- 1Pharmaceutical Sciences Research Center, Health Institute, Kermanshah University of Medical Sciences, Kermanshah, Iran
- 2Medical Biology Research Center, Health Technology Institute, Kermanshah University of Medical Sciences, Kermanshah, Iran
- 3Student Research Committee, Kermanshah University of Medical Sciences, Kermanshah, Iran
- 4College of Osteopathic Medicine, Lake Erie College of Osteopathic Medicine, Bradenton, FL, United States
Background: Tumors often progress to a more aggressive phenotype to resist drugs. Multiple dysregulated pathways are behind this tumor behavior which is known as cancer chemoresistance. Thus, there is an emerging need to discover pivotal signaling pathways involved in the resistance to chemotherapeutic agents and cancer immunotherapy. Reports indicate the critical role of the toll-like receptor (TLR)/nuclear factor-κB (NF-κB)/Nod-like receptor pyrin domain-containing (NLRP) pathway in cancer initiation, progression, and development. Therefore, targeting TLR/NF-κB/NLRP signaling is a promising strategy to augment cancer chemotherapy and immunotherapy and to combat chemoresistance. Considering the potential of phytochemicals in the regulation of multiple dysregulated pathways during cancer initiation, promotion, and progression, such compounds could be suitable candidates against cancer chemoresistance.
Objectives: This is the first comprehensive and systematic review regarding the role of phytochemicals in the mitigation of chemoresistance by regulating the TLR/NF-κB/NLRP signaling pathway in chemotherapy and immunotherapy.
Methods: A comprehensive and systematic review was designed based on Web of Science, PubMed, Scopus, and Cochrane electronic databases. The Preferred Reporting Items for Systematic Reviews and Meta-Analyses guidelines were followed to include papers on TLR/NF-κB/NLRP and chemotherapy/immunotherapy/chemoresistance by phytochemicals.
Results: Phytochemicals are promising multi-targeting candidates against the TLR/NF-κB/NLRP signaling pathway and interconnected mediators. Employing phenolic compounds, alkaloids, terpenoids, and sulfur compounds could be a promising strategy for managing cancer chemoresistance through the modulation of the TLR/NF-κB/NLRP signaling pathway. Novel delivery systems of phytochemicals in cancer chemotherapy/immunotherapy are also highlighted.
Conclusion: Targeting TLR/NF-κB/NLRP signaling with bioactive phytocompounds reverses chemoresistance and improves the outcome for chemotherapy and immunotherapy in both preclinical and clinical stages.
Introduction
Chemoresistance occurs when tumors mutate in response to cancer chemotherapy, yielding a more aggressive phenotype that results in chemotherapy failure (1). This has been a major obstacle in cancer chemotherapy and immunotherapy. Despite various attempts to overcome drug resistance and restore the sensitivity of chemotherapeutic drugs, the results thus far have been unsatisfactory (2). Several pathophysiological mechanisms and multiple dysregulated pathways are responsible for chemotherapy and immunotherapy resistance. Thus, revealing the critical dysregulated pathways in cancer chemoresistance would improve clinical outcomes and prevent/manage the development of chemoresistance, therefore limiting the progression and invasion of cancer (3). Amongst the dysregulated mediators, toll-like receptor (TLR) (4), nuclear factor-κB (NF-κB), and Nod-like receptor pyrin domain-containing (NLRP) (5), as well as the associated TLR/NF-κB/NLRP pathway, have been shown to contribute to cancer chemoresistance. In recent years, researchers have been seeking novel alternative agents with multiple targets, higher efficacy, and less side effects that can combat cancer chemoresistance.
Plant secondary metabolites are multi-targeting anticancer agents that target the cancer-associated pathways, including cellular senescence (6), Hippo signaling (7), Wnt/β-catenin (8), Janus kinase (JAK)/signal transducer and activator of transcription (STAT) (9), phosphoinositide 3-kinases (PI3K)/Akt/mammalian target of rapamycin (mTOR) (10), hypoxia-inducible factor-1α (HIF-1α) (11), and activator protein 1 (AP-1) (12). Phenolic compounds, alkaloids, terpenes/terpenoids, and sulfur-containing compounds demonstrated anticancer potential by modulating tumorigenic signaling pathways (6, 13). Emerging evidence has shown the influence of chemoresistance on cancer therapy (5, 14, 15). Although phytochemicals have exhibited critical regulatory roles in the modulation of TLR, NF-κB, and NLRP in combating cancer (16–18), there is no review report on the potential of phytochemicals in targeting TLR/NF-κB/NLRP pathway and pivotally interconnected pathways during chemoresistance. Thus, there is an imperative need to discover the precise dysregulated pathways involved in chemoresistance as well as to develop new strategies and alternative therapies to combat cancer chemoresistance. This is the first systematic and comprehensive review regarding crucial chemoresistance mechanisms and the therapeutic potential of plant secondary metabolites in combating cancer chemoresistance by targeting the TLR/NF-κB pathway and interconnected mediators. The need to develop novel phytocompound delivery systems to fight cancer chemoresistance is also highlighted.
Resistance Mechanisms in Cancer Chemotherapy
Chemoresistance is one of the critical obstacles that affects the efficacy of anticancer drugs. Several factors contribute to the development of cancer chemoresistance. The most common cause of resistance to anticancer agents is the overexpression of energy-dependent transporters that export anticancer agents from the cancer cells (1). Consequently, decreased drug accumulation is another manner of chemotherapeutic resistance, which prevents drug-induced DNA damage and cancer cell apoptosis. Reduced sensitivity to drug-associated apoptosis plays a vital role in the resistance to anticancer drugs (19). Chemotherapy failure can be contributed in part to specific genetic and epigenetic alterations in addition to host factors. Cancer cells have a variety of genetic alterations depending on the tissue and patterns of oncogene activation and tumor suppressor gene inactivation. The use of powerful anticancer agents on cancer cells with these genetic factors leads to the development of drug-resistance mechanisms and the rapid achievement of chemoresistance in various cancer types. Host elements, such as rapid metabolism, poor absorption, and drug excretion, cause low serum levels of the drugs and thus a disposition towards chemoresistance. Host factors also reduce drug delivery to the tumor site, especially in solid bulky tumors with low cell penetration and high molecular weight. Additional mechanisms of cancer cell chemoresistance include receptor loss and mutations in the drug binding site (20).
The administration of various drugs has shown promising effects on chemotherapy with high cure rates by targeting multiple mechanisms of cell entrance. However, cancer cells may develop adaptations to resist these chemotherapeutic drugs, termed multidrug resistance (MDR). MDR strategies include reduced drug accumulation within the cancer cells, decreased uptake, increased efflux, and changes in the membrane lipid properties. These MDR mechanisms limit the apoptosis in cancer cells that are typically induced by anticancer agents, reduce DNA repair mechanisms, and dysregulate the cell cycle and checkpoints in cancer cells. An alternative method to overcome MDR is the use of combination therapy (21). Multidrug resistance proteins (MRPs) decrease the efficacy of anticancer drugs and decrease drug penetration in cancer cells. An additional therapeutic approach involves identifying MDR biomarkers before or during the treatment program (22, 23).
Reduced drug uptake and cell surface molecule mutations are other drug resistance mechanisms. Methods of drug entry into cells includes endocytosis and receptor binding followed by internalization of the drug. An example of the later strategy includes immunotoxins. Cancer cells with defective endocytosis are resistant to immunotoxins and toxins (24, 25).
Drug efflux from cancer cells is accomplished through various pumps. For example, ATP-binding cassette (ABC) transporters [e.g., P-glycoprotein (P-gp)] play an essential role in drug-related clinical resistance. P-gp levels are increased in several cancers through typical molecular mechanisms and directly correlate with chemotherapy resistance (26). Thus, P-gp expressing cells develop in tumors following in vivo exposure to chemotherapeutic agents. In certain cancer types, elevated P-gp resulted in clinical relapse and decreased P-gp showed therapeutic potential. Accordingly, drugs transported by P-gp have a low chemotherapy response. Therefore, P-gp inhibitors have therapeutic potential in chemotherapy. Despite the high expression of P-gp in solid tumors (e.g., colon and renal cancer), no chemoresistance was shown, implying other methods of drug resistance are at work. Recent research has demonstrated the involvement of the ABC transporter multixenobiotic resistance (MXR) and MRPs in drug resistance. These results express the need to consider treatment with nonspecific inhibitors of ABC transporters or a cocktail of specific inhibitors with the broadest spectrum effect (27).
Another mechanism of chemoresistance is reduced intracellular drug activation and improved drug inactivation by phase I and/or II enzymes in the intestine, liver, and tumor (28). Cytochrome P450s (CYPs) are critical phase I metabolism enzymes that act as an oxidation catalyzer in many anticancer drugs. Genetic mutations in CYPs have shown significant effects on the toxicity and efficacy of anticancer agents that are primarily metabolized by CYPs. Carboxylesterase, deoxycytidine, cytidine deaminase, kinase, and epoxide hydrolase are enzymes involved in the detoxification and/or activation of some anticancer drugs. Mutations in these enzymes may alter their activity and play a role in the chemoresistance process. Cancer cells can become drug-resistant by decreasing drug activation through the reduction or mutation of kinases (29). The aforementioned dysregulated mechanisms that contribute to chemoresistance lead to rapid metabolism/excretion, poor tolerance and downstream oxidative stress, apoptosis/autophagy, and inflammation. Figure 1 summarizes the major factors involved in cancer chemoresistance.
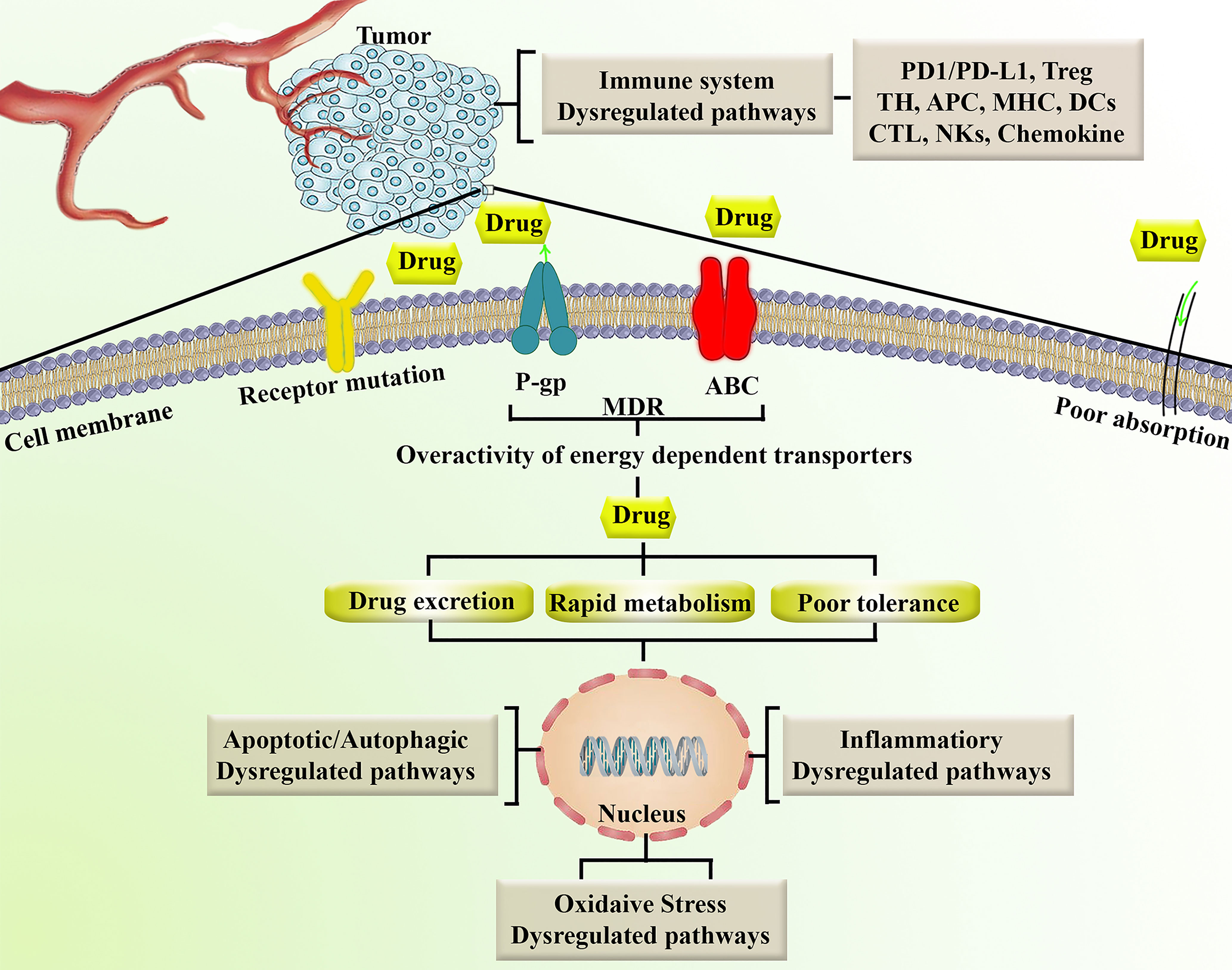
Figure 1 Representation of major factors involved in chemoresistance. ABC, ATP-binding cassette; APC, antigen-presenting cell; CTL, cytotoxic T lymphocytes; DCs, Dendritic cells; MDR, multidrug resistance; MHC, major histocompatibility complex; NKs, natural killer cells; P-gp, P-glycoproyein; PD1/PD-L1, programmed cell death-1/programmed cell death-ligand 1; TH, T helper; Treg, T regulatory.
In addition to the above mechanisms, evasion of apoptosis/autophagy/necrosis is critical to tumor resistance. In any pathological condition, programmed cell death pathways, including apoptosis, and autophagy are the cause of death through intracellular pathways. Such cell death programs may cooperatively determine the fate of malignant neoplasms. Programmed necrosis and apoptosis always contribute to cell death, however, autophagy can play either pro-death or pro-survival roles (30). The mitochondrial (intrinsic) pathway and death receptor (extrinsic) pathway are two methods of apoptosis. Initiation of both pathways ultimately proceeds through caspase-related cascades. A group of cysteine proteases plays an important role in inflammation and apoptosis by cleaving a variety of nuclear and cytoplasmic mediators. Apoptotic caspases can be either initiator or executioner caspases. These include initiator caspase-2, caspase-8, caspase-9, and caspase-10 and executioner caspase-3, caspase-6, and caspase-7. In regard to the initiator caspases, CD95 (APO-1/Fas) and tumor necrosis factor (TNF)-related apoptosis-inducing ligand (TRAIL) are members of the TNF receptor superfamily of death receptors which recruits caspase-8, forming a multimeric complex at the plasma membrane that subsequently activates caspase-3. Caspase-8 causes the release of cytochrome c by increasing the permeability of the outer mitochondrial membrane through cleavage of Bid, a BH3-only protein, and translocation to the mitochondria (31, 32). Mitochondrial apoptosis-induced channel (MAC) also increases the release of cytochrome c, which activates caspase-3 through the creation of pro-caspase-9, apoptotic protease activating factor 1 (Apaf-1), and the apoptosome. The apoptosome converts pro-caspase-9 to its active form, caspase-9, which then activates caspase-3 (31). Apaf-1 forms an oligomeric apoptosome which determines the apoptotic pathway upon binding with ATP and cytochrome c. Such apoptotic pathways are controlled by various negative and positive regulators, which play a critical role in chemoresistance (33).
The B-cell lymphoma 2 (Bcl-2) family of proteins consists of anti-apoptotic and pro-apoptotic proteins. The former includes B-cell lymphoma-extra-large (Bcl-xL), Bcl-2, and myeloid-cell leukemia 1 (Mcl-1), while the latter includes Bcl-2-associated X protein (Bax), Bcl-2 homologous antagonist/killer (Bak), and BH3. Bak and Bax enhance the formation of MAC, while Mcl-1, Bcl-2, and Bcl-xL inhibit its formation (34). Apart from the established roles of Bak and Bax in apoptosis, the ratio of pro-apoptotic/anti-apoptotic proteins is what governs apoptosis, rather than individual protein expression. In cancer cells, the upregulation of anti-apoptotic mediators allows cells to evade apoptosis. This also allows cancer cells to escape apoptosis even when exposed to chemotherapeutic drugs, which would otherwise induce apoptosis in susceptible cells (35). Thus, dysregulation of the pro-apoptotic/anti-apoptotic protein ratio is another mechanism of chemotherapy resistance due to decreased apoptosis of cancer cells.
In cancer cells, the activities of both pro- and anti-apoptotic mediators are controlled by Jun amino terminal kinase (JNK) and p38-mitogen-activated protein kinase (MAPK). The latter increases p53 and apoptosis in chemoresistant cells through the protein kinase B (Akt)/Forkhead box O3 (FoxO) pathway. Insulin-like growth factor 1 suppresses apoptosis via casein kinase 2 and PI3K/Akt pathways, which in turn arrests Smac/DIABLO release and suppresses caspase activity (36). In addition, p53 mutations reduce chemoresistance through modulatory roles on mitochondrial function, leading to increased chemosensitivity. The overexpression of tissue inhibitor of metalloproteinase-1 (TIMP) compromises chemotherapy response via NF-κB and PI3K/Akt signaling pathways (37). The actin-bundling protein, fascin, plays an important role in breast cancer chemoresistance by increasing the level of anti-apoptotic proteins and blocking the entry of the pro-apoptotic proteins caspase-3 and caspase-9 (37). Notch-1 and survivin are other signaling pathways that contribute to chemoresistance by activating targets involved in cell survival, thereby inhibiting apoptosis in tumor cells (38).
Other mechanisms of tumor resistance are related to dysregulated autophagy. Autophagy allows cells to regain ATP and vital biosynthetic factors in tumor microenvironments that are hypoxic and starved, promoting cancer cell survival. Autophagy acts as a critical modulator of intracellular hemostasis, tumor suppression, aging, cell death, and tumor chemoresistance (39). However, autophagy acts as a double-edged sword, as it also plays a role in the initiation, growth, development, and invasion of tumor cells. Accordingly, the PI3K/Akt/mTOR signaling pathway plays a crucial role in autophagy by modulating cell growth, cell survival, protein synthesis, motility, cell metabolism, cell death, and chemoresistance (39). This also downregulates the pro-apoptotic mediators Bim and Bad (40). Apoptotic mediators and autophagy are also regulated by upstream JNK and p38MAPK, thereby playing a vital role in modulating chemoresistance (41). Many anticancer drugs disrupt the balance between autophagy and apoptosis by altering the genetic/epigenetic phenotype and inhibiting PI3K/Akt/mTOR in cancer cells, thereby leading to the development of chemoresistance (41).
Resistance Mechanisms in Cancer Immunotherapy
Immunotherapy resistance is a primary and/or acquired resistance of tumor cells to immunotherapy (42). The inhibitors of programmed cell death-1/programmed cell death-ligand 1 (PD-1/PD-L1) increase the release of interferon γ (IFN-γ) and upregulate the JAK/STAT signaling pathway. This activates IFN regulatory factor 8 (IRF8), causing hyperprogression (HPD) (43). HPD is a primary form of drug resistance (44) associated with mutations of epidermal growth factor receptor (EGFR) [33], murine double minute (MDM) gene (43), and chromosome 11 region 13 (43).
Attenuation of immune checkpoints potentially stimulates regulatory T cells (Tregs), creating an immunosuppressive microenvironment and modulating autoantigenicity antigen shedding or endocytic antigens to mediate immune escape (45). Such conditions trigger the polarization of immunosuppressive cells, such as M2 macrophages, antigen-presenting cells (APCs), and myelocytes, producing immunosuppressive cytokines. This also stimulates T helper type 1 (TH1) and TH17-mediated inflammatory conditions to upregulate oncogenic pathways and accelerate tumor growth and immunotherapy resistance (43, 46). Consequently, dendritic cells (DCs), B lymphocytes, monocyte-macrophages, and other APCs such as fibroblasts, endothelial cells, mesothelial cells, and epithelial cells are interconnected with tumor-specific antigen (TSA)/tumor-associated (TAA), conferring immunogenicity and T cell infiltration in tumors. Autophagy and the endoplasmic reticulum (ER) determine the tumor-associated immunogenicity of cell death (47). Dysregulation of antigen presenting signaling pathways, including mutations of the proteasome, transporters, and major histocompatibility complex (MHC), is cross-talked with T cell activity and tumor immune escape. MHC mutations are classified into structural defects, changes in the receptor-binding domain, and epigenetic changes (48). In some cancer types, tumor cells are able to escape lysis mediated by cytotoxic T lymphocytes (CTLs) and natural killer cells (NKs) through the overexpression of MHC-I. This allows tumor cells to escape the immune system (49).
The dysregulation of emerging signaling pathways in tumor cells is another mechanism of immunotherapy resistance. For instance, IFN-γ, produced by T cells and APCs, binds to related receptors to activate JAK2 (50). This leads to interaction with STAT1, which modulates downstream cascades. IFN-γ allows tumor cells to escape the immune system by increasing the expression of PD-L1 on the surface of tumor cells (51). IFN-γ also upregulates C-X-C motif chemokine ligand (CXCL)-9 and CXCL-10 chemokines and promotes antitumor immune cell effects (51). Additionally, IFN-γ exerts pro-apoptotic and antitumor properties through binding to cell surface receptors and triggering downstream mediators to suppress tumor cells (51). In patients receiving immunotherapeutic agents, tumor cells alter IFN-γ and JAK/STAT1 signaling pathways. Tumor analysis of chemoresistant patients receiving anti-cytotoxic T-lymphocyte-associated protein 4 (CTLA-4) agents had mutations in IFN-γ pathway genes, JAK1/2, and interferon regulatory factors (52). This allowed tumor cells to evade T cells, thus resisting the anti-CTLA-4 treatment. Loss of polybromo and BRG1-associated factors (PBAF) complex increased the ability of chromatin to regulate IFN-γ as well as increased production of CXCL-9/CXCL-10 to recruit T cells to tumor tissue (53). In human cancers, expression of Pbrm1 and Arid2 is correlated with the presentation of T cell cytotoxicity genes, leading to immunotherapy resistance (53).
Spranger et al. (54) demonstrated that the infiltration of T cells and recruitment of DCs into the total mesorectal excision (TME) could be suppressed by tumor-intrinsic β-catenin activation via decreased expression of CCL4. Because DCs prevent migration into epithelial-to-mesenchymal transition (EMT), no antigen can be presented to T cells, halting their cytotoxic effects. From another mechanistic point, upregulation of MAPK signaling damages the function and infiltration of tumor-infiltrating lymphocytes through the expression of vascular endothelial growth factor (VEGF) and cytokines such as interleukin-8 (IL-8) (55). Under these conditions, induction of Tregs ultimately leads to tumor immune evasion. Loss of tumor suppressor phosphatase and tensin homolog (PTEN) leads to activation of PI3K signaling, which is associated with increased anti-inflammatory cytokines, such as VEGF and C–C motif chemokine ligand 2 (CCL2), reduced infiltration of CD8+ T cells into tumors, and decreased IFN-γ expression, conferring resistance of PD-1 blockade therapy against tumors (56).
Tumor cells develop immunotherapy resistance by altering tumor cell metabolism through multiple metabolic changes, termed tumor metabolic reprogramming (57). One such mechanism utilizes aerobic glycolysis to create a hypoxic acidic environment which prevents normal metabolism of immune cells and impairs T cell function and infiltration (58). Furthermore, glucose consumed by tumor cells may restrict T cell metabolism, which leads to inhibition of mTOR, decreased glycolytic capacity in T cells, and production of intracellular IFN-γ (59).
TLR/NF-κB/NLRP Signaling Pathway in Cancer Initiation and Progression and Chemoresistance
TLRs are members of the type I transmembrane proteins and are conserved pattern-recognition receptors (PRRs) that are activated by various pathogen-associated molecular patterns (PAMPs). These membrane proteins are heavily expressed on the surface of several cells, including monocytes, macrophages, and DCs. The three constructional domains of TLRs’ include a leucine-rich repeats (LRRs) motif, a transmembrane domain, and a cytoplasmic domain. Each of these domains have a specific function. For example, pathogen recognition is performed by the LRR motif, while signal initiation is performed by interaction of the TIR domain with the signal transduction adaptors. This receptor family is extremely important for pathogen recognition by the innate immune system (60, 61). Recently, several reports have indicated the association between cancer and TLRs. Specifically, the TLR4 signaling pathway is the most tightly linked with inflammatory response and cancer initiation and progression (16).
TLRs are involved in tumor progression, however they may display either anti- or pro-tumor metastasis and growth features (62). Activation of TLR4 increased IL-6 and IL-8 production in breast cancer (63). In some cancers, TLR4 induced the production of nitric oxide and IL-6 (64). In prostate cancer cells, TLR4 activation enhanced the expression of transforming growth factor-β1 (TGF-β1) and VEGF, which promoted tumor progression (65). Some studies have shown poorer outcomes for breast, colon, and pancreatic cancers when TLR4 is overexpressed (63, 64). The myeloid differentiation factor 88 (MyD88) pathway of TLR4 has been shown to improve carcinogenesis. Yusef et al. (66) found that TLR4 demonstrated antitumor activity in skin cancer. The role of TLR4 should be further evaluated in various cancer types. Overall, these results suggest that the release of various inflammatory mediators, cytokines, and chemokines activates TLR4 and may participate in cancer formation.
TLRs are strong actuators of the inflammatory response, activation of which triggers the production of interferons, chemokines, cytokines, and NF-κB. The NF-κB pathway plays a crucial role in various diseases through regulation of cell proliferation, differentiation, immunity, and apoptosis (67). The NF-κB family consists of five crucial parts: p50, p52, p65/RelA, c-Rel, and RelA. NF-κB acts as a transcription factor by binding DNA, which activates gene transcription. Several genes involved in the progression and development of cancer are regulated by NF-κB, such as those involved in proliferation, apoptosis, and migration. Improper or constitutive NF-κB activation has been found in many malignant human tumors (68).
Typically, NF-κB is bound to IκB (IκB) in the cytoplasm. In times of stress, reactive oxygen species (ROS) and inflammatory stimuli degrade the IkB complex to activate NF-κB, releasing inflammatory cytokines such as tumor necrosis factor-alpha (TNF-α), IL-1, IL-6, and IL-2. This inflammatory cascade suppresses apoptosis and induces cellular invasion, proliferation, and metastasis, aiding in chemoresistance [52]. Prevailing reports have shown that activation of NF-κB by tumors assists in the development of chemotherapy resistance. NF-κB activation plays a key role in hindering the effectiveness of chemotherapeutic agents. Tumor cells exposed to chemotherapeutic drugs or radiation showed increased activation of NF-κB, which enforced the expression of MDR P-gp. Meanwhile, NF-κB suppression improved the apoptotic response to radiation therapy (69).
Members of the NLR family play an essential role in the signaling pathways of the innate immune system by activating or inhibiting inflammasomes. Damage-associated molecular patterns (DAMPs) and PAMPs activate NLRs and absent in melanoma 2 (AIM-2)-like receptors (ALRs), which bind to associated cytosolic domains to activate caspases. As a result, caspases upregulate IL-18 and IL-1β, which results in apoptosis and pyroptosis (70). On the other hand, dysregulation of NLR contributes to various autoimmune and inflammatory diseases. Thus, NLR can play a role in tumor suppression or tumor promotion in the initiation, development, and regression of cancer (71). Therefore, targeting the TLR/NF-κB/NLRP signaling pathway may facilitate improvement in the regulation of cancer initiation/progression and associated chemoresistance.
TLR/NF-κB/NLRP Signaling Pathway in Cancer Immunotherapy
TLRs are part of a family of recognition receptors which play a pivotal role in the host immune system (72–74). TLRs are expressed by B cells, macrophages, monocytes, NK cells, mast cells, neutrophils, and basophils. TLRs stimulate pro-inflammatory chemokines and cytokines to activate the innate and adaptive immune systems. The activation of TLR4 can induce associated adaptor proteins, including MyD88, TIR domain-containing adapter molecule 1 (TICAM1), TIR domain-containing adapter molecule 2 (TICAM2), and TIR domain-containing adaptor protein (TIRAP). Some ligands (e.g., lipopolysaccharides and toxins) bind TLRs to activate the immune response. According to Nagai et al. (75), the co-receptor myeloid differentiation factor-2 (MD-2) increased the translocation of TLR4 to form a heterotrimer of CD14/TLR4/MD-2 (76). This may lead to two distinct signaling pathways, the MyD88 pathway and the toll/IL-1R domain-containing adapter-inducing IFN-β (TRIF) pathway. Tumor necrosis factor receptor-associated factor 6 (TRAF6) activates extracellular signal-regulated kinase (ERK), MAPKs, and the p38 signaling pathway. Alternatively, TLR4 activates the MyD88-independent pathway to upregulate NF-κB and suppress IκB kinase epsilon (IKK). MyD88-dependent and MyD88-independent pathways also contribute to host defense and engage the immune response. In addition, TLRs activate IRFs, which increase the transcription of interferon-α (IFN-α) and interferon-β (IFN-β) (77). NLRP is the downstream mediator of NF-κB, which is interconnected with the inflammasomes. Inflammasomes are receptors/sensors of the innate immune system that regulate caspase-1 activation in response to host-derived proteins. Accordingly, NLRP activates apoptosis cascades which contributes to cancer chemoresistance. Overall, TLR/NF-κB/NLRP play critical roles in the development of cancer chemoresistance mediated by the attenuation of apoptosis, inflammation, oxidative stress, and autophagy. As shown in Figure 2, the immune system is also cross-talked with dysregulated major signaling pathways of chemoresistance.
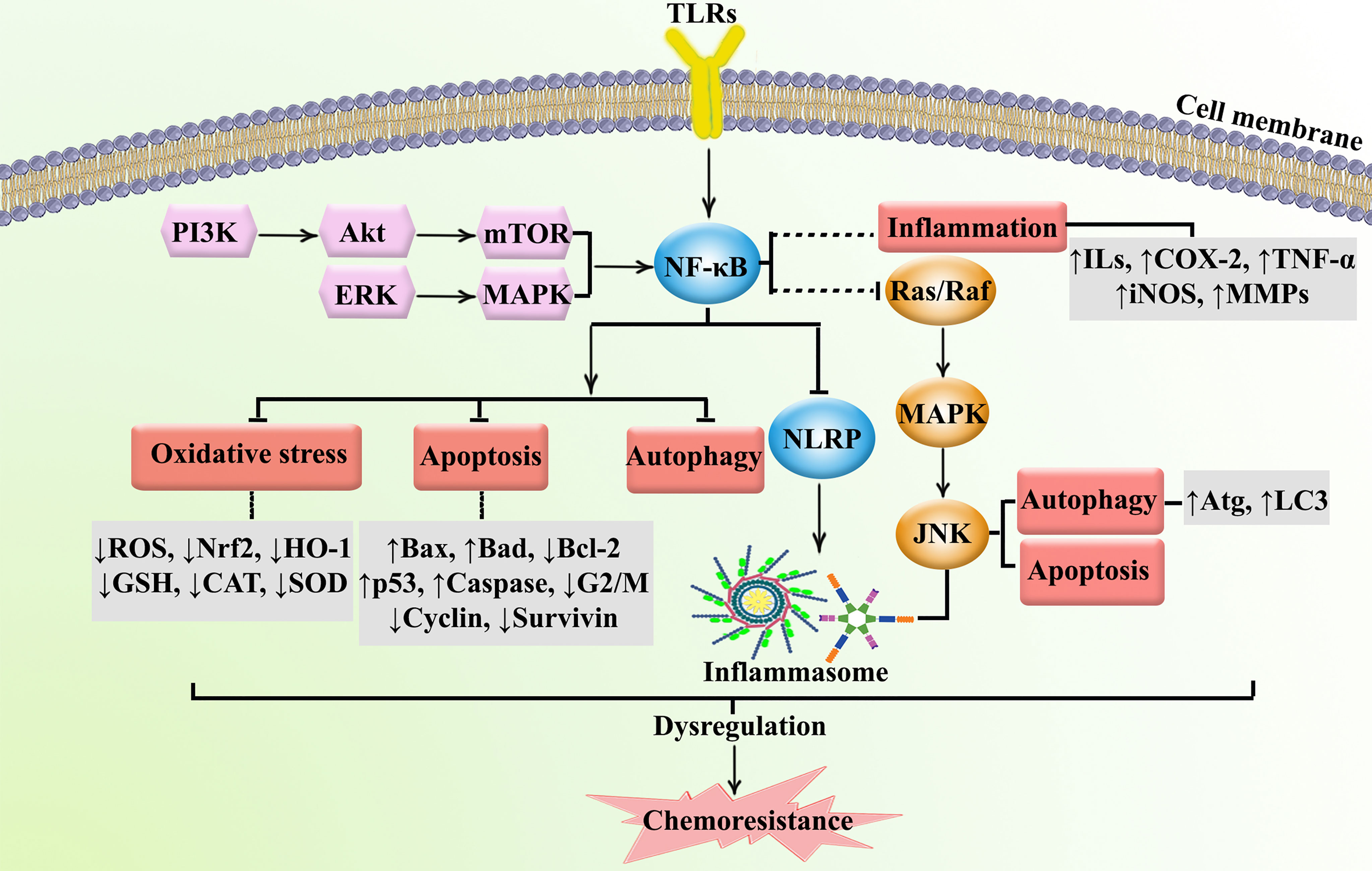
Figure 2 Major dysregulated pathways in cancer chemoresistance. Atg, autophagy-related; CAT, catalase; COX-2, cyclooxygenase; ERK, extracellular-regulated kinase; GSH, glutathione; HO-1, heme oxygenase 1; ILs, interleukins; iNOS, inducible nitric oxide synthase; JNK, c-Jun N-terminal kinase; LC3, microtubule-associated protein 1A/1B-light chain 3; MAPK, mitogen-activated protein kinase; MMP, matrix-metalloproteinase; mTOR, mammalian target of rapamycin; NLRP, nod-like receptor pyrin domain-containing; Nrf-2, nuclear factor-erythroid factor 2-related factor 2; PI3K, phosphoinositide 3-kinases; ROS, reactive oxygen species; SOD, superoxide dismutase; TNF-α, tumor necrosis factor-α.
Methodology for Literature Search on the Effect of Phytochemicals on Chemotherapy and Immunotherapy Resistance
We have performed a systematic review on vital mechanisms and the therapeutic potential of plant secondary metabolites in combating cancer chemoresistance utilizing the PRISMA guideline. Scholarly electronic databases, including Scopus, Science Direct, Cochrane, and PubMed, were used for the literature search. The search included all English language articles through October 30, 2021. The following keywords were used for the search: chemoresistance [full text] OR (cancer OR malignancy OR neoplasm OR melanoma OR leukemia OR carcinoma) [title/abstract] AND (nuclear factor kappa* OR NF-κB OR toll-like* OR nod-like receptor* OR NLRP) AND (chemotherapy OR chemoresistance OR immunotherapy OR chemo-therapy OR chemo-resistance OR immune-therapy) [title/abstract] AND (herb OR plant OR natural product OR secondary metabolite OR polyphenol* OR terpen* OR alkaloid* OR flavonoid* OR glucosinolate* OR coumarin*). Two independent authors (S.F. and S.Z.M.) designed and applied the search strategy, which was finalized by the senior author (A.B.).
Of the initial 1392 articles, 205 articles were excluded due to duplicated results, 297 articles were excluded as they were review articles, 691 articles were excluded according to their title/abstract, 229 articles were excluded according to their full text information, and 4 articles were omitted since they were not in English. Ultimately, 267 articles were included in this systematic review. Figure 3 depicts the PRISMA flowchart, which displays the literature search process and selection of relevant studies.
Multi-Targeting Phytochemicals in Cancer Therapy
Plant secondary metabolites are potential modulators of multiple dysregulated pathways due to their various pharmacological properties, including antioxidant, anti-inflammatory, and anticancer effects (78, 79). An increasing number of pre-clinical and clinical studies have shown that chemopreventive agents may regulate the aforementioned dysregulated signaling pathways, such as TLR, NF-κB, and NLRP, thereby preventing or treating multiple cancer complications (16). Considering the critical role of TLR/NF-κB/NLRP in the progression of chemotherapy and immunotherapy resistance, discovering multi-targeting therapeutic agents could assist in combating cancer chemoresistance and immunoresistance. Several reports have addressed the potential of phytochemicals in the attenuation of TLR/NF-κB/NLRP. Therefore, phenolic compounds, alkaloids, terpenes/terpenoids, and sulfur compounds have been proposed as potential agents in the prevention and treatment of chemoresistance and immunoresistance.
Phytochemicals Augment Chemotherapy and Immunotherapy Through TLR/NF-κB/NLRP Pathway
Phytochemicals may be used as alternative anticancer agents to prevent chemoresistance. This is made possible by surpassing the resistance barrier in multiple pathways, leading to increased effectiveness. Another benefit of utilizing phytochemicals is that they lower the dose frequency and thus the toxicity of chemotherapeutic agents. The principal mechanism of these phytochemical effects is through the inhibition or overexpression of certain proteins, enzymes, and other cancer cell metabolites.
Phenolic Compounds
Natural polyphenols are an important class of plant secondary metabolites that play an active role against different types of stress. The considerable volume of reported data proposed that diets rich in phenolic compounds could decrease the incidence of several cancers. Curcumin (Figure 4) is a well-known phytochemical with several important biological activities, including anticarcinogenic, neuroprotective, anti-inflammatory, and anti-SARS-CoV-2 effects (6, 13, 80–85). Curcumin suppressed the proliferation of MHCC97H liver cancer cells in vitro by promoting the formation of intracellular ROS, increasing apoptosis, and activating caspase-3, caspase-8, and TLR4/MyD-88 signaling (86). Furthermore, suppression of HSP70/TLR4 signaling was reported as another anticancer mechanism of curcumin in liver cancer (87). Curcumin also inhibited the growth of liver cancer in vivo and in vitro via diminished expression of inflammatory factors, such as cyclooxygenase-2 (COX-2), prostaglandin E2, IL-1β, and IL-6, as well as inhibition of the TLR4/NF-κB signaling pathway. Moreover, curcumin reduced VEGF, granulocyte-colony-stimulating factor (G-CSF), and granulocyte−macrophage colony-stimulating factor (GM-CSF) (88). Additionally, curcumin decreased the migration and proliferation of non-small-cell lung cancer cell (NSCLC) cells via interfering with EGFR and TLR4/MyD88 pathways and increasing cell cycle arrest in the G2/M phase (89). Treatment with curcumin has also decreased the viability of MCF-7 and MDA-MB-231 breast cancer cells, activated TLR4/TRIF/IRF-3 signaling through the inhibition of IFN-α/β, and reduced the expression of TLR4 and IRF-3 (90). In a similar study, curcumin reduced cell proliferation, inhibited NF-κB, downregulated cyclin D1, and modulated expression of TLR3 in head and neck squamous cell carcinomas (HNSCC) (91). Other reported antitumor mechanisms of curcumin include inhibition of NF-κB, cell cycle arrest, upregulation of E-cadherin, and modulation of Wnt/β-catenin signaling (92, 93). Deng et al. investigated the synergistic inflammatory and immunomodulatory activity of curcumin in combination with total ginsenosides for the treatment of HepG2 liver cancer cells in a BALB/c mice model. The results demonstrated that combination treatment inhibited the growth of liver cancer, reduced the expression of PD-L1, and suppressed the TLR4/NF-κB and NF-κB/matrix metalloproteinase-9 (MMP-9) signaling pathways (94). Curcumin exerts antineoplastic effects on various in vitro and in vivo cancer models, including lung (95), colorectal (96–98), bladder (99, 100), pancreatic (101, 102), and breast (103) cancers via interfering with the expression of TNF-α, HIF-1α, COX-2, VEGF, NF-κB, Axin2, IL-10, IL-8, and IL-6 and participating in the PI3K/Akt/mTOR/NF-κB/Wnt pathway. Additionally, curcumin promoted apoptosis, inhibited NF-κB, MMP-9, MMP-2, and MAPK, and activated sirtuin 1 (SIRT1) in HNSCC, osteoclastoma, and monocytic leukemia SHI-1 cancer cells (104–107). The known plant flavonoid quercetin is widely distributed in many vegetables, seeds, leaves, and grains and shows promising biological properties. Quercetin (Figure 4) and curcumin act synergistically together to promote apoptosis in K562 leukemia cells by interfering with the p53, TGF-α, and NF-κB pathways (108).
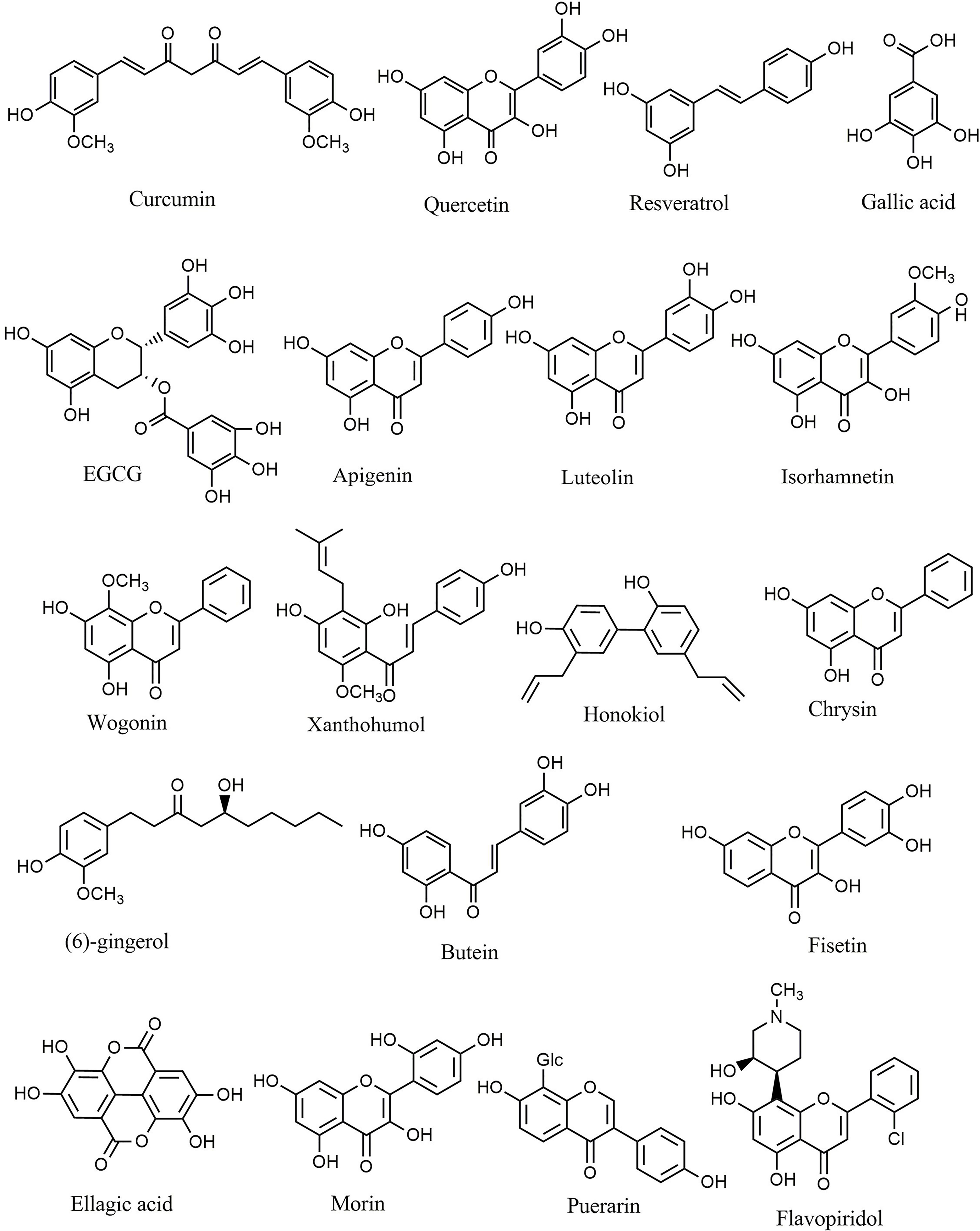
Figure 4 Chemical structures of selected phenolic compounds that modulate the TLR/NF-κB/NLRP signaling in cancer.
Resveratrol belongs to the stilbenoid group of polyphenols that exhibit high antioxidant and antitumor potential, which can be found in more than 70 plant species, particularly in grapes’ seeds and skin. Resveratrol (Figure 4) and quercetin potentiated the antineoplastic activity of curcumin in myeloid, adenocarcinoma, and HNSCC cells (109–111). Resveratrol reduced dimethylbenz(a)anthracene (DMBA) induced cutaneous carcinogenesis both in vitro and in vivo via inhibition of angiogenesis. It was reported that TLR4 is a significant mediator involved in the chemoprevention achieved by resveratrol (112). Moreover, resveratrol diminished the inflammatory responses induced by lipopolysaccharide in SW480 and Caco-2 colon cancer cell lines by reducing the activation, expression, and production of inducible NO synthase (iNOS), mRNA, TLR4, and NF-κB (113). Furthermore, resveratrol suppressed the activity of COX, AMP-activated protein kinase (AMPK), PI3K/Akt/NF-κB pathway, DNA methyltransferase, and CYP1A1 in acute myeloid leukemia (AML), colon, and pancreatic cancer cells (114–116). In similar studies, resveratrol exerted substantial antineoplastic activity against multiple cancer cell lines, including melanoma (117), lung (118, 119), glioblastoma (120), head, neck (109), hepatocellular (121), colorectal (122), and breast (123) cancer cells by interfering with dicer-like 1 (DCL1)/translationally controlled tumor protein (TCTP), Akt/NF-κB, retinoblastoma protein (pRB), VEGF, AMPK, and p21Waf1/Cip1 signaling pathways. Luteoloside (known as Cynaroside), 7-O-glucoside of luteolin, is a flavone agent that inhibited metastasis and proliferation of SNU-449, Hep3B, and mouse lung cancer cells through inhibition of caspase-1, NLRP3, and IL-1β (124).
Gallic acid is a natural antioxidant found in various fruits and tea leaves that belongs to the polyphenolic class of secondary metabolites. Various pharmacological effects of gallic acid include antioxidant, anti-inflammatory, and antineoplastic activities. There have been multiple studies which report that gallic acid (Figure 4) inhibited the progression of T24 and AGS gastric cancer cells via suppression of PI3K/Akt/NF-κB signaling and promotion of mitochondrial dysfunction (125, 126). Furthermore, quercetin inhibited the invasion and migration of Caco-2 cells via regulation of the TLR4/NF-κB pathway and decreasing MMP-2 and MMP-9 (127). In a similar study, inhibition of NF-κB, p53 induction, apoptosis, and cell cycle arrest were reported as the primary anticancer mechanisms of quercetin against the HeLa cervical cancer cell line (128). Additionally, quercetin showed antitumor activity against lung (A549 and H460) (129, 130), prostate (PC3 and LNCaP) (131), breast (MCF-7) (132), and oral SCC (133) cancer cells via induction of apoptosis and downregulation of IL-6/STAT-3 and NF-κB. The results demonstrated that combination of quercetin with chrysin suppressed the migration and invasion of nickel via downregulation of TLR4/NF-κB signaling in human lung cancer cells in vitro (134). Octyl gallate exerted significant efficacy against heat shock protein 90α (HSP90α) levels, eHSP90α–TLR4 ligation, M2-macrophages, and tumor growth in a pancreatic ductal adenocarcinoma mouse model (135).
Moreover, several studies have been performed to investigate the various biological effects of epigallocatechin 3-gallate (EGCG), a powerful polyphenolic isolated from green tea. EGCG showed significant anti-inflammatory, antioxidant, anticancer, and neuroprotective potential in different studies. Treatment with EGCG (Figure 4) downregulated the expression of NLRP1, caspase-1, and IL-1β in the melanoma cell lines HS294T and 1205Lu (136). The main antineoplastic mechanisms of EGCG includes inhibition of TNF-α and tissue factor expression (137), activation of forkhead box O3 (138), downregulation of Her-2/Neu signaling (139), decreased expression of IL-1RI (140), and modulation of MMP-2 activity (141). Furthermore, EGCG induced apoptosis and suppressed cancer cell proliferation in nasopharyngeal (142), bladder (143), hepatocellular (144), breast (145), and colon (146) cancers.
Similarly, apigenin is another polyphenolic substance with significant anticancer potential via modulating different signaling pathways in vitro and in vivo. Treatment with apigenin induced apoptosis, suppressed glycogen synthase kinase-3 (GSK-3)/NF-κB, and downregulated Bcl-xL, CCL2, CXCL-8, IL1A, Bcl-2, and VEGF in pancreatic (PANC-1 and BxPC-3) (147), prostate (PC-3) (148, 149), and breast (MDA-MB-231) cancer cells (150), as well as the athymic nu/nu nude (151) and TRAMP mice (152) cancer models. In addition to apigenin, luteolin (Figure 4) has significant anticancer properties. Luteolin interfered with the PI3K/Akt/NF-κB/Snail and MAPK pathways in the gastric adenocarcinoma cell line CRL-1739, the lung cancer cell line A549, and the AML cell line THP-1 (153–155). Additionally, luteolin 8-C-b-fucopyranoside suppressed secretion of MMP-9, IL-8, ERK/NF-κB, and ERK/AP-1 signaling in MCF-7 cancer cells in vitro (156). The in silico evaluations also showed that luteolin and other plant-derived secondary metabolites (e.g., myricetin, quercetin, apigenin, and baicalein) displayed anticancer properties via the estrogen receptor-α (157).
Isorhamnetin (Figure 4) is another polyphenolic compound that induced apoptosis and inhibited proliferation of lung (158) and breast (159) cancer cells by interfering with IL-13, NF-κB, MAPK, and Akt signaling. Similarly, wogonin (Figure 4) promoted apoptosis and suppressed the invasion and proliferation of chronic lymphocytic leukemia and the liver cancer cell lines Bel7402 and HepG2 by interfering with ERK/AKT, NF-κB/Bcl−2, and EGFR signaling pathways (160, 161). Another polyphenolic structure, xanthohumol (Figure 4), appears to have anticancer properties via significantly suppressing the angiogenesis, proliferation, and production of inflammatory mediators in breast cancer xenografts (162). Xanthohumol also reduced the expression of CXCR4 and inhibited cancer cell invasion (163). Similarly, p-hydroxycinnamic acid facilitated cell cycle arrest and suppressed the growth and migration of MDA-MB-231 cells via downregulation of NF-κB (164). The flavonoid wogonoside demonstrated anticancer effects in vitro against MCF7 and MDA-MB-231 cancer cells through inhibition of migration, invasion, and TRAF-2/TRAF-4 expression (165). In similar studies, inhibiting COX-2, EGFR, NF-κB, and the ERK pathway is the main anticancer mechanism of scutellarein in A549 cells (166). Likewise, hydroxysafflor yellow A (167), rosmarinic acid (168, 169), and magnolol (170) diminished the progression of hepatocellular carcinoma in vitro and in vivo by suppressing ERK/MAPK, ERK/NF-κB, and NF-κB signaling. Lung cancer cells treated with hexamethoxy flavanone-o-[rhamnopyranosyl-(1→4)-rhamnopyranoside, a flavonoid glycoside compound isolated from Murraya paniculata (171), hesperetin (172), honokiol (Figure 4) (173, 174), and inotilone (174) had higher levels of apoptosis-related mediators and attenuated activity of EGFR, PI3K/Akt/MAPK, and STAT3/NF-κB/COX-2 signaling pathways. Investigation into the effects of eupatilin (175, 176), polysaccharide krestin (177), tilianin (178), silibinin (179–181), chrysin (Figure 4) (182–186), (6)-gingerol (Figure 4) (187), and butein (Figure 4) (188) against gastric, breast, ovarian, pharyngeal squamous, prostate, renal, myeloid leukemia, and T cell leukemia/lymphoma cancer cells in vitro and in vivo demonstrated that these polyphenolics exert significant effects via attenuation of angiogenesis, Akt, tumor growth, AP-1, NF-κB, and TLR4.
Fisetin (Figure 4) (189–194), gallotannin (195), astragalin (196–198), ellagic acid (Figure 4) (199–201), morin (Figure 4) (202, 203), flavopiridol (Figure 4) (204), puerarin (Figure 4) (205), icariin (206), acteoside (207), and acacetin (208, 209) are some of the other polyphenolic agents that inhibited the proliferation and invasion of breast, prostate, hepatocellular, myeloma, and colon cancer cells via increased apoptosis and inhibition of TNF-α, iNOS, NF-κB, COX-2, JAK/STAT3, Akt, and IL-6 mediators and signaling pathways.
In further studies, eriodictyol (210, 211), calycosin (212), cudraflavone B (213), protocatechualdehyde (214), and naringin (215) exerted significant antitumor effects against several cancers including breast (MDA-MB-231), glioblastoma (A172, CHG-5, and U87 MG), ovarian (SKOV3), and liver (HepG2) cancer cells by promoting senescence, apoptosis, and interfering with GSK-3β, TGF-β1, SMAD2/3, SLUG, vimentin, β-catenin, NF-κB, COX-2, and cyclin D1, amongst other enzymes and signaling pathways.
In summary, polyphenols play a significant role in the prevention and treatment of cancer. Curcumin, apigenin, quercetin, and resveratrol are the most important polyphenols with reported information on their mechanisms of action and clinical trials. In several reported studies, polyphenols can interfere with a variety of anticancer pathways, including TLR/NF-κB/NLRP and interconnected pathways. Consequently, polyphenols could be considered promising treatment options in conjunction with other cancer treatment strategies. Table 1 provides the various anticancer phenolic compounds that interfere with the TLR/NF-κB/NLRP pathway to combat chemoresistance.
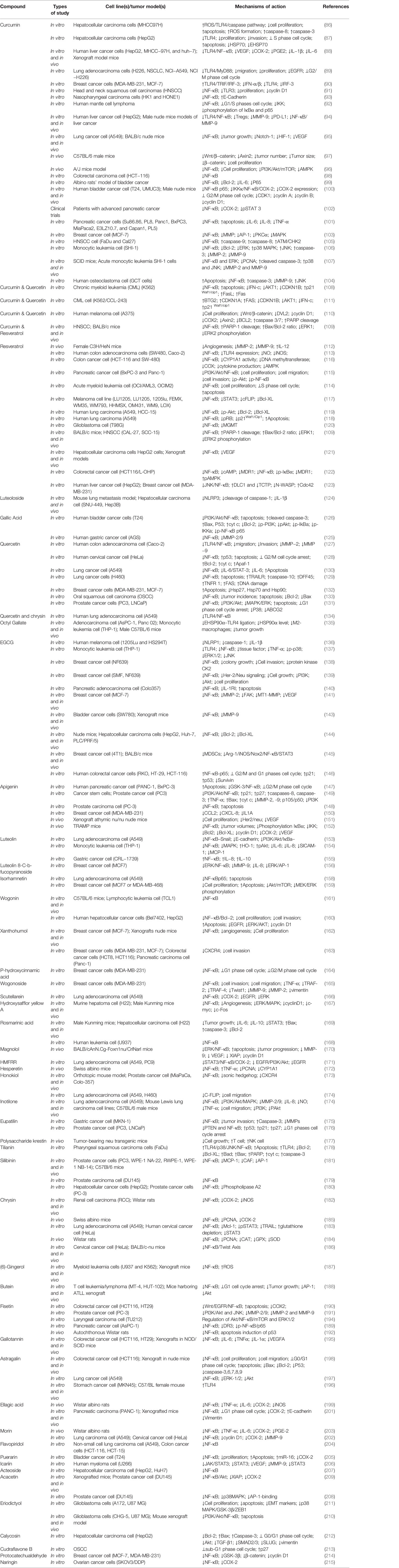
Table 1 Anticancer phenolic compounds interfering with the TLR/NF-κB/NLRP pathway and cross-talked mediators against chemoresistance.
Terpenes and Terpenoids
Terpenes and terpenoids represent large classes of natural products isolated from multiple vegetative sources. These phytochemicals exert several therapeutic effects, including anticancer, cardioprotective, neuroprotective, and hepatoprotective activities. Each terpene/terpenoid compound is composed of several isoprenes (a five-carbon unit) that are assembled in thousands of ways. Zerumbone (Figure 5), an important sesquiterpene isolated from ginger, suppressed lung and colon tumors in mice via induction of apoptosis and inhibition of proliferation, heme oxygenase 1 (HO-1), and NF-κB expression (216). Andrographolide (Figure 5) is a bioactive phytochemical obtained from Andrographis paniculata that belongs to the diterpenoid compounds. Likewise, andrographolide showed antitumor activity against B16 melanoma cells, C57BL/6J mice (217), and RIP1-Tag2 mice (218) via suppression of TLR4/NF-κB signaling, thereby reducing the expression of CXCR4 and Bcl-6. Additionally, treatment with andrographolide inhibited the proliferation of SW620 colon cancer cells in vitro via interfering with the TLR4/NF-κB/MMP-9 signaling pathway (219).
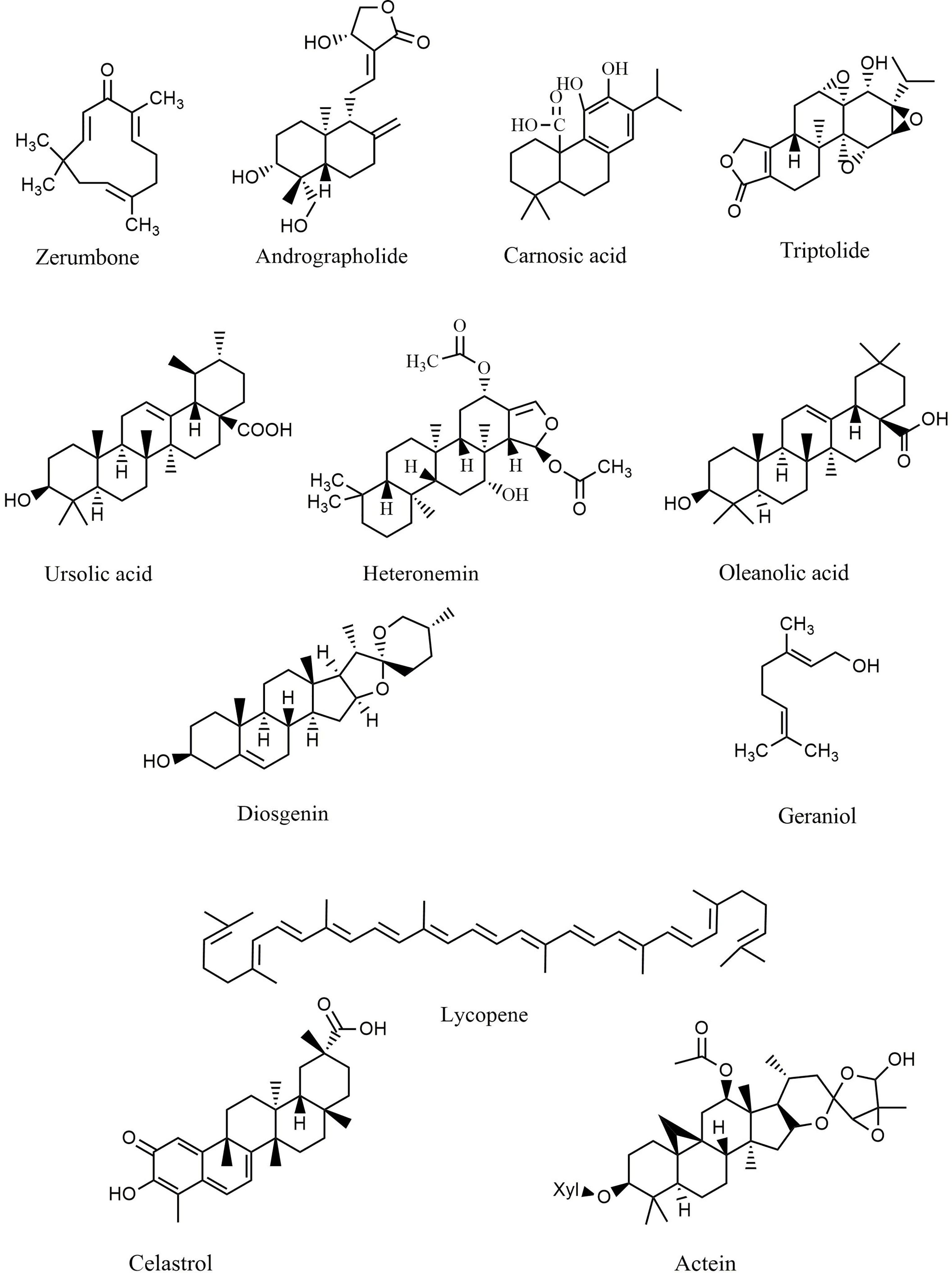
Figure 5 Chemical structures of selected terpenes/terpenoids that modulate the TLR/NF-κB/NLRP signaling in cancer.
Carnosic acid (Figure 5), one of the principal phenolic diterpenes isolated from rosmarinus officinalis, possesses antimicrobial, antioxidative, and anti-carcinogenic properties. Carnosic acid nanoparticles induced apoptosis in Bel7402 and MHCC97-H hepatic carcinoma cell lines in vitro via suppression of NF-κB, caspase-3, TLR4, MyD88, TRAF-6, interleukin 1 receptor associated kinase 1 (IRAK-1), and IRAK-4 (220). Similarly, triptolide (Figure 5) is an active natural phytochemical isolated from Tripterygium wilfordii Hook F that exhibits a wide range of pharmacological effects, including anti-diabetic, neuroprotective, anti-inflammatory, and antitumor activities. Triptolide is a well-known diterpenoid that blocks the NF-κB survival pathways and activates ERK1/2 and p38a in PC3 cancer cells (221). Additionally, triptolide decreased the expression of MMP-9, AP-1, and NF-κB signaling pathways in MCF-7 cells (222) and attenuated the angiogenesis and invasion of thyroid carcinoma cells in vitro and in vivo (223–226). Ursolic acid (Figure 5) exerts anti-inflammatory activity via suppression of the TLR4-MyD88 pathway and decreased production of inflammatory factors, including IL-1β, TNF-α, and IL-6 in abelson murine leukemia macrophage (RAW 264.7) cells (227). In a similar study, ursolic acid demonstrated significant in vitro and in vivo anticancer activity against DU145 and LNCaP prostate adenocarcinoma cells, as well as a mouse model via diminished CXCR4/CXCL-12 signaling axis and reduced activation of NF-κB (228). Furthermore, ursolic acid induced apoptosis and inhibited growth in several in vitro pancreatic and colon cancer models by interfering with PI3K/Akt/NF-κB, STAT, GSK, TRAIL, and JNK pathways (229, 230). In addition to ursolic acid, heteronemin (Figure 5) showed significant antiproliferative effects against AML cells via targeting NF-κB, Ras, MAPK, AP-1, and c-myc (231). Soyasaponins, bioactive phytochemicals found in a multitude of legumes, downregulated TLR4/MyD88 signaling and decreased TNF-α, IL-6, COX-2, NO, IL-1β, and iNOS in inflammatory macrophages (232). Oleanolic acid (Figure 5), another triterpenoid structure, and its synthetic derivative SZC014 showed considerable antitumor effects against the hepatocellular cancer cell lines HepG2, interfering with NF-κB and nuclear factor-erythroid factor 2-related factor 2 (Nrf-2)/antioxidant response element (ARE) signaling (233, 234). Additionally, inhibition of IκB kinase and suppression of NF-κB signaling are the primary anticancer mechanisms of lycopene (Figure 5) in breast and prostate cancer cells (235).
The monoterpene geraniol (Figure 5) is an acyclic isoprenoid derived from essential oils. Geraniol attenuated tongue carcinogenesis via decreased activation of NF-κB (236). Additionally, the NF-κB and STAT3 pathways are the chemopreventive mechanisms of andrographolide via inhibition of inflammatory mediators (237). Moreover, treatment with celastrol (Figure 5) downregulated NF-κB and reduced the expression of IL-6 in prostate and breast cancer cells (238, 239). In a similar study, actein (Figure 5) strongly suppressed the growth of MDA-MB-453 cells by enhancing the cytoplasmic calcium and modulating the MAPK/ERK kinase (MEK) and NF-κB pathways (240).
Diosgenin (Figure 5), a known steroidal triterpenoid with two pentacyclic rings, is found in Trigonella foenum graecum (241). Progesterone, pregnenolone, cortisone, and other steroids can be synthesized from diosgenin, which comprises more than 60% of commercial synthetic steroids (242). Diosgenin has shown several biological activities, including anticancer, antidiabetic, anti-infectious, anti-inflammatory, and anticoagulant effects (242). Diosgenin exerted significant antitumor potential via induction of apoptosis and suppression of inflammation. It also inhibited the invasion, metastasis, angiogenesis, and proliferation of various cancer cell lines. Accordingly, targeting inflammation-related pathways, including NF-κB and STAT3, is one of the main anticarcinogenic mechanisms of diosgenin (241, 242). Diosgenin displayed antiproliferative effects in HEp-2 and M4Beu cell lines via enhancing the production and release of apoptosis-inducing factors, increasing the Bax/Bcl-2 ratio, modulating caspase-3, and facilitating the activation of p53 (243). Furthermore, diosgenin induced apoptosis in the colon cancer cell lines HT-29 and HCT-116 in vitro by interfering with COX-2 signaling and increasing DNA fragmentation, caspase-3, and 5-lipoxygenase activity (244). Additionally, diosgenin sensitized colorectal cancer cells to apoptosis induced by TRAIL via activation of the p38MAPK pathway, overexpression of death receptor-5 (DR5), and downregulation of the Akt pathway (245).
Several compounds belonging to the terpenes and terpenoids classes exhibit antineoplastic properties by affecting various stages of tumor development, including suppression of the initiation and progression of tumorigenesis through promoting apoptosis, cell cycle arrest, inhibition of metastasis, angiogenesis, invasion, and downregulation of several intracellular signaling pathways, including TLR4, STAT3, NF-κB, and MMP-9. These compounds are promising therapeutic agents due to the massive progression in delineating the details of their anticancer action. Table 2 provides the various anticancer terpenes/terpenoids that interfere with the TLR/NF-κB/NLRP pathway to counter chemoresistance.
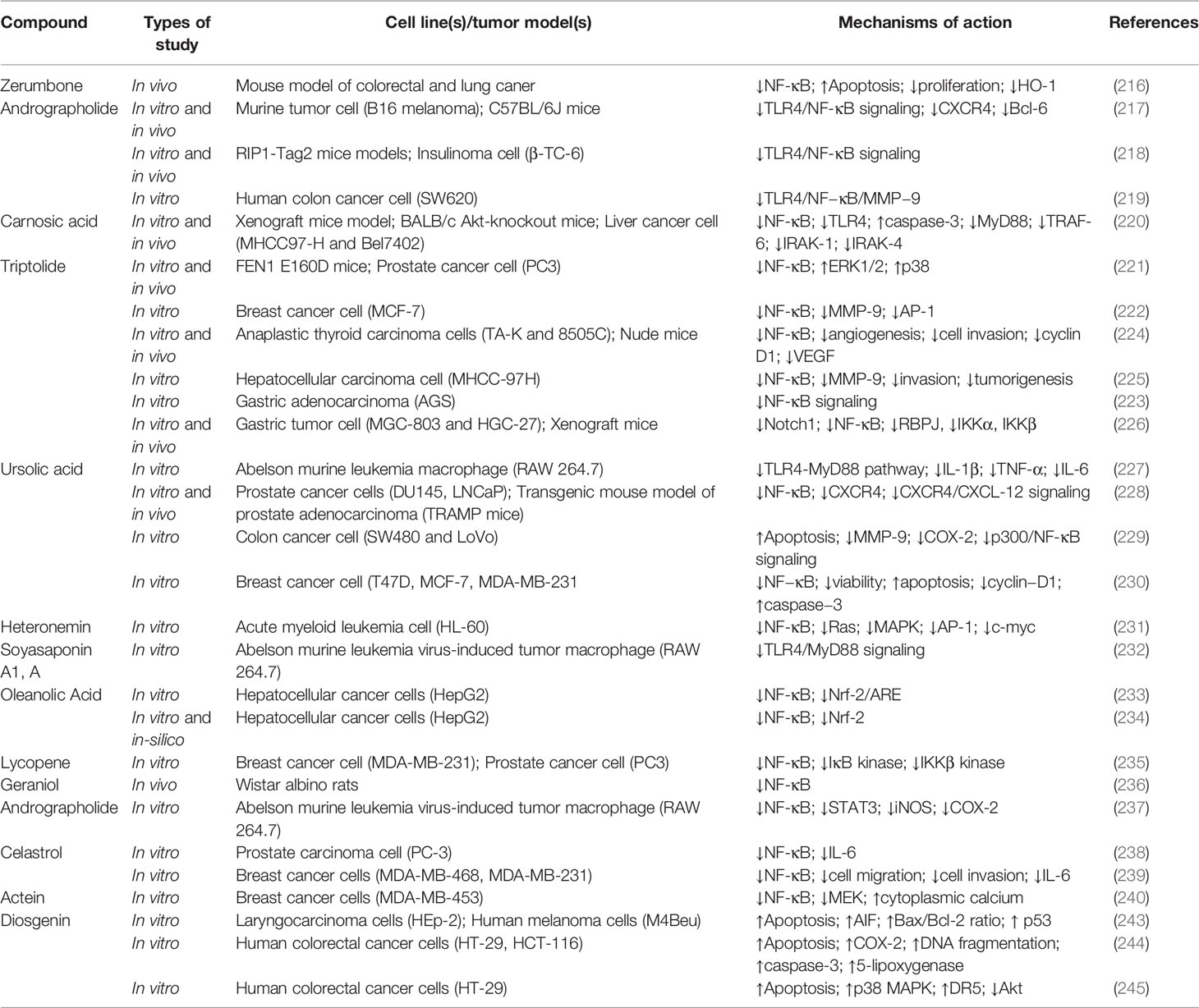
Table 2 Anticancer terpenes/terpenoids interfering with the TLR/NF-κB/NLRP pathway and cross-linked mediators against chemoresistance.
Alkaloids
Alkaloids are another group of plant secondary metabolites that hold a substantial role in the defensive and internal immune processes of plants. Antioxidative, antimicrobial, antiprotozoal, anti-inflammatory, and anticancer activities are some of the important biological activities of alkaloids. Vinblastine and camptothecin are two important compounds belonging to the alkaloid group that have been properly developed and received the Food and Drug Administration’s approval for the treatment of different cancers. Sophoridine (Figure 6) inhibited macrophage-mediated immunosuppression by interfering with the TLR4/IRF-3 pathway, downregulating IL-10, CD206, and arginase 1 (Arg-1), and upregulating IL-12α, IFN-β, and iNOS in RAW264.7 and MFC cell lines (246). Moreover, matrine (Figure 6) demonstrated anticancer activity by regulating immunity, increasing TLR8 and TLR7, and activating MyD88-dependent signaling (247). Additionally, matrine inhibited the invasion and proliferation of breast and prostate cancer cells via downregulation of VEGF/Akt, MMP-2, and MMP-9 through the NF-κB signaling pathways (248, 249). Furthermore, hypaconitine (Figure 6), another alkaloidal agent, inhibited the adhesion, invasion, and migration of the A549 cell line (250). In addition to hypaconitine, alpinetin showed significant anticancer properties, diminishing the transcription of HIF-1α, NF-κB, and the ROS/NF-κB/HIF-1α axis in breast cancer cells (251). In a similar study, berberine (Figure 6), a well-known alkaloid, inhibited the proliferation of lung cancer cells and induced apoptosis through upregulation of Bcl-2/Bax, NF-κB, COX-2, MMP-2, and Akt/ERK pathways (252–254). Likewise, berberine suppressed the NLRP3 inflammasome in MDA-MB-231 cells in vitro (255). Treatment with berberine inhibited MMP-2, MMP-9, NF-κB, focal adhesion kinase (FAK), urokinase-type plasminogen activator (u-PA), and IKK in SCC-4 cancer cells (256). Additionally, berberine prevented DMBA-induced breast carcinogenesis in Sprague Dawley rats (257) and inhibited the growth of MDA-MB-231 cells via decreased IL-6, TNF-α, and NF-κB (258). Furthermore, berberine exerted anticancer activity via targeting variant pathways, such as NF-κB/COX-2, p38/JNK, AP-2/telomerase reverse transcriptase (hTERT), cytochrome-c/caspase, and HIF-1α/VEGF signaling in human gastric and NSCLC cell lines (259, 260). The alkaloid anisodamine (Figure 6) is another anticancer compound that inhibited the growth, invasion, and proliferation of HepG2 cells and suppressed the expression and activation of IFN-γ, IL-27, NLRP3, IL-4, and TNF-α (261). In a similar study, a steroidal alkaloid, cyclopamine (Figure 6), induced apoptosis and suppressed the proliferation of HEL and TF1a cells via induction of PKC, COX-2 overexpression, PARP cleavage, and modulation of MAPK/Akt signaling (262). The main anticancer mechanisms of cepharanthine and tetrandrine (Figure 6) against Jurkat T leukemia cells (263) are the modulation of PI3K/Akt/mTOR signaling, induction of apoptosis, cell cycle arrest, and phosphorylation of JNK and p38. Another alkaloid structure, piperlongumine (Figure 6), appears to have anticancer properties via downregulating c-Met expression and NF-κB activity in renal, colon, lung, and prostate carcinoma cells (264–268). Harmine (269), fangchinoline (270), sinapine (271), gramine (272), cepharanthine (273), piperine (274, 275), lamellarin D (276), ipobscurine (277), chelerythrine (278), dihydrochelerythrine (279), tryptanthrin (280), and neferine (Figure 6) (281) are some of the other alkaloid agents that exert significant anticancer activity through modulation of VEGF, AP-1, fibroblast growth factor receptor 4 (FGFR4)/fibroblast growth factor receptor substrate 2α (FRS2α)-ERK1/2, NF-κB, Nrf-2/Kelch-like ECH-associated protein 1 (Keap-1), MMP-2, MMP-9, and STAT3.
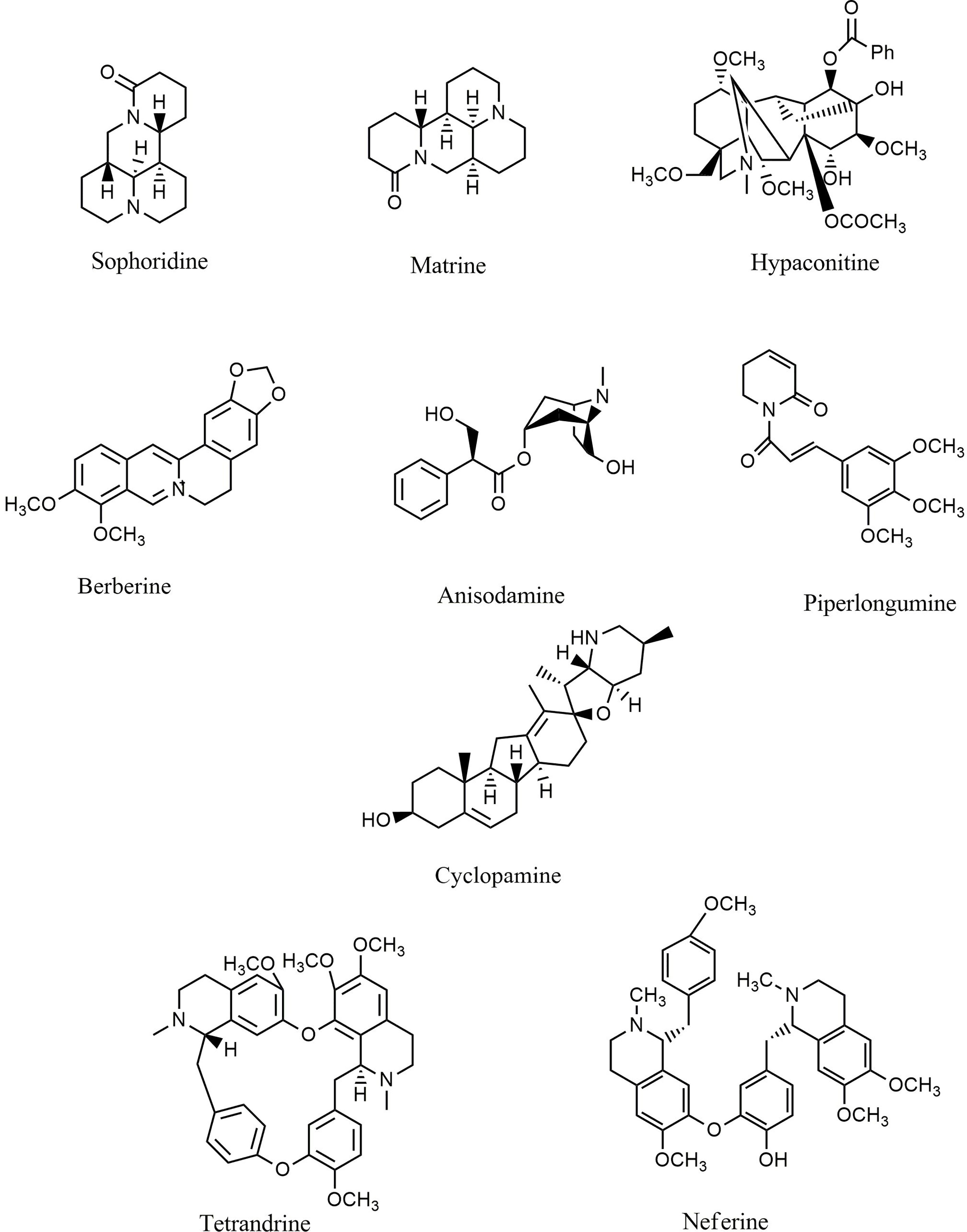
Figure 6 Chemical structures of selected alkaloids that modulate the TLR/NF-κB/NLRP signaling in cancer.
Overall, alkaloids are phytochemicals with significant potential to suppress the in vitro and in vivo growth/invasion of various cancers. By interfering with TLR/NF-κB/NLRP, alkaloids, especially berberine, matrine, and evodiamine, diminish cancer chemoresistance and facilitate the induction of apoptosis, inflammation, oxidative stress, and autophagy in cancer cells. Table 3 provides various anticancer alkaloids that interfere with the TLR/NF-κB/NLRP pathway against chemoresistance.
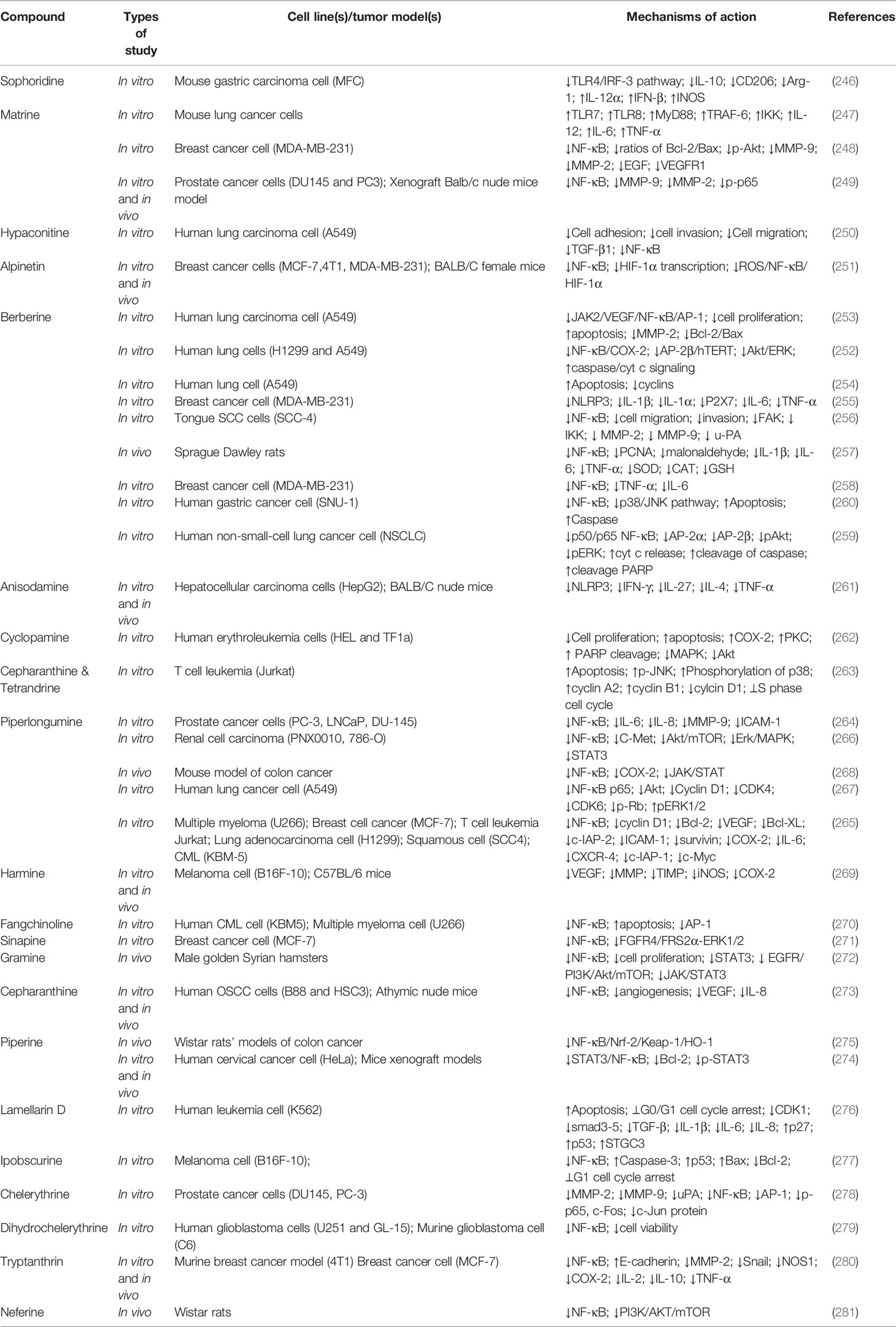
Table 3 Anticancer alkaloids interfering with the TLR/NF-κB/NLRP pathway and interconnected mediators against chemoresistance.
Sulfur-Containing Compounds and Miscellaneous Agents
Sulforaphane (Figure 7) suppressed TLR3-mediated NF-κB in PCI15A SCC cells (282). In addition, sulforaphane inhibited the expression of MMP-9, phosphorylation of IκB, and activation of NF-κB in MCF-7 cells (283). Another sulfur-containing compound, shikonin (Figure 7), appears to have anticancer properties via suppressing the migration, adhesion, viability, and invasion of gastric (MGC-803) and hepatocellular (Huh7and BEL7402) cancer cells in vitro via the TLR2/NF-κB and receptor-interacting protein 1 (RIP1)/NF-κB pathways (284, 285). In a similar study, phenethyl isothiocyanate (Figure 7) in combination with xanthohumol activated Nrf-2 and suppressed NF-κB in pancreatic cancer cells (286) and B-cell acute lymphocytic leukemia (287). Moreover, inhibition of NF-κB and MAPK signaling is the main anticancer mechanism of phenethyl isothiocyanate against AGS cell lines (288). The indolequinazoline alkaloid evodiamine is widely present in many medicinal plants belonging to the tetradium family. Evodiamine (Figure 7) and its derivatives targeted the c-Met, NF-κB, Smad2/3, and TGF-β/hepatocyte growth factor pathways in prostate, hepatocellular, lung, and melanoma carcinoma cells (289–292). It was reported that microsclerodermin A inhibited NF-κB, promoted apoptosis, and diminished cytokine release in pancreatic and breast cancer cells (293, 294). Additionally, treatment of prostate, lung, and pancreatic cancer cells (295–298) with matrine and oxymatrine inhibited angiogenesis, VEGF, NF-κB, and CXCR4. Nobiletin (Figure 7) is a citrus flavonoid with several pharmacological activities, including anticarcinogenic, antioxidative, neuroprotective, and anti-inflammatory effects. Nobiletin modulated the activity of the Cd36/STAT3/NF-κB pathway and inhibited the growth and migration of breast cancer cell lines MCF-7 and MDA-MB-231 (299). Similarly, it leads to the downregulation of Akt, HIF-1α, NF-κB, and VEGF in OVCAR-3 ovarian cancer cells and suppression of TRIF/receptor interacting serine/threonine kinase 1 (RIPK1)/Fas associated via death domain (FADD), TRIF protein, caspase-8, and TLR3/IRF-3 in LNCaP and PC-3 cell lines (300, 301). Additionally, nobiletin diminished the invasion and migration of AGS cells and downregulated FAK/PI3K/Akt, c-Raf, Rac-1, cell division control protein 42 homolog (Cdc42), as well as the NF-κB, MMP-2, and MMP-9 signaling pathways (302).
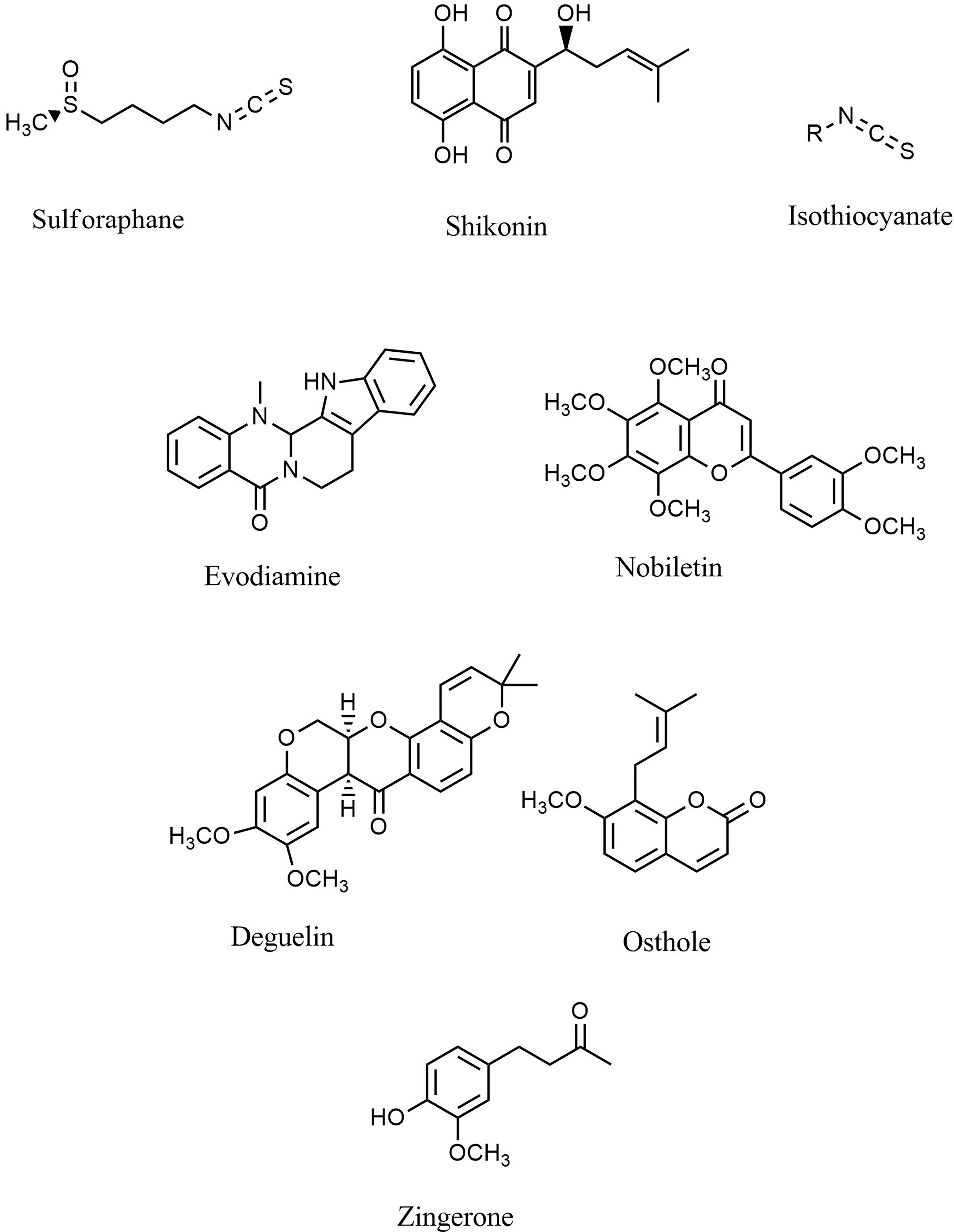
Figure 7 Chemical structures of selected sulfur-containing compounds and miscellaneous agents with effect on TLR/NF-κB/NLRP signaling in cancer.
Ulmus davidiana Nakai glycoprotein (303), phenylpropenone derivatives (304), libanoridin (305), and alisol B 23-acetate (306) decreased the proliferation and migration of colon carcinoma cells via inactivation of inflammatory and angiogenic pathways. Deguelin (Figure 7) downregulated EGFR, c-Myc, pAkt, p-ERK, p-STAT3, c-met, survivin, and NF-κB in xenograft athymic mice models of breast cancer cell lines MDA-MB-231, MDA-MB-468, BT-549, and BT-20 (307). Withaferin A, a steroidal lactone presents in Withania somnifera, exerts several pharmacological activities such as anti-inflammatory, anticancer, and cardioprotective effects. Withaferin A significantly downregulated the liver X receptor-a, NF-κB, and angiogenesis pathways in the hepatocellular carcinoma cell line QGY-7703 (308). Furthermore, withaferin A downregulated caspase-1 and AIM-2 in THP-1 cells (309). Similarly, Zingerone (vanillylacetone) (Figure 7) is another known agent with anticancer properties that inhibited NF-κB, p42/44, and MAPK/AP1signaling and attenuated the migration and invasion of human hepatocellular carcinoma cells (310). Moreover, osthole and ophiopogonin D (Figure 7) suppressed the PI3K/Akt, NF-κB, and AP-1 pathways in A549 and H1299 lung cancer cells (311–314). Embelin (315), plumbagin (316, 317), indole glucosinolates (318), thymoquinone (319, 320), decursinol angelate (321), polysaccharide agaricus blazei murill (322), and 19-a-hydroxyurs-12(13)-ene-28-oic acid-3-O-b-D-glucopyranoside (HEG) (323) are some of the other miscellaneous agents that have promising anticancer potential against MDAMB-231, pancreatic PANC1, Ehrlich ascites carcinoma, fibrosarcoma HT1080, and chronic myeloid leukemia (CML) KBM-5 cancer cell lines in vitro and in vivo.
Overall, sulfur-containing compounds demonstrate critical biological properties and therefore have meaningful potential for the prevention and treatment of cancers. These phytochemicals could target multiple signals affecting cancer progression, especially TLR/NF-κB/NLRP signaling and cross-talked pathways, to support cancer immunotherapy and chemotherapy. Table 4 provides the various anticancer sulfur and miscellaneous compounds in interfering with the TLR/NF-κB/NLRP pathway against chemoresistance.
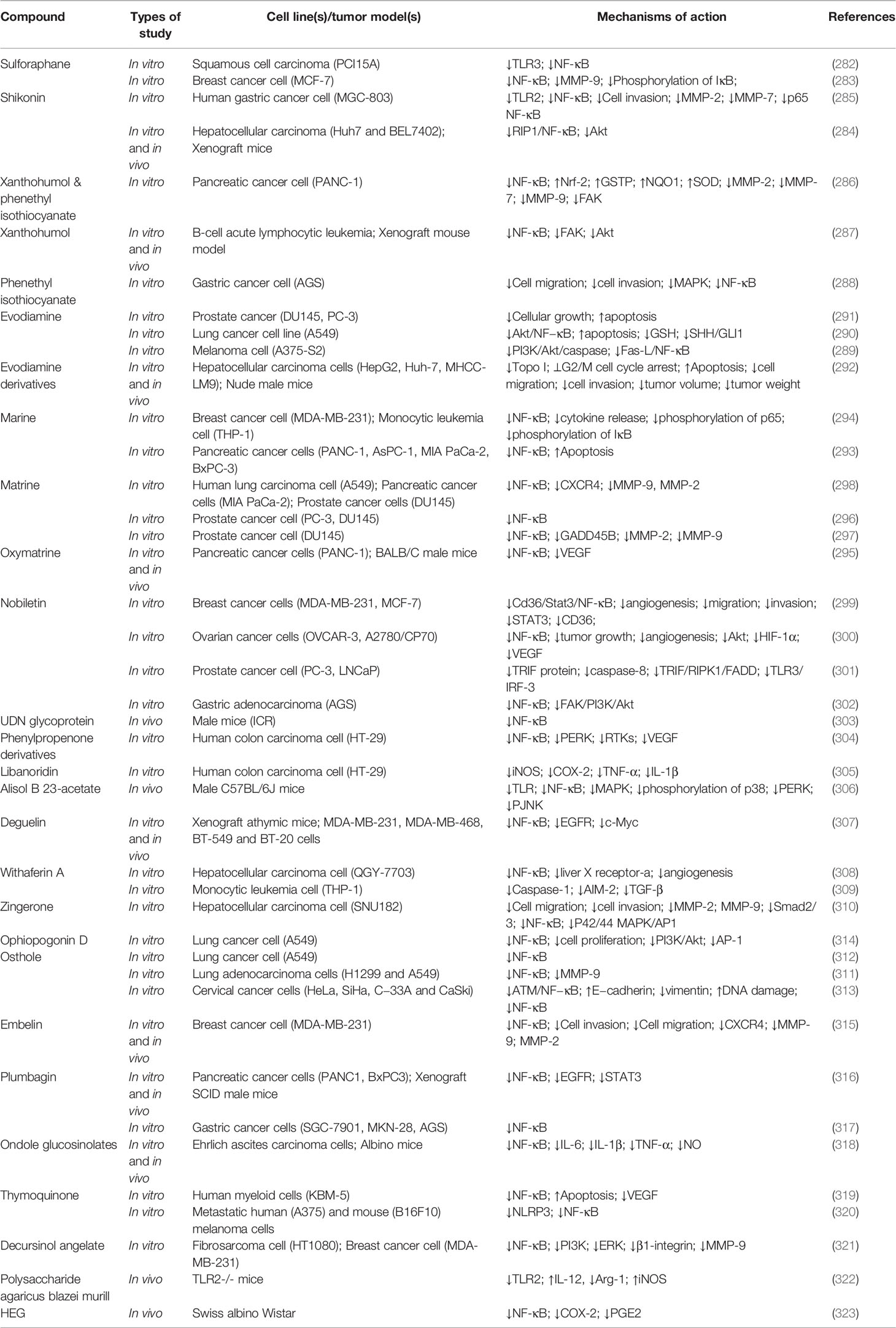
Table 4 Anticancer sulfur compounds and miscellaneous agents interfering with the TLR/NF-κB/NLRP pathway and interconnected mediators against chemoresistance.
Phytochemicals in Combination With Anticancer Drugs Potentiate Chemotherapy and Immunotherapy
Despite the recent progress in designing, synthesizing, and introducing new pharmaceutical drugs, natural products and bioactive molecules isolated from plants exert undeniable roles in the treatment of various cancers. Currently, the most important treatment option for malignant tumors is chemotherapy, which may lead to numerous side effects and promote drug resistance in patients. The use of natural products for the treatment of cancer is not only economically efficient, but also exerts multiple prophylactic, protective, and therapeutic roles in the treatment process that may reduce the side effects of chemotherapy and radiotherapy and decrease drug resistance (6, 13, 324–326).
Numerous studies have investigated the in vitro and in vivo advantages of adding curcumin to various anticancer treatment regimens. Liposomal curcumin has sensitized mouse models of cervical cancer to paclitaxel treatment (327). Curcumin also facilitated the induction of cell death by paclitaxel in MCF7 and MDA-MB-234 cell lines (328, 329). It was reported that curcumin induced cell apoptosis and increased paclitaxel sensitivity in cervical cancer cells through interfering with NF-κB, p53, and caspase-3 signaling (330). In similar studies, curcumin significantly sensitized breast cancer cells to cyclophosphamide and paclitaxel through modulation of NF-κB, protein kinase C (PKC), histone deacetylase (HDAC), and telomerase (331). Combining curcumin with oxaliplatin reversed the acquired resistance in an in vitro model of colorectal cancer by interfering with the CXC-chemokine/NF-κB pathway (332). In addition, curcumin increased the chemosensitivity of several platinum-based drugs via arresting the cell cycle in the G2/M phase, inducing apoptosis, and downregulating NF-κB (333). Similarly, co-delivery of curcumin and dasatinib led to the enhanced antitumor activity of dasatinib against colon cancer cells via diminished insulin-like growth factor type 1 receptor, c-Src, and EGFR signaling (334). Moreover, curcumin worked synergistically with tamoxifen to suppress the growth of MCF-7/LCC9 and MCF-7/LCC2 cells in an in vitro model of breast cancer by facilitating cell cycle arrest and inactivating the Akt/mTOR, Src, and NF-κB pathways (335). Furthermore, curcumin sensitized MDA-MB-231 cells to retinoic acid (336) and enhanced the efficacy, as well as diminished the toxicity, of doxorubicin (337). The anticancer potential of sorafenib against Huh7 cells and an athymic mice model of hepatocellular carcinoma was enhanced when combined with curcumin as evident by decreased expression of MMP-9 and NF-κB/p65 (338). Another well-known polyphenolic compound, resveratrol, sensitized PC3 and DU145 prostate cancer cells in vitro to cisplatin-induced apoptosis via inhibition of COX-2 and NF-κB pathways (339). In a similar study, resveratrol significantly increased the efficacy of cisplatin in xenografted mice and MDA-MB-231 cancer cells via decreased activity of p-ERK, TGF-β1, Smad2, vimentin, p-Akt, NF-κB, p-PI3K, and p-JNK (340). Furthermore, resveratrol sensitized colorectal cancer cells to 5-fluorouracil (5-FU) by inducing apoptosis and downregulating NF-κB (341). Additionally, resveratrol improved the gemcitabine-induced apoptosis of PaCa cells via inhibition of NF-κB and diminished expression of cyclin D1, VEGF, intercellular adhesion molecule-1 (ICAM-1), COX-2, and MMP-9 (342). Apigenin is another polyphenolic substance that potentiated the antitumor activity of several antineoplastic agents, including paclitaxel (343), tamoxifen (344), gemcitabine (345, 346), doxorubicin (347), and cisplatin (348) against various in vitro and in vivo cancer models. Polyphenol quercetin is another anticancer compound that works synergistically with paclitaxel (349, 350), tamoxifen (351), cisplatin (352, 353), adriamycin (354), and gemcitabine (355) to suppress the growth of various models of cancer via enhanced ROS production, cell cycle arrest, ER stress, and apoptosis. Similarly, various studies have reported the advantages of adding EGCG to enhance the antitumor activity of sunitinib, irinotecan, doxorubicin, gemcitabine, and cisplatin against human lung (A549, H460, and H1975) (356, 357), colorectal (HCT116 and RKO) (358), bladder (SW780 and T24) (359), pancreatic (MIA PaCa-2 and Panc-1) (360), and ovarian (OVCAR3 and SKOV3) (361) cancer cells, respectively. The results emphasized that EGCG could potentate the antineoplastic activity of the aforementioned drugs by increasing the sensitivity of the cancer cells, thereby enhancing their antiproliferative activity, damaging DNA, interfering with the NF-κB/MDM2/p53 pathway, inhibiting Akt, and elevating copper transporter 1 (CTR1). Likewise, cotreatment of naringin with doxorubicin (362) and paclitaxel (363) amplified their anticancer activity against human esophageal and prostate cancer cells, respectively. Moreover, baicalin improved the chemosensitivity to cisplatin (364) and doxorubicin (365) in lung and breast cancer cells, respectively, by inducing cell cycle arrest, apoptosis, and DNA damage. Furthermore, arctigenin sensitized various cancer cell lines, including SW620, HepG2, H460, HeLa, SW480, and K562, to cisplatin treatment (366–368). Morin (369), chrysin (370), and pterostilbene (371) are some of the other polyphenolic compounds that increased the cytotoxicity of antineoplastic agents in several in vitro models of cancer.
In addition to polyphenols, terpenes also showed a significant ability to increase the sensitivity of different cancer cell lines to various drugs, such as 5-FU, gefitinib, cisplatin, doxorubicin, and gemcitabine. Combination therapy of cisplatin and paclitaxel with zerumbone, a sesquiterpene agent, enhanced ROS production and p53 expression, as well as inhibited the JAK2/STAT3 pathway in prostate and lung cancer cells (372, 373). It was reported that andrographolide augmented the doxorubicin-mediated antitumor activity in different cancer cells through the blockade of JAK/STAT3 signaling (374, 375) and its coadministration with gemcitabine promoted apoptosis and inhibited STAT3 in pancreatic cancer cells (376). Furthermore, treatment with andrographolide increased cisplatin-induced antineoplastic activity against lung cancer cells (377). Carnosic acid is another triterpenoid compound that, in combination with cisplatin and tamoxifen, promoted apoptosis in lung (378) and breast (379) cancer cells. In addition to carnosic acid, triptolide showed significant anticancer properties and amplified the in vitro and in vivo anticancer activity of various chemotherapeutic agents, including cisplatin (380–382), paclitaxel (383), hydroxycamptothecin (384, 385), gemcitabine (386), and doxorubicin (387), in several cancer types, including bladder (EJ, UMUC3, and T24R2), breast (MDA-MB-231, BT549, and MCF7), lung (A549), and gastric (SC-M1) cancer cell lines. Another triterpenoid compound, ursolic acid, potentiated the therapeutic effects of gemcitabine, oxaliplatin, cisplatin, and paclitaxel in human pancreatic and colorectal cancer cells by promoting apoptosis and inhibiting the inflammatory microenvironment and NF-κB p65 signaling (388–391). Moreover, treatment with oridonin overcame antibiotic resistance and augmented the antineoplastic effects of doxorubicin, cisplatin, and gemcitabine via increased expression of Bax, induction of apoptosis, downregulation of Bcl−2, and inhibition of MMP in the in vivo and in vitro models of lung, breast, pancreatic, and ovarian cancers (392–395). In a similar study, ginsenoside Rg3 enhanced the cytotoxicity of paclitaxel in breast cancer cells by regulating the expression of Bax/Bcl-2 and suppressing NF-κB signaling (396). Additionally, ginsenoside Rg3 amplified cisplatin therapy in the lung cancer cell lines H1299, SPC-A1, and A549 by inhibiting the NF-κB pathway (397, 398). Another study found that co-administration of ginsenoside Rg3 and gefitinib increased the cytotoxicity of gefitinib against lung cancer in vitro (399). The results demonstrated that the main mechanisms by which ginsenoside Ro enhances the anti-malignant effects of 5-FU occurs by accumulating DNA damage, inhibiting DNA repair, downregulating DNA replication, and delaying the degradation of checkpoint kinase 1 (CHEK1) (400). The treatment combination of docetaxel and ginsenoside Rg3 increased activation of the apoptotic pathway in colon and prostate cancer cells via suppression of NF-κB (401, 402). Lycopene, a carotenoid agent, improved cisplatin-induced apoptosis in HeLa cancer cells via inhibition of NF-κB activation (403).
Alkaloids, like other secondary metabolites, enhance the in vitro and in vivo anticancer activities of various drugs by elevating their antiproliferative effects and promoting apoptosis and cell cycle arrest. The combined effects of doxorubicin and berberine on lung (404) and breast (405, 406) cancer cells inhibited the STAT3, high mobility group box 1 (HMGB1)-TLR4 axis and downregulated the expression of Nanog and miRNA-21. Cisplatin and berberine combined therapy also inhibited cell growth, promoted apoptosis, created DNA breaks, and interfered with miR-93/PTEN/Akt signaling in MCF-7 and A2780 cells (407, 408). Moreover, berberine increased the chemotherapy potential of irinotecan against in vitro models of colon cancer through the suppression of NF-κB (409). Another alkaloid compound, piperlongumine, induced apoptosis and potentiated the anticarcinogenic activity of doxorubicin, paclitaxel, oxaliplatin, cisplatin, and gemcitabine via suppression of the JAK2/STAT3 pathway and induction of oxidative stress in breast, intestinal, gastric, HNSCC, colorectal, and pancreatic cancer cells (410–415). In a similar study, harmine, in combination with paclitaxel, suppressed the invasion and migration of SGC-7901 and MKN-45 gastric cancer cell lines via downregulation of MMP-9 and COX-2 (416, 417). In addition, harmine suppressed the proliferation of pancreatic cancer cells in vitro by enhancing the cytotoxicity of gemcitabine (418). Likewise, several studies have reported the advantages of combining matrine with irinotecan and cisplatin to augment their antitumor activity against human colorectal (HT29) (419), urothelial bladder (EJ and T24) (420), liver (HepG2) (421), and cervical (U14) (422) cancer cell lines. The results suggested that matrine significantly potentiated the antineoplastic effects of both irinotecan and cisplatin and increased the sensitivity of the aforementioned cancer cells to treatment through induction of apoptosis, facilitation of cell cycle arrest, and enhanced activity of topoisomerase I, ROS, β−catenin, Bax, caspase-3, caspase-7, and caspase-9. Treatment with sophoridine inhibited the growth of lung cancer cells by amplifying cisplatin sensitivity via activation of Hippo and p53 signaling (423).
Sulforaphane could significantly sensitize human breast, lung, colorectal, and bladder cancer cells to variant chemotherapeutic agents via downregulation of NF-κB, induction of cell cycle arrest, and reduction of cyclin A and p-Akt (424–427). Furthermore, sulforaphane increased the in vitro and in vivo antiproliferative activity of salinomycin in colorectal cancer cells via diminished signaling of the PI3K/Akt pathway (428). Moreover, shikonin reversed gemcitabine tolerance in a xenograft model of pancreatic cancer via modulation of the NF-κB signaling pathway (429). Treatment with shikonin potentiated the antitumor efficacy of gefitinib in lung cancer cells through suppression of the PKM2/STAT3/cyclin D1 pathway (430). Shikonin also increased the sensitization of paclitaxel against esophageal cancer cells by promoting apoptosis (431). Additionally, shikonin enhanced 4-hydroxytamoxifen-induced apoptosis in breast cancer cells by activating mechanisms involved in apoptosis and its related signaling pathways (432). It was reported that co-treatment of breast cancer cells with phenethyl isothiocyanate and paclitaxel induced apoptosis, arrested the cell cycle, and inhibited cell growth (433, 434). Garcinol induced the death of the breast cancer cell lines MCF7, MDAMB231, and SKBR3 via triggering p53-dependent upregulation of Bax and downregulation of Bcl-xL (435). It also potentiated cisplatin sensitivity in HNSCC (436) and ovarian (437) cancer cells, as well as enhanced paclitaxel sensitivity in breast cancer cells (438) via inhibition of survivin, NF-κB/Twist-related protein 1 (Twist1), VEGF, caspase-3/calcium-independent phospholipase A2 (iPLA2), cyclin D1, Bcl-2, and PI3K/Akt signaling.
Hispidin (439), genistein (440, 441), guggulsterone (442), ginkgolide B (443), icariin (444), and zyflamend (445) potentiated the antineoplastic activity of gemcitabine in several cancer types, including pancreatic, osteosarcoma, and gallbladder cancers. Cisplatin in combination with tangeretin (446), galangin (447), and cepharanthine (448) decreased the proliferation and invasion of esophageal, lung, and ovarian cancer cells. Cotreatment of paclitaxel with icariside II (449) and caffeic acid (450), as well as combined treatment of 5-FU with oxymatrine (451), troxerutin (452), and calebin (453) increased the sensitivity of colon, lung, and melanoma cancer cells. Parthenolide (454) elevated oxaliplatin toxicity in A549 cells. The anticancer activity of doxorubicin was enhanced when combined with forbesione and isomorellin (455). Dioscin potentiated the effects of adriamycin in the K562 leukemia cell line (456).
In summary, phytochemicals have the potential to increase the sensitivity of various cancer cells and animal tumor models to several anticancer drugs. Phytochemicals augment chemoresistance through interfering with many processes, such as cell cycle arrest, DNA damage, angiogenesis, and variant signaling pathways, especially TLR/NF-κB/NLRP (Table 5).

Table 5 Phytochemicals in combination with anticancer drugs potentiate chemotherapy and immunotherapy: focusing on TLR/NF-κB/NLRP pathway.
Nanoformulations of Phytochemicals Against Chemoresistance and Immunotherapy Resistance
Mutations and long-term chemotherapy lead to the development of chemoresistance, prompting the need for progressively increasing dosages of anticancer drugs. Consequently, these higher concentrations of chemotherapeutic agents are toxic to noncancerous cells (457). Nanoparticles are increasingly used due to their improved bioavailability, protection of drug molecules, high specificity for cancer cells, and decreased clearance. Combining the versatile capabilities of nanoparticles with the aforementioned benefits of phytochemicals created the concept of phytonanomedicine, which revolutionized cancer therapy (458). Phytonanomedicine utilizes the valuable properties of phytochemicals merged with the nano-size, high surface area, optical activity, and surface reactivity of nanoparticles to achieve active or passive tissue-specific drug delivery. The application of phytonanocompounds may reduce the toxicity and side effects of chemotherapeutic agents, while increasing their efficacy, thereby combating chemoresistance (459).
Flavones are the most prominent natural phytochemical that regulates the functions of Bax, Bid, and Bak proteins. Additional mechanisms by which phytochemicals combat chemoresistance are altering the expression of selected genes during mitosis or meiosis, regulating the expression of mutated genes like p21 and p53, and interfering with DNA repair mechanisms. Liposomes, polymeric nanoparticles, polymeric micelles, nanodispersion, and dendrimers, among others, are efficient nanocarriers that are often used (460).
Quercetin-loaded mesoporous silica decorated with chondroitin sulfate potentiated the delivery of paclitaxel, overcoming MDR in breast cancer cells. Such co-administration successfully targeted CD44 receptor-mediated targeting with a low half-maximal inhibitory concentration (IC50) value by increasing cell cycle arrest in the G2/M phase and destroying microtubules. Quercetin also decreased paclitaxel efflux by downregulating the expression of P-gp (349). In another study, Zafar et al. (461) used phosphorylated chitosan nanoparticles loaded with α-lipoic acid to overcome chemotherapy resistance. The nanodelivery system was able to cross the cell barrier and expose the MDA-MB-231 cells to a high load of the therapeutic agent. Similar nanoparticles were also used for loading quercetin and paclitaxel simultaneously (462). The efficacy of curcumin and temozolomide co-delivery to chemoresistant cells was highlighted by Bagherian et al. (458). In this study, the curcumin nanomicelles demonstrated high cytotoxic effects against U87 cells by attenuating apoptotic and autophagic mediators. Zafar et al. (461) also used a phytochemical, thymoquinone, to prevent chemoresistance to docetaxel (e.g., endosomes escape). The neoplastic agent was loaded in chitosan nanoparticles and exhibited cytotoxicity against MCF-7 and MDA-MAB-231 cancer cell lines. Recently, a functionalized dendrimer has also been employed to co-deliver siRNA and curcumin to target HeLa cancer cells. Such co-administration increased the cytotoxicity against cancer cells by reducing Bcl-2 and improving apoptosis (463). Baicalein nanoparticles targeted folate and hyaluronic acid to display anticancer effects on paclitaxel-resistant lung cancer cells by decreasing tumor growth and cell viability (464). The nanosuspension of another flavonoid, chrysin, showed anticancer effects against HepG2 cells by blocking cell growth (465). The phytosome of luteolin, another flavonoid, exhibited anticancer activity against MDA-MB-231 cells by reducing the expression of Nrf-2/HO-1 and decreasing cell viability (466). Poly-lactic acid (PLA)-polyethylene glycol (PEG) nanoformulation of luteolin also demonstrated anticancer effects against TU212 HNSCC cells, H292 lung cancer cells, and a xenograft mouse model of head and neck cancer (467).
Nanostructured lipid carriers were also used for dual drug loading of imatinib and curcumin to target the CD20 receptor of lymphoma cells. This co-delivery system displayed promising responses in resistant tumor cells (468). By regulating oxidative stress and apoptosis, phyto-nanocomposites diffuse into the organelles of cancer cells and overcome chemoresistance through multiple signaling pathways, including TLR/NF-κB/NLRP, ERK/MAPK/JNK, stress-activated protein kinase/JNK, TRAIL, PI3K/Akt, and p53/caspase mediated apoptotic pathways (469).
Poly(lactic-co-glycolic acid nanoparticles of 4-methyl-7-hydroxy coumarin (a synthetic coumarin) and dendrosomal nanoformulation of farnesiferol c (a coumarin) demonstrated anticancer effects by decreasing cell proliferation in gastric cancer cells and by modulating the Bax/Bcl-2 ratio (470). Other phytochemicals, such as flavonolignans PEG nanoliposomes, silibinin, and glycyrrhizic acid, demonstrated anticancer effects by decreasing cell viability in HepG2 cells (471).
A nanoformulation of honokiol, a lignan, showed anticancer effects in lung cancer cell lines by inducing cell cycle arrest in the G0/G1 phase (459). Silver nanoparticles of plumbagin, a naphthoquinone, induced apoptosis in the human skin cancer cells HaCaT and A431, producing free radicals and increasing pyruvate kinase activity (472). Liposomal phytochemicals may possess anticancer effects in intravenous usage. The liposomal carrier significantly increased plasma levels of curcumin in a dose-dependent manner in cancer patients. In one clinical study, liposomal curcumin showed acceptable plasma concentration with a significant but temporary decrease in tumor markers such as prostate-specific antigen and carcinoembryonic antigen, in patients with metastatic tumors. Additionally, liposomal curcumin inhibited sphingosine kinase (473). Lipocurcumin is known to be a safe drug that significantly suppresses cancer markers via apoptosis and cell cycle arrest. The curcumin nanoparticle suppressed STAT3/NF-κB, thereby inducing apoptosis. Accordingly, the phase Ib/IIa clinical trial showed no drug-related severe toxicity while maintaining an inhibitory effect on cancer resistance. Such nanoparticles could target cancer-specific mediators in solid tumors (473).
The hyaluronic acid-modified PEGylated liposomes of doxorubicin-stigmasterol were used against chemoresistant breast cancer (474). Gold nanoparticles have shown promising potential in both cancer chemotherapy and immunotherapy (475). Resveratrol gold nanoparticles improved antitumor activity through mitochondrial accumulation and apoptosis both in vitro and in vivo. Gold nanoresveratrol also elevated the expression of caspase-8 and Bax, while reducing pro-caspase-3 and pro-caspase-9. Ginseng-derived nanoparticles are another phytonanocompound that induced M2 to M1 macrophage polarization through TLR4 and MyD88 signaling. It also produced ROS and increased apoptosis of melanoma cells (476).
Various nanoparticles may represent a novel class of nano-targeted systems in cancer immunotherapy and chemotherapy (Table 6).
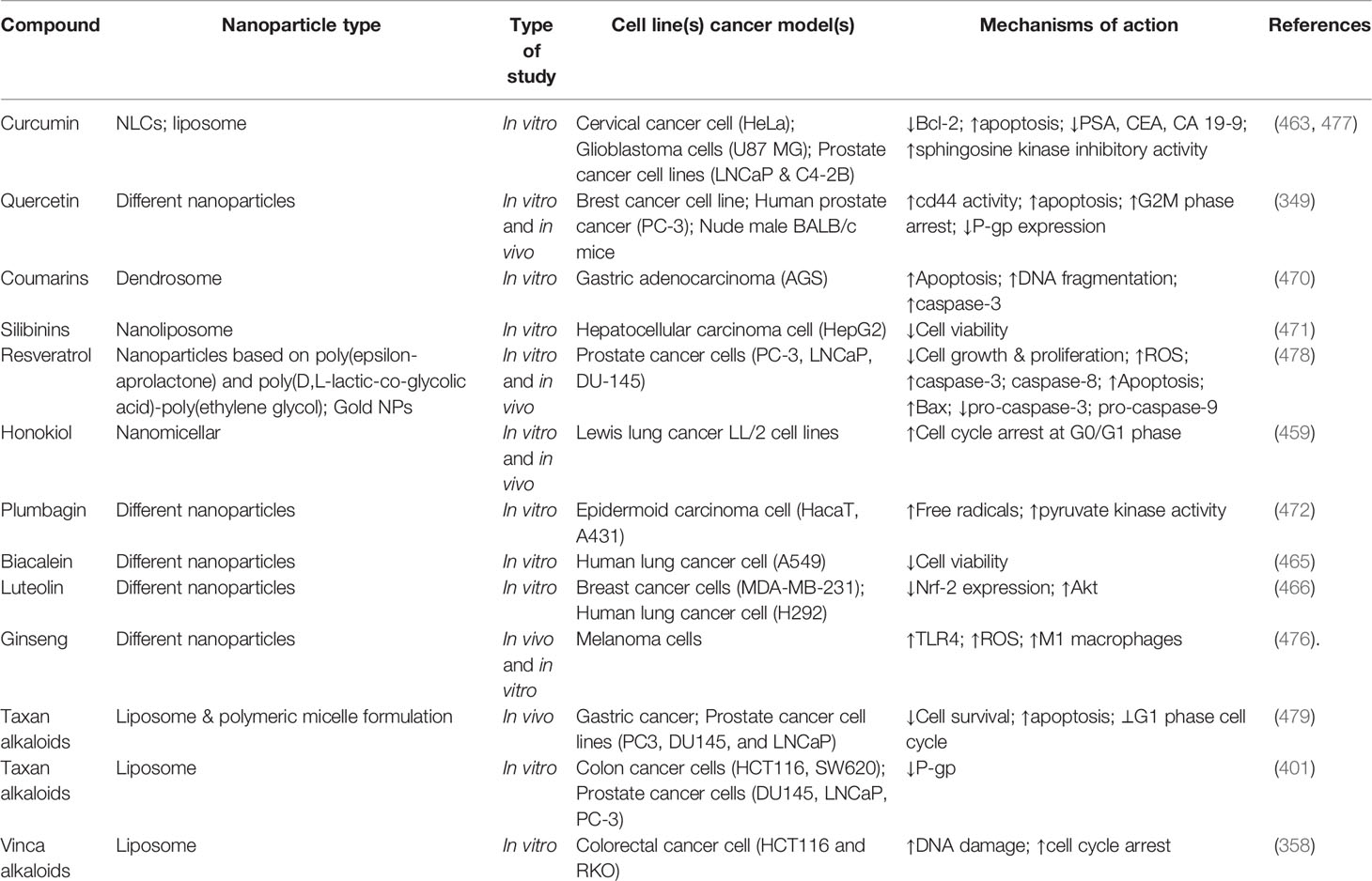
Table 6 Phytonanocompounds against chemoresistance, by targeting TLR/NF-κB/NLRP pathway and interconnected mediators.
Conclusion, Current Limitations, and Future Perspectives
Agrowing number of reports have highlighted the difficulty of chemoresistance when administering chemotherapy and immunotherapy. Several complex pathophysiological mechanisms behind chemoresistance have been discovered. Of those mechanisms, TLR/NF-κB/NLRP has been identified as a crucial aspect in the development of chemoresistance. The TLR/NF-κB/NLRP pathway is interconnected with several inflammatory, oxidative stress, and apoptotic mediators. Thus, there is an urgent need to develop novel multi-targeted agents to overcome drug resistance and restore the sensitivity of current chemotherapeutic drugs. Plant secondary metabolites (e.g., polyphenols, alkaloids, terpenes/terpenoids, and sulfur compounds) are potential multi-targeted anticancer agents that may combat chemoresistant dysregulated mediators. We have also reviewed the potential of phytochemicals in the modulation of the tumor microenvironment (13, 33, 480). Despite the effectiveness of plant secondary metabolites in regulating chemoresistance-associated pathways, their low solubility, poor bioavailability, instability, and low selectivity limit clinical efficacy and therapeutic uses in cancer treatment. The applicability of nanotechnology has greatly improved bioavailability, cellular uptake, efficacy, and specificity of anticancer phytochemicals, thereby overcome those pharmacokinetic limitations (6, 13, 457, 481). In line with this, liposomes, polymeric nanoparticles, micelles, nanodispersion, nanostructured lipid carriers, and dendrimers of plant secondary metabolites have played pivotal roles in reducing chemoresistance (482).
In this systematic and comprehensive review, the critical roles of phytochemicals have been highlighted in the modulation of TLR/NF-κB/NLRP to combat chemoresistance. The need to develop novel delivery systems of plant secondary metabolites and targeted therapy is also highlighted. Further research should be performed involving additional dysregulated mechanisms in chemoresistance which may reshape therapeutic approaches to utilize plant-derived secondary metabolites (Figure 8). Additional studies should consist of extensive in vitro and in vivo experimentation to further unveil emergent chemoresistance signaling pathways, as well as well-controlled clinical trials. Such reports may reform current therapeutic strategies, allowing treatment methods to obtain higher potency/efficacy with fewer side effects and less chemoresistance by using phytochemicals that target the TLR/NF-κB/NLRP signaling pathway.
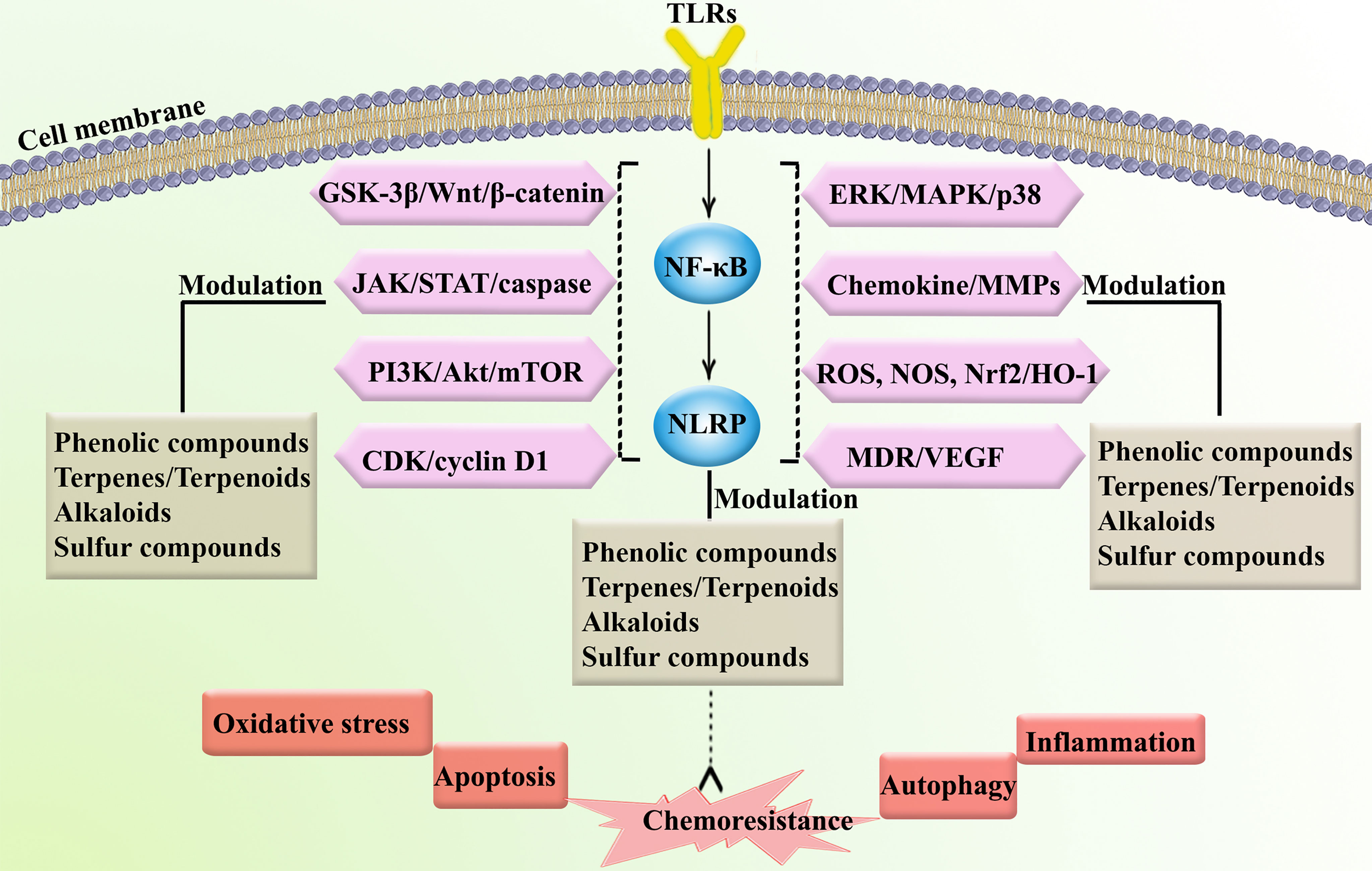
Figure 8 Targeting chemoresistance-related signaling pathways and interconnected mediators by phytochemicals. CDK, cyclin-dependent kinase; ERK, extracellular-regulated kinase; GSK-3, glycogen synthase kinase-3; HO-1, heme oxygenase 1; JAK, Janus kinase; NOS, nitric oxide synthase; MAPK, mitogen-activated protein kinase; MDR, multidrug resistance; MMP; matrix-metalloproteinase; mTOR, mammalian target of rapamycin; NLRP, nod-like receptor pyrin domain-containing; NOS, nitric oxide synthase; Nrf-2, nuclear factor-erythroid factor 2-related factor 2; PI3K, phosphoinositide 3-kinases; ROS, reactive oxygen species; STAT, signal transducer and activator of transcription; VEGF, vascular endothelial growth factor.
Data Availability Statement
The original contributions presented in this study are included in the article/supplementary material. Further inquiries can be directed to the corresponding author.
Author Contributions
Conceptualization, AB and SF. Literature search and collection: SF, SM, AY, and FN. Writing-original draft: SF, SM, AY, and FN. Writing-review and editing, SF, CW, and AB. Supervision: AB. Project administration: AB. All authors contributed to the article and approved the submitted version.
Conflict of Interest
The authors declare that the research was conducted in the absence of any commercial or financial relationships that could be construed as a potential conflict of interest.
Publisher’s Note
All claims expressed in this article are solely those of the authors and do not necessarily represent those of their affiliated organizations, or those of the publisher, the editors and the reviewers. Any product that may be evaluated in this article, or claim that may be made by its manufacturer, is not guaranteed or endorsed by the publisher.
References
1. Zheng H-C. The Molecular Mechanisms of Chemoresistance in Cancers. Oncotarget (2017) 8(35):59950. doi: 10.18632/oncotarget.19048
2. Ji X, Lu Y, Tian H, Meng X, Wei M, Cho WC. Chemoresistance Mechanisms of Breast Cancer and Their Countermeasures. Biomed Pharmacother (2019) 114:108800. doi: 10.1016/j.biopha.2019.108800
3. Cooke SL, Brenton JD. Evolution of Platinum Resistance in High-Grade Serous Ovarian Cancer. Lancet Oncol (2011) 12(12):1169–74. doi: 10.1016/S1470-2045(11)70123-1
4. Ntoufa S, Vilia MG, Stamatopoulos K, Ghia P, Muzio M. Toll-Like Receptors Signaling: A Complex Network for NF-κb Activation in B-Cell Lymphoid Malignancies. Semin Cancer Biol (2016) 39:15–25. doi: 10.1016/j.semcancer.2016.07.001
5. Li Q, Yang G, Feng M, Zheng S, Cao Z, Qiu J, et al. NF-κb in Pancreatic Cancer: Its Key Role in Chemoresistance. Cancer Lett (2018) 421:127–34. doi: 10.1016/j.canlet.2018.02.011
6. Fakhri S, Moradi SZ, Ash-Rafzadeh A, Bishayee A. Targeting Cellular Senescence in Cancer by Plant Secondary Metabolites: A Systematic Review. Pharmacol Res (2021):105961. doi: 10.1016/j.phrs.2021.105961
7. Moloudizargari M, Asghari MH, Nabavi SF, Gulei D, Berindan-Neagoe I, Bishayee A, et al. Targeting Hippo Signaling Pathway by Phytochemicals in Cancer Therapy. Semin Cancer Biol (2020). doi: 10.1016/j.semcancer.2020.05.005
8. Tewari D, Bawari S, Sharma S, DeLiberto LK, Bishayee A. Targeting the Crosstalk Between Canonical Wnt/β-Catenin and Inflammatory Signaling Cascades: A Novel Strategy for Cancer Prevention and Therapy. Pharmacol Ther (2021) 227:107876. doi: 10.1016/j.pharmthera.2021.107876
9. Bose S, Banerjee S, Mondal A, Chakraborty U, Pumarol J, Croley CR, et al. Targeting the JAK/STAT Signaling Pathway Using Phytocompounds for Cancer Prevention and Therapy. Cells (2020) 9(6):1451. doi: 10.3390/cells9061451
10. Tewari D, Patni P, Bishayee A, Sah AN, Bishayee A. Natural Products Targeting the PI3K-Akt-mTOR Signaling Pathway in Cancer: A Novel Therapeutic Strategy. Semin Cancer Biol (2019). doi: 10.1016/j.semcancer.2019.12.008
11. Yun BD, Son SW, Choi SY, Kuh HJ, Oh T-J, Park JK. Anti-Cancer Activity of Phytochemicals Targeting Hypoxia-Inducible Factor-1 Alpha. Int J Mol Sci (2021) 22(18):9819. doi: 10.3390/ijms22189819
12. Tewari D, Nabavi SF, Nabavi SM, Sureda A, Farooqi AA, Atanasov AG, et al. Targeting Activator Protein 1 Signaling Pathway by Bioactive Natural Agents: Possible Therapeutic Strategy for Cancer Prevention and Intervention. Pharmacol Res (2018) 128:366–75. doi: 10.1016/j.phrs.2017.09.014
13. Fakhri S, Moradi SZ, Farzaei MH, Bishayee A. Modulation of Dysregulated Cancer Metabolism by Plant Secondary Metabolites: A Mechanistic Review. Semin Cancer Biol (2020). doi: 10.1016/j.semcancer.2020.02.007
14. Marin JJ, Lozano E, Herraez E, Asensio M, Di Giacomo S, Romero MR, et al. Chemoresistance and Chemosensitization in Cholangiocarcinoma. Biochim Biophys Acta (BBA)-Mol Basis Dis (2018) 1864(4):1444–53. doi: 10.1016/j.bbadis.2017.06.005
15. Qiu J, Zheng Q, Meng X. Hyperglycemia and Chemoresistance in Breast Cancer: From Cellular Mechanisms to Treatment Response. Front Oncol (2021) 11:51. doi: 10.3389/fonc.2021.628359
16. Chen C-Y, Kao C-L, Liu C-M. The Cancer Prevention, Anti-Inflammatory and Anti-Oxidation of Bioactive Phytochemicals Targeting the TLR4 Signaling Pathway. Int J Mol Sci (2018) 19(9):2729. doi: 10.3390/ijms19092729
17. Chauhan A, Islam A, Prakash H, Singh S. Phytochemicals Targeting NF-κb Signaling: Potential Anti-Cancer Interventions. J Pharm Anal (2021). doi: 10.1016/j.jpha.2021.07.002
18. Seok JK, Kang HC, Cho Y-Y, Lee HS, Lee JY. Regulation of the NLRP3 Inflammasome by Post-Translational Modifications and Small Molecules. Front Immunol (2021) 11:3877. doi: 10.3389/fimmu.2020.618231
19. Bukowski K, Kciuk M, Kontek R. Mechanisms of Multidrug Resistance in Cancer Chemotherapy. Int J Mol Sci (2020) 21(9). doi: 10.3390/ijms21093233
20. Hasan S, Taha R, El Omri H. Current Opinions on Chemoresistance: An Overview. Bioinformation (2018) 14(2):80. doi: 10.6026/97320630014080
21. Khatoon E, Banik K, Harsha C, Sailo BL, Thakur KK, Khwairakpam AD, et al. Phytochemicals in Cancer Cell Chemosensitization: Current Knowledge and Future Perspectives. Semin Cancer Biol (2020). doi: 10.1016/j.semcancer.2020.06.014
22. Pietroiusti A, Campagnolo L, Fadeel B. Interactions of Engineered Nanoparticles With Organs Protected by Internal Biological Barriers. Small (2013) 9(9-10):1557–72. doi: 10.1002/smll.201201463
23. Salama L, Pastor ER, Stone T, Mousa SA. Emerging Nanopharmaceuticals and Nanonutraceuticals in Cancer Management. Biomedicines (2020) 8(9). doi: 10.3390/biomedicines8090347
24. Lyall RM, Hwang JL, Cardarelli C, FitzGerald D, Akiyama S, Gottesman MM, et al. Isolation of Human KB Cell Lines Resistant to Epidermal Growth Factor-Pseudomonas Exotoxin Conjugates. Cancer Res (1987) 47(11):2961–6.
25. Akbari B, Farajnia S, Ahdi Khosroshahi S, Safari F, Yousefi M, Dariushnejad H, et al. Immunotoxins in Cancer Therapy: Review and Update. Int Rev Immunol (2017) 36(4):207–19. doi: 10.1080/08830185.2017.1284211
26. Li S, Gao M, Li Z, Song L, Gao X, Han J, et al. P53 and P-Glycoprotein Influence Chemoresistance in Hepatocellular Carcinoma. Front Biosci (Elite Ed) (2018) 10:461–8. doi: 10.2741/e833
27. Giddings EL, Champagne DP, Wu M-H, Laffin JM, Thornton TM, Valenca-Pereira F, et al. Mitochondrial ATP Fuels ABC Transporter-Mediated Drug Efflux in Cancer Chemoresistance. Nat Commun (2021) 12(1):1–19. doi: 10.1038/s41467-021-23071-6
28. Liu X. ABC Family Transporters. Adv Exp Med Biol (2019) 1141:13–100. doi: 10.1007/978-981-13-7647-4_2
29. Guo F, Zhang H, Jia Z, Cui M, Tian J. Chemoresistance and Targeting of Growth Factors/Cytokines Signalling Pathways: Towards the Development of Effective Therapeutic Strategy for Endometrial Cancer. Am J Cancer Res (2018) 8(7):1317.
30. Hombach-Klonisch S, Mehrpour M, Shojaei S, Harlos C, Pitz M, Hamai A, et al. Glioblastoma and Chemoresistance to Alkylating Agents: Involvement of Apoptosis, Autophagy, and Unfolded Protein Response. Pharmacol Ther (2018) 184:13–41. doi: 10.1016/j.pharmthera.2017.10.017
31. Abbaszadeh F, Fakhri S, Khan H. Targeting Apoptosis and Autophagy Following Spinal Cord Injury: Therapeutic Approaches to Polyphenols and Candidate Phytochemicals. Pharmacol Res (2020) 160:105069. doi: 10.1016/j.phrs.2020.105069
32. Fakhri S, Tomas M, Capanoglu E, Hussain Y, Abbaszadeh F, Lu B, et al. Antioxidant and Anticancer Potentials of Edible Flowers: Where do We Stand? Crit Rev Food Sci Nutr (2021), 1–57. doi: 10.1080/10408398.2021.1931022
33. Fakhri S, Khodamorady M, Naseri M, Farzaei MH, Khan H. The Ameliorating Effects of Anthocyanins on the Cross-Linked Signaling Pathways of Cancer Dysregulated Metabolism. Pharmacol Res (2020) 159:104895. doi: 10.1016/j.phrs.2020.104895
34. Rahman N, Khan H, Zia A, Khan A, Fakhri S, Aschner M, et al. Bcl-2 Modulation in P53 Signaling Pathway by Flavonoids: A Potential Strategy Towards the Treatment of Cancer. Int J Mol Sci (2021) 22(21):11315. doi: 10.3390/ijms222111315
35. Ahmad I, Fakhri S, Khan H, Jeandet P, Aschner M, Yu Z-L. Targeting Cell Cycle by β-Carboline Alkaloids In Vitro: Novel Therapeutic Prospects for the Treatment of Cancer. Chem-Biol Interact (2020) 330:109229. doi: 10.1016/j.cbi.2020.109229
36. Deng J, Bai X, Feng X, Ni J, Beretov J, Graham P, et al. Inhibition of PI3K/Akt/mTOR Signaling Pathway Alleviates Ovarian Cancer Chemoresistance Through Reversing Epithelial-Mesenchymal Transition and Decreasing Cancer Stem Cell Marker Expression. BMC Cancer (2019) 19(1):1–12. doi: 10.1186/s12885-019-5824-9
37. Yang W, Liu Y, Xu QQ, Xian YF, Lin ZX. Sulforaphene Ameliorates Neuroinflammation and Hyperphosphorylated Tau Protein via Regulating the PI3K/Akt/GSK-3β Pathway in Experimental Models of Alzheimer's Disease. Oxid Med Cell Longev (2020):4754195. doi: 10.1155/2020/4754195
38. Colombo M, Platonova N, Giannandrea D, Palano MT, Basile A, Chiaramonte R. Re-Establishing Apoptosis Competence in Bone Associated Cancers via Communicative Reprogramming Induced Through Notch Signaling Inhibition. Front Pharmacol (2019) 10:145. doi: 10.3389/fphar.2019.00145
39. Xi G, Hayes E, Lewis R, Ichi S, Mania-Farnell B, Shim K, et al. CD133 and DNA-PK Regulate MDR1 via the PI3K- or Akt-NF-κb Pathway in Multidrug-Resistant Glioblastoma Cells In Vitro. Oncogene (2016) 35(2):241–50. doi: 10.1038/onc.2015.78
40. Vo TT, Letai A. BH3-Only Proteins and Their Effects on Cancer. Adv Exp Med Biol (2010) 687:49–63. doi: 10.1007/978-1-4419-6706-0_3
41. Campbell KJ, Tait SWG. Targeting BCL-2 Regulated Apoptosis in Cancer. Open Biol (2018) 8(5). doi: 10.1098/rsob.180002
42. Sharma P, Hu-Lieskovan S, Wargo JA, Ribas A. Primary, Adaptive, and Acquired Resistance to Cancer Immunotherapy. Cell (2017) 168(4):707–23. doi: 10.1016/j.cell.2017.01.017
43. Kim TY, Leem E, Lee JM, Kim SR. Control of Reactive Oxygen Species for the Prevention of Parkinson's Disease: The Possible Application of Flavonoids. Antioxid (Basel) (2020) 9(7). doi: 10.3390/antiox9070583
44. Gong J, Chehrazi-Raffle A, Reddi S, Salgia R. Development of PD-1 and PD-L1 Inhibitors as a Form of Cancer Immunotherapy: A Comprehensive Review of Registration Trials and Future Considerations. J Immunother Cancer (2018) 6(1):8. doi: 10.1186/s40425-018-0316-z
45. DuPage M, Mazumdar C, Schmidt LM, Cheung AF, Jacks T. Expression of Tumour-Specific Antigens Underlies Cancer Immunoediting. Nature (2012) 482(7385):405–9. doi: 10.1038/nature10803
46. Xu-Monette ZY, Zhang M, Li J, Young KH. PD-1/PD-L1 Blockade: Have We Found the Key to Unleash the Antitumor Immune Response? Front Immunol (2017) 8:1597:1597. doi: 10.3389/fimmu.2017.01597
47. Galluzzi L, Buqué A, Kepp O, Zitvogel L, Kroemer G. Immunological Effects of Conventional Chemotherapy and Targeted Anticancer Agents. Cancer Cell (2015) 28(6):690–714. doi: 10.1016/j.ccell.2015.10.012
48. Sokol L, Koelzer VH, Rau TT, Karamitopoulou E, Zlobec I, Lugli A. Loss of Tapasin Correlates With Diminished CD8(+) T-Cell Immunity and Prognosis in Colorectal Cancer. J Transl Med (2015) 13:279. doi: 10.1186/s12967-015-0647-1
49. Gao M, Gao L, Yang G, Tao Y, Hou J, Xu H, et al. Myeloma Cells Resistance to NK Cell Lysis Mainly Involves an HLA Class I-Dependent Mechanism. Acta Biochim Biophys Sin (Shanghai) (2014) 46(7):597–604. doi: 10.1093/abbs/gmu041
50. Rieth J, Subramanian S. Mechanisms of Intrinsic Tumor Resistance to Immunotherapy. Int J Mol Sci (2018) 19(5). doi: 10.3390/ijms19051340
51. Gao J, Shi LZ, Zhao H, Chen J, Xiong L, He Q, et al. Loss of IFN-γ Pathway Genes in Tumor Cells as a Mechanism of Resistance to Anti-CTLA-4 Therapy. Cell (2016) 167(2):397–404.e399. doi: 10.1016/j.cell.2016.08.069
52. Possick JD. Pulmonary Toxicities From Checkpoint Immunotherapy for Malignancy. Clin Chest Med (2017) 38(2):223–32. doi: 10.1016/j.ccm.2016.12.012
53. Pan D, Kobayashi A, Jiang P, Ferrari de Andrade L, Tay RE, Luoma AM, et al. A Major Chromatin Regulator Determines Resistance of Tumor Cells to T Cell-Mediated Killing. Science (2018) 359(6377):770–5. doi: 10.1126/science.aao1710
54. Spranger S, Bao R, Gajewski TF. Melanoma-Intrinsic β-Catenin Signalling Prevents Anti-Tumour Immunity. Nature (2015) 523(7559):231–5. doi: 10.1038/nature14404
55. Dhillon AS, Hagan S, Rath O, Kolch W. MAP Kinase Signalling Pathways in Cancer. Oncogene (2007) 26(22):3279–90. doi: 10.1038/sj.onc.1210421
56. Peng W, Chen JQ, Liu C, Malu S, Creasy C, Tetzlaff MT, et al. Loss of PTEN Promotes Resistance to T Cell-Mediated Immunotherapy. Cancer Discov (2016) 6(2):202–16. doi: 10.1158/2159-8290.cd-15-0283
57. Yoshida GJ. Metabolic Reprogramming: The Emerging Concept and Associated Therapeutic Strategies. J Exp Clin Cancer Res (2015) 34:111. doi: 10.1186/s13046-015-0221-y
58. Dietl K, Renner K, Dettmer K, Timischl B, Eberhart K, Dorn C, et al. Lactic Acid and Acidification Inhibit TNF Secretion and Glycolysis of Human Monocytes. J Immunol (2010) 184(3):1200–9. doi: 10.4049/jimmunol.0902584
59. Chang CH, Qiu J, O'Sullivan D, Buck MD, Noguchi T, Curtis JD, et al. Metabolic Competition in the Tumor Microenvironment Is a Driver of Cancer Progression. Cell (2015) 162(6):1229–41. doi: 10.1016/j.cell.2015.08.016
60. Kawasaki T, Kawai T. Toll-Like Receptor Signaling Pathways. Front Immunol (2014) 5:461. doi: 10.3389/fimmu.2014.00461
61. Webb JT, Behar M. Topology, Dynamics, and Heterogeneity in Immune Signaling. Wiley Interdiscip Rev: Syst Biol Med (2015) 7(5):285–300. doi: 10.1002/wsbm.1306
62. Dajon M, Iribarren K, Cremer I. Toll-Like Receptor Stimulation in Cancer: A Pro-and Anti-Tumor Double-Edged Sword. Immunobiology (2017) 222(1):89–100. doi: 10.1016/j.imbio.2016.06.009
63. Yang H, Zhou H, Feng P, Zhou X, Wen H, Xie X, et al. Reduced Expression of Toll-Like Receptor 4 Inhibits Human Breast Cancer Cells Proliferation and Inflammatory Cytokines Secretion. J Exp Clin Cancer Res (2010) 29(1):1–8. doi: 10.1186/1756-9966-29-92
64. Moradi-Marjaneh R, Hassanian SM, Fiuji H, Soleimanpour S, Ferns GA, Avan A, et al. Toll Like Receptor Signaling Pathway as a Potential Therapeutic Target in Colorectal Cancer. J Cell Physiol (2018) 233(8):5613–22. doi: 10.1002/jcp.26273
65. Zhao S, Zhang Y, Zhang Q, Wang F, Zhang D. Toll-Like Receptors and Prostate Cancer. Front Immunol (2014) 5:352. doi: 10.3389/fimmu.2014.00352
66. Yusuf N, Nasti TH, Long JA, Naseemuddin M, Lucas AP, Xu H, et al. Protective Role of Toll-Like Receptor 4 During the Initiation Stage of Cutaneous Chemical Carcinogenesis. Cancer Res (2008) 68(2):615–22. doi: 10.1158/0008-5472.CAN-07-5219
67. Park GB, Jeong JY, Kim D. Modified TLR-Mediated Downregulation of miR-125b-5p Enhances CD248 (Endosialin)-Induced Metastasis and Drug Resistance in Colorectal Cancer Cells. Mol Carcinog (2020) 59(2):154–67. doi: 10.1002/mc.23137
68. Meng Q, Liang C, Hua J, Zhang B, Liu J, Zhang Y, et al. A miR-146a-5p/TRAF6/NF-kB P65 Axis Regulates Pancreatic Cancer Chemoresistance: Functional Validation and Clinical Significance. Theranostics (2020) 10(9):3967. doi: 10.7150/thno.40566
69. Tan S-F, Dunton W, Liu X, Fox TE, Morad SA, Desai D, et al. Acid Ceramidase Promotes Drug Resistance in Acute Myeloid Leukemia Through NF-κb-Dependent P-Glycoprotein Upregulation. J Lipid Res (2019) 60(6):1078–86. doi: 10.1194/jlr.M091876
70. Lee C, Do HTT, Her J, Kim Y, Seo D, Rhee I. Inflammasome as a Promising Therapeutic Target for Cancer. Life Sci (2019) 231:116593. doi: 10.1016/j.lfs.2019.116593
71. Terlizzi M, Casolaro V, Pinto A, Sorrentino R. Inflammasome: Cancer's Friend or Foe? Pharmacol Ther (2014) 143(1):24–33. doi: 10.1016/j.pharmthera.2014.02.002
72. Balistreri CR, Ruvolo G, Lio D, Madonna R. Toll-Like Receptor-4 Signaling Pathway in Aorta Aging and Diseases:”Its Double Nature”. J Mol Cell Cardiol (2017) 110:38–53. doi: 10.1016/j.yjmcc.2017.06.011
73. Rahimifard M, Maqbool F, Moeini-Nodeh S, Niaz K, Abdollahi M, Braidy N, et al. Targeting the TLR4 Signaling Pathway by Polyphenols: A Novel Therapeutic Strategy for Neuroinflammation. Ageing Res Rev (2017) 36:11–9. doi: 10.1016/j.arr.2017.02.004
74. Cen X, Liu S, Cheng K. The Role of Toll-Like Receptor in Inflammation and Tumor Immunity. Front Pharmacol (2018) 9:878. doi: 10.3389/fphar.2018.00878
75. Nagai Y, Akashi S, Nagafuku M, Ogata M, Iwakura Y, Akira S, et al. Essential Role of MD-2 in LPS Responsiveness and TLR4 Distribution. Nat Immunol (2002) 3(7):667–72. doi: 10.1038/ni809
76. Huang L, Xu H, Peng G. TLR-Mediated Metabolic Reprogramming in the Tumor Microenvironment: Potential Novel Strategies for Cancer Immunotherapy. Cell Mol Immunol (2018) 15(5):428–37. doi: 10.1038/cmi.2018.4
77. Takeda K, Akira S. Microbial Recognition by Toll-Like Receptors. J Dermatol Sci (2004) 34(2):73–82. doi: 10.1016/j.jdermsci.2003.10.002
78. Koh Y-C, Yang G, Lai C-S, Weerawatanakorn M, Pan M-H. Chemopreventive Effects of Phytochemicals and Medicines on M1/M2 Polarized Macrophage Role in Inflammation-Related Diseases. Int J Mol Sci (2018) 19(8):2208. doi: 10.3390/ijms19082208
79. Prabhu DS, Selvam AP, Rajeswari VD. Effective Anti-Cancer Property of Pouteria Sapota Leaf on Breast Cancer Cell Lines. Biochem Biophys Rep (2018) 15:39–44. doi: 10.1016/j.bbrep.2018.06.004
80. Fakhri S, Pesce M, Patruno A, Moradi SZ, Iranpanah A, Farzaei MH, et al. Attenuation of Nrf2/Keap1/ARE in Alzheimer’s Disease by Plant Secondary Metabolites: A Mechanistic Review. Molecules (2020) 25(21):4926. doi: 10.3390/molecules25214926
81. Moradi SZ, Jalili F, Farhadian N, Joshi T, Wang M, Zou L, et al. Polyphenols and Neurodegenerative Diseases: Focus on Neuronal Regeneration. Crit Rev Food Sci Nutr (2020), 1–16. doi: 10.1080/10408398.2020.1865870
82. Moradi SZ, Momtaz S, Bayrami Z, Farzaei MH, Abdollahi M. Nanoformulations of Herbal Extracts in Treatment of Neurodegenerative Disorders. Front Bioeng Biotechnol (2020) 8:238. doi: 10.3389/fbioe.2020.00238
83. Fakhri S, Iranpanah A, Gravandi MM, Moradi SZ, Ranjbari M, Majnooni MB, et al. Natural Products Attenuate PI3K/Akt/mTOR Signaling Pathway: A Promising Strategy in Regulating Neurodegeneration. Phytomedicine (2021) 91:153664. doi: 10.1016/j.phymed.2021.153664
84. Fakhri S, Nouri Z, Moradi SZ, Akkol EK, Piri S, Sobarzo-Sánchez E, et al. Targeting Multiple Signal Transduction Pathways of SARS-CoV-2: Approaches to COVID-19 Therapeutic Candidates. Molecules (2021) 26(10):2917. doi: 10.3390/molecules26102917
85. Fakhri S, Piri S, Moradi SZ, Khan H. Phytochemicals Targeting Oxidative Stress, Interconnected Neuroinflammatory and Neuroapoptotic Pathways Following Radiation. Curr Neuropharmacol (2021). doi: 10.2174/1570159X19666210809103346
86. Li P-M, Li Y-L, Liu B, Wang W-J, Wang Y-Z, Li Z. Curcumin Inhibits MHCC97H Liver Cancer Cells by Activating ROS/TLR-4/Caspase Signaling Pathway. Asian Pac J Cancer Prev (2014) 15(5):2329–34. doi: 10.7314/APJCP.2014.15.5.2329
87. Ren B, Luo S, Tian X, Jiang Z, Zou G, Xu F, et al. Curcumin Inhibits Liver Cancer by Inhibiting DAMP Molecule HSP70 and TLR4 Signaling. Oncol Rep (2018) 40(2):895–901. doi: 10.3892/or.2018.6485
88. Tian S, Liao L, Zhou Q, Huang X, Zheng P, Guo Y, et al. Curcumin Inhibits the Growth of Liver Cancer by Impairing Myeloid−Derived Suppressor Cells in Murine Tumor Tissues. Oncol Lett (2021) 21(4):1–1.
89. Zhang L, Tao X, Fu Q, Ge C, Li R, Li Z, et al. Curcumin Inhibits Cell Proliferation and Migration in NSCLC Through a Synergistic Effect on the TLR4/MyD88 and EGFR Pathways. Oncol Rep (2019) 42(5):1843–55. doi: 10.3892/or.2019.7278
90. Eskiler GG, Özkan AD, Kaleli S, Bilir C. Inhibition of TLR4/TRIF/IRF3 Signaling Pathway by Curcumin in Breast Cancer Cells. J Pharm Pharm Sci (2019) 22:281–91. doi: 10.18433/jpps30493
91. Meyer C, Pries R, Wollenberg B. Established and Novel NF-κb Inhibitors Lead to Downregulation of TLR3 and the Proliferation and Cytokine Secretion in HNSCC. Oral Oncol (2011) 47(9):818–26. doi: 10.1016/j.oraloncology.2011.06.010
92. Shishodia S, Amin HM, Lai R, Aggarwal BB. Curcumin (Diferuloylmethane) Inhibits Constitutive NF-κb Activation, Induces G1/S Arrest, Suppresses Proliferation, and Induces Apoptosis in Mantle Cell Lymphoma. Biochem Pharmacol (2005) 70(5):700–13. doi: 10.1016/j.bcp.2005.04.043
93. Wong TS, Chan WS, Li CH, Liu RW, Tang WW, Tsao SW, et al. Curcumin Alters the Migratory Phenotype of Nasopharyngeal Carcinoma Cells Through Up-Regulation of E-Cadherin. Anticancer Res (2010) 30(7):2851–6.
94. Deng Z, Xu X-Y, Yunita F, Zhou Q, Wu Y-R, Hu Y-X, et al. Synergistic Anti-Liver Cancer Effects of Curcumin and Total Ginsenosides. World J Gastrointest Oncol (2020) 12(10):1091. doi: 10.4251/wjgo.v12.i10.1091
95. Li X, Ma S, Yang P, Sun B, Zhang Y, Sun Y, et al. Anticancer Effects of Curcumin on Nude Mice Bearing Lung Cancer A549 Cell Subsets SP and NSP Cells. Oncol Lett (2018) 16(5):6756–62. doi: 10.3892/ol.2018.9488
96. Wu X, Koh GY, Huang Y, Crott JW, Bronson RT, Mason JB. The Combination of Curcumin and Salsalate is Superior to Either Agent Alone in Suppressing Pro-Cancerous Molecular Pathways and Colorectal Tumorigenesis in Obese Mice. Mol Nutr Food Res (2019) 63(8):1801097. doi: 10.1002/mnfr.201801097
97. Hao J, Dai X, Gao J, Li Y, Hou Z, Chang Z, et al. Curcumin Suppresses Colorectal Tumorigenesis via the Wnt/β−Catenin Signaling Pathway by Downregulating Axin2. Oncol Lett (2021) 21(3):1–1. doi: 10.3892/ol.2021.12447
98. Xiang L, He B, Liu Q, Hu D, Liao W, Li R, et al. Antitumor Effects of Curcumin on the Proliferation, Migration and Apoptosis of Human Colorectal Carcinoma HCT−116 Cells. Oncol Rep (2020) 44(5):1997–2008. doi: 10.3892/or.2020.7765
99. El-Mesallamy H, Salman TM, Ashmawey AM, Osama N. Evaluating the Role of Curcum Powder as a Protective Factor Against Bladder Cancer-An Experimental Study. Asian Pac J Cancer Prev (2012) 13(10):5287–90. doi: 10.7314/APJCP.2012.13.10.5287
100. Shrestha S, Zhu J, Wang Q, Du X, Liu F, Jiang J, et al. Melatonin Potentiates the Antitumor Effect of Curcumin by Inhibiting Ikkκ/NF-κb/COX-2 Signaling Pathway. Int J Oncol (2017) 51(4):1249–60. doi: 10.3892/ijo.2017.4097
101. Bisht S, Feldmann G, Soni S, Ravi R, Karikar C, Maitra A, et al. Polymeric Nanoparticle-Encapsulated Curcumin (" Nanocurcumin"): A Novel Strategy for Human Cancer Therapy. J Nanobiotechnol (2007) 5(1):1–18. doi: 10.1186/1477-3155-5-3
102. Dhillon N, Aggarwal BB, Newman RA, Wolff RA, Kunnumakkara AB, Abbruzzese JL, et al. Phase II Trial of Curcumin in Patients With Advanced Pancreatic Cancer. Clin Cancer Res (2008) 14(14):4491–9. doi: 10.1158/1078-0432.CCR-08-0024
103. Kim JM, Noh EM, Kwon KB, Kim JS, You YO, Hwang JK, et al. Curcumin Suppresses the TPA-Induced Invasion Through Inhibition of Pkcα-Dependent MMP-Expression in MCF-7 Human Breast Cancer Cells. Phytomedicine (2012) 19(12):1085–92. doi: 10.1016/j.phymed.2012.07.002
104. Cao F, Liu T, Xu Y, Xu D, Feng S. Curcumin Inhibits Cell Proliferation and Promotes Apoptosis in Human Osteoclastoma Cell Through MMP-9, NF-κb and JNK Signaling Pathways. Int J Clin Exp Pathol (2015) 8(6):6037–45.
105. Hu A, Huang JJ, Li RL, Lu ZY, Duan JL, Xu WH, et al. Curcumin as Therapeutics for the Treatment of Head and Neck Squamous Cell Carcinoma by Activating SIRT1. Sci Rep (2015) 5:13429. doi: 10.1038/srep13429
106. Zhu GH, Dai HP, Shen Q, Ji O, Zhang Q, Zhai YL. Curcumin Induces Apoptosis and Suppresses Invasion Through MAPK and MMP Signaling in Human Monocytic Leukemia SHI-1 Cells. Pharm Biol (2016) 54(8):1303–11. doi: 10.3109/13880209.2015.1060508
107. Zhu G, Shen Q, Jiang H, Ji O, Zhu L, Zhang L. Curcumin Inhibited the Growth and Invasion of Human Monocytic Leukaemia SHI-1 Cells In Vivo by Altering MAPK and MMP Signalling. Pharm Biol (2020) 58(1):25–34. doi: 10.1080/13880209.2019.1701042
108. Yang L, Hu Z, Zhu J, Liang Q, Zhou H, Li J, et al. Systematic Elucidation of the Mechanism of Quercetin Against Gastric Cancer via Network Pharmacology Approach. BioMed Res Int (2020) 2020:3860213. doi: 10.1155/2020/3860213
109. Masuelli L, Di Stefano E, Fantini M, Mattera R, Benvenuto M, Marzocchella L, et al. Resveratrol Potentiates the In Vitro and In Vivo Anti-Tumoral Effects of Curcumin in Head and Neck Carcinomas. Oncotarget (2014) 5(21):10745–62. doi: 10.18632/oncotarget.2534
110. Srivastava NS, Srivastava RAK. Curcumin and Quercetin Synergistically Inhibit Cancer Cell Proliferation in Multiple Cancer Cells and Modulate Wnt/β-Catenin Signaling and Apoptotic Pathways in A375 Cells. Phytomedicine (2019) 52:117–28. doi: 10.1016/j.phymed.2018.09.224
111. Mutlu Altundağ E, Yılmaz AM, Serdar BS, Jannuzzi AT, Koçtürk S, Yalçın AS. Synergistic Induction of Apoptosis by Quercetin and Curcumin in Chronic Myeloid Leukemia (K562) Cells: II. Signal Transduction Pathways Involved. Nutr Cancer (2021) 73(4):703–12. doi: 10.1080/01635581.2020.1767167
112. Yusuf N, Nasti TH, Meleth S, Elmets CA. Resveratrol Enhances Cell-Mediated Immune Response to DMBA Through TLR4 and Prevents DMBA Induced Cutaneous Carcinogenesis. Mol Carcinog (2009) 48(8):713–23. doi: 10.1002/mc.20517
113. Panaro MA, Carofiglio V, Acquafredda A, Cavallo P. And Cianciulli, AAnti-Inflammatory Effects of Resveratrol Occur via Inhibition of Lipopolysaccharide-Induced NF-κb Activation in Caco-2 and SW480 Human Colon Cancer Cells. Br J Nutr (2012) 108(9):1623–32. doi: 10.1017/S0007114511007227
114. Estrov Z, Shishodia S, Faderl S, Harris D, Van Q, Kantarjian HM, et al. Resveratrol Blocks Interleukin-1β–Induced Activation of the Nuclear Transcription Factor NF-κb, Inhibits Proliferation, Causes S-Phase Arrest, and Induces Apoptosis of Acute Myeloid Leukemia Cells. Blood (2003) 102(3):987–95. doi: 10.1182/blood-2002-11-3550
115. Li W, Ma J, Ma Q, Li B, Han L, Liu J, et al. Resveratrol Inhibits the Epithelial-Mesenchymal Transition of Pancreatic Cancer Cells via Suppression of the PI-3k/Akt/NF-κb Pathway. Curr Med Chem (2013) 20(33):4185–94. doi: 10.2174/09298673113209990251
116. Salla M, Pandya V, Bhullar KS, Kerek E, Wong YF, Losch R, et al. Resveratrol and Resveratrol-Aspirin Hybrid Compounds as Potent Intestinal Anti-Inflammatory and Anti-Tumor Drugs. Molecules (2020) 25(17):3849. doi: 10.3390/molecules25173849
117. Ivanov VN, Partridge MA, Johnson GE, Huang SX, Zhou H, Hei TK. Resveratrol Sensitizes Melanomas to TRAIL Through Modulation of Antiapoptotic Gene Expression. Exp Cell Res (2008) 314(5):1163–76. doi: 10.1016/j.yexcr.2007.12.012
118. Kim YA, Lee WH, Choi TH, Rhee SH, Park KY, Choi YH. Involvement of P21waf1/CIP1, pRB, Bax and NF-kappaB in Induction of Growth Arrest and Apoptosis by Resveratrol in Human Lung Carcinoma A549 Cells. Int J Oncol (2003) 23(4):1143–9.
119. Rasheduzzaman M, Jeong JK, Park SY. Resveratrol Sensitizes Lung Cancer Cell to TRAIL by P53 Independent and Suppression of Akt/NF-κb Signaling. Life Sci (2018) 208:208–20. doi: 10.1016/j.lfs.2018.07.035
120. Huang H, Lin H, Zhang X, Li J. Resveratrol Reverses Temozolomide Resistance by Downregulation of MGMT in T98G Glioblastoma Cells by the NF-κb-Dependent Pathway. Oncol Rep (2012) 27(6):2050–6. doi: 10.3892/or.2012.1715
121. Yu HB, Zhang HF, Zhang X, Li DY, Xue HZ, Pan CE, et al. Resveratrol Inhibits VEGF Expression of Human Hepatocellular Carcinoma Cells Through a NF-Kappa B-Mediated Mechanism. Hepatogastroenterology (2010) 57(102-103):1241–6.
122. Wang Z, Zhang L, Ni Z, Sun J, Gao H, Cheng Z, et al. Resveratrol Induces AMPK-Dependent MDR1 Inhibition in Colorectal Cancer HCT116/L-OHP Cells by Preventing Activation of NF-κb Signaling and Suppressing cAMP-Responsive Element Transcriptional Activity. Tumour Biol (2015) 36(12):9499–510. doi: 10.1007/s13277-015-3636-3
123. Gao J, Ma F, Wang X, Li G. Combination of Dihydroartemisinin and Resveratrol Effectively Inhibits Cancer Cell Migration via Regulation of the DLC1/TCTP/Cdc42 Pathway. Food Funct (2020) 11(11):9573–84. doi: 10.1039/d0fo00996b
124. Fan S-H, Wang Y-Y, Lu J, Zheng Y-L, Wu D-M, Li M-Q, et al. Luteoloside Suppresses Proliferation and Metastasis of Hepatocellular Carcinoma Cells by Inhibition of NLRP3 Inflammasome. PloS One (2014) 9(2):e89961. doi: 10.1371/journal.pone.0089961
125. Ho HH, Chang CS, Ho WC, Liao SY, Wu CH, Wang CJ. Anti-Metastasis Effects of Gallic Acid on Gastric Cancer Cells Involves Inhibition of NF-kappaB Activity and Downregulation of PI3K/AKT/small GTPase Signals. Food Chem Toxicol (2010) 48(8-9):2508–16. doi: 10.1016/j.fct.2010.06.024
126. Zeng M, Su Y, Li K, Jin D, Li Q, Li Y, et al. Gallic Acid Inhibits Bladder Cancer T24 Cell Progression Through Mitochondrial Dysfunction and PI3K/Akt/NF-κb Signaling Suppression. Front Pharmacol (2020) 11:1222. doi: 10.3389/fphar.2020.01222
127. Han M, Song Y, Zhang X. Quercetin Suppresses the Migration and Invasion in Human Colon Cancer Caco-2 Cells Through Regulating Toll-Like Receptor 4/Nuclear Factor-Kappa B Pathway. Pharmacogn Mag (2016) 12(Suppl 2):S237. doi: 10.4103/0973-1296.182154
128. Priyadarsini RV, Murugan RS, Maitreyi S, Ramalingam K, Karunagaran D, Nagini S. The Flavonoid Quercetin Induces Cell Cycle Arrest and Mitochondria-Mediated Apoptosis in Human Cervical Cancer (HeLa) Cells Through P53 Induction and NF-κb Inhibition. Eur J Pharmacol (2010) 649(1-3):84–91. doi: 10.1016/j.ejphar.2010.09.020
129. Youn H, Jeong J-C, Jeong YS, Kim E-J, Um S-J. Quercetin Potentiates Apoptosis by Inhibiting Nuclear Factor-kappaB Signaling in H460 Lung Cancer Cells. Biol Pharm Bull (2013) 36(6):944–51. doi: 10.1248/bpb.b12-01004
130. Mukherjee A, Khuda-Bukhsh AR. Quercetin Down-Regulates IL-6/STAT-3 Signals to Induce Mitochondrial-Mediated Apoptosis in a Nonsmall-Cell Lung-Cancer Cell Line, A549. J Pharmacopuncture (2015) 18(1):19. doi: 10.3831/KPI.2015.18.002
131. Erdogan S, Turkekul K, Dibirdik I, Doganlar O, Doganlar ZB, Bilir A, et al. Midkine Downregulation Increases the Efficacy of Quercetin on Prostate Cancer Stem Cell Survival and Migration Through PI3K/AKT and MAPK/ERK Pathway. Biomed Pharmacother (2018) 107:793–805. doi: 10.1016/j.biopha.2018.08.061
132. Kıyga E, Şengelen A, Adıgüzel Z, Önay Uçar E. Investigation of the Role of Quercetin as a Heat Shock Protein Inhibitor on Apoptosis in Human Breast Cancer Cells. Mol Biol Rep (2020) 47(7):4957–67. doi: 10.1007/s11033-020-05641-x
133. Zhang W, Yin G, Dai J, Sun YU, Hoffman RM, Yang Z, et al. Chemoprevention by Quercetin of Oral Squamous Cell Carcinoma by Suppression of the NF-κb Signaling Pathway in DMBA-Treated Hamsters. Anticancer Res (2017) 37(8):4041–9. doi: 10.21873/anticanres.11789
134. Wu T-C, Chan S-T, Chang C-N, Yu P-S, Chuang C-H, Yeh S-L. Quercetin and Chrysin Inhibit Nickel-Induced Invasion and Migration by Downregulation of TLR4/NF-κb Signaling in A549 Cells. Chem-Biol Interact (2018) 292:101–9. doi: 10.1016/j.cbi.2018.07.010
135. Chua KV, Fan C-S, Chen C-C, Chen L-L, Hsieh S-C, Huang T-S. Octyl Gallate Induces Pancreatic Ductal Adenocarcinoma Cell Apoptosis and Suppresses Endothelial-Mesenchymal Transition-Promoted M2-Macrophages, Hsp90α Secretion, and Tumor Growth. Cells (2020) 9(1):91. doi: 10.3390/cells9010091
136. Ellis LZ, Liu W, Luo Y, Okamoto M, Qu D, Dunn JH, et al. Green Tea Polyphenol Epigallocatechin-3-Gallate Suppresses Melanoma Growth by Inhibiting Inflammasome and IL-1β Secretion. Biochem Biophys Res Commun (2011) 414(3):551–6. doi: 10.1016/j.bbrc.2011.09.115
137. Wang T, Zhou H, Xie H, Mu Y, Xu Y, Liu J, et al. Epigallocatechin-3-Gallate Inhibits TF and TNF-α Expression Induced by the Anti-β2gpi/β2gpi Complex in Human THP-1 Cells. Int J Mol Med (2014) 33(4):994–1002. doi: 10.3892/ijmm.2014.1635
138. Belguise K, Guo S, Sonenshein GE. Activation of FOXO3a by the Green Tea Polyphenol Epigallocatechin-3-Gallate Induces Estrogen Receptor α Expression Reversing Invasive Phenotype of Breast Cancer Cells. Cancer Res (2007) 67(12):5763–70. doi: 10.1158/0008-5472.CAN-06-4327
139. Pianetti S, Guo S, Kavanagh KT, Sonenshein GE. Green Tea Polyphenol Epigallocatechin-3 Gallate Inhibits Her-2/Neu Signaling, Proliferation, and Transformed Phenotype of Breast Cancer Cells. Cancer Res (2002) 62(3):652–5.
140. Hoffmann J, Junker H, Schmieder A, Venz S, Brandt R, Multhoff G, et al. EGCG Downregulates IL-1RI Expression and Suppresses IL-1-Induced Tumorigenic Factors in Human Pancreatic Adenocarcinoma Cells. Biochem Pharmacol (2011) 82(9):1153–62. doi: 10.1016/j.bcp.2011.07.063
141. Sen T, Moulik S, Dutta A, Choudhury PR, Banerji A, Das S, et al. Multifunctional Effect of Epigallocatechin-3-Gallate (EGCG) in Downregulation of Gelatinase-A (MMP-2) in Human Breast Cancer Cell Line MCF-7. Life Sci (2009) 84(7-8):194–204. doi: 10.1016/j.lfs.2008.11.018
142. Fang CY, Wu CC, Hsu HY, Chuang HY, Huang SY, Tsai CH, et al. EGCG Inhibits Proliferation, Invasiveness and Tumor Growth by Up-Regulation of Adhesion Molecules, Suppression of Gelatinases Activity, and Induction of Apoptosis in Nasopharyngeal Carcinoma Cells. Int J Mol Sci (2015) 16(2):2530–58. doi: 10.3390/ijms16022530
143. Luo KW, Wei C, Lung WY, Wei XY, Cheng BH, Cai ZM, et al. EGCG Inhibited Bladder Cancer SW780 Cell Proliferation and Migration Both In Vitro and In Vivo via Down-Regulation of NF-κb and MMP-9. J Nutr Biochem (2017) 41:56–64. doi: 10.1016/j.jnutbio.2016.12.004
144. Nishikawa T, Nakajima T, Moriguchi M, Jo M, Sekoguchi S, Ishii M, et al. A Green Tea Polyphenol, Epigalocatechin-3-Gallate, Induces Apoptosis of Human Hepatocellular Carcinoma, Possibly Through Inhibition of Bcl-2 Family Proteins. J Hepatol (2006) 44(6):1074–82. doi: 10.1016/j.jhep.2005.11.045
145. Xu P, Yan F, Zhao Y, Chen X, Sun S, Wang Y, et al. Green Tea Polyphenol EGCG Attenuates MDSCs-Mediated Immunosuppression Through Canonical and Non-Canonical Pathways in a 4T1 Murine Breast Cancer Model. Nutrients (2020) 12(4). doi: 10.3390/nu12041042
146. Saldanha SN, Kala R, Tollefsbol TO. Molecular Mechanisms for Inhibition of Colon Cancer Cells by Combined Epigenetic-Modulating Epigallocatechin Gallate and Sodium Butyrate. Exp Cell Res (2014) 324(1):40–53. doi: 10.1016/j.yexcr.2014.01.024
147. Johnson JL, De Mejia EG. Flavonoid Apigenin Modified Gene Expression Associated With Inflammation and Cancer and Induced Apoptosis in Human Pancreatic Cancer Cells Through Inhibition of GSK-3β/NF-κ B Signaling Cascade. Mol Nutr Food Res (2013) 57(12):2112–27. doi: 10.1002/mnfr.201300307
148. Shukla S, Gupta S. Suppression of Constitutive and Tumor Necrosis Factor α-Induced Nuclear Factor (NF)-κb Activation and Induction of Apoptosis by Apigenin in Human Prostate Carcinoma PC-3 Cells: Correlation With Down-Regulation of NF-κb-Responsive Genes. Clin Cancer Res (2004) 10(9):3169–78. doi: 10.1158/1078-0432.CCR-03-0586
149. Erdogan S, Doganlar O, Doganlar ZB, Serttas R, Turkekul K, Dibirdik I, et al. The Flavonoid Apigenin Reduces Prostate Cancer CD44+ Stem Cell Survival and Migration Through PI3K/Akt/NF-κb Signaling. Life Sci (2016) 162:77–86. doi: 10.1016/j.lfs.2016.08.019
150. Bauer D, Mazzio E, Soliman KF. Whole Transcriptomic Analysis of Apigenin on Tnfα Immuno-Activated MDA-MB-231 Breast Cancer Cells. Cancer Genom-Proteom (2019) 16(6):421–31. doi: 10.21873/cgp.20146
151. Mafuvadze B, Liang Y, Besch-Williford C, Zhang X, Hyder SM. Apigenin Induces Apoptosis and Blocks Growth of Medroxyprogesterone Acetate-Dependent BT-474 Xenograft Tumors. Hormones Cancer (2012) 3(4):160–71. doi: 10.1007/s12672-012-0114-x
152. Shukla S, Shankar E, Fu P, MacLennan GT, Gupta S. Suppression of NF-κb and NF-κb-Regulated Gene Expression by Apigenin Through Iκbα and IKK Pathway in TRAMP Mice. PloS One (2015) 10(9):e0138710. doi: 10.1371/journal.pone.0138710
153. Chen K-C, Chen C-Y, Lin C-J, Yang T-Y, Chen T-H, Wu L-C, et al. Luteolin Attenuates TGF-β1-Induced Epithelial–Mesenchymal Transition of Lung Cancer Cells by Interfering in the PI3K/Akt–NF-κb–Snail Pathway. Life Sci (2013) 93(24):924–33. doi: 10.1016/j.lfs.2013.10.004
154. Huang W-C, Liou C-J, Shen S-C, Hu S, Hsiao C-Y, Wu S-J. Luteolin Attenuates IL-1β-Induced THP-1 Adhesion to ARPE-19 Cells via Suppression of NF-κb and MAPK Pathways. Mediators Inflammation (2020). doi: 10.1155/2020/9421340
155. Radziejewska I, Borzym-Kluczyk M, Leszczyńska K. Luteolin Alters MUC1 Extracellular Domain, sT Antigen, ADAM−17, IL−8, IL−10 and NF−κb Expression in Helicobacter Pylori−Infected Gastric Cancer CRL−1739 Cells: A Preliminary Study. Biomed Rep (2021) 14(2):1–1. doi: 10.3892/br.2020.1395
156. Park S-H, Kim J-H, Lee D-H, Kang J-W, Song H-H, Oh S-R, et al. Luteolin 8-C-β-Fucopyranoside Inhibits Invasion and Suppresses TPA-Induced MMP-9 and IL-8 via ERK/AP-1 and ERK/NF-κb Signaling in MCF-7 Breast Cancer Cells. Biochimie (2013) 95(11):2082–90. doi: 10.1016/j.biochi.2013.07.021
157. Maruthanila V, Elancheran R, Roy NK, Bhattacharya A, Kunnumakkara AB, Kabilan S, et al. In Silico Molecular Modelling of Selected Natural Ligands and Their Binding Features With Estrogen Receptor Alpha. Curr Comput-Aided Drug Des (2019) 15(1):89–96. doi: 10.2174/1573409914666181008165356
158. Du Y, Jia C, Liu Y, Wang J, Sun K. Isorhamnetin Enhances the Radiosensitivity of A549 Cells Through Interleukin-13 and the NF-κ B Signaling Pathway. Front Pharmacol (2020) 11:2324. doi: 10.3389/fphar.2020.610772
159. Hu S, Huang L, Meng L, Sun H, Zhang W, Xu Y. Isorhamnetin Inhibits Cell Proliferation and Induces Apoptosis in Breast Cancer via Akt and Mitogen−Activated Protein Kinase Kinase Signaling Pathways. Mol Med Rep (2015) 12(5):6745–51. doi: 10.3892/mmr.2015.4269
160. Liu X, Tian S, Liu M, Jian L, Zhao L. Wogonin Inhibits the Proliferation and Invasion, and Induces the Apoptosis of HepG2 and Bel7402 HCC Cells Through NF−κb/Bcl-2, EGFR and EGFR Downstream ERK/AKT Signaling. Int J Mol Med (2016) 38(4):1250–6. doi: 10.3892/ijmm.2016.2700
161. Dürr C, Hanna BS, Schulz A, Lucas F, Zucknick M, Benner A, et al. Tumor Necrosis Factor Receptor Signaling is a Driver of Chronic Lymphocytic Leukemia That can be Therapeutically Targeted by the Flavonoid Wogonin. haematologica (2018) 103(4):688. doi: 10.3324/haematol.2017.177808
162. Monteiro R, Calhau C, Silva AOE, Pinheiro-Silva S, Guerreiro S, Gärtner F, et al. Xanthohumol Inhibits Inflammatory Factor Production and Angiogenesis in Breast Cancer Xenografts. J Cell Biochem (2008) 104(5):1699–707. doi: 10.1002/jcb.21738
163. Wang Y, Chen Y, Wang J, Chen J, Aggarwal B, Pang X, et al. Xanthohumol, a Prenylated Chalcone Derived From Hops, Suppresses Cancer Cell Invasion Through Inhibiting the Expression of CXCR4 Chemokine Receptor. Curr Mol Med (2012) 12(2):153–62. doi: 10.2174/156652412798889072
164. Yamaguchi M, Murata T, Shoji M, Weitzmann MN. The Flavonoid P-Hydroxycinnamic Acid Mediates Anticancer Effects on MDA-MB-231 Human Breast Cancer Cells In Vitro: Implications for Suppression of Bone Metastases. Int J Oncol (2015) 47(4):1563–71. doi: 10.3892/ijo.2015.3106
165. Yao Y, Zhao K, Yu Z, Ren H, Zhao L, Li Z, et al. Wogonoside Inhibits Invasion and Migration Through Suppressing TRAF2/4 Expression in Breast Cancer. J Exp Clin Cancer Res (2017) 36(1):1–14. doi: 10.1186/s13046-017-0574-5
166. Cheng CY, Hu CC, Yang HJ, Lee MC, Kao ES. Inhibitory Effects of Scutellarein on Proliferation of Human Lung Cancer A549 Cells Through ERK and Nfκb Mediated by the EGFR Pathway. Chin J Physiol (2014) 57(4):182–7. doi: 10.4077/cjp.2014.Bac200
167. Yang F, Li J, Zhu J, Wang D, Chen S, Bai X. Hydroxysafflor Yellow A Inhibits Angiogenesis of Hepatocellular Carcinoma via Blocking ERK/MAPK and NF-κb Signaling Pathway in H22 Tumor-Bearing Mice. Eur J Pharmacol (2015) 754:105–14. doi: 10.1016/j.ejphar.2015.02.015
168. Moon DO, Kim MO, Lee JD, Choi YH, Kim GY. Rosmarinic Acid Sensitizes Cell Death Through Suppression of TNF-α-Induced NF-κb Activation and ROS Generation in Human Leukemia U937 Cells. Cancer Lett (2010) 288(2):183–91. doi: 10.1016/j.canlet.2009.06.033
169. Cao W, Mo K, Wei S, Lan X, Zhang W, Jiang W. Effects of Rosmarinic Acid on Immunoregulatory Activity and Hepatocellular Carcinoma Cell Apoptosis in H22 Tumor-Bearing Mice. Kor J Physiol Pharmacol (2019) 23(6):501–8. doi: 10.4196/kjpp.2019.23.6.501
170. Tsai JJ, Chen JH, Chen CH, Chung JG, Hsu FT. Apoptosis Induction and ERK/NF-κb Inactivation are Associated With Magnolol-Inhibited Tumor Progression in Hepatocellular Carcinoma In Vivo. Environ Toxicol (2020) 35(2):167–75. doi: 10.1002/tox.22853
171. Shi Q, Jiang Z, Yang J, Cheng Y, Pang Y, Zheng N, et al. A Flavonoid Glycoside Compound From Murraya Paniculata (L.) Interrupts Metastatic Characteristics of A549 Cells by Regulating STAT3/NF-κb/COX-2 and EGFR Signaling Pathways. AAPS J (2017) 19(6):1779–90. doi: 10.1208/s12248-017-0134-0
172. Bodduluru LN, Kasala ER, Barua CC, Karnam KC, Dahiya V, Ellutla M. Antiproliferative and Antioxidant Potential of Hesperetin Against Benzo (a) Pyrene-Induced Lung Carcinogenesis in Swiss Albino Mice. Chem-Biol Interact (2015) 242:345–52. doi: 10.1016/j.cbi.2015.10.020
173. Averett C, Bhardwaj A, Arora S, Srivastava SK, Khan MA, Ahmad A, et al. Honokiol Suppresses Pancreatic Tumor Growth, Metastasis and Desmoplasia by Interfering With Tumor-Stromal Cross-Talk. Carcinogenesis (2016) 37(11):1052–61. doi: 10.1093/carcin/bgw096
174. Lv X-Q, Qiao X-R, Su L, Chen S-Z. Honokiol Inhibits EMT-Mediated Motility and Migration of Human Non-Small Cell Lung Cancer Cells In Vitro by Targeting C-FLIP. Acta Pharmacol Sin (2016) 37(12):1574–86. doi: 10.1038/aps.2016.81
175. Park BB, sun Yoon J, shil Kim E, Choi J, woong Won Y, hye Choi J, et al. Inhibitory Effects of Eupatilin on Tumor Invasion of Human Gastric Cancer MKN-1 Cells. Tumor Biol (2013) 34(2):875–85. doi: 10.1007/s13277-012-0621-y
176. Serttas R, Koroglu C, Erdogan S. Eupatilin Inhibits the Proliferation and Migration of Prostate Cancer Cells Through Modulation of PTEN and NF-κb Signaling. Anticancer Agents Med Chem (2021) 21(3):372–82. doi: 10.2174/1871520620666200811113549
177. Lu H, Yang Y, Gad E, Wenner CA, Chang A, Larson ER, et al. Polysaccharide Krestin is a Novel TLR2 Agonist That Mediates Inhibition of Tumor Growth via Stimulation of CD8 T Cells and NK Cells. Clin Cancer Res (2011) 17(1):67–76. doi: 10.1158/1078-0432.CCR-10-1763
178. Jiang H, Zeng L, Dong X, Guo S, Xing J, Li Z, et al. Tilianin Extracted From Dracocephalum Moldavica L. Induces Intrinsic Apoptosis and Drives Inflammatory Microenvironment Response on Pharyngeal Squamous Carcinoma Cells via Regulating TLR4 Signaling Pathways. Front Pharmacol (2020) 11:205. doi: 10.3389/fphar.2020.00205
179. Dhanalakshmi S, Singh RP, Agarwal C, Agarwal R. Silibinin Inhibits Constitutive and TNF Alpha-Induced Activation of NF-Alpha B and Sensitizes Human Prostate Carcinoma DU145 Cells to TNF Alpha-Induced Apoptosis. Oncogene (2002) 21(11):1759–67. doi: 10.1038/sj.onc.1205240
180. Hagelgans A, Nacke B, Zamaraeva M, Siegert G, Menschikowski M. Silibinin Down-Regulates Expression of Secreted Phospholipase A2 Enzymes in Cancer Cells. Anticancer Res (2014) 34(4):1723–9.
181. Ting H, Deep G, Kumar S, Jain AK, Agarwal C, Agarwal R. Beneficial Effects of the Naturally Occurring Flavonoid Silibinin on the Prostate Cancer Microenvironment: Role of Monocyte Chemotactic Protein-1 and Immune Cell Recruitment. Carcinogenesis (2016) 37(6):589–99. doi: 10.1093/carcin/bgw039
182. Rehman MU, Tahir M, Ali F, Khan AQ, Khan R, Lateef A, et al. Chrysin Suppresses Renal Carcinogenesis via Amelioration of Hyperproliferation, Oxidative Stress and Inflammation: Plausible Role of NF-? B. Free Radical Biol Med (2012) 53):S50. doi: 10.1016/j.freeradbiomed.2012.10.133
183. Lirdprapamongkol K, Sakurai H, Abdelhamed S, Yokoyama S, Athikomkulchai S, Viriyaroj A, et al. Chrysin Overcomes TRAIL Resistance of Cancer Cells Through Mcl-1 Downregulation by Inhibiting STAT3 Phosphorylation. Int J Oncol (2013) 43(1):329–37. doi: 10.3892/ijo.2013.1926
184. Rehman MU, Tahir M, Khan AQ, Khan R, Lateef A, Oday OH, et al. Chrysin Suppresses Renal Carcinogenesis via Amelioration of Hyperproliferation, Oxidative Stress and Inflammation: Plausible Role of NF-κb. Toxicol Lett (2013) 216(2-3):146–58. doi: 10.1016/j.toxlet.2012.11.013
185. Kasala ER, Bodduluru LN, Barua CC, Madhana RM, Dahiya V, Budhani MK, et al. Chemopreventive Effect of Chrysin, a Dietary Flavone Against Benzo(a)Pyrene Induced Lung Carcinogenesis in Swiss Albino Mice. Pharmacol Rep (2016) 68(2):310–8. doi: 10.1016/j.pharep.2015.08.014
186. Dong W, Chen A, Chao X, Li X, Cui Y, Xu C, et al. Chrysin Inhibits Proinflammatory Factor-Induced EMT Phenotype and Cancer Stem Cell-Like Features in HeLa Cells by Blocking the NF-κb/Twist Axis. Cell Physiol Biochem (2019) 52(5):1236–50. doi: 10.33594/000000084
187. Rastogi N, Gara RK, Trivedi R, Singh A, Dixit P, Maurya R, et al. (6)-Gingerolinduced Myeloid Leukemia Cell Death Is Initiated by Reactive Oxygen Species and Activation of miR-27b Expression. Free Radical Biol Med (2014) 68:288–301. doi: 10.1016/j.freeradbiomed.2013.12.016
188. Ishikawa C, Senba M, Mori N. Butein Inhibits NF-κb, AP-1 and Akt Activation in Adult T-Cell Leukemia/Lymphoma. Int J Oncol (2017) 51(2):633–43. doi: 10.3892/ijo.2017.4026
189. Murtaza I, Adhami VM, Hafeez BB, Saleem M, Mukhtar H. Fisetin, a Natural Flavonoid, Targets Chemoresistant Human Pancreatic Cancer AsPC-1 Cells Through DR3-Mediated Inhibition of NF-κb. Int J Cancer (2009) 125(10):2465–73. doi: 10.1002/ijc.24628
190. Suh Y, Afaq F, Johnson JJ, Mukhtar H. A Plant Flavonoid Fisetin Induces Apoptosis in Colon Cancer Cells by Inhibition of COX2 and Wnt/EGFR/NF-κb-Signaling Pathways. Carcinogenesis (2009) 30(2):300–7. doi: 10.1093/carcin/bgn269
191. Chien CS, Shen KH, Huang JS, Ko SC, Shih YW. Antimetastatic Potential of Fisetin Involves Inactivation of the PI3K/Akt and JNK Signaling Pathways With Downregulation of MMP-2/9 Expressions in Prostate Cancer PC-3 Cells. Mol Cell Biochem (2010) 333(1-2):169–80. doi: 10.1007/s11010-009-0217-z
192. Li J, Qu W, Cheng Y, Sun Y, Jiang Y, Zou T, et al. The Inhibitory Effect of Intravesical Fisetin Against Bladder Cancer by Induction of P53 and Down-Regulation of NF-Kappa B Pathways in a Rat Bladder Carcinogenesis Model. Basic Clin Pharmacol Toxicol (2014) 115(4):321–9. doi: 10.1111/bcpt.12229
193. Yi C, Zhang Y, Yu Z, Xiao Y, Wang J, Qiu H, et al. Melatonin Enhances the Anti-Tumor Effect of Fisetin by Inhibiting COX-2/iNOS and NF-κb/P300 Signaling Pathways. PloS One (2014) 9(7):e99943. doi: 10.1371/journal.pone.0099943
194. Zhang XJ, Jia SS. Fisetin Inhibits Laryngeal Carcinoma Through Regulation of AKT/NF-κb/mTOR and ERK1/2 Signaling Pathways. BioMed Pharmacother (2016) 83:1164–74. doi: 10.1016/j.biopha.2016.08.035
195. Al-Halabi R, Bou Chedid M, Abou Merhi R, El-Hajj H, Zahr H, Schneider-Stock R, et al. Gallotannin Inhibits NFĸB Signaling and Growth of Human Colon Cancer Xenografts. Cancer Biol Ther (2011) 12(1):59–68. doi: 10.4161/cbt.12.1.15715
196. Tian Y, Li X, Li H, Lu Q, Sun G, Chen H. Astragalus Mongholicus Regulate the Toll-Like-Receptor 4 Meditated Signal Transduction of Dendritic Cells to Restrain Stomach Cancer Cells. Afr J Tradit Complement Altern (2014) 11(3):92–6. doi: 10.4314/ajtcam.v11i3.13
197. Chen M, Cai F, Zha D, Wang X, Zhang W, He Y, et al. Astragalin-Induced Cell Death is Caspase-Dependent and Enhances the Susceptibility of Lung Cancer Cells to Tumor Necrosis Factor by Inhibiting the NF-κb Pathway. Oncotarget (2017) 8(16):26941–58. doi: 10.18632/oncotarget.15264
198. Yang M, Li W-Y, Xie J, Wang Z-L, Wen Y-L, Zhao C-C, et al. Astragalin Inhibits the Proliferation and Migration of Human Colon Cancer HCT116 Cells by Regulating the NF-κb Signaling Pathway. Front Pharmacol (2021) 12. doi: 10.3389/fphar.2021.639256
199. Umesalma S, Sudhandiran G. Differential Inhibitory Effects of the Polyphenol Ellagic Acid on Inflammatory Mediators NF-κb, iNOS, COX-2, TNF-α, and IL-6 in 1, 2-Dimethylhydrazine-Induced Rat Colon Carcinogenesis. Basic Clin Pharmacol Toxicol (2010) 107(2):650–5. doi: 10.1111/j.1742-7843.2010.00565.x
200. Anitha P, Priyadarsini RV, Kavitha K, Thiyagarajan P, Nagini S. Ellagic Acid Coordinately Attenuates Wnt/β-Catenin and NF-κb Signaling Pathways to Induce Intrinsic Apoptosis in an Animal Model of Oral Oncogenesis. Eur J Nutr (2013) 52(1):75–84. doi: 10.1007/s00394-011-0288-y
201. Cheng H, Lu C, Tang R, Pan Y, Bao S, Qiu Y, et al. Ellagic Acid Inhibits the Proliferation of Human Pancreatic Carcinoma PANC-1 Cells In Vitro and In Vivo. Oncotarget (2017) 8(7):12301–10. doi: 10.18632/oncotarget.14811
202. Manna SK, Aggarwal RS, Sethi G, Aggarwal BB, Ramesh GT. Morin (3,5,7,2',4'-Pentahydroxyflavone) Abolishes Nuclear factor-kappaB Activation Induced by Various Carcinogens and Inflammatory Stimuli, Leading to Suppression of Nuclear factor-kappaB-Regulated Gene Expression and Up-Regulation of Apoptosis. Clin Cancer Res (2007) 13(7):2290–7. doi: 10.1158/1078-0432.ccr-06-2394
203. Sharma SH, Kumar JS, Chellappan DR, Nagarajan S. Molecular Chemoprevention by Morin–A Plant Flavonoid That Targets Nuclear Factor Kappa B in Experimental Colon Cancer. Biomed Pharmacother (2018) 100:367–73. doi: 10.1016/j.biopha.2018.02.035
204. Kim DM, Koo SY, Jeon K, Kim MH, Lee J, Hong CY, et al. Rapid Induction of Apoptosis by Combination of Flavopiridol and Tumor Necrosis Factor (TNF)-α or TNF-Related Apoptosis-Inducing Ligand in Human Cancer Cell Lines. Cancer Res (2003) 63(3):621–6.
205. Liu XY, Li SG, Li YY, Cheng B, Tan B, Wang G. Puerarin Inhibits Proliferation and Induces Apoptosis by Upregulation of miR-16 in Bladder Cancer Cell Line T24. Oncol Res (2018) 26(8):1227–34. doi: 10.3727/096504018x15178736525106
206. Jung YY, Lee JH, Nam D, Narula AS, Namjoshi OA, Blough BE, et al. Anti-Myeloma Effects of Icariin Are Mediated Through the Attenuation of JAK/STAT3-Dependent Signaling Cascade. Front Pharmacol (2018) 9:531. doi: 10.3389/fphar.2018.00531
207. Peerzada KJ, Faridi AH, Sharma L, Bhardwaj SC, Satti NK, Shashi B, et al. Acteoside-Mediates Chemoprevention of Experimental Liver Carcinogenesis Through STAT-3 Regulated Oxidative Stress and Apoptosis. Environ Toxicol (2016) 31(7):782–98. doi: 10.1002/tox.22089
208. Shen KH, Hung SH, Yin LT, Huang CS, Chao CH, Liu CL, et al. Acacetin, a Flavonoid, Inhibits the Invasion and Migration of Human Prostate Cancer DU145 Cells via Inactivation of the P38 MAPK Signaling Pathway. Mol Cell Biochem (2010) 333(1-2):279–91. doi: 10.1007/s11010-009-0229-8
209. Kim HR, Park CG, Jung JY. Acacetin (5,7-Dihydroxy-4'-Methoxyflavone) Exhibits In Vitro and In Vivo Anticancer Activity Through the Suppression of NF-κb/Akt Signaling in Prostate Cancer Cells. Int J Mol Med (2014) 33(2):317–24. doi: 10.3892/ijmm.2013.1571
210. Li W, Du Q, Li X, Zheng X, Lv F, Xi X, et al. Eriodictyol Inhibits Proliferation, Metastasis and Induces Apoptosis of Glioma Cells via PI3K/Akt/NF-κb Signaling Pathway. Front Pharmacol (2020) 11:114. doi: 10.3389/fphar.2020.00114
211. Lv F, Du Q, Li L, Xi X, Liu Q, Li W, et al. Eriodictyol Inhibits Glioblastoma Migration and Invasion by Reversing EMT via Downregulation of the P38 MAPK/GSK-3β/ZEB1 Pathway. Eur J Pharmacol (2021) 900:174069. doi: 10.1016/j.ejphar.2021.174069
212. Liu Y, Piao XJ, Xu WT, Zhang Y, Zhang T, Xue H, et al. Calycosin Induces Mitochondrial-Dependent Apoptosis and Cell Cycle Arrest, and Inhibits Cell Migration Through a ROS-Mediated Signaling Pathway in HepG2 Hepatocellular Carcinoma Cells. Toxicol In Vitro (2021) 70:105052. doi: 10.1016/j.tiv.2020.105052
213. Lee HJ, Auh QS, Lee YM, Kang SK, Chang SW, Lee DS, et al. Growth Inhibition and Apoptosis-Inducing Effects of Cudraflavone B in Human Oral Cancer Cells via MAPK, NF-κb, and SIRT1 Signaling Pathway. Planta Med (2013) 79(14):1298–306. doi: 10.1055/s-0033-1350619
214. Choi J, Jiang X, Jeong JB, Lee SH. Anticancer Activity of Protocatechualdehyde in Human Breast Cancer Cells. J Med Food (2014) 17(8):842–8. doi: 10.1089/jmf.2013.0159
215. Zhu H, Zou X, Wan J, Wang L, Qian K-J, Cai L-P, et al. Reversal of Cisplatin Resistance in Ovarian Cancer Cells Mediated by Naringin-Induced COX-2 Expression Through the NF-κb Signaling Pathway. Int J Clin Exp Med (2017) 10:7590–6.
216. Kim M, Miyamoto S, Yasui Y, Oyama T, Murakami A, Tanaka T. Zerumbone, a Tropical Ginger Sesquiterpene, Inhibits Colon and Lung Carcinogenesis in Mice. Int J Cancer (2009) 124(2):264–71. doi: 10.1002/ijc.23923
217. Zhang Q-Q, Zhou D-L, Ding Y, Liu H-Y, Lei Y, Fang H-Y, et al. Andrographolide Inhibits Melanoma Tumor Growth by Inactivating the TLR4/NF-κb Signaling Pathway. Melanoma Res (2014) 24(6):545–55. doi: 10.1097/CMR.0000000000000117
218. Zhang Q-Q, Ding Y, Lei Y, Qi C-L, He X-D, Lan T, et al. Andrographolide Suppress Tumor Growth by Inhibiting TLR4/NF-κb Signaling Activation in Insulinoma. Int J Biol Sci (2014) 10(4):404. doi: 10.7150/ijbs.7723
219. Zhang R, Zhao J, Xu J, Jiao DX, Wang J, Gong ZQ, et al. Andrographolide Suppresses Proliferation of Human Colon Cancer SW620 Cells Through the TLR4/NF−κb/MMP−9 Signaling Pathway. Oncol Lett (2017) 14(4):4305–10. doi: 10.3892/ol.2017.6669
220. Tang B, Tang F, Wang Z, Qi G, Liang X, Li B, et al. Upregulation of Akt/NF-κb-Regulated Inflammation and Akt/Bad-Related Apoptosis Signaling Pathway Involved in Hepatic Carcinoma Process: Suppression by Carnosic Acid Nanoparticle. Int J Nanomed (2016) 11:6401. doi: 10.2147/IJN.S101285
221. Zheng L, Jia J, Dai H, Wan L, Liu J, Hu L, et al. Triptolide-Assisted Phosphorylation of P53 Suppresses Inflammation-Induced NF-κb Survival Pathways in Cancer Cells. Mol Cell Biol (2017) 37(15):e00149–00117. doi: 10.1128/MCB.00149-17
222. Hong O-Y, Jang H-Y, Park K-H, Jeong Y-J, Kim J-S, Chae HS. Triptolide Inhibits Matrix Metalloproteinase-9 Expression and Invasion of Breast Cancer Cells Through the Inhibition of NF-κb and AP-1 Signaling Pathways. Oncol Lett (2021) 22(1):1–9. doi: 10.3892/ol.2021.12823
223. Chang HJ, Kim MH, Baek MK, Park JS, Chung IJ, Shin BA, et al. Triptolide Inhibits Tumor Promoter-Induced uPAR Expression via Blocking NF-kappaB Signaling in Human Gastric AGS Cells. Anticancer Res (2007) 27(5a):3411–7.
224. Zhu W, Ou Y, Li Y, Xiao R, Shu M, Zhou Y, et al. A Small-Molecule Triptolide Suppresses Angiogenesis and Invasion of Human Anaplastic Thyroid Carcinoma Cells via Down-Regulation of the Nuclear Factor-κb Pathway. Mol Pharmacol (2009) 75(4):812–9. doi: 10.1124/mol.108.052605
225. Wang H, Ma D, Wang C, Zhao S, Liu C. Triptolide Inhibits Invasion and Tumorigenesis of Hepatocellular Carcinoma MHCC-97h Cells Through NF-κb Signaling. Med Sci Monit (2016) 22:1827–36. doi: 10.12659/msm.898801
226. Xiang S, Zhao Z, Zhang T, Zhang B, Meng M, Cao Z, et al. Triptonide Effectively Suppresses Gastric Tumor Growth and Metastasis Through Inhibition of the Oncogenic Notch1 and NF-κb Signaling Pathways. Toxicol Appl Pharmacol (2020) 388:114870. doi: 10.1016/j.taap.2019.114870
227. Zhao J, Zheng H, Sui Z, Jing F, Quan X, Zhao W, et al. Ursolic Acid Exhibits Anti-Inflammatory Effects Through Blocking TLR4-MyD88 Pathway Mediated by Autophagy. Cytokine (2019) 123:154726. doi: 10.1016/j.cyto.2019.05.013
228. Shanmugam MK, Manu KA, Ong TH, Ramachandran L, Surana R, Bist P, et al. Inhibition of CXCR4/CXCL12 Signaling Axis by Ursolic Acid Leads to Suppression of Metastasis in Transgenic Adenocarcinoma of Mouse Prostate Model. Int J Cancer (2011) 129(7):1552–63. doi: 10.1002/ijc.26120
229. Wang J, Guo W, Chen W, Yu W, Tian Y, Fu L, et al. Melatonin Potentiates the Antiproliferative and Pro-Apoptotic Effects of Ursolic Acid in Colon Cancer Cells by Modulating Multiple Signaling Pathways. J Pineal Res (2013) 54(4):406–16. doi: 10.1111/jpi.12035
230. Luo J, Hu YL, Wang H. Ursolic Acid Inhibits Breast Cancer Growth by Inhibiting Proliferation, Inducing Autophagy and Apoptosis, and Suppressing Inflammatory Responses via the PI3K/AKT and NF-κb Signaling Pathways In Vitro. Exp Ther Med (2017) 14(4):3623–31. doi: 10.3892/etm.2017.4965
231. Saikia M, Retnakumari AP, Anwar S, Anto NP, Mittal R, Shah S, et al. Heteronemin, a Marine Natural Product, Sensitizes Acute Myeloid Leukemia Cells Towards Cytarabine Chemotherapy by Regulating Farnesylation of Ras. Oncotarget (2018) 9(26):18115–27. doi: 10.18632/oncotarget.24771
232. Chen J, Ullah H, Zheng Z, Gu X, Su C, Xiao L, et al. Soyasaponins Reduce Inflammation by Downregulating MyD88 Expression and Suppressing the Recruitments of TLR4 and MyD88 Into Lipid Rafts. BMC Complement Med Ther (2020) 20:1–16. doi: 10.1186/s12906-020-2864-2
233. Narożna M, Krajka-Kuźniak V, Bednarczyk-Cwynar B, Kleszcz R, Baer-Dubowska W. The Effect of Novel Oleanolic Acid Oximes Conjugated With Indomethacin on the Nrf2-ARE And NF-κb Signaling Pathways in Normal Hepatocytes and Human Hepatocellular Cancer Cells. Pharmaceuticals (2021) 14(1):32. doi: 10.3390/ph14010032
234. Narożna M, Krajka-Kuźniak V, Bednarczyk-Cwynar B, Kucińska M, Kleszcz R, Kujawski J, et al. Conjugation of Diclofenac With Novel Oleanolic Acid Derivatives Modulate Nrf2 and NF-κb Activity in Hepatic Cancer Cells and Normal Hepatocytes Leading to Enhancement of Its Therapeutic and Chemopreventive Potential. Pharmaceuticals (2021) 14(7):688. doi: 10.3390/ph14070688
235. Assar EA, Vidalle MC, Chopra M, Hafizi S. Lycopene Acts Through Inhibition of Iκb Kinase to Suppress NF-κb Signaling in Human Prostate and Breast Cancer Cells. Tumor Biol (2016) 37(7):9375–85. doi: 10.1007/s13277-016-4798-3
236. Madankumar A, Tamilarasi S, Premkumar T, Gopikrishnan M, Nagabhishek N, Devaki T. Geraniol Attenuates 4NQO-Induced Tongue Carcinogenesis Through Downregulating the Activation of NF-κb in Rats. Mol Cell Biochem (2017) 434(1):7–15. doi: 10.1007/s11010-017-3030-0
237. Lee K-C, Chang H-H, Chung Y-H, Lee T-Y. Andrographolide Acts as an Anti-Inflammatory Agent in LPS-Stimulated RAW264. 7 Macrophages by Inhibiting STAT3-Mediated Suppression of the NF-κb Pathway. J Ethnopharmacol (2011) 135(3):678–84. doi: 10.1016/j.jep.2011.03.068
238. Chiang K-C, Tsui K-H, Chung L-C, Yeh C-N, Chen W-T, Chang P-L, et al. Celastrol Blocks Interleukin-6 Gene Expression via Downregulation of NF-κb in Prostate Carcinoma Cells. PloS One (2014) 9(3):e93151. doi: 10.1371/journal.pone.0093151
239. Su D, Wang WB, Wu XY, Li MY, Yan XL, Hua ZH, et al. Meriolin1 Induces Cell Cycle Arrest, Apoptosis, Autophagy and Targeting the Akt/MAPKs Pathways in Human Neuroblastoma SH-SY5Y Cells. J Pharm Pharmacol (2020) 72(4):561–74. doi: 10.1111/jphp.13224
240. Einbond LS, Mighty J, Redenti S, Wu H-A. Actein Induces Calcium Release in Human Breast Cancer Cells. Fitoterapia (2013) 91:28–38. doi: 10.1016/j.fitote.2013.07.025
241. Sung B, Prasad S, Yadav VR, Aggarwal BB. Cancer Cell Signaling Pathways Targeted by Spice-Derived Nutraceuticals. Nutr Cancer (2012) 64(2):173–97. doi: 10.1080/01635581.2012.630551
242. Yadav VR, Prasad S, Sung B, Kannappan R, Aggarwal BB. Targeting Inflammatory Pathways by Triterpenoids for Prevention and Treatment of Cancer. Toxins (2010) 2(10):2428–66. doi: 10.3390/toxins2102428
243. Corbiere C, Liagre B, Terro F, Beneytout J-L. Induction of Antiproliferative Effect by Diosgenin Through Activation of P53, Release of Apoptosis-Inducing Factor (AIF) and Modulation of Caspase-3 Activity in Different Human Cancer Cells. Cell Res (2004) 14(3):188–96. doi: 10.1038/sj.cr.7290219
244. Lepage C, Liagre B, Cook-Moreau J, Pinon A, Beneytout J-L. Cyclooxygenase-2 and 5-Lipoxygenase Pathways in Diosgenin-Induced Apoptosis in HT-29 and HCT-116 Colon Cancer Cells. Int J Oncol (2010) 36(5):1183–91. doi: 10.3892/ijo_00000601
245. Lepage C, Léger DY, Bertrand J, Martin F, Beneytout JL, Liagre B. Diosgenin Induces Death Receptor-5 Through Activation of P38 Pathway and Promotes TRAIL-Induced Apoptosis in Colon Cancer Cells. Cancer Lett (2011) 301(2):193–202. doi: 10.1016/j.canlet.2010.12.003
246. Zhuang H, Dai X, Zhang X, Mao Z, Huang H. Sophoridine Suppresses Macrophage-Mediated Immunosuppression Through TLR4/IRF3 Pathway and Subsequently Upregulates CD8+ T Cytotoxic Function Against Gastric Cancer. Biomed Pharmacother (2020) 121:109636. doi: 10.1016/j.biopha.2019.109636
247. Wang J-k, Zhao B-S, Wang M, Liu C-Y, Li Y-Q, Ma Q-T, et al. Anti-Tumor and Phenotypic Regulation Effect of Matrine on Dendritic Cells Through Regulating TLRs Pathway. Chin J Integr Med (2021) 27(7):520–6. doi: 10.1007/s11655-020-3433-8
248. Yu P, Liu Q, Liu K, Yagasaki K, Wu E, Zhang G. Matrine Suppresses Breast Cancer Cell Proliferation and Invasion via VEGF-Akt-NF-κ B Signaling. Cytotechnology (2009) 59(3):219–29. doi: 10.1007/s10616-009-9225-9
249. Huang H, Du T, Xu G, Lai Y, Fan X, Chen X, et al. Matrine Suppresses Invasion of Castration-Resistant Prostate Cancer Cells by Downregulating MMP-2/9 via NF-κb Signaling Pathway. Int J Oncol (2017) 50(2):640–8. doi: 10.3892/ijo.2016.3805
250. Hai-Tao F, Wen-Wen Z, Jin-Jian L, Yi-Tao W, Xiu-Ping C. Hypaconitine Inhibits TGF-β1-Induced Epithelial–Mesenchymal Transition and Suppresses Adhesion, Migration, and Invasion of Lung Cancer A549 Cells. Chin J Natural Medicines (2017) 15(6):427–35.
251. Zhang T, Guo S, Zhu X, Qiu J, Deng G, Qiu C. Alpinetin Inhibits Breast Cancer Growth by ROS/NF-κb/HIF-1α Axis. J Cell Mol Med (2020) 24(15):8430–40. doi: 10.1111/jcmm.15371
252. Lu JJ, Fu L, Tang Z, Zhang C, Qin L, Wang J, et al. Melatonin Inhibits AP-2β/hTERT, NF-κb/COX-2 and Akt/ERK and Activates Caspase/Cyto C Signaling to Enhance the Antitumor Activity of Berberine in Lung Cancer Cells. Oncotarget (2016) 7(3):2985–3001. doi: 10.18632/oncotarget.6407
253. Li J, Liu F, Jiang S, Liu J, Chen X, Zhang S, et al. Berberine Hydrochloride Inhibits Cell Proliferation and Promotes Apoptosis of Non-Small Cell Lung Cancer via the Suppression of the MMP2 and Bcl-2/Bax Signaling Pathways. Oncol Lett (2018) 15(5):7409–14. doi: 10.3892/ol.2018.8249
254. Kumar R, Awasthi M, Sharma A, Padwad Y, Sharma R. Berberine Induces Dose-Dependent Quiescence and Apoptosis in A549 Cancer Cells by Modulating Cell Cyclins and Inflammation Independent of mTOR Pathway. Life Sci (2020) 244:117346. doi: 10.1016/j.lfs.2020.117346
255. Yao M, Fan X, Yuan B, Takagi N, Liu S, Han X, et al. Berberine Inhibits NLRP3 Inflammasome Pathway in Human Triple-Negative Breast Cancer MDA-MB-231 Cell. BMC Complement Altern Med (2019) 19(1):1–11. doi: 10.1186/s12906-019-2615-4
256. Ho Y-T, Yang J-S, Li T-C, Lin J-J, Lin J-G, Lai K-C, et al. Berberine Suppresses In Vitro Migration and Invasion of Human SCC-4 Tongue Squamous Cancer Cells Through the Inhibitions of FAK, IKK, NF-κb, U-PA and MMP-2 and -9. Cancer Lett (2009) 279(2):155–62. doi: 10.1016/j.canlet.2009.01.033
257. Karnam KC, Ellutla M, Bodduluru LN, Kasala ER, Uppulapu SK, Kalyankumarraju M, et al. Preventive Effect of Berberine Against DMBA-Induced Breast Cancer in Female Sprague Dawley Rats. Biomed Pharmacother (2017) 92:207–14. doi: 10.1016/j.biopha.2017.05.069
258. Zhao L, Zhang C. Berberine Inhibits MDA-MB-231 Cells by Attenuating Their Inflammatory Responses. BioMed Res Int (2020). doi: 10.1155/2020/3617514
259. Fu L, Chen W, Guo W, Wang J, Tian Y, Shi D, et al. Berberine Targets AP-2/hTERT, NF-κb/COX-2, HIF-1α/VEGF and Cytochrome-C/Caspase Signaling to Suppress Human Cancer Cell Growth. PloS One (2013) 8(7):e69240. doi: 10.1371/journal.pone.0069240
260. Wang Y, Zhou M, Shang D. Berberine Inhibits Human Gastric Cancer Cell Growth via Deactivation of P38/JNK Pathway, Induction of Mitochondrial-Mediated Apoptosis, Caspase Activation and NF-κb Inhibition. J BUON (2020) 25(1):314–8.
261. Li P, Liu Y, He Q. Anisodamine Suppressed the Growth of Hepatocellular Carcinoma Cells, Induced Apoptosis and Regulated the Levels of Inflammatory Factors by Inhibiting NLRP3 Inflammasome Activation. Drug Des Dev Ther (2020) 14:1609. doi: 10.2147/DDDT.S243383
262. Ghezali L, Leger DY, Limami Y, Cook-Moreau J, Beneytout J-L. Cyclopamine and Jervine Induce COX-2 Overexpression in Human Erythroleukemia Cells But Only Cyclopamine has a Pro-Apoptotic Effect. Exp Cell Res (2013) 319(7):1043–53. doi: 10.1016/j.yexcr.2013.01.014
263. Xu W, Wang X, Tu Y, Masaki H, Tanaka S, Onda K, et al. Tetrandrine and Cepharanthine Induce Apoptosis Through Caspase Cascade Regulation, Cell Cycle Arrest, MAPK Activation and PI3K/Akt/mTOR Signal Modification in Glucocorticoid Resistant Human Leukemia Jurkat T Cells. Chem-Biol Interact (2019) 310. doi: 10.1016/j.cbi.2019.108726
264. Ginzburg S, Golovine KV, Makhov PB, Uzzo RG, Kutikov A, Kolenko VM. Piperlongumine Inhibits NF-κb Activity and Attenuates Aggressive Growth Characteristics of Prostate Cancer Cells. Prostate (2014) 74(2):177–86. doi: 10.1002/pros.22739
265. Han JG, Gupta SC, Prasad S, Aggarwal BB. Piperlongumine Chemosensitizes Tumor Cells Through Interaction With Cysteine 179 of Iκbα Kinase, Leading to Suppression of NF-κb-Regulated Gene Products. Mol Cancer Ther (2014) 13(10):2422–35. doi: 10.1158/1535-7163.mct-14-0171
266. Golovine K, Makhov P, Naito S, Raiyani H, Tomaszewski J, Mehrazin R, et al. Piperlongumine and its Analogs Down-Regulate Expression of C-Met in Renal Cell Carcinoma. Cancer Biol Ther (2015) 16(5):743–9. doi: 10.1080/15384047.2015.1026511
267. Seok JS, Jeong CH, Petriello MC, Seo HG, Yoo H, Hong K, et al. Piperlongumine Decreases Cell Proliferation and the Expression of Cell Cycle-Associated Proteins by Inhibiting Akt Pathway in Human Lung Cancer Cells. Food Chem Toxicol (2018) 111:9–18. doi: 10.1016/j.fct.2017.10.058
268. Kumar S, Agnihotri N. Piperlongumine Targets NF-κb and its Downstream Signaling Pathways to Suppress Tumor Growth and Metastatic Potential in Experimental Colon Cancer. Mol Cell Biochem (2021) 476(4):1765–81. doi: 10.1007/s11010-020-04044-7
269. Hamsa T, Kuttan G. Harmine Inhibits Tumour Specific Neo-Vessel Formation by Regulating VEGF, MMP, TIMP and Pro-Inflammatory Mediators Both In Vivo and In Vitro. Eur J Pharmacol (2010) 649(1-3):64–73. doi: 10.1016/j.ejphar.2010.09.010
270. Jung YY, Shanmugam MK, Chinnathambi A, Alharbi SA, Shair OH, Um J-Y, et al. Fangchinoline, a Bisbenzylisoquinoline Alkaloid can Modulate Cytokine-Impelled Apoptosis via the Dual Regulation of NF-κb and AP-1 Pathways. Molecules (2019) 24(17):3127. doi: 10.3390/molecules24173127
271. Guo Y, Ding Y, Zhang T, An H. Sinapine Reverses Multi-Drug Resistance in MCF-7/Dox Cancer Cells by Downregulating FGFR4/FRS2α-ERK1/2 Pathway-Mediated NF-κb Activation. Phytomedicine (2016) 23(3):267–73. doi: 10.1016/j.phymed.2015.12.017
272. Ramu A, Kathiresan S, Ramadoss H, Nallu A, Kaliyan R, Azamuthu T. Gramine Attenuates EGFR-Mediated Inflammation and Cell Proliferation in Oral Carcinogenesis via Regulation of NF-κb and STAT3 Signaling. Biomed Pharmacother (2018) 98:523–30. doi: 10.1016/j.biopha.2017.12.049
273. Harada K, Ferdous T, Itashiki Y, Takii M, Mano T, Mori Y, et al. Cepharanthine Inhibits Angiogenesis and Tumorigenicity of Human Oral Squamous Cell Carcinoma Cells by Suppressing Expression of Vascular Endothelial Growth Factor and Interleukin-8. Int J Oncol (2009) 35(5):1025–35. doi: 10.3892/ijo_00000417
274. Han SZ, Liu HX, Yang LQ, Cui LD, Xu Y. Piperine (PP) Enhanced Mitomycin-C (MMC) Therapy of Human Cervical Cancer Through Suppressing Bcl-2 Signaling Pathway via Inactivating STAT3/NF-κb. BioMed Pharmacother (2017) 96:1403–10. doi: 10.1016/j.biopha.2017.11.022
275. Rehman MU, Rashid S, Arafah A, Qamar W, Alsaffar RM, Ahmad A, et al. Piperine Regulates Nrf-2/Keap-1 Signalling and Exhibits Anticancer Effect in Experimental Colon Carcinogenesis in Wistar Rats. Biology (2020) 9(9):302. doi: 10.3390/biology9090302
276. Zhang N, Wang D, Zhu Y, Wang J, Lin H. Inhibition Effects of Lamellarin D on Human Leukemia K562 Cell Proliferation and Underlying Mechanisms. Asian Pac J Cancer Prev (2014) 15(22):9915–9. doi: 10.7314/APJCP.2014.15.22.9915
277. Hamsa T, Kuttan G. Ipobscurine, an Indole Alkaloid From Ipomoea Obscura, Inhibits Tumor Cell Invasion and Experimental Metastasis by Inducing Apoptosis. J Environ Pathol Toxicol Oncol (2011) 30(2):163–78. doi: 10.1615/JEnvironPatholToxicolOncol.v30.i2.70
278. Yang B, Zhang D, Qian J, Cheng Y. Chelerythrine Suppresses Proliferation and Metastasis of Human Prostate Cancer Cells via Modulating MMP/TIMP/NF-κb System. Mol Cell Biochem (2020) 474(1-2):199–208. doi: 10.1007/s11010-020-03845-0
279. Silva TCC, de Faria Lopes GP, de J.M.-F.N., de Oliveira DM, Pereira E, Pitanga BPS, et al. Specific Cytostatic and Cytotoxic Effect of Dihydrochelerythrine in Glioblastoma Cells: Role of NF-κb/β-Catenin and STAT3/IL-6 Pathways. Anticancer Agents Med Chem (2018) 18(10):1386–93. doi: 10.2174/1871520618666180412122101
280. Zeng Q, Luo C, Cho J, Lai D, Shen X, Zhang X, et al. Tryptanthrin Exerts Anti-Breast Cancer Effects Both In Vitro and In Vivo Through Modulating the Inflammatory Tumor Microenvironment. Acta Pharm (2021) 71(2):245–66. doi: 10.2478/acph-2021-0020
281. Sivalingam K, Amirthalingam V, Ganasan K, Huang CY, Viswanadha VP. Neferine Suppresses Diethylnitrosamine-Induced Lung Carcinogenesis in Wistar Rats. Food Chem Toxicol (2019) 123:385–98. doi: 10.1016/j.fct.2018.11.014
282. Zhu J, Ghosh A, Coyle EM, Lee J, Hahm E-R, Singh SV, et al. Differential Effects of Phenethyl Isothiocyanate and D, L-Sulforaphane on TLR3 Signaling. J Immunol (2013) 190(8):4400–7. doi: 10.4049/jimmunol.1202093
283. Lee Y-R, Noh E-M, Han J-H, Kim J-M, Hwang B-M, Kim B-S, et al. Sulforaphane Controls TPA-Induced MMP-9 Expression Through the NF-κb Signaling Pathway, But Not AP-1, in MCF-7 Breast Cancer Cells. BMB Rep (2013) 46(4):201. doi: 10.5483/bmbrep.2013.46.4.160
284. Gong K, Li W. Shikonin, a Chinese Plant-Derived Naphthoquinone, Induces Apoptosis in Hepatocellular Carcinoma Cells Through Reactive Oxygen Species: A Potential New Treatment for Hepatocellular Carcinoma. Free Radic Biol Med (2011) 51(12):2259–71. doi: 10.1016/j.freeradbiomed.2011.09.018
285. Liu JP, Liu D, Gu JF, Zhu MM, Cui L. Shikonin Inhibits the Cell viability, Adhesion, Invasion and Migration of the Human Gastric Cancer Cell Line MGC-803 via the Toll-Like Receptor 2/Nuclear Factor-Kappa B Pathway. J Pharm Pharmacol (2015) 67(8):1143–55. doi: 10.1111/jphp.12402
286. Krajka-Kuźniak V, Cykowiak M, Szaefer H, Kleszcz R, Baer-Dubowska W. Combination of Xanthohumol and Phenethyl Isothiocyanate Inhibits NF-κb and Activates Nrf2 in Pancreatic Cancer Cells. Toxicol Vitro (2020) 65:104799. doi: 10.1016/j.tiv.2020.104799
287. Benelli R, Ven R, Ciarlo M, Carlone S, Barbieri O, Ferrari N. The AKT/NF-κb Inhibitor Xanthohumol is a Potent Anti-Lymphocytic Leukemia Drug Overcoming Chemoresistance and Cell Infiltration. Biochem Pharmacol (2012) 83(12):1634–42. doi: 10.1016/j.bcp.2012.03.006
288. Yang MD, Lai KC, Lai TY, Hsu SC, Kuo CL, Yu CS, et al. Phenethyl Isothiocyanate Inhibits Migration and Invasion of Human Gastric Cancer AGS Cells Through Suppressing MAPK and NF-κb Signal Pathways. Anticancer Res (2010) 30(6):2135–43.
289. Wang C, Li S, Wang MW. Evodiamine-Induced Human Melanoma A375-S2 Cell Death was Mediated by PI3K/Akt/caspase and Fas-L/NF-kappaB Signaling Pathways and Augmented by Ubiquitin-Proteasome Inhibition. Toxicol In Vitro (2010) 24(3):898–904. doi: 10.1016/j.tiv.2009.11.019
290. Lin L, Ren L, Wen L, Wang Y, Qi J. Effect of Evodiamine on the Proliferation and Apoptosis of A549 Human Lung Cancer Cells. Mol Med Rep (2016) 14(3):2832–8. doi: 10.3892/mmr.2016.5575
291. Hwang Y, Kim HC, Shin EJ. Enhanced Neurogenesis Is Involved in Neuroprotection Provided by Rottlerin Against Trimethyltin-Induced Delayed Apoptotic Neuronal Damage. Life Sci (2020) 262:118494. doi: 10.1016/j.lfs.2020.118494
292. Fan X, Deng J, Shi T, Wen H, Li J, Liang Z, et al. Design, Synthesis and Bioactivity Study of Evodiamine Derivatives as Multifunctional Agents for the Treatment of Hepatocellular Carcinoma. Bioorg Chem (2021) 114:105154. doi: 10.1016/j.bioorg.2021.105154
293. Guzmán EA, Maers K, Roberts J, Kemami-Wangun HV, Harmody D, Wright AE. The Marine Natural Product Microsclerodermin A Is a Novel Inhibitor of the Nuclear Factor Kappa B and Induces Apoptosis in Pancreatic Cancer Cells. Investigat N Drugs (2015) 33(1):86–94. doi: 10.1007/s10637-014-0185-3
294. Sperlich J, Kerr R, Teusch N. The Marine Natural Product Pseudopterosin Blocks Cytokine Release of Triple-Negative Breast Cancer and Monocytic Leukemia Cells by Inhibiting NF-κb Signaling. Mar Drugs (2017) 15(9):262. doi: 10.3390/md15090262
295. Chen H, Zhang J, Luo J, Lai F, Wang Z, Tong H, et al. Antiangiogenic Effects of Oxymatrine on Pancreatic Cancer by Inhibition of the NF-κb-Mediated VEGF Signaling Pathway. Oncol Rep (2013) 30(2):589–95. doi: 10.3892/or.2013.2529
296. Li Q, Lai Y, Wang C, Xu G, He Z, Shang X, et al. Matrine Inhibits the Proliferation, Invasion and Migration of Castration-Resistant Prostate Cancer Cells Through Regulation of the NF-κb Signaling Pathway. Oncol Rep (2016) 35(1):375–81. doi: 10.3892/or.2015.4341
297. Huang H, Wang Q, Du T, Lin C, Lai Y, Zhu D, et al. Matrine Inhibits the Progression of Prostate Cancer by Promoting Expression of GADD45B. Prostate (2018) 78(5):327–35. doi: 10.1002/pros.23469
298. Jung YY, Um J-Y, Narula AS, Namjoshi OA, Blough BE, Kumar AP, et al. Identification of Matrine as a Novel Regulator of the Cxcr4 Signaling Axis in Tumor Cells. Int J Mol Sci (2020) 21(13):4731. doi: 10.3390/ijms21134731
299. Sp N, Kang DY, Kim DH, Park JH, Lee HG, Kim HJ, et al. Nobiletin Inhibits CD36-Dependent Tumor Angiogenesis, Migration, Invasion, and Sphere Formation Through the Cd36/Stat3/Nf-Kb Signaling Axis. Nutrients (2018) 10(6):772. doi: 10.3390/nu10060772
300. Chen J, Chen AY, Huang H, Ye X, Rollyson WD, Perry HE, et al. The Flavonoid Nobiletin Inhibits Tumor Growth and Angiogenesis of Ovarian Cancers via the Akt Pathway. Int J Oncol (2015) 46(6):2629–38. doi: 10.3892/ijo.2015.2946
301. Ozkan AD, Sarihan M, Kaleli S. Evaluation of the Effects of Nobiletin on Toll-Like Receptor 3 Signaling Pathways in Prostate Cancer In Vitro. Nutr Cancer (2021) 73(7):1138–44. doi: 10.1080/01635581.2020.1841247
302. Lee YC, Cheng TH, Lee JS, Chen JH, Liao YC, Fong Y, et al. Nobiletin, a Citrus Flavonoid, Suppresses Invasion and Migration Involving FAK/PI3K/Akt and Small GTPase Signals in Human Gastric Adenocarcinoma AGS Cells. Mol Cell Biochem (2011) 347(1-2):103–15. doi: 10.1007/s11010-010-0618-z
303. Lee S-J, Lim K-T. Inhibitory Effect of Phytoglycoprotein on Tumor Necrosis Factor-α and Interleukin-6 at Initiation Stage of Colon Cancer in 1, 2-Dimethylhydrazine-Treated ICR Mice. Toxicol Appl Pharmacol (2007) 225(2):198–205. doi: 10.1016/j.taap.2007.07.010
304. Lee J-S, Kang Y, Kim JT, Thapa D, Lee E-S, Kim J-A. The Anti-Angiogenic and Anti-Tumor Activity of Synthetic Phenylpropenone Derivatives Is Mediated Through the Inhibition of Receptor Tyrosine Kinases. Eur J Pharmacol (2012) 677(1-3):22–30. doi: 10.1016/j.ejphar.2011.12.012
305. Kang K-H, Kong C-S, Seo Y, Kim M-M, Kim S-K. Anti-Inflammatory Effect of Coumarins Isolated From Corydalis Heterocarpa in HT-29 Human Colon Carcinoma Cells. Food Chem Toxicol (2009) 47(8):2129–34. doi: 10.1016/j.fct.2009.05.036
306. Zhu H-C, Jia X-K, Fan Y, Xu S-H, Li X-Y, Huang M-Q, et al. Alisol B 23-Acetate Ameliorates Azoxymethane/Dextran Sodium Sulfate-Induced Male Murine Colitis-Associated Colorectal Cancer via Modulating the Composition of Gut Microbiota and Improving Intestinal Barrier. Front Cell Infect Microbiol (2021) 11. doi: 10.3389/fcimb.2021.640225
307. Mehta R, Katta H, Alimirah F, Patel R, Murillo G, Peng X, et al. Deguelin Action Involves C-Met and EGFR Signaling Pathways in Triple Negative Breast Cancer Cells. PloS One (2013) 8(6):e65113. doi: 10.1371/journal.pone.0065113
308. Shiragannavar VD, Gowda NGS, Kumar DP, Mirshahi F, Santhekadur PK. Withaferin A Acts as a Novel Regulator of Liver X Receptor-α in HCC. Front Oncol (2021) 10:3124. doi: 10.3389/fonc.2020.628506
309. Ngoungoure FP, Owona BA. Withaferin A Modulates AIM2 Inflammasome and Caspase-1 Expression in THP-1 Polarized Macrophages. Exp Cell Res (2019) 383(2):111564. doi: 10.1016/j.yexcr.2019.111564
310. Kim Y-J, Jeon Y, Kim T, Lim W-C, Ham J, Park YN, et al. Combined Treatment With Zingerone and its Novel Derivative Synergistically Inhibits TGF-β1 Induced Epithelial-Mesenchymal Transition, Migration and Invasion of Human Hepatocellular Carcinoma Cells. Bioorg Med Chem Lett (2017) 27(4):1081–8. doi: 10.1016/j.bmcl.2016.12.042
311. Kao SJ, Su JL, Chen CK, Yu MC, Bai KJ, Chang JH, et al. Osthole Inhibits the Invasive Ability of Human Lung Adenocarcinoma Cells via Suppression of NF-κb-Mediated Matrix Metalloproteinase-9 Expression. Toxicol Appl Pharmacol (2012) 261(1):105–15. doi: 10.1016/j.taap.2012.03.020
312. Feng H, Lu J-J, Wang Y, Pei L, Chen X. Osthole Inhibited TGF β-Induced Epithelial–Mesenchymal Transition (EMT) by Suppressing NF-κb Mediated Snail Activation in Lung Cancer A549 Cells. Cell Adhes Migr (2017) 11(5-6):464–75. doi: 10.1080/19336918.2016.1259058
313. Che Y, Li J, Li Z, Li J, Wang S, Yan Y, et al. Osthole Enhances Antitumor Activity and Irradiation Sensitivity of Cervical Cancer Cells by Suppressing ATM/Nf−κb Signaling. Oncol Rep (2018) 40(2):737–47. doi: 10.3892/or.2018.6514
314. Lee JH, Kim C, Lee S-G, Yang WM, Um J-Y, Sethi G, et al. Ophiopogonin D Modulates Multiple Oncogenic Signaling Pathways, Leading to Suppression of Proliferation and Chemosensitization of Human Lung Cancer Cells. Phytomedicine (2018) 40:165–75. doi: 10.1016/j.phymed.2018.01.002
315. Lee H, Ko JH, Baek SH, Nam D, Lee SG, Lee J, et al. Embelin Inhibits Invasion and Migration of MDA-MB-231 Breast Cancer Cells by Suppression of CXC Chemokine Receptor 4, Matrix Metalloproteinases-9/2, and Epithelial–Mesenchymal Transition. Phytother Res (2016) 30(6):1021–32. doi: 10.1002/ptr.5612
316. Hafeez BB, Jamal MS, Fischer JW, Mustafa A, Verma AK. Plumbagin, a Plant Derived Natural Agent Inhibits the Growth of Pancreatic Cancer Cells in In Vitro and In Vivo via Targeting EGFR, Stat3 and NF-κb Signaling Pathways. Int J Cancer (2012) 131(9):2175–86. doi: 10.1002/ijc.27478
317. Li J, Shen L, Lu FR, Qin Y, Chen R, Li J, et al. Plumbagin Inhibits Cell Growth and Potentiates Apoptosis in Human Gastric Cancer Cells In Vitro Through the NF-κb Signaling Pathway. Acta Pharmacol Sin (2012) 33(2):242–9. doi: 10.1038/aps.2011.152
318. Salem AZ, Medhat D, Fathy SA, Mohamed MR, El-Khayat Z, El-Daly SM. Indole Glucosinolates Exhibit Anti-Inflammatory Effects on Ehrlich Ascites Carcinoma Cells Through Modulation of Inflammatory Markers and miRNAs. Mol Biol Rep (2021) 48(10):6845–55. doi: 10.1007/s11033-021-06683-5
319. Sethi G, Ahn KS, Aggarwal BB. Targeting Nuclear Factor-κb Activation Pathway by Thymoquinone: Role in Suppression of Antiapoptotic Gene Products and Enhancement of Apoptosis. Mol Cancer Res (2008) 6(6):1059–70. doi: 10.1158/1541-7786.MCR-07-2088
320. Ahmad I, Muneer KM, Tamimi IA, Chang ME, Ata MO, Yusuf N. Thymoquinone Suppresses Metastasis of Melanoma Cells by Inhibition of NLRP3 Inflammasome. Toxicol Appl Pharmacol (2013) 270(1):70–6. doi: 10.1016/j.taap.2013.03.027
321. Kim W-J, Lee M-Y, Kim J-H, Suk K, Lee W-H. Decursinol Angelate Blocks Transmigration and Inflammatory Activation of Cancer Cells Through Inhibition of PI3K, ERK and NF-κb Activation. Cancer Lett (2010) 296(1):35–42. doi: 10.1016/j.canlet.2010.03.012
322. Liu Y, Zhang L, Zhu X, Wang Y, Liu W, Gong W. Polysaccharide Agaricus Blazei Murill Stimulates Myeloid Derived Suppressor Cell Differentiation From M2 to M1 Type, Which Mediates Inhibition of Tumour Immune-Evasion via the Toll-Like Receptor 2 Pathway. Immunology (2015) 146(3):379–91. doi: 10.1111/imm.12508
323. Verma A, Ahmed B, Anwar F, Rahman M, Patel DK, Kaithwas G, et al. Novel Glycoside From Wedelia Calendulacea Inhibits Diethyl Nitrosamine-Induced Renal Cancer via Downregulating the COX-2 and PEG 2 Through Nuclear Factor-κb Pathway. Inflammopharmacology (2017) 25(1):159–75. doi: 10.1007/s10787-017-0310-y
324. Choudhari AS, Mandave PC, Deshpande M, Ranjekar P, Prakash O. Phytochemicals in Cancer Treatment: From Preclinical Studies to Clinical Practice. Front Pharmacol (2020) 10:1614. doi: 10.3389/fphar.2019.01614
325. Rizeq B, Gupta I, Ilesanmi J, AlSafran M, Rahman MM, Ouhtit A. The Power of Phytochemicals Combination in Cancer Chemoprevention. J Cancer (2020) 11(15):4521. doi: 10.7150/jca.34374
326. Dehelean CA, Marcovici I, Soica C, Mioc M, Coricovac D, Iurciuc S, et al. Plant-Derived Anticancer Compounds as New Perspectives in Drug Discovery and Alternative Therapy. Molecules (2021) 26(4):1109. doi: 10.3390/molecules26041109
327. Sreekanth C, Bava S, Sreekumar E, Anto R. Molecular Evidences for the Chemosensitizing Efficacy of Liposomal Curcumin in Paclitaxel Chemotherapy in Mouse Models of Cervical Cancer. Oncogene (2011) 30(28):3139–52. doi: 10.1038/onc.2011.23
328. Quispe-Soto ET, Calaf GM. Effect of Curcumin and Paclitaxel on Breast Carcinogenesis. Int J Oncol (2016) 49(6):2569–77. doi: 10.3892/ijo.2016.3741
329. Calaf GM, Ponce-Cusi R, Carrión F. Curcumin and Paclitaxel Induce Cell Death in Breast Cancer Cell Lines. Oncol Rep (2018) 40(4):2381–8. doi: 10.3892/or.2018.6603
330. Dang Y-P, Yuan X-Y, Tian R, Li D-G, Liu W. Curcumin Improves the Paclitaxel−Induced Apoptosis of HPV−positive Human Cervical Cancer Cells via the NF−κb−P53−Caspase−3 Pathway. Exp Ther Med (2015) 9(4):1470–6. doi: 10.3892/etm.2015.2240
331. Roy M, Mukherjee S, Sarkar R, Biswas J. Curcumin Sensitizes Chemotherapeutic Drugs via Modulation of PKC, Telomerase, NF-κb and HDAC in Breast Cancer. Ther Deliv (2011) 2(10):1275–93. doi: 10.4155/tde.11.97
332. de Porras VR, Bystrup S, Martínez-Cardús A, Pluvinet R, Sumoy L, Howells L, et al. Curcumin Mediates Oxaliplatin-Acquired Resistance Reversion in Colorectal Cancer Cell Lines Through Modulation of CXC-Chemokine/NF-κb Signalling Pathway. Sci Rep (2016) 6(1):1–17. doi: 10.1038/srep24675
333. Wang YT, Liu HS, Su CL. Curcumin-Enhanced Chemosensitivity of FDA-Approved Platinum (II)-Based Anti-Cancer Drugs Involves Downregulation of Nuclear Endonuclease G and NF-κb as Well as Induction of Apoptosis and G2/M Arrest. Int J Food Sci Nutr (2014) 65(3):368–74. doi: 10.3109/09637486.2013.871694
334. Nautiyal J, Banerjee S, Kanwar SS, Yu Y, Patel BB, Sarkar FH, et al. Curcumin Enhances Dasatinib-Induced Inhibition of Growth and Transformation of Colon Cancer Cells. Int J Cancer (2011) 128(4):951–61. doi: 10.1002/ijc.25410
335. Jiang M, Huang O, Zhang X, Xie Z, Shen A, Liu H, et al. Curcumin Induces Cell Death and Restores Tamoxifen Sensitivity in the Antiestrogen-Resistant Breast Cancer Cell Lines MCF-7/LCC2 and MCF-7/Lcc9. Molecules (2013) 18(1):701–20. doi: 10.3390/molecules18010701
336. Thulasiraman P, McAndrews DJ, Mohiudddin IQ. Curcumin Restores Sensitivity to Retinoic Acid in Triple Negative Breast Cancer Cells. BMC Cancer (2014) 14:724. doi: 10.1186/1471-2407-14-724
337. Wang WJ, Wang SZ, Liu TL, Ma Y, Huang SJ, Lei L, et al. Resveratrol: Multi-Targets Mechanism on Neurodegenerative Diseases Based on Network Pharmacology. Front Pharmacol (2020) 11:694. doi: 10.3389/fphar.2020.00694
338. Hu XL, Guo LP, Song Q, Zhang Q, Chen Y, Wang J, et al. Kukoamine B, an Amide Alkaloid, Protects Against NMDA-Induced Neurotoxicity and Potential Mechanisms In Vitro. Neurochem Int (2015) 87:66–76. doi: 10.1016/j.neuint.2015.06.001
339. Martínez-Martínez D, Soto A, Gil-Araujo B, Gallego B, Chiloeches A, Lasa M. Resveratrol Promotes Apoptosis Through the Induction of Dual Specificity Phosphatase 1 and Sensitizes Prostate Cancer Cells to Cisplatin. Food Chem Toxicol (2019) 124:273–9. doi: 10.1016/j.fct.2018.12.014
340. Karthikeyan S, Hoti SL, Prasad NR. Resveratrol Loaded Gelatin Nanoparticles Synergistically Inhibits Cell Cycle Progression and Constitutive NF-kappaB Activation, and Induces Apoptosis in Non-Small Cell Lung Cancer Cells. Biomed Pharmacother (2015) 70:274–82. doi: 10.1016/j.biopha.2015.02.006
341. Buhrmann C, Shayan P, Kraehe P, Popper B, Goel A, Shakibaei M. Resveratrol Induces Chemosensitization to 5-Fluorouracil Through Up-Regulation of Intercellular Junctions, Epithelial-To-Mesenchymal Transition and Apoptosis in Colorectal Cancer. Biochem Pharmacol (2015) 98(1):51–68. doi: 10.1016/j.bcp.2015.08.105
342. Harikumar KB, Kunnumakkara AB, Sethi G, Diagaradjane P, Anand P, Pandey MK, et al. Resveratrol, a Multitargeted Agent, can Enhance Antitumor Activity of Gemcitabine In Vitro and in Orthotopic Mouse Model of Human Pancreatic Cancer. Int J Cancer (2010) 127(2):257–68. doi: 10.1002/ijc.25041
343. Xu Y, Xin Y, Diao Y, Lu C, Fu J, Luo L, et al. Synergistic Effects of Apigenin and Paclitaxel on Apoptosis of Cancer Cells. PloS One (2011) 6(12):e29169. doi: 10.1371/journal.pone.0029169
344. Hasani NAH, Amin IM, Kamaludin R, Rosdyd N.M.M.N.M., Ibahim MJ, Kadir SHSA. P53 and Cyclin B1 Mediate Apoptotic Effects of Apigenin AND Rutin in ERï¡+-Breast Cancer MCF-7 Cells. J Teknologi (2018) 80(1). doi: 10.11113/jt.v80.10704
345. Lee SH, Ryu JK, Lee K-Y, Woo SM, Park JK, Yoo JW, et al. Enhanced Anti-Tumor Effect of Combination Therapy With Gemcitabine and Apigenin in Pancreatic Cancer. Cancer Lett (2008) 259(1):39–49. doi: 10.1016/j.canlet.2007.09.015
346. Strouch MJ, Milam BM, Melstrom LG, McGill JJ, Salabat MR, Ujiki MB, et al. The Flavonoid Apigenin Potentiates the Growth Inhibitory Effects of Gemcitabine and Abrogates Gemcitabine Resistance in Human Pancreatic Cancer Cells. Pancreas (2009) 38(4):409–15. doi: 10.1097/MPA.0b013e318193a074
347. Ayyildiz A, Koc H, Turkekul K, Erdogan S. Co-Administration of Apigenin With Doxorubicin Enhances Anti-Migration and Antiproliferative Effects via PI3K/PTEN/AKT Pathway in Prostate Cancer Cells. Exp Oncol (2021) 43(2):125–34. doi: 10.32471/exp-oncology.2312-8852
348. Erdogan S, Turkekul K, Serttas R, Erdogan Z. The Natural Flavonoid Apigenin Sensitizes Human CD44+ Prostate Cancer Stem Cells to Cisplatin Therapy. Biomed Pharmacother (2017) 88:210–7. doi: 10.1016/j.biopha.2017.01.056
349. Liu M, Fu M, Yang X, Jia G, Shi X, Ji J, et al. Paclitaxel and Quercetin Co-Loaded Functional Mesoporous Silica Nanoparticles Overcoming Multidrug Resistance in Breast Cancer. Colloids Surf B Biointerf (2020) 196:111284. doi: 10.1016/j.colsurfb.2020.111284
350. Zhang X, Huang J, Yu C, Xiang L, Li L, Shi D, et al. Quercetin Enhanced Paclitaxel Therapeutic Effects Towards PC-3 Prostate Cancer Through ER Stress Induction and ROS Production. OncoTargets Ther (2020) 13:513. doi: 10.2147/OTT.S228453
351. Tan XH, Zhang KK, Xu JT, Qu D, Chen LJ, Li JH, et al. Luteolin Alleviates Methamphetamine-Induced Neurotoxicity by Suppressing PI3K/Akt Pathway-Modulated Apoptosis and Autophagy in Rats. Food Chem Toxicol (2020) 137. doi: 10.1016/j.fct.2020.111179
352. Li X, Guo S, Xiong XK, Peng BY, Huang JM, Chen MF, et al. Combination of Quercetin and Cisplatin Enhances Apoptosis in OSCC Cells by Downregulating xIAP Through the NF-κb Pathway. J Cancer (2019) 10(19):4509–21. doi: 10.7150/jca.31045
353. Oršolić N, Odeh D, Jembrek MJ, Knežević J, Kučan D. Interactions Between Cisplatin and Quercetin at Physiological and Hyperthermic Conditions on Cancer Cells In Vitro and In Vivo. Molecules (2020) 25(14):3271. doi: 10.3390/molecules25143271
354. Han Y, Yu H, Wang J, Ren Y, Su X, Shi Y. Quercetin Alleviates Myocyte Toxic and Sensitizes Anti-Leukemic Effect of Adriamycin. Hematol (Amsterdam Netherlands) (2015) 20(5):276–83. doi: 10.1179/1607845414Y.0000000198
355. Liu Z-j, Xu W, Han J, Liu Q-Y, Gao L-F, Wang X-H, et al. Quercetin Induces Apoptosis and Enhances Gemcitabine Therapeutic Efficacy Against Gemcitabine-Resistant Cancer Cells. Anti-Cancer Drugs (2020) 31(7):684–92. doi: 10.1097/CAD.0000000000000933
356. Singh M, Bhatnagar P, Mishra S, Kumar P, Shukla Y, Gupta KC. PLGA-Encapsulated Tea Polyphenols Enhance the Chemotherapeutic Efficacy of Cisplatin Against Human Cancer Cells and Mice Bearing Ehrlich Ascites Carcinoma. Int J Nanomed (2015) 10:6789–809. doi: 10.2147/ijn.s79489
357. Zhou Y, Tang J, Du Y, Ding J, Liu J-Y. The Green Tea Polyphenol EGCG Potentiates the Antiproliferative Activity of Sunitinib in Human Cancer Cells. Tumor Biol (2016) 37(7):8555–66. doi: 10.1007/s13277-015-4719-x
358. Wu W, Dong J, Gou H, Geng R, Yang X, Chen D, et al. EGCG Synergizes the Therapeutic Effect of Irinotecan Through Enhanced DNA Damage in Human Colorectal Cancer Cells. J Cell Mol Med (2021). doi: 10.1111/jcmm.16718
359. Luo K-W, Zhu X-H, Zhao T, Zhong J, Gao H-C, Luo X-L, et al. EGCG Enhanced the Anti-Tumor Effect of Doxorubicine in Bladder Cancer via NF-κb/MDM2/p53 Pathway. Front Cell Dev Biol (2020) 8. doi: 10.3389/fcell.2020.606123
360. Wei R, Cortez Penso NE, Hackman RM, Wang Y, Mackenzie GG. Epigallocatechin-3-Gallate (EGCG) Suppresses Pancreatic Cancer Cell Growth, Invasion, and Migration Partly Through the Inhibition of Akt Pathway and Epithelial–Mesenchymal Transition: Enhanced Efficacy When Combined With Gemcitabine. Nutrients (2019) 11(8):1856. doi: 10.3390/nu11081856
361. Wang X, Jiang P, Wang P, Yang CS, Wang X, Feng Q. EGCG Enhances Cisplatin Sensitivity by Regulating Expression of the Copper and Cisplatin Influx Transporter CTR1 in Ovary Cancer. PloS One (2015) 10(4):e0125402. doi: 10.1371/journal.pone.0125402
362. Tajaldini M, Samadi F, Khosravi A, Ghasemnejad A, Asadi J. Protective and Anticancer Effects of Orange Peel Extract and Naringin in Doxorubicin Treated Esophageal Cancer Stem Cell Xenograft Tumor Mouse Model. Biomed Pharmacother (2020) 121:109594. doi: 10.1016/j.biopha.2019.109594
363. Erdogan S, Doganlar O, Doganlar ZB, Turkekul K. Naringin Sensitizes Human Prostate Cancer Cells to Paclitaxel Therapy. Prostate Int (2018) 6(4):126–35. doi: 10.1016/j.prnil.2017.11.001
364. Yin Z, Chen E, Cai X, Gong E, Li Y, Xu C, et al. Baicalin Attenuates XRCC1-Mediated DNA Repair to Enhance the Sensitivity of Lung Cancer Cells to Cisplatin. J Recept Signal Transduct (2021), 1–10. doi: 10.1080/10799893.2021.1892132
365. Lin M-Y, Cheng W-T, Cheng H-C, Chou W-C, Chen H-I, Ou H-C, et al. Baicalin Enhances Chemosensitivity to Doxorubicin in Breast Cancer Cells via Upregulation of Oxidative Stress-Mediated Mitochondria-Dependent Apoptosis. Antioxidants (2021) 10(10):1506. doi: 10.3390/antiox10101506
366. Yao X, Zhu F, Zhao Z, Liu C, Luo L, Yin Z. Arctigenin Enhances Chemosensitivity of Cancer Cells to Cisplatin Through Inhibition of the STAT3 Signaling Pathway. J Cell Biochem (2011) 112(10):2837–49. doi: 10.1002/jcb.23198
367. Wang HQ, Jin JJ, Wang J. Arctigenin Enhances Chemosensitivity to Cisplatin in Human Nonsmall Lung Cancer H460 Cells Through Downregulation of Survivin Expression. J Biochem Mol Toxicol (2014) 28(1):39–45. doi: 10.1002/jbt.21533
368. Wang Y, Lina L, Xu L, Yang Z, Qian Z, Zhou J, et al. Arctigenin Enhances the Sensitivity of Cisplatin Resistant Colorectal Cancer Cell by Activating Autophagy. Biochem Biophys Res Commun (2019) 520(1):20–6. doi: 10.1016/j.bbrc.2019.09.086
369. Brechbuhl HM, Kachadourian R, Min E, Chan D, Day BJ. Chrysin Enhances Doxorubicin-Induced Cytotoxicity in Human Lung Epithelial Cancer Cell Lines: The Role of Glutathione. Toxicol Appl Pharmacol (2012) 258(1):1–9. doi: 10.1016/j.taap.2011.08.004
370. Bieg D, Sypniewski D, Nowak E, Bednarek I. Morin Decreases Galectin-3 Expression and Sensitizes Ovarian Cancer Cells to Cisplatin. Arch Gynecol Obstetr (2018) 298(6):1181–94. doi: 10.1007/s00404-018-4912-4
371. Mannal P, McDonald D, McFadden D. Pterostilbene and Tamoxifen Show an Additive Effect Against Breast Cancer In Vitro. Am J Surg (2010) 200(5):577–80. doi: 10.1016/j.amjsurg.2010.07.022
372. Hu Z, Zeng Q, Zhang B, Liu H, Wang W. Promotion of P53 Expression and Reactive Oxidative Stress Production is Involved in Zerumbone-Induced Cisplatin Sensitization of Non-Small Cell Lung Cancer Cells. Biochimie (2014) 107:257–62. doi: 10.1016/j.biochi.2014.09.001
373. Jorvig JE, Chakraborty A. Zerumbone Inhibits Growth of Hormone Refractory Prostate Cancer Cells by Inhibiting JAK2/STAT3 Pathway and Increases Paclitaxel Sensitivity. Anti-Cancer Drugs (2015) 26(2):160–6. doi: 10.1097/CAD.0000000000000171
374. Zhou J, Ong C-N, Hur G-M, Shen H-M. Inhibition of the JAK-STAT3 Pathway by Andrographolide Enhances Chemosensitivity of Cancer Cells to Doxorubicin. Biochem Pharmacol (2010) 79(9):1242–50. doi: 10.1016/j.bcp.2009.12.014
375. Kang X, Zheng Z, Liu Z, Wang H, Zhao Y, Zhang W, et al. Liposomal Codelivery of Doxorubicin and Andrographolide Inhibits Breast Cancer Growth and Metastasis. Mol Pharm (2018) 15(4):1618–26. doi: 10.1021/acs.molpharmaceut.7b01164
376. Bao G-Q, Shen B-Y, Pan C-P, Zhang Y-J, Shi M-M, Peng C-H. Andrographolide Causes Apoptosis via Inactivation of STAT3 and Akt and Potentiates Antitumor Activity of Gemcitabine in Pancreatic Cancer. Toxicol Lett (2013) 222(1):23–35. doi: 10.1016/j.toxlet.2013.06.241
377. Yuwen D, Mi S, Ma Y, Guo W, Xu Q, Shen Y, et al. Andrographolide Enhances Cisplatin-Mediated Anticancer Effects in Lung Cancer Cells Through Blockade of Autophagy. Anti-Cancer Drugs (2017) 28(9):967–76. doi: 10.1097/CAD.0000000000000537
378. Wen L, Tian-Cong W, Dong-Mei H, Yue H, Ting F, Wen-Jie G, et al. Carnosic Acid Enhances the Anti-Lung Cancer Effect of Cisplatin by Inhibiting Myeloid-Derived Suppressor Cells. Chin J Natural Medicines (2018) 16(12):907–15. doi: 10.1016/S1875-5364(18)30132-8
379. Han N-n, Zhou Q, Huang Q, Liu K-J. Carnosic Acid Cooperates With Tamoxifen to Induce Apoptosis Associated With Caspase-3 Activation in Breast Cancer Cells In Vitro and In Vivo. Biomed Pharmacother (2017) 89:827–37. doi: 10.1016/j.biopha.2017.01.084
380. Li C-J, Chu C-Y, Huang L-H, Wang M-H, Sheu L-F, Yeh J-I, et al. Synergistic Anticancer Activity of Triptolide Combined With Cisplatin Enhances Apoptosis in Gastric Cancer In Vitro and In Vivo. Cancer Lett (2012) 319(2):203–13. doi: 10.1016/j.canlet.2012.01.006
381. Ho J-N, Byun S-S, Lee S, Oh JJ, Hong SK, Lee SE, et al. Synergistic Antitumor Effect of Triptolide and Cisplatin in Cisplatin Resistant Human Bladder Cancer Cells. J Urol (2015) 193(3):1016–22. doi: 10.1016/j.juro.2014.09.007
382. Zhang Z, Sun C, Zhang L, Chi X, Ji J, Gao X, et al. Triptolide Interferes With XRCC1/PARP1-Mediated DNA Repair and Confers Sensitization of Triple-Negative Breast Cancer Cells to Cisplatin. Biomed Pharmacother (2019) 109:1541–6. doi: 10.1016/j.biopha.2018.11.008
383. Liu J, Cheng H, Le Han ZQ, Zhang X, Gao W, Zhao K, et al. Synergistic Combination Therapy of Lung Cancer Using Paclitaxel-and Triptolide-Coloaded Lipid–Polymer Hybrid Nanoparticles. Drug Des Dev Ther (2018) 12:3199. doi: 10.2147/DDDT.S172199
384. Yang SW, Wang W, Xie XY, Zhu WP, Li FQ. In Vitro Synergistic Cytotoxic Effect of Triptolide Combined With Hydroxycamptothecin on Pancreatic Cancer Cells. Am J Chin Med (2011) 39(1):121–34. doi: 10.1142/S0192415X11008695
385. Cheng W, Xiang W, Wang S, Xu K. Tanshinone IIA Ameliorates Oxaliplatin-Induced Neurotoxicity via Mitochondrial Protection and Autophagy Promotion. Am J Transl Res (2019) 11(5):3140–9.
386. Yang Y, Zhang L-J, Bai X-G, Xu H-J, Jin Z-L, Ding M. Synergistic Antitumour Effects of Triptolide Plus Gemcitabine in Bladder Cancer. Biomed Pharmacother (2018) 106:1307–16. doi: 10.1016/j.biopha.2018.07.083
387. Deng Y, Li F, He P, Yang Y, Yang J, Zhang Y, et al. Triptolide Sensitizes Breast Cancer Cells to Doxorubicin Through the DNA Damage Response Inhibition. Mol Carcinog (2018) 57(6):807–14. doi: 10.1002/mc.22795
388. Li J, Liang X, Yang X. Ursolic Acid Inhibits Growth and Induces Apoptosis in Gemcitabine-Resistant Human Pancreatic Cancer via the JNK and PI3K/Akt/NF-κb Pathways. Oncol Rep (2012) 28(2):501–10. doi: 10.3892/or.2012.1827
389. Prasad S, Yadav VR, Sung B, Gupta SC, Tyagi AK, Aggarwal BB. Ursolic Acid Inhibits the Growth of Human Pancreatic Cancer and Enhances the Antitumor Potential of Gemcitabine in an Orthotopic Mouse Model Through Suppression of the Inflammatory Microenvironment. Oncotarget (2016) 7(11):13182. doi: 10.18632/oncotarget.7537
390. Shan J, Xuan Y, Zhang Q, Zhu C, Liu Z, Zhang S. Ursolic Acid Synergistically Enhances the Therapeutic Effects of Oxaliplatin in Colorectal Cancer. Protein Cell (2016) 7(8):571–85. doi: 10.1007/s13238-016-0295-0
391. Li L, Hou Y, Yu J, Lu Y, Chang L, Jiang M, et al. Synergism of Ursolic Acid and Cisplatin Promotes Apoptosis and Enhances Growth Inhibition of Cervical Cancer Cells via Suppressing NF-κb P65. Oncotarget (2017) 8(57):97416. doi: 10.18632/oncotarget.22133
392. Liu D-L, Bu H-Q, Jin H-M, Zhao J-F, Li Y, Huang H. Enhancement of the Effects of Gemcitabine Against Pancreatic Cancer by Oridonin via the Mitochondrial Caspase-Dependent Signaling Pathway. Mol Med Rep (2014) 10(6):3027–34. doi: 10.3892/mmr.2014.2584
393. Ma S, Tan W, Du B, Liu W, Li W, Che D, et al. Oridonin Effectively Reverses Cisplatin Drug Resistance in Human Ovarian Cancer Cells via Induction of Cell Apoptosis and Inhibition of Matrix Metalloproteinase Expression. Mol Med Rep (2016) 13(4):3342–8. doi: 10.3892/mmr.2016.4897
394. Li J, Wu Y, Wang D, Zou L, Fu C, Zhang J, et al. Oridonin Synergistically Enhances the Anti-Tumor Efficacy of Doxorubicin Against Aggressive Breast Cancer via Pro-Apoptotic and Anti-Angiogenic Effects. Pharmacol Res (2019) 146:104313. doi: 10.1016/j.phrs.2019.104313
395. Fan X, Wang T, Ji Z, Li Q, Shen H, Wang J. Synergistic Combination Therapy of Lung Cancer Using Lipid-Layered Cisplatin and Oridonin Co-Encapsulated Nanoparticles. Biomed Pharmacother (2021) 141:111830. doi: 10.1016/j.biopha.2021.111830
396. Yuan Z, Jiang H, Zhu X, Liu X, Li J. Ginsenoside Rg3 Promotes Cytotoxicity of Paclitaxel Through Inhibiting NF-κb Signaling and Regulating Bax/Bcl-2 Expression on Triple-Negative Breast Cancer. Biomed Pharmacother (2017) 89:227–32. doi: 10.1016/j.biopha.2017.02.038
397. Jiang Z, Yang Y, Yang Y, Zhang Y, Yue Z, Pan Z, et al. Ginsenoside Rg3 Attenuates Cisplatin Resistance in Lung Cancer by Downregulating PD-L1 and Resuming Immune. Biomed Pharmacother (2017) 96:378–83. doi: 10.1016/j.biopha.2017.09.129
398. Wang J, Tian L, Khan MN, Zhang L, Chen Q, Zhao Y, et al. Ginsenoside Rg3 Sensitizes Hypoxic Lung Cancer Cells to Cisplatin via Blocking of NF-κb Mediated Epithelial–Mesenchymal Transition and Stemness. Cancer Lett (2018) 415:73–85. doi: 10.1016/j.canlet.2017.11.037
399. Dai Y, Wang W, Sun Q, Tuohayi J. Ginsenoside Rg3 Promotes the Antitumor Activity of Gefitinib in Lung Cancer Cell Lines. Exp Ther Med (2019) 17(1):953–9. doi: 10.3892/etm.2018.7001
400. Zheng K, Li Y, Wang S, Wang X, Liao C, Hu X, et al. Inhibition of Autophagosome-Lysosome Fusion by Ginsenoside Ro via the ESR2-NCF1-ROS Pathway Sensitizes Esophageal Cancer Cells to 5-Fluorouracil-Induced Cell Death via the CHEK1-Mediated DNA Damage Checkpoint. Autophagy (2016) 12(9):1593–613. doi: 10.1080/15548627.2016.1192751
401. Kim SM, Lee SY, Yuk DY, Moon DC, Choi SS, Kim Y, et al. Inhibition of NF-κb by Ginsenoside Rg3 Enhances the Susceptibility of Colon Cancer Cells to Docetaxel. Arch Pharm Res (2009) 32(5):755–65. doi: 10.1007/s12272-009-1515-4
402. Kim SM, Lee SY, Cho JS, Son SM, Choi SS, Yun YP, et al. Combination of Ginsenoside Rg3 With Docetaxel Enhances the Susceptibility of Prostate Cancer Cells via Inhibition of NF-κb. Eur J Pharmacol (2010) 631(1-3):1–9. doi: 10.1016/j.ejphar.2009.12.018
403. Aktepe OH, Şahin TK, Güner G, Arik Z, Yalçin Ş. Lycopene Sensitizes the Cervical Cancer Cells to Cisplatin via Targeting Nuclear Factorkappa B (NF-?B) Pathway. Turk J Med Sci (2021) 51(1):368–74. doi: 10.3906/sag-2005-413
404. Zhu T, Li L-L, Xiao G-F, Luo Q-Z, Liu Q-Z, Yao K-T, et al. Berberine Increases Doxorubicin Sensitivity by Suppressing STAT3 in Lung Cancer. Am J Chin Med (2015) 43(07):1487–502. doi: 10.1142/S0192415X15500846
405. Ebeid SA, Abd El Moneim NA, Ghoneim H.E.-D.M., El-Benhawy SA, Ismail SE. Combination of Doxorubicin and Berberine Generated Synergistic Anticancer Effect on Breast Cancer Cells Through Down-Regulation of Nanog and miRNA-21 Gene Expression. Middle East J Cancer (2020) 11(3):273–85.
406. Zheng X, Zhao Y, Jia Y, Shao D, Zhang F, Sun M, et al. Biomimetic Co-Assembled Nanodrug of Doxorubicin and Berberine Suppresses Chemotherapy-Exacerbated Breast Cancer Metastasis. Biomaterials (2021) 271. doi: 10.1016/j.biomaterials.2021.120716
407. Chen Q, Qin R, Fang Y, Li H. Berberine Sensitizes Human Ovarian Cancer Cells to Cisplatin Through miR-93/PTEN/Akt Signaling Pathway. Cell Physiol Biochem (2015) 36(3):956–65. doi: 10.1159/000430270
408. Zhao Y, Jing Z, Li Y, Mao W. Berberine in Combination With Cisplatin Suppresses Breast Cancer Cell Growth Through Induction of DNA Breaks and Caspase-3-Dependent Apoptosis. Oncol Rep (2016) 36(1):567–72. doi: 10.3892/or.2016.4785
409. Yu M, Tong X, Qi B, Qu H, Dong S, Yu B, et al. Berberine Enhances Chemosensitivity to Irinotecan in Colon Cancer via Inhibition of NF-κb. Mol Med Rep (2014) 9(1):249–54. doi: 10.3892/mmr.2013.1762
410. Roh J-L, Kim EH, Park JY, Kim JW, Kwon M, Lee B-H. Piperlongumine Selectively Kills Cancer Cells and Increases Cisplatin Antitumor Activity in Head and Neck Cancer. Oncotarget (2014) 5(19):9227. doi: 10.18632/oncotarget.2402
411. Wang Y, Wu X, Zhou Y, Jiang H, Pan S, Sun B. Piperlongumine Suppresses Growth and Sensitizes Pancreatic Tumors to Gemcitabine in a Xenograft Mouse Model by Modulating the NF-Kappa B Pathway. Cancer Prev Res (2016) 9(3):234–44. doi: 10.1158/1940-6207.CAPR-15-0306
412. Chen D, Ma Y, Li P, Liu M, Fang Y, Zhang J, et al. Piperlongumine Induces Apoptosis and Synergizes With Doxorubicin by Inhibiting the JAK2-STAT3 Pathway in Triple-Negative Breast Cancer. Molecules (2019) 24(12):2338. doi: 10.3390/molecules24122338
413. Chen W, Lian W, Yuan Y, Li M. The Synergistic Effects of Oxaliplatin and Piperlongumine on Colorectal Cancer are Mediated by Oxidative Stress. Cell Death Dis (2019) 10(8):1–12. doi: 10.1038/s41419-019-1824-6
414. Zhang P, Shi L, Zhang T, Hong L, He W, Cao P, et al. Piperlongumine Potentiates the Antitumor Efficacy of Oxaliplatin Through ROS Induction in Gastric Cancer Cells. Cell Oncol (2019) 42(6):847–60. doi: 10.1007/s13402-019-00471-x
415. Rawat L, Hegde H, Hoti SL, Nayak V. Piperlongumine Induces ROS Mediated Cell Death and Synergizes Paclitaxel in Human Intestinal Cancer Cells. Biomed Pharmacother (2020) 128:110243. doi: 10.1016/j.biopha.2020.110243
416. Sun K, Tang XH, Xie YK. Paclitaxel Combined With Harmine Inhibits the Migration and Invasion of Gastric Cancer Cells Through Downregulation of Cyclooxygenase−2 Expression. Oncol Lett (2015) 10(3):1649–54. doi: 10.3892/ol.2015.3425
417. Yu XJ, Sun K, Tang XH, Zhou CJ, Sun H, Yan Z, et al. Harmine Combined With Paclitaxel Inhibits Tumor Proliferation and Induces Apoptosis Through Down-Regulation of Cyclooxygenase-2 Expression in Gastric Cancer. Oncol Lett (2016) 12(2):983–8. doi: 10.3892/ol.2016.4696
418. Wu L-W, Zhang J-K, Rao M, Zhang Z-Y, Zhu H-J, Zhang C. Harmine Suppresses the Proliferation of Pancreatic Cancer Cells and Sensitizes Pancreatic Cancer to Gemcitabine Treatment. OncoTargets Ther (2019) 12:4585. doi: 10.2147/OTT.S205097
419. Duan L, Deng L, Wang D, Ma S, Li C, Zhao D. Treatment Mechanism of Matrine in Combination With Irinotecan for Colon Cancer. Oncol Lett (2017) 14(2):2300–4. doi: 10.3892/ol.2017.6407
420. Liao X-Z, Tao L-T, Liu J-H, Gu Y-Y, Xie J, Chen Y, et al. Matrine Combined With Cisplatin Synergistically Inhibited Urothelial Bladder Cancer Cells via Down-Regulating VEGF/PI3K/Akt Signaling Pathway. Cancer Cell Int (2017) 17(1):1–14. doi: 10.1186/s12935-017-0495-6
421. Hu G, Cao C, Deng Z, Li J, Zhou X, Huang Z, et al. Effects of Matrine in Combination With Cisplatin on Liver Cancer. Oncol Lett (2021) 21(1):1–1. doi: 10.3892/ol.2020.12327
422. Yang A, Zhu J, Xu F, Yang J, Wang Y, Wei M, et al. Anticancer Effect of a Combination of Cisplatin and Matrine on Cervical Cancer U14 Cells and U14 Tumor-Bearing Mice, and Possible Mechanism of Action Involved. Trop J Pharm Res (2021) 20(8):1631–8.
423. Zhu L, Huang S, Li J, Chen J, Yao Y, Li L, et al. Sophoridine Inhibits Lung Cancer Cell Growth and Enhances Cisplatin Sensitivity Through Activation of the P53 and Hippo Signaling Pathways. Gene (2020) 742:144556. doi: 10.1016/j.gene.2020.144556
424. Kaminski BM, Weigert A, Brüne B, Schumacher M, Wenzel U, Steinhilber D, et al. Sulforaphane Potentiates Oxaliplatin-Induced Cell Growth Inhibition in Colorectal Cancer Cells via Induction of Different Modes of Cell Death. Cancer Chemother Pharmacol (2011) 67(5):1167–78. doi: 10.1007/s00280-010-1413-y
425. Kim SH, Park HJ, Moon DO. Sulforaphane Sensitizes Human Breast Cancer Cells to Paclitaxel−Induced Apoptosis by Downregulating the NF−κb Signaling Pathway. Oncol Lett (2017) 13(6):4427–32. doi: 10.3892/ol.2017.5950
426. Wang F, Wang W, Li J, Zhang J, Wang X, Wang M. Sulforaphane Reverses Gefitinib Tolerance in Human Lung Cancer Cells via Modulation of Sonic Hedgehog Signaling. Oncol Lett (2018) 15(1):109–14. doi: 10.3892/ol.2017.7293
427. Justin S, Rutz J, Maxeiner S, Chun FK-H, Juengel E, Blaheta RA. Chronic Sulforaphane Administration Inhibits Resistance to the mTOR-Inhibitor Everolimus in Bladder Cancer Cells. Int J Mol Sci (2020) 21(11):4026. doi: 10.3390/ijms21114026
428. Liu F, Lv R-B, Liu Y, Hao Q, Liu S-J, Zheng Y-Y, et al. Salinomycin and Sulforaphane Exerted Synergistic Antiproliferative and Proapoptotic Effects on Colorectal Cancer Cells by Inhibiting the PI3K/Akt Signaling Pathway In Vitro and In Vivo. OncoTargets Ther (2020) 13:4957. doi: 10.2147/OTT.S246706
429. Wang Y, Zhou Y, Jia G, Han B, Liu J, Teng Y, et al. Shikonin Suppresses Tumor Growth and Synergizes With Gemcitabine in a Pancreatic Cancer Xenograft Model: Involvement of NF-κb Signaling Pathway. Biochem Pharmacol (2014) 88(3):322–33. doi: 10.1016/j.bcp.2014.01.041
430. Tang J-c, Ren Y-G, Zhao J, Long F, Chen J-Y, Jiang Z. Shikonin Enhances Sensitization of Gefitinib Against Wild-Type EGFR Non-Small Cell Lung Cancer via Inhibition PKM2/stat3/cyclinD1 Signal Pathway. Life Sci (2018) 204:71–7. doi: 10.1016/j.lfs.2018.05.012
431. Du W, Hao X, Yuan Z, Wang Y, Zhang X, Liu J. Shikonin Potentiates Paclitaxel Antitumor Efficacy in Esophageal Cancer Cells via the Apoptotic Pathway. Oncol Lett (2019) 18(3):3195–201. doi: 10.3892/ol.2019.10662
432. Lin H-Y, Han H-W, Wang Y-S, He D-L, Sun W-X, Feng L, et al. Shikonin and 4-Hydroxytamoxifen Synergistically Inhibit the Proliferation of Breast Cancer Cells Through Activating Apoptosis Signaling Pathway In Vitro and In Vivo. Chin Med (2020) 15(1):1–14. doi: 10.1186/s13020-020-00305-1
433. Liu K, Cang S, Ma Y, Chiao JW. Synergistic Effect of Paclitaxel and Epigenetic Agent Phenethyl Isothiocyanate on Growth Inhibition, Cell Cycle Arrest and Apoptosis in Breast Cancer Cells. Cancer Cell Int (2013) 13(1):1–8. doi: 10.1186/1475-2867-13-10
434. Cang S, Ma Y, Chiao J-W, Liu D. Phenethyl Isothiocyanate and Paclitaxel Synergistically Enhanced Apoptosis and Alpha-Tubulin Hyperacetylation in Breast Cancer Cells. Exp Hematol Oncol (2014) 3(1):1–6. doi: 10.1186/2162-3619-3-5
435. Choudhury B, Kandimalla R, Elancheran R, Bharali R, Kotoky J. Garcinia Morella Fruit, a Promising Source of Antioxidant and Anti-Inflammatory Agents Induces Breast Cancer Cell Death via Triggering Apoptotic Pathway. Biomed Pharmacother (2018) 103:562–73. doi: 10.1016/j.biopha.2018.04.068
436. Li F, Shanmugam MK, Siveen KS, Wang F, Ong TH, Loo SY, et al. Garcinol Sensitizes Human Head and Neck Carcinoma to Cisplatin in a Xenograft Mouse Model Despite Downregulation of Proliferative Biomarkers. Oncotarget (2015) 6(7):5147–63. doi: 10.18632/oncotarget.2881
437. Zhang J, Fang H, Zhang J, Guan W, Xu G. Garcinol Alone and in Combination With Cisplatin Affect Cellular Behavior and PI3K/AKT Protein Phosphorylation in Human Ovarian Cancer Cells. Dose-Response (2020) 18(2). doi: 10.1177/1559325820926732
438. Tu SH, Chiou YS, Kalyanam N, Ho CT, Chen LC, Pan MH. Garcinol Sensitizes Breast Cancer Cells to Taxol Through the Suppression of Caspase-3/Ipla2 and NF-κb/Twist1 Signaling Pathways in a Mouse 4T1 Breast Tumor Model. Food Funct (2017) 8(3):1067–79. doi: 10.1039/c6fo01588c
439. Chandimali N, Huynh DL, Jin WY, Kwon T. Combination Effects of Hispidin and Gemcitabine via Inhibition of Stemness in Pancreatic Cancer Stem Cells. Anticancer Res (2018) 38(7):3967–75. doi: 10.21873/anticanres.12683
440. Zhang B, Shi ZL, Liu B, Yan XB, Feng J, Tao HM. Enhanced Anticancer Effect of Gemcitabine by Genistein in Osteosarcoma: The Role of Akt and Nuclear Factor-κb. Anti-Cancer Drugs (2010) 21(3):288–96. doi: 10.1097/CAD.0b013e328334da17
441. Liang C, Li H, Shen C, Lai J, Shi Z, Liu B, et al. Genistein Potentiates the Anti-Cancer Effects of Gemcitabine in Human Osteosarcoma via the Downregulation of Akt and Nuclear Factor-κb Pathway. Anticancer Agents Med Chem (2012) 12(5):554–63. doi: 10.2174/187152012800617867
442. Ahn DW, Seo JK, Lee SH, Hwang JH, Lee JK, Ryu JK, et al. Enhanced Antitumor Effect of Combination Therapy With Gemcitabine and Guggulsterone in Pancreatic Cancer. Pancreas (2012) 41(7):1048–57. doi: 10.1097/MPA.0b013e318249d62e
443. Lou C, Lu H, Ma Z, Liu C, Zhang Y. Ginkgolide B Enhances Gemcitabine Sensitivity in Pancreatic Cancer Cell Lines via Inhibiting PAFR/NF-Кb Pathway. Biomed Pharmacother (2019) 109:563–72. doi: 10.1016/j.biopha.2018.10.084
444. Zhang DC, Liu JL, Ding YB, Xia JG, Chen GY. Icariin Potentiates the Antitumor Activity of Gemcitabine in Gallbladder Cancer by Suppressing NF-κb. Acta Pharmacol Sin (2013) 34(2):301–8. doi: 10.1038/aps.2012.162
445. Kunnumakkara AB, Sung B, Ravindran J, Diagaradjane P, Deorukhkar A, Dey S, et al. Zyflamend Suppresses Growth and Sensitizes Human Pancreatic Tumors to Gemcitabine in an Orthotopic Mouse Model Through Modulation of Multiple Targets. Int J Cancer (2012) 131(3):E292–303. doi: 10.1002/ijc.26442
446. Arafa ESA, Zhu Q, Barakat BM, Wani G, Zhao Q, El-Mahdy MA, et al. Tangeretin Sensitizes Cisplatin-Resistant Human Ovarian Cancer Cells Through Downregulation of Phosphoinositide 3-Kinase/Akt Signaling Pathway. Cancer Res (2009) 69(23):8910–7. doi: 10.1158/0008-5472.CAN-09-1543
447. Yu S, Gong LS, Li NF, Pan YF, Zhang L. Galangin (GG) Combined With Cisplatin (DDP) to Suppress Human Lung Cancer by Inhibition of STAT3-Regulated NF-κb and Bcl-2/Bax Signaling Pathways. Biomed Pharmacother (2018) 97:213–24. doi: 10.1016/j.biopha.2017.10.059
448. Zhou P, Li Z, Xu D, Wang Y, Bai Q, Feng Y, et al. Cepharanthine Hydrochloride Improves Cisplatin Chemotherapy and Enhances Immunity by Regulating Intestinal Microbes in Mice. Front Cell Infect Microbiol (2019) 9:225(JUN). doi: 10.3389/fcimb.2019.00225
449. Wu J, Guan M, Wong PF, Yu H, Dong J, Xu J. Icariside II Potentiates Paclitaxel-Induced Apoptosis in Human Melanoma A375 Cells by Inhibiting TLR4 Signaling Pathway. Food Chem Toxicol (2012) 50(9):3019–24. doi: 10.1016/j.fct.2012.06.027
450. Lin CL, Chen RF, Chen JYF, Chu YC, Wang HM, Chou HL, et al. Protective Effect of Caffeic Acid on Paclitaxel Induced Anti-Proliferation and Apoptosis of Lung Cancer Cells Involves NF-κb Pathway. Int J Mol Sci (2012) 13(5):6236–45. doi: 10.3390/ijms13056236
451. Liang L, Wu J, Luo J, Wang L, Chen ZX, Han CL, et al. Oxymatrine Reverses 5-Fluorouracil Resistance by Inhibition of Colon Cancer Cell Epithelial-Mesenchymal Transition and NF-κb Signaling In Vitro. Oncol Lett (2020) 19(1):519–26. doi: 10.3892/ol.2019.11090
452. Xu GY, Tang XJ. Troxerutin (TXN) Potentiated 5-Fluorouracil (5-Fu) Treatment of Human Gastric Cancer Through Suppressing STAT3/NF-κb and Bcl-2 Signaling Pathways. Biomed Pharmacother (2017) 92:95–107. doi: 10.1016/j.biopha.2017.04.059
453. Buhrmann C, Kunnumakkara AB, Popper B, Majeed M, Aggarwal BB, Shakibaei M. Calebin a Potentiates the Effect of 5-Fu and Tnf-ß (Lymphotoxin a) Against Human Colorectal Cancer Cells: Potential Role of NF-KB +. Int J Mol Sci (2020) 21(7). doi: 10.3390/ijms21072393
454. Fang LJ, Shao XT, Wang S, Lu GH, Xu T, Zhou JY. Sesquiterpene Lactone Parthenolide Markedly Enhances Sensitivity of Human A549 Cells to Low-Dose Oxaliplatin via Inhibition of NF-κb Activation and Induction of Apoptosis. Planta Med (2010) 76(3):258–64. doi: 10.1055/s-0029-1186083
455. Hahnvajanawong C, Wattanawongdon W, Chomvarin C, Anantachoke N, Kanthawong S, Sripa B, et al. Synergistic Effects of Isomorellin and Forbesione With Doxorubicin on Apoptosis Induction in Human Cholangiocarcinoma Cell Lines. Cancer Cell Int (2014) 14(1). doi: 10.1186/1475-2867-14-68
456. Wang LJ, Meng Q, Wang CY, Liu Q, Peng JY, Huo XK, et al. Dioscin Restores the Activity of the Anticancer Agent Adriamycin in Multidrug-Resistant Human Leukemia K562/Adriamycin Cells by Down-Regulating MDR1 via a Mechanism Involving NF-Kappa B Signaling Inhibition. J Natural Prod (2013) 76(5):909–14. doi: 10.1021/np400071c
457. Lagoa R, Silva J, Rodrigues JR, Bishayee A. Advances in Phytochemical Delivery Systems for Improved Anticancer Activity. Biotechnol Adv (2020) 38:107382. doi: 10.1016/j.biotechadv.2019.04.004
458. Bagherian A, Mardani R, Roudi B, Taghizadeh M, Banfshe HR, Ghaderi A, et al. Combination Therapy With Nanomicellar-Curcumin and Temozolomide for In Vitro Therapy of Glioblastoma Multiforme via Wnt Signaling Pathways. J Mol Neurosci (2020) 70(10):1471–83. doi: 10.1007/s12031-020-01639-z
459. Qiu N, Cai LL, Xie D, Wang G, Wu W, Zhang Y, et al. Synthesis, Structural and In Vitro Studies of Well-Dispersed Monomethoxy-Poly(Ethylene Glycol)-Honokiol Conjugate Micelles. BioMed Mater (2010) 5(6):065006. doi: 10.1088/1748-6041/5/6/065006
460. More MP, Deshmukh PK. Development of Amine-Functionalized Superparamagnetic Iron Oxide Nanoparticles Anchored Graphene Nanosheets as a Possible Theranostic Agent in Cancer Metastasis. Drug Delivery Transl Res (2020) 10(4):862–77. doi: 10.1007/s13346-020-00729-0
461. Zafar S, Akhter S, Ahmad I, Hafeez Z, Alam Rizvi MM, Jain GK, et al. Improved Chemotherapeutic Efficacy Against Resistant Human Breast Cancer Cells With Co-Delivery of Docetaxel and Thymoquinone by Chitosan Grafted Lipid Nanocapsules: Formulation Optimization, In Vitro and In Vivo Studies. Colloids Surf B Biointerf (2020) 186:110603. doi: 10.1016/j.colsurfb.2019.110603
462. Wang Y, Yu H, Wang S, Gai C, Cui X, Xu Z, et al. Targeted Delivery of Quercetin by Nanoparticles Based on Chitosan Sensitizing Paclitaxel-Resistant Lung Cancer Cells to Paclitaxel. Mater Sci Eng C Mater Biol Appl (2021) 119:111442. doi: 10.1016/j.msec.2020.111442
463. Ghaffari M, Dehghan G, Baradaran B, Zarebkohan A, Mansoori B, Soleymani J, et al. Co-Delivery of Curcumin and Bcl-2 siRNA by PAMAM Dendrimers for Enhancement of the Therapeutic Efficacy in HeLa Cancer Cells. Colloids Surf B Biointerf (2020) 188:110762. doi: 10.1016/j.colsurfb.2019.110762
464. Liu A, Wang W, Fang H, Yang Y, Jiang X, Liu S, et al. Baicalein Protects Against Polymicrobial Sepsis-Induced Liver Injury via Inhibition of Inflammation and Apoptosis in Mice. Eur J Pharmacol (2015) 748:45–53. doi: 10.1016/j.ejphar.2014.12.014
465. Li X, Huang JM, Wang JN, Xiong XK, Yang XF, Zou F. Combination of Chrysin and Cisplatin Promotes the Apoptosis of Hep G2 Cells by Up-Regulating P53. Chem Biol Interact (2015) 232:12–20. doi: 10.1016/j.cbi.2015.03.003
466. Meng G, Chai K, Li X, Zhu Y, Huang W. Luteolin Exerts Pro-Apoptotic Effect and Anti-Migration Effects on A549 Lung Adenocarcinoma Cells Through the Activation of MEK/ERK Signaling Pathway. Chem Biol Interact (2016) 257:26–34. doi: 10.1016/j.cbi.2016.07.028
467. Majumdar D, Jung KH, Zhang H, Nannapaneni S, Wang X, Amin AR, et al. Luteolin Nanoparticle in Chemoprevention: In Vitro and In Vivo Anticancer Activity. Cancer Prev Res (Phila) (2014) 7(1):65–73. doi: 10.1158/1940-6207.capr-13-0230
468. Varshosaz J, Jandaghian S, Mirian M, Sajjadi SE. Co-Delivery of Rituximab Targeted Curcumin and Imatinib Nanostructured Lipid Carriers in Non-Hodgkin Lymphoma Cells. J Liposome Res (2021) 31(1):64–78. doi: 10.1080/08982104.2020.1720718
469. Dhupal M, Oh JM, Tripathy DR, Kim SK, Koh SB, Park KS. Immunotoxicity of Titanium Dioxide Nanoparticles via Simultaneous Induction of Apoptosis and Multiple Toll-Like Receptors Signaling Through ROS-Dependent SAPK/JNK and P38 MAPK Activation. Int J Nanomed (2018) 13:6735–50. doi: 10.2147/ijn.s176087
470. Aas Z, Babaei E, Hosseinpour Feizi MA, Dehghan G. Anti-Proliferative and Apoptotic Effects of Dendrosomal Farnesiferol C on Gastric Cancer Cells. Asian Pac J Cancer Prev (2015) 16(13):5325–9. doi: 10.7314/apjcp.2015.16.13.5325
471. Ochi MM, Amoabediny G, Rezayat SM, Akbarzadeh A, Ebrahimi B. In Vitro Co-Delivery Evaluation of Novel Pegylated Nano-Liposomal Herbal Drugs of Silibinin and Glycyrrhizic Acid (Nano-Phytosome) to Hepatocellular Carcinoma Cells. Cell J (2016) 18(2):135–48. doi: 10.22074/cellj.2016.4308
472. Israelsen WJ, Vander Heiden MG. Pyruvate Kinase: Function, Regulation and Role in Cancer. Semin Cell Dev Biol (2015) 43:43–51. doi: 10.1016/j.semcdb.2015.08.004
473. Bolger GT, Licollari A, Tan A, Greil R, Vcelar B, Greil-Ressler S, et al. Pharmacokinetics of Liposomal Curcumin (Lipocurc™) Infusion: Effect of Co-Medication in Cancer Patients and Comparison With Healthy Individuals. Cancer Chemother Pharmacol (2019) 83(2):265–75. doi: 10.1007/s00280-018-3730-5
474. Gautam M, Thapa RK, Gupta B, Soe ZC, Ou W, Poudel K, et al. Phytosterol-Loaded CD44 Receptor-Targeted PEGylated Nano-Hybrid Phyto-Liposomes for Synergistic Chemotherapy. Expert Opin Drug Delivery (2020) 17(3):423–34. doi: 10.1080/17425247.2020.1727442
475. Ahn S, Lee IH, Kang S, Kim D, Choi M, Saw PE, et al. Gold Nanoparticles Displaying Tumor-Associated Self-Antigens as a Potential Vaccine for Cancer Immunotherapy. Adv Healthc Mater (2014) 3(8):1194–9. doi: 10.1002/adhm.201300597
476. Cao M, Yan H, Han X, Weng L, Wei Q, Sun X, et al. Ginseng-Derived Nanoparticles Alter Macrophage Polarization to Inhibit Melanoma Growth. J Immunother Cancer (2019) 7(1):326. doi: 10.1186/s40425-019-0817-4
477. Thangapazham RL, Puri A, Tele S, Blumenthal R, Maheshwari RK. Evaluation of a Nanotechnology-Based Carrier for Delivery of Curcumin in Prostate Cancer Cells. Int J Oncol (2008) 32(5):1119–23. doi: 10.3892/ijo.32.5.1119
478. Ashrafizadeh M, Javanmardi S, Moradi-Ozarlou M, Mohammadinejad R, Farkhondeh T, Samarghandian S, et al. .
479. Lee SW, Kim YM, Cho CH, Kim YT, Kim SM, Hur SY, et al. An Open-Label, Randomized, Parallel, Phase II Trial to Evaluate the Efficacy and Safety of a Cremophor-Free Polymeric Micelle Formulation of Paclitaxel as First-Line Treatment for Ovarian Cancer: A Korean Gynecologic Oncology Group Study (KGOG-3021). Cancer Res Treat (2018) 50(1):195–203. doi: 10.4143/crt.2016.376
480. Kooshki L, Mahdavi P, Fakhri S, Akkol EK, Khan H. Targeting Lactate Metabolism and Glycolytic Pathways in the Tumor Microenvironment by Natural Products: A Promising Strategy in Combating Cancer. BioFactors (2021). doi: 10.1002/biof.1799
481. Kashyap D, Tuli HS, Yerer MB, Sharma A, Sak K, Srivastava S, et al. Natural Product-Based Nanoformulations for Cancer Therapy: Opportunities and Challenges. Semin Cancer Biol (2021) 69:5–23. doi: 10.1016/j.semcancer.2019.08.014
482. Colone M, Calcabrini A, Stringaro A. Drug Delivery Systems of Natural Products in Oncology. Molecules (2020) 25(19):4560. doi: 10.3390/molecules25194560
Keywords: TLR - toll-like receptor, NF-κB – nuclear factor-kappa B, NLRP, phytochemicals, chemotherapy, immunotherapy, signaling pathways, molecular pharmacology
Citation: Fakhri S, Moradi SZ, Yarmohammadi A, Narimani F, Wallace CE and Bishayee A (2022) Modulation of TLR/NF-κB/NLRP Signaling by Bioactive Phytocompounds: A Promising Strategy to Augment Cancer Chemotherapy and Immunotherapy. Front. Oncol. 12:834072. doi: 10.3389/fonc.2022.834072
Received: 12 December 2021; Accepted: 26 January 2022;
Published: 01 March 2022.
Edited by:
Nand K. Roy, Case Western Reserve University, United StatesReviewed by:
Elancheran Ramakrishnan, Annamalai University, IndiaBertrand Liagre, Université de Limoges, France
Copyright © 2022 Fakhri, Moradi, Yarmohammadi, Narimani, Wallace and Bishayee. This is an open-access article distributed under the terms of the Creative Commons Attribution License (CC BY). The use, distribution or reproduction in other forums is permitted, provided the original author(s) and the copyright owner(s) are credited and that the original publication in this journal is cited, in accordance with accepted academic practice. No use, distribution or reproduction is permitted which does not comply with these terms.
*Correspondence: Anupam Bishayee, YWJpc2hheWVlQGxlY29tLmVkdQ==; YWJpc2hheWVlQGdtYWlsLmNvbQ==