- 1Departamento de Bioquímica, Centro de Investigación de Estudios Avanzados (CINVESTAV-IPN), Ciudad de Mexico, Mexico
- 2Posgrado en Ciencias Genómicas, Universidad Autónoma de la Ciudad de Mexico, Ciudad de Mexico, Mexico
- 3Programa en Biomedicina Molecular y Red de Biotecnología, Instituto Politécnico Nacional, Ciudad de Mexico, Mexico
- 4Facultad de Ciencias Químico Biológicas, Universidad Autónoma de Sinaloa, Culiacán Sinaloa, Mexico
- 5Departamento de Biología Celular y Fisiología, Instituto de Investigaciones Biomédicas, Universidad Nacional Autónoma de México, Ciudad de México, Mexico
- 6Laboratorio de Genómica, Instituto Nacional de Cancerología, Ciudad de México, Mexico
Traditional two-dimensional (2D) monolayer cell cultures have long been the gold standard for cancer biology research. However, their ability to accurately reflect the molecular mechanisms of tumors occurring in vivo is limited. Recent development of three-dimensional (3D) cell culture models facilitate the possibility to better recapitulate several of the biological and molecular characteristics of tumors in vivo, such as cancer cells heterogeneity, cell-extracellular matrix interactions, development of a hypoxic microenvironment, signaling pathway activities depending on contacts with extracellular matrix, differential growth kinetics, more accurate drugs response, and specific gene expression and epigenetic patterns. In this review, we discuss the utilization of different types of 3D culture models including spheroids, organotypic models and patient-derived organoids in gynecologic cancers research, as well as its potential applications in oncological research mainly for screening drugs with major physiological and clinical relevance. Moreover, microRNAs regulation of cancer hallmarks in 3D cell cultures from different types of cancers is discussed.
Introduction
Three-dimensional (3D) cell cultures are a breakthrough for gynecological and breast cancer research as they mimic the 3D architecture of primary tumors. For a long time, oncology research was based on 2D monolayer cultures, where cells grown on a flat solid surface. However, this culture model has limitations, such as the absence of cell-cell and cell- extracellular matrix (ECM) interactions, and tumor microenvironment, as well as unlimited access to nutrients, oxygen, and metabolites (1, 2). Additionally, cells cultured in 2D modify their morphology and cause cytoskeletal rearrangements, acquiring artificial polarity, which in turn leads to aberrant gene and protein expression (Figure 1A) (3, 4). On the other hand, 3D cultures promote cell-cell and cell-ECM interactions (5). This culture model better recapitulates the characteristics of tumor cells in vivo, such as cell heterogeneity, hypoxia, growth kinetics, signaling pathway activity and gene expression patterns (6, 7). Moreover, in 3D cultures the morphology and polarity of tumor cells are maintained, and a concentration gradient of O2, nutrients and metabolic waste is generated, making them an ideal model to study tumor cells behavior (Figure 1B) (8, 9). Several reports showed the advantages of using 3D culture systems for gynecological cancer studies, as they allow the evaluation of the effect of the extracellular matrix on the tumor, reducing the existing breach between 3D culture models and in vivo models (Table 1).
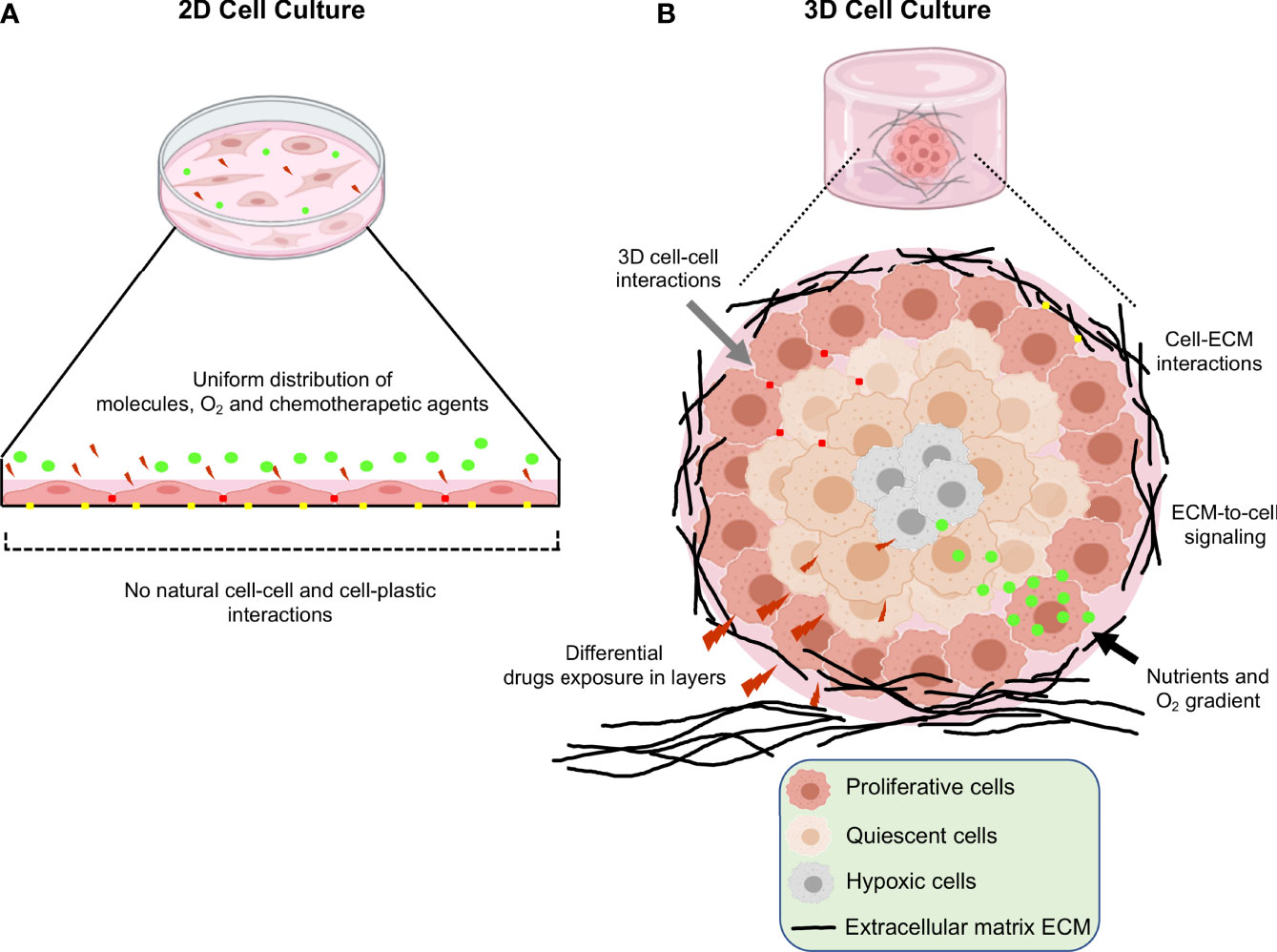
Figure 1 Schematic representation of the main differences between 2D and 3D cell cultures. (A) Traditional 2D cell culture in which flattened cells grown in a monolayer at the bottom of plastic plates. Reduced cell-cell interactions, unlimited exposure to nutrients, oxygen and drugs are limitations of this type of cultures. (B) 3D cell culture systems; in which increased cell-cell and cell-extracellular matrix interactions, limited access to nutrients, oxygen, and heterogeneity in the drugs interactions leads to better recapitulation of the tumor microenvironment occurring in vivo.
The development of 3D cultures and its more generalized utilization have permitted the evaluation of changes in gene expression mechanisms relative to 2D conditions, mainly in mRNA transcriptomes. However, scarce data on postranscriptional control of gene expression represented by microRNAs (miRNAs) have been studied in 3D cancer cell cultures. MiRNAs are small non-coding RNAs of about 21-25 nucleotides in length that function as negative regulators of gene expression at the post-transcriptional level (15). The miRNAs contain a seed region corresponding to 2-7 nucleotides, which binds by bases complementarity to conserved sites in the 3’ untranslated regions (UTR) of target mRNAs, resulting in mRNA degradation or translation repression (16). Increasing evidence shows that miRNAs regulate diverse processes involved in cancer progression, such as, cell proliferation, apoptosis, invasion, metastasis, and drug resistance (17, 18). Due to their high stability, miRNAs are also being tested in clinical trials as therapeutic agents for treatment of oncological patients (19). The objective of this review is to address the 3D modeling systems in cancer research, as well as potential applications in gynecological and breast cancers, because they are main oncological diseases affecting the female population. Finally, the differential regulation of miRNAs in 3D cultured breast and gynecological cancer cells is addressed, in order to understand its biological functions and if they could be potential therapeutic targets.
Types of 3D Culture Systems in Cancer
Nowadays, 3D culture systems are divided into three categories: spheroids, organotypic cultures and organoid models. Spheroids are commonly referred to cultures in which cancer cell lines grown in low-adherence plastic plates or over inert substrates like agarose with continuous agitation, in which no ECM is utilized as substrates. In contrast, 3D organotypic cultures of cancer cell lines are in vitro systems in which the cells are cultured on commercial matrigel containing extracellular matrix proteins which provides a semi-solid support simulating some features of the in vivo tumor microenvironment such as cell-cell and cell-ECM interactions which activate cell signaling. On the other hand, the organoids which are generally ex vivo systems mainly patient-derived explants (PDE), are cultured on matrigel that simulate the extracellular matrix and facilitate drug testing in intact human tumors. In the next sections, we will discuss the different types of 3D culture systems.
Spheroids Models
Spheroids are cell aggregates that can be grown in suspension, for example on low-adhesion plastic plates or over inert substrates such as agarose with continuous agitation without the presence of matrigel (11). The suspension culture method was developed in 1970 by Sutherland and coworkers (20). They used an in vitro 3D model system to recreate the complexities of the multicellular tumor to study the response of tumor cells to radiotherapy. In this method, ultra-low attachment plates are used or standard plastic plates coated with inert substrates, for example, agar or poly-2-hydroxyethyl methacrylate (poly-HEMA), which prevents cells from adhering to the surface of the wells, forcing cells to aggregate and form spheroids (21). On the other hand, the system from spinner flasks consists of cells suspension and a shaker element that maintains continuous movement. The liquid flow not only prevents cell adhesion, but also ensures uniform distribution of nutrients and oxygen in cells. This method produces high yields of spheroids (22, 23) (Figure 2A).
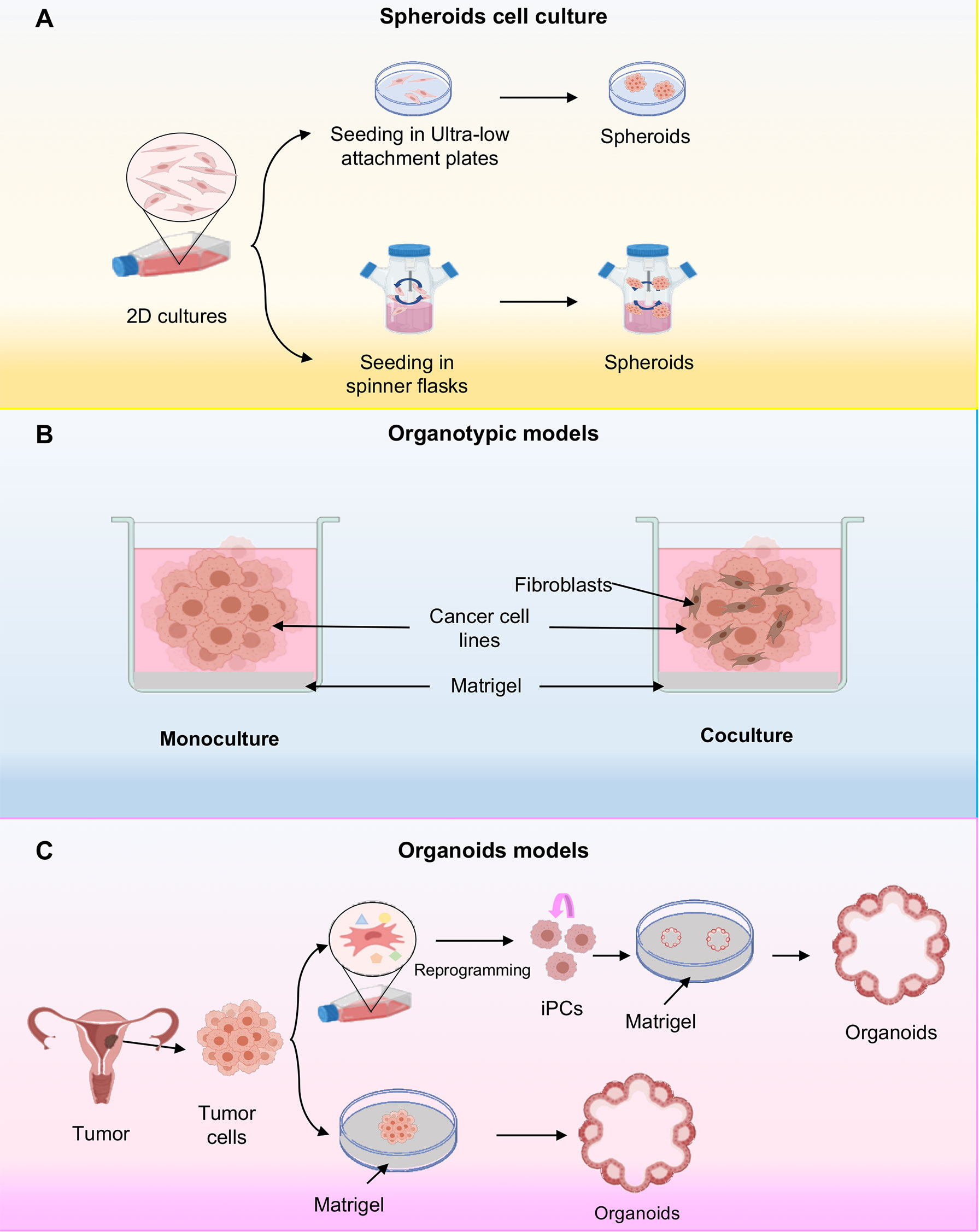
Figure 2 Three-dimensional cell cultures. (A) Scheme representing the cellular spheroids grown in ultra-low attachment plates. In this system, cancer cells are deposited on an ultralow fixation plate that prevents sticking and allowing the grown of cells in suspension; or alternatively they are placed in spinning flasks and subjected to gravitational forces also inducing the spheroids formation. (B) Schematic representing organotypic models, where organotypic models have been generated in monoculture or in combination with fibroblasts cocultures. (C) Representative schematic of organoid establishment from pluripotent stem cells (iPSCs) and cancer cells. The iPSCs first undergo reprogramming, followed by directed differentiation, and are then seeded into an extracellular matrix in a specific culture medium to initiate organoid culture. The tumor tissue organoids were processed to remove excess fat and necrotic cells and cut into small pieces. They are then seeded on Matrigel.
Organotypic Cultures of Cancer Cell Lines
The 3D culture systems or organotypic models are generated by in vitro culturing cancer cell lines in a semisolid extracellular matrix under defined culture medium conditions (Figure 2B) (24, 25). They are an attractive model as they recapitulate the characteristics of tumor cells in vivo with respect to growth kinetics, cellular heterogeneity, signaling pathway activity. Additionally, it has been shown that gene expression in 3D cultures is much closer to clinical expression profiles than those observed in traditional 2D monolayer culture (6). Interestingly, organotypic cultures show diverse morphologies depending on the inherent nature of the cell and culture conditions, for example, in breast cancer three different morphologies have been observed depending on the molecular subtype of the cell line, such as mass, grape bunch and stellate (26). Moreover, to further understand cancer biology, they have developed 3D co-culture models in order to effectively model the influences of the tumor microenvironment on drug efficacy. Thus, organotypic models possess features more appropriate for high-throughput screening assays compared to 2D conditions (9) (Figure 2B).
Organoid Models
Organoids are 3D systems that have been established for cancer research as they recapitulate the genotype, phenotype and cellular behavior of parental tissues (27). These innovative cultures models can be developed from both induced pluripotent stem cells (iPSCs), and tumor tissues (28, 29). Organoids established from induced pluripotent stem cells (iPSCs) begins with the isolation and culture of malignant cells from a primary or metastatic tumor sample (30, 31) (Figure 2C). Subsequently, reprogramming is carried out through gene transfer of SOX2, KLF4, c-MYC, and OCT4 transcription factors by means of retroviruses or lentiviruses (32). These cells then differentiate into the cell type of origin of the initial tumor. Differentiated iPSC-derived cells can be used to derive organoids. However, iPSC-derived organoids have major disadvantages because their efficacy depends on the type of cancer and the presence or absence of oncogenic mutations potentially selecting for the growth of tumor subclones and the loss of genetic heterogeneity of the tumor from which they are derived (33). In general, it is more practical to grow tumor organoids directly from tumor tissue. On the other hand, cancer tissue-derived organoids are established from the collection of tumor tissue after biopsy and placed on a matrigel-coated surface where it is embedded within the matrigel (34) (Figure 2C). The main advantage of this system is the preservation of the original tumor tissue architecture, including cellular and non-cellular components of the tumor microenvironment and cell-cell interactions However, the main disadvantage is the lack of reproducibility due to tumor heterogeneity (9, 35).
3D Cultures Systems in Gynecological Cancers
Traditional 2D cell cultures and animal models represent the experimental mainstay for gynecologic cancer research. However, their ability to reflect mechanisms occurring in vivo is limited. This is because the cellular models lack the tumor microenvironment and associated cellular interactions, which limits their application to clinical practice and research. Therefore, the development of technologies such as 3D culture will provide a novel alternative for gynecologic cancer research, as it allows to replicate several critical features of tissues including tumor morphology, differentiation, polarity, proliferation rate, gene expression, cell heterogeneity, and nutrient and oxygen gradients (2).
3D Cultures Systems in Cervical Cancer
Cervical cancer is the fourth most common cancer among women globally and therapy resistance is still a major problem to treat the disease (36). Hence, it is necessary to develop novel drugs and therapeutic approaches, as almost all drugs used today suffer from serious side effects due to drug resistance and lack of selectivity towards tumors (37). Recently, there has been an increasing interest in the development of 3D in vitro tumor models based on human cancer cells to accurately reproduce the characteristics of human cancer tissues (38). For instance, Zhao and coworkers demonstrated increased paclitaxel chemoresistance and proliferation rate in 3D cultures compared to traditional monolayer (2D) cultures of HeLa cells. In addition, HeLa cells increased the expression of matrix metalloproteinase (MMP) protein in 3D cultures (39). Similarly, Baek N and coworkers demonstrated increased resistance to doxorubicin in 3D cultures of HeLa cells compared to 2D cultures, resulting in higher IC50 values 11.2 and 9.6 μM of doxorubicin in 3D cultures at day 3 and 5, respectively, compared to monolayer cultures 1.0 μM of doxorubicin. The observed differences to doxorubicin sensitivity with respect to 2D and 3D cultures is due to monolayer cultured cells being well oxygenated, resulting in the rapid accumulation of reactive oxygen species (ROS) when exposed to DXR. In contrast, cells in the spheroid core are under hypoxic conditions, which makes them much more resistant (40). It has been shown that some plant constituents have anticancer activities, for example, Zataria essential oil (ZEO) is one of the useful essential oils that possesses extensive biological activities. The major components of ZEO have been shown to decrease the viability of breast cancer cells (41). Azadi M and coworkers demonstrated that ZEO treatment promotes inhibition of cell proliferation and promotes apoptosis in the TC1 cervical cancer cell line TC1 in both monolayer (2D) and multicellular spheroids (3D). In addition, ZEO was effective in tilting the cytokine balance in favor of T helper 1 through increased secretion of TNF-α, IFN-γ, IL-2 and decreased IL-4 (Figure 3) (42). It has been shown that bidirectional crosstalk between tumor and stroma plays an important role in the response to therapy. De Gregorio V and coworkers developed an organotypic cervical tumor model where they established this crosstalk, they developed two models 1) composed of primary human cervical fibroblasts (HCFs) embedded in the ECM, to produce normal cervical stroma (NCIS) and 2) composed of cervical cancer-associated fibroblasts (CCAFs), generating cervical cancer stroma (CCIS). They demonstrated increased gene expression of early viral E6 and E7 genes in SiHa cells when cultured in CCSI. Therefore, organotypic models of cancer can help to better understand cancer progression and establish novel anti-cancer therapeutic targets directed to tumor stroma and cancer cells (43).
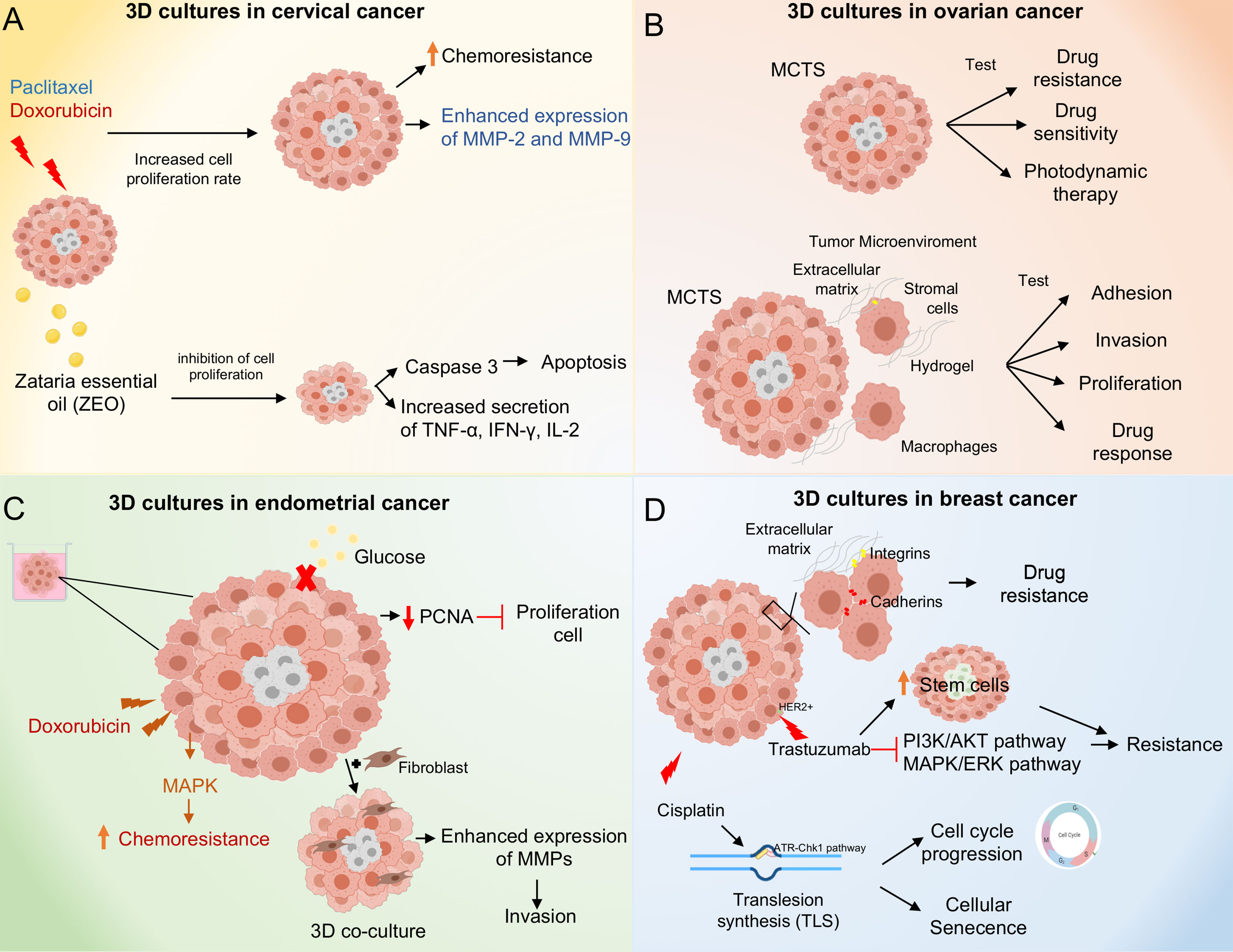
Figure 3 Molecular mechanisms activated in 3D culture systems. (A) 3D cultures of cervical cancer cells result in paclitaxel and doxorubicin chemoresistance through increased proliferation rate and overexpression of MMP-2 and 9. Zataria essential oil treatment inhibits cell proliferation in 3D cultures and induces apoptosis through activation of caspase 3. (B) 3D culture systems in ovarian cancer. The Multicellular Tumor Spheroids (MCTS) allow testing of drug and photodynamic therapies. The co-culture of MCTS with stromal cells and macrophages in combination with hydrogels as scaffold, allow to mimic the tumor microenvironment providing a model to test adhesion, invasion, proliferation processes as well as drug response. (C) 3D cultures systems in endometrial cancer. Doxorubicin treatment induces chemoresistance through activation of the MAPK pathway. Moreover, 3D cultures of endometrial cancer co-cultured with fibroblasts promote invasion through overexpression of MMPs. (D) 3D cultures systems in breast cancer. Spheroids of breast cancer cells exhibit cell-cell and extracellular cell-matrix interactions promoting drug resistance. Cisplatin treatment promotes cell cycle progression and cellular senescence through up-regulation of trans-lesion DNA polymerase (TLS) expression and activation of the ATR-Chk1 pathway. Trastuzumab treatment induces resistance in 3D cultures through inhibition of PI3K/AKT and ERK/MAPK pathways, in addition to an increase in stem cells subpopulations.
MicroRNAs Modulation in 3D Cervical Cancer Cultures
As major regulators of gene expression, its expected that miRNAs expression could be modulated in the 3D cultures, as several studies reported (44). The miR-143/145 cluster has been found downregulated in cervical cancer and overexpression of miR-143 or miR-145 inhibits cell viability, proliferation, migration, and invasion, in monolayers and 3D cultures of HeLa cervical cancer cell line. Furthermore, transfection of miRNA-145 increased pMLC levels by targeting the MYPT1 subunit of myosin regulatory phosphatase (45). Moreover, it has been shown that extracellular vesicles (EVs) secreted by 3D cultured tumor cells differ in terms of secretion dynamics and essential signaling molecular contents (RNA and DNA) compared to EVs derived from monolayer 2D cultures. These data suggested that EV small RNAs derived from 3D cultures may reflect EVs RNAs derived from in vivo tissues (46). Indeed, this is because cells in monolayer completely differ from the in vivo state where cells grow in 3D, in terms of cell morphology, cell-to-cell interactions, growth behavior and interactions with the extracellular matrix (1, 47). Thippabhotla and coworkers demonstrated that miRNAs expression profile of extracellular vesicles derived from 3D culture of HeLa cervical cancer cell line exhibited a high similarity of about 96% with circulating extracellular vesicles obtained from the plasma of cervical cancer patients, compared with the expression profile of EVs miRNAs derived from HeLa cell line growing in 2D. On the other hand, they demonstrated that culture and growth conditions do not affect genomic information, carried by EVs secretion, by DNA sequencing analysis (48). Currently, the number of studies focusing in the generation of organoids from primary cervical tissue is scarce. Recently, Lõhmussaar K, and coworkers established a protocol to generate 3D organoids from cervical tissue from both the endocervix and ectocervix that stably recapitulate cervical tissue. These organoids generated differential responses to chemotherapeutic agents, such as carboplatin, cisplatin, and gemcitabine, and grew as xenografts in mice (33, 49).
3D Cultures Systems in Ovarian Cancer
Ovarian cancer (OC) is considered the most lethal gynecological cancer due to its high metastatic potential and resistance to chemotherapeutic agents; because of these several studies have been dedicated to demonstrating the importance and influence of 3D cell cultures in the characterization and study of the OC. Due to the above, the need has arisen to develop culture supports and 3D cultures models that allow to mimic the tumor microenvironment to have greater efficiency when testing drugs, this because the use of in vitro screening methods on tissue culture polystyrene (TCPS) does not mimic the microenvironment of aggregates in vivo when evaluating the response to drugs (50). Additionally, the characteristics genotypic and phenotypic between the different cell lines, can lead to development different morphologies in the formation of spheroids and influence resistance or sensitivity in drug testing, obtaining variable results in the study between 2D and 3D models (51). Hirst and coworkers showed an increase in gene expression associated with hypoxia, drug resistance and stem cell markers in a Multicellular Tumor Spheroids (MCTS) model from epithelial ovarian cancer (EOC), interestingly, they identified that FDA-approved drugs as licofelone and glafenine reversed the gene expression found in MCST (52). The use of different techniques allows to optimize the development of tumor spheroids in OC; the co-culture between OC and mesothelial cells, promotes and facilitates OC spheroid formation in a 3D model, showing a structure of spheroids larger in 3D co-culture than OC cells in a monoculture (53). On the other hand, Angiotensin II (AGII) and its receptor AGTR1 enhanced the formation and increased the growth of OVCA429, and Isogenic highly metastatic OC (HM) tumor spheroids (54). Hydrogel supports made with Poly ethylene glycol-maleimide (PEG-MAL) allow emulation of the omentum, which has made it possible to evaluate resistance to drugs such as Pacitaxel and Mafosfamide as well as the sensitivity to drugs such as Carboplatin, Doxorubicin and LY2606368 in MCTS of SKOV-3 and Ovarian Carcinoma Ascites Spheroids (OCAS) patient-derived models, indicating a greater efficiency and potential use of 3D hydrogel omentum-based MCTS model in drug resistance and sensitivity tests compared with TCPS in OC patients (50). An 3D organotypic model omentum-based using OVCAR4 cells, showed that 3D organotypic models are a suitable tool to evaluate the delivery systems of potential nano-drugs using anti-metastatic nanoparticles with low toxicity such as RAPTA-C [Rutheniumdichloride (p-cymene) PTA] in order to optimize and increase the sensitivity in the treatment of OC (55). On the other hand, Verteporfin, a photosensitizer, was encapsulated efficiently within nanostructured lipid carriers, showing a greater sensitivity to light exposure and a higher cytotoxic effect after treatment in OVCAR3 and SKOV3 spheroids, demonstrating the application of 3D models in the search of photodynamic therapeutic prospects in OC therapy (56). The development and use of organoids models emerged as an alternative in the study of OC due to the limitations in the use of the spheroid models of cell lines. Organoids from EOC, particularly, fallopian tubes and ovarian surface epithelial cells organoids, are the main EOC organoid models used to study ovarian carcinogenesis; however, the lack of microenvironment is one of the principal limitations in the use of organoids because of that several anti-angiogenic, stromal-affecting, and immunotherapy drugs cannot be tested; the combination with the use of microfluid platform, could offer a partial solution to limitation previously mentioned (57).
The tumor microenvironment (TME) is a fundamental part in tumor development and progression, organotypic models consisting of stromal cells, such as human primary mesothelial cells (HPMC) or fibroblasts, and microfluidic models, could be a partial solution to the limitation of 2D culture. Regard 3D organotypic human mesothelium models, the key factors are the use of different ECM proteins and two mainly stromal primary cells, the purpose of this model is to study and identify potential molecules against adhesion, invasion, proliferation, and drug response on OC. The microfluidic 3D model is subjected to a continuous flow of growth factors and nutrients in order to mimic the TME by the flow of peritoneal fluid originated by OC, this model is useful mainly to evaluate the influence of macrophage infiltration in the TME development and its effect on the adhesion, tumorigenicity, proliferation, progression and trancoelomic metastasis in OC (58). The Tumor-associated macrophages (TAM) are key pieces to the survival and proliferation of free detached tumor cells from the primary tumor to form spheroids in early steps of transcoelomic metastasis, Long and coworkers established an in vitro spheroid formation assay with a co-culture system composed by GDP+F4/80+CD206+TAMs from an isolated of spheroids of ovarian cancer-bearing donor tomatoIysM-cre mouse, mixed with ID8 cells in a medium with matrigel. The model could support the lack of a tumor microenvironment in 3D models, particularly in the study of the effects of macrophage infiltration on the development and progression tumor (59). Additionally, a hetero-spheroid model, with OVCAR3 OC cells, Ovarian cancer stem cells (CSC) and CD68+ macrophages showed an increase in the expression of CD206, a M2 macrophage marker, IL-10 and WNT5B, in addition to presenting an increase in ALDH+ population and resistance to carboplatin treatment, showing a greater invasive capacity in CDS/M2 spheroids compared with OVCAR3/M0 spheroids, indicating the influence of macrophages in the modulation of the microenvironment of peritoneal fluid in the modulation of the WNT signaling and their relation with the development and progression in OC (60). Ward Rashidi and coworkers showed an increase in the population of ALDH+ from Passage 0 to 6 in an OC 3D hanging drop spheroid model of CSCs; interestingly, an increase in cisplatin resistance was observed in all spheroids serial passage, conversely, a reduction on the cell viability was observed in cells treated with 673A, an ALDH inhibitor, these finding highlights the usefulness of OC 3D model in the study of chemoresistance and tumorigenicity (61).
The gene expression profiles allow the comparative analysis of genotypic and phenotypic features between 2D and 3D cultures, as well as in primary OC tumors. An analysis of transcriptomic profiles in organotypic 3D model identified 1,182 genes differentially expressed. A comparison with primary tumors found 144 common genes that were deregulated in early metastatic colonization. The analysis of cell pathways identified the matrisome, core matrisome, ECM glycoproteins, ECM organization, matrisome associated, focal adhesion and integrin 1 as the main proteins and pathways modulated (62). In the same context, Paullin and coworkers performed a comparative transcriptomic analysis between 2D versus 3D spheroid OC models using HEY cells treated with TGFβ in order to induce the EMT. Results showed a different gene expression profile between models, among them, genes related to chemotherapy resistance (ARK1C1), ECM remodeling (PRSS35) and EMT enhancer transcription factors (SNAI1, SNAI2, ZEB2, TCF3 and SIXI) showed a differential gene expression in 3D compared with 2D culture. In relation to transcriptional networks modulated in 3D spheroids, analysis of the results identified sub-networks that include genes related to response to stress oxidative (PRDX2, CAT, SOD1 and GST01) and transcripts modulated related to heat shock response (HSP90AA1, HSPB1 and HSF1) that may contribute to stabilization of oncogenes and drug resistance (63) (Figure 3).
MicroRNAs Regulation in 3D Ovarian Cancer Cell Cultures
The 3D models can also help to study regulatory mechanisms in OC mediated by miRNAs, in this context, Yoshimura and coworkers evaluated the effect of miR-99a-5p, an microRNA overexpressed in EOC, in peritoneal dissemination, using human peritoneal mesothelial cells (HPMCs) treated with EOC-derived exosomes. Results showed that overexpression of miR-99a-5p in HMPCs promoted the EOC invasion by affecting HPMCs by fibronectin and vitronectin upregulation suggesting that it could be considered as an EOC biomarker in serum and a potential therapeutic target (64). Altogether, reports indicate various advantages of the use of the 3D models culture models for the study and characterization of the development, progression, invasion and treatments of ovarian cancer.
3D Cultures Systems in Endometrial Cancer
Endometrial cancer is the most prevalent gynecologic malignancy and a leading cause of morbidity and mortality in females (65). Endometrial cancer has been classified into two main groups, type I or type II, according to their clinicopathologic and molecular characteristics; estrogen-dependent type 1 endometrioid adenocarcinomas account for 80% of cases and are associated with endometrial hyperplasia with characteristic mutations in KRAS, PIK3CA and PTEN whereas type 2 tumors are of non-endometrioid histology, are estrogen-independent, are associated with endometrial atrophy and usually have mutations in TP53 and HER-2 (66, 67). Nowadays, there are several preclinical models in endometrial cancer to evaluate drug efficacy and predict patient outcomes. These include traditional monolayer 2D cultures, organoids, spheroids and animal models. However, some models present limitations (68, 69). For example, Chitcholtan and coworkers demonstrated reduced proliferation in 3D cultures of Ishikawa, RL95-2, EN-1078D and KLE endometrial cancer cell lines compared to 2D cultures, which correlated with decreased expression of the proliferative protein marker PCNA. In addition, altered metabolic phenotypes were observed, including decreased glucose uptake, independent of glucose transporter (GLUT) expression, and down-regulation of vascular epidermal growth factor (VEGF) secretion (70). Together, these data demonstrate that 3D cultures can affect the proliferation and metabolic behavior of endometrial cancer cells compared to 2D cells. Thus, the use of these in vitro models to assess the drug response in preclinical trials is important. The 3D cultures of RL95-2 and KLE endometrial cancer cell lines showed increased resistance to doxorubicin compared to 2D cultures, this was due to MAPK inactivation (71). Likewise, it has been shown that deletion of the ETS translocation variant 4 (ETV4), a candidate factor controlling ER genomic binding in endometrial cancer cells, led to decreased growth in 3D cultures of the Ishikawa endometrial cancer cell line (72). Nevertheless, one of the main limitations of these systems is the lack of incorporation of non-epithelial cells, which is why 3D co-cultures are now being developed, incorporating both stromal and epithelial cells. For this reason, 3D co-cultures are now being developed, incorporating both stromal and epithelial cells. These spheroids are phenotypically comparable to endometrial cancer tissue in vivo. In other study, Al-Juboori and coworkers performed a proteomic analysis to assess the biological relevance of spheroids in 3D co-culture (HESC/Ishikawa), they found 591 common proteins and canonical pathways that are closely related to endometrial biology in the 3D co-culture model compared to human endometrial tissue (73). On the other hand, the influence of fibroblasts on the invasion of endometrial cancer cells in 3D cultures has been analyzed, showing that Ishikawa endometrial cancer cells co-cultured with fibroblasts in 3D show a high invasion capacity and over-express proteins such as metalloproteinases (MMPs) and plasminogen activators (PA), compared to 3D cultures without fibroblasts (74). Other types of 3D preclinical models relevant to endometrial cancer patients are patient-derived organoids, patient-derived xenografts and patient-derived explants (75). Maru, Y and coworkers established a Matrigel bilayer organoid culture (MBOC) in gynecological tumors, demonstrating that the recovered organoids basically retained the characteristics of the original tumors (76).
3D Cultures Systems in Breast Cancer
Breast cancer is a major public health problem due to its high incidence and mortality, being the most common cancer in women worldwide (77). The search for new drugs against breast cancer remains an important field in cancer research. However, the results of the effectiveness of treatments obtained in vitro have not been reproduced in the clinic. This is largely because most pre-clinical studies are generated from 2D cultures that do not resemble the “true biology” of the tumor in vivo (78, 79). In an early study, dit Faute and coworkers demonstrated that 3D cultures resulted in decreased proliferative rate of both MCF-7 breast cancer cell line and multi-resistant cells (MDR-MCF-7), reduced drug sensitivity of MCF-7 cells, and did not affect the resistance of MDR-MCF-7 cells. In addition, transmission electron microscopy assays demonstrated that MCF-7 cells grown as spheroids had a junctional system involving E-cadherin, tight-junctions and desmosomes, promoting drug resistance. Interestingly, in MCF-7 multi-resistant cell spheroids, cell cohesion was mostly due to membrane interdigitations, induced invasive properties (80). Another study group tested the sensitivity of cisplatin of the MCF-7 cell line grown in 2D and 3D cultures. Similarly, they demonstrated that resistance to cisplatin was mainly generated in 3D cultures which seems to be generated by interactions with the tumor microenvironment. Interestingly, it was demonstrated that 3D-cultured cells were able to progress through the S-cell cycle phase, due to the upregulation of translesion (TLS) DNA polymerase expression and the activation of the ATR-Chk1 pathway. Furthermore, co-treatment with a pharmacological ATR inhibitor (VE-821) generated a response to cisplatin (81).
In other study, Lovitt and coworkers found that spheroids cells displayed more chemoresistance to doxorubicin corresponding to higher IC50 values than conventional monolayer cells in MCF-7 and MDA-MB-23 breast cancer cell lines, mediated by cell-to-ECM interactions. Interestingly, inhibition of integrin signaling in combination with doxorubicin reduced the viability of breast cancer cells (82). Recently, a 3D-μTP culture system was established; it was manufactured by seeding tumor and/or fibroblast cells on biodegradable porous microcarriers in a dynamic culture system (83). Similarly, the efficacy of doxorubicin (DOX) was evaluated in two different 3D cancer models: microtissue (3D-μTP) versus spheroid, both models were formed by co-culturing MCF-7 cell line with fibroblasts. It was demonstrated that the 3D-μTP model showed increased DOX diffusion and decreased cell viability compared to spheroid. Moreover, they demonstrated that, beside multi-cellularity, the presence of a cell-assembled ECM in the 3D-μTP model also played a crucial role in modulating the drug response (84). Another effective treatment for breast cancer is trastuzumab, a recombinant humanized monoclonal antibody targeting human epidermal growth factor receptor 2 (HER2), a gene frequently amplified in 30% of breast cancer cases, and associated with poor prognosis in breast cancer patients (85). Several studies have demonstrated that trastuzumab significantly improved the prognosis of breast cancer patients with HER2 overexpression (86). This is because trastuzumab inhibits several signaling pathways, such as phosphatidylinositol 3-kinase (PI3K)-AKT serine/threonine kinase 1 and mitogen activated protein kinase (MEK)/extracellular signal regulated kinase (ERK) (87). Tatara and coworkers demonstrated that 3D cultures better simulate the cytological and biochemical responses to trastuzumab-induced apoptosis and resistance to trastuzumab associated with the PIK3CA mutation compared to 2D cultures. They observed increased expression of poly (ADP-ribose) polymerase (PARP) cleaved only in PIK3CA-wt lines grown in 3D in response to trastuzumab, but not in PIK3CA-wt or PIK3CA-mt lines grown in 2D (88).
Likewise, Gangadhara and coworkers demonstrated that breast cancer cell lines grown in 3D Matrigel-based culture system showed resistance to trastuzumab compared to 2D cultures, generated by AKT/MAPK extracellular matrix-mediated signaling. Interestingly, MAPK suppression in 3D cultures restoring the therapeutic response (89). Finally, Rodriguez and coworkers demonstrated that the hypoxic environment developed in the spheroids modulates the response to Trastuzumab in the breast cancer cell line HER2+. Furthermore, the acquired resistance to Trastuzumab in 3D cultures was associated with an increase in the population of cancer stem cells (90). Another anti-tumor chemotherapeutic agent is the taxane paclitaxel (Ptx) which binds to- and stabilizes cytoskeleton microtubules resulting in mitosis inhibition (91). Recently, new strategies have been described that allow the drug to accumulate at the site of the tumor and simultaneously decrease the concentration in the rest of the body, thus avoiding serious side effects, such as bone marrow suppression and neurotoxicity (92). This targeted drug delivery can be achieved by magnetic drug targeting (MDT). Lugert and coworkers developed Ptx-functionalized super paramagnetic iron oxide (SPION) nanoparticles coated with lauric acid (LA) and human serum albumin (HSA; SPION LA-HSA-Ptx) and analyzed their efficacy in different breast cancer cell lines cultured in 2D and 3D. They demonstrated that the binding of the antiproliferative and antitumor agent Ptx to the biocompatible and magnetically susceptible carrier SPION LA-HSA was effective in different breast cancer cell lines and did not influence the cytotoxic efficacy of the chemotherapeutic drug. Furthermore, they found not significant differences between the 2D and 3D culture systems (93).
On the other hand, compounds of natural origin with anti-cancer activity have been investigated. An example is Ginger (Zingiber officinale Roscoe). Ginger is the rhizome of plants in the Zingiberaceae family and has been widely used as a medicinal plant for thousands of years, due to its phenolic compounds, [4], [6], [8], and [10]-gingerols (94). It has been demonstrated that gingerols, have multiple anti-cancer effects, inhibiting the cellular proliferation of MDA-MB-231 triple negative breast cancer cells compared to non-tumor cells (95). Therefore, Fuzer and coworkers analyzed the anti-cancer activity of [10]-gingerol in breast cancer HMT-3522 cells growing in lr-ECM in 3D culture. They demonstrated that [10]-gingerol promoted cytotoxicity in linear HMT-3522 (T4-2) cells compared to non-malignant S1 cells. Furthermore, [10]-gingerol induced apoptosis in the HMT-3522 (T4-2) cell line in breast cancer (96).
Interestingly, extracellular matrix signals have been demonstrated to play a crucial role in apoptotic sensitivity in response to chemotherapeutic agents for non-malignant and malignant breast cell lines in 2D and 3D culture (97, 98). This is largely because cells grown in 3D adopt morphologies similar to those of tissues in vivo. Kenny and coworkers analyzed the morphological phenotype of 25 of these breast cell lines grown in 2D and 3D cultures, and their gene expression profiles under these same conditions. They demonstrated that breast cancer cell lines grown in 2D did not show different morphologies, however, when grown in 3D they adopted four different morphologies called: Round, Massive, Grape-like and Stellate. Furthermore, the 3D microenvironment produced significant changes in the gene expression profiles of these cancer cell lines (26). In particular, genes encoding proteins involved in signal transduction were over-expressed in 3D cell cultures compared to 2D cultures. Therefore, it is important to understand that cancer is a complex process that depends both on the behavior of the cancer cells and on the function of the non-malignant supporting cells in the tumor microenvironment (99). Tumor-associated mesenchymal stromal cells (TA-MSC) are a major component of the tumor microenvironment; they contribute to cancer progression by promoting metastasis, vascularization of the tumor and contribute to cancer cell resistance to chemotherapy (100). One way to study these TA-MSC is through 3D cell cultures, because cell signaling, and drug responses differ when cells are cultured on rigid 2D substrates or using 3D cell culture systems that more closely mimic the tumor microenvironment (101). Blache and coworkers demonstrated that secretions from the 3D-cultured MDA-MB-231 breast cancer cell line convert mesenchymal stromal cells (MSC) to MSC-AT, generating an immunomodulatory phenotype that is particularly prominent in response to bone-tropic cancer cells (102). The development of 3D cultures allows us to understand the molecular mechanisms of drug resistance and the biology of breast cancer (Figure 3D).
MicroRNAs Regulation in 3D Breast Cancer Cell Cultures
The expression and function of miRNAs in breast cancer cells have long been derived from 2D cultures, which lack the tumor microenvironment. However, recently the expression of miRNAs in 3D versus 2D cultures in different breast cancer cell lines has been described. For example, Nguyen and coworkers analyzed the expression profile of miRNAs in 3D compared to 2D cultures in the MCF-7 (non-invasive) and MDA-MB-231 (invasive) breast cancer cell lines. They showed that 49 miRNAs were differentially expressed in the MCF-7 cell line in 3D cultures compared to 2D, of those 24 were upregulated and 25 were downregulated. Whereas, in the MDA-MB231 cell line, 28 miRNAs were differentially expressed, with 22 miRNAs upregulated and 6 miRNAs downregulated. In addition, two miR-200 family members, miR-141 and miR-429 were overexpressed only in 3D cultures in the MCF-7 cell line. Overexpression of miR-429 in MDA-MB231 cells attenuated their invasive stellate morphology in 3D culture. This suggests that the differential expression profile between the two cell lines is probably due to miRNAs regulating mass morphology in the MCF-7 cell line and invasive stellate morphology in MDA-MB231 cells (25). Furthermore, it has been shown that the expression profiles of miRNAs in MDA-MB-231 cell line cultured in 3D are like the changes reported in highly invasive breast tumors. For example, miR-146a-5p, which regulates cancer progression or miR-210, which is over-expressed in response to hypoxia in breast cancer. Suggesting that 3D cultures better mimic tumors in vivo than traditional 2D culture (103).
On the other hand, the use of natural compounds in the treatment of cancer is increasing. Such is the case of silibinin, which is a natural flavonoid, and the anticancer and chemopreventive effects of silibinin have been demonstrated in different types of cancer (104). Yazdi and coworkers analyzed the effect of silibinin on cell viability and miRNA expression in 3D and 2D cultures of the T47D breast cancer cell line. They demonstrated that the 3D cultures show higher drug resistance, similar to what occurs in vivo and is largely due to the fact that the cells are in different stages of growth, including proliferation, hypoxia, apoptosis, necrosis and quiescent phase. Furthermore, they demonstrated that silibinin promotes apoptosis in both 3D and 2D cultures. Finally, they demonstrated decreased expression of miR-21, miR-15a, and miR-141, in silibinin-treated cells in 3D and 2D cultures of the T47D cell line (105).
3D Cultures Applications in Gynecological and Breast Cancer Translational Research
3D culture models in gynecological and breast cancer provide a valuable platform to investigate the molecular processes leading to uncontrolled cell proliferation and metastasis which may allow for novel drugs discovery. This is of utmost importance, because resistance to chemo- and radiotherapy is common in patients with gynecological and breast cancer, in indeed a large proportion of patients undergo excessively toxic treatments with no or minimal therapeutic benefit (106). The utilization of 3D cultures and organoids will help to predict the responsiveness of patients to treatments and will allow tailoring specific treatments for each patient, resulting in personalized therapies. For example, Boretto, M and coworkers developed organoid cultures derived from endometrial cancer patients as preclinical models for screening drugs, for screening drugs, such as paclitaxel, 5-fluorouracil, carboplatin, doxorubicin) and everolimus (mTOR inhibitor), showed patient-specific responses (107). Furthermore, the STAT3 transcription factor inhibitor, BBI608 (Napabucasin), strongly inhibited the growth of patient-derived organoids through inhibition of growth receptor tyrosine kinase (108).
On the other hand, one of the main characteristics of ovarian cancer is its genetic heterogeneity, so differential responses to drugs in ovarian cancer have to be expected. The development of organoids derived from individual ovarian cancer lines will allow screening for different drugs, for example, the HGS-3.1 organoid line was sensitive to gemcitabine, adavosertib, carboplatin and paclitaxel and resistant to drugs targeting the PI3K/AKT/mTOR pathway, while the HGS-23 line showed a pattern of sensitivity to the opposite drugs. Furthermore, organoids have been demonstrated to capture tumor heterogeneity (106). The radiosensitivity of cervical cancer organoids has been investigated. Nakajima, A and coworkers demonstrated that organoid growth was inhibited in a dose-dependent manner one week after irradiation. Radiosensitivity was patient-specific and matched the response of the xenografted tumor and the patient. Interestingly, hypoxia-inducible factor 1α (HIF-1α) target gene expression was up-regulated in organoids derived from resistant cancer tissues. HIF-1α protein levels increased several hours after irradiation (109). Finally, breast cancer organoids reflect tumor heterogeneity, it has been observed that breast cancer organoids can achieve 60% or more similarity of characteristics and gene profile expression with tumors in vivo (110). Furthermore, breast cancer organoids can be used as an effective in vitro model for the study of personalized treatment. Garcia-Davis, S and coworkers evaluated the antitumor effect of laurinterol, the main compound of an ethanolic extract of Laurencia johnstonii on breast cancer organoids. They found a dose-dependent inhibition of metabolic activity, as well as morphological and nuclear changes characteristic of apoptosis. However, they observed a heterogeneous response that was associated with the individual response of each human tumor sample, being associated with intratumoral heterogeneity (111). Recently, Carter and coworkers developed an experimental protocol to infect breast cancer organoid cultures with oncolytic viruses and compared the oncolytic effects of a measles vaccine virus (MeV) and a vaccinia virus (GLV), genetically modified, allowing enzymatic conversion of the 5-fluorocytosine (5-FC) prodrug into the cytotoxic compounds 5-fluorouracil (5-FU) and 5-fluorouridine monophosphate (5-FUMP), to investigate the effects of oncolytic virotherapy. They demonstrated that oncolytic viruses significantly inhibited cell viability in organoid cultures derived from breast cancer tissue. Thus, the model provides a promising in vitro method to aid further testing of virotherapeutic vectors for in vivo use (112).
At present, breast and gynecological cancer organoids represent an optimal model for the compression of tumor biology, and their applications in the screening of new tumor drugs has significantly contributed to clinical applications (113). However, although promising breast and gynecological cancer organoids have some limitations such as: i) they lack the complete technology to simultaneously connect organoids and tumor microenvironment, ii) the generation of breast and gynecological cancer organoids from patients are mainly surgical tissue or puncture, however, it is believed that some of the cellular heterogeneity of in vivo tumors is lost in the sampling process and iii) they lack mesenchymal cells, so they do not have nervous and vascular system, presenting some differences with solid tumors (114, 115). These limitations must be overcome in the immediate future of 3D and organoids technologies to contribute to the advance of the field. In the future, 3D and organoid culture models combined with recent biotechnological progress will offer exciting improvements for the precise application of this technology.
Conclusions
Although 2D cultures have been used for a long time, they do not reflect the biology of cancer, making them an inefficient model to study the processes associated with cellular responses to chemotherapeutic exposure. On the other hand, the establishment of 3D cultures are potentially a better approach in the search for new biomarkers and new treatment strategies in breast and gynecological cancer, due to their physiological relevance bringing us closer to the goal of personalized medicine. The main contributions of 3D cultures in gynecological and breast cancer can be summarized as follows i) they have improved our understanding of cancer biology, ii) they have helped to better understand the molecular mechanisms of drug resistance by the identification on novel players in these processes, iii) they capture phenotypic heterogeneity, iv) they modify gene expression and cell behavior in a similar way to in vivo condition, v) and they mimic the tumor micro-environment in a similar way to in vivo tumors. In conclusion the development of 3D culture models in breast and gynecologic cancer will help to further understand cancer biology and to develop new drugs and predict drug response to address poor response rates and improve survival outcomes in patients.
Author Contributions
CL-C, YS-V, JV, LM, and RR-P conceived and designed the review. CL-C, YS-V, JV, YP-N, GM-L, LM, RR-P, and SN-O performed the literature review and were involved in the writing and revision of the manuscript. YS-V, YP-N, CP-P, and GM-L prepared all the figures. All authors have read and agreed to the published version of the manuscript.
Funding
This research was funded by Consejo Nacional de Ciencia y Tecnologia (CONACYT), Mexico, Grant FORDECYT-PRONACES/51207/2020. Convenio I1200/189/2020. YS-V and SN-O received scholarships 754782 and 789774, respectively, from CONACYT, Mexico.
Conflict of Interest
The authors declare that the research was conducted in the absence of any commercial or financial relationships that could be construed as a potential conflict of interest.
Publisher’s Note
All claims expressed in this article are solely those of the authors and do not necessarily represent those of their affiliated organizations, or those of the publisher, the editors and the reviewers. Any product that may be evaluated in this article, or claim that may be made by its manufacturer, is not guaranteed or endorsed by the publisher.
Acknowledgments
We acknowledge to Universidad Autonoma de la Ciudad de Mexico for support.
References
1. Pampaloni F, Reynaud EG, Stelzer EH. The Third Dimension Bridges the Gap Between Cell Culture and Live Tissue. Nat Rev Mol Cell Biol (2007) 8:839–45. doi: 10.1038/nrm2236
2. Cukierman E, Pankov R, Stevens DR, Yamada KM. Taking Cell-Matrix Adhesions to the Third Dimension. Science. (2001) 294:1708–12. doi: 10.1126/science.1064829
3. Mseka T, Bamburg JR, Cramer LP. ADF/cofilin Family Proteins Control Formation of Oriented Actin-Filament Bundles in the Cell Body to Trigger Fibroblast Polarization. J Cell Sci (2007) 120:4332–44. doi: 10.1242/jcs.017640
4. Weiswald LB, Bellet D, Dangles-Marie V. Spherical Cancer Models in Tumor Biology. Neoplasia. (2015) 17:1–15. doi: 10.1016/j.neo.2014.12.004
5. Baal N, Widmer-Teske R, McKinnon T, Preissner KT, Zygmunt MT. In Vitro Spheroid Model of Placental Vasculogenesis: Does it Work? Lab Invest (2009) 89:152–63. doi: 10.1038/labinvest.2008.126
6. Friedrich J, Seidel C, Ebner R, Kunz-Schughart LA. Spheroid-Based Drug Screen: Considerations and Practical Approach. Nat Protoc (2009) 4:309–24. doi: 10.1038/nprot.2008.226
7. Baker BM, Chen CS. Deconstructing the Third Dimension: How 3D Culture Microenvironments Alter Cellular Cues. J Cell Sci (2012) 125:3015–24. doi: 10.1242/jcs.079509
8. Mehta G, Hsiao AY, Ingram M, Luker GD, Takayama S. Opportunities and Challenges for Use of Tumor Spheroids as Models to Test Drug Delivery and Efficacy. J Control Release (2012) 164:192–204. doi: 10.1016/j.jconrel.2012.04.045
9. Nath S, Devi GR. Three-Dimensional Culture Systems in Cancer Research: Focus on Tumor Spheroid Model. Pharmacol Ther (2016) 163:94–108. doi: 10.1016/j.pharmthera.2016.03.0137
10. Kleinman HK, Philp D, Hoffman MP. Role of the Extracellular Matrix in Morphogenesis. Curr Opin Biotechnol (2003) 14:526–32. doi: 10.1016/j.copbio.2003.08.002
11. Katt ME, Placone AL, Wong AD, Xu ZS, Searson PC. In Vitro Tumor Models: Advantages, Disadvantages, Variables, and Selecting the Right Platform. Front Bioeng Biotechnol (2016) 4:12. doi: 10.3389/fbioe.2016.00012
12. Yang J, Huang S, Cheng S, Jin Y, Zhang N, Wang Y. Application of Ovarian Cancer Organoids in Precision Medicine: Key Challenges and Current Opportunities. Front Cell Dev Biol (2021) 9:701429. doi: 10.3389/fcell.2021.701429
13. Rosso F, Giordano A, Barbarisi M, Barbarisi A. From Cell-ECM Interactions to Tissue Engineering. J Cell Physiol (2004) 199:174–80. doi: 10.1002/jcp.10471
14. Antoni D, Burckel H, Josset E, Noel G. Three-Dimensional Cell Culture: A Breakthrough In Vivo. Int J Mol Sci (2015) 16:5517–27. doi: 10.3390/ijms16035517
15. Calin GA, Croce CM. MicroRNA Signatures in Human Cancers. Nat Rev Cancer (2006) 6:857–66. doi: 10.1038/nrc1997
16. Lin S, Gregory RI. MicroRNA Biogenesis Pathways in Cancer. Nat Rev Cancer (2015) 15:321–33. doi: 10.1038/nrc3932
17. Lerner RG, Petritsch C. A microRNA-Operated Switch of Asymmetric-to-Symmetric Cancer Stem Cell Divisions. Nat Cell Biol (2014) 16:212–4. doi: 10.1038/ncb2924
18. Pencheva N, Tavazoie SF. Control of Metastatic Progression by microRNA Regulatory Networks. Nat Cell Biol (2013) 15:546–54. doi: 10.1038/ncb2769
19. Bouchie A. First microRNA Mimic Enters Clinic. Nat Biotechnol (2013) 31:577. doi: 10.1038/nbt0713-577
20. Sutherland RM, McCredie JA, Inch WR. Growth of Multicell Spheroids in Tissue Culture as a Model of Nodular Carcinomas. J Natl Cancer Inst (1971) 46:113–20.
21. Kelm JM, Timmins NE, Brown CJ, Fussenegger M, Nielsen LK. Method for Generation of Homogeneous Multicellular Tumor Spheroids Applicable to a Wide Variety of Cell Types. Biotechnol Bioeng (2003) 83:173–80. doi: 10.1002/bit.10655
22. Lin RZ, Chang HY. Recent Advances in Three-Dimensional Multicellular Spheroid Culture for Biomedical Research. Biotechnol J (2008) 3:1172–84. doi: 10.1002/biot.200700228
23. Hoarau-Véchot J, Rafii A, Touboul C, Pasquier J. Halfway Between 2D and Animal Models: Are 3d Cultures the Ideal Tool to Study Cancer-Microenvironment Interactions? Int J Mol Sci (2018) 19:181. doi: 10.3390/ijms19010181
24. Lee GY, Kenny PA, Lee EH, Bissell MJ. Three-Dimensional Culture Models of Normal and Malignant Breast Epithelial Cells. Nat Methods (2007) 4:359–65. doi: 10.1038/nmeth10157
25. Nguyen HT, Li C, Lin Z, Zhuang Y, Flemington EK, Burow ME, et al. The microRNA Expression Associated With Morphogenesis of Breast Cancer Cells in Three-Dimensional Organotypic Culture. Oncol Rep (2012) 28(1):117–26. doi: 10.3892/or.2012.1764
26. Kenny PA, Lee GY, Myers CA, Neve RM, Semeiks JR, Spellman PT, et al. The Morphologies of Breast Cancer Cell Lines in Three-Dimensional Assays Correlate With Their Profiles of Gene Expression. Mol Oncol (2007) 1:84–96. doi: 10.1016/j.molonc.2007.02.004
27. Messner S, Agarkova I, Moritz W, Kelm JM. Multi-Cell Type Human Liver Microtissues for Hepatotoxicity Testing. Arch Toxicol (2013) 87:209–13. doi: 10.1007/s00204-012-0968-2
28. Xu H, Lyu X, Yi M, Zhao W, Song Y, Wu K. Organoid Technology and Applications in Cancer Research. J Hematol Oncol (2018) 11:116. doi: 10.1186/s13045-018-0662-9
29. Lancaster MA, Knoblich JA. Organogenesis in a Dish: Modeling Development and Disease Using Organoid Technologies. Science. (2014) 345:1247125. doi: 10.1126/science.1247125
30. Clevers HC. Organoids: Avatars for Personalized Medicine. Keio J Med (2019) 68:95. doi: 10.2302/kjm.68-006-ABST
31. Kim J, Hoffman JP, Alpaugh RK, Rhim AD, Reichert M, Stanger BZ, et al. An iPSC Line From Human Pancreatic Ductal Adenocarcinoma Undergoes Early to Invasive Stages of Pancreatic Cancer Progression. Cell Rep (2013) 3:2088–99. doi: 10.1016/j.celrep.2013.05.036
32. Takahashi K, Yamanaka S. A Decade of Transcription Factor-Mediated Reprogramming to Pluripotency. Nat Rev Mol Cell Biol (2016) 17:183–93. doi: 10.1038/nrm.2016.8
33. Papapetrou EP. Patient-Derived Induced Pluripotent Stem Cells in Cancer Research and Precision Oncology. Nat Med (2016) 22:1392–401. doi: 10.1038/nm.4238
34. Kondo J, Endo H, Okuyama H, Ishikawa O, Iishi H, Tsujii M, et al. Retaining Cell-Cell Contact Enables Preparation and Culture of Spheroids Composed of Pure Primary Cancer Cells From Colorectal Cancer. Proc Natl Acad Sci USA (2011) 108:6235–40. doi: 10.1073/pnas.1015938108
35. Ritter CA, Perez-Torres M, Rinehart C, Guix M, Dugger T, Engelman JA, et al. Human Breast Cancer Cells Selected for Resistance to Trastuzumab In Vivo Overexpress Epidermal Growth Factor Receptor and ErbB Ligands and Remain Dependent on the ErbB Receptor Network. Clin Cancer Res (2007) 13:4909–19. doi: 10.1158/1078-0432.CCR-07-0701
36. Vu M, Yu J, Awolude OA, Chuang L. Cervical Cancer Worldwide. Curr Probl Cancer (2018) 42:457–65. doi: 10.1016/j.currproblcancer.2018.06.003
37. Newell H, Sausville E. Cytotoxic Drugs: Past, Present and Future. Cancer Chemother Pharmacol (2016) 77:1. doi: 10.1007/s00280-015-2917-2
38. Horning JL, Sahoo SK, Vijayaraghavalu S, Dimitrijevic S, Vasir JK, Jain TK, et al. 3-D Tumor Model for In Vitro Evaluation of Anticancer Drugs. Mol Pharm (2008) 5:849–62. doi: 10.1021/mp800047v
39. Zhao Y, Yao R, Ouyang L, Ding H, Zhang T, Zhang K, et al. Three-Dimensional Printing of Hela Cells for Cervical Tumor Model In Vitro. Biofabrication (2014) 6:35001. doi: 10.1088/1758-5082/6/3/035001
40. Baek N, Seo OW, Kim M, Hulme J, An SS. Monitoring the Effects of Doxorubicin on 3D-Spheroid Tumor Cells in Real-Time. Onco Targets Ther (2016) 9:7207–18. doi: 10.2147/OTT.S112566
41. Salehi F, Behboudi H, Kavoosi G, Ardestani SK. Monitoring ZEO Apoptotic Potential in 2D and 3D Cell Cultures and Associated Spectroscopic Evidence on Mode of Interaction With DNA. Sci Rep (2017) 7:2553. doi: 10.1038/s41598-017-02633-z
42. Azadi M, Jamali T, Kianmehr Z, Kavoosi G, Ardestani SK. In-Vitro (2D and 3D Cultures) and in-Vivo Cytotoxic Properties of Zataria Multiflora Essential Oil (ZEO) Emulsion in Breast and Cervical Cancer Cells Along With the Investigation of Immunomodulatory Potential. J Ethnopharmacol (2020) 257:112865. doi: 10.1016/j.jep.2020.112865
43. De Gregorio V, La Rocca A, Urciuolo F, Annunziata C, Tornesello ML, Buonaguro FM, et al. Modeling the Epithelial-Mesenchymal Transition Process in a 3D Organotypic Cervical Neoplasia. Acta Biomater (2020) 116:209–22. doi: 10.1016/j.actbio.2020.09.006
44. Pasquinelli AE. MicroRNAs and Their Targets: Recognition, Regulation and an Emerging Reciprocal Relationship. Nat Rev Genet (2012) 13:271–82. doi: 10.1038/nrg3162
45. González-Torres A, Bañuelos-Villegas EG, Martínez-Acuña N, Sulpice E, Gidrol X, Alvarez-Salas LM. MYPT1 is Targeted by miR-145 Inhibiting Viability, Migration and Invasion in 2D and 3D HeLa Cultures. Biochem Biophys Res Commun (2018) 507:348–54. doi: 10.1016/j.bbrc.2018.11.039
46. Taylor DD, Gercel-Taylor C. MicroRNA Signatures of Tumor-Derived Exosomes as Diagnostic Biomarkers of Ovarian Cancer. Gynecol Oncol (2008) 110:13–21. doi: 10.1016/j.ygyno.2008.04.033
47. Li Y, Kilian KA. Bridging the Gap: From 2D Cell Culture to 3D Microengineered Extracellular Matrices. Adv Healthc Mater (2015) 4:2780–96. doi: 10.1002/adhm.201500427
48. Thippabhotla S, Zhong C, He M. 3D Cell Culture Stimulates the Secretion of In Vivo Like Extracellular Vesicles. Sci Rep (2019) 9:13012. doi: 10.1038/s41598-019-49671-3
49. Lõhmussaar K, Oka R, Espejo Valle-Inclan J, Smits M, Wardak H, Korving J, et al. Patient-Derived Organoids Model Cervical Tissue Dynamics and Viral Oncogenesis in Cervical Cancer. Cell Stem Cell (2021) 28:1380–1396.e6. doi: 10.1016/j.stem.2021.03.012
50. Brooks EA, Gencoglu MF, Corbett DC, Stevens KR, Peyton SR. An Omentum-Inspired 3D PEG Hydrogel for Identifying ECM-Drivers of Drug Resistant Ovarian Cancer. APL Bioeng (2019) 3:026106. doi: 10.1063/1.5091713
51. Heredia-Soto V, Redondo A, Berjón A, Miguel-Martín M, Díaz E, Crespo R, et al. High-Throughput 3-Dimensional Culture of Epithelial Ovarian Cancer Cells as Preclinical Model of Disease. Oncotarget. (2018) 9:21893–903. doi: 10.18632/oncotarget.25098
52. Hirst J, Pathak HB, Hyter S, Pessetto ZY, Ly T, Graw S, et al. Licofelone Enhances the Efficacy of Paclitaxel in Ovarian Cancer by Reversing Drug Resistance and Tumor Stem-Like Properties. Cancer Res (2018) 78:4370–85. doi: 10.1158/0008-5472.CAN-17-3993
53. Shishido A, Mori S, Yokoyama Y, Hamada Y, Minami K, Qian Y, et al. Mesothelial Cells Facilitate Cancer Stem−Like Properties in Spheroids of Ovarian Cancer Cells. Oncol Rep (2018) 40:2105–14. doi: 10.3892/or.2018.6605
54. Zhang Q, Yu S, Lam MMT, Poon T, Sun L, Jiao Y, et al. Angiotensin II Promotes Ovarian Cancer Spheroid Formation and Metastasis by Upregulation of Lipid Desaturation and Suppression of Endoplasmic Reticulum Stress. J Exp Clin Cancer Res (2019) 38:116. doi: 10.1186/s13046-019-1127-x
55. Lu M, Henry CE, Lai H, Khine YY, Ford CE, Stenzel MH. A New 3D Organotypic Model of Ovarian Cancer to Help Evaluate the Antimetastatic Activity of RAPTA-C Conjugated Micelles. Biomater Sci (2019) 7:1652–60. doi: 10.1039/c8bm01326h
56. Michy T, Massias T, Bernard C, Vanwonterghem L, Henry M, Guidetti M, et al. Verteporfin-Loaded Lipid Nanoparticles Improve Ovarian Cancer Photodynamic Therapy In Vitro and In Vivo. Cancers (Basel) (2019) 11:1760. doi: 10.3390/cancers11111760
57. Dumont S, Jan Z, Heremans R, Van Gorp T, Vergote I, Timmerman D. Organoids of Epithelial Ovarian Cancer as an Emerging Preclinical In Vitro Tool: A Review. J Ovarian Res (2019) 12:105. doi: 10.1186/s13048-019-0577-2
58. Watters KM, Bajwa P, Kenny HA. Organotypic 3d Models of the Ovarian Cancer Tumor Microenvironment. Cancers (Basel) (2018) 10:265. doi: 10.3390/cancers10080265
59. Long L, Yin M, Min W. 3d Co-Culture System of Tumor-Associated Macrophages and Ovarian Cancer Cells. Bio Protoc (2018) 8:e2815. doi: 10.21769/BioProtoc.2815
60. Raghavan S, Mehta P, Xie Y, Lei YL, Mehta G. Ovarian Cancer Stem Cells and Macrophages Reciprocally Interact Through the WNT Pathway to Promote Pro-Tumoral and Malignant Phenotypes in 3D Engineered Microenvironments. J Immunother Cancer (2019) 7:190. doi: 10.1186/s40425-019-0666-1
61. Ward Rashidi MR, Mehta P, Bregenzer M, Raghavan S, Fleck EM, Horst EN, et al. Engineered 3d Model of Cancer Stem Cell Enrichment and Chemoresistance. Neoplasia. (2019) 21:822–36. doi: 10.1016/j.neo.2019.06.005
62. Mitra S, Tiwari K, Podicheti R, Pandhiri T, Rusch DB, Bonetto A, et al. Transcriptome Profiling Reveals Matrisome Alteration as a Key Feature of Ovarian Cancer Progression. Cancers (Basel) (2019) 11:1513. doi: 10.3390/cancers11101513
63. Paullin T, Powell C, Menzie C, Hill R, Cheng F, Martyniuk CJ, et al. Spheroid Growth in Ovarian Cancer Alters Transcriptome Responses for Stress Pathways and Epigenetic Responses. PloS One (2017) 12:e0182930. doi: 10.1371/journal.pone.0182930
64. Yoshimura A, Sawada K, Nakamura K, Kinose Y, Nakatsuka E, Kobayashi M, et al. Exosomal miR-99a-5p Is Elevated in Sera of Ovarian Cancer Patients and Promotes Cancer Cell Invasion by Increasing Fibronectin and Vitronectin Expression in Neighboring Peritoneal Mesothelial Cells. BMC Cancer (2018) 18:1065. doi: 10.1186/s12885-018-4974-57
65. Mahdy H, Casey MJ, Crotzer D. Endometrial Cancer. In: StatPearls. Treasure Island (FL: StatPearls (2021).
66. Bokhman JV. Two Pathogenetic Types of Endometrial Carcinoma. Gynecol Oncol (1983) 15:10–7. doi: 10.1016/0090-8258(83)90111-7
67. Murali R, Soslow RA, Weigelt B. Classification of Endometrial Carcinoma: More Than Two Types. Lancet Oncol (2014) 15:e268–78. doi: 10.1016/S1470-2045(13)70591-6
68. Van Nyen T, Moiola CP, Colas E, Annibali D, Amant F. Modeling Endometrial Cancer: Past, Present, and Future. Int J Mol Sci (2018) 19:2348. doi: 10.3390/ijms19082348
69. Grun B, Benjamin E, Sinclair J, Timms JF, Jacobs IJ, Gayther SA, et al. Three-Dimensional In Vitro Cell Biology Models of Ovarian and Endometrial Cancer. Cell Prolif (2009) 42(2):219–28. doi: 10.1111/j.1365-2184.2008.00579.x
70. Chitcholtan K, Asselin E, Parent S, Sykes PH, Evans JJ. Differences in Growth Properties of Endometrial Cancer in Three Dimensional (3D) Culture and 2D Cell Monolayer. Exp Cell Res (2013) 319:75–87. doi: 10.1016/j.yexcr.2012.09.012
71. Chitcholtan K, Sykes PH, Evans JJ. The Resistance of Intracellular Mediators to Doxorubicin and Cisplatin Are Distinct in 3D and 2D Endometrial Cancer. J Transl Med (2012) 10:38. doi: 10.1186/1479-5876-10-38
72. Rodriguez AC, Vahrenkamp JM, Berrett KC, Clark KA, Guillen KP, Scherer SD, et al. ETV4 Is Necessary for Estrogen Signaling and Growth in Endometrial Cancer Cells. Cancer Res (2020) 80:1234–45. doi: 10.1158/0008-5472.CAN-19-1382
73. Al-Juboori AAA, Ghosh A, Jamaluddin MFB, Kumar M, Sahoo SS, Syed SM, et al. Proteomic Analysis of Stromal and Epithelial Cell Communications in Human Endometrial Cancer Using a Unique 3d Co-Culture Model. Proteomics. (2019) 19:e1800448. doi: 10.1002/pmic.201800448
74. Tanaka R, Saito T, Ashihara K, Nishimura M, Mizumoto H, Kudo R. Three-Dimensional Coculture of Endometrial Cancer Cells and Fibroblasts in Human Placenta Derived Collagen Sponges and Expression Matrix Metalloproteinases in These Cells. Gynecol Oncol (2003) 90:297–304. doi: 10.1016/s0090-8258(03)00335-4
75. Collins A, Miles GJ, Wood J, MacFarlane M, Pritchard C, Moss E. Patient-Derived Explants, Xenografts and Organoids: 3-Dimensional Patient-Relevant Pre-Clinical Models in Endometrial Cancer. Gynecol Oncol (2020) 156:251–9. doi: 10.1016/j.ygyno.2019.11.020
76. Maru Y, Tanaka N, Itami M, Hippo Y. Efficient Use of Patient-Derived Organoids as a Preclinical Model for Gynecologic Tumors. Gynecol Oncol (2019) 154:189–98. doi: 10.1016/j.ygyno.2019.05.005
77. Siegel RL, Miller KD, Fuchs HE, Jemal A. Cancer Statistics, 2021. CA Cancer J Clin (2021) 71(1):7–33. doi: 10.3322/caac.21654
78. Weeber F, Ooft SN, Dijkstra KK, Voest EE. Tumor Organoids as a Pre-Clinical Cancer Model for Drug Discovery. Cell Chem Biol (2017) 24:1092–100. doi: 10.1016/j.chembiol.2017.06.012
79. Miller DH, Sokol ES, Gupta PB. 3d Primary Culture Model to Study Human Mammary Development. Methods Mol Biol (2017) 612:139–47. doi: 10.1007/978-1-4939-7021-6_10
80. dit Faute MA, Laurent L, Ploton D, Poupon MF, Jardillier JC, Bobichon H. Distinctive Alterations of Invasiveness, Drug Resistance and Cell-Cell Organization in 3D-Cultures of MCF-7, a Human Breast Cancer Cell Line, and its Multidrug Resistant Variant. Clin Exp Metastasis (2002) 19:161–8. doi: 10.1023/a:10145948255027
81. Gomes LR, Rocha CRR, Martins DJ, Fiore A, Kinker GS, Bruni-Cardoso A, et al. ATR Mediates Cisplatin Resistance in 3D-Cultured Breast Cancer Cells via Translesion DNA Synthesis Modulation. Cell Death Dis (2019) 10:459. doi: 10.1038/s41419-019-1689-8
82. Lovitt CJ, Shelper TB, Avery VM. Doxorubicin Resistance in Breast Cancer Cells Is Mediated by Extracellular Matrix Proteins. BMC Cancer (2018) 18:41. doi: 10.1186/s12885-017-3953-6
83. Urciuolo F, Garziano A, Imparato G, Panzetta V, Fusco S, Casale C, et al. Biophysical Properties of Dermal Building-Blocks Affects Extra Cellular Matrix Assembly in 3D Endogenous Macrotissue. Biofabrication. (2016) 8:15010. doi: 10.1088/1758-5090/8/1/015010
84. Brancato V, Gioiella F, Imparato G, Guarnieri D, Urciuolo F, Netti PA. 3D Breast Cancer Microtissue Reveals the Role of Tumor Microenvironment on the Transport and Efficacy of Free-Doxorubicin In Vitro. Acta Biomater (2018) 75:200–12. doi: 10.1016/j.actbio.2018.05.055
85. Figueroa-Magalhães MC, Jelovac D, Connolly R, Wolff AC. Treatment of HER2-Positive Breast Cancer. Breast. (2014) 23:128–36. doi: 10.1016/j.breast.2013.11.011
86. Genuino AJ, Chaikledkaew U, The DO, Reungwetwattana T, Thakkinstian A. Adjuvant Trastuzumab Regimen for HER2-Positive Early-Stage Breast Cancer: A Systematic Review and Meta-Analysis. Expert Rev Clin Pharmacol (2019) 12:815–24. doi: 10.1080/17512433.2019.1637252
87. Yakes FM, Chinratanalab W, Ritter CA, King W, Seelig S, Arteaga CL. Herceptin-Induced Inhibition of Phosphatidylinositol-3 Kinase and Akt Is Required for Antibody-Mediated Effects on P27, Cyclin D1, and Antitumor Action. Cancer Res (2002) 62:4132–41.
88. Tatara T, Mukohara T, Tanaka R, Shimono Y, Funakoshi Y, Imamura Y, et al. 3d Culture Represents Apoptosis Induced by Trastuzumab Better Than 2D Monolayer Culture. Anticancer Res (2018) 38:2831–9. doi: 10.21873/anticanres.12528
89. Gangadhara S, Smith C, Barrett-Lee P, Hiscox S. 3D Culture of Her2+ Breast Cancer Cells Promotes AKT to MAPK Switching and a Loss of Therapeutic Response. BMC Cancer (2016) 16:345. doi: 10.1186/s12885-016-2377-z
90. Rodríguez CE, Berardi DE, Abrigo M, Todaro LB, Bal de Kier Joffé ED, Fiszman GL. Breast Cancer Stem Cells are Involved in Trastuzumab Resistance Through the HER2 Modulation in 3D Culture. J Cell Biochem (2018) 119:1381–91. doi: 10.1002/jcb.26298
91. Alqahtani FY, Aleanizy FS, El Tahir E, Alkahtani HM, AlQuadeib BT. Paclitaxel. Profiles Drug Subst Excip Relat Methodol (2019) 44:205–38. doi: 10.1016/bs.podrm.2018.11.001
92. Tietze R, Zaloga J, Unterweger H, Lyer S, Friedrich RP, Janko C, et al. Magnetic Nanoparticle-Based Drug Delivery for Cancer Therapy. Biochem Biophys Res Commun (2015) 468:463–70. doi: 10.1016/j.bbrc.2015.08.022
93. Lugert S, Unterweger H, Mühlberger M, Janko C, Draack S, Ludwig F, et al. Cellular Effects of Paclitaxel-Loaded Iron Oxide Nanoparticles on Breast Cancer Using Different 2D and 3D Cell Culture Models. Int J Nanomed (2018) 14:161–80. doi: 10.2147/IJN.S187886
94. Haniadka R, Rajeev AG, Palatty PL, Arora R, Baliga MS. Zingiber Officinale (Ginger) as an Anti-Emetic in Cancer Chemotherapy: A Review. J Altern Complement Med (2012) 18:440–4. doi: 10.1089/acm.2010.0737
95. Almada da Silva J, Becceneri AB, Sanches Mutti H, Moreno Martin AC, Fernandes da Silva MF, Fernandes JB, et al. Purification and Differential Biological Effects of Ginger-Derived Substances on Normal and Tumor Cell Lines. J Chromatogr B Analyt Technol BioMed Life Sci (2012) 903:157–62. doi: 10.1016/j.jchromb.2012.07.013
96. Fuzer AM, Lee SY, Mott JD, Cominetti MR. [10]-Gingerol Reverts Malignant Phenotype of Breast Cancer Cells in 3D Culture. J Cell Biochem (2017) 118:2693–9. doi: 10.1002/jcb.25906
97. Weaver VM, Lelièvre S, Lakins JN, Chrenek MA, Jones JC, Giancotti F, et al. Beta4 Integrin-Dependent Formation of Polarized Three-Dimensional Architecture Confers Resistance to Apoptosis in Normal and Malignant Mammary Epithelium. Cancer Cell (2002) 2:205–16. doi: 10.1016/s1535-6108(02)00125-3
98. Roberts S, Peyman S, Speirs V. Current and Emerging 3d Models to Study Breast Cancer. Adv Exp Med Biol (2019) 1152:413–27. doi: 10.1007/978-3-030-20301-6_22
99. Maman S, Witz IP. A History of Exploring Cancer in Context. Nat Rev Cancer (2018) 18:359–76. doi: 10.1038/s41568-018-0006-7
100. Karnoub AE, Dash AB, Vo AP, Sullivan A, Brooks MW, Bell GW, et al. Mesenchymal Stem Cells Within Tumour Stroma Promote Breast Cancer Metastasis. Nature. (2007) 449:557–63. doi: 10.1038/nature06188
101. Rijal G, Li W. 3D Scaffolds in Breast Cancer Research. Biomaterials. (2016) 81:135–56. doi: 10.1016/j.biomaterials.2015.12.016
102. Blache U, Horton ER, Xia T, Schoof EM, Blicher LH, Schönenberger A, et al. Mesenchymal Stromal Cell Activation by Breast Cancer Secretomes in Bioengineered 3D Microenvironments. Life Sci Alliance (2019) 2:e201900304. doi: 10.26508/lsa.201900304
103. Balachander GM, Rajashekar B M, Sarashetti P, Rangarajan A, Chatterjee K. MiRNomics Reveals Breast Cancer Cells Cultured on 3D Scaffolds Better Mimic Tumors in Vivo Than Conventional 2d Culture. ACS Biomater Sci Eng (2018) 4:116–27. doi: 10.1021/acsbiomaterials.7b00694
104. Kim TH, Woo JS, Kim YK, Kim KH. Silibinin Induces Cell Death Through Reactive Oxygen Species-Dependent Downregulation of Notch-1/ERK/Akt Signaling in Human Breast Cancer Cells. J Pharmacol Exp Ther (2014) 349:268–78. doi: 10.1124/jpet.113.207563
105. Yazdi Rouholamini SE, Moghassemi S, Maharat Z, Hakamivala A, Kashanian S, Omidfar K. Effect of Silibinin-Loaded Nano-Niosomal Coated With Trimethyl Chitosan on miRNAs Expression in 2D and 3D Models of T47D Breast Cancer Cell Line. Artif Cells Nanomed Biotechnol (2018) 46(3):524–35. doi: 10.1080/21691401.2017.1326928
106. Kopper O, de Witte CJ, Lõhmussaar K, Valle-Inclan JE, Hami N, Kester L, et al. An Organoid Platform for Ovarian Cancer Captures Intra- and Interpatient Heterogeneity. Nat Med (2019) 25:838–49. doi: 10.1038/s41591-019-0422-6
107. Boretto M, Maenhoudt N, Luo X, Hennes A, Boeckx B, Bui B, et al. Patient-Derived Organoids From Endometrial Disease Capture Clinical Heterogeneity and are Amenable to Drug Screening. Nat Cell Biol (2019) 21:1041–51. doi: 10.1038/s41556-019-0360-z
108. Girda E, Huang EC, Leiserowitz GS, Smith LH. The Use of Endometrial Cancer Patient-Derived Organoid Culture for Drug Sensitivity Testing Is Feasible. Int J Gynecol Cancer (2017) 27:1701–7. doi: 10.1097/IGC.0000000000001061
109. Nakajima A, Endo H, Okuyama H, Kiyohara Y, Kimura T, Kamiura S, et al. Radiation Sensitivity Assay With a Panel of Patient-Derived Spheroids of Small Cell Carcinoma of the Cervix. Int J Cancer (2015) 136:2949–60. doi: 10.1002/ijc.29349
110. Jardé T, Lloyd-Lewis B, Thomas M, Kendrick H, Melchor L, Bougaret L, et al. Wnt and Neuregulin1/ErbB Signalling Extends 3D Culture of Hormone Responsive Mammary Organoids. Nat Commun (2016) 7:13207. doi: 10.1038/ncomms13207
111. García-Davis S, Viveros-Valdez E, Díaz-Marrero AR, Fernández JJ, Valencia-Mercado D, Esquivel-Hernández O, et al. Antitumoral Effect of Laurinterol on 3D Culture of Breast Cancer Explants. Mar Drugs (2019) 7:201. doi: 10.3390/md17040201
112. Carter ME, Hartkopf AD, Wagner A, Brucker S Y, Berchtold S, Lauer UM, et al. A Three-Dimensional Organoid Model of Primary Breast Cancer to Investigate the Effects of Oncolytic Virotherapy. Front Mol Biosci (2022) 9:826302. doi: 10.3389/fmolb.2022.826302
113. Huch M, Knoblich JA, Lutolf MP, Martinez-Arias A. The Hope and the Hype of Organoid Research. Development. (2017) 144:938–41. doi: 10.1242/dev.150201
114. Weigelt B, Ghajar CM, Bissell MJ. The Need for Complex 3D Culture Models to Unravel Novel Pathways and Identify Accurate Biomarkers in Breast Cancer. Adv Drug Deliv Rev (2014) 69-70:42–51. doi: 10.1016/j.addr.2014.01.001
Keywords: 3D cultures, breast cancer, gynecological cancers, microRNAs, therapy response
Citation: Salinas-Vera YM, Valdés J, Pérez-Navarro Y, Mandujano-Lazaro G, Marchat LA, Ramos-Payán R, Nuñez-Olvera SI, Pérez-Plascencia C and López-Camarillo C (2022) Three-Dimensional 3D Culture Models in Gynecological and Breast Cancer Research. Front. Oncol. 12:826113. doi: 10.3389/fonc.2022.826113
Received: 30 November 2021; Accepted: 20 April 2022;
Published: 26 May 2022.
Edited by:
Elzbieta Pluciennik, Medical University of Lodz, PolandReviewed by:
Allen Thayakumar Basanthakumar, Dana–Farber Cancer Institute, United StatesGiorgia Imparato, Center for Advanced Biomaterials for Healthcare (IIT), Italy
Copyright © 2022 Salinas-Vera, Valdés, Pérez-Navarro, Mandujano-Lazaro, Marchat, Ramos-Payán, Nuñez-Olvera, Pérez-Plascencia and López-Camarillo. This is an open-access article distributed under the terms of the Creative Commons Attribution License (CC BY). The use, distribution or reproduction in other forums is permitted, provided the original author(s) and the copyright owner(s) are credited and that the original publication in this journal is cited, in accordance with accepted academic practice. No use, distribution or reproduction is permitted which does not comply with these terms.
*Correspondence: César López-Camarillo, Y2VzYXIubG9wZXpAdWFjbS5lZHUubXg=; orcid.org/0000-0002-9417-2609