- 1Department of Applied Cell Sciences, School of Advanced Technologies in Medicine, Tehran University of Medical Sciences, Tehran, Iran
- 2Basic and Molecular Epidemiology of Gastrointestinal Disorders Research Center, Research Institute for Gastroenterology and Liver Diseases, Shahid Beheshti University of Medical Sciences, Tehran, Iran
- 3Northern Ireland Blood Transfusion Service, Belfast, United Kingdom
- 4Department of Internal Medicine V, Medical University of Innsbruck, Innsbruck, Austria
- 5Department of Industrial Engineering, University of Trento, Trento, Italy
Natural killer cells are members of the innate immune system and promote cytotoxic activity against tumor or infected cells independently from MHC recognition. NK cells are modulated by the expression of activator/inhibitory receptors. The ratio of this activator/inhibitory receptors is responsible for the cytotoxic activity of NK cells toward the target cells. Owing to the potent anti-tumor properties of NK cells, they are considered as interesting approach in tumor treatment. Colorectal cancer (CRC) is the second most common cause of death in the world and the incidence is about 2 million new cases per year. Metastatic CRC is accompanied by a poor prognosis with less than three years of overall survival. Chemotherapy and surgery are the most adopted treatments. Besides, targeted therapy and immune checkpoint blockade are novel approach to CRC treatment. In these patients, circulating NK cells are a prognostic marker. The main target of CRC immune cell therapy is to improve the tumor cell’s recognition and elimination by immune cells. Adaptive NK cell therapy is the milestone to achieve the purpose. Allogeneic NK cell therapy has been widely investigated within clinical trials. In this review, we focus on the NK related approaches including CAR NK cells, cell-based vaccines, monoclonal antibodies and immunomodulatory drugs against CRC tumoral cells.
Introduction
Natural killer cells (NK cells) as granular cells, consist of nearly 5-15% of peripheral blood lymphocytes. These cells are classified as innate immunity cells since they can create a defensive barrier without previous exposure to a pathogen, cancer cells, or recognition by Major Histocompatibility Complex (MHC) (1).
Human NK cells are divided into two subgroups based on the expression of CD56: CD56 bright and CD56dim cells. These are functionally and phenotypically different. CD56bright NK cells are mostly found in secondary lymphoid tissues, while cytotoxic CD56dim NK cells are found in circulation (2). Although NK cells belong to the innate immune system, they also have some adaptive immune features.
Previous studies of conducted on CMV infection and on response to activatory cytokines identified two distinct populations of memory NK cells as antigen-dependent and –independent (3, 4).. The strategy to induce memory-like NK cell differentiation is a novel approach for cancer immuno therapy (5). The anti-tumor efficacy of NK cells is dependent to ratio of activating/inhibiting receptors present on their surface. Activating receptors of NK cells are NKG2D, DNM-1, Natural Cytotoxicity Receptors (NCRs) and type 2 receptor (KIR) family. NK activation releases of inflammatory cytokines as well as granules with lytic properties which cause the lysis of tumor cells (6).
One of the problem of immune cell therapy is the fact that NK cells within tumor micro environment (TME) are scanty and always suppressed (7). Tumor cells deceive NK cells in several ways: a) by increasing human leukocyte antigen E (HLA-E) (8) and HLA-G (9); b) via inhibitory immune checkpoints (10); c) decreasing cytokine expression (11); d) decreasing NK cell apoptotic activity (12); e) reducing expression of activating receptors on NK cells (13); f) increasing the expression of prostaglandin E2 by tumor-associated fibroblasts (14).
The main target of CRC immune cell therapy is to improve the tumor cell’s recognition and elimination by immune cells. Adaptive NK cell therapy is the milestone to achieve the purpose. We will review the genetically manipulated NK cells and novel immunotherapy approaches including immunomodulatory drugs, monoclonal antibodies and cancer vaccines that may enhance cytotoxicity of NK cells towards CRC.
Regulation of NK activity
Contribution of Receptor- Ligand
Activator and inhibitory receptors on NK cells detect the protein ligands on the infected and tumor cells. The ratio of these receptors will determine the activation or inhibition of NK cell cytotoxic cascades. Most healthy human nucleated cells present MHC class I loaded with self-peptides that are inhibitory ligands and will switch off NK cells. Thus, the suppression/expression of MHC molecules on the surface of most tumor cells play a key role in NK activation (Figure 1). KIRs are the key MHC receptors and regulate the development, activation, and cytotoxic features of NK cells. There are various isoforms of KIRs that, in different physiologic and pathologic conditions may play the role of inhibitory or activator receptors. For example, it is reported that the KIR2DL1 isoform is specific against HLA-C2, consisting of an Ig-like inhibitory motif (15). However, KIR 2DS5, 2DS1, and 3DS1 containing Ig-like activatory motifs are associated with an increased complete remission post-chemotherapy in metastatic CRC (16). HLA-E is a non-classical member of the MHC class-I family that presents self-peptide on almost all nucleated cells and in normal conditions, will dampen NK cell activation by interaction with NKG2A inhibitory receptor. However, in the tumor context of CRC, over-expressed HLA-E is an immune escape mechanism that inhibits NK cell activation through high-affinity interaction with the NKG2A receptor (17, 18). Activator receptors on NK cell surface play an essential role in tumor cell recognition and elimination. NKG2D is a critical activating receptor of NK cells and its expression will be upregulated during NK cell maturation and activation (19). NKG2D ligands are MHC class-I chain-related A and B molecules, also known as MICA/MICB and UL binding protein 1-6 (ULBP1-6). NKG2DLs are not expressed under normal condition on healthy tissues, and their expression is intracellular mainly (20). However, their expression on cell surface has been reported in various carcinomas due to cellular stress (21), including CRC. The same are also reported as a good prognostic biomarker (22, 23). Besides surface expression of NKG2D ligands on tumor cells, there are shedding form of MICA and MICB derived from transformed cells in TME (20). Continuous exposure of NKG2D with soluble forms of MICA and MICB results in reduced NK cells cytotoxicity, downregulating NKG2D expression. Altogether, these induce tumor cells proliferation (24). Soluble forms of NKG2DLs is due to protease cleavage of their conserved motif in the α3 domain. Two dis-integrin and metalloproteinase enzymes known as A Dis-integrin and Metalloproteinase domain-containing protein 10 and 17 (ADAM10 and 17) are crucial in making soluble forms of MICA/B, and ULBP (25). It is reported that platelets are one of the secretory origins of ADAM10 and 17. In metastatic lung cancer, these soluble platelet-derived factors impair the NK immune surveillance towards tumor cells (26).
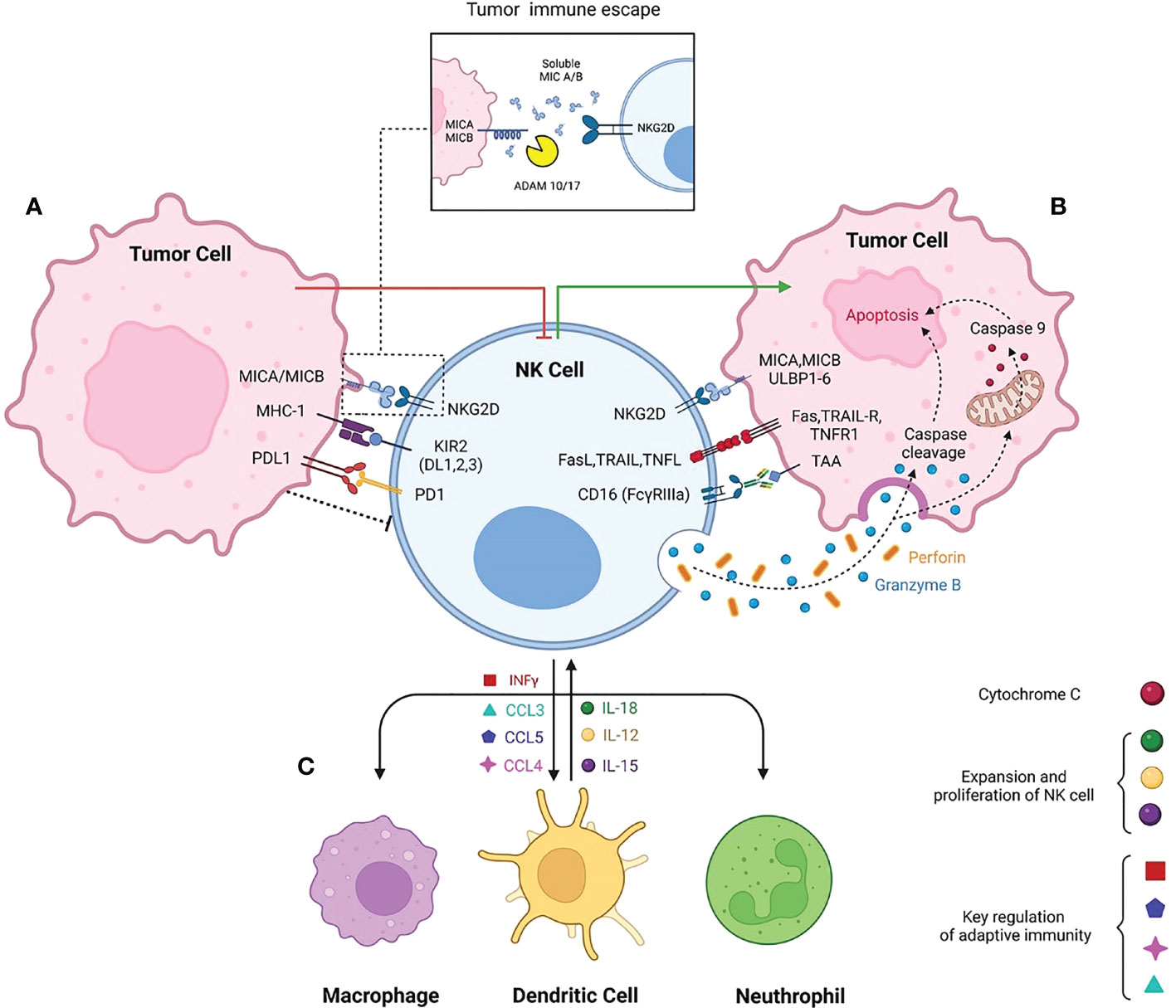
Figure 1 schematic diagram of Natural killer cell contribution to innate immunity. The balance of NK cells activatory and inhibitory receptors promotes either secreting cytotoxic molecules or making NK cells exhausted in TME respectively. (A) Immune suppressive TME is due to interaction of MHC-1 with inhibitory receptors such as KIR2. High expression of PDL-1 in tumor cells interacts with inhibitory checkpoint PD-1, inhibiting the NK cell activation. Beside the activatory role of MICA/MICB, the shedding form caused by ADAM17/10 cleavage makes the NK cells non responsive to tumor target cells. (B) NKG2D the most prominent activatory receptor of NK cells interact with MICA/MICB/ULBP. Following NK cells activation Fas ligand, TRAIL and TNF ligand highly express on NK cells to induce the apoptosis independent of perforin and granzyme. Antibody dependent cytotoxicity of NK cells promotes perforin and granzyme activation and consequently granzymes triggers apoptosis even dependent or independent of caspase cleavage. (C) Interaction of NK with other immune cells. NK cells secret INFγ, CCL3, CCL5, CCL4 to contribute with macrophages, dendritic cells and neutrophils. The innate immune cells subsequently secret IL-15, IL-18 and IL-12 to provoke NK cells expansion and activation.
The other family of no MHC restricted NK receptors are Natural cytotoxicity receptors (NCRs), including NKp46, NKp30, NKp44, and NKp80. NCRs ligands are expressed on viral infected or tumor cells and extracellular soluble forms (27). The density of NCRs on NK cells’ surfaces is coordinates with NK cells activation and cytotoxic function (28).
Receptor-ligand modulation may impair NK cells functionality in tumor elimination. Novel therapeutic strategies would selectively target this approach of NK cells in tumor context. The interactions between NK Cell Receptor and human target cell ligands are summarized in Table 1.
Cytokines induce NK cells’ cytotoxicity
Besides receptor-ligands interactions, cytokine secretion and exposure are demanded for NK cell activation (54). Several cytokines have been reported to modulate NK cell activation and proliferation. Cytokine effectiveness in NK cells has been reported mainly through JAK/STAT, CIS, and SOCS signaling pathways (55–57). The crucial cytokines activating NK cells are: IL-2, IL-12, IL-15, and IL-18. These molecules trigger their effects thanks to signal transducer and activator of transcription (STAT) proteins (58). The suppressor cytokine signaling 2 (SOCS2) inhibits JAK/STAT signaling and with negative feedback, regulates NK cell differentiation. NK cells’ cytokine signaling modulate homeostasis with IL-2, IL12, IL-15 and IL 18 and limit INFα signaling through STAT1 (59). Immature NK cells develop to mature NK cells through the acquisition of activator receptors such as NKG2D. IL-2/IL-15/IL-18 increases the cytotoxic activity of NK cells by NKG2D over expression (60). IL-12 and IL-2 enhance the NKG2D signal activation. However, IL-2 exclusively effects the activation of CD56 bright NK cells (61).
Moreover, IL-2 and IL-15 induce NKG2D, KIRDL1, and KIRDL2 expression on NK cells, T cells, and NKT cells of regional lymph nodes. This enhances NK cell cytotoxicity and increases the anti-tumor potential of innate and adaptive immune cells (62).
A cocktail-based strategy by utilization of IL-2, IL-15, and IL-18 cytokines was established to generate in vitro peripheral blood-derived NK cells with a strong cytotoxic effect (63). Systemic administration of IL-2 in cancer patients improved survival rate, however high doses was reported to be toxic (64). Intratumoral administration of IL-2 is an alternative method to systemic therapy, but this is limited to accessible tumors (65). Targeted delivery of IL-2 is a novel approach to enhance the cytotoxicity effects of tumor resident NK cells. Highly preserved glycoproteins such as fibronectin expressed in solid tumors neo-endothelial could be a target of cancer therapy. L19-IL-2 is an immune-cytokine that targets extra domain of fibronectin fused with IL-2. The IL-2 accumulates selectively in the tumor site, boosting the tumor immune response (66).
IL-15 is an immuno-stimulatory cytokine that promotes the survival and proliferation of NK cells. IL-15 receptor is a heterotrimeric complex consisting of IL-15R α, IL-15R β and common γ chain (67). Antigen-presenting cells secret IL-15/IL15Rα in two forms soluble and membrane-bound. IL-15/IL-15Rα subsequently transduces signaling through four independent mechanisms known as IL-15 trans presentation delivery (68, 69). Recombinant IL-15 had been reported to evoke anti-tumor activity of adaptive and innate immune cells in colon cancer (70) however, Short half-life and poor bioavailability limit the therapeutic potential of IL-15 systemic therapy.
It is reported that chimeric IL-15 (covalently linked IL-15 with IgG2) improves IL-15 half-life, enabling greater bioavailability for an extended period of time (71). As the delivery of IL-15 is reported through trans presentation, a novel approach investigated an immune cytokine known as KD033 that both targets PDL-1 and directly delivers IL-15/IL-15Rα complex to immune cells resident in TME. 7 days after a single dose of KD033, a dose-dependent increase in peripheral NK cells and NKT-like cells were induced (72). Furthermore, Rui Ma; et al. developed a new oncolytic herpes virus expressing IL-15/IL-15Rα and demonstrated in a murine glioblastoma model therapeutic efficacy (73).
Although there is the beneficial roles of IL-15 in immune cell stimulating, the secretion is regulated through the cytokine induced SH2-containing protein (Cish) that provides negative feedback by preventing the JAK/STAT5 signaling (57). Following CIS deletion in NK cells, hyperactive IL-15 signaling leads to increased activation of the AKT/mTOR and c-Myc signaling, inducing a high proliferation rate of NK cells (74). IL-21 and 18 are stimulate NK cell proliferation and activation. The signaling through a typical γ chain subsequently induces INFγ production in mature NK cells (75). Targeted cytokine delivery, in contrast to systemic therapy, will improve the beneficial effects of treatment with minimum side effects. A fusion of anti-PD-1 antibody and IL-21 inhibits the PD-1 immune checkpoint signaling and delivers IL-21 to targeted receptors, improving treatment efficacy (76). IL-18 receptor is highly expressed in NK cells. However, most tumors express IL-18 decoy receptor in TME, limiting the anti-tumor immunity of therapeutic recombinant IL-18. Ting Zhou; et al. have engineered a decoy resistance IL-18 receptor (DR-18); that maintains the IL-18 signaling and the compound is resistant to IL-18-decoy receptor inhibition protein. These results demonstrated that DR-18 enhanced the maturation and activation of NK cells in anti-PD-1 resistance tumors (77).
Besides the investigation of cytokines effects one by one, the compound of cytokines profile plays an essential role in the generation of different NK cell subtypes. Cooper et al. first studied cytokine-induced memory-like NK cells in 2009. They reported that IL-15/IL-18/IL-12 pre-activated NK cells showed higher INFγ production by cytokine profile re-stimulation, in contrast to pre-activated group (4). The memory-like NK cells are phenotypically different from classical cytotoxic NK cells (78). Low dose of IL-2 activates the cytokine-induced memory-like NK cells more prominently (79). Significantly metabolic features of cytokine-induced memory-like NK cells will be reprogramed during pre-activation. mTOR controls metabolic reprogramming and cytotoxicity function of NK cells. This gene will be activated during cytokine exposure (80). Due to their high cytotoxic activity and anti-tumor properties, cytokine-induced NK cells represent the novel immune cell therapy against tumors. However, their therapeutic potential may be further improved studying how they change after cytokine exposure, combination immunotherapy approaches, genetically modifications (81). Generally, the anti-tumor activity of NK cells is based on the responsiveness to growth factors and cytokines that can extremely influence the tumor immune surveillance. The specific contribution of NK cells and cytokines still needs to be better identified.
Cytoskeleton as a regulator of NK cells’ immune responses
NK cells degranulation is a multi-step process. This consists of: a) immunologic synapse formation by ligand-receptor molecules of NK cells and tumor cells; b) polarization of lytic granules toward the immunologic synapse, and directly release of cytotoxic granules toward target cells (82).
Cytolytic granules with acidic pH are kind of lysosomes that contains pore-forming molecule, granzyme, Fas Ligand, and granulysin in NK cells. Perforin is a pore-forming molecule, while granzymes are a serine protease family that induces programmed cell death (apoptosis) in tumor cells (83). Perforin mediates target cell membrane pore forming in a pH and calcium dependent manner, consequently delivering the serine protease granzyme toward the target cell (84).
NK cells are one of primary cells that induce the extrinsic apoptosis pathway by expressing tumor necrosis factor super family members such as Fas ligand (FasL), and TRAIL. Interaction of death receptor ligands will recruit adaptor proteins such as FADD (Fas-associated protein with death domain), TRADD (Tumor necrosis factor receptor type 1-associated death domain protein), and a series of downstream factors, including caspase-3, -6, -7, -8, -9 eventually leading to apoptosis (85). Independent caspase apoptosis mediated by granzymes is reported by provoking mitochondria’s permeabilization by with cytochrome C release in the cytosol and apoptosome complex formation (86).
The cytoskeleton can regulate NK cells’ immune response. NK cell migration and immune synapse formation are due to polymerization and depolymerization of actin proteins. F-actin filaments will polymerize in response to receptor-ligand interaction and trigger the downstream signaling pathways, including PLCγ, MEK, and ERK (87). Besides NK cells receptor-ligand interactions, monoclonal antibodies have been reported to induce NK lytic granule polarization by activating PLCγ, MEK, and ERK signaling pathways (88). Cytotoxic granule release is highly dependent from F-actin generation due to the mediator of actin regulatory protein, EVL (89). Recently, filamin A (FLNa) protein cross links F-actin filaments was reported playing an essential role in the degranulation of NK cells following synaptic accumulation of F-actins (90). In addition to actin proteins, myosin IIA is the next cytoskeleton protein that promotes NK cells functionality by utilizing ATP to induce the contractile force on F-actin filaments (91). Moreover, microtubule filaments consist of alpha and beta tubulin heterodimers, and utilize microtubule-associated proteins (MAPS) to facilitate the delivery of NK cells’ lytic granules in the immunologic synapse (92).
Cytoskeleton machinery abnormalities impair NK cell migration and cytotoxic activity. The relationships between NK cells and cytoskeleton should therefore be deeper investigated (93).
Colorectal cancer and impaired NK cell function
The mechanisms sustaining inflammation in CRC are not fully understood. In colitis-related colon cancers, chronic inflammation plays an influential role in the progression of the disease to malignancy (94). Innate immune system cells, including neutrophils, macrophages, NK cells, and B- and T-cells of acquired immunity, are involved in post-inflammatory cancer processes (95). According to studies conducted on CRCs, inflammation and the presence of NK cells at the tumor site play an essential role for the progression of the disease (96). In CRC microenvironment NK cells and TCD8+ cells interact through secretion of INFγ, IL-2, and HMGB1 (high-mobility group protein 1). Cross-talk between NK, TCD8+, and M1 cells are involved in the induction of a pro-inflammatory immune response that leads to the activity of TCD8+ cells in CRC microenvironment. IL-2 activated NK cells, secret INFγ, and Leptin. Leptin directly stimulates M1 cells to produce IL-1β. Subsequently, T cells synthesize the pro-inflammatory cytokine IL-6. INFγ indirectly induces IL-6 production from the macrophage (97).
Activated NK cells produce CXCL1, CCL1-3-4-5, CCL-22, and CXCL-8, chemo-attractants for other immune cells, and recruit them to the tumor site. A preclinical study in a colorectal mouse model reported that the secretions of CCL-3 from CT26 tumor cells change the inflammatory response in the early phase of tumor response. It causes mobilization of B, T-cells, dendritic cells, and CD49+ NK cells to the tumor site (98). There are differences in tumor-infiltrating immune cells among CRC on the left and right sides. Left-side tumors (LCC) are associated with a high number of CD56 bright NK cells that correlates with patient survival (99). Left-side tumors show a higher rate of response to therapy (100). It is demonstrated that CD56dim LCC infiltrated NK cells could be a prognostic biomarker in CRC (101). Mc Gilvray et al. showed NKG2D ligand expression involves in cancer immunosurveillance and associated with Prognosis (102). Furthermore, NK cells express programmed cell death protein 1 (PD-1) substantially, and is further increased after stimulation and indicates poor prognosis in digestive cancer patients (103).
Various studies have been conducted on the infiltration rate of NK cells which showed that NK cells infiltrating solid tumors were relatively low and demonstrated that the NK cell number was too low to pursue prognosis (104–107). Moreover, Sarah Nersesian et al. investigated the prognostic value of NK cells for solid tumors. They reported 1.9% found a negative association between overall survival and NK cell infiltration and 38.9% reported no impact and 59.3% positive associations between NK cell infiltration and overall survival (108). These reports demonstrate NK cells as a positive prognostic factor in solid tumors.
There are 2 types of activated macrophages: a) activated M1, which participates in immune response, and b) activated M2, which promotes tumor progression. Tumors-associated macrophages (TAMs), more similar to the M2 subtype, are the major players of cancer-related inflammation (109) and play a crucial role in NK cell suppression in the tumor milieu of CRC. Cancer-associated fibroblasts (CAFs) by enhancement of TAMs impair the NK cell function (98).
Elevated TGF-β in patients suffering metastatic CRC is reported (110). TGF-β receptor mutation causes CRC with microsatellite instability (MSI-high). This kind of mutation, is associated with better survival (111). TGF-β has been showed to impair NK cell cytotoxicity in a CRC mouse model. LY2157299, a TGF-β receptor kinase inhibitor, combined with adoptive NK cells, eradicated the liver metastasis of colon cancer in a mouse liver metastasis CRC model (112).
NK cell manipulation would be a promising target in the CRC immunotherapy context (113). As mentioned here, NK cells are innate immune cells that, without previous exposure to tumor antigens and HLA-priming, provide immunity against tumor cells (114). However, in CRC, NK cell dysfunction represents an immunological failure. NK cell dysfunction allows escape of tumor cells in colorectal, gastric, and pancreatic cancers. In all types of malignancies, a marked decrease in NKP30 NK cells has been reported (115). In cancer, several mechanisms impair NK cell function, for example: a) decreased NK cell count and b)modified phenotype, and impaired function due to inhibitory interactions with other immune cells presented in TME (Figure 2) (116).
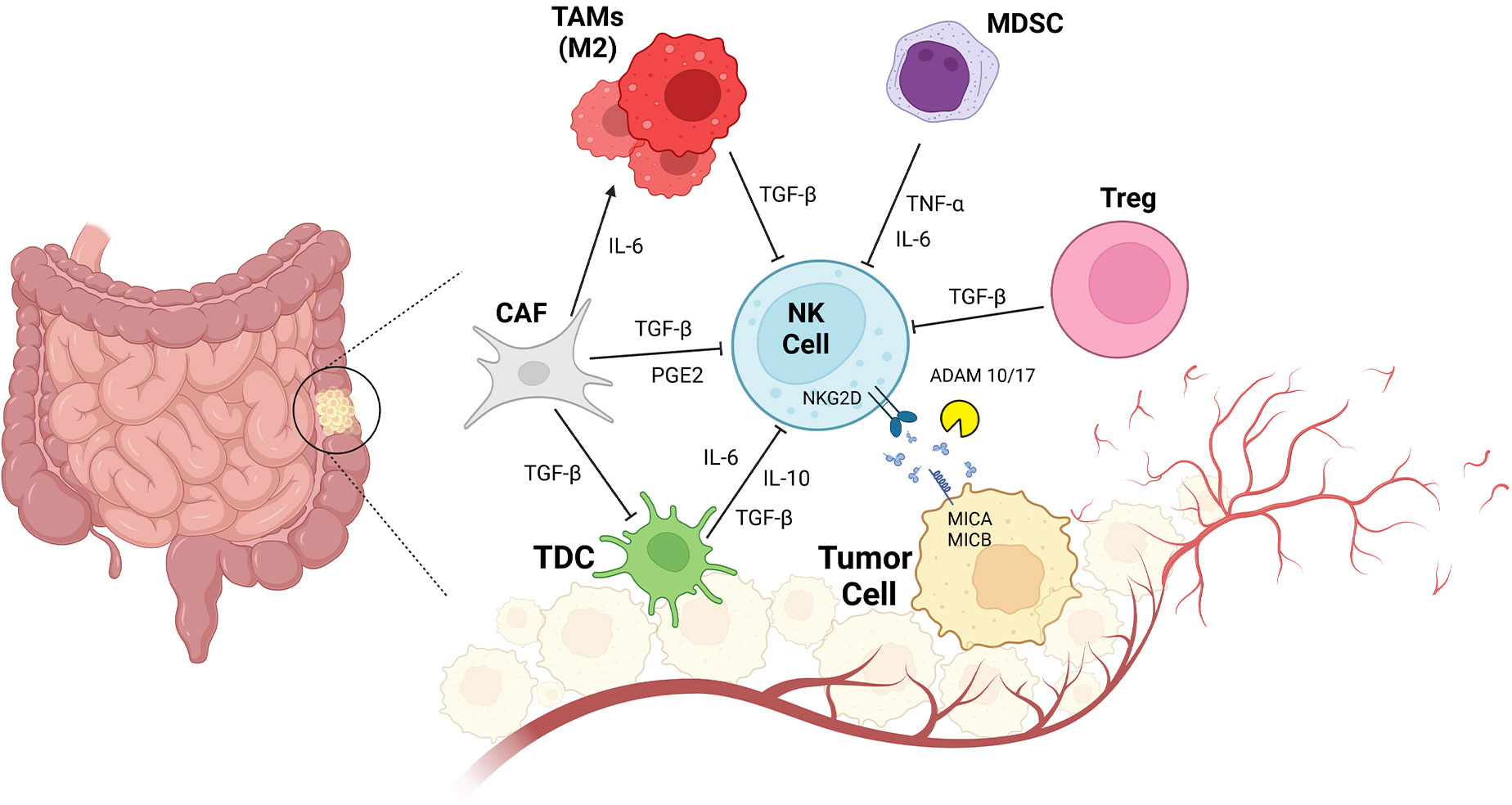
Figure 2 CRC and Impaired NK Cell Function due to innate immune cell interactions. Tumor resident innate immunity cells consist of CAF (cancer associated fibroblast), TAMs (tumor associated macrophage), MDSC (myeloid derived suppressor cells), Treg (regulatory T cells), tDC1(conventional Type1 dendritic cells) interact with NK cells. TGF-β secreted by CAf, TAMs, Treg inactivates NK cells and also DCs in TME. Other regulatory cytokines such as IL-6, IL-10 and TNFα also inhibits NK cells activation. High PGE2 levels makes the NK cells non-responder to tumor cells. Shedding forms of MICA/MICB is a tumor cell direct mechanism to escape from NK cells immune response.
NK cell count is an independent prognostic factor in CRC (11). Immune cell profile in peripheral blood of CRC patients can be a prognostic factor. It was shown an increasing percentage of circulating Tregs and a reduction of CD56dim NK cells in CRC patients. The low rate of CD16+ NK cells has been associated with shorter disease-free survival (DFS) (117). In chronic inflammation, mediators such as Prostaglandin E2 (PGE2) are associated with worse survival of CRC patients. PGE2 and upstream enzymes called COX (Cyclooxygenase) linked to CRC were first studied in 1994 (118). Acid arachidonic metabolizes to PGE2 through the COX pathway. PGE2 mechanism in CRC had been reported to stimulate cell invasion, tumor growth, and apoptosis inhibition (119). When PGE2 is produced in high amounts has an immunosuppressive role in TME produced by either immune cells and tumor cells (120). PGE2 has adverse effects on NK cell function, survival, and proliferation in tumor sites (121). PGE2 downregulates NK cell-activating receptors through cAMP-mediated PKA type I-dependent signaling (122), and it regulates IL-12 and IL-18 dependent INFγ synthesis in NK cells (123). A COX-competent animal model of CRC showed increased secretion of prostaglandin E2 and a lack of conventional type 1 dendritic cells (cDC1) mobilization to the tumor site. PGE2 impaired NK cell accumulation and its ability in tumor site to produce CCL5 chemokine. PGE2 also reduces the expression of CCL5 and CXCL1 receptors on cDC1 cells (124). Inhibiting COX2 activity by andrographolide (a natural diterpenoid from Andrographis Paniculata) decreased PGE2 release. However, the same, improved PD-1 immunotherapy in the xenograft model of CRC by enhancing the functionality of TCD4+ and TCD8+, enhancing INFγ, and increasing cytotoxic molecules such as perforin and granzymes (125). A recent case control study showed PGE2 plasma level is associated by CRC risk so the nonsteroidal anti-inflammatory drugs (NSAIDs) would be a promising personalized medicine in CRC treatment (126).
TME in CRC is a hallmark of cancer progression, immune cell dysfunction, and immunotherapy resistance. There are various physical and chemical alterations in cancers’ microenvironments, such as hypoxia, acidosis, increased extracellular matrix rigidity, and high interstitial fluid pressure (127). The acidic TME is the critical barrier created by tumor cells against immune cells. Acidification of the tumor site is caused by rapid cancer cell proliferation, a high glucose glycolytic metabolism ratio, and increased lactic acid production (128). Metastasis, tumor progression, immune cell suppression, and poor prognosis are sustained by acidotic processes (129). Acidosis in CRC contributes to tumor progression and resistance to conventional treatments (130). It is shown that lactate accumulation in metastatic CRC induces mitochondrial dysfunction and apoptosis in NK cells; it seems metabolism targeting in CRC is a promising therapeutic approach to overcome immune suppression (131). Targeting acidosis to overcome the resistance to anti-PD-1 and anti-CTLA-4 could be promising (132).
Taken together, NK cells dysfunction from different aspects play an important role in CRC immune response. NK cells exert their cytotoxic function toward impaired cells; and through cytokines and chemokines modulate the adaptive immunity too. However, NK cells fail to infiltrate properly in tumor microenvironment, and when reach the tumor site, they would be exhausted due to immune checkpoints inappropriate expression. NK cells dysfunction in tumor immunity provides the basis of new strategies to harness their immune response.
Immunomodulatory drugs potentiate NK cytotoxicity against CRC
Immune cell therapy has become a milestone in cancer therapy; however, the immunosuppressive status of the TME is one of the main barriers for the immune system’s function (133). Therefore, interfering with the TME seems to be the clue to restore the immunologic responses (134). Immunomodulatory drugs may interfere with the immunosuppressive status of the TME.
Immunomodulatory drugs that induce CD 8+ T cells proliferation can enhance cytotoxicity, activation of DCs, Th1 responses, augmentation of NK cells activity, inhibiting tumor angiogenesis, and changing the production of DCs cytokine profile (135, 136). The cross-talk between these drugs and the NK cells is mediated by IL-2 and IFNγ (137). Thalidomide, lenalidomide, and pomalidomide are examples of these drugs with anti-cancer functions. The mechanism of action of these drugs is anti-proliferative, anti-angiogenic, and proapoptotic (131, 132). The effect of these drugs has been intensively studied in acute myeloid leukemia (AML) as monotherapy or in combination with other therapies. In mice animal models where CRC was induced with CT26, lung metastasis was significantly reduced after treatment with Thalidomide, lenalidomide, and pomalidomide. Interestingly, the pre-treatment of the CT26 cell line with lenalidomide reduced the lung metastasis indicating the anti-metastatic effect of this drug (138).
These immunomodulatory drugs have also opened their way to clinical trials. For instance, in a phase II clinical study, lenalidomide was used in combination with Cetuximab in KRAS mutant patients. Lenalidomide significantly increased the number circulating NK cells. The combination of Cetuximab and Lenalidomide increased granzyme-positive NK cells more than lenalidomide only (139).
Thalidomide monotherapy or chemotherapy showed a mild effect on patients with advanced CRC. Thalidomide may affect NK cells’ cytotoxicity by increasing IL-2 and INFγ production (140, 141). Pomalidomide in CRC preclinical setting and in vitro effectively restrained the cytotoxicity of NK cells (142). However, no clinical trial with a pomalidomide-based regime has been conducted in CRC patients so far.
Immuno modulatory drugs reported positively effect on NK cells cytotoxicity by alter cytokine production, NK cells augmentation and decrease metastasis in CRC patients.
Immune checkpoint blockades improved NK cell cytotoxicity in colorectal cancer
NK cells clearly exerting cytotoxic activity against cancer cell and are a key-player of immunotherapy (143). Stimulatory and inhibitory receptors regulate the cytolytic functions of NK cells. Many of these receptors are expressed by NK cells to mount effective anti-tumor immunity (6). The activation receptors present on the NK cell surface, NCRs, include various receptors such as NKp46, NKp30, NKp44, NKG2D, and DNAM-1 (144). Tumor cells and TME adopt different approaches to evade NK cells’ immune responses and surveillance. KIRs, LIRs, NKG2A, and the classical CTLA-4 and PD-1 receptors to recently discovered B7-H7 are immune checkpoint inhibitors that compromise NK cell anti-tumor activity (145). Anti-PD1, Dostarlimab, has been reported to induce complete response with no evidence of tumor in mismatch repair-deficient, locally advanced rectal cancer patients. A clinical study (NCT04165772) was initiated in 2019, and the results demonstrated the complete treatment at least in a 6-month follow-up (146).
Therefore, targeting these inhibitory immune checkpoints, is proposed as one of the immune therapy strategies to augment NK cell effects (147).
Ipilimumab, a CTLA-4 inhibitor monoclonal antibody, is the first immune checkpoint inhibitor to gain approval with outstanding results in different cancers (148). It has been demonstrated that blocking CTLA-4 has increased the expression of IL-2 and enhanced the cytotoxicity of NK cells (149). Ipilimumab has entered clinical trials combined with nivolumab, the anti-PD-1, to treat metastatic CRC (150). Other immune checkpoint inhibitors in metastatic patients are the NKG2A inhibitor, Monalizumab, and PD-L1 inhibitors, Durvalumab, with no severe side effects, mortality, and practical clinical outcome (151). TIGIT is another cell surface inhibitor on NK cells and T cells, causing exhaustion of TIL and tumor-infiltrating NK cells. Either NK deficient TIGIT or using Blockade of this inhibitor alone or combined with Blockade of PD-L1 significantly restored the suppressed anti-tumor immunity by NK cells in CRC animal models and patients (10).
Blockade of immune checkpoints regulates the cytotoxic activity of NK cells and blockade drugs have been approved in so many malignancies; however, some patients are non-responder to these novel treatments that fundamental studies should be further investigate the effective therapies. A comprehensive overview of the immune checkpoint inhibitors and their Blockade is presented in Table 2.
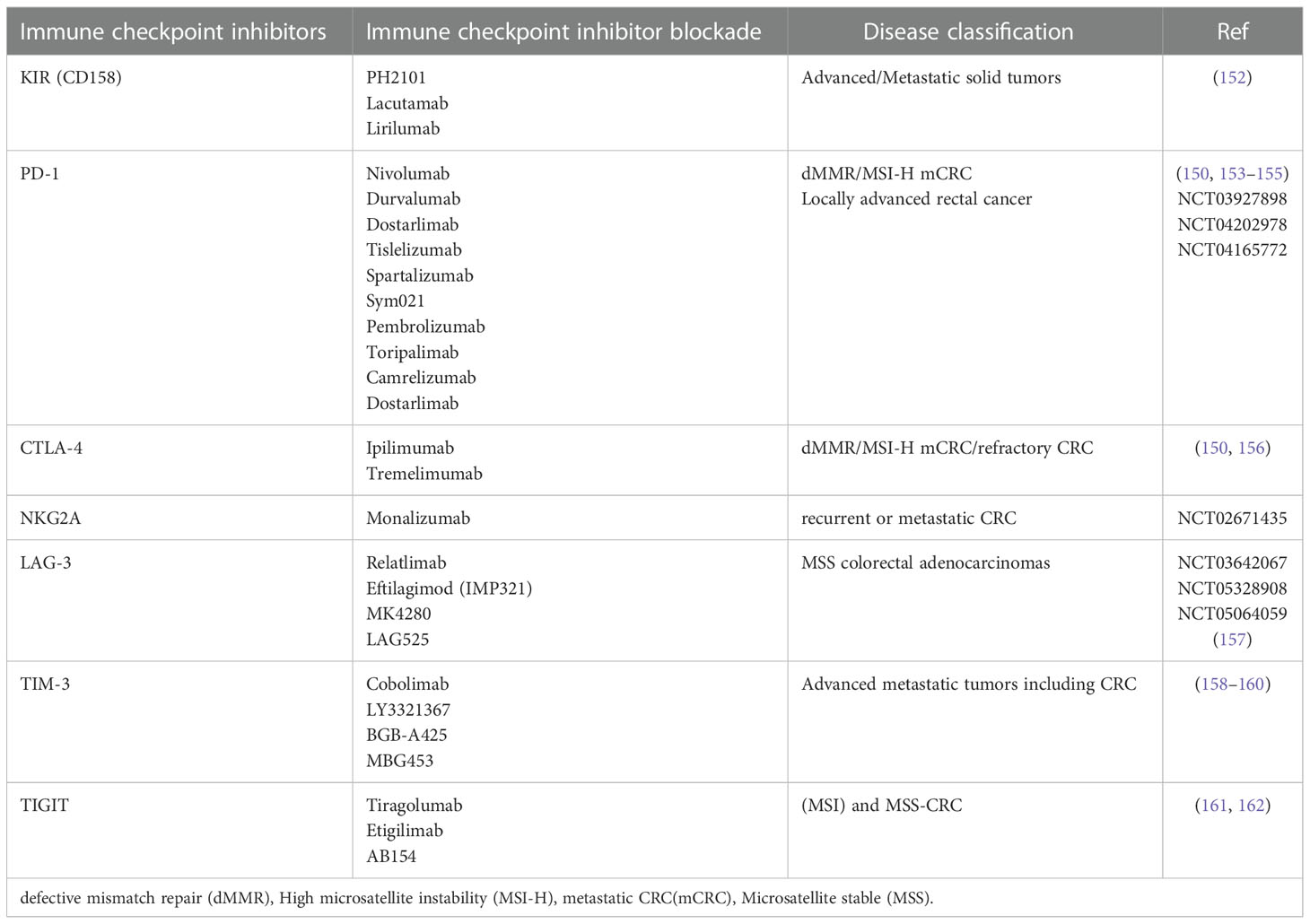
Table 2 Immune checkpoint inhibitors present on NK cells and their blockade under clinical and preclinical investigations in CRC.
Antibodies increase NK cell cytotoxicity against colorectal cancer
Antibody-dependent Cellular cytotoxicity (ADCC) is a mechanism of NK cells’ defense against tumor cells independent of MHC recognition and complement system activation. Antibodies even derived from adaptive immunity activation (a classic form of ADCC) or commercial types (monoclonal antibodies) elicit the cytotoxic activity of NK cells. NK cells interact with antibodies through FcγRIIc/CD32c and FcγRIIIa/CD16a receptors that bind to antibodies Fc portion. IgG1 sub-type comprises high affinity to FC receptors and mediates the NK cells’ ADCC activity. CD16a cross-links on NK cells following antibodies binding (163, 164). CD16a and CD32c are associated with the CD3-ζ chain containing ITAMs (Immune tyrosine-based activating motifs) residue in the cytoplasmic tail. Phosphorylated ITAMs result in NK cell degranulation, cytokine release, and tumor cell lysis by inducing TNF, FasL, and TRAIL death receptors, IFNγ secretion (165), granzyme and perforin release, caspase 8 activation and apoptosis (166). Besides induced ADCC by classical form and monoclonal antibodies, genetically manipulated NK cells expressing high-affinity CD16a will also trigger ADCC more prominently. Reportedly engineered NK-92 cell lines derived from human lymphoma expressing high-affinity CD16a, producing high amounts of IL-2 and granzymes, are highly cytotoxic against colon cancer cell lines (167).
Commercial immunoglobulin molecules are promising approaches in CRC treatment since they interfere with tumor angiogenesis and immune system modulation and induce direct immune cell cytotoxicity through NK cells (168).
CRC disease progression is highly dependent from two signaling pathways; EGFR and VEGF (169). The trastuzumab (anti-HER2), cetuximab (anti-EGFR), panitumumab (anti-EGFR), ramucirumab (anti-VEGFR2), and bevacizumab (anti-VEGF-A) are commercial specific monoclonal antibodies that are currently used in CRC treatment (170). High expression of HER-2 in CRC-derived cancer stem cells was reported with activation of the PI3K/AKT pathway exacerbating tumor cell proliferation. Recent findings showed that monoclonal antibodies in combination with other immune therapy increase treatment efficacy significantly in CRC. HER-2 targeting in combination with PI3K and MEK inhibitors induces tumor regression in avatar models of CRC (171). Cetuximab beneficially intensifies the NK cell cytotoxicity against CRC nude mice model (172).
Cetuximab combined with nivolumab was reported to be well tolerated with less efficacy in metastatic colorectal cancer (166). Panitumumab, combined with standard chemotherapy regimens (FOLFOX), is the first-line treatment in RAS mutated colorectal cancer. Re-treatment, even in the second or third line, could have potential benefits (141). The immunoglobulin backbone of panitumumab that has a low binding site affinity for CD16a, does not induce NK cells mediated ADCC (173). VEGF or VEGFR targeting is the other approach to monoclonal antibody treatment in CRC. Ramucirumab is an IgG1 monoclonal antibody targeting VEGFR-2 and is reported to be a prominent treatment in combination with chemotherapy (FOLFIRI regimens) for second and late-line treatment of metastatic CRC by inhibiting the tumor angiogenesis, improving patients overall survival (174, 175). However, it has not been reported that ramucirumab enhance NK cells’ ADCC.
Bispecific antibodies contain two binding sites against two different antigens. Some bispecific antibodies connect immune cells to tumor cells, triggering the immune cells cytotoxicity. Other bispecific antibodies can target two check points in tumor cells. Additionally, some are designed to concurrently target tumor-associated antigens and check points (176–178). Duligotuzumab, a bispecific antibody against EGFR and Her3, contains FC domains activating NK cells’ ADCC (179). One of the BIKE (bispecific NKcells’engager) antibodies recognizing CD16 on NK cells and EPCAM on tumor cells that facilitates ADCC but not the proliferation of NK cells was engineered by Joerg.U and colleagues in 2013 (180). This group incorporated a modified IL-15 cross-linker to the previous BIKE construct to create a TriKE (trispecific construct) which can improve activation, proliferation, and survival of NK cells (181). Patient derived xenograft models effectively simulate the tumors. These modelsare used to investigate the antitumor effects of immune cell therapy in combination with other agents (182). An antibody featuring simultaneous identification of two variants of CD20 and CD16 [(CD20)2xCD16] is one of the major trispecific antibodies that can effectively activate NK cells’ ADCC. More over this can be a mediator of malignant B-cells’ lysis in animal model (183).
Cytokines also play an essential role in activating NK cells’ ADCC; they are important mediators in tumorigenesis and would be used as anti-cancer treatments in CRC (184). Co-administration of IL-21 with cetuximab in phase I clinical trial of CRC increased NK cell-mediated ADCC (178). Cetuximab in combination with IL-2 and IL-15 improved the cytotoxicity of dysregulates blood NK cells in CRC patients (185). Moreover, combination therapy of rituximab and IL-2 demonstrated a synergistic role in activating NK cells (186). ALT-803 and IL-15 super agonists have also been reported to increase NK cell-mediated responses through CD16a, thus inducing ADCC (187, 188).
Following preclinical research, the synergy potential of targeted antibodies with NK cell therapy should be further investigated in clinical trials. Our research group demonstrated that pretreated checkpoint blockade NK cells could effectively enhance the NK cells’ trafficking in TME and beneficially reduce the tumor cell mitosis in gastric cancer xenograft model (189). However the same results was not achieved in chemo immune cell therapy of Intratumoral injection of NK cells in combination with capecitabine in gastric cancer xenograft model in the other study of our group (190).
Vaccines augment anti-tumor immune responses against colorectal cancer
In cancer cells, many proteins look similar to healthy proteins, which keeps the cancerous cells out of the immune system’s reach. Vaccines expose antigens to the immune system and this will trigger the immune response. Identifying the exact antigens in different cancer types is the most crucial step in developing cancer vaccines. Different types of therapeutic vaccines have been developed for CRC patients. Cell-based vaccines are either tumor lysate or immune cells modified to present tumor antigens and tumor antigens’ receptors against CRC. Molecular-based vaccines use tumor-specific antigens known as neoantigens to inhibit tumor progression. Vector-based vaccines present tumor antigens using microorganisms in immunogenic or engineered form (191).
Sipuleucel-T (Provenge) is the first autologous immune cell-based vaccine approved by FDA, 2010 (NCT00779402) for prostate cancer. Cell-based vaccines have been investigated for CRC treatment since 1990 (192, 193). Whole cell-based vaccines are the sub-group that comprise the entire tumor cells antigens and would evoke the immune system against tumor-associated antigens. The development of an universal vaccine that could protect any patients is a complex task (194). Cancer stem cells could be isolated from whole tumor cells to develop a cancer stem cell-based vaccine. A recent study demonstrated that the targeted MUC1+ CRC stem cells vaccine significantly increases NK cell infiltration and cytotoxicity. CCSC targeted vaccination promotes the release of INFγ, perforin, and granzyme B, decreasing TGF-β production (195). Furthermore, an allogenic cell base vaccine could be a valuable alternative to an autologous tumor cells vaccine. Allogeneic vaccines based on CRC cell lines (HT-29 and SW-480) were reported to induce anti-tumor immunity in CRC by increasing the NK cells’ cytotoxic activity (186).
In a preclinical study of colorectal liver metastasis, vaccination with CT26-derived tumors treated with mitoxantrone (MTX) improved NK cell and T cell infiltration to the tumor site and improved clinical response (196).
DC-based vaccines were mostly reported in clinical research to be constructed based on CEA antigens. DCs were modified to deliver CEA antigens by two CEA mRNA pulsed DCs, and CEA peptide-loaded DCs approaches. Results demonstrated that the DC vaccination was well tolerated and that NK cells level was increased (197, 198). Moreover, a clinical study of 10 patients suffering from CRC demonstrated that the CEA peptide-loaded DC vaccine could increase CEA- specific CTL and NK cell response (199).
Molecular-based vaccines have also been reported to improve NK cells’ cytotoxicity against colon tumors (200). DNA vaccines are based on direct introduction of a tumoral antigen through plasmids containing the TSA sequence. According to the U.S National library of medicine, 62% of DNA vaccine clinical trials in the United States are assigned to cancer vaccines. A DNA-based vaccine encapsulated in polyplex micelles containing TAA, SART3 (squamous cell carcinoma antigen), and C40L + GMCSF as adjuvant genes stimulated CTLs and NK cells’ efficient immune response in peritoneal metastasis of CT26-derived tumor s (200).
Vector-based vaccines composed of viral-based, bacterial-based, and yeast-based vaccines are high-tech approaches to cancer treatment. Immunogenic viruses will be engineered to express TAAs, and the antigens will be presented to cytotoxic T cells to eliminate cancer progression. It has been reported that systemic activation of NK cells and systemic anti-tumor response occur following viral-based vaccination (201). Recently a randomized phase-II clinical trial showed that CEA-targeted adenoviral vaccine in combination with Avelumab and FOLFOX6 is safe. This treatment generated specific NK cellTCD4+/TCD8+ and ki67, NKP30+. However, the trial failed to show and improvement of PFS (202).
Although therapeutic CRC vaccines have shown considerable capacity in tumor inhibition, more investigation are needed before any clinical use. Recent registered clinical research is based on combination therapy of vaccines with approved immune checkpoint blockades and chemotherapy as standard of care (203). The combination of two different antigen delivery systems in first-line treatment and vaccine booster can inhibit the immune system tolerance against the first vectors when more than one dose of vaccine is needed, especially in high recurrence cancers. The most critical issues in vaccine development are the selection of optimal antigens, adjuvant, and delivery methods. Current registered clinical trials of vaccine therapy in CRC are summarized in Table 3.
NK cells gene modification improves immunogenicity of colorectal cancer
Adoptive manipulated cell therapy has become a highly promising treatment for advanced cancers. Patients affected by Multiple Myeloma and B-lymphoma showed significant results after receiving autologous T cells (204–206). Current T-cell therapy uses gene-modified chimeric antigen receptor (CAR-T). This was approved in August 2017 for the first time by the Food and Drug Administration (207, 208). However, CAR-modified T cells still have several functional and technical limitations. It can be challenging to generate a product for one patient only, and production costs are economically not sustainable for most health care systems. In addition, autologous products require longer time to generate CAR T-cells.
Allogeneic products have the potential to overcome these limitations, and allogeneic HLA-matched T-cells can mediate graft versus host disease (GvHD) (209). During the CAR-T cell development NK cell- therapy was also considered. NK cells provide an attractive source of allogeneic cells and have become one of the hopes of the CAR engineering approach. Allogeneic NK cells with a short life span do not cause GvHD and also have less long-term adverse events (210–212). Furthermore, donor selection is based on killer cell immunoglobulin receptor (KIR)-ligand mismatch with the recipient or haplotype B KIR gene, which could be beneficial in allogeneic stem cell transplantation (213). CAR NK cell generation has been based on the CAR-T cell platform (comprising CD3ζ and T cell co-stimulatory molecules). It has been shown that these cells target cancer cells with the desired specificity and effectiveness (214). Therefore, CAR-NK-based therapy has been performed on CRC, showing that EpCAM-CAR-NK-92 cells combined with Regorafenib suppress EpCAM-positive tumor xenografts (215). Furthermore, Masayuki Shiozawa et al. demonstrated anti-CEA-CAR NK-92MI cells in a CEA-dependent manner recognized and lysed high CEA-expressing tumor cells (215, 216) and NKG2D CAR mRNA-engineered NK cells significantly improved the cytolytic activity of NK cells against tumors (217). Preclinical models are crucial stages that recapitulate the individual tumor phenotype. The organoid culture system allows long-term ex vivo expansion of gastrointestinal stem cells in a 3D extracellular matrix. In a study by Theresa E Schnalzger et al., CRC organoid was admitted to evaluate the performance of EpCAM-CAR-NK-92 and FRIZZLED-CAR-NK-92. This 3D platform is useful for evaluating CAR-engineered lymphocytes (218). Furthermore, the recent development and efficient CRISPR/Cas9 genome-editing technologies have accumulated NK cell properties and offered new opportunities to increase their susceptibility to NK surveillance. Lanlan Gao et al. demonstrated up regulation of CXCR2 and IL-2 Via CRISPR-Cas9 improved NK-92 cell anti-tumor effects, and survival time was significantly prolonged as cellular immunotherapy for CRC (219).
Engineered NK cells are the next generation of immune cell therapy products with enhanced proliferation and homing capacity and blocked suppressing signals that will enhance their tumor killing properties.
NK cell-based clinical trial of colorectal cancer
NK cell-based therapies are well developed for hematological malignancies such as Acute Myeloid Leukemia (AML) (220, 221). The FDA and EMA have represented ONKord as an off-shelf orphan drug for AML patients: the allogeneic partial HLA-matched NK cells derived from UCB-CD34 + progenitors.
Despite the successes in hematologic malignancies, NK-based therapies for CRC as a solid tumor are associated with challenges. The most difficult are NK cell source and ex vivo expansion, lymphocyte infiltration, and tumor escape from the immune surveillance. These challenges have led to fewer trials on this cancer, which has been rising in recent years (Table 4). iPSC- differentiated NK cells (iPSC-NK) are homogeneously differentiated and more suitable than NK-92. Other clinical trials (NCT03841110 and NCT04106167) examined the safety and efficacy of these cells under the name FT500 against CRC.
The first human study of NK cell transfer in CRC showed stable disease in metastasis and progression stages and was also reported to be safe with no side effects, mainly including GvHD (222–224). Furthermore, a phase-I clinical trial revealed the safety of autologous NK cell therapy and was reported tolerable in patients suffering from CRC who had failed previous standard therapy. Autologous NK cells were administered dose-escalating (dose 0.5 × 109, 1.0 × 109, 2.0 × 109 cells/injection) three times/week. The results demonstrated that adoptive NK cell monotherapy caused no clinical responses besides safety and was undesirable for patients. To improve their efficacy, combining approaches with other immune therapy agents should be considered (225).
It has become increasingly clear that NK therapy alone has limited efficacy in solid tumors. Most studies have shifted to engineered NKs and combined therapies.
Takeshi Ishikawa et al. performed a human study (N = 9) of expanded NK cells in combination with IgG1 antibody (Trastuzumab, Cetuximab). Patients received NK cells after three days of IgG1 antibody administration were infused with expanded NK cells in three steps, at doses of 0.5 × 109, 1.0 × 109, and 2.0 × 109 cells/injection at tri-weekly intervals. A decrease in tumor size in three patients and raised whole blood IFNγ production after combination therapy was observed (226).
Allogeneic NK cells combined with Cetuximab were administered in metastatic colorectal carcinoma (N = 6) to evaluate the safety and efficacy of NK cell delivery in the phase-I clinical trial. NK cells were administrated, followed by high-dose IL-2 (3×106, 8×106, and 12×106 NK cells/kg). Reportedly, NK cell-Cetuximab combination approach was well tolerated. However, clinical responses should be further investigated (227). Chimeric antigen receptor (CAR) -carrying cells have been shown to be effective in hematologic malignancy.
In comparison to CAR based T cell therapy, allogeneic CAR-NK cell therapy caused no GvHD (228, 229), accompanied by a shorter final production time (230, 231), and also the final product would be “off-the-shelf” (232). LinXiao et al. performed an open-label pilot study (NCT03415100) to assess the safety of NKG2D CAR mRNA-engineered NK Cells to improve their cytolytic activity against metastatic CRC patients. No serious adverse effects (≥grade 3 adverse events) existed in any of the three patients except grade 1 cytokine release syndrome. CAR-NK cells augmented NK-based therapy when they were administered intra -peritoneal in a dose-escalation manner to reduce off-target risks (217). Moreover, a clinical trial was recently registered (NCT02839954) to administer anti-MUC1 CAR-NK cells in patients suffering from solid tumors, including colorectal cancer. Additionally, a phase-I clinical study (NCT04319757) is recruiting patients to investigate the safety and preliminary efficacy of anti-HER2 oNK cells (ACE1702) against human HER2-expressing solid tumors as an off-the-shelf NK cell product.
The infiltration and active persistence of NK cells and genetic manipulation have been among researchers’ priorities. In recent years, the identification of checkpoint inhibitors has led to immunotherapy development, which is being evaluated in combination (NCT03841110) with FT500 to inhibit cancer immunity. Ongoing clinical trials of therapeutic NK cells in CRC have been reviewed in Table 4.
Conclusion
NK cell therapy has been widely adopted as an efficient cancer treatment, however; the limitation and challenges, especially in solid tumors, are indisputable. The major limitations reported are the non-targeted responses of NK cells, the immune suppressive tumor microenvironment, and the NK cell infiltration barriers to the tumor site. Genetic engineering strategy has developed chimeric antigen receptors NK cells; in addition, immune checkpoints recognition and development of immune blockade molecules effectively improved the adaptive NK cell therapy effectiveness. The use of NK cells to target cancer cells and understanding the NK cell–cancer interactions is constantly evolving.
Author contributions
The authors confirm responsibility for the following: study conception and design, data collection, analysis and interpretation of results, and manuscript preparation. All authors contributed to the article and approved the submitted version.
Conflict of interest
The authors declare that the research was conducted in the absence of any commercial or financial relationships that could be construed as a potential conflict of interest.
Publisher’s note
All claims expressed in this article are solely those of the authors and do not necessarily represent those of their affiliated organizations, or those of the publisher, the editors and the reviewers. Any product that may be evaluated in this article, or claim that may be made by its manufacturer, is not guaranteed or endorsed by the publisher.
References
1. Piątkiewicz P, Miłek T, Bernat–Karpińska M, Ohams M, Czech A, Ciostek P. The dysfunction of NK cells in patients with type 2 diabetes and colon cancer. Arch Immunol Ther Exp (Warsz). (2013) 61(3):245–53. doi: 10.1007/s00005-013-0222-5
2. Cooper MA, Fehniger TA, Caligiuri MA. Biol hum nat kill subsets. Trends Immunol (2001) 22:633–40. doi: 10.1016/S1471-4906(01)02060-9
3. Smith HRC, Heusel JW, Mehta IK, Kim S, Dorner BG, Naidenko OV, et al. Recognition of a virus–encoded ligand by a natural killer cell activation receptor. Proc Natl Acad Sci (2002) 99(13):8826–31. doi: 10.1073/pnas.092258599
4. Cooper MA, Elliott JM, Keyel PA, Yang L, Carrero JA, Yokoyama WM. Cytokine–induced memory–like natural killer cells. Proc Natl Acad Sci (2009) 106(6):1915–9. doi: 10.1073/pnas.0813192106
5. Becker–Hapak MK, Shrestha N, McClain E, Dee MJ, Chaturvedi P, Leclerc GM, et al. A fusion protein complex that combines IL–12, IL–15, and IL–18 signaling to induce memory–like NK cells for cancer ImmunotherapyHCW9201 induces memory–like NK cells for cancer immunotherapy. Cancer Immunol Res (2021) 9(9):1071–87. doi: 10.1158/2326-6066.CIR-20-1002
6. Sivori S, Vacca P, Del Zotto G, Munari E, Mingari MC, Moretta L. Human NK cells: surface receptors, inhibitory checkpoints, and translational applications. Cell Mol Immunol (2019) 16(5):430–41. doi: 10.1038/s41423-019-0206-4
7. Pitt JM, Vétizou M, Daillère R, Roberti MP, Yamazaki T, Routy B, et al. Resistance mechanisms to immune–checkpoint blockade in cancer: tumor–intrinsic and–extrinsic factors. Immunity. (2016) 44(6):1255–69. doi: 10.1016/j.immuni.2016.06.001
8. André P, Denis C, Soulas C, Bourbon–Caillet C, Lopez J, Arnoux T, et al. Anti–NKG2A mAb is a checkpoint inhibitor that promotes anti–tumor immunity by unleashing both T and NK cells. Cell. (2018) 175(7):1731–43. doi: 10.1016/j.cell.2018.10.014
9. Lin A, Yan W–H. Heterogeneity of HLA–G expression in cancers: facing the challenges. Front Immunol (2018) 9:2164. doi: 10.3389/fimmu.2018.02164
10. Zhang Q, Bi J, Zheng X, Chen Y, Wang H, Wu W, et al. Blockade of the checkpoint receptor TIGIT prevents NK cell exhaustion and elicits potent anti–tumor immunity. Nat Immunol (2018) 19(7):723–32. doi: 10.1038/s41590-018-0132-0
11. Tang Y, Xie M, Li K, Li J, Cai Z, Hu B. Prognostic value of peripheral blood natural killer cells in colorectal cancer. BMC Gastroenterol (2020) 20(1):1–8. doi: 10.1186/s12876-020-1177-8
12. Grzywacz B, Kataria N, Verneris M. CD56 dim CD16+ NK cells downregulate CD16 following target cell induced activation of matrix metalloproteinases. Leukemia. (2007) 21(2):356–9. doi: 10.1038/sj.leu.2404499
13. Della CM, Carlomagno S, Frumento G, Balsamo M, Cantoni C, Conte R, et al. The tryptophan catabolite l–kynurenine inhibits the surface expression of NKp46–and NKG2D–activating receptors and regulates NK–cell function. Blood. (2006) 108(13):4118–25. doi: 10.1182/blood-2006-03-006700
14. Li T, Yi S, Liu W, Jia C, Wang G, Hua X, et al. Colorectal carcinoma–derived fibroblasts modulate natural killer cell phenotype and antitumor cytotoxicity. Med Oncol (2013) 30(3):663. doi: 10.1007/s12032-013-0663-z
15. Chang C–C, Campoli M, Ferrone S. Classical and nonclassical HLA class I antigen and NK cell–activating ligand changes in malignant cells: current challenges and future directions. Adv Cancer Res (2005) 93:189–234. doi: 10.1016/S0065-230X(05)93006-6
16. De Re V, Caggiari L, De Zorzi M, Talamini R, Racanelli V, Andrea MD, et al. Genetic diversity of the KIR/HLA system and outcome of patients with metastatic colorectal cancer treated with chemotherapy. PloS One (2014) 9(1):e84940. doi: 10.1371/journal.pone.0084940
17. Eugène J, Jouand N, Ducoin K, Dansette D, Oger R, Deleine C, et al. The inhibitory receptor CD94/NKG2A on CD8+ tumor–infiltrating lymphocytes in colorectal cancer: a promising new druggable immune checkpoint in the context of HLAE/β2m overexpression. Mod Pathol (2020) 33(3):468–82. doi: 10.1038/s41379-019-0322-9
18. Kaiser BK, Pizarro JC, Kerns J, Strong RK. Structural basis for NKG2A/CD94 recognition of HLA–e. Proc Natl Acad Sci (2008) 105(18):6696–701. doi: 10.1073/pnas.0802736105
19. Diefenbach A, Tomasello E, Lucas M, Jamieson AM, Hsia JK, Vivier E, et al. Selective associations with signaling proteins determine stimulatory versus costimulatory activity of NKG2D. Nat Immunol (2002) 3(12):1142–9. doi: 10.1038/ni858
20. Ghadially H, Brown L, Lloyd C, Lewis L, Lewis A, Dillon J, et al. MHC class I chain–related protein a and b (MICA and MICB) are predominantly expressed intracellularly in tumour and normal tissue. Br J Cancer. (2017) 116(9):1208–17. doi: 10.1038/bjc.2017.79
21. Ferrari de Andrade L, Tay RE, Pan D, Luoma AM, Ito Y, Badrinath S, et al. Antibody–mediated inhibition of MICA and MICB shedding promotes NK cell–driven tumor immunity. Sci (80– ). (2018) 359(6383):1537–42. doi: 10.1126/science.aao0505
22. López–Soto A, Huergo–Zapico L, Acebes–Huerta A, Villa–Alvarez M, Gonzalez S. NKG2D signaling in cancer immunosurveillance. Int J cancer. (2015) 136(8):1741–50. doi: 10.1002/ijc.28775
23. Feng Q, Yu S, Mao Y, Ji M, Wei Y, He G, et al. High MICB expression as a biomarker for good prognosis of colorectal cancer. J Cancer Res Clin Oncol (2020) 146(6):1405–13. doi: 10.1007/s00432-020-03159-0
24. de Andrade LF, Kumar S, Luoma AM, Ito Y, da Silva PHA, Pan D, et al. Inhibition of MICA and MICB shedding elicits NK–cell–mediated immunity against tumors resistant to cytotoxic T cells. Cancer Immunol Res (2020) 8(6):769–80. doi: 10.1158/2326-6066.CIR-19-0483
25. Smith TM Jr., Tharakan A, Martin RK. Targeting ADAM10 in cancer and autoimmunity. Front Immunol (2020) 11:499. doi: 10.3389/fimmu.2020.00499
26. Maurer S, Kropp KN, Klein G, Steinle A, Haen SP, Walz JS, et al. Platelet–mediated shedding of NKG2D ligands impairs NK cell immune–surveillance of tumor cells. Oncoimmunology. (2018) 7(2):e1364827. doi: 10.1080/2162402X.2017.1364827
27. Neuss S, Bartel Y, Born C, Weil S, Koch J, Behrends C, et al. Cellular mechanisms controlling surfacing of AICL glycoproteins, cognate ligands of the activating NK receptor NKp80. J Immunol (2018) 201(4):1275–86. doi: 10.4049/jimmunol.1800059
28. Ferretti E, Carlomagno S, Pesce S, Muccio L, Obino V, Greppi M, et al. Role of the main non HLA–specific activating NK receptors in pancreatic, colorectal and gastric tumors surveillance. Cancers (Basel). (2020) 12(12):3705. doi: 10.3390/cancers12123705
29. Biassoni R. Natural killer cell receptors. multichain immune recognit. Recept Signal (2008) 640:35–52. doi: 10.1007/978-0-387-09789-3_4
30. Deschaseaux F, Delgado D, Pistoia V, Giuliani M, Morandi F, Durrbach A. HLA–G in organ transplantation: towards clinical applications. Cell Mol Life Sci (2011) 68(3):397–404. doi: 10.1007/s00018-010-0581-6
31. Quatrini L, Della Chiesa M, Sivori S, Mingari MC, Pende D, Moretta L. Human NK cells, their receptors and function. Eur J Immunol (2021) 51(7):1566–79. doi: 10.1002/eji.202049028
32. Campbell KS. Mystery checkpoint revealed: KIR3DL3 finally found a ligand in HHLA2. Cancer Immunol Res (2021) 9(2):128. doi: 10.1158/2326-6066.CIR-20-0996
33. Jung JM, Ching W, Baumdick ME, Hofmann–Sieber H, Bosse JB, Koyro T, et al. KIR3DS1 directs NK cell–mediated protection against human adenovirus infections. Sci Immunol (2021) 6(63):eabe2942. doi: 10.1126/sciimmunol.abe2942
34. Carlomagno S, Falco M, Bono M, Alicata C, Garbarino L, Mazzocco M, et al. KIR3DS1–mediated recognition of HLA–* B51: modulation of KIR3DS1 responsiveness by self HLA–b allotypes and effect on NK cell licensing. Front Immunol (2017) 8:581. doi: 10.3389/fimmu.2017.00581
35. Peipp M, Klausz K, Boje AS, Zeller T, Zielonka S, Kellner C. Immunotherapeutic targeting of activating natural killer cell receptors and their ligands in cancer. Clin Exp Immunol (2022) 209(1):22–32. doi: 10.1093/cei/uxac028
36. Garg A, Barnes PF, Porgador A, Roy S, Wu S, Nanda JS, et al. Vimentin expressed on mycobacterium tuberculosis–infected human monocytes is involved in binding to the NKp46 receptor. J Immunol (2006) 177(9):6192–8. doi: 10.4049/jimmunol.177.9.6192
37. Hecht M–L, Rosental B, Horlacher T, Hershkovitz O, De Paz JL, Noti C, et al. Natural cytotoxicity receptors NKp30, NKp44 and NKp46 bind to different heparan sulfate/heparin sequences. J Proteome Res (2009) 8(2):712–20. doi: 10.1021/pr800747c
38. Mendelson M, Tekoah Y, Zilka A, Gershoni–Yahalom O, Gazit R, Achdout H, et al. NKp46 O–glycan sequences that are involved in the interaction with hemagglutinin type 1 of influenza virus. J Virol (2010) 84(8):3789–97. doi: 10.1128/JVI.01815-09
39. Puiggros A, Blanco G, Muntasell A, Rodriguez–Rivera M, Nonell L, Puigdecanet E, et al. Reduced expression of the CD94/NKG2C NK cell receptor in chronic lymphocytic leukemia (CLL) and CLL–like monoclonal b–cell lymphocytosis (MBL). Blood. (2019) 134:5457. doi: 10.1182/blood-2019-128055
40. Norman PJ. A transcriptional signature of PDGF–DD activated natural killer cells predicts more favorable prognosis in low–grade glioma. Innate Lymphoid Cells Cancer Friends Foes? (2022) 40. doi: 10.3389/fimmu.2021.668391
41. Khanam A, Chua JV, Kottilil S. Immunopathology of chronic hepatitis b infection: Role of innate and adaptive immune response in disease progression. Int J Mol Sci (2021) 22(11):5497. doi: 10.3390/ijms22115497
42. Kumar P, Bhattacharya P, Prabhakar BS. A comprehensive review on the role of co–signaling receptors and treg homeostasis in autoimmunity and tumor immunity. J Autoimmun (2018) 95:77–99. doi: 10.1016/j.jaut.2018.08.007
43. Schwartzberg PL, Mueller KL, Qi H, Cannons JL. SLAM receptors and SAP influence lymphocyte interactions, development and function. Nat Rev Immunol (2009) 9(1):39–46. doi: 10.1038/nri2456
44. O’Neill MA, Hidalgo LG. NK cells in antibody–mediated rejection–key effector cells in microvascular graft damage. Int J Immunogenet. (2021) 48(2):110–9. doi: 10.1111/iji.12532
45. Starzer AM, Berghoff AS. New emerging targets in cancer immunotherapy: CD27 (TNFRSF7). ESMO Open (2019) 4:e000629. doi: 10.1136/esmoopen-2019-000629
46. Takeda K, Oshima H, Hayakawa Y, Akiba H, Atsuta M, Kobata T, et al. CD27–mediated activation of murine NK cells. J Immunol (2000) 164(4):1741–5. doi: 10.4049/jimmunol.164.4.1741
47. Kuklina EM. Receptor functions of semaphorin 4D. Biochem. (2019) 84(9):1021–7. doi: 10.1134/S0006297919090049
48. Mizrahi S, Markel G, Porgador A, Bushkin Y, Mandelboim O. CD100 on NK cells enhance IFNγ secretion and killing of target cells expressing CD72. PloS One (2007) 2(9):e818. doi: 10.1371/journal.pone.0000818
49. Wang Z–H, Liu H–H, Ma N, Wang L–H, Liu W, Tang B, et al. A preliminary study on the expression of CD160 on NK cells and its mechanism of mediating NK killing effect. Zhongguo shi yan xue ye xue za zhi. (2018) 26(5):1559–64. doi: 10.7534/j.issn.1009-2137.2018.05.050
50. Han Z, Huang H, Sun Y. Effect of CEACAM−1 knockdown in human colorectal cancer cells. Oncol Lett (2018) 16(2):1622–6. doi: 10.3892/ol.2018.8835
51. Mariotti FR, Quatrini L, Munari E, Vacca P, Tumino N, Pietra G, et al. Inhibitory checkpoints in human natural killer cells: IUPHAR review 28. Br J Pharmacol (2020) 177(13):2889–903. doi: 10.1111/bph.15081
52. Braud VM, Meghraoui–Kheddar A, Elaldi R, Petti L, Germain C, Anjuère F. LLT1–CD161 interaction in cancer: Promises and challenges. Front Immunol (2022) 13:847576. doi: 10.3389/fimmu.2022.847576
53. Jiang K–Y, Qi L–L, Kang F–B, Wang L. The intriguing roles of siglec family members in the tumor microenvironment. biomark Res (2022) 10(1):1–14. doi: 10.1186/s40364-022-00369-1
54. Bryceson YT, March ME, Ljunggren H–G, Long EO. Synergy among receptors on resting NK cells for the activation of natural cytotoxicity and cytokine secretion. Blood. (2006) 107(1):159–66. doi: 10.1182/blood-2005-04-1351
55. Witalisz–Siepracka A, Klein K, Prinz D, Leidenfrost N, Schabbauer G, Dohnal A, et al. Loss of JAK1 drives innate immune deficiency. Front Immunol (2019) 9:3108. doi: 10.3389/fimmu.2018.03108
56. Kortylewski M, Kujawski M, Wang T, Wei S, Zhang S, Pilon–Thomas S, et al. Inhibiting Stat3 signaling in the hematopoietic system elicits multicomponent antitumor immunity. Nat Med (2005) 11(12):1314–21. doi: 10.1038/nm1325
57. Delconte RB, Kolesnik TB, Dagley LF, Rautela J, Shi W, Putz EM, et al. CIS is a potent checkpoint in NK cell–mediated tumor immunity. Nat Immunol (2016) 17(7):816–24. doi: 10.1038/ni.3470
58. Wiedemann GM, Grassmann S, Lau CM, Rapp M, Villarino AV, Friedrich C, et al. Divergent role for STAT5 in the adaptive responses of natural killer cells. Cell Rep (2020) 33(11):108498. doi: 10.1016/j.celrep.2020.108498
59. Wiedemann GM, Santosa EK, Grassmann S, Sheppard S, Le Luduec J–B, Adams NM, et al. Deconvoluting global cytokine signaling networks in natural killer cells. Nat Immunol (2021) 22(5):627–38. doi: 10.1038/s41590-021-00909-1
60. Widowati W, Jasaputra DK, Sumitro SB, Widodo MA, Mozef T, Rizal R, et al. Effect of interleukins (IL–2, IL–15, IL–18) on receptors activation and cytotoxic activity of natural killer cells in breast cancer cell. Afr Health Sci (2020) 20(2):822–32. doi: 10.4314/ahs.v20i2.36
61. Mukherjee S, Jensen H, Stewart W, Stewart D, Ray WC, Chen S–Y, et al. In silico modeling identifies CD45 as a regulator of IL–2 synergy in the NKG2D–mediated activation of immature human NK cells. Sci Signal (2017) 10(485):eaai9062. doi: 10.1126/scisignal.aai9062
62. Vuletić A, Jovanić I, Jurišić V, Milovanović Z, Nikolić S, Spurnić I, et al. IL–2 and IL–15 induced NKG2D, CD158a and CD158b expression on T, NKT–like and NK cell lymphocyte subsets from regional lymph nodes of melanoma patients. Pathol Oncol Res (2020) 26(1):223–31. doi: 10.1007/s12253-018-0444-2
63. Liu M, Meng Y, Zhang L, Han Z, Feng X. High–efficient generation of natural killer cells from peripheral blood with preferable cell vitality and enhanced cytotoxicity by combination of IL– IL–15 and IL–18. Biochem Biophys Res Commun (2021) 534:149–56. doi: 10.1016/j.bbrc.2020.12.012
64. Alwan LM, Grossmann K, Sageser D, Van Atta J, Agarwal N, Gilreath JA. Comparison of acute toxicity and mortality after two different dosing regimens of high–dose interleukin–2 for patients with metastatic melanoma. Target Oncol (2014) 9(1):63–71. doi: 10.1007/s11523-013-0276-7
65. Yasuda K, Nirei T, Tsuno NH, Nagawa H, Kitayama J. Intratumoral injection of interleukin–2 augments the local and abscopal effects of radiotherapy in murine rectal cancer. Cancer Sci (2011) 102(7):1257–63. doi: 10.1111/j.1349-7006.2011.01940.x
66. Tijink BM, Perk LR, Budde M, Stigter–van Walsum M, Visser GWM, Kloet RW, et al. 124I–L19–SIP for immuno–PET imaging of tumour vasculature and guidance of 131I–L19–SIP radioimmunotherapy. Eur J Nucl Med Mol Imaging. (2009) 36(8):1235–44. doi: 10.1007/s00259-009-1096-y
67. Fehniger TA, Caligiuri MA. Interleukin 15: biology and relevance to human disease. Blood J Am Soc Hematol (2001) 97(1):14–32. doi: 10.1182/blood.V97.1.14
68. Stonier SW, Schluns KS. Trans–presentation: a novel mechanism regulating IL–15 delivery and responses. Immunol Lett (2010) 127(2):85–92. doi: 10.1016/j.imlet.2009.09.009
69. Wang X, Zhao X–Y. Transcription factors associated with IL–15 cytokine signaling during NK cell development. Front Immunol (2021) 12:610789. doi: 10.3389/fimmu.2021.610789
70. Hsu F–T, Liu Y–C, Tsai C–L, Yueh P–F, Chang C–H, Lan K–L. Preclinical evaluation of recombinant human IL15 protein fused with albumin binding domain on anti–PD–L1 immunotherapy efficiency and anti–tumor immunity in colon cancer and melanoma. Cancers (Basel). (2021) 13(8):1789. doi: 10.3390/cancers13081789
71. Patidar M, Yadav N, Dalai SK. Development of stable chimeric IL–15 for trans–presentation by the antigen presenting cells. Front Immunol (2021) 12:1019. doi: 10.3389/fimmu.2021.646159
72. Martomo SA, Lu D, Polonskaya Z, Luna X, Zhang Z, Feldstein S, et al. Single–dose anti–PD–L1/IL–15 fusion protein KD033 generates synergistic antitumor immunity with robust tumor–immune gene signatures and memory responses. Mol Cancer Ther (2021) 20(2):347–56. doi: 10.1158/1535-7163.MCT-20-0457
73. Ma R, Lu T, Li Z, Teng K–Y, Mansour AG, Yu M, et al. An oncolytic virus expressing IL15/IL15Rα combined with off–the–shelf EGFR–CAR NK cells targets glioblastoma. Cancer Res (2021) 81(13):3635–48. doi: 10.1158/0008-5472.CAN-21-0035
74. Daher M, Basar R, Gokdemir E, Baran N, Uprety N, Nunez Cortes AK, et al. Targeting a cytokine checkpoint enhances the fitness of armored cord blood CAR–NK cells. Blood. (2021) 137(5):624–36. doi: 10.1182/blood.2020007748
75. Parrish–Novak J, Dillon SR, Nelson A, Hammond A, Sprecher C, Gross JA, et al. Interleukin 21 and its receptor are involved in NK cell expansion and regulation of lymphocyte function. Nature. (2000) 408(6808):57–63. doi: 10.1038/35040504
76. Li Y, Cong Y, Jia M, He Q, Zhong H, Zhao Y, et al. Targeting IL–21 to tumor–reactive T cells enhances memory T cell responses and anti–PD–1 antibody therapy. Nat Commun (2021) 12(1):1–13. doi: 10.1038/s41467-021-21241-0
77. Zhou T, Damsky W, Weizman O–E, McGeary MK, Hartmann KP, Rosen CE, et al. IL–18BP is a secreted immune checkpoint and barrier to IL–18 immunotherapy. Nature. (2020) 583(7817):609–14. doi: 10.1038/s41586-020-2422-6
78. Vendrame E, Fukuyama J, Strauss–Albee DM, Holmes S, Blish CA. Mass cytometry analytical approaches reveal cytokine–induced changes in natural killer cells. Cytom Part B Clin Cytom. (2017) 92(1):57–67. doi: 10.1002/cyto.b.21500
79. Leong JW, Chase JM, Romee R, Schneider SE, Sullivan RP, Cooper MA, et al. Preactivation with IL–12, IL–15 and IL–18 induces CD25 and a functional high–affinity IL–2 receptor on human cytokine–induced memory–like natural killer cells. Biol Blood Marrow Transplant. (2014) 20(4):463–73. doi: 10.1016/j.bbmt.2014.01.006
80. Nandagopal N, Ali AK, Komal AK, Lee S–H. The critical role of IL–15–PI3K–mTOR pathway in natural killer cell effector functions. Front Immunol (2014) 5:187. doi: 10.3389/fimmu.2014.00187
81. Terrén I, Orrantia A, Astarloa–Pando G, Amarilla–Irusta A, Zenarruzabeitia O, Borrego F. Cytokine–induced memory–like NK cells: From the basics to clinical applications. Front Immunol (2022) 13:884648. doi: 10.3389/fimmu.2022.884648
82. Paul S, Lal G. The molecular mechanism of natural killer cells function and its importance in cancer immunotherapy. Front Immunol (2017) 8:1124. doi: 10.3389/fimmu.2017.01124
83. Topham NJ, Hewitt EW. Natural killer cell cytotoxicity: how do they pull the trigger? Immunology (2009) 128(1):7–15. doi: 10.1111/j.1365-2567.2009.03123.x
84. de Saint Basile G, Ménasché G, Fischer A. Molecular mechanisms of biogenesis and exocytosis of cytotoxic granules. Nat Rev Immunol (2010) 10(8):568–79. doi: 10.1038/nri2803
85. Sonar S, Lal G. Role of tumor necrosis factor superfamily in neuroinflammation and autoimmunity. Front Immunol (2015) 6:364. doi: 10.3389/fimmu.2015.00364
86. Cullen SP, Martin SJ. Mechanisms of granule–dependent killing. Cell Death Differ (2008) 15(2):251–62. doi: 10.1038/sj.cdd.4402244
87. Mace EM, Orange JS. Lytic immune synapse function requires filamentous actin deconstruction by coronin 1A. Proc Natl Acad Sci (2014) 111(18):6708–13. doi: 10.1073/pnas.1314975111
88. Kim H, Khanna V, Kucaba TA, Zhang W, Sehgal D, Ferguson DM, et al. TLR7/8 agonist–loaded nanoparticles augment NK cell–mediated antibody–based cancer immunotherapy. Mol Pharm (2020) 17(6):2109–24. doi: 10.1021/acs.molpharmaceut.0c00271
89. Wilton KM, Overlee BL, Billadeau DD. NKG2D–DAP10 signaling recruits EVL to the cytotoxic synapse to generate f–actin and promote NK cell cytotoxicity. J Cell Sci (2020) ,133(5)::jcs230508. doi: 10.1242/jcs.230508
90. Kim N, Yi E, Kwon SJ, Park HJ, Kwon H–J, Kim HS. Filamin a is required for NK cell cytotoxicity at the expense of cytokine production via synaptic filamentous actin modulation. Front Immunol (2021) 12:792334. doi: 10.3389/fimmu.2021.792334
91. Andzelm MM, Chen X, Krzewski K, Orange JS, Strominger JL. Myosin IIA is required for cytolytic granule exocytosis in human NK cells. J Exp Med (2007) 204(10):2285–91. doi: 10.1084/jem.20071143
92. Stinchcombe JC, Majorovits E, Bossi G, Fuller S, Griffiths GM. Centrosome polarization delivers secretory granules to the immunological synapse. Nature. (2006) 443(7110):462–5. doi: 10.1038/nature05071
93. Ben–Shmuel A, Sabag B, Biber G, Barda–Saad M. The role of the cytoskeleton in regulating the natural killer cell immune response in health and disease: from signaling dynamics to function. Front Cell Dev Biol (2021) 9:609532. doi: 10.3389/fcell.2021.609532
94. Friis S, Riis AH, Erichsen R, Baron JA, Sørensen HT. Low–dose aspirin or nonsteroidal anti–inflammatory drug use and colorectal cancer risk: a population–based, case–control study. Ann Intern Med (2015) 163(5):347–55. doi: 10.7326/M15-0039
95. Terzić J, Grivennikov S, Karin E, Karin M. Inflammation and colon cancer. Gastroenterology. (2010) 138(6):2101–14. doi: 10.1053/j.gastro.2010.01.058
96. Sconocchia G, Eppenberger S, Spagnoli GC, Tornillo L, Droeser R, Caratelli S, et al. NK cells and T cells cooperate during the clinical course of colorectal cancer. Oncoimmunology. (2014) 3(8):e952197. doi: 10.4161/21624011.2014.952197
97. Coppola A, Arriga R, Lauro D, Del Principe MI, Buccisano F, Maurillo L, et al. NK cell inflammation in the clinical outcome of colorectal carcinoma. Front Med (2015) 2:33. doi: 10.3389/fmed.2015.00033
98. Allen F, Rauhe P, Askew D, Tong AA, Nthale J, Eid S, et al. Ccl3 enhances antitumor immune priming in the lymph node via ifnγ with dependency on natural killer cells. Front Immunol (2017) 8:1390. doi: 10.3389/fimmu.2017.01390
99. Zhang L, Zhao Y, Dai Y, Cheng J–N, Gong Z, Feng Y, et al. Immune landscape of colorectal cancer tumor microenvironment from different primary tumor location. Front Immunol (2018) 9:1578. doi: 10.3389/fimmu.2018.01578
100. van Erning FN, Elferink MA, Bos ACRK, Lemmens VEPP. RE: primary tumor location as a prognostic factor in metastatic colorectal cancer. J Natl Cancer Inst (2015) ,107(9)::djv203. doi: 10.1093/jnci/djv203
101. Wen Z, Zhang H, Huang J, Yang J, She X, Shen X, et al. 498P differences in tumor immune microenvironment and clinical outcomes between right and left colon cancer. Ann Oncol (2020) 31:S451. doi: 10.1016/j.annonc.2020.08.608
102. McGilvray RW, Eagle RA, Watson NFS, Al–Attar A, Ball G, Jafferji I, et al. NKG2D ligand expression in human colorectal cancer reveals associations with prognosis and evidence for immunoediting. Clin Cancer Res (2009) 15(22):6993–7002. doi: 10.1158/1078-0432.CCR-09-0991
103. Liu Y, Cheng Y, Xu Y, Wang Z, Du X, Li C, et al. Increased expression of programmed cell death protein 1 on NK cells inhibits NK–cell–mediated anti–tumor function and indicates poor prognosis in digestive cancers. Oncogene. (2017) 36(44):6143–53. doi: 10.1038/onc.2017.209
104. Wang B, Wu S, Zeng H, Liu Z, Dong W, He W, et al. CD103+ tumor infiltrating lymphocytes predict a favorable prognosis in urothelial cell carcinoma of the bladder. J Urol. (2015) 194(2):556–62. doi: 10.1016/j.juro.2015.02.2941
105. Webb JR, Milne K, Watson P, DeLeeuw RJ, Nelson BH. Tumor–infiltrating lymphocytes expressing the tissue resident memory marker CD103 are associated with increased survival in high–grade serous ovarian CancerPrognostic significance of CD103+ TILs in ovarian carcinoma. Clin Cancer Res (2014) 20(2):434–44. doi: 10.1158/1078-0432.CCR-13-1877
106. Aguilar–Cazares D, Meneses–Flores M, Prado–Garcia H, Islas–Vazquez L, Rojo–Leon V, Romero–Garcia S, et al. Relationship of dendritic cell density, HMGB1 expression, and tumor–infiltrating lymphocytes in non–small cell lung carcinomas. Appl Immunohistochem Mol Morphol. (2014) 22(2):105–13. doi: 10.1097/PAI.0b013e3182849808
107. Balatoni T, Mohos A, Papp E, Sebestyén T, Liszkay G, Oláh J, et al. Tumor–infiltrating immune cells as potential biomarkers predicting response to treatment and survival in patients with metastatic melanoma receiving ipilimumab therapy. Cancer Immunol Immunother. (2018) 67(1):141–51. doi: 10.1007/s00262-017-2072-1
108. Nersesian S, Schwartz SL, Grantham SR, MacLean LK, Lee SN, Pugh–Toole M, et al. NK cell infiltration is associated with improved overall survival in solid cancers: A systematic review and meta–analysis. Transl Oncol (2021) 14(1):100930. doi: 10.1016/j.tranon.2020.100930
109. Solinas G, Germano G, Mantovani A, Allavena P. Tumor–associated macrophages (TAM) as major players of the cancer–related inflammation. J Leukoc Biol (2009) 86(5):1065–73. doi: 10.1189/jlb.0609385
110. Zhang R, Qi F, Zhao F, Li G, Shao S, Zhang X, et al. Cancer–associated fibroblasts enhance tumor–associated macrophages enrichment and suppress NK cells function in colorectal cancer. Cell Death Dis (2019) 10(4):1–14. doi: 10.1038/s41419-019-1435-2
111. de Miranda NFCC, van Dinther M, van den Akker BEWM, van Wezel T, ten Dijke P, Morreau H. Transforming growth factor β signaling in colorectal cancer cells with microsatellite instability despite biallelic mutations in TGFBR2. Gastroenterology. (2015) 148(7):1427–37. doi: 10.1053/j.gastro.2015.02.052
112. Otegbeye F, Ojo E, Moreton S, Mackowski N, Lee DA, de Lima M, et al. Inhibiting TGF–beta signaling preserves the function of highly activated, in vitro expanded natural killer cells in AML and colon cancer models. PloS One (2018) 13(1):e0191358. doi: 10.1371/journal.pone.0191358
113. Reid FSW, Egoroff N, Pockney PG, Smith SR. A systematic scoping review on natural killer cell function in colorectal cancer. Cancer Immunol Immunother. (2021) 70(3):597–606. doi: 10.1007/s00262-020-02721-6
114. Zingoni A, Molfetta R, Fionda C, Soriani A, Paolini R, Cippitelli M, et al. NKG2D and its ligands:”one for all, all for one. ” Front Immunol (2018) 9:476. doi: 10.3389/fimmu.2018.00476
115. Sahin IH, Akce M, Alese O, Shaib W, Lesinski GB, El–Rayes B, et al. Immune checkpoint inhibitors for the treatment of MSI–H/MMR–D colorectal cancer and a perspective on resistance mechanisms. Br J Cancer. (2019) 121(10):809–18. doi: 10.1038/s41416-019-0599-y
116. Devillier R, Chrétien A, Pagliardini T, Salem N, Blaise D, Olive D. Mechanisms of NK cell dysfunction in the tumor microenvironment and current clinical approaches to harness NK cell potential for immunotherapy. J Leukoc Biol (2021) 109(6):1071–88. doi: 10.1002/JLB.5MR0920-198RR
117. Krijgsman D, de Vries NL, Skovbo A, Andersen MN, Swets M, Bastiaannet E, et al. Characterization of circulating T–, NK–, and NKT cell subsets in patients with colorectal cancer: the peripheral blood immune cell profile. Cancer Immunol Immunother. (2019) 68(6):1011–24. doi: 10.1007/s00262-019-02343-7
118. Eberhart CE, Coffey RJ, Radhika A, Giardiello FM, Ferrenbach S, Dubois RN. Up–regulation of cyclooxygenase 2 gene expression in human colorectal adenomas and adenocarcinomas. Gastroenterology. (1994) 107(4):1183–8. doi: 10.1016/0016-5085(94)90246-1
119. Wang D, DuBois RN. Prostaglandins and cancer. Gut. (2006) 55(1):115–22. doi: 10.1136/gut.2004.047100
120. Finetti F, Travelli C, Ercoli J, Colombo G, Buoso E, Trabalzini L. Prostaglandin E2 and cancer: Insight into tumor progression and immunity. Biol (Basel). (2020) 9(12):434. doi: 10.3390/biology9120434
121. Wang D, DuBois RN. Role of prostanoids in gastrointestinal cancer. J Clin Invest. (2018) 128(7):2732–42. doi: 10.1172/JCI97953
122. Martinet L, Jean C, Dietrich G, Fournié J–J, Poupot R. PGE2 inhibits natural killer and γδ T cell cytotoxicity triggered by NKR and TCR through a cAMP–mediated PKA type I–dependent signaling. Biochem Pharmacol (2010) 80(6):838–45. doi: 10.1016/j.bcp.2010.05.002
123. Walker W, Rotondo D. Prostaglandin E2 is a potent regulator of interleukin–12–and interleukin–18–Induced natural killer cell interferon–γ synthesis. Immunology. (2004) 111(3):298–305. doi: 10.1111/j.1365-2567.2004.01810.x
124. Böttcher JP, Bonavita E, Chakravarty P, Blees H, Cabeza–Cabrerizo M, Sammicheli S, et al. NK cells stimulate recruitment of cDC1 into the tumor microenvironment promoting cancer immune control. Cell. (2018) 172(5):1022–37. doi: 10.1016/j.cell.2018.01.004
125. Liu W, Fan T, Li M, Zhang G, Guo W, Yang X, et al. Andrographolide potentiates PD–1 blockade immunotherapy by inhibiting COX2–mediated PGE2 release. Int Immunopharmacol. (2020) 81:106206. doi: 10.1016/j.intimp.2020.106206
126. Wang F, Wang M, Yin H, Long Z, Zhu L, Yu H, et al. Association between plasma prostaglandin E2 level and colorectal cancer. Eur J Cancer Prev (2021) 30(1):59–68. doi: 10.1097/CEJ.0000000000000583
127. Yang LV. Tumor microenvironment and metabolism. Int J Mol Sci Multidiscip Digital Publishing Institute (2017) Vol. 18:2729. doi: 10.3390/ijms18122729
128. Justus CR, Dong L, Yang LV. Acidic tumor microenvironment and pH–sensing G protein–coupled receptors. Front Physiol (2013) 4:354. doi: 10.3389/fphys.2013.00354
129. de la Cruz–López KG, Castro–Muñoz LJ, Reyes–Hernández DO, García–Carrancá A, Manzo–Merino J. Lactate in the regulation of tumor microenvironment and therapeutic approaches. Front Oncol (2019) 9:1143. doi: 10.3389/fonc.2019.01143
130. Graziano F, Ruzzo A, Giacomini E, Ricciardi T, Aprile G, Loupakis F, et al. Glycolysis gene expression analysis and selective metabolic advantage in the clinical progression of colorectal cancer. Pharmacogenomics J (2017) 17(3):258–64. doi: 10.1038/tpj.2016.13
131. Harmon C, Robinson MW, Hand F, Almuaili D, Mentor K, Houlihan DD, et al. Lactate–mediated acidification of tumor microenvironment induces apoptosis of liver–resident NK cells in colorectal liver metastasis. Cancer Immunol Res (2019) 7(2):335–46. doi: 10.1158/2326-6066.CIR-18-0481
132. Pilon–Thomas S, Kodumudi KN, El–Kenawi AE, Russell S, Weber AM, Luddy K, et al. Neutralization of tumor acidity improves antitumor responses to immunotherapy. Cancer Res (2016) 76(6):1381–90. doi: 10.1158/0008-5472.CAN-15-1743
133. Labani–Motlagh A, Ashja–Mahdavi M, Loskog A. The tumor microenvironment: a milieu hindering and obstructing antitumor immune responses. Front Immunol (2020) 11:940. doi: 10.3389/fimmu.2020.00940
134. Baghban R, Roshangar L, Jahanban–Esfahlan R, Seidi K, Ebrahimi–Kalan A, Jaymand M, et al. Tumor microenvironment complexity and therapeutic implications at a glance. Cell Commun Signal (2020) 18(1):1–19. doi: 10.1186/s12964-020-0530-4
135. Zhu D, Corral LG, Fleming YW, Stein B. Immunomodulatory drugs revlimid®(lenalidomide) and CC–4047 induce apoptosis of both hematological and solid tumor cells through NK cell activation. Cancer Immunol Immunother. (2008) 57(12):1849–59. doi: 10.1007/s00262-008-0512-7
136. Reddy N, Hernandez–Ilizaliturri FJ, Deeb G, Roth M, Vaughn M, Knight J, et al. Immunomodulatory drugs stimulate natural killer–cell function, alter cytokine production by dendritic cells, and inhibit angiogenesis enhancing the anti–tumour activity of rituximab in vivo. Br J Haematol (2008) 140(1):36–45. doi: 10.1111/j.1365-2141.2007.06841.x
137. D’Souza C, Prince HM, Neeson PJ. Understanding the role of T–cells in the antimyeloma effect of immunomodulatory drugs. Front Immunol (2021) 12:594. doi: 10.3389/fimmu.2021.632399
138. Liu WM, Henry JY, Meyer B, Bartlett JB, Dalgleish AG, Galustian C. Inhibition of metastatic potential in colorectal carcinoma in vivo and in vitro using immunomodulatory drugs (IMiDs). Br J Cancer. (2009) 101(5):803–12. doi: 10.1038/sj.bjc.6605206
139. Holubec L, Polivka J, Safanda M, Karas M, Liska V. The role of cetuximab in the induction of anticancer immune response in colorectal cancer treatment. Anticancer Res (2016) 36(9):4421–6. doi: 10.21873/anticanres.10985
140. Morris VK, Parseghian CM, Escano M, Johnson B, Raghav KPS, Dasari A, et al. Phase I/II trial of encorafenib, cetuximab, and nivolumab in patients with microsatellite stable, BRAF V600E metastatic colorectal cancer. Am Soc Clin Oncol (2022) 40(4_suppl):12–12. doi: 10.1200/JCO.2022.40.4_suppl.012
141. Aparicio J, Virgili Manrique AC, Capdevila J, Muñoz Boza F, Galván P, Richart P, et al. Randomized phase II trial of FOLFIRI–panitumumab compared with FOLFIRI alone in patients with RAS wild–type circulating tumor DNA metastatic colorectal cancer beyond progression to first–line FOLFOX–panitumumab: the BEYOND study (GEMCAD 17–01). Clin Transl Oncol (2022) 24(11):2155–65. doi: 10.1007/s12094-022-02868-x
142. Galustian C, Meyer B, Labarthe M–C, Dredge K, Klaschka D, Henry J, et al. The anti–cancer agents lenalidomide and pomalidomide inhibit the proliferation and function of T regulatory cells. Cancer Immunol Immunother. (2009) 58(7):1033–45. doi: 10.1007/s00262-008-0620-4
143. Chiossone L, Dumas P–Y, Vienne M, Vivier E. Natural killer cells and other innate lymphoid cells in cancer. Nat Rev Immunol (2018) 18(11):671–88. doi: 10.1038/s41577-018-0061-z
144. Moretta A, Bottino C, Vitale M, Pende D, Cantoni C, Mingari MC, et al. Activating receptors and coreceptors involved in human natural killer cell–mediated cytolysis. Annu Rev Immunol (2001) 19(1):197–223. doi: 10.1146/annurev.immunol.19.1.197
145. Long EO. Regulation of immune responses through inhibitory receptors. Annu Rev Immunol (1999) 17(1):875–904. doi: 10.1146/annurev.immunol.17.1.875
146. Cercek A, Lumish M, Sinopoli J, Weiss J, Shia J, Lamendola–Essel M, et al. PD–1 blockade in mismatch repair–deficient, locally advanced rectal cancer. N Engl J Med (2022) 386(25):2363–76. doi: 10.1056/NEJMoa2201445
147. Chiossone L, Vienne M, Kerdiles YM, Vivier E. Natural killer cell immunotherapies against cancer: checkpoint inhibitors and more. In: Seminars in immunology. Elsevier (2017) 31:55–63. doi: 10.1016/j.smim.2017.08.003
148. Poole RM. Pembrolizumab: first global approval. Drugs. (2014) 74(16):1973–81. doi: 10.1007/s40265-014-0314-5
149. Kohlhapp FJ, Broucek JR, Hughes T, Huelsmann EJ, Lusciks J, Zayas JP, et al. NK cells and CD8+ T cells cooperate to improve therapeutic responses in melanoma treated with interleukin–2 (IL–2) and CTLA–4 blockade. J Immunother cancer. (2015) 3(1):1–13. doi: 10.1186/s40425-015-0063-3
150. Overman MJ, Lonardi S, Wong KYM, Lenz H–J, Gelsomino F, Aglietta M, et al. Durable clinical benefit with nivolumab plus ipilimumab in DNA mismatch repair–deficient/microsatellite instability–high metastatic colorectal cancer. J Clin Oncol (2018) 36(8):773–9. doi: 10.1200/JCO.2017.76.9901
151. Segal NH, Naidoo J, Curigliano G, Patel S, Sahebjam S, Papadopoulos KP, et al. First–in–human dose escalation of monalizumab plus durvalumab, with expansion in patients with metastatic microsatellite–stable colorectal cancer. J Clin Oncol (2018) 36(15_suppl):3540. doi: 10.1200/JCO.2018.36.15_suppl.3540
152. Armand P, Lesokhin A, Borrello I, Timmerman J, Gutierrez M, Zhu L, et al. A phase 1b study of dual PD–1 and CTLA–4 or KIR blockade in patients with relapsed/refractory lymphoid malignancies. Leukemia. (2021) 35(3):777–86. doi: 10.1038/s41375-020-0939-1
153. Yarchoan M, Huang C, Zhu Q, Ferguson AK, Durham JN, Anders RA, et al. A phase 2 study of GVAX colon vaccine with cyclophosphamide and pembrolizumab in patients with mismatch repair proficient advanced colorectal cancer. Cancer Med (2020) 9(4):1485–94. doi: 10.1002/cam4.2763
154. Kawazoe A, Kuboki Y, Shinozaki E, Hara H, Nishina T, Komatsu Y, et al. Multicenter phase I/II trial of napabucasin and pembrolizumab in patients with metastatic colorectal cancer (EPOC1503/SCOOP trial). Clin Cancer Res (2020) 26(22):5887–94. doi: 10.1158/1078-0432.CCR-20-1803
155. Overman MJ, McDermott R, Leach JL, Lonardi S, Lenz H–J, Morse MA, et al. Nivolumab in patients with metastatic DNA mismatch repair–deficient or microsatellite instability–high colorectal cancer (CheckMate 142): an open–label, multicentre, phase 2 study. Lancet Oncol (2017) 18(9):1182–91. doi: 10.1016/S1470-2045(17)30422-9
156. Monjazeb AM, Giobbie–Hurder A, Lako A, Thrash EM, Brennick RC, Kao KZ, et al. A randomized trial of combined PD–L1 and CTLA–4 inhibition with targeted low–dose or hypofractionated radiation for patients with metastatic colorectal cancer. Clin Cancer Res (2021) 27(9):2470–80. doi: 10.1158/1078-0432.CCR-20-4632
157. Schöffski P, Tan DSW, Martín M, Ochoa–de–Olza M, Sarantopoulos J, Carvajal RD, et al. Phase I/II study of the LAG–3 inhibitor ieramilimab (LAG525)±anti–PD–1 spartalizumab (PDR001) in patients with advanced malignancies. J Immunother cancer. (2022) 10(2):e003776. doi: 10.1136/jitc-2021-003776
158. Harding JJ, Moreno V, Bang Y–J, Hong MH, Patnaik A, Trigo J, et al. Blocking TIM–3 in treatment–refractory advanced solid tumors: a phase ia/b study of LY3321367 with or without an anti–PD–L1 antibody. Clin Cancer Res (2021) 27(8):2168–78. doi: 10.1158/1078-0432.CCR-20-4405
159. Davar D, Boasberg P, Eroglu Z, Falchook G, Gainor J, Hamilton E. A phase 1 study of TSR–022, an anti–TIM–3 monoclonal antibody, in combination with TSR–042 (anti–PD–1) in patients with colorectal cancer and post–PD–1 NSCLC and melanoma. J Immunother Cancer. (2018) 6(Suppl 1)::115O21.
160. Gomes de Morais AL, Cerdá S, de Miguel M. New checkpoint inhibitors on the road: Targeting TIM–3 in solid tumors. Curr Oncol Rep (2022) 24(5):651–8. doi: 10.1007/s11912-022-01218-y
161. Thibaudin M, Limagne E, Hampe L, Ballot E, Truntzer C, Ghiringhelli F. Targeting PD–L1 and TIGIT could restore intratumoral CD8 T cell function in human colorectal cancer. Cancer Immunol Immunother (2022) 71(10):2549–63. doi: 10.1007/s00262-022-03182-9
162. Bolm L, Petruch N, Sivakumar S, Annels NE, Frampton AE. Gene of the month: T–cell immunoreceptor with immunoglobulin and ITIM domains (TIGIT). J Clin Pathol (2022) 75(4):217–21. doi: 10.1136/jclinpath-2021-207789
163. Ochoa MC, Minute L, Rodriguez I, Garasa S, Perez–Ruiz E, Inogés S, et al. Antibody–dependent cell cytotoxicity: immunotherapy strategies enhancing effector NK cells. Immunol Cell Biol (2017) 95(4):347–55. doi: 10.1038/icb.2017.6
164. Wang W, Erbe AK, Hank JA, Morris ZS, Sondel PM. NK cell–mediated antibody–dependent cellular cytotoxicity in cancer immunotherapy. Front Immunol (2015) 6:368. doi: 10.3389/fimmu.2015.00368
165. Schroder K, Hertzog PJ, Ravasi T, Hume DA. Interferon–γ: an overview of signals, mechanisms and functions. J Leukoc Biol (2004) 75(2):163–89. doi: 10.1189/jlb.0603252
166. Smyth MJ, Cretney E, Kelly JM, Westwood JA, Street SEA, Yagita H, et al. Activation of NK cell cytotoxicity. Mol Immunol (2005) 42(4):501–10. doi: 10.1016/j.molimm.2004.07.034
167. Jochems C, Hodge JW, Fantini M, Fujii R, Maurice YM. An NK cell line (haNK) expressing high levels of granzyme and engineered to express the high affinity CD16 allele. Oncotarget. (2016) 7(52):86359. doi: 10.18632/oncotarget.13411
168. Malik B, Ghatol A. Understanding how monoclonal antibodies work. In: StatPearls. StatPearls Publishing (2021).
169. Mousa L, Salem ME, Mikhail S. Biomarkers of angiogenesis in colorectal cancer: Supplementary issue: Biomarkers for colon cancer. biomark Cancer (2015) 7:BIC–S25250. doi: 10.4137/BIC.S25250
170. Françoso A, Simioni PU. Immunotherapy for the treatment of colorectal tumors: focus on approved and in–clinical–trial monoclonal antibodies. Drug Des Devel Ther (2017) 11:177. doi: 10.2147/DDDT.S119036
171. Mangiapane LR, Nicotra A, Turdo A, Gaggianesi M, Bianca P, Di Franco S, et al. PI3K–driven HER2 expression is a potential therapeutic target in colorectal cancer stem cells. Gut. (2022) 71(1):119–28. doi: 10.1136/gutjnl-2020-323553
172. Chen S, Li X, Chen R, Yin M, Zheng Q. Cetuximab intensifies the ADCC activity of adoptive NK cells in a nude mouse colorectal cancer xenograft model. Oncol Lett (2016) 12(3):1868–76. doi: 10.3892/ol.2016.4835
173. Kubach J, Hubo M, Amendt C, Stroh C, Jonuleit H. IgG1 anti–epidermal growth factor receptor antibodies induce CD8–dependent antitumor activity. Int J Cancer. (2015) 136(4):821–30. doi: 10.1002/ijc.29037
174. Liang Y–H, Liang J–T, Lin B–R, Huang J, Hung J–S, Lai S–L, et al. Ramucirumab plus triplet chemotherapy as an alternative salvage treatment for patients with metastatic colorectal cancer. J Formos Med Assoc (2022) 121(10):2057–64. doi: 10.1016/j.jfma.2022.02.019
175. Sobrero A, Latini L, Barone C, Capuzzo F, Banzi M, Nasroulah F, et al. Review of RAISE, a randomized, double–blind, multicenter phase III study of irinotecan, folinic acid, and 5–fluorouracil (FOLFIRI) plus ramucirumab (RAM) or placebo (PBO) in patients (pts) with metastatic colorectal carcinoma (mCRC) progressive during or. Ann Oncol (2015) 26:vi37. doi: 10.1200/jco.2015.33.3_suppl.512
176. Anderson AC, Joller N, Kuchroo VK. Lag–3, Tim–3, and TIGIT: co–inhibitory receptors with specialized functions in immune regulation. Immunity. (2016) 44(5):989–1004. doi: 10.1016/j.immuni.2016.05.001
177. Liu L, Lam C–YK, Long V, Widjaja L, Yang Y, Shah K, et al. Tumor–antigen expression–dependent activation of the CD137 costimulatory pathway by bispecific DART® proteins. Cancer Res (2017) 77(13_Supplement):3642.
178. Deak LLC, Seeber S, Perro M, Weber P, Lauener L, Chen S, et al. RG7769 (PD1–TIM3), a novel heterodimeric avidity–driven T cell specific PD–1/TIM–3 bispecific antibody lacking fc–mediated effector functions for dual checkpoint inhibition to reactivate dysfunctional T cells. Cancer Res (2020) 80(16_Supplement):2270. doi: 10.1158/1538-7445.AM2020-2270
179. Fayette J, Wirth L, Oprean C, Udrea A, Jimeno A, Rischin D, et al. Randomized phase II study of duligotuzumab (MEHD7945A) vs. cetuximab in squamous cell carcinoma of the head and neck (MEHGAN study). Front Oncol (2016) 6:232. doi: 10.3389/fonc.2016.00232
180. Vallera DA, Zhang B, Gleason MK, Oh S, Weiner LM, Kaufman DS, et al. Heterodimeric bispecific single–chain variable–fragment antibodies against EpCAM and CD16 induce effective antibody–dependent cellular cytotoxicity against human carcinoma cells. Cancer Biother Radiopharm. (2013) 28(4):274–82. doi: 10.1089/cbr.2012.1329
181. Schmohl JU, Felices M, Taras E, Miller JS, Vallera DA. Enhanced ADCC and NK cell activation of an anticarcinoma bispecific antibody by genetic insertion of a modified IL–15 cross–linker. Mol Ther (2016) 24(7):1312–22. doi: 10.1038/mt.2016.88
182. Abdolahi S, Ghazvinian Z, Muhammadnejad S, Saleh M, Asadzadeh Aghdaei H, Baghaei K. Patient–derived xenograft (PDX) models, applications and challenges in cancer research. J Transl Med (2022) 20(1):1–15. doi: 10.1186/s12967-022-03405-8
183. Glorius P, Baerenwaldt A, Kellner C, Staudinger M, Dechant M, Stauch M, et al. The novel tribody [(CD20) 2xCD16] efficiently triggers effector cell–mediated lysis of malignant b cells. Leukemia. (2013) 27(1):190–201. doi: 10.1038/leu.2012.150
184. Li J, Huang L, Zhao H, Yan Y, Lu J. The role of interleukins in colorectal cancer. Int J Biol Sci (2020) 16(13):2323. doi: 10.7150/ijbs.46651
185. Friedberg JW, Neuberg D, Gribben JG, Fisher DC, Canning C, Koval M, et al. Combination immunotherapy with rituximab and interleukin 2 in patients with relapsed or refractory follicular non–hodgkin’s lymphoma. Br J Haematol (2002) 117(4):828–34. doi: 10.1046/j.1365-2141.2002.03535.x
186. Rafieenia F, Nikkhah E, Nourmohammadi F, Hosseini S, Abdollahi A, Sharifi N, et al. Allogeneic tumor cell line–based vaccines: A good alternative to autologous and cancer stem cell vaccines in colorectal cancer. Iran J Basic Med Sci (2021) 24(9):1231. doi: 10.22038/IJBMS.2021.56732.12671
187. Pinette A, McMichael E, Courtney NB, Duggan M, Benner BN, Choueiry F, et al. An IL–Based superagonist ALT–803 enhances the NK cell response to cetuximab–treated squamous cell carcinoma of the head and neck. Cancer Immunol Immunother. (2019) 68(8):1379–89. doi: 10.1007/s00262-019-02372-2
188. Rosario M, Liu B, Kong L, Collins LI, Schneider SE, Chen X, et al. The IL–15–based ALT–803 complex enhances FcγRIIIa–triggered NK cell responses and in vivo clearance of b cell lymphomas. Clin Cancer Res (2016) 22(3):596–608. doi: 10.1158/1078-0432.CCR-15-1419
189. Abdolahi S, Ghazvinian Z, Muhammadnejad S, Ahmadvand M, Aghdaei HA, Ebrahimi–Barough S, et al. Adaptive NK cell therapy modulated by anti–PD–1 antibody in gastric cancer model. Front Pharmacol (2021) 12. doi: 10.3389/fphar.2021.733075
190. Ghazvinian Z, Abdolahi S, Ahmadvand M, Emami AH, Muhammadnejad S, Aghdaei HA, et al. Chemo–immune cell therapy by intratumoral injection of adoptive NK cells with capecitabine in gastric cancer xenograft model. BioImpacts (2022). doi: 10.34172/bi.2022.26386
191. Shahnazari M, Samadi P, Pourjafar M, Jalali A. Therapeutic vaccines for colorectal cancer: the progress and future prospect. Int Immunopharmacol. (2020) 88:106944. doi: 10.1016/j.intimp.2020.106944
192. Keenan BP, Jaffee EM. Whole cell vaccines–past progress and future strategies. in: Seminars in oncology. . Elsevier (2012) p:276–86. doi: 10.1053/j.seminoncol.2012.02.007
193. Liebrich W, Schlag P, Manasterski M, Lehner B, Stöhr M, Möller P, et al. In vitro and clinical characterisation of a Newcastle disease virus–modified autologous tumour cell vaccine for treatment of colorectal cancer patients. Eur J Cancer Clin Oncol (1991) 27(6):703–10. doi: 10.1016/0277-5379(91)90170-I
194. Jocham D, Richter A, Hoffmann L, Iwig K, Fahlenkamp D, Zakrzewski G, et al. Adjuvant autologous renal tumour cell vaccine and risk of tumour progression in patients with renal–cell carcinoma after radical nephrectomy: phase III, randomised controlled trial. Lancet. (2004) 363(9409):594–9. doi: 10.1016/S0140-6736(04)15590-6
195. Guo M, Luo B, Pan M, Li M, Xu H, Zhao F, et al. Colorectal cancer stem cell vaccine with high expression of MUC1 serves as a novel prophylactic vaccine for colorectal cancer. Int Immunopharmacol. (2020) 88:106850. doi: 10.1016/j.intimp.2020.106850
196. Qin J, Kunda NM, Qiao G, Tulla K, Prabhakar BS, Maker AV. Vaccination with mitoxantrone–treated primary colon cancer cells enhances tumor–infiltrating lymphocytes and clinical responses in colorectal liver metastases. J Surg Res (2019) 233:57–64. doi: 10.1016/j.jss.2018.07.068
197. Morse MA, Nair SK, Mosca PJ, Hobeika AC, Clay TM, Deng Y, et al. Immunotherapy with autologous, human dendritic cells transfected with carcinoembryonic antigen mRNA. Cancer Invest. (2003) 21(3):341–9. doi: 10.1081/CNV-120018224
198. Sakakibara M, Kanto T, Hayakawa M, Kuroda S, Miyatake H, Itose I, et al. Comprehensive immunological analyses of colorectal cancer patients in the phase I/II study of quickly matured dendritic cell vaccine pulsed with carcinoembryonic antigen peptide. Cancer Immunol Immunother. (2011) 60(11):1565–75. doi: 10.1007/s00262-011-1051-1
199. Hunyadi J, András C, Szabó I, Szántó J, Szluha K, Sipka S, et al. Autologous dendritic cell based adoptive immunotherapy of patients with colorectal cancer–a phase I–II study. Pathol Oncol Res (2014) 20(2):357–65. doi: 10.1007/s12253-013-9704-3
200. Furugaki K, Cui L, Kunisawa Y, Osada K, Shinkai K, Tanaka M, et al. Intraperitoneal administration of a tumor–associated antigen SART3, CD40L, and GM–CSF gene–loaded polyplex micelle elicits a vaccine effect in mouse tumor models. PloS One (2014) 9(7):e101854. doi: 10.1371/journal.pone.0101854
201. Fujihara A, Kurooka M, Miki T, Kaneda Y. Intratumoral injection of inactivated Sendai virus particles elicits strong antitumor activity by enhancing local CXCL10 expression and systemic NK cell activation. Cancer Immunol Immunother. (2008) 57(1):73–84. doi: 10.1007/s00262-007-0351-y
202. Redman JM, Tsai Y–T, Weinberg BA, Donahue RN, Gandhy S, Gatti–Mays ME, et al. A randomized phase II trial of mFOLFOX6+ bevacizumab alone or with AdCEA vaccine+ avelumab immunotherapy for untreated metastatic colorectal cancer. Oncologist. (2022) 27(3):198–209. doi: 10.1093/oncolo/oyab046
203. Thomas S, Prendergast GC. Cancer vaccines: a brief overview. Vaccine Des (2016) 1403:755–61. doi: 10.1007/978-1-4939-3387-7_43
204. Brentjens RJ, Davila ML, Riviere I, Park J, Wang X, Cowell LG, et al. CD19–targeted T cells rapidly induce molecular remissions in adults with chemotherapy–refractory acute lymphoblastic leukemia. Sci Transl Med (2013) 5(177):177ra38–177ra38. doi: 10.1126/scitranslmed.3005930
205. Grupp SA, Kalos M, Barrett D, Aplenc R, Porter DL, Rheingold SR, et al. Chimeric antigen receptor–modified T cells for acute lymphoid leukemia. N Engl J Med (2013) 368(16):1509–18. doi: 10.1056/NEJMoa1215134
206. Moretti C. CAR–T cell therapy may have ‘Big future’in Relapsed/Refractory multiple. Myeloma (2022).
207. Neelapu SS, Locke FL, Bartlett NL, Lekakis LJ, Miklos DB, Jacobson CA, et al. Axicabtagene ciloleucel CAR T–cell therapy in refractory large b–cell lymphoma. N Engl J Med (2017) 377(26):2531–44. doi: 10.1056/NEJMoa1707447
208. Rezvani K. Adoptive cell therapy using engineered natural killer cells. Bone Marrow Transplant. (2019) 54(2):785–8. doi: 10.1038/s41409-019-0601-6
209. Goulmy E. Human minor histocompatibility antigens: new concepts for marrow transplantation and adoptive immunotherapy. Immunol Rev (1997) 157(1):125–40. doi: 10.1111/j.1600-065X.1997.tb00978.x
210. Olson JA, Leveson–Gower DB, Gill S, Baker J, Beilhack A, Negrin RS. NK cells mediate reduction of GVHD by inhibiting activated, alloreactive T cells while retaining GVT effects. Blood J Am Soc Hematol (2010) 115(21):4293–301. doi: 10.1182/blood-2009-05-222190
211. Miller JS, Soignier Y, Panoskaltsis–Mortari A, McNearney SA, Yun GH, Fautsch SK, et al. Successful adoptive transfer and in vivo expansion of human haploidentical NK cells in patients with cancer. Blood. (2005) 105(8):3051–7. doi: 10.1182/blood-2004-07-2974
212. Curti A, Ruggeri L, D’Addio A, Bontadini A, Dan E, Motta MR, et al. Successful transfer of alloreactive haploidentical KIR ligand–mismatched natural killer cells after infusion in elderly high risk acute myeloid leukemia patients. Blood J Am Soc Hematol (2011) 118(12):3273–9. doi: 10.1182/blood-2011-01-329508
213. Sekine T, Marin D, Cao K, Li L, Mehta P, Shaim H, et al. Specific combinations of donor and recipient KIR–HLA genotypes predict for large differences in outcome after cord blood transplantation. Blood J Am Soc Hematol (2016) 128(2):297–312. doi: 10.1182/blood-2016-03-706317
214. Liu E, Marin D, Banerjee P, Macapinlac HA, Thompson P, Basar R, et al. Use of CAR–transduced natural killer cells in CD19–positive lymphoid tumors. N Engl J Med (2020) 382(6):545–53. doi: 10.1056/NEJMoa1910607
215. Zhang Q, Zhang H, Ding J, Liu H, Li H, Li H, et al. Combination therapy with EpCAM–CAR–NK–92 cells and regorafenib against human colorectal cancer models. J Immunol Res (2019) 2019:2070562. doi: 10.1155/2018/4263520
216. Shiozawa M, Chang C–H, Huang Y–C, Chen Y–C, Chi M–S, Hao H–C, et al. Pharmacologically upregulated carcinoembryonic antigen–expression enhances the cytolytic activity of genetically–modified chimeric antigen receptor NK–92MI against colorectal cancer cells. BMC Immunol (2018) 19(1):1–13. doi: 10.1186/s12865-018-0262-z
217. Xiao L, Cen D, Gan H, Sun Y, Huang N, Xiong H, et al. Adoptive transfer of NKG2D CAR mRNA-engineered natural killer cells in colorectal cancer patients. Mol Ther (2019) 27(6):1114–25. doi: 10.1016/j.ymthe.2019.03.011
218. Schnalzger TE, de Groot MHP, Zhang C, Mosa MH, Michels BE, Röder J, et al. 3D model for CAR–mediated cytotoxicity using patient–derived colorectal cancer organoids. EMBO J (2019) 38(12):e100928. doi: 10.15252/embj.2018100928
219. Gao L, Yang L, Zhang S, Ge Z, Su M, Shi Y, et al. Engineering NK–92 cell by upregulating CXCR2 and IL–2 via CRISPR–Cas9 improves its antitumor effects as cellular immunotherapy for human colon cancer. J Interf Cytokine Res (2021) 41(12):450–60. doi: 10.1089/jir.2021.0078
220. Chen Y–C, Fang W–L, Wang R–F, Liu C–A, Yang M–H, Lo S–S, et al. Clinicopathological variation of Lauren classification in gastric cancer. Pathol Oncol Res (2016) 22(1):197–202. doi: 10.1007/s12253-015-9996-6
221. Melero I, Rouzaut A, Motz GT, Coukos G. T–Cell and NK–cell infiltration into solid tumors: a key limiting factor for efficacious cancer immunotherapy. Cancer Discovery (2014) 4(5):522–6. doi: 10.1158/2159-8290.CD-13-0985
222. Krause SW, Gastpar R, Andreesen R, Gross C, Ullrich H, Thonigs G, et al. Treatment of colon and lung cancer patients with ex vivo heat shock protein 70–peptide–activated, autologous natural killer cells: a clinical phase I trial. Clin Cancer Res (2004) 10(11):3699–707. doi: 10.1158/1078-0432.CCR-03-0683
223. Barkholt L, Alici E, Conrad R, Sutlu T, Gilljam M, Stellan B, et al. Safety analysis of ex vivo–expanded NK and NK–like T cells administered to cancer patients: a phase I clinical study. Immunotherapy. (2009) 1(5):753–64. doi: 10.2217/imt.09.47
224. Liem NT, Van Phong N, Kien NT, Anh BV, Huyen TL, Thao CT, et al. Phase I clinical trial using autologous ex vivo expanded NK cells and cytotoxic T lymphocytes for cancer treatment in Vietnam. Int J Mol Sci (2019) 20(13):3166. doi: 10.3390/ijms20133166
225. Sakamoto N, Ishikawa T, Kokura S, Okayama T, Oka K, Ideno M, et al. Phase I clinical trial of autologous NK cell therapy using novel expansion method in patients with advanced digestive cancer. J Transl Med (2015) 13(1):1–13. doi: 10.1186/s12967-015-0632-8
226. Ishikawa T, Okayama T, Sakamoto N, Ideno M, Oka K, Enoki T, et al. Phase I clinical trial of adoptive transfer of expanded natural killer cells in combination with I g G 1 antibody in patients with gastric or colorectal cancer. Int J Cancer. (2018) 142(12):2599–609. doi: 10.1002/ijc.31285
227. Adotevi O, Godet Y, Galaine J, Lakkis Z, Idirene I, Certoux JM, et al. In situ delivery of allogeneic natural killer cell (NK) combined with cetuximab in liver metastases of gastrointestinal carcinoma: A phase I clinical trial. Oncoimmunology. (2018) 7(5):e1424673. doi: 10.1080/2162402X.2018.1424673
228. Rubnitz JE, Inaba H, Ribeiro RC, Pounds S, Rooney B, Bell T, et al. NKAML: a pilot study to determine the safety and feasibility of haploidentical natural killer cell transplantation in childhood acute myeloid leukemia. J Clin Oncol (2010) 28(6):955. doi: 10.1200/JCO.2009.24.4590
229. Ruggeri L, Capanni M, Urbani E, Perruccio K, Shlomchik WD, Tosti A, et al. Effectiveness of donor natural killer cell alloreactivity in mismatched hematopoietic transplants. Sci (80– ). (2002) 295(5562):2097–100. doi: 10.1126/science.1068440
230. Rezvani K, Rouce R, Liu E, Shpall E. Engineering natural killer cells for cancer immunotherapy. Mol Ther (2017) 25(8):1769–81. doi: 10.1016/j.ymthe.2017.06.012
231. Rezvani K, Rouce RH. The application of natural killer cell immunotherapy for the treatment of cancer. Front Immunol (2015) 6:578. doi: 10.3389/fimmu.2015.00578
Keywords: natural killer cell, colorectal cancer, CAR-NK cell, check point inhibitor, cancer vaccine, cancer immune-cell therapy, adoptive cell immunotherapy
Citation: Ghazvinian Z, Abdolahi S, Tokhanbigli S, Tarzemani S, Piccin A, Reza Zali M, Verdi J and Baghaei K (2023) Contribution of natural killer cells in innate immunity against colorectal cancer. Front. Oncol. 12:1077053. doi: 10.3389/fonc.2022.1077053
Received: 22 October 2022; Accepted: 13 December 2022;
Published: 04 January 2023.
Edited by:
Zhiyuan Zhang, Fudan University, ChinaReviewed by:
Dainius Characiejus, Vilnius University, LithuaniaDaniela Montagna, Laboratory of Immunology and Transplantation (IRCCS), Italy
Copyright © 2023 Ghazvinian, Abdolahi, Tokhanbigli, Tarzemani, Piccin, Reza Zali, Verdi and Baghaei. This is an open-access article distributed under the terms of the Creative Commons Attribution License (CC BY). The use, distribution or reproduction in other forums is permitted, provided the original author(s) and the copyright owner(s) are credited and that the original publication in this journal is cited, in accordance with accepted academic practice. No use, distribution or reproduction is permitted which does not comply with these terms.
*Correspondence: Kaveh Baghaei, a2F2ZWhiYWdoYWlAZ21haWwuY29t
†These authors have contributed equally to this work and share first authorship