- 1Department of Cellular Biology and Anatomy, Louisiana State University Health Sciences Center, Shreveport, LA, United States
- 2Feist-Weiller Cancer Center, Louisiana State University Health Shreveport, Shreveport, LA, United States
Targeted therapies have significantly improved survival rates and quality of life for many cancer patients. However, on- and off-target side toxicities in normal tissues, and precocious activation of the immune response remain significant issues that limit the efficacy of molecular targeted agents. Extracellular vesicles (EVs) hold great promise as the mediators of next-generation therapeutic payloads. Derived from cellular membranes, EVs can be engineered to carry specific therapeutic agents in a targeted manner to tumor cells. This review highlights the progress in our understanding of basic EV biology, and discusses how EVs are being chemically and genetically modified for use in clinical and preclinical studies.
Introduction
Budding from the membranes of prokaryotic and eukaryotic cells, extracellular vesicles (EVs) act as intercellular messengers (1). They carry information in the form of proteins, lipids, RNA, and DNA to cells in the local environment and at distant sites, and retain many of the features of their parental cell of origin. Such information imprinting means that EVs can regulate processes during normal homeostasis; conversely, deregulation of EV function can translate to pathology in EV-targeted cells. This is exemplified by EV-dependent perturbations of the immune response, organ development, reproduction, and vasculogenesis (2, 3). EVs can also modulate the tumor microenvironment, leading to either enhanced or reduced tumorigenesis (4, 5). By understanding the fundamental biological processes that govern EV behavior, we will be able to exploit EVs to use them as conduits of anticancer therapeutics. By definition EVs are biocompatible, and researchers in the field are now fine-tuning EVs via chemical and genetic strategies to transform them into drug delivery systems (6). The pros and cons of EVs in the context of cancer therapy is the main focus of this review. We draw upon examples from basic and translational research to highlight the advantages and limitations of EV use in the preclinical and clinical settings. Our aim is to provide a conceptual framework to spur on novel research approaches in the EV field, with the ultimate aim of improving survival rates in cancer patients.
Biochemical properties of EVs
EV secretion has been observed in virtually all kingdoms of life. EV biogenesis and cargo loading are tightly linked processes, and these steps are strictly regulated to ensure that the subsequent interaction with target cells produces the desired biological effects (7). Cargos as diverse as proteins, lipids, nucleic acids, cytokines and even organelles provide an information-rich payload that can be exploited to achieve downstream cellular effects (3). Indeed, the ExoCarta database contains 9,690 proteins, 3,300 mRNAs, and 1,010 different types of lipids that have been identified in exosomes, which underscores the complexity and heterogeneity of these nanostructures (8). Specific examples of select cargos are discussed below.
Small non-coding RNAs have been found within EVs, and may control molecular events in recipient cells by regulating the activity of target mRNAs, for example (9). These RNA cargos could be exploited as potential non-invasive biomarkers for multiple disorders such as those that affect the immune system (10). EV has been isolated from most cell types and body fluids, including saliva. Saliva diagnostics is a rapidly expanding field and the non-invasive saliva testing could greatly facilitate the early diagnosis of many diseases, including cancers (11, 12).
The lipid composition of EVs is very similar to that of their parental cells; this provides an ideal physical barrier to protect internal cargo from premature degradation. In addition to providing EV integrity, the large amounts of sphingomyelin and cholesterol prevent EV cargos from degradation by both nucleases and proteases (13). Such lipids also act as physiological buffers that ensure the pH and osmotic balance within EVs is maintained (14, 15). These properties could be exploited to effectively increase the amount of drug that is delivered to a particular tumor.
A suite of fatty acids in EVs provide the building blocks for the generation of signaling intermediates that regulate multiple physiological processes (16). Consistent with the coordinated regulation of EV biogenesis and function, the EVs also contain the enzymes required for conversion of fatty acids into bioactive products such as esterified fatty acids and eicosanoids (17). These features underlie the ability of EVs to regulate inflammatory processes involved in chronic tissue remodeling, cancer, asthma, rheumatoid arthritis and autoimmune disorders (18).
Strategies to functionalize EVs
Despite their advantageous small size and ability to cross the blood brain barrier, natural EVs require further optimization before they can be used for drug delivery. Major limitations include the lack of target cell specificity and an inability to store sufficient payload quantities. Great strides have been made with regard to improving the loading of heterogenous cargo (19). EV membranes can be decorated with a wide variety of molecules that increase target cell specificity and/or reduce the likelihood of EV destruction by host macrophages during immune surveillance (20, 21). Genetic and non-genetic manipulation can lead to a release of modified EVs by introducing targeting moieties such as bispecific antibody, single-domain antibody (nanobody), single-chain variable fragment (scFv), affibody, or targeting peptide that can bind to molecules on target cells (Figure 1).
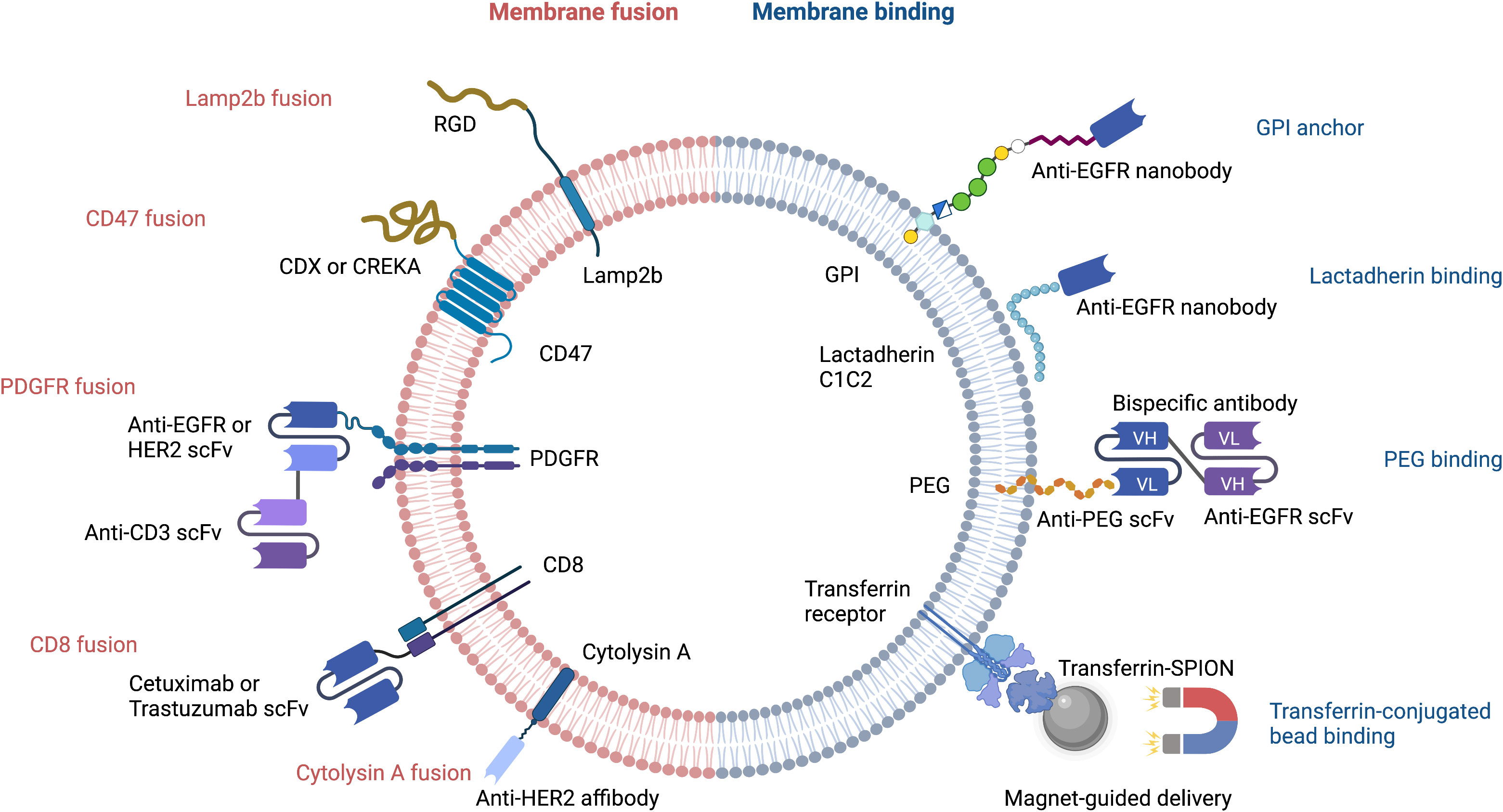
Figure 1 Strategies for targeted delivery of EVs. RGD, Arg-Gly-Asp peptide; CDX, d-peptide ligand of the nicotine acetylcholine receptor; CREKA, pentapeptide Cys-Arg-Glu-Lys-Ala; EGFR, epidermal growth factor receptor; HER2, human epidermal growth factor receptor 2; scFv, single-chain variable fragment; PDGFR, platelet-derived growth factor receptor; GPI, glycosylphosphatidylinositol; PEG, polyethylene glycol; Transferrin-SPION, transferrin-conjugated superparamagnetic iron oxide nanoparticle.
Genetic manipulation of parental cells
Cellular nanoporation (CNP) is the method of choice for delivery of nucleic acids, including large mRNAs, to EVs (19). The mRNAs may encode tumor suppressor genes that comprise a therapeutic payload, or may encode fusion proteins that have a tumor cell-targeting domain and a bioactive domain. Portions of naturally occurring EV membrane proteins are often used in these fusion constructs; the N-terminus of Lamp2b and CD47 are two common examples. Peptides containing the Arg-Gly-Asp (RGD) integrin recognition motif have been fused to Lamp2b to increase the tumor homing capability of EVs (Figure 1). This has been used successfully to deliver the chemotherapeutic, doxorubicin, to tumor cells (22). In a similar approach, CDX and CREKA, two other tumor-targeting peptides, have been fused to CD47 to facilitate delivery of mRNA encoding the tumor suppressor, PTEN, to glioblastoma cells (19).
The single-chain transmembrane glycoprotein PDGFR has been exploited for breast cancer immunotherapy by Cheng et al., who designed nanoscale controllers termed synthetic multivalent antibody retargeted exosomes (SMART-Ex) (23). Using the PDGFR transmembrane domain as an anchor, single-chain variable fragments (scFv) that recognize either CD3, EGFR, or HER2 have been introduced to EV membranes (23, 24) (Figure 1). The CD8 transmembrane region has also been used to generate chimeric antigen receptor (CAR)-expressing EVs that target either EGFR or HER2; the CAR-EVs promoted tumor regression and did not elicit significant toxicity (25).
Interestingly, EV-type structures from non-mammalian organisms are also being evaluated for their potential as drug carriers. A case in point are bacterial outer membrane vesicles that have been engineered to express a HER2-specific antibody fused to the transmembrane region of the bacteria pore-forming protein, cytolysin A (26).
Modification of EV membranes
The therapeutic efficacy of EVs can be increased by bioconjugation of nanobodies or bispecific antibodies that bind to specific molecules and receptors on target cells. The ExoMAb approach exemplifies this strategy of engineering EVs to express non-natural antigens (27). Briefly, lactadherin contains a C1C2 domain which binds phosphatidylserine moieties in EV membranes. This provides the basis for decorating the EV membrane with exogenous proteins that are fused to C1C2 domain. This has been used to express tumor antigens at high-levels on EV membranes surface antigens, which could be exploited to generate tumor-specific antibodies. Anti-EGFR nanobodies have also been conjugated to glycosylphosphatidylinositol (GPI) anchor signal peptides or to the lactadherin C1C2 domain. This approach led to a remarkable improvement in selective cargo delivery to EGFR-positive cells (Figure 1) (28, 29).
Circulating EVs play a continuous ‘cat and mouse’ game with patrolling macrophages, which can phagocytose EVs upon recognition. This clearly reduces the effective half-life of EVs in the body. CD47 (mentioned above) can again be exploited here: by binding to signal regulatory protein alpha (SIRPα) on the surface of macrophages, CD47 sets up a ‘don’t eat me’ signal (21, 30). Thus, CD47-decorated EVs have longer circulatory half-lives and are more likely to reach their target cancer cells to deliver a therapeutic payload (Figure 2). An extremely innovative approach builds on this by combining CD47-loaded red blood cell (RBC) membranes with membranes from cancer cells themselves (31). Since CD47 is overexpressed on the surface of RBC, chimeric EVs derived from RBC-MCF7 hybrid membrane protect EVs from phagocytosis. These hybrid EVs have a longer half-life, but also exploit antigens from the cancer cell membranes to re-target the original cancer cells (31). This has demonstrable therapeutic efficacy, as DOX-loaded hybrid EVs were able to induce tumor regression in a preclinical mouse model.
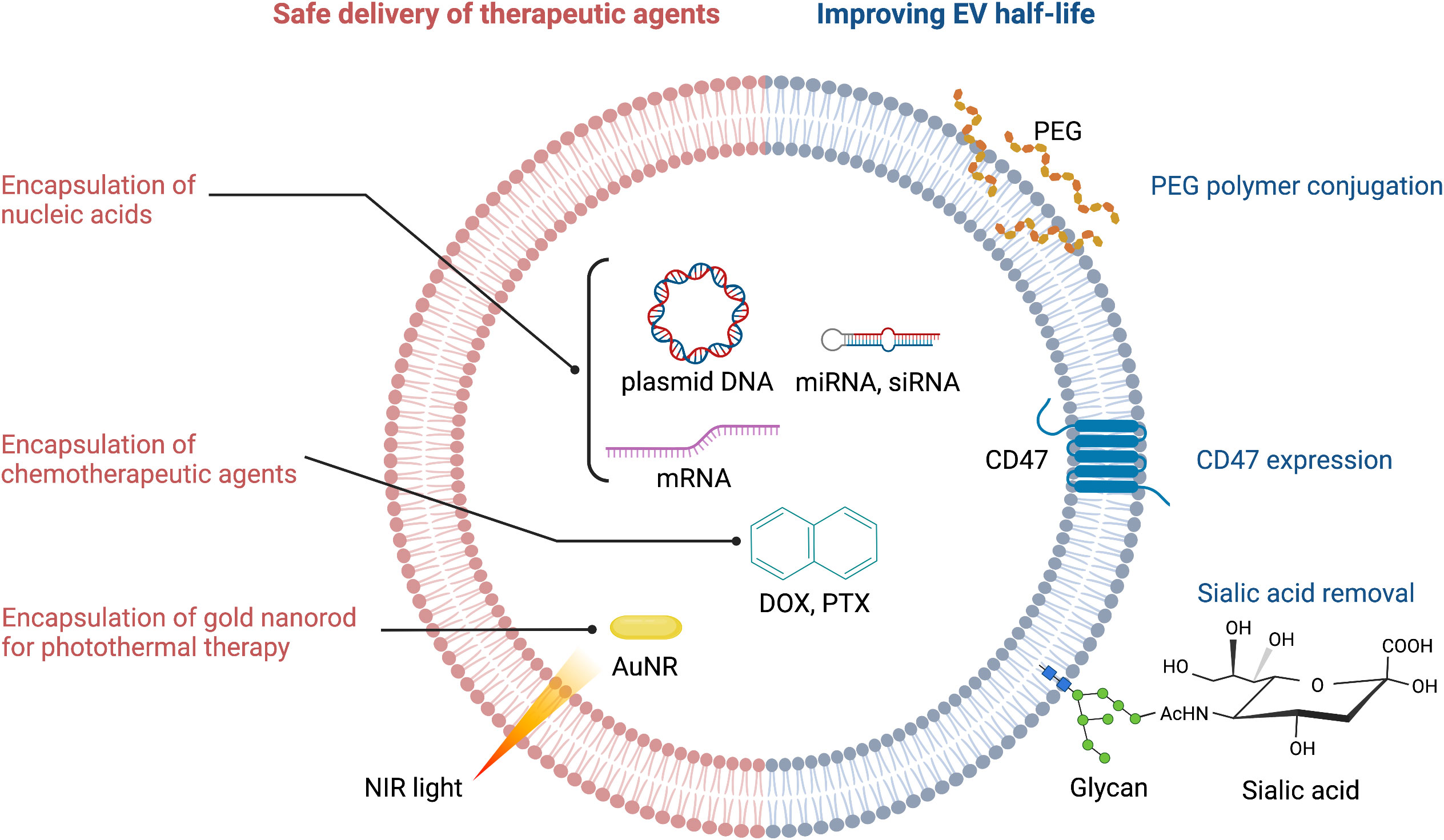
Figure 2 Strategies for improving therapeutic efficacy of EVs. PEG, polyethylene glycol; NIR, near infrared; AuNR, gold nanorod; DOX, doxorubicin; PTX, paclitaxel.
The EV membrane surface can be chemically or enzymatically modified to either increase EV half-life, or to alter its targeting selectivity. For example, polyethylene glycol (PEG) conjugation to the surface of EVs increases their half-life (32) (Figure 2). PEG can also be used as a ‘recognition signal’ for bispecific antibodies. Proof-of-concept is provided with a study using a PEG/EGFR bispecific antibody that increased specific targeting of PEG-loaded EVs to EGFR-positive tumors (33) (Figure 1). One can see here that PEG’s effects on EV half-life and antibody targeting is a powerful combination. Altering the surface glycan composition of EVs via enzymatic removal of sialic acid moieties also changes their biodistribution (34); this could ultimately be exploited to achieve tumor-specific targeting of EVs (Figure 2).
Transferrin receptors are highly enriched on the surface of reticulocyte-derived EVs. This can be exploited to enrich and purify EVs during production, or to facilitate EV-dependent drug delivery. For example, transferrin-conjugated superparamagnetic iron oxide nanoparticles (SPIONs) have been used for targeted delivery of chemotherapeutic drugs (35) (Figure 1).
Fusion to liposomes or micelles offers an alternative to chemical modification of EV membranes. For example, fusion of EVs to liposomes using the freeze-thaw method increases the cellular uptake of these hybrid EVs (36). Micelles have been used to deliver PEG-conjugated EGFR single-chain Fab fragment (scFab) to EVs, thereby expanding the spectrum of therapeutics amenable to an EV approach (33).
Loading of exogenous molecules into EVs
Encapsulation of chemotherapeutic agents and safe delivery to the target tumor are the common challenges in EV-mediated cancer chemotherapy (Figure 2). Drugs such as nucleic acids can be loaded via passive methods, such as combining drugs with the lipid bilayer after incubation, or conjugating drugs to the EV surface. Alternatively, for drugs with high molecular weight (i.e. DOX, PTX, AuNR), active methods of loading such as mechanical or chemical opening of the EV membrane via sonication, electroporation, saponin treatment or freeze-thaw cycles can be employed (37). The active methods create temporary pores through which therapeutic payloads can enter. However, this is not without risk: the internal composition of EVs and their cargos can be compromised, which may reduce efficacy (32). Ultimately, the success of both the passive and active methods is determined by the source of EVs, the specific drug involved, and the experimental design (38).
For the evaluation of EVs in clinical trials, drug loading must be achieved using a robust protocol, and at an industrial scale to meet demands (39). These prerequisites have presented challenges, because EVs lack the intracellular structures of whole cells, and therefore are not amenable to approaches such as conventional transfection.
Sources of EVs
In this section, we describe the wide variety of systems from which EVs can be sourced. As will become evident, the precise choice of system will depend on the downstream application. We also provide several examples of the application of EVs to cancer therapy.
Despite their substantial contribution to cancer treatment, chemotherapeutic agents are prone to rapid clearance, are poorly biocompatible, and suffer from low intra-tumoral delivery; they also induce systemic side effects and their efficacy is compromised by the onset of drug resistance (40). To overcome these challenges, various types of nanoscale delivery vehicles have been developed. These vehicles are associated with efficient drug delivery and tumor-specific targeting ability, and many have been evaluated in human clinical oncology trials (Table 1).
Tumor-derived EVs (TEX)
Tumor-derived EVs (TEX) preferentially home to and target the tumor cells from which they were derived (50) (Table 2). For example, accumulation of DOX in HT1080 xenografts was higher when the drug was delivered by HT1080-derived TEX rather than by those obtained from HeLa cells. Successful inhibition of tumor growth in other preclinical models using TEX carrying a chemotherapeutic payload has been independently confirmed (46). Furthermore, TEX have been used to deliver DOX-loaded porous silicon nanoparticles (PSiNPs) in mouse models of hepatocarcinoma, melanoma, and breast cancer (54). In each of these situations, beneficial attributes of EVs were observed. These included enhanced accumulation of drugs within tumors, more efficient extravasation and tissue penetration and striking anti-tumor activities.
Glioma is a devastating malignant brain tumor with a high mortality rate due to low penetration and efficacy of the available chemotherapeutics. TEX are already having an impact in this tumor type. For example, TEX-mediated delivery of IMV-001 (an antisense oligonucleotide against the transmembrane receptor, IGF1R) to patients with recurrent malignant glioma was more effective than other delivery methods due as it was associated with an increased number of tumor-infiltrating lymphocytes and enhanced anti-tumor immunity (57, 58). In glioblastoma, co-delivery of TEX with α-galactosylceramide enhanced the efficacy of a dendritic cell vaccine, likely due to its stimulation of immunomodulatory factor release into the tumor microenvironment (51).
In the liver cancer setting, administration of functionalized TEX loaded with an adjuvant, high mobility group nucleosome-binding protein 1 (HMGN1), reduced tumor size and potentiated immunogenicity in a mouse model of hepatocellular carcinoma (53) (Table 2). Furthermore, treatment of end-stage extrahepatic cholangiocarcinoma patients with malignant biliary obstruction with MTX-loaded TEX was efficacious and relieved biliary obstruction (48).
Pleural effusions and ascites are abnormal fluid collections within the thoracic and peritoneal cavity, respectively. They are frequent in terminal stage malignancies, and require aspiration and paracentesis to manage disease conditions. TEX may be particularly suited to drug delivery for pleural effusions. For example, A549 tumor cell-derived EVs loaded with methotrexate (MTX) induced neutrophil recruitment in the effusion fluid and improved primary malignant pleural effusion (MPE) in non-small cell lung cancer patients (49). Additionally, two clinical trials have been conducted in patients with MPE to explore the anti-tumor effects of methotrexate- or cisplatin-loaded TEX (NCT01854866, NCT02657460) (Table 1). End-stage lung cancer patients with metastatic malignant pleural effusion (MPE) and resistance to cisplatin were treated with cisplatin-loaded TEX (47). This led to a 95% reduction in the number of tumor cells in the malignant fluids, and was associated with increased survival rate without significant side effects.
In addition to tumor cells themselves, TEX are also present in ascites. These ascites-derived TEX can also be exploited for cancer treatment, as exemplified in a clinical trial (43) (Table 1). This study demonstrated that treatment of advanced colorectal cancer with TEX from malignant ascites plus GM-CSF induced a specific anti-tumor T cell response.
Mesenchymal stem cell-derived EVs (STEX)
Mesenchymal stem cell (MSC)-derived EVs (STEX) arise from different tissues in normal and disease conditions and have beneficial effects in wound healing (59), myocardial infarction (60), acute kidney injury (61), hepatic injury (62), neonatal lung injury (63) and optic nerve injury (64). STEX may be particularly suitable for therapeutic applications due to their excellent safety profile, low immunogenicity, and their ability to cross biological barriers (65). They have been used to successfully deliver both chemical and genetic payloads, as we discuss below.
Human umbilical cord STEX enhance drug-induced apoptosis of leukemic cells in vitro (66) and increase their sensitivity to the anticancer drug imatinib through caspase activation (67). STEX can also deliver genetic cargos. For example, STEX-mediated delivery of miR-379 significantly suppressed breast cancer growth in vivo by decreasing cyclooxygenase (COX)-2 expression (68) (Table 3). Furthermore, an ongoing phase I clinical trial in KRAS G12D-mutant pancreatic cancer is evaluating the optimal dose and adverse effects of STEX loaded with KRAS G12D siRNA (NCT03608631) (Table 1).
Adipose tissue-derived mesenchymal stromal cells (AD-MSCs) are one of the most studied STEX sources because they are easier to obtain and harvest through subcutaneous lipoaspiration; this is much less painful and less ethically sensitive than collecting bone marrow or embryonic stem cells (80, 81). In the first animal study of its kind, Ko et al. found that AD-MSC-derived EVs significantly increased the number of circulating NKT cells and inhibited HCC tumor growth (70). Following demonstrations that AD-MSCs have anti-tumor effects in bladder cancer (82), prostate cancer (83) and breast cancer (71) via induction of apoptosis, there has been a surge of interest in the use of AD-MSCs for cancer therapy. This is highlighted by the finding that delivery of miR-101 by AD-MSC-derived EVs downregulated BCL6 and inhibited the metastasis of osteosarcoma to the lungs in a preclinical mouse model (72) (Table 3).
Bone marrow-derived STEX carrying GRP78 siRNA enhanced sorafenib sensitivity in an HCC xenograft mouse model (73) (Table 3). Moreover, miR-125b-loaded EVs derived from AD-MSCs specifically reduced HCC cell proliferation in vitro by activation of the p53 tumor suppressor (84). STEX are currently under investigation in clinical trials beyond cancer, including those focused on neurological and cardiovascular diseases, and SARS-CoV-2 (85).
HEK293-derived EVs (HEX)
HEK293T cells have been widely used as EV producer cells due to their inherent rapid proliferation, high EV yield, and ease of genetic manipulation (86–90). HEK293-derived EVs (HEX) have been used to deliver gene therapies including miRNA for breast cancer (91) and both chemotherapeutics and therapeutic protein constructs in a schwannoma model (92). Notably, HEX are more readily taken up by human neural stem cells when compared to mature neurons, suggesting that they might be used to modulate undifferentiated neurons in future therapeutic applications (93).
Administration of HEX loaded with a miR-21 sponge construct (which prevents miR-21 binding to its natural target) significantly reduced tumor burden in a C6 glioma rat model (78) (Table 3). Since miR-21 is overexpressed in glioma, we infer that miR-21 sponge-loaded EVs will reduce the proliferation and malignant metastatic behavior of tumor cells in patients. Additionally, HEX loaded with an antisense oligonucleotide (ASO) targeting STAT6 selectively silenced expression of this transcription factor in colorectal cancer and HCC mouse models; combined with anti-PD1 immunotherapy, this led to greater than 90% inhibition of tumor growth (74) (Table 3). With the same strategy, an ongoing phase I clinical trial is evaluating the pharmacokinetics and pharmacodynamics of exoASO-STAT6 (CDK-004) in advanced HCC, colorectal cancer, and liver metastases from gastric cancer (NCT05375604) (Table 1).
Dendritic cell-derived EVs (DEX)
Cancer vaccines are used to boost the endogenous human immune response to cancer through enhanced recognition of tumor cell-related antigens. Adding to the therapeutic arsenal in this area are dendritic cell-derived EVs (DEX), which express MHC-I- and MHC-II-bound antigen peptides as well as other adhesion molecules (94). Pioneering work demonstrated that EVs derived from tumor peptide-pulsed bone marrow dendritic cells (BMDC) could prime cytotoxic T cells and thereby facilitate tumor growth inhibition in syngeneic tumor mouse models (95) (Table 4).
DEX can activate CD4+ T cells by inducing Th1 and Th2 immune responses, irrespective of the maturity of the DCs (102). However, large and small DEX constructs differ in their capacity to induce a T cell response, favoring cytokine secretion by Th2 and Th1 cell subtypes, respectively. A Phase II clinical trial evaluating DEX loaded with tumor antigen in patients with unresectable non-small cell lung cancer has recently been completed. The trial revealed activation of both innate and adaptive immunity, and over 4 months of progression-free survival in 32% of patients (NCT01159288) (45) (Table 1). Another Phase I trial in this cancer type showed that DEX therapy was well-tolerated and elicited only minor adverse effects (41).
NK cell-derived EVs (NEX)
NK cell-derived EVs (NEX) can carry cargo such as cytotoxic proteins, miRNAs, and cytokines that employ multiple mechanisms to kill tumor cells (103). They also exhibit immunomodulatory activity by stimulating other immune cells. Prior studies have demonstrated that NEX contain not only FasL and perforin but also TNFα; these molecules can all trigger melanoma cell death (96, 104) (Table 4). NEX exposed to neuroblastoma cells can ‘teach’ naive NK cells to recognize and kill these cancer cells, thereby overcoming immune resistance (97). Moreover, tumor cell growth is blocked by NEX containing the tumor suppressor miR-186, and these NEX also derepress TGFβ1-dependent inhibition of NK cells (98). These findings suggest that, in addition to their role as drug carriers, NEX can act as immunotherapeutic agents.
Macrophage-derived EVs (MEX)
Macrophages can be categorized into anti-tumor M1 and protumor M2 subtypes, and reprogramming between these phenotypes has been exploited for anticancer therapy (74). Macrophage-derived EVs (MEX) derived from the M1 macrophage cell line, RAW264.7, accumulate at the tumor site and promote M2-to-M1 macrophage reprogramming (100). MEX loaded with DOX also induced apoptosis and suppressed the growth of xenografts in a murine model of breast cancer (101) (Table 4).
Plant-derived EVs (PEX)
Fruits and vegetables have been used historically as medicines to treat numerous diseases. Following the discovery of plant-derived EVs (PEX), which can be isolated by extracting apoptotic vesicles from leaf, sunflower seeds, and roots (105), it was a natural progression to investigate whether they could be harnessed for therapeutic benefit (106). The choice of a specific PEX is determined by the type of health-promoting molecule that is enriched within each plant; this selection must match the disease to be treated. Specific examples follow below.
The consumption of grapes may reduce the impact of risk factors associated with multiple diseases, including cancer (107). This has been attributed to the presence of anti-inflammatory flavonoids (107), phenolic acids and polyphenols that exert anti-cancer activity by scavenging reactive oxygen species (108). It is therefore not surprising that PEX from grapes and grapefruit exhibit anti-inflammatory properties (109, 110) and can reduce colitis in murine models (109, 111).
Ginger contains gingerol, which has powerful antioxidant and anti-inflammatory properties (112); together with other compounds in ginger root, this may explain the ability of extracts from this plant to inhibit oxidative stress, arthritis, inflammation, and various types of infection (113). Ginger may also decrease the risk of cancer and diabetes (112, 114). As highlighted for grapes, ginger PEX are also able to attenuate inflammation (115) and reduce the pathologies associated with colitis (116).
Although their anti-inflammatory effects are well established, the evaluation of PEX as cancer therapeutics is still in the early stages. However, initial results are encouraging. For example, PEX from citrus lemons suppressed chronic myeloid leukemia xenograft growth by inducing TRAIL-mediated cell death (117) (Table 5). Additionally, an ongoing phase I clinical trial (NCT01294072) is evaluating whether PEX can be used to deliver curcumin (a constituent of the spice, turmeric) to perturb tumor cell metabolism and modulate the immune response in colon cancer patients (Table 1). There is a strong underlying preclinical evidence for this, because curcumin was reported to suppress colon cancer in a murine xenograft model by inhibiting Wnt/β-catenin signaling (122). A separate in vitro study showed that curcumin significantly arrested the growth of human colon cancer cells (123). These promising early data provide the rationale for further study of the therapeutic effects of PEX in diverse diseases.
Application of EVs in cancer therapy
Following the discussion of the sources of EVs above, we now present several examples of how EVs are being used to complement and enhance current anti-cancer approaches. It should become apparent from this discussion that the targeting potential of EVs, as well as some of their innate abilities to penetrate cell membranes, offers great promise in the realm of cancer therapy.
Tumor-specific targeting
A major obstacle to effective cancer chemotherapy is a lack of tumor-specific targeting, which is associated with toxicity in normal tissues. Superparamagnetic iron oxide nanoparticles (SPIONs) have great potential utility as magnetic nanoplatforms for targeted drug delivery (124), as they can be directed to the required tissue area through the use of external magnets. SPIONs conjugated with transferrin can readily be attached to EVs, which express high surface levels of the transferrin receptor (125). The SPION-decorated EVs can be loaded with a chemotherapeutic agent and then directed to the tumor site using magnets. This has been elegantly demonstrated by Zhang et al., who observed DOX-dependent tumor regression and increased survival in a preclinical model using this approach (120). The SPION approach has also been used to enhance the cytotoxic effect of the pro-apoptotic cytokine, TNFα, in melanoma cells (69).
An alternative to SPIONs with regard to tumor-specific targeting is the use of aptamers. Aptamers are short single-stranded DNA or RNA that can bind to a specific target molecule (126). Aptamers can be used for therapeutic purposes in the same way as monoclonal antibodies. For example, EVs loaded with siRNA against the anti-apoptotic molecule, survivin, have been specifically delivered to prostate cancer cells in vivo using prostate-specific membrane antigen (PSMA) aptamers (76) (Table 3). In a similar manner, EGFR aptamer-conjugated EVs loaded with survivin siRNA inhibited tumor growth in an orthotopic breast cancer mouse model (76). We believe that the aptamer approach could readily be exploited to treat other cancer types. For example, the interleukin-3 receptor (IL3R) is overexpressed on chronic myeloid leukemia (CML) cells, but is absent or expressed at low levels on normal hematopoietic stem cells. This suggests that IL3R could serve as a receptor target for EV-based cancer drug delivery systems (127–129).
Overcoming drug resistance
Following several rounds of chemotherapy, the onset of drug resistance leads to reduced survival. Several lines of evidence suggest that EVs may offer breakthroughs in this challenging area. For example, HEX modified with lipidomimetic chain-grafted hyaluronic acid can efficiently deliver DOX and reverse multi-drug resistance in breast cancer cells (77) (Table 3). In a 5-FU-resistant HER2-positive colorectal cancer mouse model, co-delivery of an miR-21 inhibitor using EVs decorated with a LAMP2-HER2 affibody fusion significantly enhanced cytotoxicity and restored 5-FU sensitivity (79). Multidrug-resistant Madin-Darby canine kidney cells can be re-sensitized to paclitaxel when the drug is delivered via EVs (99). Finally, HEX loaded with imatinib and siRNA to the driver oncogene, BCR-ABL, have been used to re-sensitize CML xenografts to imatinib in a preclinical model (75).
Hard-to-treat tumors
Primary brain tumors and tumors that metastasize to the brain are difficult to treat due to poor drug penetrance across the blood brain barrier (BBB). EVs may offer a natural solution to this problem because they are nanosized membrane vesicles that can easily pass through the BBB. The combination of BBB penetration and tumor cell-selective targeting using EVs has been compellingly demonstrated in glioma. Specifically, EVs decorated with RGERPPR, a peptide ligand for neuropilin-1 which is highly expressed on glioma cells but not other neuronal cells (130, 131), were able to target glioma cells in an orthotopic mouse model (132). Another example of EV success in brain tumors is the selective targeting of glioblastoma cells, which overexpress integrin αVβ3 receptors (133). EVs loaded with paclitaxel (PTX) and conjugated with RGD peptides which have high affinity for αVβ3 receptors were able to target orthotopic glioblastomas and prolong survival in a mouse model (121).
Triple negative breast cancer (TNBC) has the worst prognosis of all breast cancers, primarily because it lacks estrogen and progesterone receptors, and has extremely low levels of HER2 (134, 135). However, TNBC cells express high levels of the c-Met tyrosine kinase oncogene, which is associated with poor prognosis and drug resistance (136, 137), and could be targeted by EVs. Proof-of-concept has been demonstrated via the delivery of DOX to TNBC xenografts using MEX coated with the c-Met binding peptide, CBP (52, 138).
Enhancing other therapeutic modalities
EVs can be used in combination with cutting edge drug delivery and activation technologies, such as photothermal and photodynamic therapies. Gold nanorods (AuNRs) have unique properties that make them attractive for applications in bioimaging, drug delivery, and photothermal therapy in cancer (139). Irradiating AuNRs with near-infrared light (NIR) produces a moderate temperature rise in the target region that leads to selective damage in tumor tissues (Figure 2). Since NIR lasers can be manipulated precisely, this activation mechanism provides an ideal and versatile platform to simultaneously deliver heat and anticancer drugs with control over the exposure area, time, and dosage. An alternative photothermal agent is the vanadium carbide quantum dot (V2C QD). This compound has higher photothermal conversion efficiency than AuNRs, and V2C QD-loaded EVs have superior biocompatibility and long circulation times combined with advanced antitumor activity (55) (Table 2).
Photodynamic therapy (PDT) requires light and a photosensitizing chemical substance that produces an oxygen radical to elicit cell death (140). EVs conjugated with the photosensitizer protoporphyrin IX (PpIX) and nuclear localization signal (NLS) peptide have good biocompatibility and the ability to target the nucleus. This subcellular targeting strategy and the cytotoxic reactive oxygen species generated by the photosensitizer enhances the efficacy of PDT, opening a new window for cancer therapy (119).
Conclusion and future perspective
There is great demand for safe, efficient, and versatile drug delivery systems for cancer therapy. Engineered EVs hold great promise in this regard, given their ability to specifically target and efficiently transfer therapeutic agents into cancer cells. However, more evaluation is required to produce and incorporate engineered EVs into clinically relevant systems, weighing the potential risks and benefits of this new approach. While the use of allogenic EVs appears feasible, the selection of parental cells, assessment of immunologic and oncogenic effects, and risk of viral contamination need to be carefully considered.
Successful clinical translation of EVs depends on the availability of reliable methods for large-scale production, isolation, and characterization to minimize lot-to-lot variations in drug-loaded EVs (141). A potential solution to many of these challenges is the use of EV mimics or artificial EV generated by chemical or genetic modification. Fusion of drug-loaded liposomes with EVs can enhance drug loading and targeting abilities (142), while customized production of EVs by exogenously implanted cells offers a new route for the production of engineered EVs in vivo (143). Although further studies are required to develop safer, more efficient, and cost-effective methods for generating engineered EVs for practical applications in oncology, the future is decidedly bright for this next-generation cancer therapy.
Author contributions
The author confirms being the sole contributor of this work and has approved it for publication.
Funding
The work was supported by National Institutes of Health Grants (R03 DE029272), Feist-Weiller Cancer Center Foundation Legacy Fund, and LSU Collaborative Cancer Research Initiative (CCRI) Fund to TN.
Acknowledgments
The work was supported by National Institutes of Health Grants (R03 DE029272), Feist-Weiller Cancer Center Foundation Legacy Fund, and LSU Collaborative Cancer Research Initiative (CCRI) Fund to TN. Figures were created with BioRender.com.
Conflict of interest
The author declares that the research was conducted in the absence of any commercial or financial relationships that could be construed as a potential conflict of interest.
Publisher’s note
All claims expressed in this article are solely those of the authors and do not necessarily represent those of their affiliated organizations, or those of the publisher, the editors and the reviewers. Any product that may be evaluated in this article, or claim that may be made by its manufacturer, is not guaranteed or endorsed by the publisher.
References
1. Colombo M, Raposo G, Thery C. Biogenesis, secretion, and intercellular interactions of exosomes and other extracellular vesicles. Annu Rev Cell Dev Biol (2014) 30:255–89. doi: 10.1146/annurev-cellbio-101512-122326
2. Amabile N, Guerin AP, Leroyer A, Mallat Z, Nguyen C, Boddaert J, et al. Circulating endothelial microparticles are associated with vascular dysfunction in patients with end-stage renal failure. J Am Soc Nephrol (2005) 16(11):3381–8. doi: 10.1681/ASN.2005050535
3. Yanez-Mo M, Siljander PR, Andreu Z, Zavec AB, Borras FE, Buzas EI, et al. Biological properties of extracellular vesicles and their physiological functions. J Extracell Vesicles (2015) 4:27066. doi: 10.3402/jev.v4.27066
4. Kalluri R. The biology and function of exosomes in cancer. J Clin Invest (2016) 126(4):1208–15. doi: 10.1172/JCI81135
5. Tkach M, Thery C. Communication by extracellular vesicles: where we are and where we need to go. Cell (2016) 164(6):1226–32. doi: 10.1016/j.cell.2016.01.043
6. Kalluri R, LeBleu VS. The biology, function, and biomedical applications of exosomes. Science (2020) 367(6478). doi: 10.1126/science.aau6977
7. Valadi H, Ekstrom K, Bossios A, Sjostrand M, Lee JJ, Lotvall JO. Exosome-mediated transfer of mrnas and micrornas is a novel mechanism of genetic exchange between cells. Nat Cell Biol (2007) 9(6):654–9. doi: 10.1038/ncb1596
8. Keerthikumar S, Chisanga D, Ariyaratne D, Saffar H, Anand S, Zhao K, et al. Exocarta: A web-based compendium of exosomal cargo. J Mol Biol (2015) 428. doi: 10.1016/j.jmb.2015.09.019
9. O'Brien K, Breyne K, Ughetto S, Laurent LC, Breakefield XO. Rna delivery by extracellular vesicles in mammalian cells and its applications. Nat Rev Mol Cell Biol (2020) 21(10):585–606. doi: 10.1038/s41580-020-0251-y
10. Turchinovich A, Drapkina O, Tonevitsky A. Transcriptome of extracellular vesicles: state-of-the-art. Front Immunol (2019) 10:202. doi: 10.3389/fimmu.2019.00202
11. Nonaka T, Wong DTW. Liquid biopsy in head and neck cancer: Promises and challenges. J Dent Res (2018) 97(6):701–8. doi: 10.1177/0022034518762071
12. Nonaka T, Wong DTW. Saliva diagnostics. Annu Rev Anal Chem (Palo Alto Calif) (2022) 15(1):107–21. doi: 10.1146/annurev-anchem-061020-123959
13. Niemelä P, Hyvönen MT, Vattulainen I. Structure and dynamics of sphingomyelin bilayer: Insight gained through systematic comparison to phosphatidylcholine. Biophys J (2004) 87(5):2976–89. doi: 10.1529/biophysj.104.048702
14. Fathali H, Dunevall J, Majdi S, Cans AS. Extracellular osmotic stress reduces the vesicle size while keeping a constant neurotransmitter concentration. ACS Chem Neurosci (2017) 8(2):368–75. doi: 10.1021/acschemneuro.6b00350
15. Parolini I, Federici C, Raggi C, Lugini L, Palleschi S, De Milito A, et al. Microenvironmental ph is a key factor for exosome traffic in tumor cells. J Biol Chem (2009) 284(49):34211–22. doi: 10.1074/jbc.M109.041152
16. Record M, Carayon K, Poirot M, Silvente-Poirot S. Exosomes as new vesicular lipid transporters involved in cell-cell communication and various pathophysiologies. Biochim Biophys Acta (2014) 1841(1):108–20. doi: 10.1016/j.bbalip.2013.10.004
17. Boilard E. Extracellular vesicles and their content in bioactive lipid mediators: more than a sack of microrna. J Lipid Res (2018) 59(11):2037–46. doi: 10.1194/jlr.R084640
18. Harizi H, Corcuff JB, Gualde N. Arachidonic-acid-derived eicosanoids: roles in biology and immunopathology. Trends Mol Med (2008) 14(10):461–9. doi: 10.1016/j.molmed.2008.08.005
19. Yang Z, Shi J, Xie J, Wang Y, Sun J, Liu T, et al. Large-scale generation of functional mrna-encapsulating exosomes via cellular nanoporation. Nat BioMed Eng (2020) 4(1):69–83. doi: 10.1038/s41551-019-0485-1
20. Alvarez-Erviti L, Seow Y, Yin H, Betts C, Lakhal S, Wood MJA. Delivery of sirna to the mouse brain by systemic injection of targeted exosomes. Nat Biotechnol (2011) 29(4):341–5. doi: 10.1038/nbt.1807
21. Kamerkar S, LeBleu VS, Sugimoto H, Yang S, Ruivo CF, Melo SA, et al. Exosomes facilitate therapeutic targeting of oncogenic kras in pancreatic cancer. Nature (2017) 546(7659):498–503. doi: 10.1038/nature22341
22. Tian Y, Li S, Song J, Ji T, Zhu M, Anderson GJ, et al. A doxorubicin delivery platform using engineered natural membrane vesicle exosomes for targeted tumor therapy. Biomaterials (2014) 35(7):2383–90. doi: 10.1016/j.biomaterials.2013.11.083
23. Cheng Q, Shi X, Han M, Smbatyan G, Lenz HJ, Zhang Y. Reprogramming exosomes as nanoscale controllers of cellular immunity. J Am Chem Soc (2018) 140(48):16413–7. doi: 10.1021/jacs.8b10047
24. Shi X, Cheng Q, Hou T, Han M, Smbatyan G, Lang JE, et al. Genetically engineered cell-derived nanoparticles for targeted breast cancer immunotherapy. Mol Ther (2020) 28(2):536–47. doi: 10.1016/j.ymthe.2019.11.020
25. Fu W, Lei C, Liu S, Cui Y, Wang C, Qian K, et al. Car Exosomes derived from effector car-t cells have potent antitumour effects and low toxicity. Nat Commun (2019) 10(1):4355. doi: 10.1038/s41467-019-12321-3
26. Gujrati V, Kim S, Kim SH, Min JJ, Choy HE, Kim SC, et al. Bioengineered bacterial outer membrane vesicles as cell-specific drug-delivery vehicles for cancer therapy. ACS Nano (2014) 8(2):1525–37. doi: 10.1021/nn405724x
27. Delcayre A, Estelles A, Sperinde J, Roulon T, Paz P, Aguilar B, et al. Exosome display technology: applications to the development of new diagnostics and therapeutics. Blood Cells Mol Dis (2005) 35(2):158–68. doi: 10.1016/j.bcmd.2005.07.003
28. Kooijmans SA, Aleza CG, Roffler SR, van Solinge WW, Vader P, Schiffelers RM. Display of Gpi-anchored anti-egfr nanobodies on extracellular vesicles promotes tumour cell targeting. J Extracell Vesicles (2016) 5:31053. doi: 10.3402/jev.v5.31053
29. Kooijmans SAA, Gitz-Francois J, Schiffelers RM, Vader P. Recombinant phosphatidylserine-binding nanobodies for targeting of extracellular vesicles to tumor cells: a plug-and-play approach. Nanoscale (2018) 10(5):2413–26. doi: 10.1039/c7nr06966a
30. Koh E, Lee EJ, Nam GH, Hong Y, Cho E, Yang Y, et al. Exosome-sirpalpha, a cd47 blockade increases cancer cell phagocytosis. Biomaterials (2017) 121:121–9. doi: 10.1016/j.biomaterials.2017.01.004
31. Zhang K-L, Wang Y-J, Sun J, Zhou J, Xing C, Huang G, et al. Artificial chimeric exosomes for anti-phagocytosis and targeted cancer therapy. Chem Sci (2019) 10(5):1555–61. doi: 10.1039/C8SC03224F
32. Kooijmans SAA, Fliervoet LAL, van der Meel R, Fens M, Heijnen HFG, van Bergen En Henegouwen PMP, et al. Pegylated and targeted extracellular vesicles display enhanced cell specificity and circulation time. J Control Release (2016) 224:77–85. doi: 10.1016/j.jconrel.2016.01.009
33. Su Y-C, Burnouf P-A, Chuang K-H, Chen B-M, Cheng T-L, Roffler SR. Conditional internalization of pegylated nanomedicines by peg engagers for triple negative breast cancer therapy. Nat Commun (2017) 8(1):15507. doi: 10.1038/ncomms15507
34. Royo F, Cossío U, Ruiz de Angulo A, Llop J, Falcon-Perez JM. Modification of the glycosylation of extracellular vesicles alters their biodistribution in mice. Nanoscale (2019) 11(4):1531–7. doi: 10.1039/c8nr03900c
35. Qi H, Liu C, Long L, Ren Y, Zhang S, Chang X, et al. Blood exosomes endowed with magnetic and targeting properties for cancer therapy. ACS Nano (2016) 10(3):3323–33. doi: 10.1021/acsnano.5b06939
36. Sato YT, Umezaki K, Sawada S, S-a M, Sasaki Y, Harada N, et al. Engineering hybrid exosomes by membrane fusion with liposomes. Sci Rep (2016) 6(1):21933. doi: 10.1038/srep21933
37. Fuhrmann G, Serio A, Mazo M, Nair R, Stevens MM. Active loading into extracellular vesicles significantly improves the cellular uptake and photodynamic effect of porphyrins. J Control Release (2015) 205:35–44. doi: 10.1016/j.jconrel.2014.11.029
38. Doyle LM, Wang MZ. Overview of extracellular vesicles, their origin, composition, purpose, and methods for exosome isolation and analysis. Cells (2019) 8(7):727. doi: 10.3390/cells8070727
39. Armstrong JPK, Holme MN, Stevens MM. Re-engineering extracellular vesicles as smart nanoscale therapeutics. ACS Nano (2017) 11(1):69–83. doi: 10.1021/acsnano.6b07607
40. Senapati S, Mahanta AK, Kumar S, Maiti P. Controlled drug delivery vehicles for cancer treatment and their performance. Signal Transduct Target Ther (2018) 3:7. doi: 10.1038/s41392-017-0004-3
41. Morse MA, Garst J, Osada T, Khan S, Hobeika A, Clay TM, et al. A phase I study of dexosome immunotherapy in patients with advanced non-small cell lung cancer. J Transl Med (2005) 3(1):9–. doi: 10.1186/1479-5876-3-9
42. Escudier B, Dorval T, Chaput N, André F, Caby M-P, Novault S, et al. Vaccination of metastatic melanoma patients with autologous dendritic cell (dc) derived-exosomes: results of thefirst phase I clinical trial. J Transl Med (2005) 3(1):10–. doi: 10.1186/1479-5876-3-10
43. Dai S, Wei D, Wu Z, Zhou X, Wei X, Huang H, et al. Phase I clinical trial of autologous ascites-derived exosomes combined with gm-csf for colorectal cancer. Mol Ther (2008) 16(4):782–90. doi: 10.1038/mt.2008.1
44. Viaud S, Terme M, Flament C, Taieb J, Andre F, Novault S, et al. Dendritic cell-derived exosomes promote natural killer cell activation and proliferation: a role for nkg2d ligands and Il-15ralpha. PloS One (2009) 4(3):e4942. doi: 10.1371/journal.pone.0004942
45. Besse B, Charrier M, Lapierre V, Dansin E, Lantz O, Planchard D, et al. Dendritic cell-derived exosomes as maintenance immunotherapy after first line chemotherapy in nsclc. Oncoimmunology (2015) 5(4):e1071008-e. doi: 10.1080/2162402X.2015.1071008
46. Tang K, Zhang Y, Zhang H, Xu P, Liu J, Ma J, et al. Delivery of chemotherapeutic drugs in tumour cell-derived microparticles. Nat Commun (2012) 3(1):1282. doi: 10.1038/ncomms2282
47. Ma J, Zhang Y, Tang K, Zhang H, Yin X, Li Y, et al. Reversing drug resistance of soft tumor-repopulating cells by tumor cell-derived chemotherapeutic microparticles. Cell Res (2016) 26(6):713–27. doi: 10.1038/cr.2016.53
48. Gao Y, Zhang H, Zhou N, Xu P, Wang J, Gao Y, et al. Methotrexate-loaded tumour-cell-derived microvesicles can relieve biliary obstruction in patients with extrahepatic cholangiocarcinoma. Nat Biomed Eng (2020) 4(7):743–53. doi: 10.1038/s41551-020-0583-0
49. Xu P, Tang K, Ma J, Zhang H, Wang D, Zhu L, et al. Chemotherapeutic tumor microparticles elicit a neutrophil response targeting malignant pleural effusions. Cancer Immunol Res (2020) 8(9):1193–205. doi: 10.1158/2326-6066.CIR-19-0789
50. Qiao L, Hu S, Huang K, Su T, Li Z, Vandergriff A, et al. Tumor cell-derived exosomes home to their cells of origin and can be used as trojan horses to deliver cancer drugs. Theranostics (2020) 10(8):3474–87. doi: 10.7150/thno.39434
51. Liu H, Chen L, Liu J, Meng H, Zhang R, Ma L, et al. Co-delivery of tumor-derived exosomes with alpha-galactosylceramide on dendritic cell-based immunotherapy for glioblastoma. Cancer Lett (2017) 411:182–90. doi: 10.1016/j.canlet.2017.09.022
52. Li S, Wu Y, Ding F, Yang J, Li J, Gao X, et al. Engineering macrophage-derived exosomes for targeted chemotherapy of triple-negative breast cancer. Nanoscale (2020) 12(19):10854–62. doi: 10.1039/D0NR00523A
53. Zuo B, Qi H, Lu Z, Chen L, Sun B, Yang R, et al. Alarmin-painted exosomes elicit persistent antitumor immunity in large established tumors in mice. Nat Commun (2020) 11(1):1790. doi: 10.1038/s41467-020-15569-2
54. Yong T, Zhang X, Bie N, Zhang H, Zhang X, Li F, et al. Tumor exosome-based nanoparticles are efficient drug carriers for chemotherapy. Nat Commun (2019) 10(1):3838. doi: 10.1038/s41467-019-11718-4
55. Cao Y, Wu T, Zhang K, Meng X, Dai W, Wang D, et al. Engineered exosome-mediated near-infrared-ii region v(2)c quantum dot delivery for nucleus-target low-temperature photothermal therapy. ACS Nano (2019) 13(2):1499–510. doi: 10.1021/acsnano.8b07224
56. Wang K, Ye H, Zhang X, Wang X, Yang B, Luo C, et al. An exosome-like programmable-bioactivating paclitaxel prodrug nanoplatform for enhanced breast cancer metastasis inhibition. Biomaterials (2020) 257:120224. doi: 10.1016/j.biomaterials.2020.120224
57. Andrews DW, Resnicoff M, Flanders AE, Kenyon L, Curtis M, Merli G, et al. Results of a pilot study involving the use of an antisense oligodeoxynucleotide directed against the insulin-like growth factor type I receptor in malignant astrocytomas. J Clin Oncol (2001) 19(8):2189–200. doi: 10.1200/JCO.2001.19.8.2189
58. Andrews DW, Judy KD, Scott CB, Garcia S, Harshyne LA, Kenyon L, et al. Phase Ib clinical trial of igv-001 for patients with newly diagnosed glioblastoma. Clin Cancer Res (2021) 27(7):1912–22. doi: 10.1158/1078-0432.CCR-20-3805
59. Zhang Y, Liu Y, Liu H, Tang W. Exosomes: Biogenesis, biologic function and clinical potential. Cell Bioscience (2019) 9. doi: 10.1186/s13578-019-0282-2
60. Liu L, Jin X, Hu CF, Li R, Zhou Z, Shen CX. Exosomes derived from mesenchymal stem cells rescue myocardial ischaemia/reperfusion injury by inducing cardiomyocyte autophagy Via ampk and akt pathways. Cell Physiol Biochem (2017) 43(1):52–68. doi: 10.1159/000480317
61. van Koppen A, Joles JA, van Balkom BW, Lim SK, de Kleijn D, Giles RH, et al. Human embryonic mesenchymal stem cell-derived conditioned medium rescues kidney function in rats with established chronic kidney disease. PloS One (2012) 7(6):e38746. doi: 10.1371/journal.pone.0038746
62. Tan CY, Lai RC, Wong W, Dan YY, Lim SK, Ho HK. Mesenchymal stem cell-derived exosomes promote hepatic regeneration in drug-induced liver injury models. Stem Cell Res Ther (2014) 5(3):76. doi: 10.1186/scrt465
63. Willis GR, Mitsialis SA, Kourembanas S. "Good Things come in small packages": application of exosome-based therapeutics in neonatal lung injury. Pediatr Res (2018) 83(1-2):298–307. doi: 10.1038/pr.2017.256
64. Mead B, Tomarev S. Bone marrow-derived mesenchymal stem cells-derived exosomes promote survival of retinal ganglion cells through mirna-dependent mechanisms. Stem Cells Transl Med (2017) 6(4):1273–85. doi: 10.1002/sctm.16-0428
65. Gowen A, Shahjin F, Chand S, Odegaard KE, Yelamanchili SV. Mesenchymal stem cell-derived extracellular vesicles: challenges in clinical applications. Front Cell Dev Biol (2020) 8:149. doi: 10.3389/fcell.2020.00149
66. Phetfong J, Tawonsawatruk T, Kamprom W, Ontong P, Tanyong D, Borwornpinyo S, et al. Bone marrow-mesenchymal stem cell-derived extracellular vesicles affect proliferation and apoptosis of leukemia cells in vitro. FEBS Open Bio (2022) 12(2):470–9. doi: 10.1002/2211-5463.13352
67. Liu Y, Song B, Wei Y, Chen F, Chi Y, Fan H, et al. Exosomes from mesenchymal stromal cells enhance imatinib-induced apoptosis in human leukemia cells via activation of caspase signaling pathway. Cytotherapy (2017) 20. doi: 10.1016/j.jcyt.2017.11.006
68. O'Brien KP, Khan S, Gilligan KE, Zafar H, Lalor P, Glynn C, et al. Employing mesenchymal stem cells to support tumor-targeted delivery of extracellular vesicle (ev)-encapsulated microrna-379. Oncogene (2018) 37(16):2137–49. doi: 10.1038/s41388-017-0116-9
69. Zhuang M, Chen X, Du D, Shi J, Deng M, Long Q, et al. Spion decorated exosome delivery of tnf-α to cancer cell membranes through magnetism. Nanoscale (2020) 12(1):173–88. doi: 10.1039/C9NR05865F
70. Ko SF, Yip HK, Zhen YY, Lee CC, Lee CC, Huang CC, et al. Adipose-derived mesenchymal stem cell exosomes suppress hepatocellular carcinoma growth in a rat model: apparent diffusion coefficient, natural killer t-cell responses, and histopathological features. Stem Cells Int (2015) 2015:853506. doi: 10.1155/2015/853506
71. Li T, Zhou X, Wang J, Liu Z, Han S, Wan L, et al. Adipose-derived mesenchymal stem cells and extracellular vesicles confer antitumor activity in preclinical treatment of breast cancer. Pharmacol Res (2020) 157:104843. doi: 10.1016/j.phrs.2020.104843
72. Zhang K, Dong C, Chen M, Yang T, Wang X, Gao Y, et al. Extracellular vesicle-mediated delivery of mir-101 inhibits lung metastasis in osteosarcoma. Theranostics (2020) 10(1):411–25. doi: 10.7150/thno.33482
73. Li H, Yang C, Shi Y, Zhao L. Exosomes derived from sirna against grp78 modified bone-marrow-derived mesenchymal stem cells suppress sorafenib resistance in hepatocellular carcinoma. J Nanobiotechnology (2018) 16(1):103. doi: 10.1186/s12951-018-0429-z
74. Kamerkar S, Leng C, Burenkova O, Jang SC, McCoy C, Zhang K, et al. Exosome-mediated genetic reprogramming of tumor-associated macrophages by exoaso-stat6 leads to potent monotherapy antitumor activity. Sci Adv (2022) 8(7):eabj7002. doi: 10.1126/sciadv.abj7002
75. Bellavia D, Raimondo S, Calabrese G, Forte S, Cristaldi M, Patinella A, et al. Interleukin 3- receptor targeted exosomes inhibit in vitro and in vivo chronic myelogenous leukemia cell growth. Theranostics (2017) 7(5):1333–45. doi: 10.7150/thno.17092
76. Pi F, Binzel DW, Lee TJ, Li Z, Sun M, Rychahou P, et al. Nanoparticle orientation to control rna loading and ligand display on extracellular vesicles for cancer regression. Nat Nanotechnol (2018) 13(1):82–9. doi: 10.1038/s41565-017-0012-z
77. Liu J, Ye Z, Xiang M, Chang B, Cui J, Ji T, et al. Functional extracellular vesicles engineered with lipid-grafted hyaluronic acid effectively reverse cancer drug resistance. Biomaterials (2019) 223:119475. doi: 10.1016/j.biomaterials.2019.119475
78. Monfared H, Jahangard Y, Nikkhah M, Mirnajafi-Zadeh J, Mowla SJ. Potential therapeutic effects of exosomes packed with a mir-21-sponge construct in a rat model of glioblastoma. Front Oncol (2019) 9:782. doi: 10.3389/fonc.2019.00782
79. Liang G, Zhu Y, Ali DJ, Tian T, Xu H, Si K, et al. Engineered exosomes for targeted co-delivery of mir-21 inhibitor and chemotherapeutics to reverse drug resistance in colon cancer. J Nanobiotechnology (2020) 18(1):10. doi: 10.1186/s12951-019-0563-2
80. Gimble JM, Katz AJ, Bunnell BA. Adipose-derived stem cells for regenerative medicine. Circ Res (2007) 100(9):1249–60. doi: 10.1161/01.RES.0000265074.83288.09
81. Miana VV, Gonzalez EAP. Adipose tissue stem cells in regenerative medicine. Ecancermedicalscience (2018) 12:822. doi: 10.3332/ecancer.2018.822
82. Wang T, Yu X, Lin J, Qin C, Bai T, Xu T, et al. Adipose-derived stem cells inhibited the proliferation of bladder tumor cells by s phase arrest and wnt/beta-catenin pathway. Cell Reprogram (2019) 21(6):331–8. doi: 10.1089/cell.2019.0047
83. Rigotti G, Marchi A, Sbarbati A. Adipose-derived mesenchymal stem cells: past, present, and future. Aesthetic Plast Surg (2009) 33(3):271–3. doi: 10.1007/s00266-009-9339-7
84. Baldari S, Di Rocco G, Magenta A, Picozza M, Toietta G. Extracellular vesicles-encapsulated microrna-125b produced in genetically modified mesenchymal stromal cells inhibits hepatocellular carcinoma cell proliferation. Cells (2019). doi: 10.3390/cells8121560
85. Lee B-C, Kang I, Yu K-R. Therapeutic features and updated clinical trials of mesenchymal stem cell (msc)-derived exosomes. J Clin Med (2021) 10(4):711. doi: 10.3390/jcm10040711
86. Faruqu FN, Xu L, Al-Jamal KT. Preparation of exosomes for sirna delivery to cancer cells. J Vis Exp (2018). doi: 10.3791/58814
87. Ferguson S, Kim S, Lee C, Deci M, Nguyen J. The phenotypic effects of exosomes secreted from distinct cellular sources: a comparative study based on mirna composition. AAPS J (2018) 20(4):67. doi: 10.1208/s12248-018-0227-4
88. Johnsen KB, Gudbergsson JM, Skov MN, Pilgaard L, Moos T, Duroux M. A comprehensive overview of exosomes as drug delivery vehicles - endogenous nanocarriers for targeted cancer therapy. Biochim Biophys Acta (2014) 1846(1):75–87. doi: 10.1016/j.bbcan.2014.04.005
89. Liu Y, Li D, Liu Z, Zhou Y, Chu D, Li X, et al. Targeted exosome-mediated delivery of opioid receptor mu sirna for the treatment of morphine relapse. Sci Rep (2015) 5:17543. doi: 10.1038/srep17543
90. Zhu X, Badawi M, Pomeroy S, Sutaria DS, Xie Z, Baek A, et al. Comprehensive toxicity and immunogenicity studies reveal minimal effects in mice following sustained dosing of extracellular vesicles derived from hek293t cells. J Extracell Vesicles (2017) 6(1):1324730. doi: 10.1080/20013078.2017.1324730
91. Ohno S, Takanashi M, Sudo K, Ueda S, Ishikawa A, Matsuyama N, et al. Systemically injected exosomes targeted to EGFR deliver antitumor microrna to breast cancer cells. Mol Ther (2013) 21(1):185–91. doi: 10.1038/mt.2012.180
92. Mizrak A, Bolukbasi MF, Ozdener GB, Brenner GJ, Madlener S, Erkan EP, et al. Genetically engineered microvesicles carrying suicide mrna/protein inhibit schwannoma tumor growth. Mol Ther (2013) 21(1):101–8. doi: 10.1038/mt.2012.161
93. Jurgielewicz BJ, Yao Y, Stice SL. Kinetics and specificity of hek293t extracellular vesicle uptake using imaging flow cytometry. Nanoscale Res Lett (2020) 15(1):170. doi: 10.1186/s11671-020-03399-6
94. Pitt JM, Andre F, Amigorena S, Soria JC, Eggermont A, Kroemer G, et al. Dendritic cell-derived exosomes for cancer therapy. J Clin Invest (2016) 126(4):1224–32. doi: 10.1172/JCI81137
95. Zitvogel L, Regnault A, Lozier A, Wolfers J, Flament C, Tenza D, et al. Eradication of established murine tumors using a novel cell-free vaccine: dendritic cell-derived exosomes. Nat Med (1998) 4(5):594–600. doi: 10.1038/nm0598-594
96. Zhu L, Kalimuthu S, Gangadaran P, Oh JM, Lee HW, Baek SH, et al. Exosomes derived from natural killer cells exert therapeutic effect in melanoma. Theranostics (2017) 7(10):2732–45. doi: 10.7150/thno.18752
97. Shoae-Hassani A, Hamidieh AA, Behfar M, Mohseni R, Mortazavi-Tabatabaei SA, Asgharzadeh S. Nk cell-derived exosomes from nk cells previously exposed to neuroblastoma cells augment the antitumor activity of cytokine-activated nk cells. J Immunother (2017) 40(7):265–76. doi: 10.1097/cji.0000000000000179
98. Neviani P, Wise PM, Murtadha M, Liu CW, Wu CH, Jong AY, et al. Natural killer-derived exosomal mir-186 inhibits neuroblastoma growth and immune escape mechanisms. Cancer Res (2019) 79(6):1151–64. doi: 10.1158/0008-5472.Can-18-0779
99. Kim MS, Haney MJ, Zhao Y, Mahajan V, Deygen I, Klyachko NL, et al. Development of exosome-encapsulated paclitaxel to overcome mdr in cancer cells. Nanomedicine (2016) 12(3):655–64. doi: 10.1016/j.nano.2015.10.012
100. Choo YW, Kang M, Kim HY, Han J, Kang S, Lee JR, et al. M1 macrophage-derived nanovesicles potentiate the anticancer efficacy of immune checkpoint inhibitors. ACS Nano (2018) 12(9):8977–93. doi: 10.1021/acsnano.8b02446
101. Fan Z, Xiao K, Lin J, Liao Y, Huang X. Functionalized DNA enables programming exosomes/vesicles for tumor imaging and therapy. Small (2019) 15(47):1903761. doi: 10.1002/smll.201903761
102. Tkach M, Kowal J, Zucchetti AE, Enserink L, Jouve M, Lankar D, et al. Qualitative differences in t-cell activation by dendritic cell-derived extracellular vesicle subtypes. EMBO J (2017) 36(20):3012–28. doi: 10.15252/embj.201696003
103. Wu F, Xie M, Hun M, She Z, Li C, Luo S, et al. Natural killer cell-derived extracellular vesicles: novel players in cancer immunotherapy. Front Immunol (2021) 12:658698. doi: 10.3389/fimmu.2021.658698
104. Lugini L, Cecchetti S, Huber V, Luciani F, Macchia G, Spadaro F, et al. Immune surveillance properties of human nk cell-derived exosomes. J Immunol (Baltimore Md 1950) (2012) 189(6):2833–42. doi: 10.4049/jimmunol.1101988
105. Chen Y-S, Lin E-Y, Chiou T-W, Harn H-J. Exosomes in clinical trial and their production in compliance with good manufacturing practice. Ci Ji Yi Xue Za Zhi (2019) 32(2):113–20. doi: 10.4103/tcmj.tcmj_182_19
106. Rome S. Biological properties of plant-derived extracellular vesicles. Food Funct (2019) 10(2):529–38. doi: 10.1039/c8fo02295j
107. Vislocky LM, Fernandez ML. Biomedical effects of grape products. Nutr Rev (2010) 68(11):656–70. doi: 10.1111/j.1753-4887.2010.00335.x
108. Dinicola S, Cucina A, Antonacci D, Bizzarri M. Anticancer effects of grape seed extract on human cancers: a review. J Carcinog Mutagen (2014). doi: 10.4172/2157-2518.S8-005
109. Ju S, Mu J, Dokland T, Zhuang X, Wang Q, Jiang H, et al. Grape exosome-like nanoparticles induce intestinal stem cells and protect mice from dss-induced colitis. Mol Ther (2013) 21(7):1345–57. doi: 10.1038/mt.2013.64
110. Perez-Bermudez P, Blesa J, Soriano JM, Marcilla A. Extracellular vesicles in food: experimental evidence of their secretion in grape fruits. Eur J Pharm Sci (2017) 98:40–50. doi: 10.1016/j.ejps.2016.09.022
111. Wang B, Zhuang X, Deng ZB, Jiang H, Mu J, Wang Q, et al. Targeted drug delivery to intestinal macrophages by bioactive nanovesicles released from grapefruit. Mol Ther (2014) 22(3):522–34. doi: 10.1038/mt.2013.190
112. Mashhadi NS, Ghiasvand R, Askari G, Hariri M, Darvishi L, Mofid MR. Anti-oxidative and anti-inflammatory effects of ginger in health and physical activity: review of current evidence. Int J Prev Med (2013) 4(Suppl 1):S36–42.
113. Dugasani S, Pichika MR, Nadarajah VD, Balijepalli MK, Tandra S, Korlakunta JN. Comparative antioxidant and anti-inflammatory effects of [6]-gingerol, [8]-gingerol, [10]-gingerol and [6]-shogaol. J Ethnopharmacol (2010) 127(2):515–20. doi: 10.1016/j.jep.2009.10.004
114. Mao QQ, Xu XY, Cao SY, Gan RY, Corke H, Beta T, et al. Bioactive compounds and bioactivities of ginger (zingiber officinale roscoe). Foods (2019) 8(6). doi: 10.3390/foods8060185
115. Chen X, Zhou Y, Yu J. Exosome-like nanoparticles from ginger rhizomes inhibited nlrp3 inflammasome activation. Mol Pharm (2019) 16(6):2690–9. doi: 10.1021/acs.molpharmaceut.9b00246
116. Zhang M, Viennois E, Prasad M, Zhang Y, Wang L, Zhang Z, et al. Edible ginger-derived nanoparticles: a novel therapeutic approach for the prevention and treatment of inflammatory bowel disease and colitis-associated cancer. Biomaterials (2016) 101:321–40. doi: 10.1016/j.biomaterials.2016.06.018
117. Raimondo S, Naselli F, Fontana S, Monteleone F, Lo Dico A, Saieva L, et al. Citrus limon-derived nanovesicles inhibit cancer cell proliferation and suppress cml xenograft growth by inducing trail-mediated cell death. Oncotarget (2015) 6(23):19514–27. doi: 10.18632/oncotarget.4004
118. Wang Q, Zhuang X, Mu J, Deng ZB, Jiang H, Zhang L, et al. Delivery of therapeutic agents by nanoparticles made of grapefruit-derived lipids. Nat Commun (2013) 4:1867. doi: 10.1038/ncomms2886
119. Cheng H, Fan J-H, Zhao L-P, Fan G-L, Zheng R-R, Qiu X-Z, et al. Chimeric peptide engineered exosomes for dual-stage light guided plasma membrane and nucleus targeted photodynamic therapy. Biomaterials (2019) 211:14–24. doi: 10.1016/j.biomaterials.2019.05.004
120. Zhang J, Ji C, Zhang H, Shi H, Mao F, Qian H, et al. Engineered neutrophil-derived exosome-like vesicles for targeted cancer therapy. Sci Adv (2022) 8(2):eabj8207. doi: 10.1126/sciadv.abj8207
121. Zhu Q, Ling X, Yang Y, Zhang J, Li Q, Niu X, et al. Embryonic stem cells-derived exosomes endowed with targeting properties as chemotherapeutics delivery vehicles for glioblastoma therapy. Adv Sci (2019) 6(6):1801899. doi: 10.1002/advs.201801899
122. Dou H, Shen R, Tao J, Huang L, Shi H, Chen H, et al. Curcumin suppresses the colon cancer proliferation by inhibiting wnt/beta-catenin pathways via Mir-130a. Front Pharmacol (2017) 8:877. doi: 10.3389/fphar.2017.00877
123. Mosieniak G, Adamowicz M, Alster O, Jaskowiak H, Szczepankiewicz AA, Wilczynski GM, et al. Curcumin induces permanent growth arrest of human colon cancer cells: link between senescence and autophagy. Mech Ageing Dev (2012) 133(6):444–55. doi: 10.1016/j.mad.2012.05.004
124. Wahajuddin, Arora S. Superparamagnetic iron oxide nanoparticles: magnetic nanoplatforms as drug carriers. Int J Nanomed (2012) 7:3445–71. doi: 10.2147/IJN.S30320
125. Thery C, Zitvogel L, Amigorena S. Exosomes: composition, biogenesis and function. Nat Rev Immunol (2002) 2(8):569–79. doi: 10.1038/nri855
126. Keefe AD, Pai S, Ellington A. Aptamers as therapeutics. Nat Rev Drug Discovery (2010) 9(7):537–50. doi: 10.1038/nrd3141
127. Nievergall E, Ramshaw HS, Yong AS, Biondo M, Busfield SJ, Vairo G, et al. Monoclonal antibody targeting of il-3 receptor alpha with csl362 effectively depletes cml progenitor and stem cells. Blood (2014) 123(8):1218–28. doi: 10.1182/blood-2012-12-475194
128. Testa U, Pelosi E, Frankel A. Cd 123 is a membrane biomarker and a therapeutic target in hematologic malignancies. biomark Res (2014) 2(1):4. doi: 10.1186/2050-7771-2-4
129. Testa U, Riccioni R, Militi S, Coccia E, Stellacci E, Samoggia P, et al. Elevated Expression of Il-3ralpha in Acute Myelogenous Leukemia Is Associated with Enhanced Blast Proliferation, Increased Cellularity, and Poor Prognosis. Blood (2002) 100(8):2980–8. doi: 10.1182/blood-2002-03-0852
130. Chen L, et al. Inhibitory effect of neuropilin-1 monoclonal antibody (NRP-1 MAb) on glioma tumor in mice. J BioMed Nanotechnol (2013) 9(4):551–8. doi: 10.1166/jbn.2013.1623
131. Chen L, Zhang G, Shi Y, Qiu R, Khan AA. Neuropilin-1 (Nrp-1) and magnetic nanoparticles, a potential combination for diagnosis and therapy of gliomas. Curr Pharm Des (2015) 21(37):5434–49. doi: 10.2174/1381612821666150917092658
132. Jia G, Han Y, An Y, Ding Y, He C, Wang X, et al. Nrp-1 targeted and cargo-loaded exosomes facilitate simultaneous imaging and therapy of glioma in vitro and in vivo. Biomaterials (2018) 178:302–16. doi: 10.1016/j.biomaterials.2018.06.029
133. Zhong Y, Wang C, Cheng R, Cheng L, Meng F, Liu Z, et al. cRGD-directed, NIR-responsive and robust AuNR/PEG-PCL hybrid nanoparticles for targeted chemotherapy of glioblastoma in vivo. J Control Release (2014) 195:63–71. doi: 10.1016/j.jconrel.2014.07.054
134. Li X, Yang J, Peng L, Sahin AA, Huo L, Ward KC, et al. Triple-negative breast cancer has worse overall survival and cause-specific survival than non-triple-negative breast cancer. Breast Cancer Res Treat (2017) 161(2):279–87. doi: 10.1007/s10549-016-4059-6
135. Dent R, Trudeau M, Pritchard KI, Hanna WM, Kahn HK, Sawka CA, et al. Triple-negative breast cancer: clinical features and patterns of recurrence. Clin Cancer Res (2007) 13(15 Pt 1):4429–34. doi: 10.1158/1078-0432.CCR-06-3045
136. Sohn J, Liu S, Parinyanitikul N, Lee J, Hortobagyi GN, Mills GB, et al. Cmet activation and egfr-directed therapy resistance in triple-negative breast cancer. J Cancer (2014) 5(9):745–53. doi: 10.7150/jca.9696
137. Zagouri F, Bago-Horvath Z, Rossler F, Brandstetter A, Bartsch R, Papadimitriou CA, et al. High met expression is an adverse prognostic factor in patients with triple-negative breast cancer. Br J Cancer (2013) 108(5):1100–5. doi: 10.1038/bjc.2013.31
138. Wu Y, Fan Q, Zeng F, Zhu J, Chen J, Fan D, et al. Peptide-functionalized nanoinhibitor restrains brain tumor growth by abrogating mesenchymal-epithelial transition factor (met) signaling. Nano Lett (2018) 18(9):5488–98. doi: 10.1021/acs.nanolett.8b01879
139. Liao S, Yue W, Cai S, Tang Q, Lu W, Huang L, et al. Improvement o fgold nanorods in photothermal therapy: recent progress and perspective. Front Pharmacol (2021) 12:664123. doi: 10.3389/fphar.2021.664123
140. Li X, Lovell JF, Yoon J, Chen X. Clinical development and potential of photothermal and photodynamic therapies for cancer. Nat Rev Clin Oncol (2020) 17(11):657–74. doi: 10.1038/s41571-020-0410-2
141. Akuma P, Okagu OD, Udenigwe CC. Naturally occurring exosome vesicles as potential delivery vehicle for bioactive compounds. Front Sustain Food Syst (2019) 3.
142. Piffoux M, Silva AKA, Lugagne JB, Hersen P, Wilhelm C, Gazeau F. Extracellular vesicle production loaded with nanoparticles and drugs in a trade-off between loading, yield and purity: towards a personalized drug delivery system. Adv Biosyst (2017) 1(5):e1700044. doi: 10.1002/adbi.201700044
Keywords: extracellular vesicle, exosome, engineered EV, drug delivery, cancer
Citation: Nonaka T (2022) Application of engineered extracellular vesicles to overcome drug resistance in cancer. Front. Oncol. 12:1070479. doi: 10.3389/fonc.2022.1070479
Received: 14 October 2022; Accepted: 09 November 2022;
Published: 15 December 2022.
Edited by:
Rana Jahanban-Esfahlan, Tabriz University of Medical Sciences, IranReviewed by:
Xinlei Li, Nationwide Children’s Hospital, United StatesAdeleh Taghi Khani, City of Hope, United States
Migara Jayasinghe, National University of Singapore, Singapore
Copyright © 2022 Nonaka. This is an open-access article distributed under the terms of the Creative Commons Attribution License (CC BY). The use, distribution or reproduction in other forums is permitted, provided the original author(s) and the copyright owner(s) are credited and that the original publication in this journal is cited, in accordance with accepted academic practice. No use, distribution or reproduction is permitted which does not comply with these terms.
*Correspondence: Taichiro Nonaka, dGFpY2hpcm8ubm9uYWthQGxzdWhzLmVkdQ==