- Department of Cell Biology and Genetics, School of Basic Medical Sciences, Xi’an Jiaotong University, Xi’an, China
Canonical histone H3.1 and variant H3.3 deposit at different sites of the chromatin via distinct histone chaperones. Histone H3.1 relies on chaperone CAF-1 to mediate replication-dependent nucleosome assembly during S-phase, while H3.3 variant is regulated and incorporated into the chromatin in a replication-independent manner through HIRA and DAXX/ATRX. Current literature suggests that dysregulated expression of histone chaperones may be implicated in tumor progression. Notably, ectopic expression of CAF-1 can promote a switch between canonical H3.1 and H3 variants in the chromatin, impair the chromatic state, lead to chromosome instability, and impact gene transcription, potentially contributing to carcinogenesis. This review focuses on the chaperone proteins of H3.1 and H3.3, including structure, regulation, as well as their oncogenic and tumor suppressive functions in tumorigenesis.
Introduction
In eukaryotes, histone proteins wrap around the DNA to form nucleosomes, which are the building blocks of chromatin. Each nucleosome core particle is composed of around 147bp of DNA and an octamer of histones that is formed of two H3/H4 dimers and two H2A/H2B dimers. Histone H1 is a linker histone that connects adjacent nucleosomes (1). Aside from the canonical histones, H2A, H2B, H3, and H4, histone variants play a pivotal role in regulating chromatin dynamics and the accessibility of the underlying DNA in a locus-specific manner (2). In contrast to the canonical histone, non-canonical histone variant genes locate outside the histone gene clusters, contain introns and their mRNAs have poly(A) tails, which increase the histone diversity. In addition, canonical histones are expressed and incorporated into the chromatin during DNA replication in the S phase (3–6), whereas the assembly of histone variants is replication-independent and spans all phases of the cell cycle (2, 7). Thus non-canonical histone variants may play important roles in other DNA-dependent processes outside the S phase, such as transcription initiation and elongation (8).
In human somatic cells, seven variants of H3 (H3.1, H3.2, H3.3, CENP-A, H3.1T, H3.X, and H3.Y) have been identified (6). Canonical histone variants H3.1 and H3.2 are termed replication-coupled histones because they are incorporated during DNA replication. In addition, H3.2 differs from H3.1 by only one amino acid. Non-canonical histone variant H3.3 is encoded by H3F3A and H3F3B in humans, and it differs from H3.1 by only five amino acids (6). H3.3 dysregulation is implicated in a variety of biological processes: embryonic stem cell differentiation, epigenetic reprogramming, neuron plasticity, centromere maintenance, and DNA damage response (9). To ensure the temporal and spatial correctness of histone functions, histone needs the chaperones to bind histones directly after their synthesis. By definition, histone chaperones are a group of proteins that neutralize the positive charge of histones to prevent non-specific interactions between histones and DNA (10, 11). They are involved in the storage, exchange, and deposition of histones on DNA for assembly (12). Different histone chaperones mediate the deposition of canonical histones and histone variants. Canonical histone H3.1 relies on chaperone anti-silencing factor 1A (CAF-1) to incorporate into chromatin in a replication-dependent manner. H3.3 variant uses specific chaperones: HIRA, DAXX/ATRX complex, and DEK to incorporate into the chromatin in a replication-independent manner (13). Chaperones have been shown to prefer distinct sites for H3.3 assembly. For example, H3.3 requires HIRA to promote its deposition at transient nucleosome-free regions, while DAXX/ATRX is necessary for H3.3 enrichment at heterochromatin (13, 14). DEK maintains chromatin integrity by controlling H3.3 deposition into specific genomic regions (6, 15, 16). Increasing numbers of researchers have demonstrated that histone chaperones are frequently mutated in tumors, indicating that they play a key role in tumorigenesis. Here, we focus on the potential functional roles of histone chaperone proteins CAF-1, HIRA, and ATRX/DAXX in carcinogenesis (Figure 1).
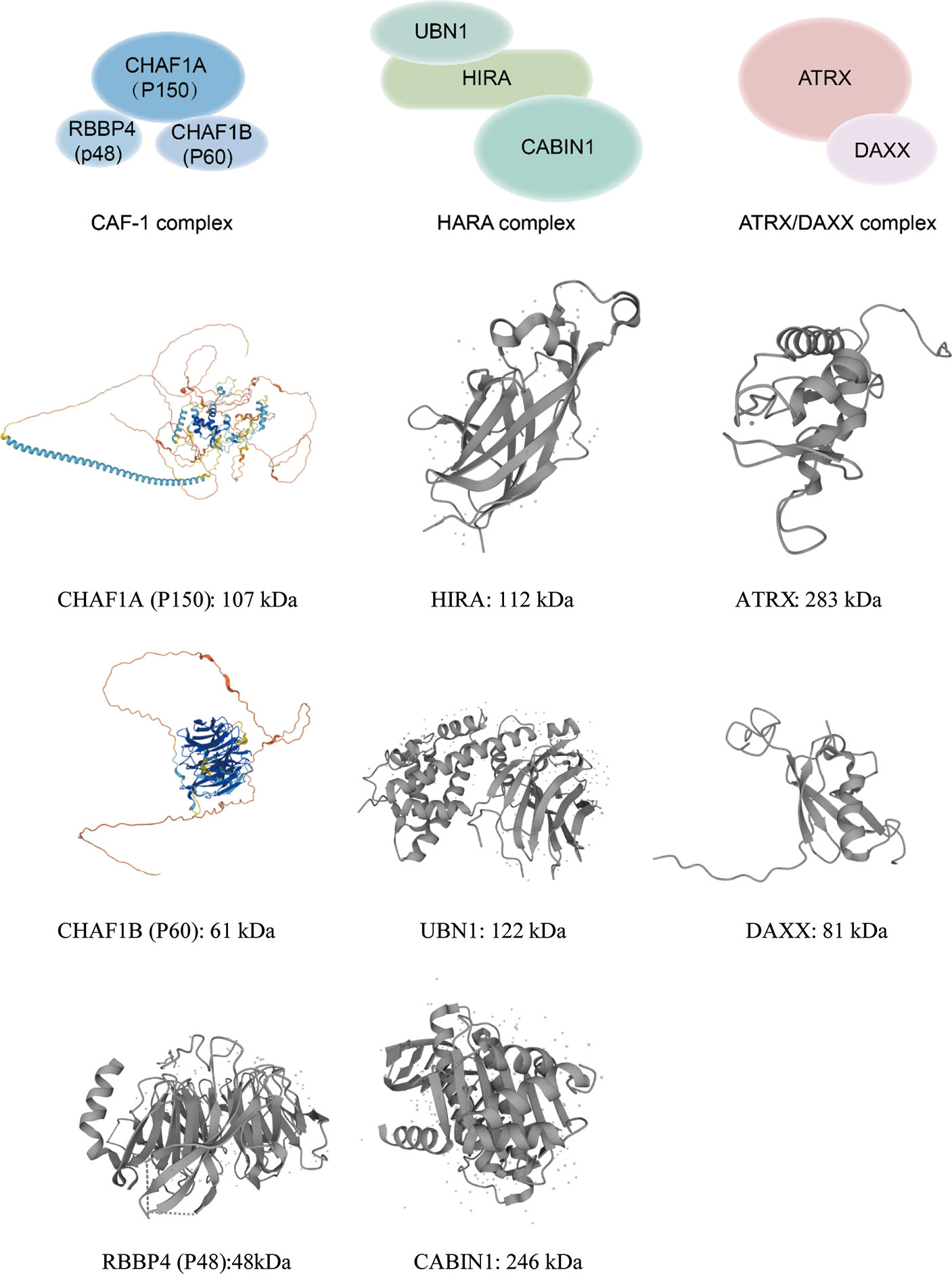
Figure 1 This graph illustrates annotated domains of histone chaperone complexes, along with individual 3D structures of chaperone subunits (3D images from Uniprot 2021).
Histone H3.1 Chaperone: CAF-1 Complex
As an evolutionarily conserved H3/H4 histone chaperone, CAF-1 complex was first identified in DNA replication experiments (17). In humans, CAF-1 consists of three subunits, P150(CHAF1A), P60(CHAF1B), and P48(RBBP4), that were named based on their apparent molecular weight following gel electrophoresis (17). The CHAF1A subunit contains a Winged-Helix Domain (WHD) that binds DNA in a sequence-independent manner. CHAF1A can interact with Proliferating Cell Nuclear Antigen (PCNA) to target the CAF-1 complex at the replication fork. The CHAF1B subunit can deliver H3/H4 by directly interacting with anti-silencing function 1 (ASF1). The P48 subunit contributes to the interaction of histone-modifying enzymes and their substrates. P48 subunit binds independently to fragments of H3/H4 using different interaction surfaces. Overall, the function of CAF-1 complex is to deliver newly synthesized H3/H4 dimers to the replication fork during S phase of cell cycle (18) and participate in DNA damage repair.
CAF-1 and Cancer
The CHAF1B subunit is overexpressed in a variety of tumors, including high-grade glioma, melanomas, prostatic, renal, cervical carcinomas, endometrial tumors, hepatocellular, squamous cell carcinoma, salivary gland tumors, leukemia, and breast cancer (19–29) (Table 1). Moreover, CHAF1B is a major factor for driving metastasis in many different human tumors, as increased protein levels can be used to accurately predict whether or not these tumors will metastasize (18). In hepatocellular carcinoma (HCC), knockdown of the CHAF1B gene reduced the migration and invasion ability of HCC cells, suggesting that the CAF-1 may function as an oncogene (22). In breast cancer, CAF-1 has been shown to be a useful proliferation marker (24). However, in another study, the downregulation of CAF-1 was found to promote the progression of breast cancer (30). Gomes et al. revealed that extracellular regulated protein kinases (ERK) -dependent transfer signal promotes a switch in H3 variants incorporated into chromatin by down-regulating histone chaperones CAF-1 (31). In carcinoma cell lines, the ERK2 signaling reduces the levels of H3.1/H3.2 by suppressing CHAF1B transcription, thus creating the “space” for gap-filling with H3.3, leading to a HIRA-dependent H3.3 enrichment at the promoter of EMT, resulting in tumor progression and metastasis formation. There are few studies focused on CHAF1A, although some have reported its reduction in squamous cell carcinoma (26) and breast cancer (30), indicating potential and anti-cancer effects. Overall these studies indicate that histone chaperones may be valuable therapeutic targets for aggressive tumors.
Histone H3.3 Chaperone: HIRA Complex
The histone cell cycle regulator (HIRA) is an evolutionarily conserved H3/H4 histone chaperone (32, 33). Human HIRA was originally identified in DiGeorge syndrome patients, who commonly have heart and brain abnormalities (34), and later described as a histone chaperone (35). The HIRA complex is composed of HIRA, Ubinuclein-1 (UBN1), and calcineurin-binding protein 1 (CABIN1), which coordinate with ASF1 to bind and deposit H3.3/H4 into the chromatin in a DNA replication-independent manner (36, 37). The HIRA subunit can enhance the binding affinity of UBN1 towards H3.3. The UBN1 subunit is mainly responsible for specific recognition and direct binding of H3.3 (38, 39). In addition to these core partners, HIRA can also directly interact with ASF1b or ASF1a and transfer H3.3/H4 dimers to HIRA complexes (40).
The HIRA complex deposits H3.3 mainly at euchromatin regions such as promoters, enhancers, actively transcribed gene bodies, gene regulatory regions, developmentally regulated genes, and areas of DNA and chromatin damage and repair (37, 41, 42). The HIRA complex interacts with the single-stranded DNA (ssDNA)-binding protein replication factor A (RPA) to deposit newly synthesized H3.3 at gene transcription regulatory elements (42). It has been reported that HIRA can promote transcription recovery after DNA damage as well as maintain global nucleosomal architecture and genomic integrity (32, 43, 44). Furthermore, HIRA binds to naked DNA in vitro and non-nucleosomal regions in vivo, suggesting that deposition of H3.3-gap filling is HIRA-dependent (45). Interestingly, some has suggested that HIRA-mediated H3.3 deposition may be a mechanism to maintain genomic stability when chaperone protein CAF-1 mediated H3.1 deposition is impaired during S-phase (6). Various lines of research suggest that HIRA is involved in a range of processes including embryonic development (46, 47), angiogenesis (48, 49), cellular senescence (50, 51), and early neural development (52).
HIRA and Cancer
HIRA is involved in cellular senescence and is closely related to cancer carcinogenesis (Table 2). Cellular senescence is an irreversible proliferation arrest triggered by short chromosome telomeres, activated oncogenes, and cellular stress. Furthermore, cellular senescence is a known tumor suppressor mechanism. Hall et al. demonstrated that HIRA can interact with Cyclin-CDK2, whose expression blocks S-phase progression and promotes cellular senescence (54). HIRA can interact with ASF1a to form facultative heterochromatin called senescence-associated heterochromatin foci (SAHF), thereby inhibiting cell proliferation and causing cell senescence (50, 51). Another study found that the HIRA mRNA overexpression in chronic myeloid leukemia (CML). Majumder et al. demonstrated that the downregulation of HIRA could induce the differentiation of CML cells and inhibit their proliferation (53). Similarly, in a metastasis-induced breast cancer model, a pronounced upregulation of HIRA and a decrease of CAF-1 can be observed (30). As a histone chaperone that mediates H3.3 gap-filling, knockdown of HIRA suppresses the migration and invasion of human breast cancer cell lines LM2 (30). These studies suggest that HIRA may serve as a potentially effective therapeutic target for metastatic cancer.
Histone H3.3 Chaperone: ATRX/DAXX Complex
In addition to HIRA, the ATRX/DAXX complex is another H3.3 chaperone protein. Alpha-thalassemia X-linked intellectual disability (ATRX) and death domain-associated (DAXX) proteins localize to promyelocytic leukemia nuclear bodies (PMLNBs), which are multipurpose subnuclear domains implicated in transcriptional activation, DNA replication, apoptosis, and viral infection (55–57). The function of the ATRX/DAXX complex as an H3.3-specific deposition complex was identified through the purification of histone variant chaperone complexes (58–60). The ATRX gene was first identified in patients with the ATRX syndrome (61). ATRX encodes a 2,492 amino acid protein with a molecular weight of 282,586 Da (62). The ATRX protein is a chromatin remodeling factor initially described as a putative helicase protein due to sequence homology with the DNA repair and recombination Rad54 protein (63, 64). The ATRX protein contains two highly conserved domains, an ADD (ATRX-DNMT3-DNMT3L) domain in the N-terminal and an ATPase/helicase domain in C-terminal (65–68). The ADD domain can recognize H3K9me3containing nucleosomes in the absence of H3K4 methylation (69), so ATRX itself is an efficient reader of the H3K9me3 histone mark via this domain (66, 70–75). The ATPase/helicase domain belongs to the SWI/SNF2 (SWItch/Sucrose Non Fermentable) family of chromatin remodeling proteins (76). It also contains a plant homeodomain (PHD) zinc finger domain, which is most similar to the DNA methyltransferase 3 family of proteins (77). When ATRX binds to nucleosomes or DNA, the ATPase chromatin remodeling activity of the ATPase/helicase domain can be activated (77, 78).
DAXX was originally identified as a fatty acid synthase (FAS) binding protein that induced apoptosis via Jun N-terminal kinase (JNK) pathway (79) and further work identified it as a chaperone of histone variant H3.3 (58). DAXX preferentially binds to promoter regions and regulates H3.3 loading of immediate early genes after neuronal stimulation (14, 80). DAXX is a transcription repressor that interacts with histone deacetylases (HDAC) and DNA methyltransferases (81). DAXX has four domains: the DAXX helical bundle (DHB); DAXX histone-binding domain (HBD); DAXX acidic domain and SUMO-interacting motifs (SIMs) (82). The DHB domain contains a defined binding surface for a number of DAXX-interacting proteins such as ATRX, Ras-association domain family 1 isoform C (RASSF1C), p53, and mouse double minutes 2 homolog (MDM2) (83). The HBD domain binds the H3.3/H4 dimer for H3.3-specific recognition (84). Crystal structure analysis revealed that DAXX distinguishes H3.3 through direct interaction with the variant-specific residues (87-90) in the core histone fold of H3.3 (6, 60). The DAXX acidic domain appears to increase the binding affinity to the H3.3/H4 dimer. DAXX has two SIMs, located at the N- and C-terminus respectively. The four domains are closely related to the regulation of DAXX transcription.
The Interaction of DAXX and ATRX
The ATRX/DAXX complex is an ATP-dependent chromatin remodeling complex, with ATRX being the core ATPase subunit and DAXX being the targeting subunit (77). DAXX binds to the linker region of ATRX (residues between 1,189 and 1,326) located between the ADD and ATPase domains through its N-terminal DHB domain (77, 85) (Figure 2). ATRX binds H3K9me3 via its ADD domain and heterochromatin protein 1 (HP1) via the PxVxL motif, thereby recruiting DAXX to heterochromatin regions (86). Besides, the binding affinity of DAXX/ATRX is stronger than DAXX and RASSF1C, p53, or MDM2, mainly due to additional electrostatic interactions between positively charged residues in 4HB and negatively charged residues in ATRX (81, 83, 85, 87).
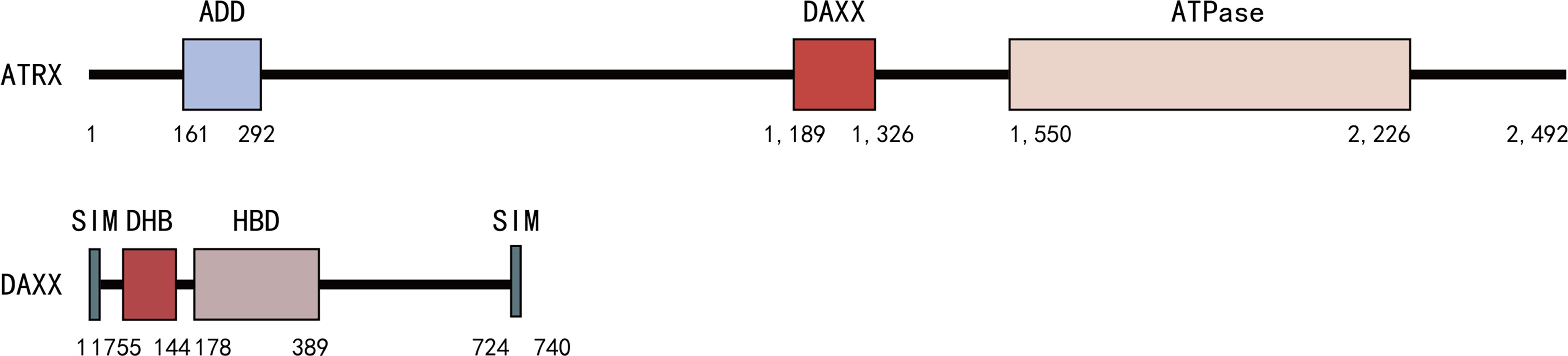
Figure 2 DAXX binds to the linker region of ATRX (residues between 1,189 and 1,326) located between the ADD and ATPase domains through its N-terminal DHB domains.
ATRX and Cancer
There are two known Telomere Maintenance Mechanisms (TMMs): telomerase-mediated telomere maintenance and telomerase-independent telomere maintenance mechanism termed alternative lengthening of telomeres (ALT) (88, 89). The ALT pathway in cancer was first identified in 1997 (90). Unlike the HIRA complex, the ATRX/DAXX complex facilitates H3.3 deposition at heterochromatin, such as telomeres (7, 60). As a histone chaperone, the loss of ATRX/DAXX will impair H3.3 loading at telomeres, leading to ALT and chromosomal instability (CIN) (91–95). Through telomere-specific fluorescence in situ hybridization (FISH), Heaphy et al. revealed that all pancreatic neuroendocrine tumors (PanNETs) samples with ATRX or DAXX gene mutations displayed large, ultrabright telomere FISH signals. This is a universal feature of ALT (92), suggesting that the mutations of ATRX and DAXX are highly related to the ALT. The ALT was associated with DAXX or ATRX mutations in adult adrenocortical tumorigenesis, but exclusively with ATRX mutations in pediatric cases (96, 97). ATRX represses ALT and is required to maintain genomic stability (98). However, Liu et al. demonstrated that there is no significant association between ATRX mutation/loss of expression and ALT in adult diffuse astrocytic tumors (99). In addition, another study showed that in mouse embryonic stem cells, although ATRX loss causes extensive genomic instability, it does not on its own cause ALT or cancer (94, 100). Consistent with this, a study led by Schwartzentruber et al. demonstrated that the simultaneous presence of ATRX/H3F3A/TP53 mutations was highly related to ALT (101). The gene H3F3A, which encodes histone variant H3.3, was recurrently mutated in the pediatric glioblastoma multiforme and led to the critical amino acid mutations at the histone tail (K27M, G34R/V). Of note, mutations in ATRX overlapped significantly with mutations in H3F3A and tumor suppressor TP53 (99, 101, 102), suggesting that there may be collaborative effects among them (76). Intriguingly, H3.3-G34R/V mutations co-occur with ATRX mutations (69, 101, 103), whereas H3.3-K27M mutations did not (69). H3.3K27 often undergo important post-translational modifications like methylation, which is commonly associated with transcriptional repression (101). H3.3 mutations induce chromatin remodeling to produce different gene expression profiles for the K27 and G34 mutations. Whole-exome sequencing showed that genes involved in development and differentiation are distinct among H3.3-K27 and H3.3-G34 mutants (101). ATRX loss-of-function will impair H3.3 loading at telomeres and disrupt the heterochromatic state, facilitating ALT (101).
In recent years, with whole-genome sequencings in cancer, ATRX mutations/losses have been detected in a variety of cancers, such as PanNETs (104–106), Glioblastoma multiforme (GBM) (101), neuroblastoma (107), adrenocortical tumor (96, 97), pediatric osteosarcoma (108), angiosarcomas (109), and Gliomas (99). In PanNETs, there is a high ratio of inactivated to missense mutations in DAXX/ATRX, suggesting that they function as tumor suppressor genes. Intriguingly, patients with DAXX/ATRX mutations often show prolonged survival than patients without those mutations (105). In addition, whole-genome sequencing suggests that ATRX is recurrently mutated in osteosarcoma and is also associated with ALT (108). These loss-of-function mutations ranged from point mutations to frameshift insertions/deletions and were mainly localized within the ADD and C-terminal helicase domain (69, 101, 105, 110). As discussed above, while ATRX loss-of-function is found in various tumors, overexpression of ATRX has been reported in colorectal cancer cell lines (64, 111) (Table 3). For instance, Athwal et al. demonstrated that ATRX is overexpressed in colon cancer SW480 cells (111).
DAXX and Cancer
DAXX mutations more frequently occur in the regions that interact with ATRX and the H3.3/H4 dimer, suggesting that the loss of H3.3 chaperone function of DAXX may lead to abnormal chromatin structures, epigenetic dysregulation, and chromosome instability (82). DAXX mutations are relatively rare compared to H3F3A and ATRX (101). For example, in neuroblastomas, ATRX loss-of-function mutations play a role in ALT which is related to worse prognosis (117). However, DAXX mutations were not detected in neuroblastomas. DAXX gene expression is not significantly changed in ALT-positive neuroblastomas (117). In addition, mutations in ATRX and DAXX were mutually exclusive (92, 101, 105), confirming that they function together in the same pathway. The expression of DAXX is often dysregulated in tumor cells (Table 4). For example, DAXX is overexpressed in many types of cancer such as prostate cancer (118, 120, 130), ovarian cancer (121, 122), gastric cancer (123), and gliomas (14). DAXX is downregulated in advanced gastric cancer (125) and human colon adenocarcinoma cells (126, 127), lung cancer (128), and PanNETs (129). One study suggests that DAXX binds to anaphase promoting complex (APC) coactivators Cdc20 and Cdh1 to inhibit the degradation of APC, thereby promoting chromosome instability during prostate cancer development (118). In addition, Puto et al. demonstrated that in prostate cancer, DAXX binds to DNA methyltransferase (DNMT1) resulting in hypermethylation of the promoter regions of the apoptosis-and autophagy-relevant genes, represses autophagy, and promotes tumorigenicity (119, 120). Overexpression of DAXX promoted ovarian cancer cell proliferation, colony formation, and migration, whereas DAXX depletion by RNA interference had the opposite effects (121). DAXX acts as an oncogene by interacting with PML to protect ovarian cancer cells from DNA damage (121). A subsequent study showed that DAXX promotes ovarian cancer ascites cell proliferation by activating the ERK pathway and directly binding to CCAAT enhancer binding protein-beta (CEBP-β) (122). Benitez et al. proposed a model that DAXX removes H3.3 from the chromatin by competing for chromatin binding to promote oncogene transcription in PTEN-deficient PTEN-null cells (14). In oral squamous cell carcinoma (OSCC) human samples and cell lines, DAXX expression was frequently upregulated. A study showed that DAXX silencing in OSCC cells suppresses cyclin D1 expression via the DAXX-TCF4 (transcription factor 4) interaction, thereby reducing tumor growth (124). In gastric cancer, nuclear/cytoplasmic ratio (NCR) of DAXX expression was found higher in gastric cancer tissues than adjacent normal tissues (123). However, the expression of DAXX was decreased in advanced gastric cancer samples. The upregulation of DAXX in gastric cancer cells inhibited proliferation, migration, invasion, and epithelial-mesenchymal transition (EMT). DAXX overexpression inhibited the growth of gastric cancer through downregulating snail family transcriptional repressor 3 (SNAI3), a key inducer of EMT, by recruiting HDAC-1 into the nucleus (125). Similarly, a study lead by Tzeng et al. demonstrated that DAXX suppresses Tcf4 transcriptional activity and induces G1 arrest of colon cancer cells, functions as tumor suppressor (127), and the knockdown of DAXX caused significant cell proliferation and promote metastasis (126). In PanNETs, DAXX/H3.3 complex suppresses target genes including Stanniocalcin 2 (STC2) by promoting H3K9me3 (129), suggesting that DAXX acts as a tumor suppressor. In another study, DAXX functions as a tumor suppressor by inhibiting the HIF-1a/HDAC1/Slug axis in hypoxia-induced lung cancer cells (128). Furthermore, DAXX mutations usually mark the increase of malignancy (119, 129). Similarly, the depletion of H3.3 leads to loss of DAXX, because the HBD domain does not establish a stable conformation without H3.3/H4 binding, revealing that the physiological level of H3.3 is necessary for maintaining the level of DAXX protein (81). Therefore, increased H3.3 levels in cancer cells may augment the oncogenic function of DAXX through increasing protein stability (82).
Conclusions
Histone chaperones play a critical role in the maintenance of global nucleosomal architecture. Histone chaperone CAF-1 facilitates histone H3.1 deposition in a DNA-synthesis-dependent manner. Mutation of CAF-1 protein reduces the incorporation of H3.1 and H3.2, leading to the increased incorporation of H3.3. Histone variants H3.3 chaperone protein HIRA and ATRX/DAXX mediate DNA-synthesis-independent nucleosome assembly. The chaperone HIRA promotes H3.3 deposition at transient nucleosome-free regions (13). This could be a salvage pathway to maintain chromatin integrity when CAF-1 mediated H3.1 deposition is impaired during DNA replication (6). Although histone variant H3.3 was initially thought to be a marker of transcriptional activation (59), it was later discovered to be deposited into heterochromatic regions via ATRX/DAXX, indicating that H3.3 deposition in repetitive regions may contribute to chromatin stability (64). Mutations of histone chaperones DAXX and ATRX reduce the level of histone variant H3.3 (92) and active the ALT pathway in telomerase-negative cancers, suggesting that the incorporation of H3.3 is necessary for telomere maintenance (80, 131–133). In addition, inactivation mutation of ATRX/DAXX can cause a shift towards HIRA-mediated H3.3 deposition (2). Aside from mutations, imbalances between H3.3 and H3.1 or H3.3/H3.1 and its chaperones may also have detrimental effects on genome stability (134, 135). Nye et al. proposed a “chaperone competition” model, in which changes in chaperone expression cause their target histone variants to bind to non-homologous partners, the location of histone variants, thereby potentially promoting tumorigenesis (136). Histone chaperone competition may lead to the incorrect deposition of canonical histones and histone variants, thus results in activating the expression of oncogenes and promoting the occurrence of cancer.
Taken together, both the up-and down-regulation of expression of chaperone proteins can potentially contribute to the occurrence of tumor. The contradictory conclusions discussed above indicate that carcinogenesis is an extremely complex process involving the interaction of multiple proteins and signaling pathways. In addition, the chaperones play an important role in the malignant transformation of tumors and may serve as targets for cancer prevention and treatment. The mechanisms of histone chaperones in tumorigenesis remain to be fully elucidated. Further study should be done in histone chaperones to explore the molecular mechanisms underlying carcinogenesis and chromatin regulation.
Author Contributions
QC and TW conceived the topic and wrote the manuscript. All authors contributed to the article and approved the submitted version.
Funding
This study is funded by “Young Talent Support Plan” of Xi’an Jiaotong University No. YX6J010.
Conflict of Interest
The authors declare that the research was conducted in the absence of any commercial or financial relationships that could be construed as a potential conflict of interest.
Publisher’s Note
All claims expressed in this article are solely those of the authors and do not necessarily represent those of their affiliated organizations, or those of the publisher, the editors and the reviewers. Any product that may be evaluated in this article, or claim that may be made by its manufacturer, is not guaranteed or endorsed by the publisher.
Glossary
CAF-1: Anti-silencing factor 1A
HIRA: Histone cell cycle regulator
DAXX: Death domain-associated protein
ATRX: Alpha-thalassemia X-linked intellectual disability
DNA: Deoxyribonucleic acid
mRNA: Messenger RNA
poly(A): Polyadenylic acid
CENP-A: Centromere protein-A
H. sapiens: Homo sapiens
WHD: Winged-Helix Domain
PCNA: Proliferating Cell Nuclear Antigen
ASF1: Anti-silencing function 1
UBN1: Ubinuclein-1
CABIN1: Calcineurin-binding protein 1
ssDNA: Single-stranded DNA
RPA: Replication factor A
CDK2: Cyclin-dependent kinase 2
SAHF: Senescence-associated heterochromatin foci
CML: Chronic myeloid leukemia
PMLNBs: Promyelocytic leukemia nuclear bodies
ADD: ATRX-DNMT3-DNMT3L
SWI/SNF2: SWItch/Sucrose Non Fermentable
PHD: Plant homeodomain
FAS: Fatty acid synthase
JNK: Jun N-terminal kinase
HDAC: Histone deacetylases
DHB: DAXX helical bundle
HBD: DAXX histone-binding domain
SIMs: SUMO-interacting motifs
RASSF1C: Ras-association domain family 1 isoform C
MDM2: Mouse double minutes 2 homolog
HP1: Heterochromatin Protein 1
TMMs: Telomere Maintenance Mechanisms
ALT: Alternative lengthening of telomeres
CIN: Chromosomal instability
FISH: Fluorescence in situ hybridization
PanNETs: Pancreatic neuroendocrine tumors
GBM: Glioblastoma multiforme
ERK: Extracellular regulated protein kinases
CEBP-β: CCAAT enhancer binding protein-beta
OSCC: Oral squamous cell carcinoma
TCF4: Transcription factor 4
NCR: Nuclear/Cytoplasmic Ratio
EMT: Epithelial-mesenchymal transition
SNAI3: Snail family transcriptional repressor 3
STC2: Stanniocalcin 2
References
1. Luger K, Mäder AW, Richmond RK, Sargent DF, Richmond TJ. Crystal Structure of the Nucleosome Core Particle at 2.8 A Resolution. Nature (1997) 389(6648):251–60. doi: 10.1038/38444
2. Buschbeck M, Hake SB. Variants of Core Histones and Their Roles in Cell Fate Decisions, Development and Cancer. Nat Rev Mol Cell Biol (2017) 18(5):299–314. doi: 10.1038/nrm.2016.166
3. Akhmanova AS, Bindels PC, Xu J, Miedema K, Kremer H, et al. Structure and Expression of Histone H3.3 Genes in Drosophila Melanogaster and Drosophila Hydei. Genome (1995) 38(3):586–600. doi: 10.1139/g95-075
4. Frank D, Doenecke D, Albig W. Differential Expression of Human Replacement and Cell Cycle Dependent H3 Histone Genes. Gene (2003) 312:135–43. doi: 10.1016/s0378-1119(03)00609-7
5. Krimer DB, Cheng G, Skoultchi AI. Induction of H3.3 Replacement Histone mRNAs During the Precommitment Period of Murine Erythroleukemia Cell Differentiation. Nucleic Acids Res (1993) 21(12):2873–9. doi: 10.1093/nar/21.12.2873
6. Shi L, Wen H, Shi X. The Histone Variant H3.3 in Transcriptional Regulation and Human Disease. J Mol Biol (2017) 429(13):1934–45. doi: 10.1016/j.jmb.2016.11.019
7. Fang HT, El Farran CA, Xing QR, Zhang LF, Li H, Lim, et al. Global H3.3 Dynamic Deposition Defines Its Bimodal Role in Cell Fate Transition. Nat Commun (2018) 9(1):1537. doi: 10.1038/s41467-018-03904-7
8. Tagami H, Ray-Gallet D, Almouzni G, Nakatani Y. Histone H3.1 and H3.3 Complexes Mediate Nucleosome Assembly Pathways Dependent or Independent of DNA Synthesis. Cell (2004) 116(1):51–61. doi: 10.1016/s0092-8674(03)01064-x
9. Delaney K, Mailler J, Wenda JM, Gabus C, Steiner FA. Differential Expression of Histone H3.3 Genes and Their Role in Modulating Temperature Stress Response in Caenorhabditis Elegans. Genetics (2018) 209(2):551–65. doi: 10.1534/genetics.118.300909
10. Burgess RJ, Zhang Z. Histones, Histone Chaperones and Nucleosome Assembly. Protein Cell (2010) 1(7):607–12. doi: 10.1007/s13238-010-0086-y
11. Burgess RJ, Zhang Z. Histone Chaperones in Nucleosome Assembly and Human Disease. Nat Struct Mol Biol (2013) 20(1):14–22. doi: 10.1038/nsmb.2461
12. Shandilya J, Gadad S, Swaminathan V, Kundu TK. Histone Chaperones in Chromatin Dynamics: Implications in Disease Manifestation. Subcell Biochem (2007) 41:111–24. doi: 10.1007/1-4020-5466-1_6
13. Filipescu D, Müller S, Almouzni G. Histone H3 Variants and Their Chaperones During Development and Disease: Contributing to Epigenetic Control. Annu Rev Cell Dev Biol (2014) 30:615–46. doi: 10.1146/annurev-cellbio-100913-013311
14. Benitez JA, Ma J, D'Antonio M, Boyer A, Camargo MF, Zanca C, et al. PTEN Regulates Glioblastoma Oncogenesis Through Chromatin-Associated Complexes of DAXX and Histone H3.3. Nat Commun (2017) 8:15223. doi: 10.1038/ncomms15223
15. Ivanauskiene K, Delbarre E, McGhie JD, Küntziger T, Wong LH, et al. The PML-Associated Protein DEK Regulates the Balance of H3.3 Loading on Chromatin and Is Important for Telomere Integrity. Genome Res (2014) 24(10):1584–94. doi: 10.1101/gr.173831.114
16. Sawatsubashi S, Murata T, Lim J, Fujiki R, Ito S, Suzuki E, et al. A Histone Chaperone, DEK, Transcriptionally Coactivates a Nuclear Receptor. Genes Dev (2010) 24(2):159–70. doi: 10.1101/gad.1857410
17. Smith S, Stillman B. Purification and Characterization of CAF-I, a Human Cell Factor Required for Chromatin Assembly During DNA Replication In Vitro. Cell (1989) 58(1):15–25. doi: 10.1016/0092-8674(89)90398-x
18. Volk A, Crispino JD. The Role of the Chromatin Assembly Complex (CAF-1) and Its P60 Subunit (CHAF1b) in Homeostasis and Disease. Biochim Biophys Acta (2015) 8(1849):979–86. doi: 10.1016/j.bbagrm.2015.05.009
19. de Tayrac M, Aubry M, Saïkali S, Etcheverry A, Surbled C, Guénot F, et al. A 4-Gene Signature Associated With Clinical Outcome in High-Grade Gliomas. Clin Cancer Res (2011) 17(2):317–27. doi: 10.1158/1078-0432.ccr-10-1126
20. de Tayrac M, Saikali S, Aubry M, Bellaud P, Boniface R, Quillien, et al. Prognostic Significance of EDN/RB, HJURP, P60/CAF-1 and PDLI4, Four New Markers in High-Grade Gliomas. PloS One (2013) 8(9):e73332. doi: 10.1371/journal.pone.0073332
21. Mascolo M, Vecchione ML, Ilardi G, Scalvenzi M, Molea G, Di Benedetto M, et al. Overexpression of Chromatin Assembly Factor-1/P60 Helps to Predict the Prognosis of Melanoma Patients. BMC Cancer (2010) 10:63. doi: 10.1186/1471-2407-10-63
22. Peng X, Fu H, Yin J, Zhao Q. CHAF1B Knockdown Blocks Migration in a Hepatocellular Carcinoma Model. Oncol Rep (2018) 40(1):405–13. doi: 10.3892/or.2018.6437
23. Polo SE, Theocharis SE, Grandin L, Gambotti L, Antoni G, Savignoni A, et al. Clinical Significance and Prognostic Value of Chromatin Assembly Factor-1 Overexpression in Human Solid Tumours. Histopathology (2010) 57(5):716–24. doi: 10.1111/j.1365-2559.2010.03681.x
24. Polo SE, Theocharis SE, Klijanienko J, Savignoni A, Asselain B, Vielh P, et al. Chromatin Assembly Factor-1, a Marker of Clinical Value to Distinguish Quiescent From Proliferating Cells. Cancer Res (2004) 64(7):2371–81. doi: 10.1158/0008-5472.can-03-2893
25. Staibano S, Mascolo M, Mancini FP, Kisslinger A, Salvatore G, Di Benedetto M, et al. Overexpression of Chromatin Assembly Factor-1 (CAF-1) P60 Is Predictive of Adverse Behaviour of Prostatic Cancer. Histopathology (2009) 54(5):580–9. doi: 10.1111/j.1365-2559.2009.03266.x
26. Staibano S, Mignogna C, Lo Muzio L, Mascolo M, Salvatore G, Di Benedetto M, et al. Chromatin Assembly Factor-1 (CAF-1)-Mediated Regulation of Cell Proliferation and DNA Repair: A Link With the Biological Behaviour of Squamous Cell Carcinoma of the Tongue? Histopathology (2007) 50(7):911–9. doi: 10.1111/j.1365-2559.2007.02698.x
27. Staibano S, Mascolo M, Rocco A, Lo Muzio L, Ilardi G, Siano M, et al. The Proliferation Marker Chromatin Assembly Factor-1 Is of Clinical Value in Predicting the Biological Behaviour of Salivary Gland Tumours. Oncol Rep (2011) 25(1):13–22.
28. Volk A, Liang K, Suraneni P, Li X, Zhao J, Bulic M, et al. A CHAF1B-Dependent Molecular Switch in Hematopoiesis and Leukemia Pathogenesis. Cancer Cell (2018) 34(5):707–723.e707. doi: 10.1016/j.ccell.2018.10.004
29. Yang S, Long Q, Chen M, Liu X, Zhou H. CAF-1/P150 Promotes Cell Proliferation, Migration, Invasion and Predicts a Poor Prognosis in Patients With Cervical Cancer. Oncol Lett (2020) 20(3):2338–46. doi: 10.3892/ol.2020.11775
30. Gomes AP, Ilter D, Low V, Rosenzweig A, Shen ZJ, Schild T, et al. Dynamic Incorporation of Histone H3 Variants Into Chromatin Is Essential for Acquisition of Aggressive Traits and Metastatic Colonization. Cancer Cell (2019) 36(4):402–417.e413. doi: 10.1016/j.ccell.2019.08.006
31. Simeonova I, Almouzni G. Dynamic Histone H3 Incorporation Fuels Metastatic Progression. Trends Mol Med (2019) 25(11):933–5. doi: 10.1016/j.molmed.2019.09.003
32. Gal C, Moore KM, Paszkiewicz K, Kent NA, Whitehall SK. The Impact of the HIRA Histone Chaperone Upon Global Nucleosome Architecture. Cell Cycle (2015) 14(1):123–34. doi: 10.4161/15384101.2014.967123
33. Nie X, Wang H, Li J, Holec S, Berger F. The HIRA Complex That Deposits the Histone H3.3 Is Conserved in Arabidopsis and Facilitates Transcriptional Dynamics. Biol Open (2014) 3(9):794–802. doi: 10.1242/bio.20148680
34. Lamour V, Lécluse Y, Desmaze C, Spector M, Bodescot M, Aurias A, et al. A Human Homolog of the S. Cerevisiae HIR1 and HIR2 Transcriptional Repressors Cloned From the DiGeorge Syndrome Critical Region. Hum Mol Genet (1995) 4(5):791–9. doi: 10.1093/hmg/4.5.791
35. Lorain S, Quivy JP, Monier-Gavelle F, Scamps C, Lécluse Y, Almouzni, et al. Core Histones and HIRIP3, a Novel Histone-Binding Protein, Directly Interact With WD Repeat Protein HIRA. Mol Cell Biol (1998) 18(9):5546–56. doi: 10.1128/mcb.18.9.5546
36. Rai TS, Puri A, McBryan T, Hoffman J, Tang Y, Pchelintsev NA, et al. Human CABIN1 Is a Functional Member of the Human HIRA/UBN1/ASF1a Histone H3.3 Chaperone Complex. Mol Cell Biol (2011) 31(19):4107–18. doi: 10.1128/mcb.05546-11
37. Ricketts MD, Marmorstein R. A Molecular Prospective for HIRA Complex Assembly and H3.3-Specific Histone Chaperone Function. J Mol Biol (2017) 429(13):1924–33. doi: 10.1016/j.jmb.2016.11.010
38. Ricketts MD, Frederick B, Hoff H, Tang Y, Schultz DC, Singh Rai T, et al. Ubinuclein-1 Confers Histone H3.3-Specific-Binding by the HIRA Histone Chaperone Complex. Nat Commun (2015) 6:7711. doi: 10.1038/ncomms8711
39. Xiong C, Wen Z, Yu J, Chen J, Liu CP, Zhang X, et al. UBN1/2 of HIRA Complex Is Responsible for Recognition and Deposition of H3.3 at Cis-Regulatory Elements of Genes in Mouse ES Cells. BMC Biol (2018) 16(1):110. doi: 10.1186/s12915-018-0573-9
40. Torné J, Ray-Gallet D, Boyarchuk E, Garnier M, Le Baccon P, Coulon A, et al. Two HIRA-Dependent Pathways Mediate H3.3 De Novo Deposition and Recycling During Transcription. Nat Struct Mol Biol (2020) 27(11):1057–68. doi: 10.1038/s41594-020-0492-7
41. Pchelintsev NA, McBryan T, Rai TS, van Tuyn J, Ray-Gallet D, Almouzni, et al. Placing the HIRA Histone Chaperone Complex in the Chromatin Landscape. Cell Rep (2013) 3(4):1012–9. doi: 10.1016/j.celrep.2013.03.026
42. Zhang H, Gan H, Wang Z, Lee JH, Zhou H, Ordog T, et al. RPA Interacts With HIRA and Regulates H3.3 Deposition at Gene Regulatory Elements in Mammalian Cells. Mol Cell (2017) 65(2):272–84. doi: 10.1016/j.molcel.2016.11.030
43. Adam S, Polo SE, Almouzni G. Transcription Recovery After DNA Damage Requires Chromatin Priming by the H3.3 Histone Chaperone HIRA. Cell (2013) 155(1):94–106. doi: 10.1016/j.cell.2013.08.029
44. Anderson HE, Wardle J, Korkut SV, Murton HE, López-Maury L, Bähler J, et al. The Fission Yeast HIRA Histone Chaperone Is Required for Promoter Silencing and the Suppression of Cryptic Antisense Transcripts. Mol Cell Biol (2009) 29(18):5158–67. doi: 10.1128/mcb.00698-09
45. Ray-Gallet D, Woolfe A, Vassias I, Pellentz C, Lacoste N, Puri A, et al. Dynamics of Histone H3 Deposition In Vivo Reveal a Nucleosome Gap-Filling Mechanism for H3.3 to Maintain Chromatin Integrity. Mol Cell (2011) 44(6):928–41. doi: 10.1016/j.molcel.2011.12.006
46. Lin CJ, Koh FM, Wong P, Conti M, Ramalho-Santos M. Hira-Mediated H3.3 Incorporation Is Required for DNA Replication and Ribosomal RNA Transcription in the Mouse Zygote. Dev Cell (2014) 30(3):268–79. doi: 10.1016/j.devcel.2014.06.022
47. Roberts C, Sutherland HF, Farmer H, Kimber W, Halford S, Carey A, et al. Targeted Mutagenesis of the Hira Gene Results in Gastrulation Defects and Patterning Abnormalities of Mesoendodermal Derivatives Prior to Early Embryonic Lethality. Mol Cell Biol (2002) 22(7):2318–28. doi: 10.1128/mcb.22.7.2318-2328.2002
48. Dutta D, Ray S, Home P, Saha B, Wang S, Sheibani N, et al. Regulation of Angiogenesis by Histone Chaperone HIRA-Mediated Incorporation of Lysine 56-Acetylated Histone H3.3 at Chromatin Domains of Endothelial Genes. J Biol Chem (2010) 285(53):41567–77. doi: 10.1074/jbc.M110.190025
49. Majumder A, Syed KM, Joseph S, Scambler PJ, Dutta D. Histone Chaperone HIRA in Regulation of Transcription Factor Runx1. J Biol Chem (2015) 290(21):13053–63. doi: 10.1074/jbc.M114.615492
50. Banumathy G, Somaiah N, Zhang R, Tang Y, Hoffmann J, Andrake M, et al. Human UBN1 Is an Ortholog of Yeast Hpc2p and Has an Essential Role in the HIRA/ASF1a Chromatin-Remodeling Pathway in Senescent Cells. Mol Cell Biol (2009) 29(3):758–70. doi: 10.1128/mcb.01047-08
51. Ye X, Zerlanko B, Zhang R, Somaiah N, Lipinski M, Salomoni P, et al. Definition of pRB- and P53-Dependent and -Independent Steps in HIRA/ASF1a-Mediated Formation of Senescence-Associated Heterochromatin Foci. Mol Cell Biol (2007) 27(7):2452–65. doi: 10.1128/mcb.01592-06
52. Li Y, Jiao J. Histone Chaperone HIRA Regulates Neural Progenitor Cell Proliferation and Neurogenesis via β-Catenin. J Cell Biol (2017) 216(7):1975–92. doi: 10.1083/jcb.201610014
53. Majumder A, Dharan AT, Baral I, Varghese PC, Mukherjee A, Subhadradevi L, et al. Histone Chaperone HIRA Dictate Proliferation vs Differentiation of Chronic Myeloid Leukemia Cells. FASEB Bioadv (2019) 1(9):525–37. doi: 10.1096/fba.2019-00014
54. Hall C, Nelson DM, Ye X, Baker K, DeCaprio JA, Seeholzer S, et al. HIRA, the Human Homologue of Yeast Hir1p and Hir2p, Is a Novel Cyclin-Cdk2 Substrate Whose Expression Blocks S-Phase Progression. Mol Cell Biol (2001) 21(5):1854–65. doi: 10.1128/mcb.21.5.1854-1865.2001
55. Bernardi R, Pandolfi PP. Structure, Dynamics and Functions of Promyelocytic Leukaemia Nuclear Bodies. Nat Rev Mol Cell Biol (2007) 8(12):1006–16. doi: 10.1038/nrm2277
56. Ishov AM, Sotnikov AG, Negorev D, Vladimirova OV, Neff N, Kamitani T, et al. PML Is Critical for ND10 Formation and Recruits the PML-Interacting Protein Daxx to This Nuclear Structure When Modified by SUMO-1. J Cell Biol (1999) 147(2):221–34. doi: 10.1083/jcb.147.2.221
57. Ishov AM, Vladimirova OV, Maul GG. Heterochromatin and ND10 Are Cell-Cycle Regulated and Phosphorylation-Dependent Alternate Nuclear Sites of the Transcription Repressor Daxx and SWI/SNF Protein ATRX. J Cell Sci (2004) 117 (:Pt17):3807–20. doi: 10.1242/jcs.01230
58. Drané P, Ouararhni K, Depaux A, M.Hamiche A. The Death-Associated Protein DAXX Is a Novel Histone Chaperone Involved in the Replication-Independent Deposition of H3.3. Genes Dev (2010) 24(12):1253–65. doi: 10.1101/gad.566910
59. Goldberg AD, Banaszynski LA, Noh KM, Lewis PW, Elsaesser SJ, Stadler S, et al. Distinct Factors Control Histone Variant H3.3 Localization at Specific Genomic Regions. Cell (2010) 140(5):678–91. doi: 10.1016/j.cell.2010.01.003
60. Lewis PW, Elsaesser SJ, Noh KM, Stadler SC, Allis CD. Daxx is an H3.3-Specific Histone Chaperone and Cooperates With ATRX in Replication-Independent Chromatin Assembly at Telomeres. Proc Natl Acad Sci USA (2010) 107(32):14075–80. doi: 10.1073/pnas.1008850107
61. Gibbons RJ, Picketts DJ, Villard L, Higgs DR. Mutations in a Putative Global Transcriptional Regulator Cause X-Linked Mental Retardation With Alpha-Thalassemia (ATR-X Syndrome). Cell (1995) 80(6):837–45. doi: 10.1016/0092-8674(95)90287-2
62. Garrick D, Samara V, McDowell TL, Smith AJ, Dobbie L, Higgs, et al. A Conserved Truncated Isoform of the ATR-X Syndrome Protein Lacking the SWI/SNF-Homology Domain. Gene (2004) 326:23–34. doi: 10.1016/j.gene.2003.10.026
63. Stayton CL, Dabovic B, Gulisano M, Gecz J, Broccoli V, Giovanazzi S, et al. Cloning and Characterization of a New Human Xq13 Gene, Encoding a Putative Helicase. Hum Mol Genet (1994) 3(11):1957–64. doi: 10.1093/hmg/3.11.1957
64. Watson LA, Goldberg H, Bérubé NG. Emerging Roles of ATRX in Cancer. Epigenomics (2015) 7(8):1365–78. doi: 10.2217/epi.15.82
65. Aapola U, Kawasaki K, Scott HS, Ollila J, Vihinen M, Heino M, et al. Isolation and Initial Characterization of a Novel Zinc Finger Gene, DNMT3L, on 21q22.3, Related to the Cytosine-5-Methyltransferase 3 Gene Family. Genomics (2000) 65(3):293–8. doi: 10.1006/geno.2000.6168
66. Argentaro A, Yang JC, Chapman L, Kowalczyk MS, Gibbons RJ, Higgs DR, et al. Structural Consequences of Disease-Causing Mutations in the ATRX-DNMT3-DNMT3L (ADD) Domain of the Chromatin-Associated Protein ATRX. Proc Natl Acad Sci USA (2007) 104(29):11939–44. doi: 10.1073/pnas.0704057104
67. Picketts DJ, Tastan AO, Higgs DR, Gibbons RJ. Comparison of the Human and Murine ATRX Gene Identifies Highly Conserved, Functionally Important Domains. Mamm Genome (1998) 9(5):400–3. doi: 10.1007/s003359900781
68. Xie S, Wang Z, Okano M, Nogami M, Li Y, He WW, et al. Cloning, Expression and Chromosome Locations of the Human DNMT3 Gene Family. Gene (1999) 236(1):87–95. doi: 10.1016/s0378-1119(99)00252-8
69. Dyer MA, Qadeer ZA, Valle-Garcia D, Bernstein E. ATRX and DAXX: Mechanisms and Mutations. Cold Spring Harb Perspect Med (2017) 7:3. doi: 10.1101/cshperspect.a026567
70. Clynes D, Higgs DR, Gibbons RJ. The Chromatin Remodeller ATRX: A Repeat Offender in Human Disease. Trends Biochem Sci (2013) 38(9):461–6. doi: 10.1016/j.tibs.2013.06.011
71. Dhayalan A, Tamas R, Bock I, Tattermusch A, Dimitrova E, Kudithipudi S, et al. The ATRX-ADD Domain Binds to H3 Tail Peptides and Reads the Combined Methylation State of K4 and K9. Hum Mol Genet (2011) 20(11):2195–203. doi: 10.1093/hmg/ddr107
72. Eustermann S, Yang JC, Law MJ, Amos R, Chapman LM, Jelinska C, et al. Combinatorial Readout of Histone H3 Modifications Specifies Localization of ATRX to Heterochromatin. Nat Struct Mol Biol (2011) 18(7):777–82. doi: 10.1038/nsmb.2070
73. Iwase S, Xiang B, Ghosh S, Ren T, Lewis PW, Cochrane JC, et al. ATRX ADD Domain Links an Atypical Histone Methylation Recognition Mechanism to Human Mental-Retardation Syndrome. Nat Struct Mol Biol (2011) 18(7):769–76. doi: 10.1038/nsmb.2062
74. Mitson M, Kelley LA, Sternberg MJ, Higgs DR, Gibbons RJ. Functional Significance of Mutations in the Snf2 Domain of ATRX. Hum Mol Genet (2011) 20(13):2603–10. doi: 10.1093/hmg/ddr163
75. Sarma K, Cifuentes-Rojas C, Ergun A, Del Rosario A, Jeon Y, White F, et al. ATRX Directs Binding of PRC2 to Xist RNA and Polycomb Targets. Cell (2014) 159(4):869–83. doi: 10.1016/j.cell.2014.10.019
76. Haase S, Garcia-Fabiani MB, Carney S, Altshuler D, Núñez FJ, Méndez FM, et al. Mutant ATRX: Uncovering a New Therapeutic Target for Glioma. Expert Opin Ther Targets (2018) 22(7):599–613. doi: 10.1080/14728222.2018.1487953
77. Tang J, Wu S, Liu H, Stratt R, Barak OG, Shiekhattar R, et al. A Novel Transcription Regulatory Complex Containing Death Domain-Associated Protein and the ATR-X Syndrome Protein. J Biol Chem (2004) 279(19):20369–77. doi: 10.1074/jbc.M401321200
78. Xue Y, Gibbons R, Yan Z, Yang D, McDowell TL, Sechi S, et al. The ATRX Syndrome Protein Forms a Chromatin-Remodeling Complex With Daxx and Localizes in Promyelocytic Leukemia Nuclear Bodies. Proc Natl Acad Sci USA (2003) 100(19):10635–40. doi: 10.1073/pnas.1937626100
79. Yang X, Khosravi-Far R, Chang HY, Baltimore D. Daxx, a Novel Fas-Binding Protein That Activates JNK and Apoptosis. Cell (1997) 89(7):1067–76. doi: 10.1016/s0092-8674(00)80294-9
80. Michod D, Bartesaghi S, Khelifi A, Bellodi C, Berliocchi L, Nicotera P, et al. Calcium-Dependent Dephosphorylation of the Histone Chaperone DAXX Regulates H3.3 Loading and Transcription Upon Neuronal Activation. Neuron (2012) 74(1):122–35. doi: 10.1016/j.neuron.2012.02.021
81. Hoelper D, Huang H, Jain AY, Patel DJ, Lewis PW. Structural and Mechanistic Insights Into ATRX-Dependent and -Independent Functions of the Histone Chaperone DAXX. Nat Commun (2017) 8(1):1193. doi: 10.1038/s41467-017-01206-y
82. Mahmud I, Liao D. DAXX in Cancer: Phenomena, Processes, Mechanisms and Regulation. Nucleic Acids Res (2019) 47(15):7734–52. doi: 10.1093/nar/gkz634
83. Escobar-Cabrera E, Lau DK, Giovinazzi S, Ishov AM, McIntosh LP. Structural Characterization of the DAXX N-Terminal Helical Bundle Domain and Its Complex With Rassf1C. Structure (2010) 18(12):1642–53. doi: 10.1016/j.str.2010.09.016
84. Elsässer SJ, Huang H, Lewis PW, Chin JW, C.D.Patel DJ. DAXX Envelops a Histone H3.3-H4 Dimer for H3.3-Specific Recognition. Nature (2012) 491(7425):560–5. doi: 10.1038/nature11608
85. Wang X, Zhao Y, Zhang J, Chen Y. Structural Basis for DAXX Interaction With ATRX. Protein Cell (2017) 8(10):767–71. doi: 10.1007/s13238-017-0462-y
86. Lechner MS, Schultz DC, Negorev D, Maul GG, Rauscher FJ 3rd. The Mammalian Heterochromatin Protein 1 Binds Diverse Nuclear Proteins Through a Common Motif That Targets the Chromoshadow Domain. Biochem Biophys Res Commun (2005) 331(4):929–37. doi: 10.1016/j.bbrc.2005.04.016
87. Li Z, Zhao D, Xiang B, Li H. Structural and Biochemical Characterization of DAXX-ATRX Interaction. Protein Cell (2017) . 8(10):762–6. doi: 10.1007/s13238-017-0463-x
88. De Vitis M, Berardinelli F, Sgura A. Telomere Length Maintenance in Cancer: At the Crossroad Between Telomerase and Alternative Lengthening of Telomeres (ALT). Int J Mol Sci (2018) 19:2. doi: 10.3390/ijms19020606
89. Heaphy CM, Subhawong AP, Hong SM, Goggins MG, Montgomery EA, Gabrielson E, et al. Prevalence of the Alternative Lengthening of Telomeres Telomere Maintenance Mechanism in Human Cancer Subtypes. Am J Pathol (2011) 179(4):1608–15. doi: 10.1016/j.ajpath.2011.06.018
90. Bryan TM, Englezou A, Dalla-Pozza L, Dunham MA, Reddel RR. Evidence for an Alternative Mechanism for Maintaining Telomere Length in Human Tumors and Tumor-Derived Cell Lines. Nat Med (1997) 3(11):1271–4. doi: 10.1038/nm1197-1271
91. Amorim JP, Santos G, Vinagre J, Soares P. The Role of ATRX in the Alternative Lengthening of Telomeres (ALT) Phenotype. Genes (Basel) (2016) 7:9. doi: 10.3390/genes7090066
92. Heaphy CM, de Wilde RF, Jiao Y, Klein AP, Edil BH, Shi C, et al. Altered Telomeres in Tumors With ATRX and DAXX Mutations. Science (2011) 333(6041):425. doi: 10.1126/science.1207313
93. Koschmann C, Lowenstein PR, Castro MG. ATRX Mutations and Glioblastoma: Impaired DNA Damage Repair, Alternative Lengthening of Telomeres, and Genetic Instability. Mol Cell Oncol (2016) 3(3):e1167158. doi: 10.1080/23723556.2016.1167158
94. Lovejoy CA, Li W, Reisenweber S, Thongthip S, Bruno J, de Lange T, et al. Loss of ATRX, Genome Instability, and an Altered DNA Damage Response are Hallmarks of the Alternative Lengthening of Telomeres Pathway. PloS Genet (2012) 8(7):e1002772. doi: 10.1371/journal.pgen.1002772
95. Ramamoorthy M, Smith S. Loss of ATRX Suppresses Resolution of Telomere Cohesion to Control Recombination in ALT Cancer Cells. Cancer Cell (2015) 28(3):357–69. doi: 10.1016/j.ccell.2015.08.003
96. Assié G, Letouzé E, Fassnacht M, Jouinot A, Luscap W, Barreau O, et al. Integrated Genomic Characterization of Adrenocortical Carcinoma. Nat Genet (2014) 46(6):607–12. doi: 10.1038/ng.2953
97. Pinto EM, Chen X, Easton J, Finkelstein D, Liu Z, Pounds S, et al. Genomic Landscape of Paediatric Adrenocortical Tumours. Nat Commun (2015) 6:6302. doi: 10.1038/ncomms7302
98. Napier CE, Huschtscha LI, Harvey A, Bower K, Noble JR, Hendrickson EA, et al. ATRX Represses Alternative Lengthening of Telomeres. Oncotarget (2015) 6(18):16543–58. doi: 10.18632/oncotarget.3846
99. Liu XY, Gerges N, Korshunov A, Sabha N, Khuong-Quang DA, Fontebasso AM, et al. Frequent ATRX Mutations and Loss of Expression in Adult Diffuse Astrocytic Tumors Carrying IDH1/IDH2 and TP53 Mutations. Acta Neuropathol (2012) 124(5):615–25. doi: 10.1007/s00401-012-1031-3
100. Clynes D, Jelinska C, Xella B, Ayyub H, Taylor S, Mitson M, et al. ATRX Dysfunction Induces Replication Defects in Primary Mouse Cells. PloS One (2014) 9(3):e92915. doi: 10.1371/journal.pone.0092915
101. Schwartzentruber J, Korshunov A, Liu XY, Jones DT, Pfaff E, Jacob K, et al. Driver Mutations in Histone H3.3 and Chromatin Remodelling Genes in Paediatric Glioblastoma. Nature (2012) 482(7384):226–31. doi: 10.1038/nature10833
102. Elsässer SJ, Allis CD, Lewis PW. Cancer. New Epigenetic Drivers of Cancers. Science (2011) 331(6021):1145–6. doi: 10.1126/science.1203280
103. Wu G, Diaz AK, Paugh BS, Rankin SL, Ju B, Li Y, et al. The Genomic Landscape of Diffuse Intrinsic Pontine Glioma and Pediatric Non-Brainstem High-Grade Glioma. Nat Genet (2014) 46(5):444–50. doi: 10.1038/ng.2938
104. Chen SF, Kasajima A, Yazdani S, Chan MS, Wang L, He YY, et al. Clinicopathologic Significance of Immunostaining of α-Thalassemia/Mental Retardation Syndrome X-Linked Protein and Death Domain-Associated Protein in Neuroendocrine Tumors. Hum Pathol (2013) 44(10):2199–203. doi: 10.1016/j.humpath.2013.04.025
105. Jiao Y, Shi C, Edil BH, de Wilde RF, Klimstra DS, Maitra A, et al. DAXX/ATRX, MEN1, and mTOR Pathway Genes Are Frequently Altered in Pancreatic Neuroendocrine Tumors. Science (2011) 331(6021):1199–203. doi: 10.1126/science.1200609
106. Marinoni I, Kurrer AS, Vassella E, Dettmer M, Rudolph T, Banz V, et al. Loss of DAXX and ATRX Are Associated With Chromosome Instability and Reduced Survival of Patients With Pancreatic Neuroendocrine Tumors. Gastroenterology (2014) 146(2):453–460.e455. doi: 10.1053/j.gastro.2013.10.020
107. Cheung NK, Zhang J, Lu C, Parker M, Bahrami A, Tickoo SK, et al. Association of Age at Diagnosis and Genetic Mutations in Patients With Neuroblastoma. JAMA (2012) . 307(10):1062–71. doi: 10.1001/jama.2012.228
108. Chen X, Bahrami A, Pappo A, Easton J, Dalton J, Hedlund E, et al. Recurrent Somatic Structural Variations Contribute to Tumorigenesis in Pediatric Osteosarcoma. Cell Rep (2014) 7(1):104–12. doi: 10.1016/j.celrep.2014.03.003
109. Panse G, Chrisinger JS, Leung CH, Ingram DR, Khan S, Wani K, et al. Clinicopathological Analysis of ATRX, DAXX and NOTCH Receptor Expression in Angiosarcomas. Histopathology (2018) 72(2):239–47. doi: 10.1111/his.13337
110. Qadeer ZA, Harcharik S, Valle-Garcia D, Chen C, Birge MB, Vardabasso C, et al. Decreased Expression of the Chromatin Remodeler ATRX Associates With Melanoma Progression. J Invest Dermatol (2014) 134(6):1768–72. doi: 10.1038/jid.2014.45
111. Athwal RK, Walkiewicz MP, Baek S, Fu S, Bui M, Camps J, et al. CENP-A Nucleosomes Localize to Transcription Factor Hotspots and Subtelomeric Sites in Human Cancer Cells. Epigenet Chromatin (2015) 8:2. doi: 10.1186/1756-8935-8-2
112. Ahvenainen TV, Mäkinen NM, von Nandelstadh P, Vahteristo MEA, Pasanen AM, Bützow RC, et al. Loss of ATRX/DAXX Expression and Alternative Lengthening of Telomeres in Uterine Leiomyomas. Cancer (2018) 124(24):4650–6. doi: 10.1002/cncr.31754
113. Graham MK, Kim J, Da J, Brosnan-Cashman JA, Rizzo A, Baena Del Valle JA, et al. Functional Loss of ATRX and TERC Activates Alternative Lengthening of Telomeres (ALT) in LAPC4 Prostate Cancer Cells. Mol Cancer Res (2019) 17(12):2480–91. doi: 10.1158/1541-7786.mcr-19-0654
114. Liau JY, Tsai JH, Yang CY, Lee JC, Liang CW, H.H.Jeng YM. Alternative Lengthening of Telomeres Phenotype in Malignant Vascular Tumors is Highly Associated With Loss of ATRX Expression and Is Frequently Observed in Hepatic Angiosarcomas. Hum Pathol (2015) 46(9):1360–6. doi: 10.1016/j.humpath.2015.05.019
115. Akaike K, Toda-Ishii M, Suehara Y, Mukaihara K, Kubota D, Mitani K, et al. TERT Promoter Mutations Are a Rare Event in Gastrointestinal Stromal Tumors. Springerplus (2015) 4:836. doi: 10.1186/s40064-015-1606-2
116. Kim HS, Lee HS, Nam KH, Choi J, Kim WH. Telomere Length Abnormalities and Telomerase RNA Component Expression in Gastroenteropancreatic Neuroendocrine Tumors. Anticancer Res (2015) 35(6):3501–10.
117. Ackermann S, Cartolano M, Hero B, Welte A, Kahlert Y, Roderwieser A, et al. A Mechanistic Classification of Clinical Phenotypes in Neuroblastoma. Science (2018) 362(6419):1165–70. doi: 10.1126/science.aat6768
118. Kwan PS, Lau CC, Chiu YT, Man C, Liu J, Tang KD, et al. Daxx Regulates Mitotic Progression and Prostate Cancer Predisposition. Carcinogenesis (2013) 34(4):750–9. doi: 10.1093/carcin/bgs391
119. Puto LA, Brognard J, Hunter T. Transcriptional Repressor DAXX Promotes Prostate Cancer Tumorigenicity via Suppression of Autophagy. J Biol Chem (2015) 290(25):15406–20. doi: 10.1074/jbc.M115.658765
120. Puto LA, Benner C, Hunter T. The DAXX Co-Repressor is Directly Recruited to Active Regulatory Elements Genome-Wide to Regulate Autophagy Programs in a Model of Human Prostate Cancer. Oncoscience (2015) 2(4):362–72. doi: 10.18632/oncoscience.152
121. Pan WW, Zhou JJ, Liu XM, Xu Y, Guo LJ, Yu C, et al. Death Domain-Associated Protein DAXX Promotes Ovarian Cancer Development and Chemoresistance. J Biol Chem (2013) 288(19):13620–30. doi: 10.1074/jbc.M112.446369
122. Liu SB, Lin XP, Xu Y, Shen ZF, Pan WW. DAXX Promotes Ovarian Cancer Ascites Cell Proliferation and Migration by Activating the ERK Signaling Pathway. J Ovarian Res (2018) 11(1):90. doi: 10.1186/s13048-018-0462-4
123. Xu JF, Zhao ZG, Ye LL, Zhuge W, Han Z, Zhang TM, et al. Prognostic Significance of Daxx NCR (Nuclear/Cytoplasmic Ratio) in Gastric Cancer. Cancer Med (2017) 6(9):2063–75. doi: 10.1002/cam4.1144
124. Lin GJ, Huang YS, Lin CK, Huang SH, Shih HM, Sytwu HK, et al. Daxx and TCF4 Interaction Links to Oral Squamous Cell Carcinoma Growth by Promoting Cell Cycle Progression via Induction of Cyclin D1 Expression. Clin Oral Investig (2016) 20(3):533–40. doi: 10.1007/s00784-015-1536-y
125. Wu C, Ding H, Wang S, Li Y, Liu SB, Wang X, et al. DAXX Inhibits Cancer Stemness and Epithelial-Mesenchymal Transition in Gastric Cancer. Br J Cancer (2020) . 122(10):1477–85. doi: 10.1038/s41416-020-0800-3
126. Chen YC, Lee TH, Tzeng SL. Reduced DAXX Expression Is Associated With Reduced CD24 Expression in Colorectal Cancer. Cells (2019) 8:10. doi: 10.3390/cells8101242
127. Tzeng SL, Cheng YW, Li CH, Lin YS, Hsu HC, Kang JJ. Physiological and Functional Interactions Between Tcf4 and Daxx in Colon Cancer Cells. J Biol Chem (2006) 281(22):15405–11. doi: 10.1074/jbc.M601807200
128. Lin CW, Wang LK, Wang SP, Chang YL, Wu YY, Chen HY, et al. Daxx Inhibits Hypoxia-Induced Lung Cancer Cell Metastasis by Suppressing the HIF-1α/HDAC1/Slug Axis. Nat Commun (2016) 7:13867. doi: 10.1038/ncomms13867
129. Ueda H, Akiyama Y, Shimada S, Mogushi K, Serizawa M, Matsumura S, et al. Tumor Suppressor Functions of DAXX Through Histone H3.3/H3K9me3 Pathway in Pancreatic NETs. Endocr Relat Cancer (2018) 25(6):619–31. doi: 10.1530/erc-17-0328
130. Jamali L, Moradi A, Ganji M, Ayati M, Kazeminezhad B, Fazeli Attar Z, et al. Potential Prognostic Role for SPOP, DAXX, RARRES1, and LAMP2 as an Autophagy Related Genes in Prostate Cancer. Urol J (2020) 17(2):156–63. doi: 10.22037/uj.v0i0.4935
131. Morozov VM, Giovinazzi S, Ishov AM. CENP-B Protects Centromere Chromatin Integrity by Facilitating Histone Deposition via the H3.3-Specific Chaperone Daxx. Epigenet Chromatin (2017) 10(1):63. doi: 10.1186/s13072-017-0164-y
132. Tang SY, Wan YP, Wu YM. Death Domain Associated Protein (Daxx), a Multi-Functional Protein. Cell Mol Biol Lett (2015) 20(5):788–97. doi: 10.1515/cmble-2015-0048
133. Wasylishen AR, Estrella JS, Pant V, Chau GP, Lozano G. Daxx Functions Are P53-Independent In Vivo. Mol. Cancer Res (2018) 16(10):1523–9. doi: 10.1158/1541-7786.Mcr-18-0281
134. Nye J, Sturgill D, Athwal R, Dalal Y. HJURP Antagonizes CENP-A Mislocalization Driven by the H3.3 Chaperones HIRA and DAXX. PloS One (2018) 13(10):e0205948. doi: 10.1371/journal.pone.0205948
135. Chen D, Chen QY, Wang Z, Zhu Y, Kluz T, Tan W, Li J, et al. Polyadenylation of Histone H3.1 mRNA Promotes Cell Transformation by Displacing H3.3 from Gene Regulatory Elements. iScience (2020) 23(9):101518. doi:10.1016/j.isci.2020.101518
Keywords: histone, histone variants, H3.1, H3.3, chaperone, cancer
Citation: Wen T and Chen QY (2022) Dynamic Activity of Histone H3-Specific Chaperone Complexes in Oncogenesis. Front. Oncol. 11:806974. doi: 10.3389/fonc.2021.806974
Received: 01 November 2021; Accepted: 15 December 2021;
Published: 11 January 2022.
Edited by:
Keqiang Zhang, City of Hope National Medical Center, United StatesReviewed by:
A. Arockia Jeyaprakash, University of Edinburgh, United KingdomSongbai Liu, Suzhou Vocational Health College, China
Copyright © 2022 Wen and Chen. This is an open-access article distributed under the terms of the Creative Commons Attribution License (CC BY). The use, distribution or reproduction in other forums is permitted, provided the original author(s) and the copyright owner(s) are credited and that the original publication in this journal is cited, in accordance with accepted academic practice. No use, distribution or reproduction is permitted which does not comply with these terms.
*Correspondence: Qiao Yi Chen, cXljaGVuMjAzQHhqdHUuZWR1LmNu