- 1Dipartimento di Farmacia (DIFARMA), Università degli Studi di Salerno, Fisciano, Italy
- 2Departamento de Biomiméticos, Instituto de Química Médica, Madrid, Spain
- 3Dipartimento di Farmacia, Università degli Studi di Napoli “Federico II”, Naples, Italy
The transient melastatin receptor potential (TRPM) ion channel subfamily functions as cellular sensors and transducers of critical biological signal pathways by regulating ion homeostasis. Some members of TRPM have been cloned from cancerous tissues, and their abnormal expressions in various solid malignancies have been correlated with cancer cell growth, survival, or death. Recent evidence also highlights the mechanisms underlying the role of TRPMs in tumor epithelial-mesenchymal transition (EMT), autophagy, and cancer metabolic reprogramming. These implications support TRPM channels as potential molecular targets and their modulation as an innovative therapeutic approach against cancer. Here, we discuss the general characteristics of the different TRPMs, focusing on current knowledge about the connection between TRPM channels and critical features of cancer. We also cover TRPM modulators used as pharmaceutical tools in biological trials and an indication of the only clinical trial with a TRPM modulator about cancer. To conclude, the authors describe the prospects for TRPM channels in oncology.
Introduction
The TRP melastatin (TRPM) channel family comprises eight members (TRPM1-8), representing the largest and most diverse subfamily of the TRP channels. TRPMs are very different in terms of selectivity, activation, and physiological functions. The members of this family share some common characteristics: 1) they are non-selective calcium-permeable cation channels, and almost all family-members conduct Ca2+; 2) they are activated by different temperatures, voltages, ion, and lipids such as phosphatidyl inositol(4,5)bisphosphate (PIP2); and 3) some of these channels are organized into cytoskeletal complexes (1–3).
TRPM proteins are subdivided based on their structural similarity into four groups: TRPM1/3, TRPM6/7, TRPM4/5, and TRPM2/8 (4) (Figure 1A). Through cryo-EM techniques, the complete structures of TRPM2, TRPM4, and TRPM8, and a partial one of TRPM7 have been determined. This allowed approaching the modality of channel assembly, ionic permeation, and modulation binding modes (5–18). Briefly (Figure 1A), TRPM proteins contain an N-terminal melastatin homology region domain (MHR), which is involved in channel assembly and external stimuli sensing (9, 13). The transmembrane domain (TMD) comprises six helices with two functional regions, the pore-forming loop between helices S5-S6, and a region in S4-S5 involved in in the activation of these channels. TMD domain is the binding site of Ca2+ in three of these channels, TRPM2, TRPM4, and TRPM8, as well as the PIP2 and other pharmacological compounds in TRPM8 (9).
TRPMs’ C-terminal domain varies greatly among members of the subfamily. C-terminal domains contain a highly conserved sequence, the TRP box, implicated in channel anchoring to the plasma membrane, and a coiled-coiled sequence (CC), which contributes to the functional tetrameric assembly (19, 20). In addition, the integrity of these C-terminal domains is required for the cold-induced opening of the TRPM8 channel (21).
After the CC sequence, TRPM2 includes an additional motif, NUDT9-H, which has high homology (50% sequence identity) to the mitochondrial NUDT9 ADPR pyrophosphatase. This region, indispensable for channel function, does not show enzymatic activity functioning as an intracellular ligand-binding site for nucleotides (22, 23). TRPM6 and TRPM7 channels contain a serine/threonine rich α-kinase substrate (S/T) domain and an α-kinase domain (KD) with an interesting enzymatic activity (see below) (24). C-terminal domain of TRPM1/TRPM3, and TRPM4 contains interaction partners for G proteins and ligand-binding sites for nucleotides, respectively (13, 25, 26).
TRPMs are involved in diversified biological processes based on regulating Ca2+ signaling, ion homeostasis, interactomes, and/or kinase activity. Many of the TRPMs are involved in cell/organ sensory transduction processes, including the perception of temperature (TRPM2, TRPM3, TRPM4, TRPM5, and TRPM8) and pain (TRPM2, TRPM3, and TRPM8) (27–32), vision (TRPM1) (33), taste (TRPM5) (27, 34), and mechanical forces (TRPM2, TRPM4, TRPM7) (2, 4, 35). Some of them are also involved in ion Mg2+ uptake and reabsorption (TRPM6 and TRPM7) (36), and besides modulate secretory processes in various cells all over the body (TRPM3, TRPM2, TRPM3, TRPM4, TRPM5) (31, 37–40). At the same time, mutations or anomalies in the expression and function of these channels could contribute to diverse pathologies, including organ dysfunction, cardiovascular and neurodegenerative disorders, other channelopathies, and cancer (41–47). In the following section, we summarize the functions and characteristics of the channels to proceed to an in-depth analysis of the main pathways modulated by the channels during the initiation and progression of neoplastic diseases.
TRPM channels: Characteristics and functions
TRPM1/TRPM3
TRPM1 and TRPM3 share 75% identical amino acids (9). TRPM1 is a non-selective channel, permeable to Ca2+, Mn2+, and Mg2+. TRPM3 shows tissue-specific permeability to mono- and divalent cations related to alternative splicing (48–50).
TRPM1 or melastatin was the first protein of this subfamily to be identified while searching for loci associated with melanoma (51). Trpm1 maps in chromosome 15 (15q13.3) and encodes five protein isoforms, containing between 1516 and 1643 amino acids, and an intronic microRNA (miR-211) co-expressed with TRPM1 proteins (52). TRPM1 is expressed mainly in melanocytes and in the retina (33, 53). The TRPM1 channel is negatively coupled to mGluR6/Go, through direct interaction with the Gβγ dimer, which, released by Gα(o) dissociation upon mGluR6 activation, closes the channel (25, 54) (Figure 1B).
TRPM3 gene, located in chromosome 9 (9q21.12-13), encodes many protein isoforms, most of which have unknown functionality yet, and, analogously to TRPM1, also encodes a microRNA (miR-204) (55–57). TRPM1 and miR-204 are co-expressed in eye cells and pancreatic βcells and are regulated by transcription factors such as Pax6 and MITF (57–59). TRPM3 is also expressed in the kidney, nociceptive neurons, and vasculature muscular layer, as well as in the brain, prostate, ovary, and in sensory bladder afferents, odontoblasts, adipocytes, ciliary body, and oral mucosa (47, 60–63).
TRPM3 is activated by natural ligands, such as sphingosine-1 sulfate, pregnenolone sulfate (PS), nifedipine, the synthetic ligand CIM0216, as well as by noxious heat (29, 31, 40). Combined stimulation by two of these ligands leads to activation of the central pore, which is permeable to Ca2+, and an alternative permeation pathway that mediated monovalent cation current and that involves the voltage-sensing domain of TRPM3 (64, 65). 17β-estradiol, progesterone, and its metabolites non-competitively inhibit TRPM3 activation, while dihydrotestosterone behaves as a PS competitive antagonist. TRPM3 activation increases [Ca2+]i, which induces activation of different kinases and transcription factors such as ERK, Raf, JNK, CREB, AP-1, Elk-1, and Egr-1 (66–69). Intracellular divalent cations such as cytosolic Ca2+ inhibit TRPM1 and TRPM3, whereas Zn2+ inhibits TRPM1 but not TRPM3, and Mg2+ inhibits TRPM3 activity (47, 70). Analogously to TRPM1, the activation of Gi/o, Gs, or Gq coupled receptors GPCRs inhibits TRPM3 via Gβγ liberation (26, 71) (Figure 1B).
TRPM2/TRPM8
TRPM2 are non-voltage-activated channels, porous to monovalent and divalent cations, such as Na+, K+, Ba2+, Ca2+, and Mg2+. TRPM2 gene is positioned on chromosome 21 (21q22.3) and encodes a 1503 amino acid protein in humans (72). Several alternative splice variants of this protein include TRPM2-S, TRPM2-ΔN, TRPM2-ΔC, TRPM2-SSF, and TRPM2-TE (73–76). TRPM2-S overexpression to suppress endogenous TRPM2 formation and activity has been used to question TRPM2 roles as a regulator of cellular functions mediated by ROS-induced Ca2+ signaling (77, 78). TRPM2 is broadly expressed in the brain, including the thalamus, cerebral cortex, hippocampus, striatum, and microglia (79–81). This channel is also detected in the heart, lung, bone marrow, liver, spleen, endometrium, placenta, gastrointestinal tract, and in different pancreatic β-like cells, the salivary gland, endothelial cells, heart, vasculature, and in immune cells (80, 82–84).
H2O2 and other agents producing reactive oxygen/nitrogen (ROS/RNS) species activate TRPM2 (85). TRPM2 promotes Ca2+ influx after activation by ROS and responds to RNS releasing adenosine diphosphate ribose (ADPR) from mitochondria and overproducing TNF-α (85, 86). This activation occurs through the direct binding of the ADPR to the channel’s enzymatic NUDT9-H domain (5, 87, 88). ADRP-induced TRPM2 activation is potentiated by [Ca2+]i and arachidonic acid, allowing TRPM2s’ response to changes in intracellular stores-released Ca2+ levels, and integrating intracellular signaling events (23, 89). TRPM2 is negatively regulated by adenosine monophosphate (AMP) and cellular acidification (85, 87, 90–93). TRPM2 is inhibited in cells external or internally exposed to pH values of 5-6 (91, 93), while divalent metal cations, Cu2+, Hg2+, and Zn2+ also inhibit TRPM2 by blocking its pore domain (94, 95) (Figure 1B).
TRPM8, one of the most studied TRPM channels, was identified during screening of a prostate cDNA library (96). TRPM8 gene is located at 2q37 and harbors genetic diversity with potential functional and phenotypic consequences (97). TRPM8 is a Ca2+-permeant but non-selective cation channel (Cs+, K+, Na+, Ba2+, Ca2+, and Mg2+) identified as the physiological sensor of environmental cold (98, 99). TRPM8 is functionally expressed in dorsal root and trigeminal ganglia in skin, teeth, the oral cavity, epithelium, tongue, nasal mucosa, and cornea (100, 101). The channel is also expressed in the colon, lung, liver, kidney, and pancreas, as well as in the male urinary, bladder, genital tracts tissues, and sperm (100, 102, 103). These gender differences in TRPM8 expression in the urinary tract have also been observed in neutrophils expressing the TRPM2 channel, which is lower in neutrophils from older women (104). TRPM8 was also detected in immune system cells, including macrophages (105, 106), bone marrow mesenchymal stem endoplasmic reticulum (ER) membranes (107), and in several areas of mouse brain (108). TRPM8, along with TRPV4, seems also to regulate microglia activities (109).
TRPM8 channels are activated by different stimuli, such as noxious to innocuous (8- 26°C) cold temperatures (28, 98, 99), membrane depolarization (110), increased extracellular osmolarity (111), and in addition by natural and synthetic cooling agents (101). TRPM8 activation/deactivation processes are regulated by post-translational modifications (112, 113), splice variants (97, 114), and modulatory regions (115). Phosphatidylinositol-4,5-bisphosphate (PIP2) changes the voltage-dependent sensitivity of the channel to cold and menthol, acting as a positive endogen TRPM8 modulator (116–118). Nuclear testosterone-androgen receptors (119), interacting proteins (120, 121), and G protein-coupled receptor signaling cascades are also involved in these inhibition/desensitization processes (106, 107). Phospholipase C (PLC) activation and subsequent hydrolysis and depletion of PIP2, or channel phosphorylation by protein kinase C (PKC) are other possible mechanisms of TRPM8 modulation (117, 118, 122, 123). TRPM8 also is inhibited by some GqPCR activation processes through the direct binding of Gαq protein to the channel (116, 124) (Figure 1B).
TRPM4/TRPM5
TRPM4 and TRPM5 are only permeable to monovalent cations and do not conduct Ca2+ (125, 126), thus differing from the other TRPM channels. TRPM4 gene is in chromosome 19 (19q13.33), and encodes a 1214 amino acid protein, which works as a voltage-modulated Ca2+-activated channel (125, 127, 128). TRPM4 is vastly expressed in the heart, colon, and prostate (125, 128), but also in the central nervous system (129), and in cells of the immunes system (130–132).
Direct activation of TRPM4 by intracellular Ca2+ leads to an influx of Na+ (133), while ATP, calmodulin, IP3, and protein kinase C-dependent phosphorylation can modify the Ca2+-induced TRPM4 activation (134, 135). TRPM4 and TRPM5 gating is also regulated by transmembrane voltage, so that depolarization causes the channel’s activation, whereas hyperpolarization deactivates it (128, 133, 136). H2O2 induces TRPM4 sustained activation resulting in an increased cell vulnerability to necrotic death (137) (Figure 1B).
TRPM5 gene, carried on chromosome 11 (11p15.5), encodes an 1165 amino acids protein (138). TRPM5 is a voltage-modulated Ca2+-activated channel that carries monovalent Na+, K+, and Cs+ ions, mediating transient membrane depolarization (127, 136). The TRPM5 channel is expressed in fetal liver, brain, and kidney, and in adult testis, prostate, colon, ovary peripheral blood leukocytes, and taste buds (79, 138). TRPM5 is likewise expressed in insulin-secreting β-cells and in the central nervous system (34, 139, 140).
TRPM5 is physiologically activated by intracellular calcium and heat (136, 141–143). Increasing temperature in the 15-35°C range greatly potentiates TRPM5 inward currents (27). TRPM5 is blocked by extracellular acidification at pH 6.2-5.9 intervals (144). Structurally, residues E830 and H934 in the S5-S6 and S3-S4 linkers are involved in the extracellular pH sensitivity of TRPM5. Interestingly, these residues are not conserved in TRPM4 (144) (Figure 1B).
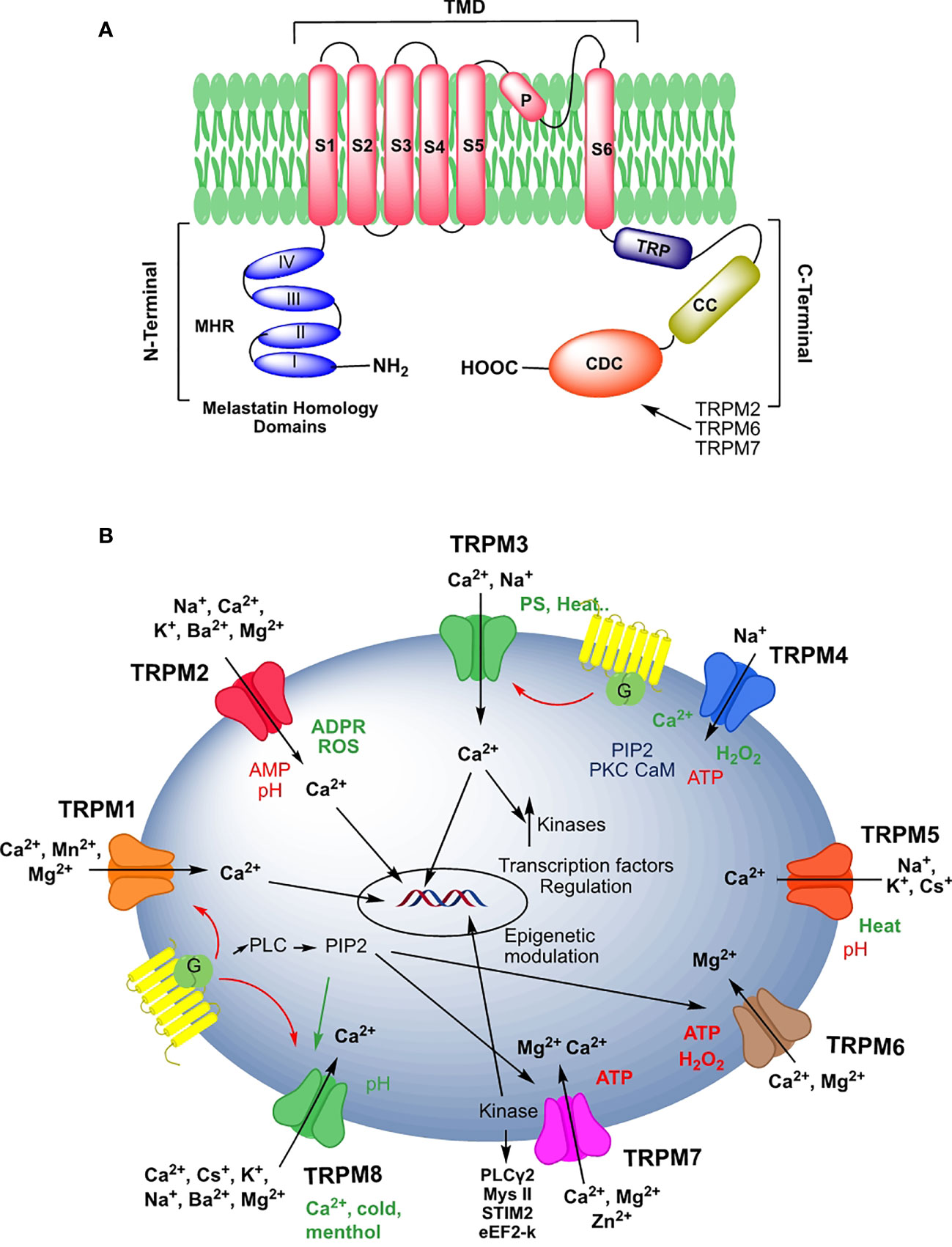
Figure 1 (A) Schematic representation of the structural domains of a monomer of the human TRPM family according to the design of Hang et al. (9). (B). Schematic illustrations of ion permeabilities and regulation processes of TRP channels. Color codes, red: channel inactivators; green: channel activators. The designs have been made using Chem Draw Professional 16.0.
TRPM6/TRPM7
TRPM6 and TRPM7 exhibit a unique dual functionality within the TRP family, acting as ion channels and kinases (145–147). TRPM6 gene, located on chromosome 9 (9q21.13) codifies for a protein of 2022 amino acids. TRPM6 is permeable to Ca2+ and Mg2+ and regulated by intracellular levels of Mg2+ (148). In this channel, the selectivity filter seems regulated by the amino acid sequence 1028GEIDVC1033, where the two negative-charged residues D1031 and E1024, are determinants for cation permeation through TRPM6 (149). TRPM6 is expressed in the intestine’s epithelial cells and in the nephron’s distal convoluted tubule (DCT) consistent with its central role in controlling Mg2+ homeostasis (79, 124, 148). TRPM6 expression is regulated by hormones such as estrogen, Ang II, and insulin (36, 150–152). Epidermal growth factor (EGF) amplifies the TRPM6 expression and activity, through the signaling pathway ERK/AP-1 (153), while uromodulin increases TRPM6 activity during Mg2+ deficiency periods (154). TRPM6-negative endogenous regulators are H2O2 and ATP-mediated P2X4 receptor signaling (155, 156) (Figure 1B).
TRPM7, also named TRP-PLIK, LTRPC7, and ChaK1, was identified by three different groups (145, 157, 158). TRPM7 gene is located on chromosome 15 (15q21.2), encoding an 1863-amino acid protein. TRPM7 is constitutively active and selectively permeable to divalent ions Mg2+, Ca2+, and traces of Zn2+ (157–160). Amino acid residues 1047EVY1049 in the pore loop provide the TRPM7 selectivity filter (161, 162). Under physiological conditions, TRPM7 inward currents are weak due to substantial downregulation of these channels by high Mg2+ or Mg·ATP concentrations. The decrease in intracellular [Mg2+]i, free or bound to ATP, increases the current carried by TRPM7 channels (158, 163). The gating of TRPM7 and TRPM6 is controlled by PIP2 (164, 165). Depletion of PIP2 after activation of PLC-linked GPCRs results in the inactivation of both channels. TRPM6 interacts with PIP2 at the TRP domain through the basic residue R1088, while in TRPM7, the specific contact residues have not been identified yet (164). However, TRPM7 is activated and not inhibited by PLC-coupled GPCR agonists (166). Takezawa et al. reported that GPCR-coupled adenylyl cyclase also enhances TRPM7 activity, and this effect is arbitrated by protein kinase A and cAMP (167).
TRPM7 activity is sensitive to pH (168), polyamines (169), mechanical stretch (170–174), osmotic gradients (175), and chloride and related halides (176). TRPM7 regulates the increase in cytosolic Zn2+ efflux induced by increased ROS, as well as blockade of cytosolic Zn2+ influx due to decreased glutathione. Oxidative stress perception as well as the release of Zn2+ from intracellular storing compartments are also TRPM7-dependent process (160).
TRPM7’s catalytic activity required Mg2+ or Mn2+ (177). In vitro, TRPM7 phosphorylates myosin II isoforms, annexin A1, tropomodulin, eukaryotic elongation factor-2 kinase (eEF2-k), phospholipase C gamma 2 (PLCγ2), and stromal interaction molecule 2 (STIM2) (178–182). TRPM7 kinase auto-phosphorylates threonine and serine residues located in its kinase substrate domain (147, 179, 183, 184). Proteolytic cleavage by caspase results in the release of the kinase domain, which potentiates T cells apoptosis induced by Fas (185). In addition, circulating kinase can translocate to the cell nucleus, where it can phosphorylate histones, thus modulating the chromatin epigenetic landscape, likely in a Zn2+-dependent mode (160, 186).
Contingent to the tissue, the structural similarity between TRPM6 and TRPM7 allows the formation of heterotetrametric complexes after the phosphorylation of TRPM7 by TRPM6 (148, 187–189). The TRPM6/7 complex differs from the homomeric TRPM6 and TRPM7 in their permeability to Ni2+, conductance, and pore structure. In these channels, the different sensitivity to low pH depends on the difference between the number of negatively charged residues in the pore domain, 7 in TRPM7 and 8 in TRPM6 (36, 157, 162). These assembled channels can modulate the pathophysiological role of both channels (43, 190) (Figure 1B).
TRPM and cancer
In this section, we discuss recent results involving TRPM channels in oncologic processes, the signaling pathways modulated by these proteins, their mechanisms, and their functions. To facilitate reading, we have summarized in Figure 2 the cancer types/TRPM channel expression (potential effects of agonists or antagonists) relationships and in Table 1 the pro/suppressive oncological functions of these channels in certain cell lines, where TRPM modulators have been used as anti-cancer pharmacological tools.
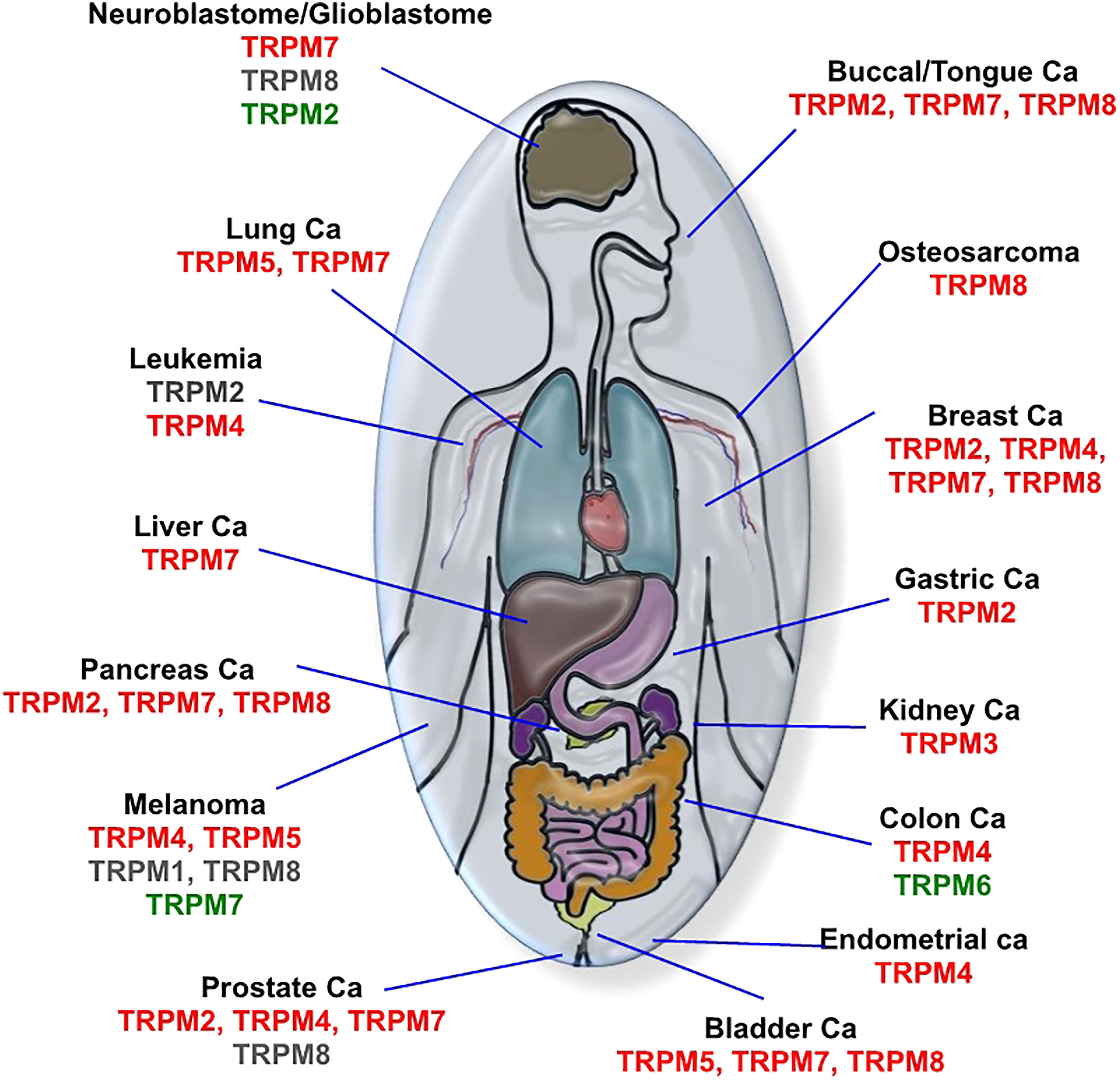
Figure 2 Relation between cancer types and TRPM channel expression. Overexpressed channels with the potential beneficial role of antagonists are indicated in red; down-regulated channels with a possible beneficial effect of agonists are in green; the controversial role of channel according to similar benefits of agonists and antagonists are in grey.
TRPM 1
Melanoma
Since its discovery, TRPM1 has been associated with malignant skin pathologies (47, 51). In normal melanocytes, TRPM1 knockdown resulted in reduced [Ca2+]i, tyrosinase activity, and intracellular melanin pigment, showing that TRPM1 is involved in Ca2+ homeostasis and melanogenesis. Induction of the p53 tumor suppressor by transfection or UVB radiation triggered inhibition of TRPM1 expression and a diminution in both intracellular Ca2+ mobilization, and extracellular Ca2+ uptake (209). TRPM1 is highly expressed in dysplastic and benign nevi, as well as in cutaneous melanomas, and variably extracted from invasive melanoma, while reduced expression was found in more advanced melanomas (210–212). According to Levi et al., the increase in the miR-211 expression reduces the invasion activity of several cutaneous malignant melanoma cell lines, by decreasing the expression of growth factor receptors IGF2R and TGFBR2, and nuclear factor of activated T-cells 5 (NFAT5) genes (213). Vemurafenib, a BRAF inhibitor, significantly augments the expression of miR-211-5p in tumor-derived extracellular vesicles (EVs) by increasing MIFT expression. Regulation of the TRPM1 gene by MIFT triggers the activation of the survival pathway (214). In melanoma cells, miR-211 transfection decreases sensitivity to vemurafenib, while in a vemurafenib-resistant cell line, inhibiting the mature form of miR-211 (miR-211-5p), diminishes cell prolifer (214). However, in patients with acral melanoma, the increased TRPM1 expression was associated with shorter survival due to tumor progression (215). TRPM1 elevates [Ca2+]-cytosolic levels and activates Ca2+/calmodulin-dependent protein kinase IIδ (CaMKIIδ), promoting AKT activation after CaMKIIδ/AKT interaction, cell mobility, colony formation, and tumor growth in an in vivo (xenograft) melanoma cell model (215).
TRPM2
TRPM2 has been implicated in several pathological pathways engaging oxidative stress (41, 89, 216–218). After activation of TRPM2 by oxidative stress, the resulting ADPR production can increase [Ca2+]i, leading to cell death by enhanced cytokines production, aggravating inflammation and tissue injury (219–223). Other authors suggested that TRPM2-induced Ca2+ entry may have a protective role for injured tissues (224, 225).
Neuroblastoma and glioblastoma
In neuroblastoma tumor cells and xenograft mice model, TRPM2 modulates both antioxidant response and ROS production, prompting cell survival (78, 216, 226, 227). TRPM2 activation results in the expression of transcription factors and kinases such as HIF-1/2α, CREB, Nrf2, Pyk2, and Src, which contribute to cell survival and proliferation (226–228). TRPM2 inhibition or depletion induces an increase in ROS by mitochondrial dysfunction, while antioxidant diminution results in cell death. Treatment of TRPM2-knockout neuroblastoma cells with doxorubicin decreased cell viability, RNAs encoding for transcription factors, E2F1/2 and FOXM1, and cell cycle regulators, including CDK1, Cyclin B1, CKS1, and PLK (229). Wild-type TRPM2 reestablished the number of living cells, as well as the expression of FOXM1, E2F1, and DNA repair proteins. On the other hand, treatment of DBTRG glioblastoma cells with selenium (Se) and docetaxel (DTX) increases markers involved in ROS production, mitochondrial membrane depolarization, and apoptosis (230). Additive therapy prompted cell death in the glioblastoma cells, via TRPM2-mediated increases in oxidative stress and [Ca2+]i. These effects were reverted after treatment with N-(p-amyl cinnamoyl) anthranilic acid (ACA, Table 1), a TRPM2 antagonist.
Leukemia
In in vitro and in vivo models of acute myeloid leukemia (AML), TRPM2 showed a mechanism of action similar to that observed in neuroblastoma. TRPM2 deletion in cells provokes a decrease in mitochondrial potential and calcium uptake, with a reduced antioxidant response (enhanced mitochondrial ROS levels and decreased Nrf2), autophagy inhibition (decreased ULK1, Atg7, and Atg5 protein levels), and bioenergetic modifications (reduced ATF4 and CREB levels). These effects inhibited leukemia proliferation and increased sensitivity to doxorubicin, an effect that was reversed after reconstitution with TRPM2 (231).
In a Trpm2(-/-) mouse model of AML driven by MLL-AF9, the loss of TRPM2 had neither a substantial effect on the progression of leukemic disease nor a synergistic effect with the cytotoxic therapy (232). These divergent results could be attributed to the models used in both experiments.
In Jurkat lymphohematopoietic cancer cells, stably expressing apoptosis-resistant Bcl-2, treatment with N-(p-amylcinnamoyl) anthranilic acid (ACA), a TRPM2 inhibitor, followed by irradiation (IR), reduced the CAMKII phosphorylation and hampered the radiation-assisted inactivation of cdc2, which is dependent on the indicated phosphorylation (233). IR stimulated a TRPM2-mediated Ca2+-entry that resulted in cell cycle arrest in G2/M, while inhibition of this channel induced cell release from the G2/M phase, promoting cell death (233).
Pancreatic cancer
The mutated TRPM2 gene is strongly associated with the survival of patients with pancreatic ductal adenocarcinoma (PDAC), compared to the standard control group. Upregulation of TRPM2 channels could stimulate pancreatic cancer processes (proliferation, migration, and invasion), independently of the tumor cell type (PANC-1, BxPC-3), and of the tumor-bearing mice model. TRPM2 level is significantly increased in PA tissues and negatively correlated to overall survival (234, 235). The action of TRPM2 may be directly due to the activation of PKCα by calcium or indirectly triggered by PKCϵ and PKCδ, through increased DAG, which most likely activates the downstream MAPK/MEK pathway, stimulating cell survival (235).
Prostate cancer
TRPM2 is markedly expressed in prostate cancer cells (PCa) compared to the normal prostate epithelium, and its expression fuels in parallel with increasing clinical tumor grade (192, 236). In PC3 cells, H2O2 addition triggers intracellular Ca2+ increase, decreased autophagy marker LC3-II, and induced apoptotic cell death (237). The TRPM2-Ca2+-CaMKII cascade is activated by oxidative stress, intensifying the production of intracellular ROS radicals, which are responsible for mitochondrial fragmentation and modification of the mitochondrial membrane potential. The inhibition of early autophagy induction was also observed, managing cell death in TRPM2-expressing tumor cells. Under oxidative stress pressure, TRPM2 knockdown cells shift from cell death to autophagy to help in cell survival (237). Silencing of TRPM2 by siRNA transfection causes a significant increase in autophagic and apoptotic gene expression (ULK1/2, AMBRA1, ATG5/10, BECN1, BAX) at the mRNA level (192). Also, the inhibition of TRPM2 by 8-Br-ADPR led to a significant reduction in the cell viability of PC3 cells (Table 1).
Breast cancer
TRPM2 was also identified in the foci of human breast cancers (MDA-MB-231 and MCF-7 cells), and inhibition with 2-APB, or silencing through RNAi, reduced tumor cell multiplication (191; Table 1). Compared to normal breast cells, TRPM2 inhibition in tumor breast cells produces a significant increase in damaged DNA levels, hypothesizing that TRPM2 activity in the nucleus could facilitate the integrity of genomic DNA, by promoting nuclear calcium influx. In MDA-MB-231 cells, TRPM2 inhibition led to cell death after treatment with doxorubicin or N-methyl-N’-nitro-N-nitrosoguanidine. Silencing TRPM2 selectively increases cell death in both MDA-MB-231 and MCF-7 cells after tamoxifen and doxorubicin treatment, respectively (191, 238). In another appealing work, Gershkovitz et al. demonstrated that the neutrophil cytotoxicity induced by H2O2 is Ca2+- and TRPM2-dependent in several tumor cell lines (239). Silencing TRPM2 in MDA-MB-231 cells showed growth retardation, utter resistance to H2O2 and neutrophil cytotoxicity, seeding metastatic tumors more efficiently.
Gastric cancers and other cancers
The survival of gastric cancer (GS) patients has negatively been correlated to the TRPM2 expression (240). In MKN-45 and AGS cells, TRPM2 knockdown increases apoptosis, and reduces cell growth and mitochondrial metabolism, as denoted by the reduction of both ATP production and mitochondrial oxygen consumption. Concomitantly, a decrease in autophagy and mitophagy-induced proteins (ATG, LC3A/B II, BNIP3) downregulated the c-Jun N- terminal kinase (JNK) pathway, causing accumulation of damaged mitochondria and gastric cancer cell’s death. Downregulation of TRPM2 also sensitized these tumor cells to chemotherapeutic agents, like doxorubicin and paclitaxel (240). These findings are consistent with earlier observations by Wang et al., which demonstrated that the activation of the TRPM2-Ca2+-CAMK2 cascade by oxidative stress resulted in phosphorylation of the BECN1 protein, which ultimately inhibited autophagy in liver cells and promotes cell death (241). TRPM2, via AKT-mediated epithelial-mesenchymal transition (EMT), also contributes to GC cell invasion and metastasis (242). TRPM2 channel silencing considerably reduced the expression of EMT markers (N- and E-cadherin, twist, and snail), while increasing tumor suppressor PTEN activities. In a mice model, the TRPM2 knockdown eradicated AGS’s tumor proliferation capacity, and produced deregulation of metastatic markers. The same results were obtained in non-small lung cancer (NSCLC) cell lines, A549 and H1299, and in a human lung tumor xenograft SCID mice model (243).
In the tongue carcinoma (SCC) cell line SCC9, it was observed an enhanced expression of TRPM2 located at the nucleus of cancer cells, in contrast with non-malignant human tongue samples. Treatment of SCC9 with H2O2 for 24 hours induced an increased number of apoptotic cells, while TRPM2 knockdown inhibited SCC9 survival and migration, independently of the apoptotic p53-p21 pathway (244).
TRPM3
TRPM3 is connected to the control of renal Ca2+ ion homeostasis (60), and its expression is upregulated in a type of renal cell carcinoma (ccRCC), with loss mutation of von Hippel-Lindau ligase (VHL, 59). VHL mutation induces a state of pseudohypoxia in the cells due to an increase in the cytoplasmic levels of hypoxia-inducible factor (HIF), which triggers autophagy (245). In human ccRCC cell lines (A498 and 786-O), TRPM3 silencing or treatment with mefenamic acid, a TRPM3 inhibitor, disrupted the formation and growth of tumors (Table 1). Mechanistically, Ca2+ influx through TRPM3 channels stimulates pro-survival autophagy, with upstream stimulation of Atg-related ULK1 protein, an essential pathway for autophagosome biogenesis, but without mTOR involvement (59). This process was negatively controlled by two VHL-regulated microRNA types (mir-204 and mir-214). VHL loss results in both miR’s lack and autophagy pathway activation (59).
TRPM4
Reduced expression of the TRPM4 channel drops the proliferation of a cervical cancer-derived HeLa cell line (246). In these cells, TRPM4 suppression stimulated the degradation of β-catenin by GSK-3β, lowering the transcription process dependent on the β-catenin/Tcf/Lef system. Compared to control transfected cells, reduced expression of TRPM4 correlates with a decrease in the number of cells in S phase, and a more significant number of cells in the G1 phase. TRPM4 knockdown reduced the expression levels of survivin and cyclin D1, while TRPM4 overexpression in T-REx 293 cells led to high β-catenin levels and increased cell proliferation (246).
Endometrial cancer
TRPM4 has been identified as a protective prognostic gene in endometrial cancer (EC) (247). Low TRPM4 expression in EC patient tissues s was associated with both worse recurrence-free survival and overall survival (248). In AN3CA cells, TRPM4 silencing significantly increases EC progression by up-regulation of mesenchymal markers, N-cadherin, and vimentin. and by decreasing cytokeratin expression, inducing cell proliferation and migration. TRPM4 silencing also leads to reduced p53 and PI3K/AKT/mTOR signaling pathways, strongly implicated in EC pathogenesis.
Prostate cancer
TRPM4 channel is among the five candidate driver genes implicated in non-hormonal prostate cancer (PCa) (249). Increased expression of TRPM4 was found in prostate cancer and prostatic intraepithelial neoplasia tissues, compared to non-malignant tissues. In fact, a higher risk of recurrence in PCa patients was associated to this TRPM4 overexpression (249–251). In prostate epithelial (PEC) and prostate cancer (DU145) cells, TRPM4 knockdown significantly increased store-operated Ca2+ entry (SOCE). In PC3 and DUA145 androgen-insensitive PCa cell lines, silencing the TRPM4 ion channel decreased cell migration, but not proliferation. Stable CRISPR/Cas9-mediated TRPM4 knockdown DU145 cells showed a rounder shape, lower proliferation, migration, and viability, as well as reduced cell adhesion. However, current TRPM4 inhibitors (CBA, LBA and NBA) did not elicit specific TRPM4 effects in DU145 cells, questioning the function of the ion conductivity of TRPM4 in PCa. In line with the HeLa study (246), decreased TRPM4 levels lead to decreased Akt1 phosphoactivation, probably impaired by an alteration in the EGFR-calcium/calmodulin axis, and by reduced GSK-3β activity. Therefore, the total levels of β-catenin protein are reduced, which, together with a decrease in the transcriptional activity of Tcf/Tcf, induces a diminished proliferation of PC3 cells (252). TRPM4 overexpression in androgen-sensitive LNCaP cells enhances the total levels of β-catenin and the phosphorylation of GSK-3β. Silencing TRPM4 in PC3 cells also decreased migration/invasion ability, via a partial reversion of the EMT process, including a decrease in the expression of Snail1, and a substantial change in the MMP9, E-cadherin/N-cadherin, and vimentin expression. Overexpressing TRPM4 in LNCaP cells increases the transcription factor Snail, promoting the repression of E-cadherin and an augment in their migration potential (253).
In PCa tissues, Hong and Yu observed higher and lower TRPM4 and miR-150 expression, respectively, EMT stimulation, as well as β-catenin signaling pathway activation. In PC-3, DU-145, BPH-1, PC-3M-2B4 and LNCaP cells, the upregulation of miR-150 led to the inactivation of the β-catenin signaling pathway (254). Furthermore, either upregulation of miR-150 or knockdown of TRPM4 suppresses proliferation, migration, invasion and EMT in vitro, while in vivo restrains tumor growth and metastasis (254).
The TRPM4 ion channel is recognized as part of the adhesoma, the protein machinery involved in focal adhesions (FAs) necessary for contractility and migration (255). Blanco et al. propose the interaction of TRPM4 with the microtubule plus-end tracking EB1 and EB2 proteins, which are required for TRPM4 trafficking and functional activity (256). Mutations or inhibition of the TRPM4-EB interaction reduced TRPM4 expression in the plasma membrane and the distribution of channels in ER. In a B16-F10 melanoma model, these mutations diminishes TRPM4-dependent focal adhesion, disassembly rates and cell invasion effects, confirming the TRPM4 channel as an adhesome component (255, 256). The effects of TRPM4 on cell migration are partially mediated by the activation of a GTPase, Rac1. Upon silencing of TRPM4, the serum-induced activation of Rac1 was significantly reduced, diminishing cellular spreading (256).
Breast cancer
TRPM4 channel is overexpressed in breast cancer. This expression was associated with EMT and estrogen response gene sets (257), and correlated to negative clinical evolution (257, 258). TRPM4 and K+ channel tetramerization domain 5 (KCTD5) protein expressions are increased in different breast cancer samples. KCTD5 positively regulates TRPM4 activity by enhancing its Ca2+ sensitivity and promoting cell migration and contractility (258). In addition, KCTD5, as a putative adaptor for the ubiquitin ligase Cullin3-E3, could also promote TRPM4 turnover through ubiquitination (259, 260). In breast cancer stem cells, Verigos et al. hypothesize the involvement of TRPM4 in cell chemoresistance, tumor recurrence, and metastasis. TRPM4 gene was overexpressed in tumorspheres enriched in breast cancer stem cells (bCSCs), and the TRPM4 gene knock-down revealed potential anti-tumor effects by directly reducing stemness properties of bCSCs in vitro (261).
Colorectal cancer
In colorectal cancer (CRC), the overexpression of TRPM4 has been related to characteristic adverse tumor patterns, such as epithelial-mesenchymal transition and hence infiltrative growth (262). In HCT116 cells, lacking p53 expression, TRPM4 acts as the primary CAN current source conducting large Na+ currents. Transient overexpression of p53 reverses this phenotype (263). Silencing TRPM4 in HCT116 resulted in an increased store-operated Ca2+ entry as well as in reduced cell viability, proliferation, and invasion, with respect to normal HCT116 cells. Knockout of TRPM4 in the same cells, also induced a shift in the cell cycle towards G1 phase, which seems to be dependent on p53 expression (262, 263). Furthermore, in prostate cells expressing endogenous p53, LNCaPs, a p53 overexpression diminished the currents mediated by TRPM4 channels (263). These authors also observed that the silencing TRPM4-mediated cell cycle switch is abolished in the event of p53 loss, indicating that TRPM4 expression is repressed by p53 (262, 263). TRPM4 regulates Ca2+-induced exocytosis in HCT116 cells. which depends on TRPM4 ion conductivity in TRPM4-containing vesicles. Both exocytosis and the delivery of TRPM4 channels to the plasma membrane are mediated by SNARE proteins (264–266). Genome sequencing of affected individuals in different families, non-bearing known CRC predisposing genes mutations, identified variants in the CYBA gene and the TRPM4 gene, leading to a premature stop codon and truncated protein (267). Functional characterization of these variants revealed that TRPM4 knockdown reduced the generation of radical oxygen species in HT-29 and LS174T cell lines, and decreased the production of MUC2 protein, an important component of the intestinal mucus barrier (267).
Leukemia
Transcriptome analysis on the impact of azacitidine treatment on four acute myeloid leukemia cell lines identified five up-regulated coding genes, among which TRPM4 is the only surface protein up-regulated (268). In MLL-rearranged leukemia cells, the knockdown of TRPM4 arrested the cell cycle at the G0/G1 phase, impairing tumoral cell growth and proliferation. The authors suggested that this channel could be involved in the regulation of the AKT/GLI1/Cyclin D1 pathway, and that it is behind the pathogenesis of this leukemia (269).
In patients with CD5+ subtypes of diffuse large B-cell lymphoma (DLBCL), the upregulation of TRPM4 mRNA was related to a poorer prognosis compared to CD5− patients (270). The TRPM4 protein was detected in epithelial cells of reactive tonsils, hyperplastic prostates (luminal epithelial cells), kidney distal tubules, and endometrial glands, but was not identified in normal B cells located in lymphoid tissues (271). In activated B cell-like of non-Hodgkin lymphoma DLBCL subtype, the TRPM4 overexpression was linked to reduced overall and progression-free survival (271).
TRPM5
Single nucleotide polymorphisms (SNP) present in TRPM5 (CG or GG genotype) have been associated with a reduced risk of suffering childhood leukemia compared to the CC genotype (272). In contrast to normal bladder, TRPM5 mRNA is considerably lower in bladder cancer tissues (273). However, TRPM5 protein was not identified in bladder tissues from cancer patients or control subjects. In public-available databanks, the increased TRPM5 mRNA expression was linked to shorter survival in gastric and melanoma cancer patients, but this type of correlation was not found in patients with colorectal, ovarian, breast, or lung cancers (194). TRPM5 protein is highly expressed in BL6 cells, a metastatic B16 melanoma variant. In silencing TRPM5 B16 melanoma cells, these authors observed a reduction of MMP-9 expression, a hallmark of solid tumors associated with EMT, induced by the acidic extracellular pH (194). Mice injected with TRMP5-overexpressed B16-BL6 cells showed an increased degree of acidic pHe-induced MMP-9 expression and lung metastasis. The crucial pHe for MMP-9 induction was not modified by genetic manipulation but merely amplified the inducible MMP-9 percentage, at each pHe (194). In mice, the treatment with triphenylphosphine oxide (TPPO), a known inhibitor of the TRPM5 channel, resulted in a significant reduction of NF-kB activities, lower expression of EMT-associated genes (Vim, Mmp9, Cdh2), and attenuation of spontaneous lung metastasis (194).
TRPM6
TRPM6 could play oncogenic/tumor suppressive roles through its ability to mediate Mg2+ homeostasis and its kinase functions (274, 275).
Colon and colorectal cancers
Colon carcinoma LoVo and doxorubicin-resistant LoVo cells showed different cytosolic Mg2+ levels. In resistant cells, the total magnesium concentration is higher, but the entry capacity is poorer, than in sensitive cells. In resistant cells, there are decreased TRPM6 and TRPM7 levels due to transcriptional regulation and post-transcriptional events, respectively (276).
In colorectal cancer (CRC), the expression of Mg2+ transporters has been investigated in numerous studies (274, 275). TRPM6 mRNA was downregulated in CRC tissues and, therefore, the high expression of TRPM6 channels in CRC patients was correlated to prolonged overall survival (277, 278). These authors also identified hsa-let-7f-1 and hsa-let-7g as the regulatory miRNAs of TRPM6. Pugliese et al. reported that TRPM6 expression was higher in inflammatory (IBD) tumor tissues than in non-IBD CRC, but these facts could not be associated with tumor stage or grade (279). Always in the digestive system, TRPM6 is expressed in the human hepatoma cell lines HepG2 and Huh-7 (280). A study by Pietropaolo et al. hypothesizes that downregulation of TRPM6 contributes to severe hypomagnesemia in cancer patients treated with the monoclonal antibody targeting EGFR, cetuximab (CTX). In human colon carcinoma CaCo-2 cells, CTX reduced the TRPM6-mediated Mg2+ influx through interference with the EGF signaling path (281).
TRPM7
One of the first studies about the implication of TRPM7 in cancer progression informs on the association of an SNP TRPM7 polymorphism (Thr1482Ile) and an increased risk of developing hyperplastic polyps or colorectal adenoma, particularly in patients with a high Ca2+/Mg2+ intake diet (282). Recent data also identified TRPM7 expression as an indicator of the predisposition to colorectal cancer (CRC) onset and progression in patients with inflammatory bowel disease (279, 283).
Prostate cancer
Compared to prostate hyperplasia cells and tissues, the TRPM7 channel is overexpressed in prostate cancer (PCa) cells and tissues and this upregulation has been correlated with poor survival of patients (284). The activation of TRPM7 channels increases the Ca2+/Mg2+ ratio in serum, promoting cell proliferation in PCa (285). Knockdown of TRPM7 in DU145 and PC3 cells suppressed migration and invasion by reversing the EMT state, thus is, downregulating EMT activators (MMP2 and MMP9) and overexpressing suppressor proteins (E-cadherin) (284). TRPM7 was also required for TGFβ‐induced EMT, which subsequently promoted cell invasion (286). Under hypoxic conditions, TRPM7 knockdown promoted proteasomal HIF-1α degradation and inhibited EMT changes in DU145 and PC3 cells. TRPM7 knockdown increased RACK1 phosphorylation, reinforcing RACK1-HIF-1α interaction, and attenuating the connection between HSP90 and HIF-1α. Deletion of both TRPM7 and HIF-1α suppressed TRPM7-HIF-1α-Annexin-1 signaling, inhibiting hypoxia-induced cell migration and invasion (287).
Pancreatic cancer
TRPM7 is essential for pancreatic ductal adenocarcinoma (PDAC) progression and invasion. The over-expression of these channels has been correlated with the increase in tumor size and the advancement of tumor stages, and hence, inversely connected to patient survival (288, 289). In PANC‐1 and MIA PaCa‐2 aggressive PDAC cells, TRPM7 channels mediated the Mg2+ entry, which has no effects on cell viability but was a requisite for cell invasion (290). This Mg2+ entry prompted heat‐shock protein 90α (Hsp90α) secretion, with the subsequent stabilization of both urokinase plasminogen and pro‐MMP2 activator pathways, stimulating the extracellular matrix degeneration and the PDAC cell invasiveness. In consequence, silencing TRPM7 in PDAC cell lines reduced cancer cell invasion (290). Analogously, in cancer stem (CSCs)-like and metastatic lung cancer cells, TRPM7 silencing induced EMT inhibition, stemness markers, phenotypes suppression, and concomitantly Hsp90α/uPA/MMP2 deregulation (196). TRPM7 channels are implicated in AG-9/VG-6-stimulated MIAPaCa-2 cell migration. Elastin-derived peptides (EDP), AG-9 and VG-6, bind to the ribosomal protein elastin receptor (RPSA), which is overexpressed in human pancreatic tumor tissues, where its interconnection with alpha-6 integrin (ITGA6) regulates the invasion process of PDAC cells (291, 292). This cell migration effect is avoided by TRPM7 channel silencing. EDP treatment did not modify TRPM7 expression but activated the channel without stimulating divalent cation influx into the cytosol. In addition. EDPs treatment induced the co-localization of the TRPM7 channel and the ribosomal protein SA (RBSA). Co-treatment with an RPSA inhibitor, EGCG, and AG-9 prevented the co-localization (292).
Breast cancer
In human breast ductal adenocarcinoma (hBDA), the high levels of TRPM7 expression have been interconnected with the Ki67 proliferation index, Scarff-Bloom-Richardson (SBR) cancer grade, and tumor size (293). High TRPM7 mRNA levels have also been linked to lower recurrence‐ and distant metastasis‐free survival. In xenografts, immunodeficient Rag2−/−IL2rg−/− mice, harboring human MDA‐MB‐231 cells, these high TRPM7 levels were required for cancer proliferation and metastasis (294). More recently, it has been described that the increased expression of TRPM7 channels predicts reduced survival in patients suffering from Luminal A breast cancer (295). The authors found that the methylation frequency of the channel has a mean of 42.7% in the whole cohort, while it is much lesser in Luminal A cancer versus other subtypes (33.3% vs. 45.7% in Luminal B, 46.9% in Her2+, and 51.3% in Basal-like). In addition, TRPM7 methylation has been negatively related to metastasis at the lymph node, disease recurrence, and final cancer-induced death. In this type of cancer, de-adhesion of cell-matrix interactions and myosin-II-based cell strains are TRPM7-dependent, and in a murine model of breast cancer, TRPM7 is required for cell metastasis into the lung (296). TRPM7 is also involved in the migration and invasion of MDA-MB-435 cells, in which the regulation of Src and MAPK kinase pathways are dependent on TRPM7 level (297). This suggests that TRPM7’s role is independent of Ca2+ entry and involves the channel α-kinase activity, which is required for the phosphorylation of myosin-IIA heavy-chain (179, 296, 297). TRPM7 maintains mesenchymal phenotype in MDA-MB-231 and Hs 578T cells by regulating the EMT transcription factor SOX4 (298). In this respect, the TRPM7 channel reduces the cytoskeletal stress by inhibiting myosin II activity, which mechanistically activates SOX4 expression and, therefore, contributes to metastatic processes of breast cancer cells (298). These results indicate the involvement of TRPM7 channels in both the alteration of mechanical adhesion dynamics and in the cytoskeletal tension reduction, which in the end resulted in increased cell migration (294, 296, 298).
Nasopharyngeal carcinoma, ovarian cancer, and neck squamous carcinoma
The TRPM7 role in breast cancer cell migration was also found in other tumors, such as ovarian cancer, and nasopharyngeal and neck squamous carcinomas (195, 299, 300). In ovarian and neck squamous carcinoma samples, the TRPM7 expression was negatively interrelated with the expression of E-cadherin, but positively correlated with N-cadherin, twist, and vimentin expression (195, 228). In ovarian cancer SKOV3 and OVCAR3 cell lines, TRPM7 depletion inhibited migration and invasion, and decreased metastasis to the lung in SKOV3 tumors, therefore prolonging the survival of mice with this type of tumors. Reduction of TRPM7 expression by treatment with MK886, a 5-lipoxygenase inhibitor, or with the intracellular calcium chelator BAPTA-AM, decreased EGF-mediated migration, invasion, and EMT/insulin-like growth factors (IGF), by decreasing the levels of [Ca2+]i. TRPM7 silencing also attenuated calcium-related PI3K/AKT activation, which was enhanced by treatment with LY2904002, a PI3K inhibitor (301). The same group proposes TRPM7 as a modulator of metabolic reprogramming pathways in ovarian cancer (195, 302–304). TRPM7 expression levels in SKOV3 and HO8910 cells were positively correlated with glycolysis-related protein (HK2, PDK1) levels, but negatively correlated with oxidative phosphorylation (OXPHOS) pathway-related protein (IDH3B, UQCRC1) levels, suggesting that in ovarian cancer TRPM7 channels promote glycolysis (195). TRPM7 knockout significantly reversed these relationships by shifting glycolysis to OXPHOS. In vitro and in vivo, silencing TRPM7 increased the activation of AMPK and stimulated the ubiquitination and degradation of HIF-1α, thus attenuating the HIF-1α-enhanced glycolysis, and inhibiting the proliferation of ovarian cancer cells (195). These outcomes may indicate that the glucose metabolic reprogramming in ovarian cancer is regulated by the TRPM7/AMPK/HIF-1α axis (195). TRPM7 silencing not only diminished the glucose uptake, but also ECAR and lactic acid production, while increased ATP, ROS, OCR levels, and NAD+/NADH ratios. Consistently, in a xenograft mice ovarian cancer model, the pharmacological inhibition of the TRPM7 activity with carvacrol also decreased the 18F-FDG uptake (195, Table 1).
TRPM7 is highly expressed in neck squamous carcinoma (HNSCC) tissues, especially in invasive tissues, and overexpressed in hypopharynx squamous carcinoma (FaDu), tongue squamous carcinoma (SAS), and buccal carcinoma (TW2.6) cell lines, which was associated with poor overall survival rates. The knockdown of TRPM7 negatively regulates the expression of genes and proteins correlated to the calcineurin/NFAT pathway (i.e. NOTCH1, NFATC3) and reduced the percentage of migrating and invasive SAS cells (305). Silencing TRPM7 significantly decreased the E-cadherin/vimentin ratio and suppressed dynamic processes, such as migration, colony and tumorsphere formation, and hence, SAS invasion. These effects involve a negative regulation of the expression of several proteins, like Snail, cyclin D1, c-Myc, SOX2, NANOG, and OCT4. TRPM7-knocked cells alone or treated with cis-platin significantly reduced colony and tumorsphere formation, compared with untreated or cis-platin-treated wild-type SAS cells (305).
Bladder cancer
Different works related TRPM7 upregulation to bladder cancer (Bca) cells’ proliferation, motility, and apoptosis (200, 201, 306). TRPM7 knockdown reduced the migration and invasion ability of UMUC3 and T24 cells by suppression of JNK (c-Jun N-terminal kinase), Akt, and Src phosphorylation. Treatment with carvacrol inhibited TRPM7 activity and restricted the tumor size in a xenograft model (201; Table 1). Another TRPM7 inhibitor, oridonin, suppressed the proliferative activity, as well as colony-formation and migration capacities of T24 cells, eliciting wide-ranging apoptosis in vitro, and delayed tumor growth in vivo (200; Table 1). On the other hand, treatment with oridonin appreciably improved p53 expression levels, increased caspase-3 activity, and reduced the expression of p-AKT, p-ERK, and TRPM7 channels.
Non-small cell lung cancer
In the non-small cell lung cancer (NSCLC) cell line A549, the TRPM7 channel is over-expressed after stimulation of epidermal growth factor (EGF), and an increased cell migration is observed. TRPM7-knockout attenuates the effects of EGF stimulation (307). In TRPM7-rich 95D cells, treatment with Waixenicin A repressed survivin, vimentin, STAT3, uPA, HSP90α, TRPM7, and MMP2 expression levels, while TRPM7-knockout enhanced the anti-CSCs effect above mentioned (196; Table 1). Luanpitpong et al. proposed an interesting TRPM7/O-GlcNAcylation regulatory axis as a potential target against lung carcinoma (308). In different cell lines and patient-derived primary cells, the inhibition of TRPM7 and of the enzyme O-GlcNAc transferase (OGT) suppresses the cells’ motility. TRPM7 inhibition also decreases Cav-1 expression, a component of the plasma membrane micro-domains, overexpressed in lung carcinoma, and connected with tumor invasiveness and patients’ poor survival. This inhibition can be reversed by activating O-GlcNAcylation, defining the TRPM7/O-GlcNAc/Cav-1 pathway. Hypo-O-GlcNAcylation of Cav-1, following post-translational TRPM7 inhibition, promotes Cav-1 ubiquitination and subsequent proteasomal degradation, a crosstalk that was also observed with c-Myc. In vitro and in experimental lung metastasis in vivo, when TRPM7 inhibition repressed O-GlcNAcylation, c-Myc and Cav-1, ubiquitination and then their proteasomal degradation increased, therefore inhibiting cell migration and invasion (308).
Samar et al. also propose the regulatory Ca2+influx/O-GlcNAcylation axis, which directly targets ITGA4 and ITGB7 human integrins, as a potential target against the motility and dissemination of myeloma (MM) cells (309). Silencing the TRPM7 channel and, therefore, inhibition of Ca2+ entry, efficiently reduced MM cells’ spreading in vivo. These results suggest a potential clinical application for both TRPM7 inhibitors and O-GlcNAcylation modulators to treat MM (309).
Liver cancer
Inhibition of the TRPM7/myocardin-related transcription factors A and B (MRTFs) axis is another promising strategy to curb hepatocellular carcinoma (HCC) growth (310). The expression of genes implicated in cell proliferation, dissemination, and differentiation is mediated by the serum response factor (SRF), which has MRTFs as coactivators (197). Using HuH7, HuH6, and TRPM7 knockout HAP1 cells, Voringer et al. showed that TRPM7 activation induces RhoA activation, and, successively, the polymerization of F-actin; permits the formation of MRTF-A-Filamin A complex and stimulates MRTF-A/SRF transcriptional activity. The authors hypothesize that the TRPM7 channel activity is necessary to increase Mg2+ concentration, required for optimal kinase activity, which is essential for both productive TRPM7-RhoA interaction and MRTF transcriptional activity. Treatment of HuH7 and HuH6 cells with NS8593, a TRPM7 blocker, strongly reduced proliferation by cell cycle arrest in G1 phase. This fact, together with the increase in senescence-associated ß-galactosidase activity, ERK phosphorylation, GTP loading of Ras, and TNFSF10 and p16INK4a expression, indicated that TRPM7 lack of function contributes to oncogene-induced senescence of hepatocarcinoma (HCC) cells. The same results were obtained in vivo in the corresponding mice model of HCC xenograft, derived from HuH7 cells (310).
Neuroblastoma and glioblastoma
In the human neuroblastoma cell line SHEP-21N, the expression of N-myc oncogene correlated with TRPM7 but not with TRPM6 mRNA expression, probably due to the few numbers of malignant tumors with significant TRPM6 expression (311). N-Myc expression is related to increased cell growth and overexpression of both TRPM6 and TRPM7 channels. N-Myc knockout SHEP-21N cells showed a basal expression of both channels, which was significantly enriched, especially that of TRPM6, by the up-regulation of N-Myc. Analyzing membrane currents, they found that the endogenous TRPM6/TRPM7 currents show decreased Mg·ATP suppression, amplified sensitivity to Mg2+, and weak sensitivity to 2-APB inhibition (311). These data support both Ca2+ and Mg2+ uptake, consistently with the increase of heteromeric TRPM6/TRPM7 channels mediated by N-Myc. Accordingly, the silencing of TRPM6/TRPM7 in these cells induced decreased cell proliferation.
In glioblastoma cells U87, treatment with the TRPM7 activator naltriben induced TRPM7‐like currents through Ca2+ influx. It did not alter cells’ viability or proliferation, but increased migration and dissemination (198; Table 1). Concomitantly, the author observed improved activation in the MAPK/ERK signaling pathway proteins but not in PI3K/AKT. Treatment of U87 cells with the TRPM7 inhibitor carvacrol decreased cell growth and viability, migration, and dissemination, and induced TRPM7-mediated apoptosis. Carvacrol may regulate dynamic cell processes through the decrease of MMP-2 protein expression and the increased levels of p-cofilin (199).
In xenograft GBM mouse models injected with U87 or U251 cells, the treatment with carvacrol showed a significantly reduced tumor size in both mice, decreased expression of the p-Akt protein, and increased levels of GSK3β (312). TRPM7 expression and activity are not only required for glioma cell proliferation and migration/invasion but also drive glioblastoma stem cells (GSC) plasticity through Notch and STAT3 proliferative activities (313–317). In A172 cells, data from a miRNA microarray analysis revealed down- and up-regulated miRNA whose transcripts are significantly changed after TRPM7 knockdown. There are two TRPM7 mutants with an inactive kinase domain, the Δkinase and the K1648R transfected glioma cells, which have reduced cell invasion, thus indicating the need for an active TRPM7 channel for glioma cell growth, while for cell migration and invasion it seems necessary a functional kinase domain. Overexpression of miR-28-5p suppressed glioma cells’ proliferation and invasion, by upregulating the target Rap1b gene (313). In addition, TRPM7 knockout in A172 glioma cells induced the regulation of a series of lncRNAs (318), of which HOX transcript antisense intergenic RNA (HOTAIR) was the most positively affected by TRPM7 depletion (319). TRPM7-mediated HOTAIR overexpression promoted glioma cell proliferation and invasion. HOTAIR exerted the oncogenic effects partially through TRPM7/HOTAIR/miR301a-3p/FOSL1 axis. TRPM7 mediates the Ca2+ influx necessary for NF-κB activation, which transcriptionally activates lncHOTAIR. In turn, lncHOTAIR directly inhibits miR-301a-3p expression, which functionally impedes FOSL1 gene expression activity. As FOSL1 is a key glioma regulator, it is expected that other TRPM7-mediated FOSL1 activations could contribute to glioma pathogenesis, other than HOTAIR and miR-301a-3p.
Differently, Xing et al. showed the mechanisms responsible for autophagy and tumorigenesis process induced by TRPM7 (320). Pharmacological and genetic TRPM7 activation disturbs lysosomes/autophagosome fusion, thus inhibiting the autophagy. TRPM7 activation umpires the releases to the cytosolic media of intracellular Zn2+, which abolishes the interaction between VAMP8 and syntaxin 17 (Stx17), two soluble N-ethylmaleimide-sensitive factor-attachment protein receptors (SNARE) located, respectively, in the lysosome and in the autophagosome, and therefore arresting autophagy flux (320). In a panel of cancer and regular cell lines, as well as in xenograft and melanoma lung-metastasis animal models, the autophagy inhibition mediated by TRPM7 activated cell death and blocked cancel cells’ metastasis in vitro. In vivo, TRPM7 activation limited tumor proliferation and metastasis by inducing ROS production, cell cycle arrest, and apoptosis (321).
TRPM8
Prostate cancer
TRPM8 is overexpressed in prostate tumors compared to non-malignant prostate tissues, and this channel is present in hormone-refractory prostate cancer with higher Gleason grading scores (96, 322, 323). In prostate cells TRPM8 gene expression is dependent on androgen. The androgen receptor (AR), when bound to an androgen (DHT), directly activates the TRPM8 gene promoter (324, 325). Biochemical findings have reported a direct TRPM8 interaction with androgens or their receptors (326). In prostate cancer LNCaP cells, while overexpression of TRPM8 transcript is observed, TRPM8 protein was internalized from its normal plasma membrane localization, ubiquitinated, and degraded via proteasomal and lysosomal pathways (327). High internalization and degradation of TRPM8 correlates with greater severity of human prostate cancer cases.
In AR+ prostatic carcinoma cell line LNCaP, silencing TRPM8 or capsazepine blockade of TRPM8 limited cell viability and provoked apoptotic nuclei formation (324). Working with different prostatic tumor cells, Valero et al. reported that TRPM8 knockdown decreased cellular proliferation and arrested cells in the G0/G1 phases, impairing cell cycle progression. The TRPM8 blockers BCTC, AMT, and JNJ41876666 reduce proliferation rates in LNCaP, PC3, and DU145 cell lines, while showing a minimal effect on proliferation in a normal prostate cell line, PNT1A (205, 328). In LNCaP and PC3 cells, TRPM8 depletion inhibited cell proliferation and promoted the chemosensitivity of these cells towards epirubicin, through an increase of p38 and JNK proteins phosphorylation (329). Over-expression of TRPM8 produces anti-proliferative, apoptotic, and anti-migratory effects in PC-3 cells, which are androgen-independent. In these cells, ectopic TRPM8 expression induced G0/G1 cell cycle arrest and expedited starvation-induced cell apoptosis through focal-adhesion kinase inactivation (330). These discrepant effects (activator or suppressor) of TRPM8 on cancer cell growth and survival should depend on the cancer cell types, the molecular phenotype, and the intermediatiors by which TRPM8 channel expression and activity are modulated, without forgetting the contribution of TRPM8 isoforms to the modulation of the whole process (331–333). Treatment of PC3 cells with menthol, a TRPM8 agonist, together with TRPM8 overexpression or AR inhibition, showed an amplified anti-proliferative effect. Another TRPM8 agonist, WS12, when encapsulated into lipid nanocapsules, impaired cancer cell migration ability (334, 335). In a prostate xenograft mouse model, the same treatment limits cell proliferation but also the spread of TRPM8-positive cells to metastatic sites by impairing both the focal adhesion via Cdc42, FAK Rac1 and ERK pathway’s inhibition, and the cytoskeleton dynamics (336).
A new TRPM8-controlled anti-invasion mechanism by direct TRPM8/small GTPase Rap1A interaction (PPI) was also described (337). In PC3 cells, the complex formed by TRPM8 and Rap1A, trapped in its GDP-bound inactive form, prevents cell migration and adhesion thus avoiding channel activation at the plasma membrane. Structurally, critical residues for the PPI interaction are Y32 in the sequence of Rap1A and E207 and Y240 in that of TRPM8. These interactions were also found in breast (MCF-7) and cervical (HeLa) cancer cell lines (337).
Alaimo et al. had already shown the positive effects induced by TRPM8 agonists in combination with radio-, hormone- or chemotherapy (208). Extracellular and intracellular stimuli, if are prolonged over time and produce minimal increases in [Ca2+]i, may be behind of the damaging cellular stress. In LNCaPFGC cells overexpressing the channel, treatment with WS-12 (48 h) in combination with either enzalutamide or docetaxel increases cell death percentage, from about 20% after single treatments to nearly 60% (208). We also found that tryptophane-derived TRPM8 agonists were able to show anti-PC potential in LNCaP cells, despite antagonists appeared more potent and reliable compounds in the same model (202).
Petrocelli et al. identified a powerful and selective tetrahydroisoquinoline-based TRPM8 antagonist, with strong antiproliferative activity in LNCaP prostate cancer cells (204). Analogously, tryptophane-derived TRPM8 antagonists inhibit proliferation in the LNCaP cell line as well as in metastatic and resistant tumor cell C4-2B, 22Rv1, and DU-CaP lines. In AR+ LNCaP, selected TRPM8 modulators mitigated migration and invasiveness (202). These effects are maintained in both LNCaP spheroids expressing AR and the castration-resistant prostate cancer (CRPC) model. This model is usually resistant to deprivation of androgen therapy (ADT) and is considered the most aggressive form of PCa. No effects were detected in PCa cells devoid of the AR receptor (202, 203). These TRPM8 antagonists interfere with AR/TRPM8 crosstalk by a non-genomic mechanism abolishing the AR/TRPM8 complex assembly, and counteracting the increase in intracellular Ca2+ levels.
To our knowledge, only a modulator of TRPM8 (D3263, Dendreon Corporation) reached a clinical trial (phase I) for the management of advanced solid tumors (NCT00839631, 101). D3263 is an orally active, TRPM8 agonist able to slow down tumor progression in advanced prostate cancer patients and in benign prostatic hyperplasia. No results have been published to date about this clinical study.
Pancreatic cancers
Pancreatic adenocarcinoma (PC) cell lines overexpressed TRPM8 channels, and this fact has been correlated to advanced TNM, vast tumor size, and distant metastasis. Patients having high TRPM8 expression show worse DFS and OS than patients with low TRPM8 levels (323, 338). In pancreatic adenocarcinoma PANC-1 and BxPC-3 cell lines, silencing TRPM8 reduced cell proliferation and showed impaired cell cycle progression, causing cells to arrest in the G1 phase and, hence, decreasing the number of cells entering S phase (339). Consistently, it was observed an increase of p21CDKN2A and p27CDKN2B cyclin-dependent kinases. However, an increase in the proportion of apoptotic cells compared to control cells was not observed, but they exhibited features of replicative senescence by inducing senescence-associated β-galactosidase (SAβG) expression. These findings indicate that the TRPM8 channel is essential for sustaining uncontrolled cancer cell growth, by regulating cell cycle phases and replicative senescence (288). TRPM8 regulation by LCK, a crucial lymphocyte-specific tyrosine kinase in regulating T-cell functions, reveals that the phosphorylation at Y1022 of the TRPM8 protein is important for pancreatic tumor cell proliferation, migration, and tumorigenesis (340). The phosphorylation process, as well as the 14-3-3ζ/TRPM8 interaction, regulates TRPM8 multi-merization and, therefore, increased current density. LCK significantly enhanced this interaction, whereas mutant TRPM8-Y1022F and knockdown of the 14-3-3ζ reduced LCK-induced TRPM8 oligomerization. In addition, the phosphorylation of TRPM8 at Y1022 inhibited, in turn, LCK Tyr505 phosphorylation, thus modulating LCK ubiquitination and activity. In AsPC-1 and PANC-1 cells stably expressing WT-TRPM8, control vector, or TRPM8-Y1022F mutant, it was observed that WT-TRPM8 considerably increased tumor cell proliferation and showed a significantly higher migration capacity in PANC-1, compared to control vector-containing cells. Diversely, the TRPM8-Y1022F mutant impaired cell proliferation and migration processes in this cell line. In vivo assays in a mice PANC-1 cell-derived xenograft model of pancreatic tumors, expressing the control vector, WT, and Y1022F-TRPM8, showed up-regulated TRPM8 mRNA expression in tumors expressing WT or Y1022F. Histopathologic analyses indicated a significant increase in tumor volumes and weights, as well as in the Ki67 antigen expression, in WT-TRPM8 tumor tissues compared to controls. In mutant TRPM8-Y1022F tissues, a smaller increase in volume, weight, and Ki67 antigen expression was observed compared to WT-TRPM8 tumors (340).
Breast cancer
In ER+ grade I breast adenocarcinomas, TRPM8 expression is normally upregulated (293, 341), with comparatively higher expression of TRPM8 mRNA in the highly invasive MDA-MB-231 cell line. In MDA-MB-231 cells, TRPM8 knockout reduces migration and invasion, decreasing the EMT-related markers (GSK-3ß phosphorylation, Snail, and Akt). Contrastingly, in the low-aggressive MCF-7 cell line, TRPM8 overexpression enhances invasion and migration, stimulating EMTs (293). TRPM8 activity might be hormone-dependent in these cells, and its expression is regulated by estrogen receptor alpha (ERα) and estrogens, and correlated with the tumors’ estrogen receptor status (293, 341, 342). TRPM8 promotes metastasis in MDA-MB-231 or MCF-7 cells by regulating epithelial-mesenchymal transition (EMT) via AKT/GSK-3β pathway activation (342, 343). However, it has also been described that TRPM8 was not expressed in MDA-MB-231 cells and that the TRPM8 transcript is absent in half of the studied breast cancer cell lines, emphasizing that the relevance of TRPM8 as a therapeutic target is very limited in this case (344).
Glioblastoma cancer
TRPM8 affects glioblastoma (GBM) cell migration rate by stimulation with specific agonists, such as menthol and icilin, which mediated a substantial increase in [Ca2+]i (345, 346), while contributes to proliferation, survival, and local tumor invasion in the case of U251 glioblastoma cells (347). In T98G and U-87MG cell lines, the activation of TRPM8 channels by icilin produced an important increase in migration speed and chemotaxis, Consistently, TRPM8 downregulation by RNAi or through a specific channel blocker, BCTC, decreases both cell migration and transfer chemotaxis (346, Table 1). The stimulation of large-conductance Ca2+-activated K+ ion channels (BK channels), could be a possible mechanism behind the cell migration in glioma, induced by TRPM8-mediated Ca2+ influx (345). TRPM8 activation by agonists increased the probable opening of single BK channels (345, 346), while TRPM8 activation and upregulation by ionizing radiation increased the Ca2+ influx (346). In human glioblastoma cells, the TRPM8 channel stimulated cell progression to the S phase, and mitosis, inducting cyclin-dependent kinase CDC2, Ca2+/calmodulin-dependent protein kinase II (CaMKII) isoforms, and phosphatase CDC25C (346). In contrast, TRPM8 knockdown or inhibition impaired cell cycle progression, DNA repair, and clonogenic survival, while inducing apoptotic cell death (346).
Osteosarcoma
The proliferative role of TRPM8 in osteosarcoma (OSS) is demonstrated in osteosarcoma cancer cells lines, MG-63 and U2OS, where TRPM8 is aberrantly over-expressed (348). TRPM8 knockdown in these cells impaired regulation of the [Ca2+]i, and decreased cyclinD1, Cdk4 expression, therefore blocking cell cycle in G0/G1, and also p-GSK-3β and p-Akt expression, inhibiting metastasis. In silencing TRPM8 cells treated with epirubicin, the inhibition of Akt-GSK-3β pathway with suppression of FAK and p44/p42 phosphorylation, amplified epirubicin-induced cell apoptosis (349). Overexpression of TRPM8 transcript and protein is concomitant to higher clinical stages, distant metastasis, and feebler disease-free survival (350).
Treatment of several osteosarcoma cell lines (143B, U2OS, HOS, and MG-63), with AMTB, a TRPM8 antagonist, results in suppressed proliferation and metastasis, and induces cellular apoptosis (207). In 143B and U2OS, incubation with AMTB for 24 h increases apoptosis rate, raising both poly (ADP-ribose) polymerase (PARP) and cleaved caspase-3 levels (207). In nude mice xenograft model, AMTB treatment augmented tumor cells’ sensitivity to cisplatin, by smodering Smad2 and Smad3 phosphorylation and, therefore, repressing the activation of TGFβ signaling, which was implicated in tumorigenesis and tumor progression (207, 351).
Squamous cell carcinoma
TRPM8 is overexpressed at the plasma membrane and in the intracellular region of oral squamous cell carcinoma (SCC), promoting the proliferation of these cancer cells. RQ-00203078, a potent and selective antagonist of TRPM8, strongly inhibits migration and invasion capability of HSC3 and HSC4 SCC lines, markedly reducing clonogenic potential and calcium entry (206; Table 1).
Recently, Huang et al. observed that the overexpression of TRPM8 channels increased basal autophagy levels, while TRPM8 knockdown reduced it, in different types of mammalian cancer cells, including cervical cancer (HeLa), breast cancer (MDA-MB-231 and MCF7), embryonic kidney (HEK293) and colorectal carcinoma (HCT116) (9). The mechanism of TRPM8 autophagy regulation involves the activation of autophagy-associated signaling proteins, AMPK and ULK1, and phagophore formation. In breast cancer cell lines, the authors hypothesized the formation through the cytoplasmic C-terminus of TRPM8 of a TRPM8-AMPK complex protein, which stimulates AMPK phosphorylation and activation, and subsequent the ULK1 activation to enhance basal autophagy (352).
Perspective
During the last decade, accumulative research indicates the close relationship between the cancer hallmarks and the deregulation of one or more ion channels. TRPM proteins are part of that family that plays a crucial role in the mechanisms of cancer cell proliferation, invasion, and survival. However, forthcoming treatments with TRPM modulators still need more comprehensive and coordinated basic and applied investigations.
We think that the recently disclosed structural data, and the new structures that undoubtedly will come soon, will aid in a more rational design of modulators. As proof, we refer to the channels with more information on structural data, including right now an open channel state (353), and a more significant number of modulators: TRPM8. This information will also be valuable in the case of TRPM6 and TRPM7 due to the complexity of their interactions and the biological effects derived.
TRPM channels may represent key support for the success of more conventional anticancer strategies such as chemotherapy and radiotherapy. By damaging target cells, these therapies trigger many stress responses, such as ion channel activation. Stimulation of sensitizing channels (TRPM2 or TRPM8) can intensify insults provoked by these therapies, whereas modulation of other channels can circumvent intrinsic chemo(radio)resistance.
Since the TRPM8 receptor is closely related to the control of core body temperature, the possibility of oral or systemic treatments based on TRPM8 antagonists is minimal, as it has been shown with compounds that have reached, but not passed, early clinical trials (354). However, TRP modulators in topical preparations can be an alternative solution. Two cosmetic preparations have recently been marketed to help in restoring the balance of nociceptors in chemotherapy-induced peripheral neuropathy, related to the overexpression of TRPM8 channels (Alodia creams, https://atikapharma.com/es/products/alodia-manos). The preparation of TRPM-based biosensors for cancer diagnosis and the development of tumor-targeted nanomedicines containing TRPM modulators, alone or in combination with known chemotherapeutic agents, could also be contemplated soon in this field.
As described in this review, several miRNA/TRP channel pairs seem to play a key role in tumor biology such as TRPM1, TRPM3, and TRPM4. New therapies based on siRNA and antisense oligonucleotides should be considered in the search for specific anti-tumor targets and modulators centered on TRPM channels (317).
Improved knowledge of the TRPM/receptors interactome as well as on the functional association between different ion channels, is another fundamental aspect to discover cellular pathways operating in TRPM-associated cancers. The search for synergy between channels/receptors/proteins or between different channels involved in the regulation of the same or complementary cancer signaling pathways can be the basis for the design of more effective TRPM modulators.
Another issue is finding the most suitable animal model to validate TRPMs as potential targets and translate efficacy and safety discoveries to human studies. It is commonly accepted that integrating relevant data from humans at the early stages of the drug discovery process strengthens the probability of success (355, 356). In this regard, the recent availability of multi-omics epidemiological tools and large-scale clinical datasets will allow a more reliable prediction of concerns related to the direct modulation of a particular target in humans (357). The efficacy and safety signal results from these “virtual” trials are likely to drive discussions about the benefits and risks for each individual TRPM channel.
In summary, the functional modulation of TRPM channels will provide promising opportunities for new antitumor agents’ development, and behind cancer, for peripheral neuropathies and other disabling diseases.
Author contributions
All authors have read and agreed to the published version of the manuscript.
Fundings
This work was supported by grants from Regione Campania-PON Campania FESR 2014-2020 “Combattere la resistenza tumorale: piattaforma integrate multidisciplinare per un approccio tecnologico innovativo alle oncoterapie-Campania Oncoterapie (Project N. B61G18000470007), and Spanish Ministry of Science and Innovation (RTI2018-097189B-C22 and PID2021-126423OB-C22).
Conflict of interest
The authors declare that the research was conducted in the absence of any commercial or financial relationships that could be construed as a potential conflict of interest.
Publisher’s note
All claims expressed in this article are solely those of the authors and do not necessarily represent those of their affiliated organizations, or those of the publisher, the editors and the reviewers. Any product that may be evaluated in this article, or claim that may be made by its manufacturer, is not guaranteed or endorsed by the publisher.
Glossary
References
2. Venkatachalam K, Montell C. TRP channels. Annu Rev Biochem (2007) 76:387–417. doi: 10.1146/annurev.biochem.75.103004.142819
3. Kuipers AJ, Middelbeek J, van Leeuwen FN. Mechanoregulation of cytoskeletal dynamics by TRP channels. Eur J Cell Biol (2012) 91:834–46. doi: 10.1016/j.ejcb.2012.05.006
4. Nilius B, Owsianik G. The transient receptor potential family of ion channels. Genome Biol (2011) 12:218. doi: 10.1186/gb-2011-12-3-218
5. Wang L, Fu TM, Zhou Y, Xia S, Greka A, Wu H. Structures and gating mechanism of human TRPM2. Science (2018) 362:eaav4809. doi: 10.1126/science.aav4809
6. Zhang Z, Toth B, Szollosi A, Chen J, Csanady L. Structure of a TRPM2 channel in complex with Ca(2+) explains unique gating regulation. Elife (2018) 7:e36409. doi: 10.7554/eLife.36409
7. Huang Y, Winkler PA, Sun W, Lu W, Du J. Architecture of the TRPM2 channel and its activation mechanism by ADP-ribose and calcium. Nature (2018) 562:45–149. doi: 10.1038/s41586-018-0558-4
8. Huang Y, Roth B, Lu BW, Du J. Ligand recognition and gating mechanism through three ligand-binding sites of human TRPM2 channel. Elife (2019) 8:e50175. doi: 10.7554/eLife.50175
9. Huang Y, Fliegert R, Guseb AH, Lüa W, Dua J. A structural overview of the ion channels of the TRPM family. Cell Calcium (2020) 85:102111. doi: 10.1016/j.ceca.2019.102111
10. Yin Y, Wu M, Zubcevic L, Borschel WF, Lander GC, Lee S-Y. Structure of the cold- and menthol-sensing ion channel TRPM8. Science (2018) 359:237–41. doi: 10.1126/science.aan4325
11. Yin Y, Le SC, Hsu AL, Borgnia MJ, Yang H, Lee S-Y. Structural basis of cooling agent and lipid sensing by the cold-activated TRPM8 channel. Science (2019) 363:eaav9334. doi: 10.1126/science.aav9334
12. Yin Y, Wu M, Hsu AL, Borschel WF, Borgnia MJ, Lander GC, et al. Visualizing structural transitions of ligand-dependent gating of the TRPM2 channel. Nat Commun (2019) 10:3740. doi: 10.1038/s41467-019-11733-5
13. Winkler PA, Huang Y, Sun W, Du J, Lu W. Electron cryo-microscopy structure of a human TRPM4 channel. Nature (2017) 552:200–4. doi: 10.1038/nature24674
14. Guo J, She J, Zeng W, Chen Q, Bai XC, Jiang Y. Structures of the calcium-activated, non-selective cation channel TRPM4. Nature (2017) 552:205–9. doi: 10.1038/nature24997
15. Autzen HE, Myasnikov AG, Campbell MG, Asarnow D, Julius D, Cheng Y. Structure of the human TRPM4 ion channel in a lipid nanodisc. Science (2018) 359:228–32. doi: 10.1126/science.aar4510
16. Diver MM, Cheng Y, Julius D. Structural insights into TRPM8 inhibition and desensitization. Science (2019) 365:1434–40. doi: 10.1126/science.aax6672
17. Duan J, Li Z, Li J, Hulse RE, Santa-Cruz A, Valinsky WC, et al. Structure of the mammalian TRPM7, a magnesium channel required during embryonic development. Proc Natl Acad Sci USA (2018) 115:E8201–10. doi: 10.1073/pnas.1810719115
18. Zhao C, Xie Y, Xu L, Ye F, Xu X, Yang W, et al. Structures of a mammalian TRPM8 in closed state. Nat Commun (2022) 13:3113. doi: 10.1038/s41467-022-30919-y
19. Jiang LH. Subunit interaction in channel assembly and functional regulation of transient receptor potential melastatin (TRPM) channels. Biochem Soc Trans (2007) 35:86–8. doi: 10.1042/BST0350086
20. Fujiwara Y, Minor DL. X-Ray crystal structure of a TRPM assembly domain reveals an antiparallel four-stranded coiled-coil. J Mol Biol (2008) 383:854–70. doi: 10.1016/j.jmb.2008.08.059
21. Díaz-Franulic I, Raddatz N, Castillo K, González-Nilo FD, Latorre R. A folding reaction at the c-terminal domain drives temperature sensing in TRPM8 channels. Proc Natl Acad Sci U S A (2020) 117:20298–304. doi: 10.1073/pnas.2004303117
22. Iordanov I, Mihályi C, Tóth B, Csanády L. The proposed channel- enzyme transient receptor potential melastatin 2 does not possess ADP ribose hydrolase activity. Elife (2016) 5:e17600. doi: 10.7554/eLife.17600
23. Belrose JC, Jackson MJ. TRPM2: a candidate therapeutic target for treating neurological diseases. Acta Pharmacol Sin (2018) 39:722–32. doi: 10.1038/aps.2018.31
24. Chubanov V, Mittermeier L, Gudermann T. Role of kinase-coupled TRP channels in mineral homeostasis. Pharmacol Ther (2018) 184:159–76. doi: 10.1016/j.pharmthera.2017.11.003
25. Shen Y, Rampino MAF, Carroll RC, Nawy S. G-Protein-mediated inhibition of the trp channel TRPM1 requires the gβγ dimer. Proc Natl Acad Sci U S A (2012) 109:8752–7. doi: 10.1073/pnas.111743310
26. Badheka D, Yudin Y, Borbiro I, Hartle CM, Yazici A, Mirshahi T, et al. Inhibition of transient receptor potential melastatin 3 ion channels by G-protein βγ subunits. Elife (2017) 6:e26147. doi: 10.7554/eLife.26147
27. Talavera K, Yasumatsu K, Voets T, Droogmans G, Shigemura N, Ninomiya Y, et al. Heat activation of TRPM5 underlies thermal sensitivity of sweet taste. Nature (2005) 438:1022–5. doi: 10.1038/nature04248e
28. Bautista DM, Siemens J, Glazer JM, Tsuruda PR, Basbaum AI, Stucky CL, et al. The menthol receptor TRPM8 is the principal detector of environmental cold. Nature (2007) 448:204–8. doi: 10.1038/nature05910
29. Vriens J, Owsianik G, Hofmann T, Philipp TE, Stab J, Chen X, et al. TRPM3 is a nociceptor channel involved in the detection of noxious heat. Neuron (2011) 70:482–94. doi: 10.1016/j.neuron.2011.02.051
30. Knowlton WM, Palkar R, Lippoldt EK, McCoy DD, Baluch F, et al. A sensory-labeled line for cold: TRPM8-expressing sensory neurons define the cellular basis for cold, cold pain, and cooling-mediated analgesia. J Neurosci (2013) 33:2837–48. doi: 10.1523/JNEUROSCI.1943-12.2013
31. Held K, Kichko T, DeClercq K, Klaassen H, VanBree R, Vanherck JC, et al. Activation of TRPM3 by a potent synthetic ligand reveals a role in peptide release. Proc Natl Acad Sci USA (2015) 112:E1363–72. doi: 10.1073/pnas.141984511
32. Tan CH, McNaughtonm PA. TRPM2 and warm sensation. Pflugers Archiv (2018) 470:787–798. doi: 10.1007/s00424-018-2139-7
33. Morgans CW, Zhang J, Jeffrey BG, Nelson SM, Burke NS, Duvoisin RM, et al. TRPM1 is required for the depolarizing light response in retinal ON-bipolar cells. Proc Natl Acad Sci USA (2009) 106:19174–8. doi: 10.1073/pnas.0908711106
34. Liman ER. Mammalian transient receptor potential (TRP) cation channels. In: Handb. exp. pharmacol, vol. 222. . Berlin, Heidelberg: Springer (2014).
35. Canales J, Morales D, Blanco C, Rivas J, Díaz N, Angelopoulos I, et al. A TR(i)P to cell migration: New roles of TRP channels in mechanotransduction and cancer. Front Physiol (2019) 10:757. doi: 10.3389/fphys.2019.00757
36. Schlingmann KP, Waldegger S, Konrad M, Chubanov V, Gudermann T. TRPM6 and TRPM7–gatekeepers of human magnesium metabolism. Biochim Biophys Acta (2007) 1772:813–21. doi: 10.1016/j.bbadis.2007.03.009
37. Philippaert K, Pironet A, Mesuere M, Sones W, Vermeiren L, Kerselaers S, et al. Steviol glycosides enhance pancreatic beta-cell function and taste sensation by potentiation of TRPM5 channel activity. Nat Commun (2017) 8:14733. doi: 10.1038/ncomms14733
38. Uchida K, Dezaki K, Damdindorj B, Inada H, Shiuchi T, Mori Y, et al. Lack of TRPM2 impaired insulin secretion and glucose metabolisms in mice. Diabetes (2011) 60:119–26. doi: 10.2337/db10-0276
39. Mathar I, Vennekens R, Meissner M, Kees F, van der Mieren ,G, et al. Increased catecholamine secretion contributes to hypertension in TRPM4-deficient mice. J Clin Invest (2010) 120:3267–79. doi: 10.1172/JCI41348
40. Wagner TFJ, Loch S, Lambert S, Straub I, Mannebach S, Mathar I, et al. Transient receptor potential M3 channels are ionotropic steroid receptors in pancreatic β cells. Nat Cell Biol (2008) 10:1421–30. doi: 10.1038/ncb1801
41. Simon F, Varela D, Cabello-Verrugio C. Oxidative stress-modulated TRPM ion channels in cell dysfunction and pathological conditions in humans. Cell Signal (2013) 25:1614–24. doi: 10.1016/j.cellsig.2013.03.023
42. Uchida K, Tominaga M. The role of TRPM2 in pancreatic β-cells and the development of diabetes. Cell Calcium (2014) 56:332–9. doi: 10.1016/j.ceca.2014.07.001
43. Chubanov V, Waldegger S, Mederos y Schnitzler M, Vitzthum H, Sassen MC, Seyberth HW, et al. Disruption of TRPM6/TRPM7 complex formation by a mutation in the TRPM6 gene causes hypomagnesemia with secondary hypocalcemia. Proc Natl Acad Sci U S A (2004) 101:2894–9. doi: 10.1073/pnas.0305252101
44. Hantute-Ghesquier A, Haustrate A, Prevarskaya N, Lehen’kyi V. TRPM family channels in cancer. Pharmaceuticals (2018) 11:58. doi: 10.3390/ph11020058. V.
45. Tai YS, Yang SC, Hsieh YC, Huang YB, Wu PC, Tsai MJ, et al. A novel model for studying voltage-gated ion channel gene expression during reversible ischemic stroke. Int J Med Sci (2019) 16:60–7. doi: 10.7150/ijms.27442
46. Hof T, Chaigne S, Récalde A, Sallé L, Brette F, Guinamard R. Transient receptor potential channels in cardiac health and disease. Na.t Rev Cardiol (2019) 16:344–60. doi: 10.1038/s41569-018-0145-2
47. Jimenez I, Prado Y, Marchant F, Otero C, Eltit F, Cabello-Verrugio C, et al. TRPM channels in human diseases. Cells (2020) 9:2604. doi: 10.3390/cells9122604
48. Owsianik G, Talavera K, Voets T, Nilius B. Permeation and selectivity of TRP channels. Annu Rev Physiol (2006) 68:685–717. doi: 10.1146/annurev.physiol.68.040204.101406
49. Oberwinkler J, Lis A, Giehl KM, Flockerzi V, Philipp SE. Alternative splicing switches the divalent cation selectivity of TRPM3 channels. J Biol Chem (2005) 280:22540–8. doi: 10.1074/jbc.M503092200
50. Lee N, Chen J, Sun L, Wu S, Gray KR, Rich A, et al. Expression and characterization of human transient receptor potential melastatin 3 (hTRPM3). J Biol Chem (2003) 278:20890–7. doi: 10.1074/jbc.M211232200
51. Duncan LM, Deeds J, Hunter J, Shao J, Holmgren LM, Woolf EA, et al. Down-regulation of the novel gene melastatin correlates with potential for melanoma metastasis. Cancer Res (1998) 58:1515–20.
52. Hunter JJ, Shao J, Smutko JS, Dussault BJ, Nagle DL, Woolf EA, et al. Chromosomal localization and genomic characterization of the mouse melastatin gene (Mlsn1). Genomics (1998) 5:116–23. doi: 10.1006/geno.1998.5549
53. Devi S, Markandeya Y, Maddodi N, Dhingra A, Vardi N, Balijepalli RC, et al. Metabotropic glutamate receptor 6 signaling enhances TRPM1 calcium channel function and increases melanin content in human melanocytes. Pigment Cell Melanoma Res (2013) 26:348–56. doi: 10.1111/pcmr.12083
54. Koike C, Obara T, Uriu Y, Numata T, Sanuki R, Miyata K, et al. TRPM1 is a component of the retinal ON bipolar cell transduction channel in the mGluR6 cascade. Proc Natl Acad Sci USA (2010) 107:332–7. doi: 10.1073/pnas.0912730107
55. Rodriguez A. Identification of mammalian microRNA host genes and transcription units. Genome Res (2004) 14:1902–10. doi: 10.1101/gr.2722704
56. Oberwinkler J, Philipp SE. TRPM3. Handb. Exp Pharmacol (2014) 222:427–59. doi: 10.1007/978-3-642-54215-2_17
57. Shiels A. TRPM3_miR-204: a complex locus for eye development and disease. Hum Genom (2020) 14:7. doi: 10.1186/s40246-020-00258-4
58. Shaham O, Gueta K, Mor E, Oren-Giladi P, Grinberg D, Xie Q, et al. Pax6 regulates gene expression in the vertebrate lens through miR-204. PloS Genet (2013) 9:e1003357. doi: 10.1371/journal.pgen.1003357
59. Hall DP, Cost NG, Hegde S, Kellner E, Mikhaylova O, Stratton Y, et al. TRPM3 and miR-204 establish a regulatory circuit that controls oncogenic autophagy in clear cell renal cell carcinoma. Cancer Cell (2014) 26:738 –753. doi: 10.1016/j.ccell.2014.09.015
60. Grimm C, Kraft R, Sauerbruch S, Schultz G, Harteneck C. Molecular and functional characterization of the melastatin-related cation channel TRPM3. J Biol Chem (2003) 278:21493–501. doi: 10.1074/jbc.M300945200
61. Hoffmann A, Grimm C, Kraft R, Goldbaum O, Wrede A, Nolte C, et al. TRPM3 is expressed in sphingosine-responsive myelinating oligodendrocytes. J Neurochem (2010) 114:654–65. doi: 10.1111/j.1471-4159.2010.06644.x
62. Vangeel L, Benoit M, Miron Y, Miller PE, De Clercq K, Chaltin P, et al. Functional expression and pharmacological modulation of TRPM3 in human sensory neurons. Br J Pharmacol (2020) 177:2683–95. doi: 10.1111/bph.14994
63. Vanneste M, Mulier M, Nogueira Freitas AC, Van Ranst N, Kerstens A, Voets T, et al. TRPM3 is expressed in afferent bladder neurons and is upregulated during bladder inflammation. Int J Mol Sci (2022) 23(1):107. doi: 10.3390/ijms23010107
64. Vriens J, Held K, Janssens A, Tóth BI, Kerselaers S, Nilius B, et al. Opening of an alternative ion permeation pathway in a nociceptor TRP channel. Nat Chem Biol (2014) 10:188–95. doi: 10.1038/nchembio.1428
65. Held K, Gruss F, Aloi VD, Janssens A, Ulens C, Voets T, et al. Mutations in the voltage-sensing domain affect the alternative ion permeation pathway in the TRPM3 channel. J Physiol (2018) 596:2413–32. doi: 10.1113/JP274124
66. Thiel G, Rubil S, Lesch A, Guethlein LA, Rössler OG. Transient receptor potential TRPM3 channels: Pharmacology, signaling, and biological functions. Pharmacol Res (2017) 124:92–9. doi: 10.1016/j.phrs.2017.07.014
67. Lesch A, Rubil S, Thiel G. Activation and inhibition of transient receptor potential TRPM3-induced gene transcription. Br J Pharmacol (2014) 171:2645–58. doi: 10.1111/bph.12524
68. Mayer SI, Müller I, Mannebach S, Endo T, Thiel G. Signal transduction of pregnenolone sulfate in insulinoma cells: Activation of egr-1 expression involving TRPM3, voltage-gated calcium channels, ERK, and ternary complex factors. J Biol Chem (2011) 286:10084–96. doi: 10.1074/jbc.M110.202697
69. Lesch A, Rössler OG, Thiel G. Extracellular signal-regulated protein kinase, c-jun n-terminal protein kinase, and calcineurin regulate transient receptor potential M3 (TRPM3) induced activation of AP-1. J Cell Biochem (2017) 118:2409–19. doi: 10.1002/jcb.25904
70. Lambert S, Drews A, Rizun O, Wagner TFJ, Lis A, Mannebach S, et al. Transient receptor potential melastatin 1 (TRPM1) is an ion-conducting plasma membrane channel inhibited by zinc ions. J Biol Chem (2011) 286:12221–33. doi: 10.1074/jbc.M110.202945
71. Alkhatib O, da Costa R, Gentry C, Quallo T, Bevan S, Andersson DA. Promiscuous G-protein-coupled receptor inhibition of transient receptor potential melastatin 3 ion channels by gβγ subunits. J Neurosci (2019) 39:7840–52. doi: 10.1523/JNEUROSCI.0882-19.2019
72. Nagamine K, Kudoh J, Minoshima S, Kawasaki K, Asakawa S, Ito F, et al. Molecular cloning of a novel putative Ca2+ channel protein (TRPC7) highly expressed in brain. Genomics (1998) 54:124–31. doi: 10.1006/geno.1998.5551
73. Orfanelli U, Wenke AK, Doglioni C, Russo V, Bosserhoff AK, Lavorgna G. Identification of novel sense and antisense transcription at the TRPM2 locus in cancer. Cell Res (2008) 18:1128–40. doi: 10.1038/cr.2008.296
74. Uemura T, Kudoh J, Noda S, Kanba S, Shimizu N. Characterization of human and mouse TRPM2 genes: identification of a novel n-terminal truncated protein specifically expressed in human striatum. Biochem Biophys Res Commun (2005) 328:1232–43. doi: 10.1016/j.bbrc.2005.01.086
75. Zhang W, Chu X, Tong Q, Cheung JY, Conrad K, Masker K, et al. A novel TRPM2 isoform inhibits calcium influx and susceptibility to cell death. J Biol Chem (2003) 278:16222–9. doi: 10.1074/jbc.M300298200
76. Wehage E, Eisfeld J, Heiner I, Jüngling E, Zitt C, Lückhoff A. Activation of the cation channel long transient receptor potential channel 2 (LTRPC2) by hydrogen peroxide. a splice variant reveals a mode of activation independent of ADP-ribose. J Biol Chem (2002) 277:23150–6. doi: 10.1074/jbc.M112096200
77. Hecquet CM, Zhang M, Mittal M, Vogel SM, Di A, Gao X, et al. Cooperative interaction of trp melastatin channel transient receptor potential (TRPM2) with its splice variant TRPM2 short variant is essential for endothelial cell apoptosis. Circ Res (2014) 114:469–79. doi: 10.1161/CIRCRESAHA.114.302414
78. Hirschler-Laszkiewicz I, Chen SJ, Bao L, Wang J, Zhang XQ, Shanmughapriya S, et al. The human ion channel TRPM2 modulates neuroblastoma cell survival and mitochondrial function through Pyk2, CREB, and MCU activation. Am J Physiol Cell Physiol (2018) 315:C571–86. doi: 10.1152/ajpcell.00098.2018
79. Fonfria E, Murdock PR, Cusdin FS, Benham CD, Kelsell RE, McNulty S. Tissue distribution profiles of the human TRPM cation channel family. J Recept. Signal Transduction Res (2006) 26:159–78. doi: 10.1080/10799890600637506
80. Faouzi M, Penner R. Mammalian transient receptor potential (TRP) cation channels. In: Nilius B, Flockerzi V, editors. Handb. exp. pharmacol, vol. 222 . Berlin, Heidelberg: Springer (2014).
81. Li X, Jiang LH. Multiple molecular mechanisms form a positive feedback loop driving amyloid β42 peptide-induced neurotoxicity via activation of the TRPM2 channel in hippocampal neurons. Cell Death Dis (2018) 9:195. doi: 10.1038/s41419-018-0270-1
82. Yamamoto S, Shimizu S. Targeting TRPM2 in ROS-coupled diseases. Pharmaceuticals (2016) 9:57. doi: 10.3390/ph9030057
83. Manna PT, Munsey TS, Abuarab N, Li F, Asipu A, Howell G, et al. TRPM2-mediated intracellular Zn2+ release triggers pancreatic β-cell death. Biochem J (2015) 466:537–46. doi: 10.1042/BJ20140747
84. Abuarab N, Munsey TS, Jiang LH, Li J, Sivaprasadarao A. High glucose-induced ROS activates TRPM2 to trigger lysosomal membrane permeabilization and Zn2+-mediated mitochondrial fission. Sci Signal (2017) 10:eaal4161. doi: 10.1126/scisignal.aal4161
85. Perraud AL, Fleig A, Dunn CA, Bagley LA, Launay P, Schmitz C, et al. ADP-ribose gating of the calcium-permeable LTRPC2 channel revealed by nudix motif homology. Nature (2001) 411:595–9. doi: 10.1038/35079100
86. Harrision D, Gravells P, Thompson R, Bryant HE. Poly(ADP-ribose) glycohydrolase (PARG) vs. poly(ADP-ribose) polymerase (PARP) – function in genome maintenance and relevance of inhibitors for anti-cancer therapy. Front Mol Biosci (2020) 2020:191. doi: 10.3389/fmolb.2020.00191
87. Kolisek M, Beck A, Fleig A, Penner R. Cyclic ADP-ribose and hydrogen peroxide synergize with ADP-ribose in the activation of TRPM2 channels. Mol Cell (2005) 18:61–9. doi: 10.1016/j.molcel.2005.02.033
88. Fliegert R, Watt JM, Schöbel A, Rozewitz MD, Moreau C, Kirchberger T, et al. Ligand-induced activation of human TRPM2 requires the terminal ribose of ADPR and involves Arg1433 and Tyr1349. Biochem J (2017) 474:2159–75. doi: 10.1042/BCJ20170091
89. Jiang LH, Li X, Syed Mortadza SA, Lovatt M, Yang W. The TRPM2 channel nexus from oxidative damage to alzheimer’s pathologies: An emerging novel intervention target for age-related dementia. Ageing Res Rev (2018) 47:67–79. doi: 10.1016/j.arr.2018.07.002
90. Yang W, Zou J, Xia R, Vaal ML, Seymour VA, Luo J, et al. State-dependent inhibition of TRPM2 channel by acidic pH. J Biol Chem (2010) 285:30411–8. doi: 10.1074/jbc.M110.139774
91. Starkus JG, Fleig A, Penner R. The calcium-permeable non-selective cation channel TRPM2 is modulated by cellular acidification. J Physiol (2010) 588:1227–40. doi: 10.1113/jphysiol.2010.187476
92. Lange I, Penner R, Fleig A, Beck A. Synergistic regulation of endogenous TRPM2 channels by adenine dinucleotides in primary human neutrophils. Cell Calcium (2008) 44:604–15. doi: 10.1016/j.ceca.2008.05.001
93. Du J, Xie J, Yue L. Modulation of TRPM2 by acidic pH and the underlying mechanisms for pH sensitivity. J Gen Physiol (2009) 134:471–88. doi: 10.1085/jgp.200910254
94. Zeng B, Chen G-L, Xu S-Z. Divalent copper is a potent extracellular blocker for TRPM2 channel. Biochem Biophys Res Commun (2012) 424:279–84. doi: 10.1016/j.bbrc.2012.06.107
95. Yang W, Manna PT, Zou J, Luo J, Beech DJ, Sivaprasadarao A, et al. Zinc inactivates melastatin transient receptor potential 2 channels via the outer pore. J Biol Chem (2011) 286:23789–98. doi: 10.1074/jbc.M111.247478
96. Tsavaler L, Shapero MH, Morkowski S, Laus R. Trp-p8, a novel prostate-specific gene,is up-regulated in prostate cancer and other malignancies and shares high homology with transient receptor potential calcium channel proteins. Cancer Res (2001) 61:3760–9.
97. Blanquart S, Borowiec A, Delcourt P, Figeac M, Emerling CA, Meseguer AS, et al. Evolution of the human cold/menthol receptor. TRPM8. Mol Phylogenet Evol (2019) 136:104–18. doi: 10.1016/j.ympev.2019.04.011
98. Peier AM, Moqrich A, Hergarden AC, Reeve AJ, Andersson DA. A TRP channel that senses cold stimuli and menthol. Cell (2002) 108:705–15. doi: 10.1016/s0092-8674(02)00652-9
99. McKemy DD, Neuhausser WM, Julius D. Identification of a cold receptor reveals a general role for TRP channels in thermosensation. Nature (2002) 416:52–8. doi: 10.1038/nature719
100. Almaraz L, Manenschijn JA, de la Peña E, Viana F. TRPM8. Handb. Exp Pharmacol (2014) 222:547–79. doi: 10.1007/978-3-642-54215-2_22
101. Izquierdo C, Martín-Martínez M, Gómez-Monterrey IM, González-Muñiz R. TRPM8 channels: Advances in structural studies and pharmacological modulation. Int J Mol Sci (2021) 22:8502. doi: 10.3390/ijms22168502
102. Vanneste M, Segal A, Voets T, Everaerts W. Transient receptor potential channels in sensory mechanisms of the lower urinary tract. Nat Rev Urol (2021) 18:139–59. doi: 10.1038/s41585-021-00428-6
103. Amato A, Terzo S, Lentini L, Marchesa P, Mule F. TRPM8 channel activation reduces the spontaneous contractions in human distal colon. Int J Mol Sci (2020) 21:5403. doi: 10.3390/ijms21155403
104. Vaízquez-Prieto MA, Lascurais-Santamariía N, Fernaíndez-Eufrasio NB, Montiel-Condado D, Garibay-Escobar A, et al. Sex-dependent effect of aging on calcium signaling and expression of TRPM2 and CRAC channels in human neutrophils. Hum Immunol (2022) 83:645–55. doi: 10.1016/j.humimm.2022.05.002
105. Khalil M, Alliger K, Weidinger C, Yerinde C, Wirtz S, Becker C, et al. Functional role of transient receptor potential channels in immune cells and epithelia. Front Immunol (2018) 9:174. doi: 10.3389/fimmu.2018.00174
106. Hornsby E, Peiris M, Peiris M, King HW, Wing ES, Lindsay JO, et al. P016 constitutive activity of the cation channel TRPM8 regulates monocyte to macrophage transition in humans to control intestinal inflammation. J Crohn’s Colitis (2019) 13:S094. doi: 10.1093/ecco-jcc/jjy222.140
107. Bidaux G, Gordienko D, Shapovalov G, Farfariello V, Borowiec A-S, Iamshanova O, et al. 4TM-TRPM8 channels are new gatekeepers of the ER-mitochondria Ca2+ transfer. Biochim Biophys Acta Mol Cell Res (2018) 1865:981–94. doi: 10.1016/j.bbamcr.2018.04.007
108. Ordás P, Hernández-Ortego P, Vara H, Fernández-Peña C, Reimúndez A, Morenilla-Palao C, et al. Expression of the cold thermoreceptor TRPM8 in rodent brain thermoregulatory circuits. J Comp Neurol (2021) 529:234–56. doi: 10.1038/cr.2008.296
109. Chakraborty R, Goswami C. Both heat-sensitive TRPV4 and cold-sensitive TRPM8 ion channels regulate microglial activity. Biochem Biophys Res Commun (2022) 611:132–9. doi: 10.1016/j.bbrc.2022.04.032
110. Raddatz N, Castillo JP, Gonzalez C, Alvarez O, Latorre R. Temperature and voltage coupling to channel opening in transient receptor potential melastatin 8 (TRPM8). J Biol Chem (2014) 289:35438–54. doi: 10.1074/jbc.M114.612713
111. Quallo T, Vastani N, Horridge E, Gentry C, Parra A, Moss S, et al. TRPM8 is a neuronal osmosensor that regulates eye blinking in mice. Nat Commun (2015) 6:7150. doi: 10.1038/ncomms8150
112. Cao C, Yudin Y, Bikard Y, Chen W, Liu T, Li H, et al. Polyester modification of the mammalian trpm8 channel protein: Implications for structure and function. Cell Rep (2013) 4:302–15. doi: 10.1016/j.celrep.2013.06.022
113. Pertusa M, Madrid R, Morenilla-Palao C, Belmonte C, Viana F. N-glycosylation of TRPM8 ion channels modulates temperature sensitivity of cold thermoreceptor neurons. J Biol Chem (2012) 287:18218–29. doi: 10.1074/jbc.M111.312645
114. Bidaux G, Beck B, Zholos A, Gordienko D, Flourakis M, Roudbaraki M, et al. Regulation of activity of transient receptor potential melastatin 8 (TRPM8) channel by its short isoforms. J Biol Chem (2012) 287:2948–62. doi: 10.1074/jbc.M111.270256
115. Pertusa M, González A, Hardy P, Madrid R, Viana F. Bidirectional modulation of thermal and chemical sensitivity of TRPM8 channels by the initial region of the n-terminal domain. J Biol Chem (2014) 289:21828–43. doi: 10.1074/jbc.M114.565994
116. Liu L, Rohacs T. Regulation of the cold-sensing TRPM8 channels by phosphoinositides and gq-coupled receptors. Channels (2020) 14:79–86. doi: 10.1080/19336950.2020.1734266
117. Daniels RL, Takashima Y, McKemy DD. Activity of the neuronal cold sensor TRPM8 is regulated by phospholipase c via the phospholipid phosphoinositol 4,5-bisphosphate. J Biol Chem (2009) 284:1570–82. doi: 10.1074/jbc.M807270200
118. Rohács T, Lopes CMB, Michailidis I, Logothetis DE. PI(4,5)P2 regulates the activation and desensitization of TRPM8 channels through the TRP domain. Nat Neurosci (2005) 8:626–34. doi: 10.1038/nn1451
119. Gkika D, Lolignier S, Grolez GP, Bavencoffe A, Shapovalov G, Gordienko D, et al. Testosterone-androgen receptor: The steroid link inhibiting TRPM8-mediated cold sensitivity. FASEB J (2020) 34:7483–99. doi: 10.1096/fj.201902270R
120. Tang Z, Kim A, Masuch T, Park K, Weng H, Wetzel C, et al. Pirt functions as an endogenous regulator of TRPM8. Nat Commun (2013) 4:217. doi: 10.1038/ncomms3179
121. Zhang X. Direct gαq gating is the sole mechanism for TRPM8 inhibition caused by bradykinin receptor activation. Cell Rep (2019) 27:3672–3683.e4. doi: 10.1016/j.celrep.2019.05.080
122. Manolache A, Selescu T, Maier GL, Neacsu C, Babes A, et al. Regulation of TRPM8 channel activity by src-mediated tyrosine phosphorylation. J Cell Physiol (2020) 235:5192–203. doi: 10.1002/jcp.29397
123. Yudin Y, Lukacs V, Cao C, Rohacs T. Decrease in phosphatidylinositol 4,5-bisphosphate levels mediates desensitization of the cold sensor TRPM8 channels. J Physiol (2011) 589:6007–27. doi: 10.1113/jphysiol.2011.220228
124. Luongo F, Pietropaolo G, Gautier M, Dhennin-Duthille I, Ouadid-Ahidouch H, Wolf FI, et al. TRPM6 is essential for magnesium uptake and epithelial cell function in the colon. Nutrients (2018) 10:784. doi: 10.3390/nu10060784
125. Launay P, Fleig A, Perraud AL, Scharenberg AM, Penner R, Kinet JP. TRPM4 is a Ca2+-activated nonselective cation channel mediating cell membrane depolarization. Cell (2002) 109:39. doi: 10.1016/s0092-8674(02)00719-5
126. Guinamard R, Sallé L, Simard C. The non-selective monovalent cationic channels TRPM4 and TRPM5. Adv Exp Med Biol (2011) 704:147–71. doi: 10.1007/978-94-007-0265-3_8
127. Ullrich ND, Voets T, Prenen J, Vennekens R, Talavera K, Droogmans G, et al. Comparison of functional properties of the Ca2+-activated cation channels TRPM4 and TRPM5 from mice. Cell Calcium (2005) 37:267–78. doi: 10.1016/j.ceca.2004.11.001
128. Nilius B, Prenen J, Droogmans G, Voets T, Vennekens R, Freichel M, et al. Voltage dependence of the Ca2+-activated cation channel TRPM4. J Biol Chem (2003) 278:30813–20. doi: 10.1074/jbc.M305127200
129. Earley S, Waldron BJ, Brayden JE. Critical role for transient receptor potential channel TRPM4 in myogenic constriction of cerebral arteries. Circ Res (2004) 95:922–9. doi: 10.1161/01.RES.0000147311.54833.03
130. Vennekens R, Olausson J, Meissner M, Bloch W, Mathar I, Philipp SE, et al. Increased IgE-dependent mast cell activation and anaphylactic responses in mice lacking the calcium-activated nonselective cation channel TRPM4. Nat Immunol (2007) 8:312–20. doi: 10.1038/ni1441
131. Weber KS, Hildner K, Murphy KM, Allen PM. Trpm4 differentially regulates Th1 and Th2 function by altering calcium signaling and NFAT localization. J Immunol (2010) 185:2836–46. doi: 10.4049/jimmunol.1000880
132. Barbet G, Demion M, Moura IC, Serafini N, Léger T, Vrtovsnik F, et al. The calcium-activated nonselective cation channel TRPM4 is essential for the migration but not the maturation of dendritic cells. Nat Immunol (2008) 9:1148–56. doi: 10.1038/ni.1648
133. Mathar I, Jacobs G, Kecskes M, Menigoz A, Philippaert K, Vennekens R. TRPM4. in: Nilius, b., flockerzi, v. (eds) mammalian transient receptor potential (TRP) cation channels. In: Handb. exp. pharmacol, vol. 222. . Berlin, Heidelberg: Springer (2014).
134. Nilius B, Prenen J, Tang J, Wang C, Owsianik G, Janssens A, et al. Regulation of the Ca2+ sensitivity of the nonselective cation channel TRPM4. J Biol Chem (2005) 280:6423–33. doi: 10.1074/jbc.M411089200
135. Zhang Z, Okawa H, Wang Y, Liman ER. Phosphatidylinositol 4,5-bisphosphate rescues TRPM4 channels from desensitization. J Biol Chem (2005) 280:39185–92. doi: 10.1074/jbc.M506965200
136. Hofmann T, Chubanov V, Gudermann T, Montell C. TRPM5 is a voltage-modulated and Ca2+-activated monovalent selective cation channel. Curr Biol (2003) 13:1153–8. doi: 10.1016/s0960-9822(03)00431-7
137. Simon F, Leiva-Salcedo E, Armisen R, Riveros A, Cerda O, Varela D, et al. Hydrogen peroxide removes TRPM4 current desensitization conferring increased vulnerability to necrotic cell death. J Biol Chem (2010) 285:37150–8. doi: 10.1074/jbc.M110.155390
138. Prawitt D, Enklaar T, Klemm G, Gärtner B, Spangenberg C, Winterpacht A, et al. Identification and characterization of MTR1, a novel gene with homology to melastatin (MLSN1) and the trp gene family located in the BWS-WT2 critical region on chromosome 11p15.5 and showing allele specific expression. Hum Mol Genet (2000) 9:203–16. doi: 10.1093/hmg/9.2.203
139. Kim YS, Kang E, Makino Y, Park S, Shin JH. Characterizing the conductance underlying depolarization-induced slow current in cerebellar purkinje cells. J Neurophysiol (2012) 109:1174–81. doi: 10.1152/jn.01168.2011
140. Kaske ,S, Krasteva G, Konig P, Kummer W, Hofmann T, Gudermann T, et al. TRPM5, a taste-signaling transient receptor potential ion-channel, is a ubiquitous signaling component in chemosensory cells. BMC Neurosci (2007) 8:49. doi: 10.1186/1471-2202-8-49
141. Zhang Z, Zhao Z, Margolskee R, Liman E. The transduction channel TRPM5 is gated by intracellular calcium in taste cells. J Neurosci (2007) 27:5777–86. doi: 10.1523/JNEUROSCI.4973-06.2007
142. Prawitt D, Monteilh-Zoller MK, Brixel L, Spangenberg C, Zabel B, Fleig A, et al. TRPM5 is a transient Ca2+-activated cation channel responding to rapid changes in [Ca2+]i. Proc Natl Acad (2003) 100:15166–71. doi: 10.1073/pnas.2334624100
143. Liu D, Liman ER. Intracellular Ca2+ and the phospholipid PIP2 regulate the taste transduction ion channel TRPM5. Proc Natl Acad Sci USA (2003) 100:15160–5. doi: 10.1073/pnas.2334159100
144. Liu D, Zhang Z, Liman ER. Extracellular acid block and acid-enhanced inactivation of the Ca2+-activated cation channel TRPM5 involve residues in the S3-S4 and S5-S6 extracellular domains. J Biol Chem (2005) 280:20691–9. doi: 10.1074/jbc.M414072200
145. Ryazanov G, Ward MD, Mendola CE, Pavur KS, Dorovkov MV, Wiedmann M, et al. Identification of a new class of protein kinases represented by eukaryotic elongation factor-2 kinase. Proc Natl Acad Sci USA (1997) 94:4884–9. doi: 10.1073/pnas.94.10.4884
146. Riazanova LV, Pavur KS, Petrov AN, Dorovkov MV, Ryazanov AG. Novel type of signaling molecules: Protein kinases covalently linked to ion channels. Mol Biol (2001) 35:271–83. doi: 10.1023/A:1010499720185
147. Drennan D, Ryazanov AG. Alpha-kinases: analysis of the family and comparison with conventional protein kinases. Prog Biophys Mol Biol (2004) 85:1–32. doi: 10.1016/S0079-6107(03)00060-9
148. Voets T, Nilius B, Hoefs S, van der Kemp AWCM, Droogmans G, Bindels RJ. TRPM6 forms the Mg2+ influx channel involved in intestinal and renal Mg2+ absorption. J Biol Chem (2004) 279:9–25. doi: 10.1074/jbc.M311201200
149. Topala CN, Groenestege WT, Thébault S, Van den Berg D, Nilius B, Hoenderop JG, et al. Molecular determinants of permeation through the cation channel TRPM6. Cell Calcium (2007) 41:513–23. doi: 10.1016/j.ceca.2006.10.003
150. Touyz RM, He Y, Montezano AC, Yao G, Chubanov V, Gudermann T, et al. Differential regulation of TRPM6/7 cation channels by ang II in vascular smooth muscle cells from spontaneously hypertensive rats. Am J Physiol Regul Integr Comp Physiol (2005) 290:R73–78. doi: 10.1152/ajpregu.00515.2005
151. van der Wijst J, Hoenderop JG, Bindels RJ. Epithelial Mg2+ channel TRPM6: insight into the molecular regulation. Magnes. Res (2009) 22:127–32. doi: 10.1684/mrh.2009.0174
152. Nair AV, Hocher B, Verkaart S, van Zeeland F, Pfab T, Slowinski T, et al. Loss of insulin-induced activation of TRPM6 magnesium channels results in impaired glucose tolerance during pregnancy. Proc Natl Acad Sci USA (2012) 109:11324–9. doi: 10.1073/pnas.1113811109
153. Ikari A, Sanada A, Okude C, Sawada H, Yamazaki Y, Sugatani J, et al. Up-regulation of TRPM6 transcriptional activity by AP-1 in renal epithelial cells. J Cell Physiol (2010) 222:481–7. doi: 10.1002/jcp.21988
154. Nie M, Bal MS, Liu J, Yang Z, Rivera C, Wu XR, et al. Uromodulin regulates renal magnesium homeostasis through the ion channel transient receptor potential melastatin 6 (TRPM6). J Biol Chem (2018) 2018:16488–502. doi: 10.1074/jbc.RA118.003950
155. De Baaij JHF, Blanchard MG, Lavrijsen M, Leipziger J, Bindels RJM, Hoenderop JGJ. P2X4 receptor regulation of transient receptor potential melastatin type 6 (TRPM6) Mg2+ channels. Pflug. Arch (2014) 466:1941–52. doi: 10.1007/s00424-014-1440-3
156. Cao G, Lee KP, van der Wijst J, de Graaf M, van der Kemp A, Bindels RJ, et al. Methionine sulfoxide reductase B1 (MsrB1) recovers TRPM6 channel activity during oxidative stress. J Biol Chem (2010) 285:26081–7. doi: 10.1074/jbc.M110.103655
157. Runnels LW, Yue L, Clapham DE. TRP-PLIK, a bifunctional protein with kinase and ion channel activities. Science (2001) 291:1043–7. doi: 10.1126/science.1058519
158. Nadler MJ, Hermosura MC, Inabe K, Perraud AL, Zhu Q, Stokes AJ, et al. LTRPC7 is a Mg.ATP- regulated divalent cation channel required for cell viability. Nature (2001) 411:590–5. doi: 10.1038/35079092
159. Monteilh-Zoller MK, Hermosura MC, Nadler MJ, Scharenberg AM, Penner R, Fleig A. TRPM7 provides an ion channel mechanism for cellular entry of trace metal ions. J Gen Physiol (2003) 121:49–60. doi: 10.1085/jgp.20028740
160. Abiria AA, Krapivinsky G, Sah R, Santa-Cruz AG, Chaudhuri D, Zhang J, et al. TRPM7 senses oxidative stress to release Zn2+ from unique intracellular vesicles. Proc Natl Acad Sci USA (2017) 114:E6079–88. doi: 10.1073/pnas.1707380114
161. Mederos y Schnitzler M, Waring J, Gudermann T, Chubanov V. Evolutionary determinants of divergent calcium selectivity of TRPM channels. FASEB J (2008) 22:1540–51. doi: 10.1096/fj.07-9694com
162. Li M, Du J, Jiang J, Ratzan W, Su LT, Runnels LW, et al. Molecular determinants of Mg2+ and Ca2+ permeability and pH sensitivity in TRPM6 and TRPM7. J Biol Chem (2007) 282:25817–30. doi: 10.1074/jbc.M608972200
163. Macianskiene R, Martisiene I, Zablockaite D, Gendviliene V. Characterization of Mg2+-regulated TRPM7-like current in human atrial myocytes. J Biomed Sci (2012) 19:75. doi: 10.1186/1423-0127-19-75
164. Xie J, Sun B, Du J, Yang W, Chen H-C, Overton JD, et al. Phosphatidylinositol 4,5-bisphosphate (PIP2) controls magnesium gatekeeper TRPM6 activity. Sci Rep (2011) 1:146. doi: 10.1038/srep00146
165. Runnels LW, Yue L, Clapham DE. The TRPM7 channel is inactivated by PIP(2) hydrolysis. Nat Cell Biol (2002) 4:329–36. doi: 10.1038/ncb781
166. Langeslag M, Clark K, Moolenaar WH, van Leeuwen FN, Jalink K. Activation of TRPM7 channels by phospholipase c-coupled receptor agonists. J Biol Chem (2007) 282:232–9. doi: 10.1074/jbc.M605300200
167. Takezawa R, Schmitz C, Demeuse P, Scharenberg AM, Penner R, Fleig A. Receptor-mediated regulation of the TRPM7 channel through its endogenous protein kinase domain. Proc Natl Acad Sci USA (2004) 101:6009–14. doi: 10.1073/pnas.0307565101
168. Jiang J, Li M, Yue L. Potentiation of TRPM7 inward currents by protons. J Gen Physiol (2005) 126:137–50. doi: 10.1042/BST0350086
169. Kerschbaum HH, Kozak JA, Cahalan MD. Polyvalent cations as permeant probes of MIC and TRPM7 pores. Biophys J (2003) 84:2293–305. doi: 10.1016/S0006-3495(03)75035-8
170. Oancea E, Wolfe JT, Clapham DE. Functional TRPM7 channels accumulate at the plasma membrane in response to fluid flow. Circul. Res (2006) 98:245–53. doi: 10.1161/01.RES.0000200179.29375.cc
171. Numata T, Shimizu T, Okada Y. Direct mechano-stress sensitivity of TRPM7 channel. Cel Physiol Biochem (2007) 19:1–8. doi: 10.1159/000099187
172. Kim TJ, Joo C, Seong J, Vafabakhsh R, Botvinick EL, Berns MW, et al. Distinct mechanisms regulating mechanical force-induced Ca(2+) signals at the plasma membrane and the ER in human MSCs. Elife (2015) 4:e04876. doi: 10.7554/eLife.04876
173. Xiao E, Yang HQ, Gan YH, Duan DH, He LH, Guo Y, et al. Brief reports: TRPM7 senses mechanical stimulation inducing osteogenesis in human bone marrow mesenchymal stem cells. Stem Cells (2015) 32:615–21. doi: 10.1002/stem.1858
174. Liu YS, Liu YA, Huang CJ, Yen MH, Tseng CT, Chien S, et al. Mechanosensitive TRPM7 mediates shear stress and modulates osteogenic differentiation of mesenchymal stromal cells through osterix pathway. Sci Rep (2015) 5:16522. doi: 10.1038/srep16522
175. Bessac BF, Fleig A. TRPM7 channel is sensitive to osmotic gradients in human kidney cells. J Physiol (2007) 582:1073–86. doi: 10.1113/jphysiol.2007.130534
176. Yu H, Zhang Z, Lis A, Penner R, Fleig A. TRPM7 is regulated by halides through its kinase domain. Cel. Mol Life Sci (2013) 70:2757–71. doi: 10.1007/s00018-013-1284-6
177. Riazanova LV, Dorovkov MV, Ansari A, Ryazanov AG. Characterization of the protein kinase activity of TRPM7/ChaK1, a protein kinase fused to the transient receptor potential ion channel. J Biol Chem (2004) 279:3708–16. doi: 10.1074/jbc.M308820200
178. Dorovkov MV, Ryazanov AG. Phosphorylation of annexin I by TRPM7 channel-kinase. J Biol Chem (2004) 279:50643–6. doi: 10.1074/jbc.C400441200
179. Clark K, Middelbeek J, Lasonder E, Dulyaninova NG, Morrice NA, Ryazanov AG, et al. TRPM7 regulates myosin IIA filament stability and protein localization by heavy chain phosphorylation. J Mol Biol (2008) 378:790–803. doi: 10.1016/j.jmb.2008.02.057
180. Deason-Towne F, Perraud AL, Schmitz C. Identification of Ser/Thr phosphorylation sites in the C2-domain of phospholipase c gamma2 (PLCgamma2) using TRPM7-kinase. Cel. Sign (2012) 24:2070–5. doi: 10.1016/j.cellsig.2012.06.015
181. Perraud AL, Zhao X, Ryazanov AG, Schmitz C. The channel-kinase TRPM7 regulates phosphorylation of the translational factor eEF2 via eEF2-k. Cell Signalling (2011) 23:586–93. doi: 10.1016/j.cellsig.2010.11.011
182. Faouzi M, Kilch T, Horgen FD, Fleig A, Penner R. The TRPM7 channel kinase regulates store-operated calcium entry. J Physiol (2017) 595:3165–80. doi: 10.1113/JP274006
183. Cai N, Bai Z, Nanda V, Runnels LW. Mass spectrometric analysis of TRPM6 and TRPM7 phosphorylation reveals regulatory mechanisms of the channel-kinases. Sci Rep (2017) 7:42739. doi: 10.1038/srep42739
184. Matsushita M, Kozak JA, Shimizu Y, McLachlin DT, Yamaguchi H, Wei FY, et al. Channel function is dissociated from the intrinsic kinase activity and autophosphorylation of TRPM7/ChaK1. J Biol Chem (2005) 280:20793–803. doi: 10.1074/jbc.M413671200
185. Desai BN, Krapivinsky G, Navarro B, Krapivinsky L, Carter BC, Febvay S, et al. Cleavage of TRPM7 releases the kinase domain from the ion channel and regulates its participation in fas-induced apoptosis. Develop.Cell (2012) 22:1149–62. doi: 10.1016/j.devcel.2012.04.006
186. Krapivinsky G, Krapivinsky L, Manasian Y, Clapham DE. The TRPM7 chanzyme is cleaved to release a chromatin-modifying kinase. Cell (2014) 157:1061–72. doi: 10.1016/j.cell.2014.03.046
187. Brandao K, Deason-Towne F, Zhao X, Perraud AL, Schmitz C. TRPM6 kinase activity regulates TRPM7 trafficking and inhibits cellular growth under hypomagnesic conditions. Cell Mol Life Sci (2014) 71:4853–67. doi: 10.1007/s00018-014-1647-7
188. Schmitz C, Dorovkov MV, Zhao X, Davenport BJ, Ryazanov AG, Perraud A-L. The channel kinases TRPM6 and TRPM7 are functionally nonredundant. J Biol Chem (2005) 280:37763–71. doi: 10.1074/jbc.M509175200
189. Van der Wijst J, Blanchard MG, Woodroof HI, Macartney TJ, Gourlay R, Hoenderop JG, et al. Kinase and channel activity of TRPM6 are co-ordinated by a dimerization motif and pocket interaction. Biochem J (2014) 460:165–75. doi: 10.1042/BJ20131639
190. Li M, Jiang J, Yue L. Functional characterization of homo-and heteromeric channel kinases TRPM6 and TRPM7. J Gen Physiol (2006) 127:525–37. doi: 10.1085/jgp.200609502
191. Hopkins MM, Feng X, Liu M, Parker LP, Koh DW. Inhibition of the transient receptor potential melastatin-2 channel causes increased DNA damage and decreased proliferation in breast adenocarcinoma cells. Int J Oncol (2015) 46:2267–76. doi: 10.3892/ijo.2015.2919
192. Tektemur A, Ozaydin S, Onalan EE, Kaya N, Kuloglu T, Ozercan İH, et al. TRPM2 mediates disruption of autophagy machinery and correlates with the grade level in prostate cancer. Cancer Re.s Clin Oncol (2019) 145:1297–311. doi: 10.1007/s00432-019-02898-z
193. Borgström A, Hauert B, Kappel S, Zoni E, Kiener M, Stokłosa P, et al. Small molecular inhibitors block TRPM4 currents in prostate cancer cells, with limited impact on cancer hallmark functions. J Mol Biol (2020) 2020:12. doi: 10.1016/j.jmb.2020.09.024
194. Maeda T, Suzuki A, Koga K, Miyamoto C, Maehata Y, Ozawa S, et al. TRPM5 mediates acidic extracellular pH signaling and TRPM5 inhibition reduces spontaneous metastasis in mouse B16-BL6 melanoma cells. Oncotarget (2017) 8:78312–26. doi: 10.18632/oncotarget.20826
195. Chen Y, Liu L, Xia L, Wu N, Wang Y, Li H, et al. TRPM7 silencing modulates glucose metabolic reprogramming to inhibit the growth of ovarian cancer by enhancing AMPK activation to promote HIF-1α degradation. Exp Clin Cancer Res (2022) 41:44. doi: 10.1186/s13046-022-02252-1
196. Liu K, Xu SH, Chen Z, Zeng QX, Li ZJ, Chen ZM. TRPM7 overexpression enhances the cancer stem cell-like and metastatic phenotypes of lung cancer through modulation of the Hsp90α/uPA/MMP2 signaling pathway. BMC Cancer (2018) 18:1167. doi: 10.1186/s12885-018-5050-x
197. Mittermeier C, Konopa A, Muehlich S. Molecular mechanisms to target cellular senescence in hepatocellular carcinoma. Cells (2020) 9:2540. doi: 10.3390/cells9122540
198. Wong R, Turlova E, Feng ZP, Rutka JT, Sun HS. Activation of TRPM7 by naltriben enhances migration and invasion of glioblastoma cells. Oncotarget (2017) 8:11239–48. doi: 10.18632/oncotarget.14496
199. Chen WL, Barszczyk A, Turlova E, Deurloo M, Liu B, Yang BB, et al. Inhibition of TRPM7 by carvacrol suppresses glioblastoma cell proliferation, migration and invasion. Oncotarget (2015) 6:6321–40. doi: 10.18632/oncotarget.3872
200. Che X, Zhan J, Zhao F, Zhong Z, Chen M, Han R, et al. Oridonin promotes apoptosis and restrains the viability and migration of bladder cancer by impeding TRPM7 expression via the ERK and AKT signaling pathways. Biomed Res Int (2021) 2021:4340950. doi: 10.1155/2021/4340950
201. Lee EH, Chun SY, Kim B, Yoon BH, Lee JN, Kim BS, et al. Knockdown of TRPM7 prevents tumor growth, migration, and invasion through the src, akt, and JNK pathway in bladder cancer. BMC Urol (2020) 20:145. doi: 10.1186/s12894-020-00714-2
202. Di Donato M, Ostacolo C, Giovannelli P, Di Sarno V, Gomez-Monterrey I, Campiglia P, et al. Therapeutic potential of TRPM8 antagonists in prostate cancer. Sci Rep (2021) 11:23232. doi: 10.1038/s41598-021-02675-4
203. Di Sarno V, Giovannelli P, Medina-Peris A, Ciaglia T, Di Donato M, Musella S, et al. New TRPM8 blockers exert anticancer activity over castration-resistant prostate cancer models. Eur J Med Chem (2022) 238:114435. doi: 10.1016/j.ejmech.2022.114435
204. De Petrocellis L, Arroyo FJ, Orlando P, Schiano Moriello A, Vitale RM, Amodeo P, et al. Tetrahydroisoquinoline-derived urea and 2,5-diketopiperazine derivatives as selective antagonists of the transient receptor potential melastatin 8 (TRPM8) channel receptor and antiprostate cancer agents. J Med Chem (2016) 59:5661–83. doi: 10.1021/acs.jmedchem.5b01448
205. Liu T, Fang Z, Wang G, Shi M, Wang X, Jiang K, et al. Anti-tumor activity of the TRPM8 inhibitor BCTC in prostate cancer DU145 cells. Oncol Lett (2016) 11:182–8. doi: 10.3892/ol.2015.3854
206. Okamoto Y, Ohkubo T, Ikebe T, Yamazaki J. Blockade of TRPM8 activity reduces the invasion potential of oral squamous carcinoma cell lines. Int J Oncol (2012) 40:1431–40. doi: 10.3892/ijo.2012.1340
207. Liu Y, Leng A, Li L, Yang B, Shen S, Chen H, et al. AMTB, a TRPM8 antagonist, suppresses growth and metastasis of osteosarcoma through repressing the TGFβ signaling pathway. Cell Death Dis (2022) 13:288. doi: 10.1038/s41419-022-04744-6
208. Alaimo A, Lorenzoni M, Ambrosino P, Bertossi A, Bisio A, Macchia A, et al. Calcium cytotoxicity sensitizes prostate cancer cells to standard-of-care treatments for locally advanced tumors. Cell Death Dis (2020) 11:1039. doi: 10.1038/s41419-020-03256-5
209. Devi S, Kedlaya R, Maddodi N, Bhat KMR, Weber CS, Valdivia H, et al. Calcium homeostasis in human melanocytes: role of transient receptor potential melastatin 1 (TRPM1) and its regulation by ultraviolet light. Am J Physiol Cell Physiol (2009) 297:C679–87. doi: 10.1152/ajpcell.00092.2009
210. Erickson LA, Letts GA, Shah JB, Shackelton JB, Duncan LM. TRPM1 (Melastatin-1/MLSN1) mRNA expression in Spitz nevi and nodular melanomas. Mod Pathol (2009) 22:969–76. doi: 10.1038/modpathol.2009.56
211. Miller AJ, Du J, Rowan S, Hershey CL, Widlund HR, Fisher DE. Transcriptional regulation of the melanoma prognostic marker melastatin (TRPM1) by MITF in melanocytes and melanoma. Cancer Res (2004) 64:509–516. doi: 10.1158/0008-5472.can-03-2440
212. Deeds J, Cronin F, Duncan LM. Patterns of melastatin mRNA expression in melanocytic tumors. Hum Pathol (2000) 31:1346–56. doi: 10.1016/S0046-8177(00)80003-9
213. Levy C, Khaled M, Iliopoulos D, Janas MM, Schuber S, Pinner S, et al. Intronic miR-211 assumes the tumor suppressive function of its host gene in melanoma. Mol Cell (2010) 40:841–9. doi: 10.1016/j.molcel.2010.11.020
214. Lunavat TR, Cheng L, Einarsdottir BO, Olofsson, Bagge R, Veppil Muralidharan S, Sharples RA, et al. BRAF(V600) inhibition alters the microRNA cargo in the vesicular secretome of malignant melanoma cells. Proc Natl Acad Sci USA (2017) 114:E5930–9. doi: 10.1073/pnas.1705206114
215. Hsieh C-C, Su ,Y-C, Jiang K-Y, Ito T, Li T-W, Kaku-Ito Y, et al. TRPM1 promotes tumor progression in acral melanoma by activating the Ca2+/CaMKIIδ/AKT pathway. J Adv Res (2023) 43:45–57. doi: 10.1016/j.jare.2022.03.005
217. Mortadza SAS, Wang L, Li D, Lin-Hua Jiang L-H. TRPM2 channel-mediated ROS-sensitive Ca2+ signaling mechanisms in immune cells. Front Immunol (2015) 6:407. doi: 10.3389/fimmu.2015.00407
218. Gorrini C, Harris IS, Mak TW. Modulation of oxidative stress as an anticancer strategy. Nat Rev Drug Discovery (2013) 12:931–47. doi: 10.1038/nrd4002
219. Hara Y, Wakamori M, Ishii M, Maeno E, Nishida M, Yoshida T, et al. LTRPC2 Ca2+-permeable channel activated by changes in redox status confers susceptibility to cell death. Mol Cell (2002) 9:163–73. doi: 10.1016/s1097-2765(01)00438-5
220. Yamamoto S, Shimizu S, Kiyonaka S, Takahashi N, Wajima T, Hara Y, et al. TRPM2- mediated Ca2+ influx induces chemokine production in monocytes that aggravates inflammatory neutrophil infiltration. Nat Med (2008) 14:738–47. doi: 10.1038/nm1758
221. Sumoza-Toledo A, Penner R. TRPM2: a multifunctional ion channel for calcium signalling. J Physiol (2011) 589:1515–25. doi: 10.1113/jphysiol.2010.201855
222. Ferrera L, Raffaella Barbieri R, Picco C, Zuccolini P, Remigante A, Bertelli S, et al. TRPM2 oxidation activates two distinct potassium channels in melanoma cells through intracellular calcium increase. Int J Mol Sci (2021) 22:8359. doi: 10.3390/ijms22168359
223. Sun L, Zhang Z, Zhao H, Qiu M, Wen Y, Yao X, et al. Identification of TRPM2 as a marker associated with prognosis and immune infiltration in kidney renal clear cell carcinoma. Front Mol Biosci (2022) 8:774905. doi: 10.3389/fmolb.2021.774905
224. Di A, Gao X-P, Qian F, T Kawamura T, Han J, Hecquet C, et al. The redox-sensitive cation channel TRPM2 modulates phagocyte ROS production and inflammation. Nat Immunol (2012) 13:29–35. doi: 10.1038/ni.2171
225. Miller BA, Cheung JY. TRPM2 protects against tissue damage following oxidative stress and ischemia-reperfusion. J Physiol (2016) 594:4181–91. doi: 10.1113/JP270934
226. Bao L, Chen SJ, Conrad K, Keefer K, Abraham T, Lee JP, et al. Depletion of the human ion channel TRPM2 in neuroblastoma demonstrates its key role in cell survival through modulation of mitochondrial reactive oxygen species and bioenergetics. J Biol Chem (2016) 291:24449–64. doi: 10.1074/jbc.M116.747147
227. Chen SJ, Hoffman NE, Shanmughapriya S, Bao L, Keefer K, Conrad K, et al. A splice variant of the human ion channel TRPM2 modulates neuroblastoma tumor growth through hypoxia-inducible factor (HIF)-1/2alpha. J Biol Chem (2014) 289:36284–302. doi: 10.1074/jbc.M114.620922
228. Liu L, Yudin Y, Nagwekar J, Kang C, Shirokova N, Rohacs T. Gaq sensitizes TRPM8 to inhibition by PI(4,5)P2 depletion upon receptor activation. J Neurosci (2019) 2019:2304–18. doi: 10.1523/JNEUROSCI.2304-18.2019
229. Hirschler-Laszkiewicz I, Festa F, Huang S, Moldovan G-L, Nicolae C, Dhoonmoon A, et al. The human ion channel TRPM2 modulates cell survival in neuroblastoma through E2F1 and FOXM1. Sci Rep (2022) 12:6311. doi: 10.1152/ajpcell.00098.2018
230. Ertilav K, Nazıroğlu M, Ataizi ZS, Braidy N. Selenium enhances the apoptotic efficacy of docetaxel through activation of TRPM2 channel in DBTRG glioblastoma cells. Neurotox Res (2019) 35:797–808. doi: 10.1007/s12640-019-0009-5
231. Chen S, Bao L, Keefer K, Shanmughapriya S, Chen L, Lee J, et al. Transient receptor potential ion channel TRPM2 promotes AML proliferation and survival through modulation of mitochondrial function, ROS, and autophagy. Cell Death Dis (2020) 11:247. doi: 10.1038/s41419-020-2454-8
232. Haladyna JN, Pastuer T, Riedel SS, Perraud AL, Bernt KM. Transient potential receptor melastatin-2 (Trpm2) does not influence murine MLL-AF9-driven AML leukemogenesis or in vitro response to chemotherapy. Exp Hematol (2016) 44:596–602 e593. doi: 10.1016/j.exphem.2016.03.006
233. Klumpp D, Misovic M, Szteyn K, Shumilina E, Rudner J, Huber SM. Targeting TRPM2 channels impairs radiation-induced cell cycle arrest and fosters cell death of T cell leukemia cells in a bcl-2- dependent manner. Oxid Med Cel. Long (2016) 2016:8026702. doi: 10.1155/2016/8026702
234. Lin R, Wang Y, Chen Q, Liu Z, Xiao S, Wang B, et al. TRPM2 promotes the proliferation and invasion of pancreatic ductal adenocarcinoma. Mol Med Rep (2018) 17:7537–44. doi: 10.3892/mmr.2018.8816
235. Lin R, Bao X, Wang H, Zhu S, Liu Z, Chen Q, et al. TRPM2 promotes pancreatic cancer by PKC/MAPK pathway. Cell Death Dis (2021) 12:585. doi: 10.1038/s41419-021-03856-9
236. Zeng X, Sikka SC, Huang L, Sun C, Xu C, Jia D, et al. A novel role for the transient receptor potential channel TRPM2 in prostate cancer cell proliferation. Prostate Cancer Prostatic Dis (2010) 13:195–201. doi: 10.1038/pcan.2009.55
237. Wang Q, Huang L, Yue J. Oxidative stress activates the TRPM2-Ca2+-CaMKII-ROS signaling loop to induce cell death in cancer cells. Biochim Biophys Acta Mol Cell Res (2017) 1864:957–67. doi: 10.1016/J.BBAMCR.2016.12.014
238. Koh DW, Powell DP, Blake SD, Hoffman JL, Hopkins MM, Feng X. Enhanced cytotoxicity in triple-negative and estrogen receptor positive breast adenocarcinoma cells due to inhibition of the transient receptor potential melastatin-2 channel. Oncol Rep (2015) 34:1589–98. doi: 10.3892/or.2015.4131
239. Gershkovitz M, Caspi Y, Fainsod-Levi T, Katz B, Michaeli J, Khawaled S, et al. TRPM2 mediates neutrophil killing of disseminated tumor cells. Cancer Res (2018) 78:2680–90. doi: 10.1158/0008-5472.CAN-17-3614
240. Almasi S, Kennedy BE, El-Aghil M, Sterea AM, Gujar S, Partida-Sánchez S, et al. TRPM2 channel-mediated regulation of autophagy maintains mitochondrial function and promotes gastric cancer cell survival via the JNK-signaling pathway. J Biol Chem (2018) 293:3637–50. doi: 10.1074/jbc.M117.817635
241. Wang Q, Guo W, Hao B, Shi X, Lu Y, Wong CW, et al. Mechanistic study of TRPM2-Ca(2+)-CAMK2-BECN1 signaling in oxidative stress-induced autophagy inhibition. Autophagy (2016) 12:1340–54. doi: 10.1080/15548627.2016.1187365
242. Almasi S, Sterea AM, Fernando W, Clements DR, Marcato P, Hoskin DW, et al. TRPM2 ion channel promotes gastric cancer migration, invasion, and tumor growth through the AKT signaling pathway. Sci Rep (2019) 9:4182. doi: 10.1038/s41598-019-40330-1
243. Almasi S, Long CY, Sterea A, Clements DR, Gujar S, El Hiani Y. TRPM2 silencing causes G2/M arrest and apoptosis in lung cancer cells via increasing intracellular ROS and RNS levels and activating the JNK pathway. Cell Physiol Biochem (2019) 52:742–57. doi: 10.33594/000000052
244. Zhao LY, Xu WL, Xu ZQ, Qi C, Li Y, Cheng J, et al. The overexpressed functional transient receptor potential channel TRPM2 in oral squamous cell carcinoma. Sci Rep (2016) 6:38471. doi: 10.1038/srep38471
245. Bacigalupa ZA, Rathmell WK. Beyond glycolysis: Hypoxias signaling as a master regulator of alternative metabolic pathways and the implications in clear renal cell carcinoma. Cancer Lett (2020) 489:19–28. doi: 10.1016/j.canlet.2020.05.034
246. Armisén R, Marcelain K, Simon F, Tapia JC, Toro J, Quest AF, et al. TRPM4 enhances cell proliferation through up-regulation of the β-catenin signaling pathway. J Cell Physiol (2011) 226:103–9. doi: 10.1002/jcp.22310
247. Liu L, Lin J, Hongying H. Identification of potential crucial genes associated with the pathogenesis and prognosis of endometrial cancer. Front Genet (2019) 10:373. doi: 10.3389/fgene.2019.00373
248. Li X-C, Cheng Y, Yang X, Zhou J-Y, Dong Y-Y, et al. Decreased expression of TRPM4 is associated with unfavorable prognosis and aggressive progression of endometrial carcinoma. Am J Trans Res (2020) 12:3926–39.
249. Schinke EN, Bii V, Nalla A, Rae DT, Tedrick L, Meadows GG, et al. A novel approach to identify driver genes involved in androgen-independent prostate cancer. Mol Cancer (2014) 13:120. doi: 10.1186/1476-4598-13-120
250. Berg KD, Soldini D, Jung M, Dietrich D, Stephan C, Jung K, et al. TRPM4 protein expression in prostate cancer: A novel tissue biomarker associated with risk of biochemical recurrence following radical prostatectomy. Virchows Arch (2016) 468:345–55. doi: 10.1007/s00428-015-1880-y
251. Holzmann C, Kappel S, Kilch T, Jochum MM, Urban SK, Jung V, et al. Transient receptor potential melastatin 4 channel contributes to migration of androgen-insensitive prostate cancer cells. Oncotarget (2015) 6:41783–93. doi: 10.18632/oncotarget.6157
252. Sagredo AI, Sagredo EA, Cappelli C, Báez P, Andaur RE, Blanco C, et al. TRPM4 regulates Akt/GSK3-β activity and enhances β-catenin signaling and cell proliferation in prostate cancer cells. Mol Oncol (2018) 12:151–65. doi: 10.1002/1878-0261.12100
253. Sagredo AI, Sagredo EA, Pola V, Echeverría C, Andaur R, Michea L, et al. TRPM4 channel is involved in regulating epithelial to mesenchymal transition, migration, and invasion of prostate cancer cell lines. J Cell Physiol (2019) 234:2037–50. doi: 10.1002/jcp.27371
254. Hong X, Yu J-J. MicroRNA-150 suppresses epithelial-mesenchymal transition, invasion, and metastasis in prostate cancer through the TRPM4-mediated β-catenin signaling pathway. 2019. Am J Physiol Cell Physiol (2019) 316:C463–80. doi: 10.3892/ijo.2015.2919
255. Cáceres M, Ortiz L, Recabarren T, Romero A, Colombo A, Leiva-Salcedo E, et al. TRPM4 is a novel component of the adhesome required for focal adhesion disassembly, migration and contractility. PloS One (2015) 10:e0130540. doi: 10.1371/journal.pone.0130540
256. Blanco C, Morales D, Mogollones I, Vergara-Jaque A, Vargas C, Álvarez A, et al. EB1- and EB2-dependent anterograde trafficking of TRPM4 regulates focal adhesion turnover and cell invasion. FASEB J (2019) 33:9434–52. doi: 10.1096/fj.201900136R
257. Wong KK, Hussain FA. TRPM4 is over-expressed in breast cancer associated with estrogen response and epithelial-mesenchymal transition gene sets. PloS One (2020) 15:e0233884. doi: 10.1371/journal.pone.0233884
258. Rivas J, Díaz N, Silva I, Morales D, Lavanderos B, Álvarez A, et al. KCTD5, a novel TRPM4-regulatory protein required for cell migration as a new predictor for breast cancer prognosis. FASEB J (2020) 34:7847–65. doi: 10.1096/fj.201901195RRR
259. Canales J, Cruz P, Díaz N, Riquelme D, Leiva-Salcedo E. K+ channel tetramerization domain5 (KCTD5) protein regulates cell migration, focal adhesion dynamics and spreading through modulation of Ca2+ signaling and Rac1 activity. Cells (2020) 9:2273. doi: 10.3390/cells9102273
260. Chinigò G, Pla AF, Gkika D. TRP channels and small GTPases interplay in the main hallmarks of metastatic cancer. Front Pharmacol (2020) 11:581455. doi: 10.3389/fphar.2020.581455
261. Verigos J, Kordias D, Papadaki S, Magklara A. Transcriptional profiling of tumorspheres reveals TRPM4 as a novel stemness regulator in breast cancer. Biomedicines (2021) 9:1368. doi: 10.3390/biomedicines9101368
262. Kappel S, Stokłosa P, Hauert B, Kaschitza DR, Borgström A, Baur R, et al. TRPM4 is highly expressed in human colorectal tumor buds and contributes to proliferation, cell cycle, and invasion of colorectal cancer cells. Mol Oncol (2019) 13:2393–405. doi: 10.1002/1878-0261.12566
263. Kappel S, Kaschitza DR, Hauert B, Rother K, Peinelt C. p53 alters intracellular Ca 2+ signaling through regulation of TRPM4. Cell Calcium (2022) 104:102591. doi: 10.1016/j.ceca.2022.102591
264. Stokłosa P, Kappel S, Peinelt C. A novel role of the TRPM4 ion channel in exocytosis. Cells (2022) 11:1793. doi: 10.3390/cells11111793
265. Marchi S, Giorgi C, Galluzzi L, Pinton P. Ca2+ fluxes and cancer. Mol Cell (2020) 78:1055–69. doi: 10.1016/j.molcel.2020.04.017
266. Vennekens R, Nilius B. Insights into TRPM4 function, regulation and physiological role. In: Flockerzi V, Nilius B, editors. Transient receptor potential (TRP) channels. handbook of experimental pharmacology. United States: Springer (2007). p. 269–85.
267. Zhu L, Miao B, Dymerska D, Kuswik M, Bueno-Martínez E, Sanoguera-Miralles L, et al. Germline variants of CYBA and TRPM4 predispose to familial colorectal cancer. Cancers (2022) 14:670. doi: 10.3390/cancers14030670
268. Leung KK, Nguyen A, Shi T, Tang L, Ni X, Escoubet L, et al. Multiomics of azacitidine-treated AML cells reveals variable and convergent targets that remodel the cell-surface proteome. Proc Natl Acad Sci U S A (2019) 116:695–700. doi: 10.1073/pnas.1813666116
269. Wang F, Wu P, Gon S, Chen Y, Gao J. Aberrant TRPM4 expression in MLL-rearranged acute myeloid leukemia and its blockade induces cell cycle arrest via AKT/GLI1/Cyclin D1 pathway. Cell Signal (2020) 72:109643. doi: 10.1016/j.cellsig.2020.109643
270. Suguro M, Tagawa H, Kagami Y, Okamoto M, Ohshima K, Shiku H, et al. Expression profiling analysis of the CD5+ diffuse large b-cell lymphoma subgroup: development of a CD5 signature. Cancer Sci (2006) 97:868–74. doi: 10.1111/j.1349-7006.2006.00267.x
271. Loo SK, Ch’ng ES, MdSalleh MS, Banham AH, Pedersen LM, Møller MB, et al. TRPM4 expression is associated with activated b cell subtype and poor survival in diffuse large b cell lymphoma. Histopathology (2017) 71:98–111. doi: 10.1111/his.13204
272. Han S, Koo HH, Lan Q, Lee K-M, Park AK, Park SK, et al. Common variation in genes related to immune response and risk of childhood leukemia. Hum Immunol (2012) 73:316–9. doi: 10.1016/j.humimm.2011.12.018
273. Ceylan GG, Önalan EE, Kuloğlu T, Aydoğ G, Keleş I, Tonyali S, et al. Potential role of melastatin-related transient receptor potential cation channel subfamily m gene expression in the pathogenesis of urinary bladder cancer. Oncol Lett (2016) 12:5235–9. doi: 10.3892/ol.2016.5359
274. Stokłosa P, Borgström A, Kappel S, Peinelt C. TRP channels in digestive tract cancers. Int J Mol Sci (2020) 21:1877. doi: 10.3390/ijms21051877
275. Auwercx J, Rybarczyk P, Kischel P, Dhennin-Duthille I, Chatelain D, Sevestre H, et al. Mg2+ transporters in digestive cancers. Nutrients (2021) 13:210. doi: 10.3390/nu13010210
276. Castiglioni S, Cazzaniga A, Trapani V, Cappadone C, Farruggia G, Merolle L, et al. Magnesium homeostasis in colon carcinoma LoVo cells sensitive or resistant to doxorubicin. Sci Rep (2015) 5:16538. doi: 10.1038/srep16538
277. Xie B, Zhao R, Bai B, Wu Y, Xu Y, Lu S, et al. Identification of key tumorigenesis-related genes and their microRNAs in colon cancer. Oncol Rep (2018) 40:3551–60. doi: 10.3892/or.2018.6726
278. Ibrahim S, Dakik H, Vandier C, Chautard R, Paintaud G, Mazurier F, et al. Expression profiling of calcium channels and calcium-activated potassium channels in colorectal cancer. Cancers (2019) 11:561. doi: 10.3390/cancers11040561
279. Pugliese D, Armuzzi A, Castri F, Benvenuto R, Mangoni A, Guidi L, et al. TRPM7 is overexpressed in human IBD-related and sporadic colorectal cancer and correlates with tumor grade. Dig. Liver Dis (2020) 52:1188–94. doi: 10.1016/j.dld.2020.05.027
280. El Boustany C, Bidaux G, Enfissi A, Delcourt P, Prevarskaya N, Capiod T. Capacitative calcium entry and transient receptor potential canonical 6 expression control human hepatoma cell proliferation. Hepatology (2006) 47:2068–77. doi: 10.1002/hep.22263
281. Pietropaolo G, Pugliese D, Armuzzi A, Guidi L, Gasbarrini A, Rapaccini GL, et al. Magnesium absorption in intestinal cells: Evidence of cross-talk between EGF and TRPM6 and novel implications for cetuximab therapy. Nutrients (2020) 12:3277. doi: 10.3390/nu12113277
282. Dai Q, Shrubsole MJ, Ness RM, Schlundt D, Cai Q, Smalley WE, et al. The relation of magnesium and calcium intakes and a genetic polymorphism in the magnesium transporter to colorectal neoplasia risk. Am J Clin Nutr (2007) 86:743–51. doi: 10.1093/ajcn/86.3.743
283. Su F, Wang B-F, Zhang T, Hou X-M, Feng M-H. TRPM7 deficiency suppresses cell proliferation, migration, and invasion in human colorectal cancer via regulation of epithelial-mesenchymal transition. Cancer biomark (2019) 26:451–60. doi: 10.3233/CBM-190666
284. Chen L, Cao R, Wang G, Yuan L, Qian G, Guo Z, et al. Downregulation of TRPM7 suppressed migration and invasion by regulating epithelial-mesenchymal transition in prostate cancer cells. Med Oncol (2017) 34:127. doi: 10.1007/s12032-017-0987-1
285. Sun Y, Selvaraj S, Varma A, Derry S, Sahmoun AE, Singh BB. Increase in serum Ca2+/Mg2+ ratio promotes the proliferation of prostate cancer cells by activating TRPM7 channels. J Biol Chem (2013) 288:255–63. doi: 10.1074/jbc.M112.393918
286. Sun Y, Schaar A, Sukumaran P, Dhasarathy A, Sing BB. TGFbeta-induced epithelial-to-mesenchymal transition in prostate cancer cells is mediated via TRPM7 expression. Mol Oncol (2018) 57:752–61. doi: 10.1002/mc.22797
287. Yang F, Cai J, Zhan H, Situ J, Li W, Mao Y, et al. Suppression of TRPM7 inhibited hypoxia-induced migration and invasion of androgen-independent prostate cancer cells by enhancing RACK1-mediated degradation of HIF-1α. Oxid Med Cell Longev (2020) 2020:6724810. doi: 10.1155/2020/6724810
288. Yee NS, Brown RD, Lee MS, Zhou W, Jensen C, Gerke H, et al. TRPM8 ion channel is aberrantly expressed and required for preventing replicative senescence in pancreatic adenocarcinoma: Potential role of TRPM8 as a biomarker and target. Cancer Biol Ther (2012) 13:592–9. doi: 10.4161/cbt.20079
289. Yee NS, Kazi AA, Li Q, Yang Z, Berg A, Yee RK. Aberrant over-expression of TRPM7 ion channels in pancreatic cancer: Required for cancer cell invasion and implicated in tumor growth and metastasis. Biol Open (2015) 4:507–14. doi: 10.1242/bio.20137088
290. Rybarczyk P, Vanlaeys A, Brassart B, Dhennin-Duthille I, Chatelain D, Sevestre H, et al. The transient receptor potential melastatin 7 channel regulates pancreatic cancer cell invasion through the Hsp90α/uPA/MMP2 pathway. Neoplasia (2017) 19:288–300. doi: 10.1016/j.neo.2017.01.004
291. Wu Y, Tan X, Liu P, Yang Y, Huang Y, Liu X, et al. ITGA6 and RPSA synergistically promote pancreatic cancer invasion and metastasis via PI3K and MAPK signaling pathways. Exp Cell Res 379 (2019), 30–47. doi: 10.1016/j.yexcr.2019.03.022
292. Lefebvre T, Rybarczyk P, Bretaudeau C, Vanlaeys A, Cousin R, Brassart-Pasco S, et al. TRPM7/RPSA complex regulates pancreatic cancer cell migration. Front Cell Dev Biol (2020) 8:549. doi: 10.3389/fcell.2020.00549
293. Dhennin-Duthille I, Gautier M, Faouzi M, Guilbert A, Brevet M, Vaudry D, et al. High expression of transient receptor potential channels in human breast cancer epithelial cells and tissues: Correlation with pathological parameters. Cell Physiol Biochem (2011) 28:813–22. doi: 10.1159/000335795
294. Middelbeek J, Kuipers AJ, Henneman L, Visser D, Eidhof I, van Horssen R, et al. TRPM7 is required for breast tumor cell metastasis. Cancer Res (2012) 72:4250–61. doi: 10.1158/0008-5472.CAN-11-3863
295. Wang Y, Lu R, Chen P, Cui R, Ji M, Zhang X, et al. Promoter methylation of transient receptor potential melastatin-related 7 (TRPM7) predicts a better prognosis in patients with luminal a breast cancers. BMC Cancer (2022) 22:951. doi: 10.1186/s12885-022-10038-z
296. Guilbert A, Gautier M, Dhennin-Duthille I, Rybarczyk P, Sahni J, Sevestre H, et al. Transient receptor potential melastatin 7 is involved in oestrogen receptor-negative metastatic breast cancer cells migration through its kinase domain. Eur J Cancer (2013) 49:3694–707. doi: 10.1016/j.ejca.2013.07.008
297. Meng X, Cai C, Wu J, Cai S, Ye C, Chen H, et al. TRPM7 mediates breast cancer cell migration and invasion through the MAPK pathway. Cancer Lett (2013) 333:96–102. doi: 10.1016/j.canlet.2013.01.031
298. Kuipers AJ, Middelbeek J, Vrenken K, Pérez-González C, Poelmans G, Klarenbeek J, et al. TRPM7 controls mesenchymal features of breast cancer cells by tensional regulation of SOX4. Biochim Biophys Acta (2018) 1864:2409–19. doi: 10.1016/j.bbadis.2018.04.017
299. Chen J-P, Luan Y, You C-X, Chen X-H, Luo R-C, Li R. TRPM7 regulates the migration of human nasopharyngeal carcinoma cells by mediating Ca2+ influx. Cell Calcium (2010) 47:425–32. doi: 10.1016/j.ceca.2010.03.003
300. Wang J, Liao Q-J, Zhang Y, Zhou H, Luo CH, Tang J, et al. TRPM7 is required for ovarian cancer cell growth, migration and invasion. Biochem Biophys Res Commun (2014) 454:547–53. doi: 10.1016/j.bbrc.2014.10.118
301. Liu L, Wu N, Wang Y, Zhang X, Xia B, Tang J, et al. TRPM7 promotes the epithelial-mesenchymal transition in ovarian cancer through the calcium-related PI3K / AKT oncogenic signaling. J Exp Clin Cancer Res (2019) 38:106. doi: 10.1186/s13046-019-1061-y
302. Huang Y, Li S, Jia Z, Li S, He W, Zhou C, et al. TRIM4 interacts with TRPM8 and regulates its channel function through K423-mediated ubiquitination. J Cell Physiol (2021) 236:2934–2949. doi: 10.1093/ecco-jcc/jjy222.140
303. Jia D, Lu M, Jung KH, Park JH, Yu L, Onuchic JN, et al. Elucidating cancer metabolic plasticity by coupling gene regulation with metabolic pathways. Proc Natl Acad Sci USA (2019) 116:3909–18. doi: 10.1073/pnas.1816391116
304. Vander Heiden MG, DeBerardinis RJ. Understanding the intersections between metabolism and cancer biology. Cell (2017) 168:657–69. doi: 10.1016/j.cell.2016.12.039
305. Chen TM, Huang CM, Hsieh MS, Lin CS, Lee WH, Yeh CT, et al. TRPM7 via calcineurin/NFAT pathway mediates metastasis and chemotherapeutic resistance in head and neck squamous cell carcinoma. Aging (2022) 14:5250–70. doi: 10.18632/aging.204154
306. Gao SL, Kong CZ, Zhang Z, Li ZL, Bi JB, Liu XK. TRPM7 is overexpressed in bladder cancer and promotes proliferation, migration, invasion and tumor growth. Oncol Rep (2017) 38:1967–76. doi: 10.3892/or.2017.5883
307. Gao H, Chen X, Du X, Guan B, Liu Y, Zhang H. EGF enhances the migration of cancer cells by up-regulation of TRPM7. Cell Calcium (2011) 50:559–68. doi: 10.1016/j.ceca.2011.09.003
308. Luanpitpong S, Rodboon N, Samart P, Vinayanuwattikun C, Klamkhlai S, Chanvorachote P, et al. A novel TRPM7/O-GlcNAc axis mediates tumour cell motility and metastasis by stabilising c-myc and caveolin-1 in lung carcinoma. Br J Cancer (2020) 123:s1289–1301. doi: 10.1038/s41416-020-0991-7
309. Samart P, Luanpitpong S, Rojanasakul Y, Issaragrisil S. O- GlcNAcylation homeostasis controlled by calcium influx channels regulates multiple myeloma dissemination. J Exp Clin Cancer Res (2021) 40:100. doi: 10.1186/s13046-021-01876-z
310. Voringer S, Schreyer L, Nadolni W, Meier MA, Woerther K, Mittermeier C, et al. Inhibition of TRPM7 blocks MRTF/SRF-dependent transcriptional and tumorigenic activity. Oncogene (2020) 39:2328–44. doi: 10.1038/s41388-019-1140-8
311. Zhang Z, Faouzi M, Huang J, Geerts D, Yu H, Fleig A, et al. N-myc-induced up-regulation of TRPM6/TRPM7 channels promotes neuroblastoma cell proliferation. Oncotarget (2014) 5:625–7634. doi: 10.18632/oncotarget.2283
312. Alanazi R, Nakatogawa H, Wang H, Ji D, Luo Z, Golbourn B, et al. Inhibition of TRPM7 with carvacrol suppresses glioblastoma functions in vivo. Eur J Neurosci (2022) 55:1483–91. doi: 10.1111/ejn.15647
313. Wan J, Guo AA, Chowdhury I, Guo S, Hibbert J, Wang G, et al. TRPM7 induces mechanistic target of Rap1b through the downregulation of miR-28-5p in glioma proliferation and invasion. Front Oncol (2019) 9:1413. doi: 10.3389/fonc.2019.01413
314. Wan J, King P, Guo S, Saafir T, Jing Y, Liu M. TRPM7 induces tumorigenesis and stemness through notch activation in glioma. Front Pharmacol (2020) 11:590723. doi: 10.3389/fphar.2020.590723
315. Liu M, Inoue K, Leng T, Guo S, Xiong ZG. TRPM7 channels regulate glioma stem cells through STAT3 and notch signaling pathways. Cell Signal (2014) 26:2773–81. doi: 10.1016/j.cellsig.2014.08.020
316. Leng TD, Li MH, Shen JF, Liu ML, Li XB, Sun HW, et al. Suppression of TRPM7 inhibits proliferation, migration, and invasion of malignant human glioma cells. CNS Neurosci Ther (2015) 21:252–61. doi: 10.1111/cns.12354
317. Santoni G, Amantini C, Nabissi M, Maggi F, Morelli MB. MicroRNA signature targeting transient receptor potential channels in the prognosis and therapy of cancer. J Cell Immunol (2020) 2:74–9. doi: 10.33696/immunology.2.023
318. Panni S, Lovering RC, Porras P, Orchard S. Non-coding RNA regulatory networks. Biochim Biophys Acta Gene Regul Mech (2020) 1863:194417. doi: 10.1016/j.bbagrm.2019.194417
319. Guo S, King P, Liang E, Guo AA, Liu M. LncRNA HOTAIR sponges miR-301a-3p to promote glioblastoma proliferation and invasion through upregulating FOSL1. Cel. Signal (2022) 94:110306. doi: 10.1016/j.cellsig.2022.110306
320. Xing Y, Wei X, Wang M, Liu Y, Sui Z, Wang X, et al. Stimulating TRPM7 suppresses cancer cell proliferation and metastasis by inhibiting autophagy. Cancer Lett (2022) 525:179–97. doi: 10.1016/j.canlet.2021.10.043
321. Dower CM, Wills CA, Frisch SM, Wang HG. Mechanisms and context underlying the role of autophagy in cancer metastasis. Autophagy (2018) 14:1110–28. doi: 10.1080/15548627.2018.1450020
322. Zhang L, Barritt GJ. TRPM8 in prostate cancer cells: a potential diagnostic and prognostic marker with a secretory function? Endocr. Relat Cancer (2006) 13:27–38. doi: 10.1677/erc.1.01093
323. Yee NS. TRPM8 ion channels as potential cancer biomarker and target in pancreatic cancer. Adv Prot Chem Struct Biol (2016) 104:127–55. doi: 10.1016/bs.apcsb.2016.01.001
324. Zhang L, Barritt GJ. Evidence that TRPM8 is an androgen-dependent Ca2+ channel required for the survival of prostate cancer cells. Cancer Res (2004) 64:8365–73. doi: 10.1158/0008-5472.CAN-04-2146
325. Bidaux G, Roudbaraki M, Merle C, Crépin A, Delcourt P, Slomianny C, et al. Evidence for specific TRPM8 expression in human prostate secretory epithelial cells: functional androgen receptor requirement. Endocr. Relat Cancer (2005) 12:367–82. doi: 10.1677/erc.1.00969.10.1677/erc.1.00969
326. Asuthkar S, Velpula KK, Elustondo PA, Demirkhanyan L, Zakharian E. TRPM8 channel as a novel molecular target in androgen-regulated prostate cancer cells. Oncotarget (2015) 6:17221–36. doi: 10.18632/oncotarget.3948
327. Asuthkar S, Demirkhanyan L, Mueting SR, Cohen A, Zakharian E. High-throughput proteome analysis reveals targeted TRPM8 degradation in prostate cancer. Oncotarget (2017) 8(8):12877–90. doi: 10.18632/oncotarget.14178
328. Valero MLI, Mello de Queiroz F, Stuhmer W, Viana F, Pardo LA. TRPM8 ion channels differentially modulate proliferation and cell cycle distribution of normal and cancer prostate cells. PloS One (2012) 7:e51825. doi: 10.1371/journal.pone.0051825
329. Liu T, Liao Y, Tao H, Zeng J, Wang G, Yang Z, et al. RNA Interference-mediated depletion of TRPM8 enhances the efficacy of epirubicin chemotherapy in prostate cancer LNCaP and PC3 cells. Oncol Lett (2018) 15:4129–36. doi: 10.3892/ol.2018.7847
330. Yang Z-H, Wang X-H, Wang H-P, Hu H-Q. Effects of TRPM8 on the proliferation and motility of prostate cancer PC-3 cells. Asian J Androl (2009) 11:157–65. doi: 10.1038/aja.2009.1
331. Bidaux G, Borowiec AS, Dubois C, Delcourt P, Schulz C, Vanden Abeele F, et al. Targeting of short TRPM8 isoforms induces 4TM-TRPM8-dependent apoptosis in prostate cancer cells. Oncotarget (2016) 7:29063–80. doi: 10.18632/oncotarget.8666
332. Peng M, Wang Z, Yang Z, Tao L, Liu Q, Yi LU, et al. Overexpression of short TRPM8 variant alpha promotes cell migration and invasion and decreases starvation-induced apoptosis in prostate cancer LNCaP cells. Oncol Lett (2015) 10:1378–84. doi: 10.3892/ol.2015.3373
333. Bidaux G, Flourakis M, Thebault S, Zholos A, Beck B, Gkika D, et al. Prostate cell differentiation status determines transient receptor potential melastatin member 8 channel subcellular localization and function. J Clin Invest (2007) 117:1647–57. doi: 10.1172/JCI30168
334. Grolez GP, Hammadi M, Barras A, Gordienko D, Slomianny C, Völkel P, et al. Encapsulation of a TRPM8 agonist, WS12, in lipid nanocapsules potentiates PC3 prostate cancer cell migration inhibition through channel activation. Sci Rep (2019) 9:7926. doi: 10.1038/s41598-019-44452-4
335. Grolez GP, Gordiendko DV, Clarisse M, Hammadi M, Desruelles E, Fromont G, et al. TRPM8-androgen receptor association within lipid rafts promotes prostate cancer cell migration. Cell Death Dis (2019) 10:652. doi: 10.1038/s41419-019-1891-8
336. Grolez GP, Chinigò G, Barras A, Hammadi M, Noyer L, Kondratska K, et al. TRPM8 as an anti-tumoral target in prostate cancer growth and metastasis dissemination. Int J Mol Sci (2022) 23:6672. doi: 10.3390/ijms23126672
337. Chinigò G, Grolez GP, Audero M, Bokhobza A, Bernardini M, Cicero J, et al. TRPM8-Rap1A interaction sites as critical determinants for adhesion and migration of prostate and other epithelial cancer cells. Cancers (2022) 14:2261. doi: 10.3390/cancers14092261
338. Du JD, Zheng X, Chen YL, Huang ZQ, Cai SW, Jiao HB, et al. Elevated transient receptor potential melastatin 8 (TRPM8) expression is correlated with poor prognosis in pancreatic cancer. Med Sci Monit (2018) 24:3720–5. doi: 10.12659/MSM.909968
339. Yee NS, Zhou W, Lee M. Transient receptor potential channel TRPM8 is over-expressed and required for cellular proliferation in pancreatic adenocarcinoma. Cancer Lett (2010) 297:49–55. doi: 10.1016/j.canlet.2010.04.023
340. Huang Y, Li S, Liu Q, Wang Z, Li S, Liu L, et al. The LCK-14-3-3ζ-TRPM8 axis regulates TRPM8 function/assembly and promotes pancreatic cancer malignancy. Cell Death Dis (2022) 13:524. doi: 10.1038/s41419-022-04977-5
341. Chodon D, Guilbert A, Dhennin-Duthille I, Gautier M, Telliez MS, Sevestre H, et al. Estrogen regulation of TRPM8 expression in breast cancer cells. BMC Cancer (2010) 10:212. doi: 10.1186/1471-2407-10-212
342. Liu J, Chen Y, Shuai S, Ding D, Li R, Luo R. TRPM8 promotes the aggressiveness of breast cancer cells by regulating EMT via activating AKT/GSK-3beta pathway. Tumour Biol (2014) 35:8969–77. doi: 10.1007/s13277-014-2077-8
343. Saldías MP, Maureira D, Orellana-Serradell O, Silva I, Lavanderos B, Cruz P, et al. TRP channels interactome as a novel therapeutic target in breast cancer. Front Oncol (2021) 11:621614. doi: 10.3389/fonc.2021.621614
344. Yapa K, Deuis J, Peters AA, Kenny PA, Roberts-Thomson SJ, Vetter I, et al. Assessment of the TRPM8 inhibitor AMTB in breast cancer cells and its identification as an inhibitor of voltage gated sodium channels. Life Sci (2018) 198:198128–35. doi: 10.1016/j.lfs.2018.02.030
345. Wondergem R, Bartley JW. Menthol increases human glioblastoma intracellular Ca2+, BK channel activity and cell migration. J Biomed Sci (2009) 16:1–7. doi: 10.1186/1423-0127-16-90
346. Klumpp D, Frank SC, Klumpp L, Sezgin EC, Eckert M, Edalat L, et al. TRPM8 is required for survival and radio-resistance of glioblastoma cells. Oncotarget (2017) 8(56):95896–913. doi: 10.18632/oncotarget.21436
347. Zeng J, Wu Y, Zhuang S, Qin L, Hua S, Mungur R, et al. Identification of the role of TRPM8 in glioblastoma and its effect on proliferation, apoptosis and invasion of the U251 human glioblastoma cell line. Oncol Rep (2019) 42:1517–26. doi: 10.3892/or.2019.7260
348. Yee NS. Roles of TRPM8 ion channels in cancer: Proliferation, survival, and invasion. Cancers (Basel) (2015) 7:2134–46. doi: 10.3390/cancers7040882
349. Wang Y, Yang Z, Meng Z, Cao H, Zhu G, Liu T, et al. Knockdown of TRPM8 suppresses cancer malignancy and enhances epirubicin-induced apoptosis in human osteosarcoma cells. Int J Biol Sci (2014) 10:90–102. doi: 10.7150/ijbs.7738
350. Zhao W, Xu H. High expression of TRPM8 predicts poor prognosis in patients with osteosarcoma. Oncol Lett (2016) 12:1373–9. doi: 10.3892/ol.2016.4764
351. Ikushima H, Miyazono K. TGF beta signalling: a complex web in cancer progression. Nat Rev Cancer (2010) 10:415–24. doi: 10.1038/nrc2853
352. Huang Y, Li S, Jia Z, Zhao W, Zhou C, Zhang R, et al. Transient receptor potential melastatin 8 (TRPM8) channel regulates proliferation and migration of breast cancer cells by activating the AMPK-ULK1 pathway to enhance basal autophagy. Front Oncol (2020) 10:573127. doi: 10.3389/fonc.2020.573127
353. Yin Y, Zhang F, Feng S, Butay KJ, Borgnia MJ, Im W, et al. Activation mechanism of the mouse cold-sensing TRPM8 channel by cooling agonist and PIP2. Science (2022) 378:154. doi: 10.1126/science.add1268
354. Fernández-Carvajal A, González-Muñiz R, Gregorio Fernández-Ballester G, Ferrer-Montiel A. Investigational drugs in early phase clinical trials targeting thermotransient receptor potential (thermoTRP) channels. Exp Opin Investig Drugs (2020) 29:1209–22. doi: 10.1080/13543784.2020.1825680
355. Mak IW, Evaniew N, Ghert M. Lost in translation: animal models and clinical trials in cancer treatment. Am J Transl Res (2014) 6:114–8.
356. Journigan VB, Alarcón-Alarcón D, Feng Z, Wang Y, Liang T, Dawley DC, et al. Structural and in vitro functional characterization of a menthyl TRPM8 antagonist indicates species-dependent regulation. ACS Med Chem Lett (2021) 12:758–67. doi: 10.1021/acsmedchemlett.1c0000
Keywords: TRPM channels, modulators, cancer, cell proliferation, autophagy
Citation: Ciaglia T, Vestuto V, Bertamino A, González-Muñiz R and Gómez-Monterrey I (2023) On the modulation of TRPM channels: Current perspectives and anticancer therapeutic implications. Front. Oncol. 12:1065935. doi: 10.3389/fonc.2022.1065935
Received: 27 October 2022; Accepted: 15 December 2022;
Published: 09 February 2023.
Edited by:
Simone Patergnani, University of Ferrara, ItalyReviewed by:
Hongmei Wang, Southeast University, ChinaGrigori Rychkov, University of Adelaide, Australia
David Crottès, Université de Tours, France
Copyright © 2023 Ciaglia, Vestuto, Bertamino, González-Muñiz and Gómez-Monterrey. This is an open-access article distributed under the terms of the Creative Commons Attribution License (CC BY). The use, distribution or reproduction in other forums is permitted, provided the original author(s) and the copyright owner(s) are credited and that the original publication in this journal is cited, in accordance with accepted academic practice. No use, distribution or reproduction is permitted which does not comply with these terms.
*Correspondence: Isabel Gómez-Monterrey, aW1nb21lekB1bmluYS5pdA==; Rosario González-Muñiz, aXFtZzMxM0BpcW0uY3NpYy5lcw==