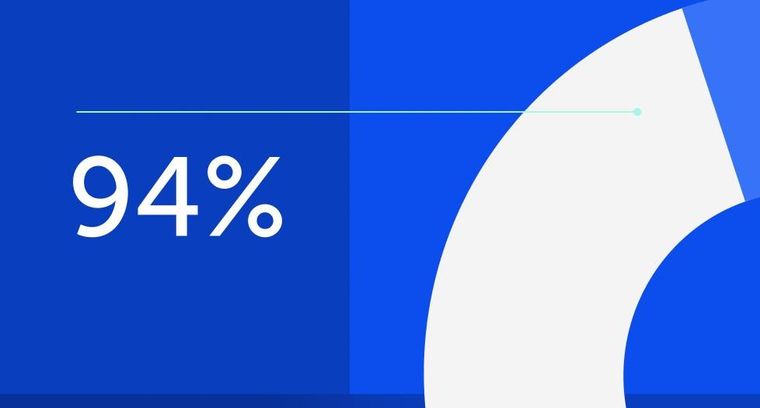
94% of researchers rate our articles as excellent or good
Learn more about the work of our research integrity team to safeguard the quality of each article we publish.
Find out more
REVIEW article
Front. Oncol., 14 December 2022
Sec. Thoracic Oncology
Volume 12 - 2022 | https://doi.org/10.3389/fonc.2022.1016217
In the US, there are ~250,000 new lung cancer diagnoses and ~130,000 deaths per year, and worldwide there are an estimated 1.6 million deaths per year from this deadly disease. Lung cancer is the most common cause of cancer death worldwide, and it accounts for roughly a quarter of all cancer deaths in the US. Non-small cell lung cancer (NSCLC) represents 80-85% of these cases. Due to an enormous tobacco cessation effort, NSCLC rates in the US are decreasing, and the implementation of lung cancer screening guidelines and other programs have resulted in a higher percentage of patients presenting with potentially curable locoregional disease, instead of distant disease. Exciting developments in molecular targeted therapy and immunotherapy have resulted in dramatic improvement in patients’ survival, in combination with new surgical, pathological, radiographical, and radiation techniques. Concurrent platinum-based doublet chemoradiation therapy followed by immunotherapy has set the benchmark for survival in these patients. However, despite these advances, ~50% of patients diagnosed with locally advanced NSCLC (LA-NSCLC) survive long-term. In patients with local and/or locoregional disease, chemoradiation is a critical component of curative therapy. However, there remains a significant clinical gap in improving the efficacy of this combined therapy, and the development of non-overlapping treatment approaches to improve treatment outcomes is needed. One potential promising avenue of research is targeting cancer metabolism. In this review, we will initially provide a brief general overview of tumor metabolism as it relates to therapeutic targeting. We will then focus on the intersection of metabolism on both oxidative stress and anti-tumor immunity. This will be followed by discussion of both tumor- and patient-specific opportunities for metabolic targeting in NSCLC. We will then conclude with a discussion of additional agents currently in development that may be advantageous to combine with chemo-immuno-radiation in NSCLC.
In the US, there are ~250,000 new lung cancer diagnoses and ~130,000 deaths per year, and worldwide there are an estimated 1.6 million deaths per year from this disease. Lung cancer is the most common cause of cancer death worldwide, and it accounts for roughly a quarter of all cancer deaths in the US (1, 2). Non-small cell lung cancer (NSCLC) represents 80-85% of these cases. Due to an enormous tobacco cessation effort, NSCLC rates in the US are decreasing, and the implementation of lung cancer screening guidelines and other programs have resulted in a higher percentage of patients presenting with potentially curable locoregional disease, instead of distant disease (3). Exciting developments in molecular targeted therapy and immunotherapy have resulted in dramatic improvement in patients’ survival, in combination with new surgical, pathological, radiographical, and radiation techniques. Concurrent platinum-base doublet chemoradiation therapy followed by immunotherapy has set the benchmark for survival in these patients (4). However, despite these advances, ~50% of patients diagnosed with locally advanced NSCLC (LA-NSCLC) survive long-term (4). In patients with local and/or locoregional disease, chemoradiation is a critical component of curative therapy. However, there remains a significant clinical gap in improving the efficacy of this combined approach, and the development of non-overlapping treatment approaches to improve treatment outcomes is needed.
One potential promising avenue of research is targeting cancer metabolism. In this review, we will initially provide a brief general overview of tumor metabolism as it relates to therapeutic targeting. We will then focus on the intersection of metabolism on both oxidative stress and anti-tumor immunity. This will be followed by discussion of both tumor- and patient-specific opportunities for metabolic targeting in NSCLC. We will then conclude with a discussion of additional agents currently in development that may be advantageous to combine with chemo-immuno-radiation in NSCLC.
As first detailed by Otto Warburg nearly a century ago, metabolic activity within tumor cells diverges significantly from normal eukaryotic homeostasis (5). Whereas normal cells balance energy and biomass to support specialized functions and proliferation, tumor cells disproportionately favor high proliferative activity. This results in a metabolic program designed to generate high biomass turnover at the expense of balanced energy production (6). Common metabolic pathways including glycolysis, pentose phosphate, tricarboxylic acid (TCA) cycle, and oxidative phosphorylation are all affected, and their activity is coordinated to support enhanced proliferation even at the expense of energetic efficiency. Intermittent and variable hypoxia, which is ubiquitous in solid tumors, further exacerbates this phenotype.
Cancer cells frequently encounter hypoxic conditions which result in mitochondrial membrane hyperpolarization, leading to inhibition of mitochondrial transition pores that normally release pro-apoptotic factors. In these hypoxic conditions, hypoxia-induced factor 1-alpha (HIF1α) is activated, which increases glucose transporter expression. This allows increased glucose into the cell, amplifies transcription of glycolytic enzymes, and prompts a switch to aerobic glycolysis to produce ATP (7, 8). HIF1α activates pyruvate dehydrogenase kinase 1 (PDK1) which inhibits pyruvate dehydrogenase (PDH), and therefore reduces the supply of acetyl-CoA entering the TCA cycle, shunting carbon flux elsewhere (7). This mitochondrial dysregulation, in both normoxic and hypoxic conditions, allows cancer cells to use excess pyruvate for anabolic synthesis (7). Cancer cells additionally show increased glutamine uptake for glutaminolysis, which refills TCA intermediates that are shunted into heightened biosynthetic processes (9).
Cancer cells also undergo metabolic shifts during treatment with radiation and chemotherapy (10). Radiation’s therapeutic effect is the result of unrepaired DNA damage leading to cell death, and cells must use DNA damage repair pathways to avoid death. DNA damage repair is an energy intensive process, and cancer cells already have a high energy demand making it difficult to allocate excess energy for this process (11, 12). Radiation also induces PI3K and NF-kB pathways along with epithelial-mesenchymal transition transcription factors (SNAI1, HIF1, ZEB1, and STAT3) which can result in metabolic reprograming (11, 13, 14). The effect of chemotherapies on metabolism is vast, depends upon the agent in question, and has been extensively reviewed previously (8, 15). Different chemotherapies can affect glycolysis, fatty acid synthases, glutaminolysis, and other metabolic pathways (15). Chemotherapies promote metabolism shifts that result in drug resistance (16).
Although well designed for maximal proliferation, these metabolic adaptations toward higher biomass turnover present interesting opportunities for therapeutic intervention. First, it is important to note that under conditions of stress such as that generated by chemoradiation, the biomass requirements for survival may surpass even those of normal cancer proliferation and thus present an opportunity for effective combinatorial strategies. Second, targeting of biomass-generating pathways may be preferentially effective in cancer cells while limiting toxicity in normal tissue. It is important to remember that metabolic pathways which are partially dysregulated can become severely sensitive to disruption even with limited inhibition. As such, a third opportunity arises from minimal inhibition of a generally altered pathway (e.g., oxidative phosphorylation) to generate disproportionate reductions in energy and biomass resulting in catastrophic tumor cell death.
How tumor cells regulate intra-cellular ROS remains unclear, but it appears to require a careful interplay between intrinsic tumor biology and the tumor microenvironment (TME). The TME includes the surrounding host immune cells, extracellular matrix (ECM), blood vessels, fibroblasts, lymphocytes, other bone marrow-derived inflammatory cells, and signaling molecules (17). Often, in solid tumors, the TME is characterized by hypoxia, low pH, and high interstitial fluid pressure (18). These conditions further the shift from oxidative respiration to glycolysis and increase lactic acid production resulting in an acidic TME (19). This acidosis boosts reactive oxygen species (ROS) formations leading to RAS-RAF-MEK-MAPK pathway activation, thereby promoting cell proliferation (19, 20).
However, free radicals are a double-edged sword in the context of solid tumors. On the one hand, tumor cells–particularly aggressive, highly proliferative and metastatic cells–appear to thrive in the presence of slightly elevated ROS levels, suggesting that higher ROS levels may be pro-tumorigenic (21). On the other hand, conventional chemotherapy and radiation generate much of their anti-tumorigenic activity by dramatically increasing ROS levels above those which tumor cells can absorb without catastrophic damage to their DNA (22). The TME is a site of significant interaction between these cancer therapeutics and cancer metabolism, and the TME can promote cancer survival by impairing the efficacy of radiation, chemotherapy, and immunotherapy (18, 23–25). Since the cytotoxic effects of radiation are potentiated by oxygen, hypoxic tumor stromal regions of the TME can drive radioresistance and local treatment failure (18). The acidification of the TME promotes cancer cell metabolic reprogramming, selects for cancer cells that are resistant to hypoxic conditions, and therefore promotes chemoresistance and metastatic development (25). The TME and ROS production present a targetable area for cancer therapeutics that will be discussed further in this review.
Even in the absence of immunotherapy, a functional immune system is important for maximal response to treatment in solid tumors, including NSCLC (26). In patients with NSCLC, the presence of tumor-infiltrating lymphocytes (TILs) is associated with improved distant-free survival and overall survival (27–30). Radiation improves antitumoral immunity in the TME by increasing the release of tumor antigens and allowing antigen-presenting cells to activate CD8+ T-cells. This ultimately results in tumor cell death (31, 32).
As demonstrated in the PACIFIC trial, the benefit of adjuvant PD-L1 blockade using durvalumab after chemoradiation in NSCLC is thought to be at least partially due to its ability to block inhibition of CD8+ cytotoxic T cells, which are presumably activated following chemoradiation. The interplay between a broadly immunosuppressive therapy – namely chemoradiation – with these immunogenic characteristics leads to a complicated and not completely understood interaction. The timing of immune-checkpoint blockade is critical as initial studies in other cancers that used immune-checkpoint blockade concurrently with chemoradiation have underperformed (33), unlike in the consolidation setting of immune checkpoint blockade in PACIFIC.
Adding to this complexity is the effect of tumor metabolism on the TME. In the TME, excess lactate is immunosuppressive (25, 34). The tumor stroma suppresses host immune responses against malignant tumor cells: restricting T-cells from making contact with the cancer cells, secreting immunosuppressive cytokines, and metabolic competition between tumor cells and immune cells (32). Members of the host immune system such as B-cells, T-cells, NK cells, dendritic cells, and macrophages are present in the TME, yet the interactions between malignant and non-malignant cells in the TME create a carcinogenic environment with metabolic stress and often render immune cells less functional (24). The TME induces host immune cells to become tolerogenic to cancer cells, and the immune cells become unproductive in cancer suppression. This ultimately results in decreased immunotherapy effectiveness (35).
Drugs targeting cancer metabolism may synergistically enhance immunotherapy due to the significant overlap with the TME (36). Indeed, agents targeting tumor metabolism could have multiple benefits on immune response both by creating a TME more favorable to immune function as well as directly affecting the tumor-infiltrating lymphocytes to improve their cytotoxic function (37). Some examples of metabolic intervention tactics to improve immunotherapy response include: 1) PI3K-AKT-mTOR blockade which can simultaneously inhibit cancer metabolism and disinhibit the immune system via inhibition of regulatory T cells (38) and 2) targeting glutaminolysis via glutaminase (GLS) or arginase 1 to promote differentiation and function of CD4 and CD8 T cells (39–42). In an in-depth analysis, Guerra et al. argue that different players in the TME use or modify energetic pathways in different types of cancers which could be used to design cancer-specific immune metabolic modulators. These approaches in metabolic manipulation in combination with chemoradiation may enhance immune cell fitness in the TME. Specifically, because radio- and chemotherapy induce tumor cell death, more nutrients become available in the TME for immune cells to use to their own advantage (35).
The complexity of the immune system with multiple immune cell types using different metabolic pathways and responding to different stimuli should not be understated (43). Metabolic manipulation must be done in a thoughtful way to promote anti-tumor immunity and not blunt its response (14, 44). For example, once stimulated, effector T cells increase glycolysis and macronutrient production (45), but memory T cells rely on OXPHOS with a lower glycolysis rate (45, 46). Tumor cells have also been demonstrated to competitively uptake glucose to inhibit TILs (47), whereas PD-1 blockade increases glucose in TILs and promotes glycolysis (35). An in-depth review of different immune cell metabolic profiles has been previously published (48). These findings suggest caution when using metabolic modulation anti-cancer therapies in combination with immunotherapy, and that temporal and spatial interaction will be important.
As noted previously, cancer’s metabolic adaptations to the tumor microenvironment create barriers to effective immunotherapy applications, supporting the addition of metabolic modulators to therapeutic regimens for NSCLC (34). Metabolic modulator use in the treatment of NSCLC may improve radiotherapy and immunotherapy efficacy, and the interplay between therapeutic agents in the TME supports the continued exploration of chemoradiation, immunotherapy, and metabolic modulators.
Completion of TCGA-led efforts over the last decade have unraveled the genomic, transcriptomic, and proteomic landscape of lung cancer in a manner never previously thought possible (49, 50). Among NSCLC subtypes, adenocarcinomas have a higher incidence of actionable mutations and are more prevalent in non-smokers (50). Squamous cell carcinomas have a higher frequency of non-actionable mutations such as TP53 (~80-90% of cases) and are more common in smokers (49, 51). The two most commonly mutated genes in NSCLC are TP53 and KRAS, with KRAS being almost exclusively in adenocarcinomas. The two most commonly mutated signaling pathways are PI3K-Akt-mTOR and RAF-MEK-MAPK (50). By examining these mutations, potential candidates for metabolic modulation can be identified.
TP53 is mutated in 46% of NSCLC tumors (50), and mutated TP53 is associated with worse patient outcomes (52). Although not directly actionable currently, TP53 mutations result in loss of normal protein function which provide several unique opportunities for metabolic intervention. First, mutated TP53 results in reduced apoptosis by TP53-induced glycolysis and apoptosis regulator (TIGAR) suppression and increased glycolysis and glucose transport by expression of phosphoglucomutase (PGM), losing the ability to block G6PD activity (53). Second, when glycolysis is pharmacologically inhibited, mutant TP53 NSCLC tumor cells lines are unable to upregulate OXPHOS compared to wild type TP53, suggesting a mechanism to target mutant TP53 (54). The high prevalence of TP53 mutations makes it an attractive target in NSCLC.
The RAS-RAF-MEK-MAPK pathway is mutated in 58% of all NSCLC tumors and 76% of lung adenocarcinomas (50, 55). In lung adenocarcinoma, activating mutations in oncogenes KRAS (32%), EGFR (11%), and BRAF (7%) are common (50). It should be noted that KRAS and EGFR mutations are mutually exclusive. Alteration in this pathway results in heightened expression of central glycolytic enzymes. Oncogenic RAS mutations keep RAS membrane proteins in their GTP-bound, active state, resulting in uncontrolled growth (8). KRAS mutations have higher prevalence in smokers, but are still seen in non-smokers (56). Mutated KRAS is associated with radioresistance in NSCLC and other cancers (57–59). Mutated KRAS NSCLC tumors are associated with higher expression of PD-L1/2, promoting an immunoevasive environment (60). Once thought to be untargetable, a newly approved KRAS small-molecular inhibitor for patients with KRASC12C demonstrated an objective response rate of ~33%, and provides hope of being able to improve outcomes in these patients (61). Mutated KRAS affects reactive oxygen species (ROS) regulation and promotes radio- and chemoresistance (59, 62).
The PI3K-Akt-mTOR signal transduction pathway manages glucose regulation and activates oncogenic mutations leading to angiogenesis, proliferation, and differentiation among other downstream effects (8). Therefore, it provides a direct link between tumorigenesis, treatment response, and metabolic targeting. In NSCLC, common mutations of this pathway include serine/threonine kinase 11 (STK11 aka LKB1) which is inactivated in 17% of cases, PTEN (3%), PIK3CA (4%), and AKT1 (1%) (50). STK11 encodes the tumor suppressor enzyme serine/threonine kinase 11, which when lost can increase flux of glucose-derived carbon towards serine biosynthesis, support DNA methylation, and promote oncogenic metabolic phenotypes (63). Loss of STK11 is associated with locoregional recurrence after radiation (64).The mechanism of resistance is in part driven by the Kelch-like ECH-associated protein 1 (Keap1)/nuclear factor erythroid-2-related factor 2 (NFR2) pathway (64, 65).
The Keap1/NRF2 pathway is essential in metabolic and ROS regulation. In homeostatic conditions, Keap1 with other proteins ubiquitinates Nrf2 leading to its degradation via proteosomes (66). When cells undergo an oxidative stress, Keap1 is oxidized preventing Nrf2 ubiquitination which results in Nrf2 accumulation (67). Nrf2 is able to translocate to the nucleus, interacts with nuclear proteins, and transcriptionally activates antioxidant gene response (68). Nrf2 regulates Mdm2, NAD(P)H quinone dehydrogenase 1 (NQO1), heme oxygenase 1 (HMOX1), and glutamate-cysteine ligase modifier subunit (GCLM) among others (69–72). As an oxidative stress regulator, Nrf2 is important in the regulation of cancer metabolism. It up-regulates metabolic pathways including glycolysis/gluconeogenesis, pyruvate metabolism, pentose phosphate pathway, glutathione metabolism, and others leads to increased production of catabolic building blocks of nucleic acids (73), carbohydrates, amino acids, and lipids which are vital for tumor survival (65). Because KEAP1 is inactivated by high levels of ROS resulting in activation of Nrf2, Nrf2 is one of the master regulators of anti-oxidative transcription factors.
In NSCLC, loss-of-function mutations in Keap1 and gain-of-functions of Nrf2 have been observed in 11.3% and 3.5% of patients, respectively (74). These mutations result in a constitutively active Nrf2 (75, 76). In patients with lung adenocarcinoma, patients with high Keap1 and low Nrf2 have better clinical outcomes than patients with low Keap1 and high Nrf2 (74, 76). In NSCLC, mutated KRAS leads to NRF2 upregulation promoting chemoresistance and radioresistance (62, 77). Nrf-2 inhibition has been demonstrated to be a radiosensitizer in other cancers as well (78). Co-mutation of KRAS and KEAP1/NFE2L2 predicts worse survival to chemotherapy and immunotherapy (79), and Nrf-2-regulated ROS homeostasis has been linked to chemotherapy resistance in NSCLC and other cancers (76, 80–83). Knockdown of NRF2 in NSCLC cell lines results in higher basal ROS causing radiosensitization (84). When examining subtype-specific transcriptional phenotypes in both KRAS mutant and wild type NSCLC, the proliferative tumor subtype were STK11/KEAP1 deficient in ~90% and ~75% of KRAS mutant and wild type tumors, respectively (85), and the proliferative tumor subtype appeared to respond to MEK inhibition (85). The small molecule inhibitor of Nrf-2, IM3829, resulted in radiosensitization in in vitro and in vivo experiments in NSCLC and demonstrated expected increase in ROS and apoptosis (86). Due to Nrf-2 promoting both radioresistance and chemoresistance, NRF-2 inhibition makes for a rational target for improving NSCLC chemoradiation. Targeting the KEAP1/NFR2 pathway by inhibiting glutaminase may radiosensitize tumors with the STK11 mutation (64). Glutaminase (GLS) inhibition blocks the conversion of glutamine to glutamate, decreasing glutathione production. Inhibition of GLS by CB-938 leads to NSCLC tumor cell sensitization to radiation (87–89). GLS inhibitors are currently in clinical trials in solid tumors with chemotherapy combination, but not currently any with radiation (90, 91). This example demonstrates the potential for metabolic modulators to improve concurrent chemoradiation.
Moreover, bioinformatic analysis of multiple smoking related malignancies including NSCLC has identified a strong correlation between Nrf2 hyperactivation and a suppressive tumor immune microenvironment, depleted of effector immunocytes (Sandulache et al., In press). Experimental data both in vitro and in immunocompetent pre-clinical models have identified glutathione peroxidase 2 (GPX2), an Nrf2 target and a critical regulator of oxidative stress response, as a primary driver of suppressed immunity, manifested through reduced cytokine and chemokine production by tumor cells, development of tumors depleted of cytotoxic T cells, and enriched for myeloid derived suppressor cells.
AMP-activated protein kinase (AMPK) is a key regulator of cellular energy metabolism implicated in the PI3K-Akt-mTOR cell signaling pathway, an important molecule in both metabolic and genomic signaling pathways (92–94), and induces p53 activation promoting cell survival and senescence (95–97). AMPK is targeted in metabolic syndrome and type 2 diabetes mellitus, and it has been proposed as a metabolic tumor suppressor in cancer treatment (98). In cancer microenvironments, insulin activates the PI3K/Akt signal transduction pathway and inhibits GSK3, a tumor suppressor that inactivates glycogen synthase by phosphorylation (99, 100). GSK3 inhibition results in cancer cells storing and synthesizing glycogen in abnormally high quantities, promoting cancer cell survival (101). SKT11 (aka LKB1) is upstream of AMPK, normally leading to its activation, however this effect may not be present in the setting of STK11 mutation (102). As radiation normally activates AMPK, and reversal of this activation leads to radioresistance, this may be a mechanism by which STK11 mutation drives therapeutic response independent of Keap1/Nrf2 (92, 93).
The high rates of mutations of TP53 and KRAS and dysregulation of PI3K-Akt-mTOR and RAF-MEK-MAPK mechanistically supports and demonstrates the importance of enhanced biomass requirements of rapidly proliferating cells. This is particularly seen in conditions of energy deprivation seen in the TME (e.g., AMPK sensing low ATP levels). Although aberrant in cancer, NSCLC must tightly regulate the metabolism, otherwise, the most likely outcome is a catastrophic metabolic disruption resulting in cell death. By exploiting this careful balance, metabolic modulators may enhance the effects of radiation, chemotherapy, and immunotherapy.
Metabolic dysfunction is a risk factor for many types of cancer. Hyperinsulinemia and hyperglycemia predict for increased cancer incidence and worse patient survival outcomes in a variety of cancers (103, 104). Diabetic cancer patients experience worse prognoses than non-diabetic patients, resulting in a 0-40% increase in overall mortality for different types of cancer (105). Diabetes mellitus is an independent prognostic factor for worse outcomes in patients with locally advanced NSCLC (106), and hyperglycemia predicted for worse survival in these patients when treated with concurrent chemoradiation (107). Paradoxically, obesity is linked with decreased risk of lung cancer and better outcomes. This has been attributed to confounders such as smoking and lung cancer-associated weight loss (108–110). In metabolic syndrome (characterized by three of five characteristics of abdominal obesity, hypertriglyceridemia, hyperlipidemia, hypertension and/or hyperglycemia), there has also been association with increased risk of certain cancers (111). Both obesity and metabolic dysfunction are associated with a state of chronic inflammation (111, 112). Emerging evidence demonstrates that obesity creates an immunosuppressive environment in the TME (113), but there is evidence demonstrating patients with obesity have better responses to immunotherapy, especially in NSCLC (114). Because of these links between metabolic dysregulation and cancer, investigation into effective metabolic modulators is warranted to potentially incorporate them into cancer therapeutics and understand the full implications between these two diseases.
In patients with type 2 diabetes, metformin is considered the initial drug of choice for management of hyperglycemia (115). Metformin inhibits hepatic gluconeogenesis via interaction with the mitochondrial electron transport chain and disrupts cAMP-PKA signaling (116). Epidemiologic studies in diabetic patients show that metformin use is associated with decreased risk of cancer in comparison to other anti-diabetic treatments (117), and specifically in NSCLC incidence (118–120). In diabetic patients taking metformin there is a survival benefit compared to diabetic patients not taking metformin or the nondiabetic population in all cancers (121). In other solid cancer subsites, metformin use is associated with improved outcomes with radiotherapy and chemoradiotherapy (122–124). In advanced NSCLC, patients with type II diabetes taking metformin had improved PFS and OS compared to those patients taking insulin or other anti-diabetic medications (125). In patients with diabetes and non-operable NSCLC, metformin use is associated with significantly longer overall survival suggesting that metformin may have an anti-tumorigenic effect (126).
In preclinical models, metformin suppresses oncogenic pathways such as EGFR signaling, insulin-like growth factor, and acts as a radiation sensitizer in NSCLC by activating AMPK, suppressing mTOR, and inducing G1 cell-cycle arrest (98, 127–129). Metformin inhibits NSCLC cancer growth and increases radiosensitivity with limited effect on normal lung cells (128). The mechanism of action of metformin is thought to be inhibition of the mitochondrial complex I of oxidative phosphorylation via mitochondrial complex I (130). Phenformin, a mitochondrial inhibitor and analog of metformin, has been shown to selectively induce apoptosis in STK11-deficient NSCLC cells and prolong survival in murine models of NSCLC tumors with KRAS and STK11 mutations (131).
Metformin also demonstrates potentiation of antitumor immunity. In a preclinical model of head and neck cancer, long-term metformin treatment reduces tumor growth velocity, increases tumor infiltrating lymphocyte levels, and increases the CD8+/T-reg ratio (a measure of immune cell upregulation) thereby creating local and systemic immune effects (132). Furthermore, given that hypoxia in the TME acts as a barrier to immunotherapy, a recent study determined that metformin led to reduction of tumor hypoxia which improved the efficacy of PD-1 blockade and could reduce immunotherapy resistance (133).
Based on this promising preclinical work and retrospective clinical data, metformin has been pursued as a therapeutic sensitizing agent. Metformin is one of the top ten prescribed pharmaceuticals in the US, with a favorable adverse effects profile. Metformin is associated with low rates of hypoglycemia and is generally well-tolerated, with most common side effects being gastrointestinal. While rare, lactic acidosis is the most severe side effect of metformin use as a diabetic treatment. This safety and side effect profile made metformin an optimal target for incorporation with chemoradiation in NSCLC clinical trials in non-diabetic NSCLC patients and its potential synergy with immunotherapy.
Unfortunately, the integration of metformin into clinical trials for cancers has underperformed. In the locally advanced NSCLC definitive chemoradiation setting, recent clinical trials demonstrate no benefit of metformin use. NRG-LU001, a phase II clinical trial of non-diabetic patients with locally advanced unresectable NSCLC, tested the addition of metformin to concurrent chemoradiation. It demonstrated no significant difference in PFS or OS at one year compared to standard chemoradiation, failing to meet its primary endpoint (134, 135). The OCOG-ALMERA trial, a phase II clinical trial in non-diabetic patients with unresectable locally advanced NSCLC, demonstrated that concurrent and adjuvant metformin with chemoradiation compared to chemoradiation alone had worse 1-year treatment failure, 69.2% versus 42.9%, p =0.05, and 1-year PFS, 34.8% versus 63.0%, respectively (136). There were also higher rates of grade 3+ adverse events in the metformin arm (136). These trials demonstrate that metformin does not improve outcomes with standard of care chemoradiation in an unselected non-diabetic patient population.
A phase II clinical trial in inoperable early-stage NSCLC tested the addition of neoadjuvant and concurrent metformin to hypofractionated radiation (50 Gy in 4 fractions or 70 Gy in 10 fractions). Metformin resulted in increased SUV in tumors at mid-treatment PET after neoadjuvant metformin use, contrary to its expected effect on most physiologic tissues. Metabolic responses determined with PERCIST criteria on PET imaging show complete metabolic response in 69% of the metformin cohort 6 months post-radiation treatment (137). In a Phase II trial of non-diabetic stage IIIB/IV patients with EGFR-mutated lung adenocarcinoma, the addition of metformin to EGFR tyrosine kinase resulted in improved PFS and OS (138). These results offer hope for properly selected NSCLC patient populations such as those patients with EGFR or KRAS/STK11 mutations (139).
Metformin became an attractive target for investigation in the clinical setting for two reasons. First, the preclinical data in multiple disease models was fairly compelling both in the single agent setting and when combined with chemoradiation (98, 127–129). Second, metformin has an unparalleled safety profile as a systemic agent used for DM management. This made it perfect for repurposing. Unfortunately, metformin failed to benefit an unselected patient population in combination with chemoradiation in NSCLC. This highlights the complexity of interactions between radiation, chemotherapy, cancer metabolism, and the TME. However, results such as those in EGFR-mutated lung adenocarcinoma gives hope that in an appropriately selected patient population, metformin could improve outcomes.
Most strategies rely on inhibition of major metabolic pathways, predominantly glycolysis with the exception of the Nrf2 based strategies outlined above. This poses substantial challenges as they relate to both efficacy and normal tissue toxicity. Multiple potential metabolic enzyme-targeting therapies currently in preclinical and clinical trials not involving NSCLC are shown in Table 1. Those being tested in NSCLC are discussed here and summarized in Table 2. Future exploration focuses on inhibition of glucose transport and metabolism and inhibition of monocarboxylate transport to prevent lactate excretion.
Glucose transporters (GLUT) inhibitors (fasentin, phloretin, STF-31, DRB18 and WZB117) are in preclinical development for lung cancer (8). WZB117 and DRB18 demonstrated in vitro and in vivo inhibition in NSCLC cancer models (149, 150). Pyruvate kinase M2 (PKM2) activation results in a higher ratio of glycolysis to glucose oxidation and appears to be inactivated in cancer (7). Shikonin, a PMK2 inhibitor, demonstrated response rates in late-stage lung cancer in patients who were not candidates for surgery, radiotherapy, or chemotherapy (152, 153). Pyruvate dehydrogenase kinase 1 (PDK1) blocks PDH activity. Dichloroacetate (DCA), a PDK inhibitor, is an orally available drug that shows potential benefit in many cancer types, including reduced tumor growth, increased apoptosis in vivo, and a shift from glycolysis to OXPHOS in NSCLC 7 (Table 1) (154). However, a phase II clinical trial in metastatic NSCLC or breast cancer investigating DCA response demonstrated safety and risk concerns and was stopped prematurely (8). Lactate dehydrogenase A (LDHA), which converts cytosolic pyruvate into lactate (157), has been associated with radioresistance and chemoresistance in other cancers (158, 158). LDHA is clinically targetable by PSTMB, which demonstrates induction of apoptosis in NSCLC cancer cell lines (8). It has been proposed that LDHA inhibition must be done with careful dose and temporal control due to the significant off-target side effects of this molecule (159). Due to the importance of these metabolic proteins, this might apply to a substantial number of metabolic inhibitors and possibly limit the translation to the clinic. Another potential metabolic target is fructose-1,6-bisphosphatase (FBP1), which when aberrant causes dysfunction in NK cells of the immune system. Inhibition of FBP1 suggests restoration of NK cell function during tumor growth (160). However, it remains unclear whether these approaches can achieve significant efficacy without normal tissue toxicity.
Although most of this discussion has focused on the addition of potential metabolic modulators to concurrent chemoradiation, the use of metabolic imaging is vital for target delineation of current radiation therapy. Molecular imaging in lung cancer has been discussed extensively in these previous reviews (161–163). Fluorodeoxyglucose (FDG)-PET scans are standard of care for the appropriate staging of newly diagnosed patients with NSCLC. Use of PET-CT has been demonstrated to increase compliance with stage appropriate treatment and result in a decreased 5-year mortality (164). When used in conjunction with invasive mediastinal staging techniques, FDG-PET has a high sensitivity and specificity for mediastinal lymph node staging, allowing for improved target delineation when designing radiation treatment plans. By treating only involved mediastinal lymph nodes and avoiding traditional elective lymph nodes, PET-CT radiation planning technique has demonstrated at least equivalent local-regional control rate with decreased pneumonitis (165–167). Current studies are examining the role of mid-treatment FDG PET-guided imaging to guide treatment intensification (168).
Different metabolic pathways are currently under investigation for improving NSCLC prognostication and prediction. As discussed above, tumor hypoxia is an area of radioresistance within a tumor. Metabolic hypoxia PET tracers are being studied to guide radiation intensification. The most commonly used hypoxia markers are the nitroimidazole class of compounds with (18)F-fluoromisonidazole ((18)FMISO-) (169, 170) being the most studied. When using both FDG-PET and (18)F-FMISO imaging, a combined pattern can predict for risk of recurrence after SBRT (171). In head and neck cancer (18), F-FMISO PET/CT has been used to identify tumor areas with hypoxia requiring dose escalation (172).
Additionally, over the last decade, MRI of hyperpolarized 13C pyruvate - lactate conversion has matured in a technology which can be used to identify high risk solid tumors in early stage clinical trials (173, 174). In preclinical studies, 13C pyruvate HP-MRI has been advanced as a near real-time readout of intra-tumoral shifts in oxidative stress, and corresponding changes in carbon flux from pyruvate into lactate are able to be determined. At a basic level, HP-MRI leverages all of the aspects of altered solid tumor metabolism outlined above and may provide the first real-time biomarker of chemotherapy and radiation response in solid tumors. This may be more challenging to implement in lung cancer based upon motion artifact, but, along with CT-based imaging with dedicated radiotracers, represents an interesting avenue to explore (175–177).
Cancer cells have significant changes in glucose transport and metabolism, glycolysis, catabolic metabolism, mitochondrial oxidative cycle and aerobic fermentation, and the cancer TME. A better understanding of these complex interactions can help developing cancer therapeutics to improve chemoradiation and immunotherapy in patients with NSCLC. In this subset of locally advanced patients requiring chemoradiation, the prognosis has improved significantly with the addition of immunotherapy, but it still remains poor.
Metformin generated significant interest because initial retrospective data in diabetic patients suggested that metformin improved outcomes in patients receiving chemoradiation. Unfortunately, recent phase II clinical trials in nondiabetic patients did not show benefit of the addition of metformin. Taken together, these results demonstrate that in unselected nondiabetic patient populations, metformin does not improve outcomes with chemoradiation. So, how do we explain these findings and where does this leave metformin in NSCLC? Due to metformin being first-line treatment for type II diabetes mellitus, retrospective analysis could possibly be confounded in patients receiving appropriate management or patients taking metformin have less severe disease. As discussed previously, obesity is associated with type II diabetes mellitus, and the possibility that the previously discussed obesity paradox impacted patient outcomes instead of metformin cannot be excluded. Also, the hyperglycemia and hyperinsulinemia state in diabetic patients could cause differential effect on tumors such as the known increase in AMPK. The question if metformin will be useful in any clinical situations will likely depend on patient selection. As discussed previously, metformin may work better in certain patient populations such as KRAS and STK11 mutations, suggesting there may be certain genetic mutations that would benefit. However, more preclinical and clinical data will be needed to confirm this. It remains to be seen how metabolic modulators can be used improve immunotherapy outcomes. Could metformin be added to adjuvant immunotherapy after chemoradiation to improve outcomes? Does the concurrent and adjuvant timing matter? Now that adjuvant immunotherapy is the standard of care, this question will become more important.
Incorporation of future metabolic modulators into chemoradiation therapy is not ready for prime time. Further work-up will be needed before incorporation of many of these metabolic modulators into clinical trials. However, there is rationale for incorporation in chemoradiation and immunotherapy which deserves significant consideration.
AD and PP contributed equally to this manuscript. HS, AD, and PP were responsible for conceptualization, methodology, original draft and visualization. VS was responsible for conceptualization and reviewing/editing. HS is responsible for supervision. All authors contributed to the article and approved the submitted version.
The authors declare that the research was conducted in the absence of any commercial or financial relationships that could be construed as a potential conflict of interest.
All claims expressed in this article are solely those of the authors and do not necessarily represent those of their affiliated organizations, or those of the publisher, the editors and the reviewers. Any product that may be evaluated in this article, or claim that may be made by its manufacturer, is not guaranteed or endorsed by the publisher.
1. Torre LA, Bray F, Siegel RL, Ferlay J, Lortet-Tieulent J, Jemal A. Global cancer statistics, 2012. CA: A Cancer J Clin (2015) 65(2):87–108. doi: 10.3322/caac.21262
2. Siegel RL, Miller KD, Jemal A. Cancer statistics, 2018. CA: A Cancer J Clin (2018) 68(1):7–30. doi: 10.3322/caac.21442
3. Siegel RL, Miller KD, Fuchs HE, Jemal A. Cancer statistics, 2022. CA: A Cancer J Clin (2022) 72(1):7–33. doi: 10.3322/caac.21708
4. Faivre-Finn C, Vicente D, Kurata T, Planchard D, Paz-Ares L, Vansteenkiste JF, et al. Four-year survival with durvalumab after chemoradiotherapy in stage III NSCLC–an update from the PACIFIC trial. J Thorac Oncol (2021) 16(5):860–7. doi: 10.1016/j.jtho.2020.12.015
5. Warburg O, Wind F, Negelein E. The metabolism of tumors in the body. J Gen Physiol (1927) 8(6):519–30. doi: 10.1085/jgp.8.6.519
6. Heiden MGV, DeBerardinis RJ. Understanding the intersections between metabolism and cancer biology. Cell (2017) 168(4):657–69. doi: 10.1016/j.cell.2016.12.039
7. Kinnaird A, Michelakis ED. Metabolic modulation of cancer: a new frontier with great translational potential. J Mol Med (2015) 93(2):127–42. doi: 10.1007/s00109-014-1250-2
8. Vanhove K, Graulus GJ, Mesotten L, Thomeer M, Derveaux E, Noben JP, et al. The metabolic landscape of lung cancer: New insights in a disturbed glucose metabolism. Front Oncol (2019) 9:1215. doi: 10.3389/fonc.2019.01215
9. Schulze A, Harris AL. How cancer metabolism is tuned for proliferation and vulnerable to disruption. Nature (2012) 491(7424):364–73. doi: 10.1038/nature11706
10. van Gisbergen MW, Zwilling E, Dubois LJ. Metabolic rewiring in radiation oncology toward improving the therapeutic ratio. Front Oncol (2021) 11:653621. doi: 10.3389/fonc.2021.653621
11. Tang L, Wei F, Wu Y, He Y, Shi L, Xiong F, et al. Role of metabolism in cancer cell radioresistance and radiosensitization methods. J Exp Clin Cancer Res (2018) 37(1):87. doi: 10.1186/s13046-018-0758-7
12. Qin L, Fan M, Candas D, Jiang G, Papadopoulos S, Tian L, et al. CDK1 enhances mitochondrial bioenergetics for radiation-induced DNA repair. Cell Rep Elsevier; (2015) 13(10):2056–63. doi: 10.1016/j.celrep.2015.11.015
13. Edwards E, Geng L, Tan J, Onishko H, Donnelly E, Hallahan DE. Phosphatidylinositol 3-kinase/Akt signaling in the response of vascular endothelium to ionizing radiation. Cancer Res (2002) 62(16):4671–7.
14. Xia L, Tan S, Zhou Y, Lin J, Wang H, Oyang L, et al. Role of the NFκB-signaling pathway in cancer. Onco Targets Ther (2018) 11:2063–73. doi: 10.2147/OTT.S161109
15. Desbats MA, Giacomini I, Prayer-Galetti T, Montopoli M. Metabolic plasticity in chemotherapy resistance. Front Oncol (2020) 10:281. doi: 10.3389/fonc.2020.00281
16. Zaal EA, Berkers CR. The influence of metabolism on drug response in cancer. Front Oncol (2018) 8:500. doi: 10.3389/fonc.2018.00500
17. Arneth B. Tumor microenvironment. medicina Vol. 56. Basel, Switzerland: Multidisciplinary Digital Publishing Institute (2020). p. 15.
18. Matsuo M, Matsumoto S, Mitchell JB, Krishna MC, Camphausen K. Magnetic resonance imaging of the tumor microenvironment in radiotherapy: Perfusion, hypoxia, and metabolism. Semin Radiat Oncol (2014) 24(3):210–7. doi: 10.1016/j.semradonc.2014.02.002
19. Riemann A, Schneider B, Ihling A, Nowak M, Sauvant C, Thews O, et al. Acidic environment leads to ROS-induced MAPK signaling in cancer cells. PloS One (2011) 6(7):e22445. doi: 10.1371/journal.pone.0022445
20. Zhang W, Liu HT. MAPK signal pathways in the regulation of cell proliferation in mammalian cells. Cell Res (2002) 12(1):9–18. doi: 10.1038/sj.cr.7290105
21. Aggarwal V, Tuli HS, Varol A, Thakral F, Yerer MB, Sak K, et al. Role of reactive oxygen species in cancer progression: Molecular mechanisms and recent advancements. Biomolecules (2019) 9(11):735. doi: 10.3390/biom9110735
22. Kim W, Lee S, Seo D, Kim D, Kim K, Kim E, et al. Cellular stress responses in radiotherapy. Cells (2019) 8(9):1105. doi: 10.3390/cells8091105
23. Sauvant C, Nowak M, Wirth C, Schneider B, Riemann A, Gekle M, et al. Acidosis induces multi-drug resistance in rat prostate cancer cells (AT1) in vitro and in vivo by increasing the activity of the p-glycoprotein via activation of p38. Int J Cancer (2008) 123(11):2532–42. doi: 10.1002/ijc.23818
24. Balkwill FR, Capasso M, Hagemann T. The tumor microenvironment at a glance. J Cell Sci (2012) 125(23):5591–6. doi: 10.1242/jcs.116392
25. Sertorio M, Perentesis JP, Vatner RE, Mascia AE, Zheng Y, Wells SI. Cancer cell metabolism: Implications for X-ray and particle radiation therapy. Int J Particle Ther (2018) 5(1):40–8. doi: 10.14338/IJPT-18-00023.1
26. Carbone DP, Gandara DR, Antonia SJ, Zielinski C, Paz-Ares L. Non–small cell lung cancer: Role of the immune system and potential for immunotherapy. J Thorac Oncol (2015) 10(7):974–84. doi: 10.1097/JTO.0000000000000551
27. Ruffini E, Asioli S, Filosso PL, Lyberis P, Bruna MC, Macrì L, et al. Clinical significance of tumor-infiltrating lymphocytes in lung neoplasms. Ann Thorac Surg (2009) 87(2):365–72. doi: 10.1016/j.athoracsur.2008.10.067
28. Horne ZD, Jack R, Gray ZT, Siegfried JM, Wilson DO, Yousem SA, et al. Increased levels of tumor-infiltrating lymphocytes are associated with improved recurrence-free survival in stage 1A non-small-cell lung cancer. J Surg Res (2011) 171(1):1–5. doi: 10.1016/j.jss.2011.03.068
29. Hiraoka K, Miyamoto M, Cho Y, Suzuoki M, Oshikiri T, Nakakubo Y, et al. Concurrent infiltration by CD8+ T cells and CD4+ T cells is a favourable prognostic factor in non-small-cell lung carcinoma. Br J Cancer (2006) 94(2):275–80. doi: 10.1038/sj.bjc.6602934
30. Zhuang X, Xia X, Wang C, Gao F, Shan N, Zhang L, et al. A high number of CD8+ T cells infiltrated in NSCLC tissues is associated with a favorable prognosis. Appl Immunohistochem Mol Morphol (2010) 18(1):24–8. doi: 10.1097/PAI.0b013e3181b6a741
31. Nardone V, Pastina P, Giannicola R, Agostino R, Croci S, Tini P, et al. How to increase the efficacy of immunotherapy in NSCLC and HNSCC: Role of radiation therapy, chemotherapy, and other strategies. Front Immunol (2018) 9:2941. doi: 10.3389/fimmu.2018.02941
32. Menon H, Ramapriyan R, Cushman TR, Verma V, Kim HH, Schoenhals JE, et al. Role of radiation therapy in modulation of the tumor stroma and microenvironment. Front Immunol (2019) 10:193. doi: 10.3389/fimmu.2019.00193
33. Lee NY, Ferris RL, Psyrri A, Haddad RI, Tahara M, Bourhis J, et al. Avelumab plus standard-of-care chemoradiotherapy versus chemoradiotherapy alone in patients with locally advanced squamous cell carcinoma of the head and neck: a randomised, double-blind, placebo-controlled, multicentre, phase 3 trial. Lancet Oncol Elsevier; (2021) 22(4):450–62. doi: 10.1016/S1470-2045(20)30737-3
34. Romero-Garcia S, Moreno-Altamirano MMB, Prado-Garcia H, Sánchez-García FJ. Lactate contribution to the tumor microenvironment: Mechanisms, effects on immune cells and therapeutic relevance. Front Immunol (2016) 7:52. doi: 10.3389/fimmu.2016.00052
35. Guerra L, Bonetti L, Brenner D. Metabolic modulation of immunity: A new concept in cancer immunotherapy. Cell Rep (2020) 32(1):107848. doi: 10.1016/j.celrep.2020.107848
36. Li X, Wenes M, Romero P, Huang SCC, Fendt SM, Ho PC. Navigating metabolic pathways to enhance antitumour immunity and immunotherapy. Nat Rev Clin Oncol (2019) 16(7):425–41. doi: 10.1038/s41571-019-0203-7
37. Rangel Rivera GO, Knochelmann HM, Dwyer CJ, Smith AS, Wyatt MM, Rivera-Reyes AM, et al. Fundamentals of T cell metabolism and strategies to enhance cancer immunotherapy. Front Immunol (2021) 12:645242. doi: 10.3389/fimmu.2021.645242
38. O’Donnell JS, Massi D, Teng MWL, Mandala M. PI3K-AKT-mTOR inhibition in cancer immunotherapy, redux. Semin Cancer Biol (2018) 48:91–103. doi: 10.1016/j.semcancer.2017.04.015
39. Johnson MO, Wolf MM, Madden MZ, Andrejeva G, Sugiura A, Contreras DC, et al. Distinct regulation of Th17 and Th1 cell differentiation by glutaminase-dependent metabolism. Cell Elsevier; (2018) 175(7):1780–95. doi: 10.1016/j.cell.2018.10.001
40. Nabe S, Yamada T, Suzuki J, Toriyama K, Yasuoka T, Kuwahara M, et al. Reinforce the antitumor activity of CD8+ T cells via glutamine restriction. Cancer Sci (2018) 109(12):3737–50. doi: 10.1111/cas.13827
41. Tannir NM, Motzer RJ, Agarwal N, Liu PY, Whiting SH, O’Keeffe B, et al. CANTATA: A randomized phase 2 study of CB-839 in combination with cabozantinib vs. placebo with cabozantinib in patients with advanced/metastatic renal cell carcinoma. JCO (2018) 36(15_suppl):TPS4601–TPS4601. doi: 10.1200/JCO.2019.37.7_suppl.TPS682
42. Steggerda SM, Bennett MK, Chen J, Emberley E, Huang T, Janes JR, et al. Inhibition of arginase by CB-1158 blocks myeloid cell-mediated immune suppression in the tumor microenvironment. J Immunother Cancer BMJ Specialist Journals; (2017) 5(1):101. doi: 10.1186/s40425-017-0308-4
43. Ghesquière B, Wong BW, Kuchnio A, Carmeliet P. Metabolism of stromal and immune cells in health and disease. Nature (2014) 511(7508):167–76. doi: 10.1038/nature13312
44. Xia L, Oyang L, Lin J, Tan S, Han Y, Wu N, et al. The cancer metabolic reprogramming and immune response. Mol Cancer (2021) 20(1):28. doi: 10.1186/s12943-021-01316-8
45. Pearce EL, Poffenberger MC, Chang CH, Jones RG. Fueling immunity: insights into metabolism and lymphocyte function. Science (2013) 342(6155):1242454. doi: 10.1126/science.1242454
46. Tan S, Li S, Min Y, Gisterå A, Moruzzi N, Zhang J, et al. Platelet factor 4 enhances CD4+ T effector memory cell responses via akt-PGC1α-TFAM signaling-mediated mitochondrial biogenesis. J Thromb Haemostasis (2020) 18(10):2685–700. doi: 10.1111/jth.15005
47. Qiu J, Villa M, Sanin DE, Buck MD, O’Sullivan D, Ching R, et al. Acetate promotes T cell effector function during glucose restriction. Cell Rep (2019) 27(7):2063–2074.e5. doi: 10.1016/j.celrep.2019.04.022
48. Pearce EL, Pearce EJ. Metabolic pathways in immune cell activation and quiescence. Immunity (2013) 38(4):633–43. doi: 10.1016/j.immuni.2013.04.005
49. Hammerman PS, Lawrence MS, Voet D, Jing R, Cibulskis K, Sivachenko A, et al. Comprehensive genomic characterization of squamous cell lung cancers. Nature (2012) 489(7417):519–25. doi: 10.1038/nature11404
50. Collisson EA, Campbell JD, Brooks AN, Berger AH, Lee W, Chmielecki J, et al. Comprehensive molecular profiling of lung adenocarcinoma. Nature (2014) 511(7511):543–50. doi: 10.1038/nature13385
51. Herbst RS, Morgensztern D, Boshoff C. The biology and management of non-small cell lung cancer. Nature (2018) 553(7689):446–54. doi: 10.1038/nature25183
52. Gu J, Zhou Y, Huang L, Ou W, Wu J, Li S, et al. TP53 mutation is associated with a poor clinical outcome for non-small cell lung cancer: Evidence from a meta-analysis. Mol Clin Oncol (2016) 5(6):705–13. doi: 10.3892/mco.2016.1057
53. Jiang P, Du W, Wang X, Mancuso A, Gao X, Wu M, et al. p53 regulates biosynthesis through direct inactivation of glucose-6-phosphate dehydrogenase. Nat Cell Biol (2011) 13(3):310–6. doi: 10.1038/ncb2172
54. Sinthupibulyakit C, Ittarat W, St. Clair WH, St. Clair DK. p53 protects lung cancer cells against metabolic stress. Int J Oncol (2010) 37(6):1575–81. doi: 10.3892/ijo_00000811
55. Sinkala M, Nkhoma P, Mulder N, Martin DP. Integrated molecular characterisation of the MAPK pathways in human cancers reveals pharmacologically vulnerable mutations and gene dependencies. Commun Biol (2021) 4(1):1–16. doi: 10.1038/s42003-020-01552-6
56. Riely GJ, Kris MG, Rosenbaum D, Marks J, Li A, Chitale DA, et al. Frequency and distinctive spectrum of KRAS mutations in never smokers with lung adenocarcinoma. Clin Cancer Res (2008) 14(18):5731–4. doi: 10.1158/1078-0432.CCR-08-0646
57. Bernhard EJ, Stanbridge EJ, Gupta S, Gupta AK, Soto D, Bakanauskas VJ, et al. Direct evidence for the contribution of activated n-ras and K-ras oncogenes to increased intrinsic radiation resistance in human tumor cell lines. Cancer Res (2000) 60(23):6597–600.
58. Cassidy RJ, Zhang X, Patel PR, Shelton JW, Escott CE, Sica GL, et al. Next-generation sequencing and clinical outcomes of patients with lung adenocarcinoma treated with stereotactic body radiotherapy. Cancer (2017) 123(19):3681–90. doi: 10.1002/cncr.30794
59. Wang M, Han J, Marcar L, Black J, Liu Q, Li X, et al. Radiation resistance in KRAS-mutated lung cancer is enabled by stem-like properties mediated by an osteopontin-EGFR pathway. Cancer Res (2017) 77(8):2018–28. doi: 10.1158/0008-5472.CAN-16-0808
60. Calles A, Liao X, Sholl LM, Rodig SJ, Freeman GJ, Butaney M, et al. Expression of PD-1 and its ligands, PD-L1 and PD-L2, in smokers and never smokers with KRAS-mutant lung cancer. J Thorac Oncol (2015) 10(12):1726–35. doi: 10.1097/JTO.0000000000000687
61. Skoulidis F, Li BT, Dy GK, Price TJ, Falchook GS, Wolf J, et al. Sotorasib for lung cancers with KRAS p.G12C mutation. New Engl J Med (2021) 384(25):2371–81. doi: 10.1056/NEJMoa2103695
62. Tao S, Wang S, Moghaddam SJ, Ooi A, Chapman E, Wong PK, et al. Oncogenic KRAS confers chemoresistance by upregulating NRF2. Cancer Res (2014) 74(24):7430–41. doi: 10.1158/0008-5472.CAN-14-1439
63. Skoulidis F, Heymach JV. Co-Occurring genomic alterations in non-small cell lung cancer biology and therapy. Nat Rev Cancer (2019) 19(9):495–509. doi: 10.1038/s41568-019-0179-8
64. Sitthideatphaiboon P, Galan-Cobo A, Negrao MV, Qu X, Poteete A, Zhang F, et al. STK11/LKB1 mutations in NSCLC are associated with KEAP1/NRF2-dependent radiotherapy resistance targetable by glutaminase inhibition. Clin Cancer Res (2021) 27(6):1720–33. doi: 10.1158/1078-0432.CCR-20-2859
65. Zhao J, Lin X, Meng D, Zeng L, Zhuang R, Huang S, et al. Nrf2 mediates metabolic reprogramming in non-small cell lung cancer. Front Oncol (2020) 10:578315. doi: 10.3389/fonc.2020.578315
66. Itoh K, Ye P, Matsumiya T, Tanji K, Ozaki T. Emerging functional cross-talk between the Keap1-Nrf2 system and mitochondria. J Clin Biochem Nutr (2015) 56(2):91–7. doi: 10.3164/jcbn.14-134
67. Sporn MB, Liby KT. NRF2 and cancer: the good, the bad and the importance of context. Nat Rev Cancer (2012) 12(8):564–71. doi: 10.1038/nrc3278
68. Yamamoto M, Kensler TW, Motohashi H. The KEAP1-NRF2 system: a thiol-based sensor-effector apparatus for maintaining redox homeostasis. Physiol Rev (2018) 98(3):1169–203. doi: 10.1152/physrev.00023.2017
69. Venugopal R, Jaiswal AK. Nrf1 and Nrf2 positively and c-fos and Fra1 negatively regulate the human antioxidant response element-mediated expression of NAD(P)H:quinone oxidoreductase1 gene. Proc Natl Acad Sci U S A (1996) 93(25):14960–5. doi: 10.1073/pnas.93.25.14960
70. Lignitto L, LeBoeuf SE, Homer H, Jiang S, Askenazi M, Karakousi TR, et al. Nrf2 activation promotes lung cancer metastasis by inhibiting the degradation of Bach1. Cell (2019) 178(2):316–329.e18. doi: 10.1016/j.cell.2019.06.003
71. Solis WA, Dalton TP, Dieter MZ, Freshwater S, Harrer JM, He L, et al. Glutamate-cysteine ligase modifier subunit: mouse gclm gene structure and regulation by agents that cause oxidative stress. Biochem Pharmacol (2002) 63(9):1739–54. doi: 10.1016/S0006-2952(02)00897-3
72. You A, won Nam C, Wakabayashi N, Yamamoto M, Kensler TW, Kwak MK. Transcription factor Nrf2 maintains the basal expression of Mdm2: An implication of the regulation of p53 signaling by Nrf2. Arch Biochem Biophysics (2011) 507(2):356–64. doi: 10.1016/j.abb.2010.12.034
73. Namani A, Liu K, Wang S, Zhou X, Liao Y, Wang H, et al. Genome-wide global identification of NRF2 binding sites in A549 non-small cell lung cancer cells by ChIP-seq reveals NRF2 regulation of genes involved in focal adhesion pathways. Aging (Albany NY) (2019) 11(24):12600–23. doi: 10.18632/aging.102590
74. Frank R, Scheffler M, Merkelbach-Bruse S, Ihle MA, Kron A, Rauer M, et al. Clinical and pathological characteristics of KEAP1- and NFE2L2-mutated non-small cell lung carcinoma (NSCLC). Clin Cancer Res (2018) 24(13):3087–96. doi: 10.1158/1078-0432.CCR-17-3416
75. Singh A, Misra V, Thimmulappa RK, Lee H, Ames S, Hoque MO, et al. Dysfunctional KEAP1–NRF2 interaction in non-Small-Cell lung cancer. PloS Med Public Library Sci; (2006) 3(10):e420. doi: 10.1371/journal.pmed.0030420
76. Ohta T, Iijima K, Miyamoto M, Nakahara I, Tanaka H, Ohtsuji M, et al. Loss of Keap1 function activates Nrf2 and provides advantages for lung cancer cell growth. Cancer Res (2008) 68(5):1303–9. doi: 10.1158/0008-5472.CAN-07-5003
77. Yang L, Shen C, Estrada-Bernal A, Robb R, Chatterjee M, Sebastian N, et al. Oncogenic KRAS drives radioresistance through upregulation of NRF2-53BP1-mediated non-homologous end-joining repair. Nucleic Acids Res (2021) 49(19):11067–82. doi: 10.1093/nar/gkab871
78. Zhou S, Ye W, Shao Q, Zhang M, Liang J. Nrf2 is a potential therapeutic target in radioresistance in human cancer. Crit Rev Oncol/Hematol (2013) 88(3):706–15. doi: 10.1016/j.critrevonc.2013.09.001
79. Arbour KC, Jordan E, Kim HR, Dienstag J, Yu HA, Sanchez-Vega F, et al. Effects of Co-occurring genomic alterations on outcomes in patients with KRAS-mutant non-small cell lung cancer. Clin Cancer Res (2018) 24(2):334–40. doi: 10.1158/1078-0432.CCR-17-1841
80. Chen X, Wu Q, Chen Y, Zhang J, Li H, Yang Z, et al. Diosmetin induces apoptosis and enhances the chemotherapeutic efficacy of paclitaxel in non-small cell lung cancer cells via Nrf2 inhibition. Br J Pharmacol (2019) 176(12):2079–94. doi: 10.1111/bph.14652
81. Tang X, Wang H, Fan L, Wu X, Xin A, Ren H, et al. Luteolin inhibits Nrf2 leading to negative regulation of the Nrf2/ARE pathway and sensitization of human lung carcinoma A549 cells to therapeutic drugs. Free Radical Biol Med (2011) 50(11):1599–609. doi: 10.1016/j.freeradbiomed.2011.03.008
82. Zhong Y, Zhang F, Sun Z, Zhou W, Li ZY, You QD, et al. Drug resistance associates with activation of Nrf2 in MCF-7/DOX cells, and wogonin reverses it by down-regulating Nrf2-mediated cellular defense response. Mol Carcinogenesis (2013) 52(10):824–34. doi: 10.1002/mc.21921
83. Homma S, Ishii Y, Morishima Y, Yamadori T, Matsuno Y, Haraguchi N, et al. Nrf2 enhances cell proliferation and resistance to anticancer drugs in human lung cancer. Clin Cancer Res (2009) 15(10):3423–32. doi: 10.1158/1078-0432.CCR-08-2822
84. Singh A, Bodas M, Wakabayashi N, Bunz F, Biswal S. Gain of Nrf2 function in non-small-cell lung cancer cells confers radioresistance. Antioxid Redox Signal (2010) 13(11):1627–37. doi: 10.1089/ars.2010.3219
85. Daemen A, Cooper JE, Myrta S, Wongchenko MJ, Lin E, Long JE, et al. Transcriptional subtypes resolve tumor heterogeneity and identify vulnerabilities to MEK inhibition in lung adenocarcinoma. Clin Cancer Res (2021) 27(4):1162–73. doi: 10.1158/1078-0432.CCR-20-1835
86. Lee S, Lim MJ, Kim MH, Yu CH, Yun YS, Ahn J, et al. An effective strategy for increasing the radiosensitivity of human lung cancer cells by blocking Nrf2-dependent antioxidant responses. Free Radical Biol Med (2012) 53(4):807–16. doi: 10.1016/j.freeradbiomed.2012.05.038
87. Kery M, Papandreou I. Emerging strategies to target cancer metabolism and improve radiation therapy outcomes. BJR (2020) 93(1115):20200067. doi: 10.1259/bjr.20200067
88. Boysen G, Jamshidi-Parsian A, Davis MA, Siegel ER, Simecka CM, Kore RA, et al. Glutaminase inhibitor CB-839 increases radiation sensitivity of lung tumor cells and human lung tumor xenografts in mice. Int J Radiat Biol (2019) 95(4):436–42. doi: 10.1080/09553002.2018.1558299
89. Sappington DR, Siegel ER, Hiatt G, Desai A, Penney RB, Jamshidi-Parsian A, et al. Glutamine drives glutathione synthesis and contributes to radiation sensitivity of A549 and H460 lung cancer cell lines. Biochim Biophys Acta (BBA) - Gen Subjects (2016) 1860(4):836–43. doi: 10.1016/j.bbagen.2016.01.021
90. Eads JR, Krishnamurthi SS, Saltzman JN, Bajor DL, Vinayak S, Barnholtz-Sloan J, et al. Phase I clinical trial of the glutaminase inhibitor CB-839 plus capecitabine in patients with advanced solid tumors. JCO (2018) 36(15_suppl):2562–2. doi: 10.1200/JCO.2018.36.15_suppl.2562
91. Calithera Biosciences, Inc. A phase 1b/2 open label, dose escalation and expansion study of the glutaminase inhibitor CB-839 in combination with the PARP inhibitor talazoparib in patients with advanced or metastatic solid tumors (2022). Available at: https://clinicaltrials.gov/ct2/show/NCT03875313.
92. Sanli T, Rashid A, Liu C, Harding S, Bristow RG, Cutz JC, et al. Ionizing radiation activates AMP-activated kinase (AMPK): A target for radiosensitization of human cancer cells. Int J Radiat Oncol Biol Phys (2010) 78(1):221–9. doi: 10.1016/j.ijrobp.2010.03.005
93. Sanli T, Steinberg GR, Singh G, Tsakiridis T. AMP-activated protein kinase (AMPK) beyond metabolism. Cancer Biol Ther (2014) 15(2):156–69. doi: 10.4161/cbt.26726
94. Storozhuk Y, Sanli T, Hopmans SN, Schultz C, Farrell T, Cutz JC, et al. Chronic modulation of AMP-kinase, akt and mTOR pathways by ionizing radiation in human lung cancer xenografts. Radiat Oncol (2012) 7:71. doi: 10.1186/1748-717X-7-71
95. Jones RG, Plas DR, Kubek S, Buzzai M, Mu J, Xu Y, et al. AMP-activated protein kinase induces a p53-dependent metabolic checkpoint. Mol Cell (2005) 18(3):283–93. doi: 10.1016/j.molcel.2005.03.027
96. Thoreen CC, Sabatini DM. AMPK and p53 help cells through lean times. Cell Metab (2005) 1(5):287–8. doi: 10.1016/j.cmet.2005.04.009
97. Lee DH, Lee TH, Jung CH, Kim YH. Wogonin induces apoptosis by activating the AMPK and p53 signaling pathways in human glioblastoma cells. Cell Signal (2012) 24(11):2216–25. doi: 10.1016/j.cellsig.2012.07.019
98. Li W, Saud SM, Young MR, Chen G, Hua B. Targeting AMPK for cancer prevention and treatment. Oncotarget (2015) 6(10):7365–78. doi: 10.18632/oncotarget.3629
99. Ferreira LMR. Cancer metabolism: The warburg effect today. Exp Mol Pathol (2010) 89(3):372–80. doi: 10.1016/j.yexmp.2010.08.006
100. Jope RS, Yuskaitis CJ, Beurel E. Glycogen synthase kinase-3 (GSK3): Inflammation, diseases, and therapeutics. Neurochem Res (2007) 32(4):577–95. doi: 10.1007/s11064-006-9128-5
101. Pelletier J, Bellot G, Gounon P, Lacas-Gervais S, Pouyssegur J, Mazure N. Glycogen synthesis is induced in hypoxia by the hypoxia-inducible factor and promotes cancer cell survival. Front Oncol (2012) 2:18. doi: 10.3389/fonc.2012.00018
102. Shackelford DB, Shaw RJ. The LKB1–AMPK pathway: metabolism and growth control in tumour suppression. Nat Rev Cancer Nat Publishing Group; (2009) 9(8):563–75. doi: 10.1038/nrc2676
103. Weiser MA, Cabanillas ME, Konopleva M, Thomas DA, Pierce SA, Escalante CP, et al. Relation between the duration of remission and hyperglycemia during induction chemotherapy for acute lymphocytic leukemia with a hyperfractionated cyclophosphamide, vincristine, doxorubicin, and dexamethasone/methotrexate–cytarabine regimen. Cancer (2004) 100(6):1179–85. doi: 10.1002/cncr.20071
104. Derr RL, Ye X, Islas MU, Desideri S, Saudek CD, Grossman SA. Association between hyperglycemia and survival in patients with newly diagnosed glioblastoma. JCO (2009) 27(7):1082–6. doi: 10.1200/JCO.2008.19.1098
105. van de Poll-Franse LV, Houterman S, Janssen-Heijnen MLG, Dercksen MW, Coebergh JWW, Haak HR. Less aggressive treatment and worse overall survival in cancer patients with diabetes: A large population based analysis. Int J Cancer (2007) 120(9):1986–92. doi: 10.1002/ijc.22532
106. Imai H, Kaira K, Mori K, Ono A, Akamatsu H, Matsumoto S, et al. Prognostic significance of diabetes mellitus in locally advanced non-small cell lung cancer. BMC Cancer (2015) 15:989. doi: 10.1186/s12885-015-2012-4
107. Bergamino M, Rullan AJ, Saigí M, Peiró I, Montanya E, Palmero R, et al. Fasting plasma glucose is an independent predictor of survival in patients with locally advanced non-small cell lung cancer treated with concurrent chemoradiotherapy. BMC Cancer (2019) 19(1):165. doi: 10.1186/s12885-019-5370-5
108. Lam VK, Bentzen SM, Mohindra P, Nichols EM, Bhooshan N, Vyfhuis M, et al. Obesity is associated with long-term improved survival in definitively treated locally advanced non-small cell lung cancer (NSCLC). Lung Cancer (2017) 104:52–7. doi: 10.1016/j.lungcan.2016.11.017
109. Yu D, Zheng W, Johansson M, Lan Q, Park Y, White E, et al. Overall and central obesity and risk of lung cancer: A pooled analysis. J Natl Cancer Inst (2018) 110(8):831–42. doi: 10.1093/jnci/djx286
110. Mavridis K, Michaelidou K. The obesity paradox in lung cancer: is there a missing biological link? J Thorac Dis (2019) 11(Suppl 3):S363–6. doi: 10.21037/jtd.2018.12.69
111. Esposito K, Chiodini P, Colao A, Lenzi A, Giugliano D. Metabolic syndrome and risk of cancer. Diabetes Care (2012) 35(11):2402–11. doi: 10.2337/dc12-0336
112. Ellulu MS, Patimah I, Khaza’ai H, Rahmat A, Abed Y. Obesity and inflammation: the linking mechanism and the complications. Arch Med Sci (2017) 13(4):851–63. doi: 10.5114/aoms.2016.58928
113. Ringel AE, Drijvers JM, Baker GJ, Catozzi A, García-Cañaveras JC, Gassaway BM, et al. Obesity shapes metabolism in the tumor microenvironment to suppress anti-tumor immunity. Cell (2020) 183(7):1848–1866.e26. doi: 10.1016/j.cell.2020.11.009
114. Yoo SK, Chowell D, Valero C, Morris LGT, Chan TA. Outcomes among patients with or without obesity and with cancer following treatment with immune checkpoint blockade. JAMA Network Open (2022) 5(2):e220448. doi: 10.1001/jamanetworkopen.2022.0448
115. American Diabetes Association. Standards of medical care in diabetes–2020 abridged for primary care providers. Clin Diabetes (2020) 38(1):10–38. doi: 10.2337/cd20-as01
116. Pernicova I, Korbonits M. Metformin–mode of action and clinical implications for diabetes and cancer. Nat Rev Endocrinol (2014) 10(3):143–56. doi: 10.1038/nrendo.2013.256
117. DeCensi A, Puntoni M, Goodwin P, Cazzaniga M, Gennari A, Bonanni B, et al. Metformin and cancer risk in diabetic patients: A systematic review and meta-analysis. Cancer Prev Res (2010) 3(11):1451–61. doi: 10.1158/1940-6207.CAPR-10-0157
118. Hsieh MC, Lee TC, Cheng SM, Tu ST, Yen MH, Tseng CH. The influence of type 2 diabetes and glucose-lowering therapies on cancer risk in the Taiwanese. Exp Diabetes Res (2012) 2012:413782. doi: 10.1155/2012/413782
119. Mazzone PJ, Rai H, Beukemann M, Xu M, Jain AK, Sasidhar M. The effect of metformin and thiazolidinedione use on lung cancer in diabetics. BMC Cancer (2012) 12(1):410. doi: 10.1186/1471-2407-12-410
120. Noto H, Goto A, Tsujimoto T, Noda M. Cancer risk in diabetic patients treated with metformin: a systematic review and meta-analysis. PloS One (2012) 7(3):e33411. doi: 10.1371/journal.pone.0033411
121. Currie CJ, Poole CD, Jenkins-Jones S, Gale EAM, Johnson JA, Morgan CL. Mortality after incident cancer in people with and without type 2 diabetes: impact of metformin on survival. Diabetes Care (2012) 35(2):299–304. doi: 10.2337/dc11-1313
122. Sandulache VC, Hamblin JS, Skinner HD, Kubik MW, Myers JN, Zevallos JP. Association between metformin use and improved survival in patients with laryngeal squamous cell carcinoma. Head Neck (2014) 36(7):1039–43. doi: 10.1002/hed.23409
123. Skinner HD, Crane CH, Garrett CR, Eng C, Chang GJ, Skibber JM, et al. Metformin use and improved response to therapy in rectal cancer. Cancer Med (2013) 2(1):99–107. doi: 10.1002/cam4.54
124. Skinner HD, McCurdy MR, Echeverria AE, Lin SH, Welsh JW, O’Reilly MS, et al. Metformin use and improved response to therapy in esophageal adenocarcinoma. Acta Oncol (2013) 52(5):1002–9. doi: 10.3109/0284186X.2012.718096
125. Tan BX, Yao WX, Ge J, Peng XC, Du XB, Zhang R, et al. Prognostic influence of metformin as first-line chemotherapy for advanced nonsmall cell lung cancer in patients with type 2 diabetes. Cancer (2011) 117(22):5103–11. doi: 10.1002/cncr.26151
126. Chuang MC, Yang YH, Tsai YH, Hsieh MJ, Lin YC, Lin CK, et al. Survival benefit associated with metformin use in inoperable non-small cell lung cancer patients with diabetes: A population-based retrospective cohort study. PloS One (2018) 13(1):e0191129. doi: 10.1371/journal.pone.0191129
127. Levy A, Doyen J. Metformin for non-small cell lung cancer patients: Opportunities and pitfalls. Crit Rev Oncol Hematol (2018) 125:41–7. doi: 10.1016/j.critrevonc.2018.03.001
128. Storozhuk Y, Hopmans SN, Sanli T, Barron C, Tsiani E, Cutz JC, et al. Metformin inhibits growth and enhances radiation response of non-small cell lung cancer (NSCLC) through ATM and AMPK. Br J Cancer (2013) 108(10):2021–32. doi: 10.1038/bjc.2013.187
129. Song CW, Lee H, Dings RPM, Williams B, Powers J, Santos TD, et al. Metformin kills and radiosensitizes cancer cells and preferentially kills cancer stem cells. Sci Rep (2012) 2:362. doi: 10.1038/srep00362
130. Wheaton WW, Weinberg SE, Hamanaka RB, Soberanes S, Sullivan LB, Anso E, et al. Metformin inhibits mitochondrial complex I of cancer cells to reduce tumorigenesis. eLife (2014) 3:e02242. doi: 10.7554/eLife.02242
131. Shackelford DB, Abt E, Gerken L, Vasquez DS, Seki A, Leblanc M, et al. LKB1 inactivation dictates therapeutic response of non-small cell lung cancer to the metabolism drug phenformin. Cancer Cell (2013) 23(2):143–58. doi: 10.1016/j.ccr.2012.12.008
132. Veeramachaneni R, Yu W, Newton JM, Kemnade JO, Skinner HD, Sikora AG, et al. Metformin generates profound alterations in systemic and tumor immunity with associated antitumor effects. J Immunother Cancer (2021) 9(7):e002773. doi: 10.1136/jitc-2021-002773
133. Scharping NE, Menk AV, Whetstone RD, Zeng X, Delgoffe GM. Efficacy of PD-1 blockade is potentiated by metformin-induced reduction of tumor hypoxia. Cancer Immunol Res (2017) 5(1):9–16. doi: 10.1158/2326-6066.CIR-16-0103
134. Skinner H, Hu C, Tsakiridis T, Santana-Davila R, Lu B, Erasmus J, et al. OA12.03 initial reporting of NRG-LU001, randomized phase II trial of concurrent chemoradiotherapy +/- metformin HCL in locally advanced NSCLC. J Thorac Oncol (2019) 14(10):S237–8. doi: 10.1016/j.jtho.2019.08.473
135. Skinner H, Hu C, Tsakiridis T, Santana-Davila R, Lu B, Erasmus JJ, et al. Addition of metformin to concurrent chemoradiation in patients with locally advanced non–small cell lung cancer: The NRG-LU001 phase 2 randomized clinical trial. JAMA Oncol (2021) 7(9):1324–32. doi: 10.1001/jamaoncol.2021.2318
136. Tsakiridis T, Pond GR, Wright J, Ellis PM, Ahmed N, Abdulkarim B, et al. Metformin in combination with chemoradiotherapy in locally advanced non–small cell lung cancer: The OCOG-ALMERA randomized clinical trial. JAMA Oncol (2021) 7(9):1333–41. doi: 10.1001/jamaoncol.2021.2328
137. Chun SG, Liao Z, Jeter MD, Chang JY, Lin SH, Komaki RU, et al. Metabolic responses to metformin in inoperable early-stage non–small cell lung cancer treated with stereotactic Radiotherapy : Results of a randomized phase II clinical trial. Am J Clin Oncol (2020) 43(4):231–5. doi: 10.1097/COC.0000000000000632
138. Arrieta O, Barrón F, Padilla MÁS, Avilés-Salas A, Ramírez-Tirado LA, Arguelles Jiménez MJ, et al. Effect of metformin plus tyrosine kinase inhibitors compared with tyrosine kinase inhibitors alone in patients with epidermal growth factor receptor–mutated lung adenocarcinoma: A phase 2 randomized clinical trial. JAMA Oncol (2019) 5(11):e192553. doi: 10.1001/jamaoncol.2019.2553
139. Eze C, Belka C, Manapov F. Forging a path for metformin use in inoperable locally advanced non–small cell lung cancer. JAMA Oncol (2021) 7(9):1341–2. doi: 10.1001/jamaoncol.2021.2316
140. Rohle D, Popovici-Muller J, Palaskas N, Turcan S, Grommes C, Campos C, et al. An inhibitor of mutant IDH1 delays growth and promotes differentiation of glioma cells. Science (2013) 340(6132):626–30. doi: 10.1126/science.1236062
141. Wang F, Travins J, DeLaBarre B, Penard-Lacronique V, Schalm S, Hansen E, et al. Targeted inhibition of mutant IDH2 in leukemia cells induces cellular differentiation. Science (2013) 340(6132):622–6. doi: 10.1126/science.1234769
142. Dang L, Yen K, Attar EC. IDH mutations in cancer and progress toward development of targeted therapeutics. Ann Oncol (2016) 27(4):599–608. doi: 10.1093/annonc/mdw013
143. Luengo A, Gui DY, Vander Heiden MG. Targeting metabolism for cancer therapy. Cell Chem Biol (2017) 24(9):1161–80. doi: 10.1016/j.chembiol.2017.08.028
144. Beloueche-Babari M, Casals Galobart T, Delgado-Goni T, Wantuch S, Parkes HG, Tandy D, et al. Monocarboxylate transporter 1 blockade with AZD3965 inhibits lipid biosynthesis and increases tumour immune cell infiltration. Br J Cancer (2020) 122(6):895–903. doi: 10.1038/s41416-019-0717-x
145. Porporato PE, Dhup S, Dadhich RK, Copetti T, Sonveaux P. Anticancer targets in the glycolytic metabolism of tumors: A comprehensive review. Front Pharmacol (2011) 2:49. doi: 10.3389/fphar.2011.00049
146. Brenner A, Falchook G, Patel M, Infante J, Arkenau HT, Dean E, et al. Abstract P6-11-09: Heavily pre-treated breast cancer patients show promising responses in the first in human study of the first-in-class fatty acid synthase (FASN) inhibitor, TVB-2640 in combination with paclitaxel. Cancer Res (2017) 77(4_Supplement):P6-11–09. doi: 10.1158/1538-7445.SABCS16-P6-11-09
147. Tsuji A, Akao T, Masuya T, Murai M, Miyoshi H. IACS-010759, a potent inhibitor of glycolysis-deficient hypoxic tumor cells, inhibits mitochondrial respiratory complex I through a unique mechanism. J Biol Chem (2020) 295(21):7481–91. doi: 10.1074/jbc.RA120.013366
148. Berruti A, Bitossi R, Gorzegno G, Bottini A, Alquati P, De Matteis A, et al. Time to progression in metastatic breast cancer patients treated with epirubicin is not improved by the addition of either cisplatin or lonidamine: Final results of a phase III study with a factorial design. JCO Wolters Kluwer; (2002) 20(20):4150–9. doi: 10.1200/JCO.2002.08.012
149. Liu Y, Cao Y, Zhang W, Bergmeier S, Qian Y, Akbar H, et al. A small-molecule inhibitor of glucose transporter 1 downregulates glycolysis, induces cell-cycle arrest, and inhibits cancer cell growth In vitro and In vivo. Mol Cancer Ther (2012) 11(8):1672–82. doi: 10.1158/1535-7163.MCT-12-0131
150. Shriwas P, Roberts D, Li Y, Wang L, Qian Y, Bergmeier S, et al. A small-molecule pan-class I glucose transporter inhibitor reduces cancer cell proliferation in vitro and tumor growth in vivo by targeting glucose-based metabolism. Cancer Metab (2021) 9(1):14. doi: 10.1186/s40170-021-00248-7
151. Wood TE, Dalili S, Simpson CD, Hurren R, Mao X, Saiz FS, et al. A novel inhibitor of glucose uptake sensitizes cells to FAS-induced cell death. Mol Cancer Ther (2008) 7(11):3546–55. doi: 10.1158/1535-7163.MCT-08-0569
152. Guo XP, Zhang XY, Zhang SD. Clinical trial on the effects of shikonin mixture on later stage lung cancer. Zhong Xi Yi Jie He Za Zhi (1991) 11(10):598–9.
153. Chen J, Xie J, Jiang Z, Wang B, Wang Y, Hu X. Shikonin and its analogs inhibit cancer cell glycolysis by targeting tumor pyruvate kinase-M2. Oncogene (2011) 30(42):4297–306. doi: 10.1038/onc.2011.137
154. Bonnet S, Archer SL, Allalunis-Turner J, Haromy A, Beaulieu C, Thompson R, et al. A mitochondria-k+ channel axis is suppressed in cancer and its normalization promotes apoptosis and inhibits cancer growth. Cancer Cell (2007) 11(1):37–51. doi: 10.1016/j.ccr.2006.10.020
155. Garon EB, Christofk HR, Hosmer W, Britten CD, Bahng A, Crabtree MJ, et al. Dichloroacetate should be considered with platinum-based chemotherapy in hypoxic tumors rather than as a single agent in advanced non-small cell lung cancer. J Cancer Res Clin Oncol (2014) 140(3):443–52. doi: 10.1007/s00432-014-1583-9
156. Kim EY, Chung TW, Han CW, Park SY, Park KH, Jang SB, et al. A novel lactate dehydrogenase inhibitor, 1-(Phenylseleno)-4-(Trifluoromethyl) benzene, suppresses tumor growth through apoptotic cell death. Sci Rep (2019) 9(1):3969. doi: 10.1038/s41598-019-40617-3
157. Tennant DA, Durán RV, Gottlieb E. Targeting metabolic transformation for cancer therapy. Nat Rev Cancer (2010) 10(4):267–77. doi: 10.1038/nrc2817
158. Koukourakis MI, Giatromanolaki A, Panteliadou M, Pouliliou SE, Chondrou PS, Mavropoulou S, et al. Lactate dehydrogenase 5 isoenzyme overexpression defines resistance of prostate cancer to radiotherapy. Br J Cancer (2014) 110(9):2217–23. doi: 10.1038/bjc.2014.158
159. Chen Y, Maniakas A, Tan L, Cui M, Le X, Niedzielski JS, et al. Development of a rational strategy for integration of lactate dehydrogenase a suppression into therapeutic algorithms for head and neck cancer. Br J Cancer (2021) 124(10):1670–9. doi: 10.1038/s41416-021-01297-x
160. Cong J, Wang X, Zheng X, Wang D, Fu B, Sun R, et al. Dysfunction of natural killer cells by FBP1-induced inhibition of glycolysis during lung cancer progression. Cell Metab (2018) 28(2):243–255.e5. doi: 10.1016/j.cmet.2018.06.021
161. Geets X, Sterpin E, Wanet M, Di Perri D, Lee J. Metabolic imaging in non-small-cell lung cancer radiotherapy. Cancer/Radiothérapie (2014) 18(5):402–5. doi: 10.1016/j.canrad.2014.07.146
162. Kaseda K. Recent and current advances in FDG-PET imaging within the field of clinical oncology in NSCLC: A review of the literature. Diagnostics (Basel) (2020) 10(8):561. doi: 10.3390/diagnostics10080561
163. van Genugten EAJ, Weijers JAM, Heskamp S, Kneilling M, van den Heuvel MM, Piet B, et al. Imaging the rewired metabolism in lung cancer in relation to immune therapy. Front Oncol (2022) 11:786089. doi: 10.3389/fonc.2021.786089
164. Vella M, Meyer CS, Zhang N, Cohen BE, Whooley MA, Wang S, et al. Association of receipt of positron emission tomography-computed tomography with non-small cell lung cancer mortality in the veterans affairs health care system. JAMA Netw Open (2019) 2(11):e1915828. doi: 10.1001/jamanetworkopen.2019.15828
165. Rosenzweig KE, Sim SE, Mychalczak B, Braban LE, Schindelheim R, Leibel SA. Elective nodal irradiation in the treatment of non-small-cell lung cancer with three-dimensional conformal radiation therapy. Int J Radiat Oncol Biol Phys (2001) 50(3):681–5. doi: 10.1016/S0360-3016(01)01482-1
166. Yuan S, Sun X, Li M, Yu J, Ren R, Yu Y, et al. A randomized study of involved-field irradiation versus elective nodal irradiation in combination with concurrent chemotherapy for inoperable stage III nonsmall cell lung cancer. Am J Clin Oncol (2007) 30(3):239–44. doi: 10.1097/01.coc.0000256691.27796.24
167. Nestle U, Schimek-Jasch T, Kremp S, Schaefer-Schuler A, Mix M, Küsters A, et al. Imaging-based target volume reduction in chemoradiotherapy for locally advanced non-small-cell lung cancer (PET-plan): a multicentre, open-label, randomised, controlled trial. Lancet Oncol (2020) 21(4):581–92. doi: 10.1016/S1470-2045(20)30013-9
168. Kong FMS, Hu C, Ten Haken R, Xiao Y, Matuszak M, Hirsh V, et al. NRG-RTOG 1106/ACRIN 6697: A phase IIR trial of standard versus adaptive (mid-treatment PET-based) chemoradiotherapy for stage III NSCLC–results and comparison to NRG-RTOG 0617 (non-personalized RT dose escalation). JCO (2021) 39(15_suppl):8548–8. doi: 10.1200/JCO.2021.39.15_suppl.8548
169. Bell C, Dowson N, Fay M, Thomas P, Puttick S, Gal Y, et al. Hypoxia imaging in gliomas with 18F-fluoromisonidazole PET: toward clinical translation. Semin Nucl Med (2015) 45(2):136–50. doi: 10.1053/j.semnuclmed.2014.10.001
170. Rajendran JG, Krohn KA. F-18 fluoromisonidazole for imaging tumor hypoxia: Imaging the microenvironment for personalized cancer therapy. Semin Nucl Med (2015) 45(2):151–62. doi: 10.1053/j.semnuclmed.2014.10.006
171. Watanabe S, Inoue T, Okamoto S, Magota K, Takayanagi A, Sakakibara-Konishi J, et al. Combination of FDG-PET and FMISO-PET as a treatment strategy for patients undergoing early-stage NSCLC stereotactic radiotherapy. EJNMMI Res (2019) 9:104. doi: 10.1186/s13550-019-0578-6
172. Welz S, Paulsen F, Pfannenberg C, Reimold M, Reischl G, Nikolaou K, et al. Dose escalation to hypoxic subvolumes in head and neck cancer: A randomized phase II study using dynamic [18F]FMISO PET/CT. Radiother Oncol (2022) 171:30–6. doi: 10.1016/j.radonc.2022.03.021
173. van Zijl PCM, Brindle K, Lu H, Barker PB, Edden R, Yadav N, et al. Hyperpolarized MRI, functional MRI, MR spectroscopy and CEST to provide metabolic information in vivo. Curr Opin Chem Biol (2021) 63:209–18. doi: 10.1016/j.cbpa.2021.06.003
174. Bankson JA, Walker CM, Ramirez MS, Stefan W, Fuentes D, Merritt ME, et al. Kinetic modeling and constrained reconstruction of hyperpolarized [1-13C]-Pyruvate offers improved metabolic imaging of tumors. Cancer Res (2015) 75(22):4708–17. doi: 10.1158/0008-5472.CAN-15-0171
175. Sandulache VC, Skinner HD, Wang Y, Chen Y, Dodge CT, Ow TJ, et al. Glycolytic inhibition alters anaplastic thyroid carcinoma tumor metabolism and improves response to conventional chemotherapy and radiation. Mol Cancer Ther (2012) 11(6):1373–80. doi: 10.1158/1535-7163.MCT-12-0041
176. Sandulache VC, Chen Y, Lee J, Rubinstein A, Ramirez MS, Skinner HD, et al. Evaluation of hyperpolarized [1-13C]-pyruvate by magnetic resonance to detect ionizing radiation effects in real time. PloS One (2014) 9(1):e87031. doi: 10.1371/journal.pone.0087031
Keywords: lung cancer, radiation, chemo-radiotherapy, metabolism, metformin, immunotherapy
Citation: Dhawan A, Pifer PM, Sandulache VC and Skinner HD (2022) Metabolic targeting, immunotherapy and radiation in locally advanced non-small cell lung cancer: Where do we go from here? Front. Oncol. 12:1016217. doi: 10.3389/fonc.2022.1016217
Received: 10 August 2022; Accepted: 24 November 2022;
Published: 14 December 2022.
Edited by:
Yuen Yee Cheng, University of Technology Sydney, AustraliaReviewed by:
Johann Matschke, Essen University Hospital, GermanyCopyright © 2022 Dhawan, Pifer, Sandulache and Skinner. This is an open-access article distributed under the terms of the Creative Commons Attribution License (CC BY). The use, distribution or reproduction in other forums is permitted, provided the original author(s) and the copyright owner(s) are credited and that the original publication in this journal is cited, in accordance with accepted academic practice. No use, distribution or reproduction is permitted which does not comply with these terms.
*Correspondence: Heath D. Skinner, c2tpbm5lcmhAdXBtYy5lZHU=
†These authors have contributed equally to this work
Disclaimer: All claims expressed in this article are solely those of the authors and do not necessarily represent those of their affiliated organizations, or those of the publisher, the editors and the reviewers. Any product that may be evaluated in this article or claim that may be made by its manufacturer is not guaranteed or endorsed by the publisher.
Research integrity at Frontiers
Learn more about the work of our research integrity team to safeguard the quality of each article we publish.