Bevacizumab in Children With Newly Diagnosed Diffuse Intrinsic Pontine Glioma or High-Grade Glioma. Pediatr Blood Cancer (2020) 67(6):e28283. doi: 10.1002/pbc.28283
165. Gururangan S, Chi SN, Young Poussaint T, Onar-Thomas A, Gilbertson RJ, Vajapeyam S, et al. Lack of Efficacy of Bevacizumab Plus Irinotecan in Children With Recurrent Malignant Glioma and Diffuse Brainstem Glioma: A Pediatric Brain Tumor Consortium Study. J Clin Oncol (2010) 28(18):3069–75. doi: 10.1200/JCO.2009.26.8789
166. Levy AS, Krailo M, Chi S, Villaluna D, Springer L, Williams-Hughes C, et al. Temozolomide With Irinotecan Versus Temozolomide, Irinotecan Plus Bevacizumab for Recurrent Medulloblastoma of Childhood: Report of a COG Randomized Phase II Screening Trial. Pediatr Blood Cancer (2021) 68(8):e29031. doi: 10.1002/pbc.29031
167. Chinot OL, Wick W, Cloughesy T. Bevacizumab for Newly Diagnosed Glioblastoma. N Engl J Med (2014) 370(21):2049. doi: 10.1056/NEJMc1403303
168. Gilbert MR, Sulman EP, Mehta MP. Bevacizumab for Newly Diagnosed Glioblastoma. N Engl J Med (2014) 370(21):2048–9. doi: 10.1056/NEJMc1403303
169. Kreisl TN, Kim L, Moore K, Duic P, Royce C, Stroud I, et al. Phase II Trial of Single-Agent Bevacizumab Followed by Bevacizumab Plus Irinotecan at Tumor Progression in Recurrent Glioblastoma. J Clin Oncol (2009) 27(5):740–5. doi: 10.1200/JCO.2008.16.3055
170. Friedman HS, Prados MD, Wen PY, Mikkelsen T, Schiff D, Abrey LE, et al. Bevacizumab Alone and in Combination With Irinotecan in Recurrent Glioblastoma. J Clin Oncol (2009) 27(28):4733–40. doi: 10.1200/JCO.2008.19.8721
171. Ravanpay AC, Gust J, Johnson AJ, Rolczynski LS, Cecchini M, Chang CA, et al. EGFR806-CAR T Cells Selectively Target a Tumor-Restricted EGFR Epitope in Glioblastoma. Oncotarget (2019) 10(66):7080–95. doi: 10.18632/oncotarget.27389
172. Westphal M, Maire CL, Lamszus K. EGFR as a Target for Glioblastoma Treatment: An Unfulfilled Promise. CNS Drugs (2017) 31(9):723. doi: 10.1007/s40263-017-0456-6
173. McCrea HJ, Ivanidze J, O’Connor A, Hersh EH, Boockvar JA, Gobin YP, et al. Intraarterial Delivery of Bevacizumab and Cetuximab Utilizing Blood-Brain Barrier Disruption in Children With High-Grade Glioma and Diffuse Intrinsic Pontine Glioma: Results of a Phase I Trial. J Neurosurg: Pediatr (2021) 28(4):371–9. doi: 10.3171/2021.3.PEDS20738
174. Massimino M, Biassoni V, Miceli R, Schiavello E, Warmuth-Metz M, Modena P, et al. Results of Nimotuzumab and Vinorelbine, Radiation and Re-Irradiation for Diffuse Pontine Glioma in Childhood. J Neurooncol (2014) 118(2):305–12. doi: 10.1007/s11060-014-1428-z
175. van den Bent M, Gan HK, Lassman AB, Kumthekar P, Merrell R, Butowski N, et al. Efficacy of Depatuxizumab Mafodotin (ABT-414) Monotherapy in Patients With EGFR-Amplified, Recurrent Glioblastoma: Results From a Multi-Center, International Study. Cancer Chemother Pharmacol (2017) 80(6):1209–17. doi: 10.1007/s00280-017-3451-1
176. Padovan M, Eoli M, Pellerino A, Rizzato S, Caserta C, Simonelli M, et al. Depatuxizumab Mafodotin (Depatux-M) Plus Temozolomide in Recurrent Glioblastoma Patients: Real-World Experience From a Multicenter Study of Italian Association of Neuro-Oncology (AINO). Cancers (Basel) (2021) 13(11):2773. doi: 10.3390/cancers13112773
177. Saxena M, van der Burg SH, Melief CJM, Bhardwaj N. Therapeutic Cancer Vaccines. Nat Rev Cancer (2021) 21(6):360–78. doi: 10.1038/s41568-021-00346-0
178. Hashii Y, Sato E, Ohta H, Oka Y, Sugiyama H, Ozono K. WT1 Peptide Immunotherapy for Cancer in Children and Young Adults. Pediatr Blood Cancer (2010) 55(2):352–5. doi: 10.1002/pbc.22522
179. Hashii Y, Sato-Miyashita E, Matsumura R, Kusuki S, Yoshida H, Ohta H, et al. WT1 Peptide Vaccination Following Allogeneic Stem Cell Transplantation in Pediatric Leukemic Patients With High Risk for Relapse: Successful Maintenance of Durable Remission. Leukemia (2012) 26(3):530–2. doi: 10.1038/leu.2011.226
180. Akazawa Y, Hosono A, Yoshikawa T, Kaneda H, Nitani C, Hara J, et al. Efficacy of the NCCV Cocktail-1 Vaccine for Refractory Pediatric Solid Tumors: A Phase I Clinical Trial. Cancer Sci (2019) 110(12):3650–62. doi: 10.1111/cas.14206
181. Kopp LM, Malempati S, Krailo M, Gao Y, Buxton A, Weigel BJ, et al. Phase II Trial of the Glycoprotein non-Metastatic B-Targeted Antibody-Drug Conjugate, Glembatumumab Vedotin (CDX-011), in Recurrent Osteosarcoma AOST1521: A Report From the Children’s Oncology Group. Eur J Cancer (2019) 121:177–83. doi: 10.1016/j.ejca.2019.08.015
182. Sawada A, Inoue M, Kondo O, Yamada-Nakata K, Ishihara T, Kuwae Y, et al. Feasibility of Cancer Immunotherapy With WT1 Peptide Vaccination for Solid and Hematological Malignancies in Children. Pediatr Blood Cancer (2016) 63(2):234–41. doi: 10.1002/pbc.25792
183. Kushner BH, Cheung IY, Modak S, Kramer K, Ragupathi G, Cheung NK. Phase I Trial of a Bivalent Gangliosides Vaccine in Combination With β-Glucan for High-Risk Neuroblastoma in Second or Later Remission. Clin Cancer Res (2014) 20(5):1375–82. doi: 10.1158/1078-0432.CCR-13-1012
184. Krishnadas DK, Shusterman S, Bai F, Diller L, Sullivan JE, Cheerva AC, et al. A Phase I Trial Combining Decitabine/Dendritic Cell Vaccine Targeting MAGE-A1, MAGE-A3 and NY-ESO-1 for Children With Relapsed or Therapy-Refractory Neuroblastoma and Sarcoma. Cancer Immunol Immunother (2015) 64(10):1251–60. doi: 10.1007/s00262-015-1731-3
185. Cacciavillano W, Sampor C, Venier C, Gabri MR, de Dávila MT, Galluzzo ML, et al. A Phase I Study of the Anti-Idiotype Vaccine Racotumomab in Neuroblastoma and Other Pediatric Refractory Malignancies. Pediatr Blood Cancer (2015) 62(12):2120–4. doi: 10.1002/pbc.25631
186. Dohnal AM, Witt V, Hugel H, Holter W, Gadner H, Felzmann T. Phase I Study of Tumor Ag-Loaded IL-12 Secreting Semi-Mature DC for the Treatment of Pediatric Cancer. Cytotherapy (2007) 9(8):755–70. doi: 10.1080/14653240701589221
187. Kong Z, Wang Y, Ma W. Vaccination in the Immunotherapy of Glioblastoma. Hum Vaccines Immunother (2017) 14(2):255–68. doi: 10.1080/21645515.2017.1388481
188. Vleeschouwer SD, Fieuws S, Rutkowski S, Van Calenbergh F, Van Loon J, Goffin J, et al. Postoperative Adjuvant Dendritic Cell–Based Immunotherapy in Patients With Relapsed Glioblastoma Multiforme. Clin Cancer Res (2008) 14(10):3098–104. doi: 10.1158/1078-0432.CCR-07-4875
189. Ardon H, De Vleeschouwer S, Van Calenbergh F, Claes L, Kramm CM. Adjuvant Dendritic Cell-Based Tumour Vaccination for Children With Malignant Brain Tumours. Pediatr Blood Cancer (2010) 54(4):519–25. doi: 10.1002/pbc.22319
Keywords: immunotherapy, pediatric cancer, adoptive cell therapy, antibody therapy, neurotoxicity, vaccine therapy
Citation: Shalabi H, Nellan A, Shah NN and Gust J (2022) Immunotherapy Associated Neurotoxicity in Pediatric Oncology. Front. Oncol. 12:836452. doi: 10.3389/fonc.2022.836452
Received: 15 December 2021; Accepted: 20 January 2022;
Published: 21 February 2022.
Edited by:
Nobuko Hijiya, Columbia University Irving Medical Center, United StatesReviewed by:
Andres Morales La Madrid, Hospital Sant Joan de Déu Barcelona, SpainPietro Merli, Bambino Gesù Children’s Hospital (IRCCS), Italy
Copyright © 2022 Shalabi, Nellan, Shah and Gust. This is an open-access article distributed under the terms of the Creative Commons Attribution License (CC BY). The use, distribution or reproduction in other forums is permitted, provided the original author(s) and the copyright owner(s) are credited and that the original publication in this journal is cited, in accordance with accepted academic practice. No use, distribution or reproduction is permitted which does not comply with these terms.
*Correspondence: Haneen Shalabi, aGFuZWVuLnNoYWxhYmlAbmloLmdvdg==; Juliane Gust, anVsaWFuZS5ndXN0QHNlYXR0bGVjaGlsZHJlbnMub3Jn