- 1Department of Orthopedics, Tongji Hospital, Tongji Medical College, Huazhong University of Science and Technology, Wuhan, China
- 2Department of Oncology, Tongji Hospital, Tongji Medical College, Huazhong University of Science and Technology, Wuhan, China
Osteosarcoma is the most common primary bone malignancy in adolescents. Its high propensity to metastasize is the leading cause for treatment failure and poor prognosis. Although the research of osteosarcoma has greatly expanded in the past decades, the knowledge and new therapy strategies targeting metastatic progression remain sparse. The prognosis of patients with metastasis is still unsatisfactory. There is resonating urgency for a thorough and deeper understanding of molecular mechanisms underlying osteosarcoma to develop innovative therapies targeting metastasis. Toward the goal of elaborating the characteristics and biological behavior of metastatic osteosarcoma, it is essential to combine the diverse investigations that are performed at molecular, cellular, and animal levels from basic research to clinical translation spanning chemical, physical sciences, and biology. This review focuses on the metastatic process, regulatory networks involving key molecules and signaling pathways, the role of microenvironment, osteoclast, angiogenesis, metabolism, immunity, and noncoding RNAs in osteosarcoma metastasis. The aim of this review is to provide an overview of current research advances, with the hope to discovery druggable targets and promising therapy strategies for osteosarcoma metastasis and thus to overcome this clinical impasse.
Introduction
Osteosarcoma (OS) is the most common primary malignant bone tumor in children and adolescents and characterized by mesenchymal cells or osteogenic progenitor cells producing osteoid and immature bone (1). The estimated incidence rate of OS is 2–4/million/year worldwide, with first peak at age of 15–19 years (incidence: 8–11/million/year) and second minor peak at age of >60 years (2, 3). OS most commonly occurs in the metaphysis of long extremity bone, such as distal femur, proximal tibia, proximal femur, and proximal humerus, while it rarely arise in axial skeleton and other sites. The 5-year survival rate has reached a plateau in OS patients with localized disease ranging from 60%–70% since the introduction of systematic chemotherapy (4). However, the survival rate of around 20% is still dismal in patients with metastasis (5–7). More importantly, almost all patients are presumed to have subclinical micrometastatic lesions at diagnosis, whereas only 15%–20% of newly diagnosed OS are successfully detected with metastasis (8–10).
The OS cells show a high propensity to disseminate to develop metastasis, which appears to be the most important intrinsic factor for poor outcome of OS patients (5). OS can virtually metastasize to any sites or organs, mostly to lungs and occasionally to bone or lymph nodes (6, 11). The metastatic OS cells from the primary tumor undergo a series of critical steps to colonize and grow in the second site and finally progress into clinically detectable lesions. The biological behavior of metastasis is quite different from primary tumor with respect to cell cycle, differentiation, karyotype, metabolism and surrounding microenvironment, which is caused by differentially expressed genes, shift of molecule profiles, and interaction with microenvironment (12, 13). In the last few decades, a lot of research has been carried out to uncover potential mechanisms underlying OS metastasis and has made encouraging progress. Under the unremitting efforts, more and more biomarkers that are involved in metastasis and prognosis have been discovered. Functional experiments in cells and animal models further validate some remarkable genes and signaling pathways as well as their regulatory patterns responsible for metastasis (14–17). Based on these basic and preclinical studies, many clinical trials have also been initiated to identify novel therapeutic strategies against metastasis. Early diagnosis of metastasis especially micrometastasis will significantly innovate therapeutic modality and doubtlessly improve the prognosis of patients.
In this review, we performed a thorough literature search on OS metastasis and mainly discussed those studies that have been validated in vivo. Our focus was primarily on the biological behavior and underlying mechanisms of metastasis. We illustrated the panorama of OS metastasis from various perspectives, such as microenvironment, osteoclast, angiogenesis, metabolism, and immunity. Also, the role of noncoding RNAs in OS metastasis was also discussed, including microRNAs (miRNAs), long noncoding RNAs (lncRNAs), and circular RNAs (circRNAs). The aim of our study is to provide an overall insight into the cross-talk regulatory network in metastasis and to identify core nodes as potential targets for the development of novel therapies. Only breakthroughs in the diagnosis and treatment of metastatic OS can further improve the survival of patients.
Process of Metastasis
The dissemination of cancer cells from the primary tumor to a secondary site requires a set of multiple steps, and these metastatic cells exhibit completely distinct characteristics from primary tumor. The pulmonary metastasis process can be divided into three stages, including escape of cancer cells from primary tumor, transit within circulation system, and colonization and establishment of metastatic lesions in lung. Although a large number of tumor cells may gain the potential to enter this metastatic cascade, there are only a few cells that can survive to successfully form metastasis due to the limited efficiency in each step of metastatic cascade (18, 19). Schematic diagram of OS metastasis to the lung is shown in Figure 1.
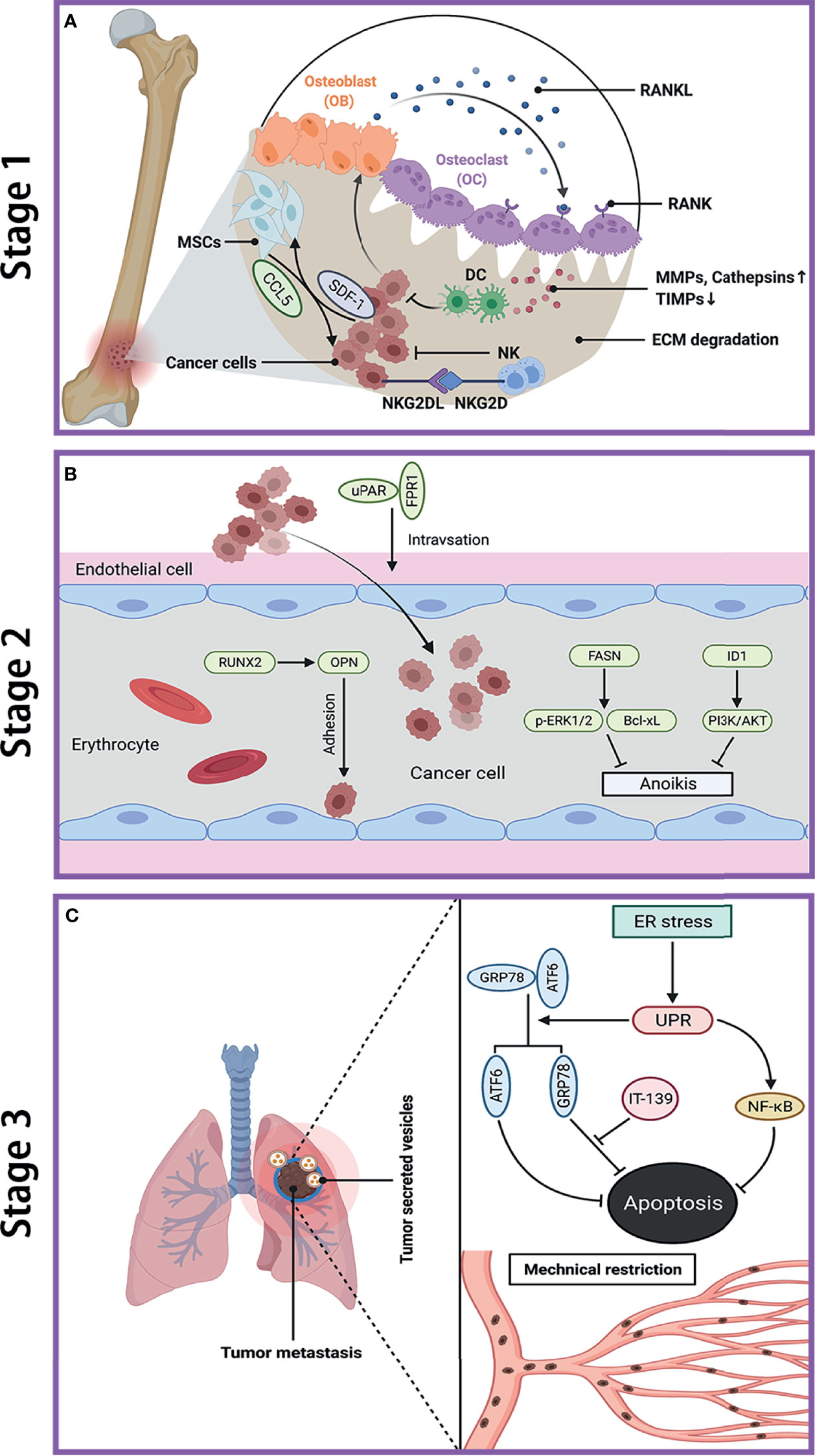
Figure 1 Metastatic cascade of OS to the lung. (A) Stage 1: dissemination of metastatic OS cells from primary site. Cancer cells induce OB to secrete RNAKL, which binds to OC, and lead to bone resorption. The increase of MMPs and cathepsins while decrease of TIMPs cause ECM degradation. Cancer cells secret SDF-1 to recruit MSCs, which in turn promote tumor growth and metastasis by secreting CCL-5. NK cells kill cancer cell through the interaction between NKG2D receptor and NKG2D ligands (NKG2DL). (B) Stage 2: transfer of OS cells in blood. The interaction between uPAR and FPR1 enhances invasion of cancer cell and their entry into blood. RUNX2/OPN axis promotes adhesion of cancer cell to endothelia cell in lung. FASN and ID1 increase anoikis resistance in cancer cell by upregulating p-ERK1/2 and Bcl-xL and by activating PI3K/AKT pathway, respectively. (C) Stage 3: colonization of OS cells in lung. The ER stress-initiated UPR protects cancer cell from apoptosis by activating GRP78 and ATF6, as well as through NF-κB pathway. The mechanical restriction of circulating cancer cells within lung microvasculature partly accounts for the propensity of lung metastasis. Tumor-secreted vesicles reach the lung in advance and direct cancer cell to transfer to the lung. OS, osteosarcoma; OB, osteoblast; OC, osteoclast; RANK, receptor activator of NF-κB; RANKL, receptor activator of NF-κB ligand; MMPs, matrix metalloproteinases; TIMPs, tissue inhibitor of metalloproteinases; ECM, extracellular matrix; SDF-1, stromal cell-derived factor-1; MSCs, mesenchymal stem cells; CCL-5, C–C motif chemokine ligand 5; NKG2D, natural killer group 2 member D; uPAR, urokinase-type plasminogen activator; FPR1, formyl peptide receptor type 1; RUNX2, runt‐related transcription factor 2; OPN, osteopontin; FASN, fatty acid synthase; ID1, inhibitor of differentiation or DNA binding; ERK, extracellular signal-regulated kinase; Bcl-xL, B-cell lymphoma-extra large; ER, endoplasmic reticulum; UPR, unfolded protein response; GRP78, glucose-regulated protein 78KD; ATF6, activating transcription factor 6; IT-139, a novel small molecule that inhibit GRP78.
Stage 1: Dissemination From the Primary Tumor
At the first stage of metastasis, OS cells with an invasive phenotype migrate away from primary tumor and then invade into surrounding tissues. Such process of cancer invasion is critically dependent on the destruction and degradation of basement membrane and extracellular matrix (ECM), which is catalyzed by pericellular and extracellular proteases, mainly by the matrix metalloprotease (MMP) family. Remarkably, a series of studies have suggested that many proteins and microRNAs can promote OS metastasis via upregulating various MMPs, including MMP-9 (20, 21), MMP-13 (22, 23), membrane-associated MT1-MMP (also called MMP14) (24), and MMP-16 (25). Cathepsin is another proteolytic enzyme to be involved in OS metastasis (26). Furthermore, some inhibitors targeting MMPs have been explored to suppress OS aggressive behavior by blocking this stage, such as tissue inhibitor of metalloproteinases (TIMPs) (27) and Nobiletin (28). In addition, the interactions between tumor cells and microenvironment such as endothelial cells (29) and mesenchymal stem cells (MSCs) (30, 31) may promote tumorigenicity, whereas interactions with primed dendritic cells (32) and natural killer (NK) cells (33) have antitumor effects. For instance, MSCs promote OS metastasis by the interaction of CCL5 from MSCs and SDF-1 from OS cells via autocrine/paracrine communication (31). Tumor lysate-pulsed dendritic cells have been studied in combination with other candidate strategies, such as agonist antiglucocorticoid-induced tumor necrosis factor receptor (anti-GITR) antibody (32), anti-cytotoxic T-lymphocyte antigen-4 (anti-CTLA-4) antibody (33), and antitransforming growth factor-β (anti-TGF-β) antibody (34), to further improve OS treatment via enhancing antitumor immunity. In addition, NK cells can kill OS cells, including tumor-initiating cells, through the interaction between natural killer group 2 member D (NKG2D) receptor and its ligand (NKG2DL) (35). The significance of osteoclast-mediated bone destruction and resorption has been revealed throughout the OS development and progression (36). Nevertheless, the exact role of osteoclast in OS metastasis remains controversial and subject to further clarification. A more detailed discussion would be presented in the subsequent part of osteoclasts and metastasis in this review. Figure 1A depicts the processes of OS cell invasion and migration at primary site.
Stage 2: Transit Within Circulation System
Firstly, OS cells need to intravasate into microvasculature such as blood vasculature by crossing endothelial cells and basement membrane and then travel within blood flow. The circulating tumor cells arrest and eventually extravasate out from blood into the target secondary organ. Several studies have investigated the interactions between tumor cells and endothelia cells and identified a few related molecules, including runt-related transcription factor 2 (RUNX2), osteopontin (OPN), urokinase-type plasminogen activator (uPAR), and formyl peptide receptor type 1 (FPR1), all of which are further shown to facilitate metastasis in vivo. In order to survive in blood vessels, OS cells must acquire anoikis resistance property, which is regulated by many genes (37) such as FASN and ID1. Additionally, tumor cells also encounter with various physical hemodynamic forces (e.g., fluid shear stress) during transit (38). The time and intensity of circulating tumor cells exposure to fluids will affect their fates either survival or apoptosis (39). The development of bone adapts to mechanical load, suggesting that OS cells might be sensitive to their surround physical stimuli (40, 41). Moreover, the changes in physical stimuli could not only affect biological behaviors of OS cells but also influence their response to chemotherapy and radiation therapy (42, 43). The transfer of OS cells within vasculature is shown in Figure 1B.
Stage 3: Colonization and Establishment of Metastasis in Lung
Under a poorly defined mechanism, the majority of circulating tumor cells arrive and arrest in lung microvasculature and subsequently extravasate into lung tissues, whereas only a minority of tumor cells can survive and eventually generate detectable metastasis (19, 44). Compared with primary site, the microenvironment at the secondary site presents a lot of differences, including oxygen tension, nutrition supply, and other physiochemical features. Lung is a foreign microenvironment, where tumor cells will undergo many challenges and face various fates, including apoptosis or death (e.g., immune clearance), dormancy, and proliferation into micrometastasis. In terms of micrometastases, they also have several fates either entering into angiogenic dormancy, or regression, or proliferation to form vascularized macrometastatic lesions (45, 46).
A few studies have attempted to elucidate the potential mechanisms by which OS cells conquer the selective pressures to successfully survive and proliferate in lungs. The response of OS cells to different stresses encountered in lungs is diverse. Endoplasmic reticulum (ER) stress can alter environment in ER lumen and disturb protein folding (47). The unfolded protein response (UPR) and UPR-related signaling pathways are also identified to dysregulated in OS (48, 49). Compared with low metastatic counterparts, OS cells with high metastatic potential express a higher level of ER chaperone protein HSPA5, whose inhibition can reduce lung metastasis (50). The ER stress may even partly account for the resistance of chemotherapy in OS (51). Moreover, the mechanism remains poorly understood by which OS cells preferentially metastasize to lungs. Based on current research, the mechanical restriction of circulating tumor cells within lung microvasculature might play a crucial role in lung tropism (52, 53). Another possible explanation of lung tropism is the notion of premetastatic niche (54). Extracellular vesicles, specifically exosomes released from cancer and stromal cells provide a favorable scenario for initiating organ-directed metastasis in several cancers, including melanoma (55), gastric cancer (56), pancreatic cancer (57), and cervical squamous cell carcinoma (58). A few reviews have discussed the role of exosomes in metastatic organotropism (59, 60), among which one publication focused on bone sarcomas (61). Moreover, Hoshino et al. revealed that tumor-derived exosomes could be uptaken by organ-specific cells to prepare the premetastatic niche, which is mediated by different exosomal integrins depending on the metastatic organs (62). Although their results were mainly from breast cancer, they also profiled the proteome of OS-derived exosome. Recently, several research groups have demonstrated that OS-derived exosomes enhance OS lung metastasis and some circulating plasma exosomal biomarkers are detected to be dysregulated in metastatic OS (63–66). Another study further confirmed the preferential seeding of OS-secreted EVs in lung tissue by fluorescence microscopy with lifetime imaging in vivo (67). Thus, we speculate that exosomes derived from metastatic OS cells may preferentially retain in lung to create a premetastatic niche by interacting with resident cells, and thus attract OS cells to migrate to lung and support their survival. More studies are needed to investigate the biodistribution of OS-secreted exosomes and whether molecules within exosomes direct OS cells to lung. A brief description of OS lung metastasis is presented in Figure 1C.
Molecular Mechanisms and Promising Targets
As mentioned above, each step of metastatic cascade is rate limited and therefore the key nodes in these steps can serve as potential targets for drug design. Some druggable targets have been summarized in other reviews based on which step the drug acts on (13, 68). Considering the clinical scenario that the majority of patients already have micrometastasis, novel therapy focusing on the later step of metastasis may be more effective. Herein, we provide an overview of biology behavior and regulatory networks of metastasis from various perspectives and summarize current research advances and explore potential targets. The data are extracted from articles, where the results have been validated in animal experiments. Based on the relevance and similarity among these data, we list the core genes and involved signaling pathways or events in Table 1. These genes serve as nodes to weave a biological regulatory network of OS metastasis through related pathways, which will continue to be extended and improved with further research. Promising therapy targeting such hub genes or pathways may open a new avenue to treat OS metastasis.
Microenvironment and Metastasis
Bone is a type of connective tissue with complex components, including various cells, soluble factors, and extracellular matrix (ECM). These components interact and influence with each other, by which the bone tissue maintains homeostasis in physiological conditions. The appropriate balance between bone formation and destruction determines normal structure of bone. Indeed, OS occurrence and metastatic initiation arise in such a complex bone environment. Additionally, metastatic cells also interact with surrounding microenvironment in each step of metastatic process, primarily in lung. Therefore, study on the interactions between microenvironment and metastatic cells will expand our understanding of OS biology. Increasing evidence has indicated that metastatic cells elicit and receive signals to and from microenvironment, which lead to metastasis inhibition or promotion. Figure 2 provides a brief overview of the interactions between metastatic OS cells and microenvironment.
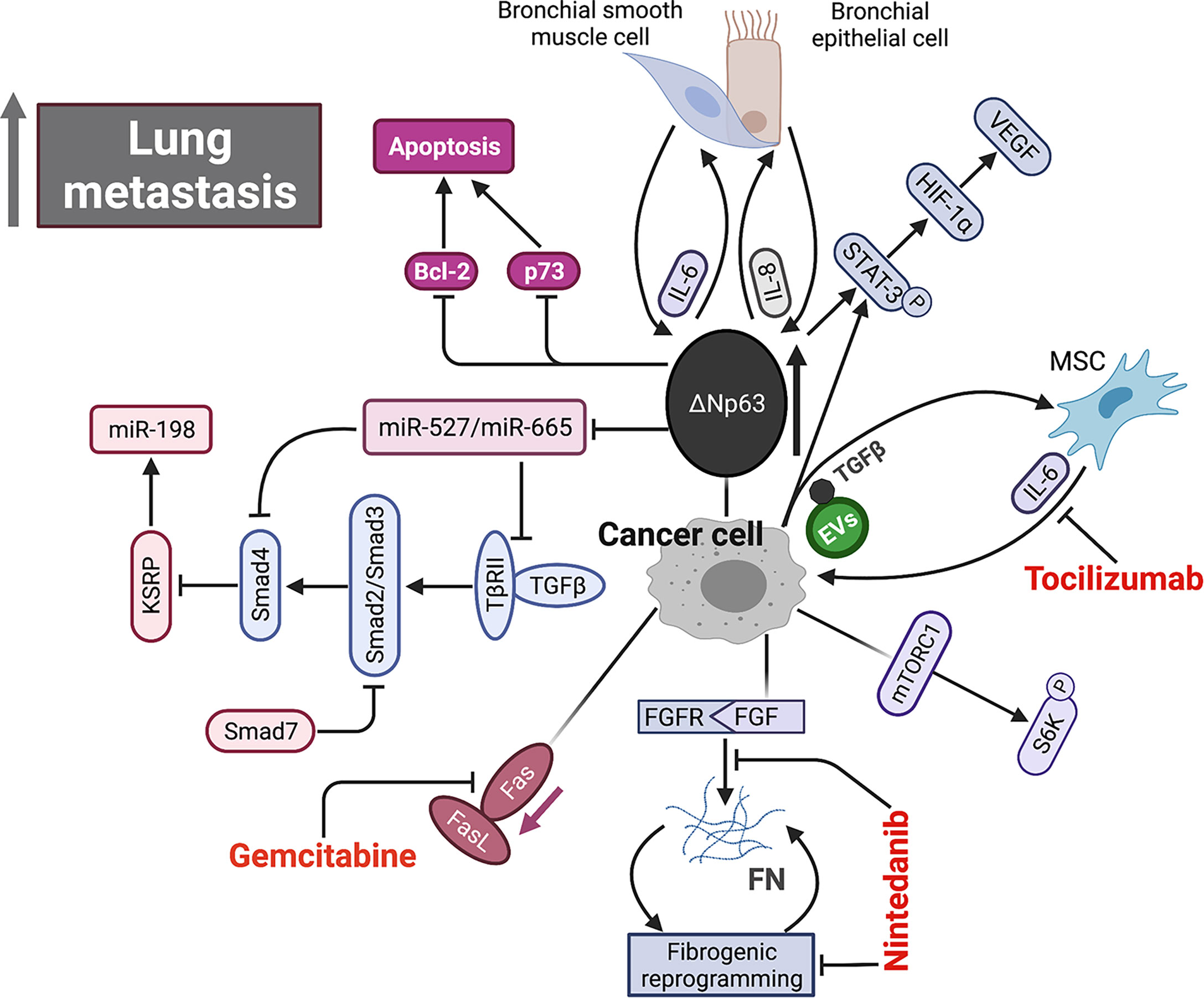
Figure 2 Schematic representation of signaling pathways within microenvironment underlying OS metastasis. Aberrant overexpression of ΔNp63 in cancer cell directly drives feed-forward amplification of IL-6 and IL-8 production by the interactions between cancer cell and both primary bronchial epithelial cell and bronchial smooth muscle cell. ΔNp63 overexpression leads to elevated phosphorylation of STAT-3, which further activates HIF-1α/VEGF axis. High expression of ΔNp63 promotes cancer cell survival by inhibiting Bcl-2 and p73-depedent apoptosis. In addition, ΔNp63 represses miR-527 and miR-665, leading to the upregulation of two TGF-β effectors, Smad4 and TβRII, which inhibits anti-metastasis miR-198 by suppressing its regulatory factor, KSRP. FGF signaling initiates and FN signaling sustains fibrotic reprogramming. Nintedanib targets the pan FGFR-FN axis to inhibit OS lung metastasis. Fas-negative OS cells are selected during metastasis by evading elimination in lung where Fas ligand (FasL) is constitutively expressed. Gemcitabine appears to be a promising agent by upregulating Fas expression. EVs secreted by OS cells selectively incorporate a membrane-associated form of TGF-β and induce IL-6 production by MSCs, which in turn promotes OS progression. IL-6, interleukin-6; IL-8, interleukin-8; STAT-3, signal transducer and activator of transcription 3; HIF-1α, hypoxia-inducible factor 1α; VEGF, vascular endothelial growth factor; TGF-β, tumor growth factor β; FGF, fibroblast growth factor; FN, fibronectin; EVs, extracellular vesicle.
TP63 is a member of the well-known tumor suppressor gene p53 family, and its splice variant ΔNTP63 is characterized by the lack of N-terminal transactivation domain. Bid and colleagues have shown that ΔNTP63 overexpression in OS cells increases phosphorylation of signal transducer and activator of transcription 3 (STAT3) by enhancing interleukin-6 (IL-6) and interleukin-8 (IL-8) secretion (123). In addition, phosphorylation of STAT3 can stabilize hypoxia-inducible factor 1-alpha (HIF1-α) and induce vascular endothelial growth factor (VEGF) secretion. Combined with clinical data, these results reveal a prometastatic role of ΔNTP63 in OS. Furthermore, the suppression of above cytokine/chemokine signaling pathways can reduce OS metastasis. In a mouse model, the inhibitions of IL-6 and C–X–C motif chemokine 8 (CXCL8, also called IL-8) significantly prolong survival by decreasing their deaths from metastasis (121). Moreover, the combination of inhibitors against IL-6 and CXCL8 achieves an intensive antimetastatic efficiency whereas each inhibitor alone only shows a modest effect. Another laboratory has reported that the metastatic OS cells expressing ΔNTP63α disseminate in a transforming growth factor beta (TGFβ)-rich microenvironment by upregulation of Smad4 and TβRII via suppressing miRNA-527/665 (120). In addition, TGF-β expression on the surface of extracellular vesicles (EVs), derived from OS cells, can induce the IL-6 secretion from mesenchymal stem cells (MSCs), which in turn facilitated proliferation of metastatic cells by activating STAT3. The administration of anti-IL-6 antibody disturbs this cross-talk signaling to reduce metastasis in mice (153). A research group recently has reported that myofibroblastic reprogramming of OS cells contribute to the formation of lung metastasis. This fibrotic reprogramming could be initiated by the activation of fibroblast growth factor (FGF) signaling and sustained by the resultant fibronectin (FN) deposition. They also demonstrated the efficacy of nintedanib in disrupting lung metastasis, but not in primary bone lesion, by blocking fibrotic reprogramming through inhibiting pan FGFR-FN axis (199).
Kleinerman and colleagues have indicated a noteworthy connection between OS cells and resident cells within lung, that is, the metastatic cells expressing Fas will be eliminated by binding to ligand (FasL), which is consistently expressed in lung (76). Under such selective pressure, only those Fas-negative OS cells or cells with nonfunctional Fas signaling can evade this defense mechanism and survive in lung (200). Thus, it is feasible to find agents that can induce Fas expression on OS cells as an alternative therapeutic approach against lung metastasis. This research group has identified two agents, chemotherapeutic agent gemcitabine and histone deacetylase inhibitor entinostat, both of which induce the regression of lung metastasis in wild-type mice by upregulating Fas expression (201, 202). However, such therapeutic efficacy was not observed in FasL-deficient mice since a FasL+ lung microenvironment is a prerequisite for this treatment (78, 203, 204). Moreover, another study confirmed this result again in a canine model with lung metastasis (205). These findings suggest that incorporating lung microenvironment as part of the therapy strategy may benefit patients with established lung metastasis. As an important factor in microenvironment, EVs like tumor-derived exosomes contain various components (e.g., proteins and nucleic acids) and play an essential role in intercellular communication and metastatic progression in a variety of cancers (206). Notably, the expression profiles of EVs from OS samples with low metastatic potential are significantly distinguished from that in high metastatic potential samples by transcriptome analysis (207). A study further indicated that culture medium supplemented with exosomes would change secretome of OS cells and affect their aggressive properties (208). Interestingly, proteomic analysis of the EVs derived from highly metastatic OS cells shows the enrichment of metastasis-related proteins. The EVs from highly metastatic clonal variants of OS can even be internalized into low metastatic cells and thereby endow them with metastatic ability through horizontal phenotypic transfer (67). Also, an in vivo metastatic model further confirmed the potential of OS-derived EVs to promote metastasis (65). The exosomes released by OS cells, carrying programmed death-ligand 1 (PD-L1) and N-cadherin, could also stimulate pulmonary metastasis (63), and a recent study found that the plasma exosomal sentrin SUMO-specific protease 1 (SENP1) level is closely related to pulmonary metastasis in OS patients (66). As such, OS cells preferentially migrate and localize to lung directed by the EVs they have secreted, which may partly account for lung tropism of metastasis. The role of cancer-derived exosomes in cancer development and progression was systematically discussed in a review by Kok et al., mainly elaborating on the trafficking of enriched genetic signals carried by exosomal cargo in fostering cancer progression in several tumor types, including OS (209). In addition to exosomes derived from the tumor itself, exosomes from other cells such as bone marrow mesenchymal stem cells (210), adipose-derived mesenchymal stem cells (211) and tumor-associated macrophages can also affect metastasis of osteosarcoma (212, 213). OS cells and non-OS cells in tumor microenvironment could influence themselves or each other by releasing EVs through autocrine/paracrine pathways, shaping tumor microenvironment, modulating cell biological behaviors, especially the aggressiveness of OS cells. The secreted CXCLs within microenvironment selectively recruit different types of cells through binding to their transmembrane receptors. Tumor cells and leukocytes expressing CXCRs migrate following CXCL gradient (214). The CXCL/CXCR axis plays an essential role in leukocyte trafficking, immune homeostasis maintenance like T-cell homing, directional migration of tumor cells. It is well documented that the binding of CXCL12 (also known as SDF-1) to its receptor like CXCR4 or CXCR7 remarkably promotes tumor progression including OS (100, 118, 215). In primary bone site, OS epigenetically downregulates CXCL12 expression by DNMT1, impairs cytotoxic T-cell homing to the tumor site and this chemokine gradient of CXCL12 drives the metastasis of OS cells to the lung (Figure 3A), where CXCL12 is highly expressed. The constitutive expression of CXCL12 in lung may largely determine lung as the main site of OS metastasis (Figure 3B). In addition to CXCR4, CXCR7 is another receptor for CXCL12 and also participate in OS lung metastasis (110). The coexpression of CXCR7 and CXCR4 on OS cells could enhance their metastatic ability, since a chemokine gradient of CXCL12 between bone and lung is produced through CXCR7-mediated CXCL12 scavenging in primary bone site (116).Moreover, osteoprotegerin could induce SDF-1 secretion from endothelial cells, which further promotes OS development by increasing neovascularization via SDF-1/CXCR4 axis (216). Nigris et al. indicated that transcriptional repressor Yin Yang 1 protein (YY1) enhances metastatic potential of OS cells by activating VEGF/CXCR4 axis (112). Another study also suggested that VEGF secreted from MSCs promotes CXCR4-mediated metastasis (108). In addition, IL-8 produced by MSCs increases anoikis resistance and metastasis of OS cells by regulating CXCR1/Akt signaling pathway (104). Gozo and colleagues has demonstrated that forkhead box protein C2 (FOXC2) maintains OS cells in a stem-like state and promotes metastasis by increasing CXCR4 (114). More importantly, the inhibition of CXCR4 by its antibody (98, 106) or inhibitor (like AMD300) (217) can successfully reduce metastasis. Due to the prominent role of CXCL/CXCR axis in metastasis and its close association with angiogenesis, anoikis, and immune response, novel therapy targeting this axis might be promising for treating metastasis.
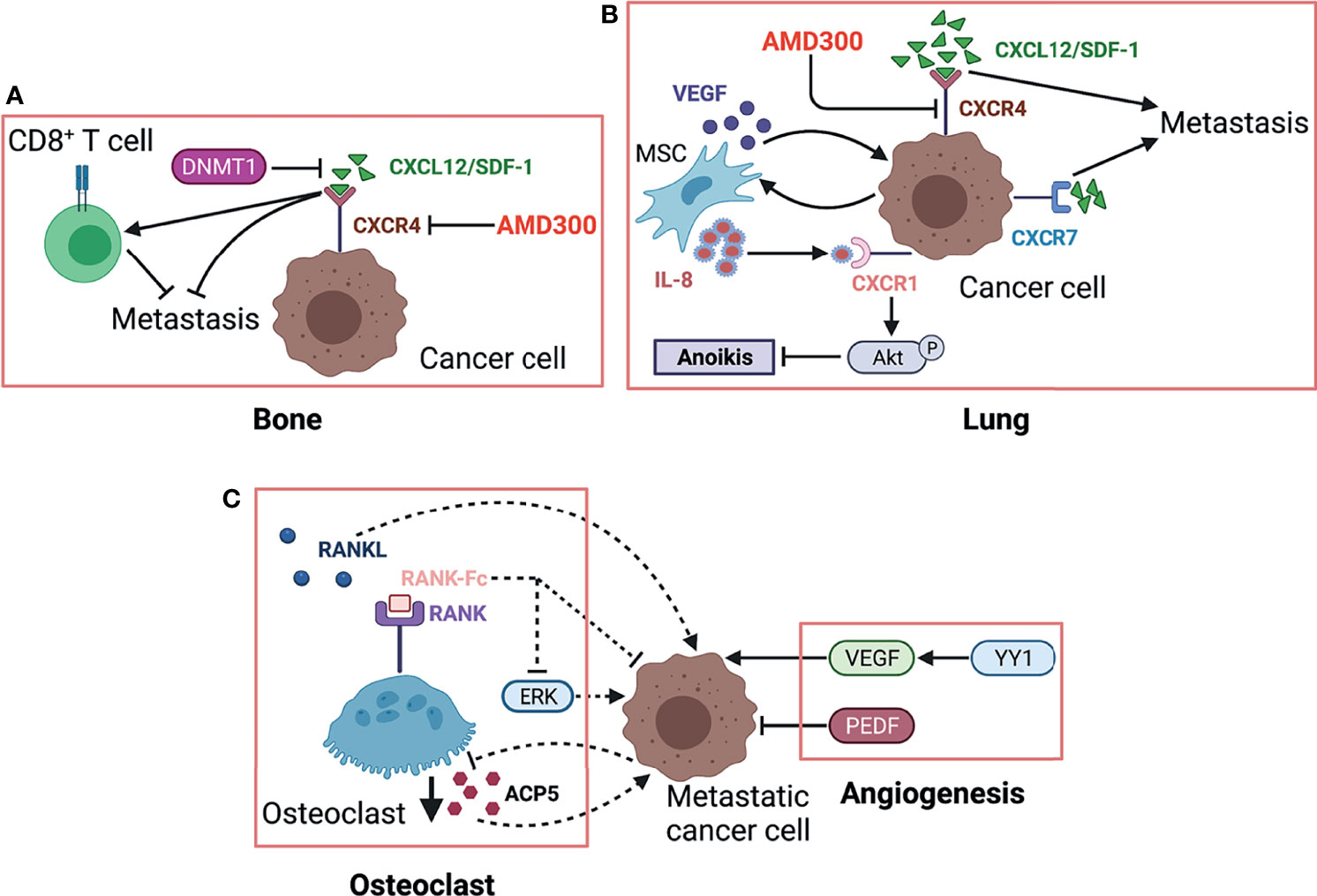
Figure 3 The role of osteoclast and CXCR/CXCL axis in OS metastasis. (A) Highly expressed CXCL12. The CXCR4/CXCL12 (SDF-1) interaction is critical for OS metastasis in the lung, which is further strengthened by MSC via secreting VEGF. MSC-derived IL-8 induces OS cell anoikis resistance by activating CXCR1/Akt signaling. Another receptor CXCR7 expressed on OS cells promotes lung metastasis and enhances the malignancy activity of CXCR4. (B) RANK-Fc binds to RANK as a potent RANKL antagonist to inhibit osteoclast formation and activity, which can reduce OS metastasis, partly by suppressing ERK. Controversially, metastasis-competent OS cells induce loss of ACP5+ osteoclasts, which in turn enhances metastasis. Herein, we used dotted lines to indicate this contradiction. VEGF exhibits prometastatic effects on OS cells while PEDF shows the opposite by regulating angiogenesis. (C) In primary bone site, OS epigenetically downregulates CXCL12 expression by DNMT1, impairs cytotoxic T-cell homing to the tumor site, and this chemokine gradient of CXCL12 drives the metastasis of OS cells to the lung. ACP5/TRAP, osteoclast-specific tartrate-resistant acid phosphatase 5; PEDF, pigment epithelium-derived factor; YY1, Yin Yang 1 protein; CXCL12, C–X–C motif chemokine 12; DNMT1, DNA methyltransferase 1; CXCR4, chemokine receptor 4; AMD3100, CXCR4 antagonist.
Osteoclasts and Metastasis
The progression of bone tumor leads to osteolysis (218), which in turn promotes the dissemination of tumor cells and thus forms by a vicious cycle. Breaking this vicious cycle between osteoclasts and tumor cells may be one of the promising ways to treat OS metastasis. Several studies have used RANK-Fc to perturb TNFRSF11A/TNFSF11 (also called RANK/RANKL) axis and successfully reduced metastasis by inducing anoikis and apoptosis of OS cells (107, 109, 111). In addition, other agents that can restrict bone resorption are also expected to become potential candidates to inhibit metastasis, such as inhibitors against transcription factor Sp7, TNFSF10 and TGF-β/Smad signaling. Anti-osteoclast drug like zoledronic acid could be synergistically used to enhance the therapy efficacy for OS progression (219). One publication has discussed the prospects of novel therapeutic strategy targeting osteoclast activity for OS (220). However, Munoz et al. suggested the tendency of mutual restrain between metastatic OS cells and osteoclasts and that metastasis-competent OS cells induce the loss of ACP5+ osteoclasts, which in turn facilitate metastasis (119). Notably, EVs derived from OS could suppress osteoclastogenesis and further enhance its metastasis (65). An in vitro study was performed to directly explore the reciprocal modulation between OS and osteoclastic cells by a co-culture system (221). More studies are needed to further clarify the exact role of osteoclast in OS metastasis. The interaction between osteoclasts and OS cells was displayed in Figure 3C.
Angiogenesis and Metastasis
Angiogenesis is an essential component for tumor growth and progression by supplying adequate blood and nutrients (75, 222). Additionally, these new blood vessels provide the principal route by which cancer cells exit the primary site and enter circulation (223). Angiogenesis is also required for tumor colonization at the site of metastasis. In OS metastasis, intensive investigation has explored the role of angiogenesis and interaction between proangiogenic and antiangiogenic factors, in order to develop potential optimum targets for antiangiogenic therapy (Figure 3A). A meta-analysis including nine articles revealed that VEGF is positively associated with tumor metastasis and a higher tumor grade (70). Two publications also suggested that angiopoietin-like protein 2 and YY1 accelerate metastasis via VEGF-mediated angiogenesis (112, 224). Furthermore, VEGF knockdown (73) restricts OS metastasis. Also, the blockade of VEGF receptor 2 (VEGFR2) by anlotinib, a tyrosine kinase inhibitor, results in metastasis suppression in a preclinical study (225). In contrast to VEGF-related angiogenesis, pigment epithelium-derived factor (PEDF) or PEDF-derived synthetic peptides both exhibited antimetastasis activity by inhibiting angiogenesis (77, 79, 81, 226). Antiangiogenesis therapy seems to be an appealing and attractive alternative strategy to manage OS metastasis. However, the safety and efficacy of antiangiogenic therapy has not been confirmed in clinical trials, more work is needed to achieve clinical transformation of this treatment. Current research progress and application limitation in antiangiogenesis therapy for OS can refer to other reviews (227, 228).
Metabolism and Metastasis
The metabolic program of primary tumor cells is quite different from that of metastatic cells in many ways such as nutritional availability, energy demand, oxygenation level, metabolites, and metabolic pathways, all of which participate in tumor metastasis (229). An advanced metabolomics has shed light on the study of biochemical status and reprogrammed metabolism during metastasis. Metabolomics covers a wide range of metabolites, mainly including sugars, amino acids, and fatty acids. Giang et al. have observed the Warburg effect in several human OS cell lines (230), a common phenomenon of aerobic glycolysis in cancer cells that means pyruvate is transformed into lactic acid even in the presence of oxygen (231). Further study in mice by Hua and colleagues found the dynamic metabolic reprogramming throughout tumor occurrence and progression (232). They identified a number of differentially expressed metabolic biomarkers in serum prior and postmetastasis. Their results suggested the metabolic reprogramming in OS metastasis, characterized by lowered carbohydrate and amino acid metabolism, while an elevated lipid metabolism. Moreover, the serum metabolic profile of lung metastasis is distinct from that of primary tumor. Mice developing lung metastasis have a higher level of lipid metabolites in serum compared with mice without metastasis (232). Consistently, a global analysis of lipidomic reveals the alteration of lipid profiles in metastatic OS cells (233). Previous studies using synvinolin (inhibitor of de novo cholesterol synthesis) observed metastasis reduction, which further supported a critical role of lipid metabolism in tumor metastasis (234, 235). The inositol pathway is significantly downregulated in highly metastatic OS cells (236). Functional study both in ex vivo lung culture and in vivo mouse model has indicated that the administration of inositol hexaphosphate, which will be converted to inositol once entering cells, can reduce lung metastasis by suppressing MAPK and PI3K signaling pathways.
HIF1-α expression is positively associated with metastasis while negatively with survival. Naggar et al. has shown that Y-box-binding protein 1 (YB-1) facilitates metastasis by direct translational activation of epithelial-to-mesenchymal transition (EMT) and HIF1-α, which then induces CXCR4 expression (194). Functionally, HIF1-α enhances invasion of OS cells in hypoxia via increasing VEGF-A (237). Another pathway, HIF1-α/CXCR4 is also proven to facilitate metastasis in vitro and in vivo (238). Additionally, HIF-1α binds to AP-1-binding motif within Cyr61 (also called CCN1) promoter and induces Cyr61 expression, which plays a prometastatic role in human melanoma cells under hypoxia (239). Hypoxia is one of the prominent features of many malignant tumors and also provides an opportunity to develop agents that target hypoxic region. For instance, hypoxia-activated prodrug TH-302 can reduce OS metastasis as a single agent or in combination with chemotherapy (240). The high expression of Cyr61 indicates poor survival in OS patients (91) and promotes metastasis via activating PI-3K/Akt/GSK3β, IGF1/IGFR signaling pathways, facilitating angiogenesis characterized by an increase in VEGF, FGF2, PECAM, and a decrease in TSP-1 and SPARC (93) and promoting EMT-like process (95). Of note, WW domain containing oxidoreductase (WWOX) as tumor suppressor has been revealed to maintain mitochondrial respiration and attenuate Warburg effect by inhibiting HIF1-α (241) and c-Jun (242) by physical interaction with them. Recently, a research group further found that WWOX inhibits OS metastasis in vitro and in vivo through downregulation of RUNX2 (243). The suppression of c-Jun activity can inhibit metastasis by increasing apoptosis (85, 89) and chemosensitivity in OS cells (87). Tang and colleagues demonstrated that cadherin-4 (CDH4) overexpression would activate c-Jun via the JNK pathway (83). In addition to c-Jun, proteins from several families like c-Fos, ATF, and MAF, can form transcriptional complex AP-1, and their activation may be one of the mechanisms underlying metastasis (244). Leaner et al. have shown a higher activity of AP-1 in highly metastatic OS cells, compared with low metastatic counterparts (245). In terms of OS, AP-1 promotes metastasis by upregulating podoplanin and TGF-β (246). Another study also reported that FGFR1 silence, a downstream target of c-Fos/AP-1 complex, could significantly reduce lung metastasis (247). The growth and progression of malignant tumors largely rely on glycolysis for energy, resulting in an acidic tumor microenvironment, which may in turn affect the biological behaviors of tumor cells. Several studies have investigated how OS cells adapt to acidic microenvironment. Brief exposure of OS cells to acidic condition results in cell death, whereas prolonged exposure reverses cell death, and even fosters tumor invasiveness (40). The adaption of OS cells to acidosis leads to a metabolic reprogramming with epigenetic stability (248). The underlying mechanism has been further explored, by which acid microenvironment activates a stress-regulated switch to support cell survive. Studies found that cIAP proteins and NF-κB pathway are activated in OS cells in response to acid tumor microenvironment (249, 250). Metabolism-related regulatory networks are shown in Figure 4.
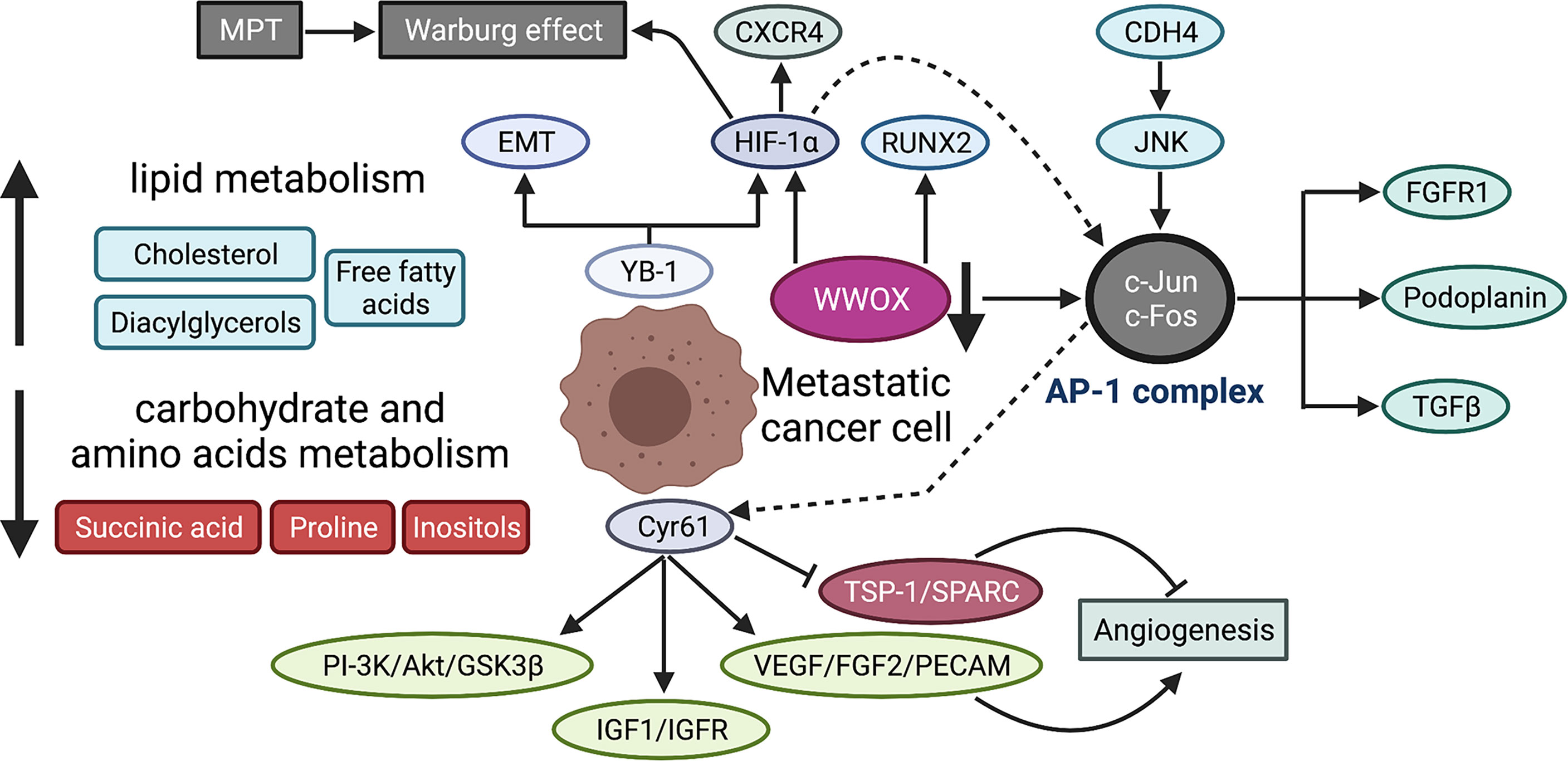
Figure 4 Metabolic reprogramming during OS metastasis. MPT promotes Warburg effect in OS cells by suppressing mitochondrial function. The serum metabolic profile of lung metastasis shows lowered carbohydrate and amino acid metabolism but an elevated lipid metabolism. YB-1 contributes to metastasis by translational activation of EMT and HIF-1α, which then induces CXCR4 expression. Cyr61 enhances the metastatic potential of OS cells through multiple signaling pathways, including PI-3K/Akt/GSK3β, IGF1/IGFR, and angiogenesis-associated signaling (increased VEGF, FGF2, PECAM and reduced TSP-1, SPARC). WWOX maintains mitochondrial respiration and inhibits Warburg effect by physical interaction with HIF-1α. WWOX also suppresses c-Jun activity by physical association while CDH4 overexpression activates c-Jun via the JNK pathway. AP-1 is a transcriptional complex, mainly composed of c-Jun and c-Fos, which promotes metastatic potential by upregulating several downstream effectors, including FGFR1, podoplanin, and TGFβ. The dotted lines indicate the mechanistic study is performed in melanoma cells, that is, HIF-1α interacts with AP-1, which then binds to AP-1-binding motif within the Cyr61 promoter and induces Cyr61 expression. MPT, mitochondrial permeability transition; YB-1, Y-box binding protein 1; EMT, epithelial-to-mesenchymal transition; Cyr61, cysteine-rich protein 61; IGF-1, insulin-like growth factor 1; FGF2, fibroblast growth factor 2; PECAM, platelet endothelial cell adhesion molecule; TSP-1, thrombospondin-1; SPARC, secreted protein acidic and rich in cysteine; WWOX, WW domain-containing oxidoreductase; CDH4, cadherin-4; AP-1, activating protein-1; FGFR1, fibroblast growth factor receptor 1.
As mentioned above, redox stress is a metabolic challenge in lung microenvironment for tumor cells. Studies shown that the excess reactive oxidative species (251, 252) and reactive nitrogen species (253, 254), both impair mitochondrial function. In order to overcome such oxidative pressures, metastatic OS cells trigger their antioxidant response by upregulating redox-related enzymes or glutathione-related metabolic pathways (255, 256). However, the previous mentioned study by Hua et al. reported the contradictory result that glutathione pathway is suppressed at the metastasis phase (232). More studies are urgently needed to further elucidate the exact role of metabolism especially glucose metabolism in tumor cells during dynamic metastatic process. Thereby, the exploration of metabolism reprogramming and metabolic vulnerability may provide novel targets for treating metastasis.
Immunity and Metastasis
It is well known that immune system both innate and adaptive immunity plays a key role in tumorigenesis and progression, and even to a large extent determines the fate of tumor cells. With the advance in basic and preclinical research, increasing immune-based therapies are being approved for clinical use in various cancers, which yield encouraging outcomes in those patients who are resistant to conventional treatment. Nevertheless, the application of immunotherapy in metastatic OS is far from satisfactory to date. Recently, a series of studies identified several immune cells, including innate immunocytes (e.g., macrophages and dendritic cells) (257–259) and adaptive immunocytes (e.g., T lymphocytes) (260), all of which putatively participate in immune response during OS metastatic progression (Figure 5). The number, function, state of immune cells, and their interactions with each other collectively determine whether they promote or inhibit metastasis. Researchers have also recognized some immunocytes as diagnostic or prognostic biomarkers. For instance, the increase of M2-polarized tumor-associated macrophages (TAMs) maintains OS stemness and metastatic property (261). In addition, TAMs activated by mifamurtide (262) or M2-type macrophages suppressed by all-trans retinoic acid (ATRA) (263, 264) both can inhibit tumorigenicity and progression of OS cells. However, the inconsistent results among studies are available, which require more research to further elucidate the dynamic and complex role of TAMs in OS. In addition to TAMs, tumor-infiltrating lymphocytes (TILs) within OS microenvironment also affect OS progression (261, 265). The increased CD8+ TILs as well as the high ratio of CD8+/FOXP3+ TILs both predict a better prognosis in OS patients (266, 267). However, the higher density of TILs is found to be within metastatic sites compared with primary tumor, which constitutes a special immune niche (82, 267). It should be noted that the interaction between PD-L1 (expressed in metastatic OS cells) and PD-1 (expressed in tumor-infiltrating cytotoxic T lymphocytes) limits antitumor function of T cells and thus promotes OS metastasis by evading immune surveillance. The dynamic and intricate regulation of immune system seems to be decisive for metastasis with respect to synthesis of immune factors, spatiotemporal expression of surface markers and phenotypic transition of immunocytes, and their interactions with surrounding compositions. With increasing understanding of immunomodulatory mechanism underlying OS metastasis, researchers are constantly exploring the key immune checkpoints to develop potential immunotherapy strategies for OS with the aim of improving prognosis. A recent review has discussed the mechanisms and status of immunotherapy for OS (268).
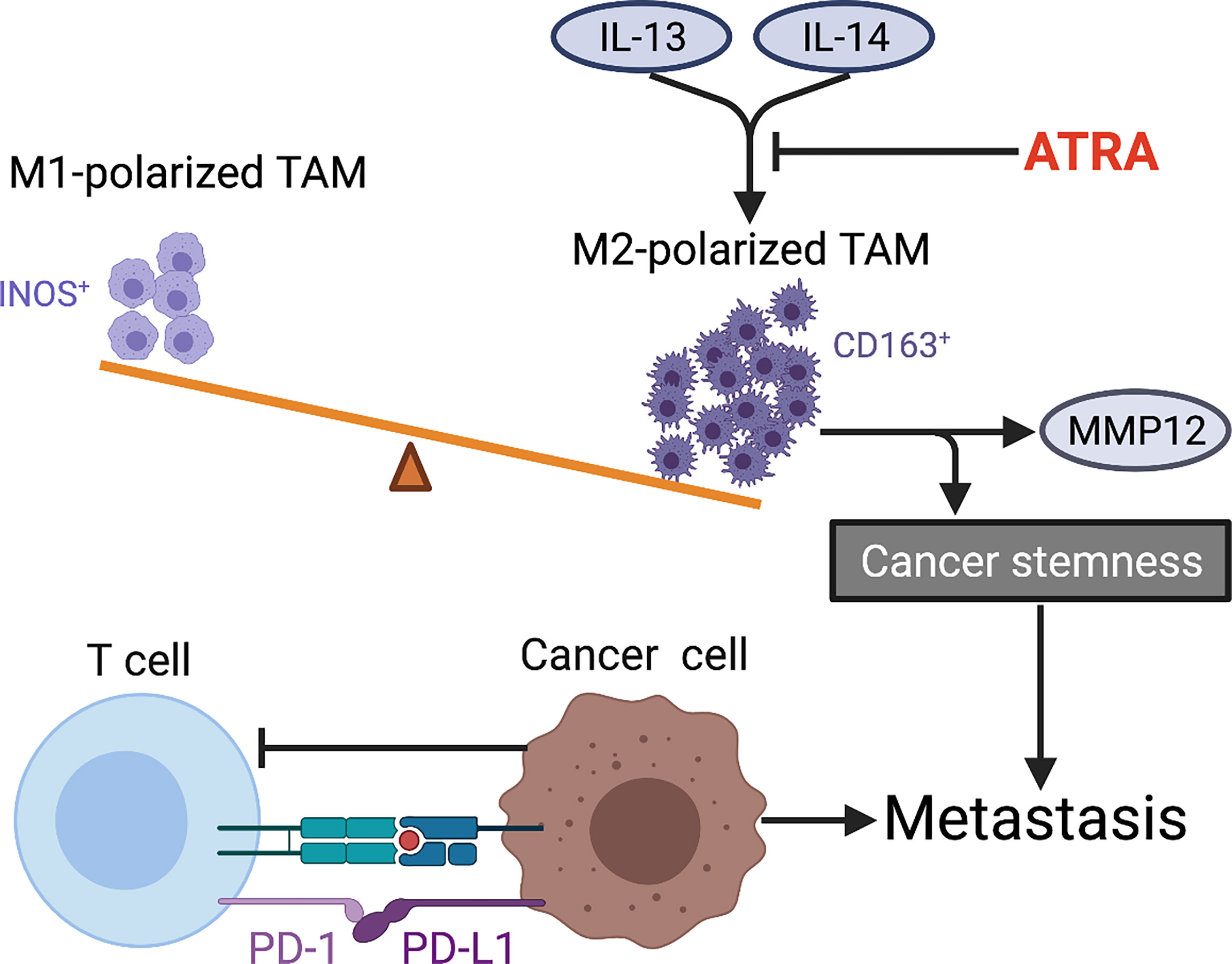
Figure 5 Tumor immune microenvironment characteristics within OS metastasis. The imbalance of M1 (INOS+)/M2 (CD163+)-polarized TAMs in favor of M2 subtype is observed in metastatic OS. ATRA suppresses IL-13-induced secretion of MMP12 from M2-polarized macrophages and also weakens cancer stemness by preventing M2 polarization of TAMs in OS. The interaction between PD-L1 (expressed in metastatic OS cells) and PD-1 (expressed in tumor-infiltrating CTLs) limits antitumor function of T cells and thus promotes OS metastasis by evading immune surveillance. TAMs, tumor-associated macrophages; ATRA, all-trans retinoic acid; PD-L1, programmed death ligand 1; PD-1, programmed death receptor-1; CTLs, cytotoxic T lymphocytes.
Noncoding RNA
Noncoding RNAs are a large group of RNAs characterized by the lack of ability to encode proteins, which can be divided into regulatory and housekeeping noncoding RNAs (269). Here, we focused on the role of the former in OS metastasis, including microRNAs (miRNAs), long noncoding RNAs (lncRNAs), and circular RNAs (circRNAs). We further outlined the biological function of these noncoding RNAs in OS metastasis and the underlying molecular mechanism. Table 2 summarizes the noncoding RNAs that have been validated in vivo.
MicroRNA
MiRNAs contain 18–25 bases and most of them fell within 21–23 bases. Typically, miRNAs bind to 3′untranslated region (3′UTR) of mRNAs by complementarily pairing to destabilize mRNAs or block translation and thus reduce their expressions (349–351). A single miRNA can target multiple mRNAs and vice versa, one mRNA can be regulated by various miRNAs. Thereby, miRNAs may indirectly affect various biological processes such as proliferation, differentiation, apoptosis, and angiogenesis. The unique signatures of miRNA expression profile have been studied in OS metastasis as diagnostic and/or prognostic biomarkers. More importantly, an enormous growing body of studies have explored potential mechanisms, by which miRNAs participate in OS metastasis.
A minority of the miRNAs that we retrieved from literature have been demonstrated for their effects on OS metastasis in vivo. Also, only few miRNAs show prometastatic activity in OS, while the majority of miRNAs inhibit metastasis. A study reported that miR-195 suppressed metastatic potential by targeting FASN (352). However, other studies found a high level of miR-195 in serum from OS patients (353, 354). Thus, a single miRNA might play a dual role in different stages of OS progression or the seemingly contradictory results are probably caused by small number of participants. Further studies are needed to confirm the exact function of such miRNAs like miR-195. Whether miRNAs promote or inhibit metastasis depends on proteins they target. Intriguingly, miR-27a* is the first passenger miRNA strand and shows prometastatic activity in OS cells, which is encoded by MIR27 and directly connects with CBFA2T3, the same as miR-27a (355). Furthermore, the injection of OS cells expressing miR-27a to mice can generate metastatic nodules within lung and bone in vivo (356, 357). In turn, miRNAs are also regulated by metastasis-associated genes such as MYC, TP53, and TGFB1. The epigenetic modulation affects the miRNA expression as well. One research team found that apurinic/apyrimidinic endodeoxyribonuclease 1 (APEX1) participated in multiple biological processes of OS by shifting miRNA expression profiles (358). Some drugs used to treat OS-like epirubicin (359) and diallyltrisulfide (360) can also alter miRNA expression profiles.
Unfortunately, miRNA-mediated therapy still remains the first phase of clinical trial at best despite so much basic and preclinical research. The main issue needed to be solved is how to deliver miRNAs efficiently and precisely to the sites of interest. Current methods generally show the following deficiencies, including low transfection efficiency, rapid degradation, and abnormal accumulation in nonspecific tissues and organs. Using hyaluronic acid-associated liposomes as carrier may accelerate the entry of miRNAs into cells and prevent them from degradation (361). An alternative approach is to apply viral vectors with hairpin molecules that will be processed into mature miRNAs (362).
Long Noncoding RNA
LncRNAs are a large group of noncoding RNAs with more than 200 nucleotides and emerging evidence demonstrates its crucial role in multiple biological processes, particularly in tumorigenesis (363, 364). Briefly, lncRNAs in nucleus can regulate gene expression both at genetic and epigenetic levels by affecting transcription factors and chromatin-modifying complexes to bind specific gene loci. In cytoplasm, lncRNAs modulate the stability and translation activity of mRNAs either directly or indirectly. The majority of lncRNAs included in our study compete with miRNAs to bind target mRNAs as sponges. Recently, lncRNAs have engaged much attention in the field of tumor aggressiveness, including migration, invasion, and metastasis. Unlike miRNAs, most of lncRNAs involved in OS are positively associated with metastatic property. We outlined those lncRNAs that have been widely studied in OS metastasis and confirmed in vivo.
Metastasis-associated lung adenocarcinoma transcript 1 (MALAT1), also named noncoding nuclear-enriched abundant transcript 2 (NEAT2), is located in chromosome 11q13 with a length of 8.7kb (365). The MALAT1 overexpression is closely correlated with advanced clinical stage and distant metastasis in OS patients (366). Furthermore, a meta-analysis confirmed the predictive value of MALAT1 for metastasis and poor prognosis in six different types of cancer, including OS (367). Consistent with this result, another meta-analysis only including OS also showed MALAT1 as a valuable biomarker for prognosis (368). MALAT1 knockdown inhibits OS metastasis in vitro and in vivo by affecting various downstream pathways, such as downregulation of PI3K/Akt/MMP-9 signaling pathway (334, 335) and upregulation of E-cadherin and several miRNAs (336, 369). Also, high dose of 17β-estradiol inhibits metastatic potential of OS cells by suppressing MALAT1 while low dose presents the opposite effect (370, 371). It is noteworthy that the most widely studied lncRNAs is the large family of small nucleolar RNA host gene (SNHG), of which all members are considered to promote OS progression and metastasis by sponging various miRNAs. SNHG1, for example, activates Nin one binding protein (NOB1) by sponging miRNA-326 and thus promotes migration/invasion in OS cells and metastasis in murine models (341). Consistently, additional research further shows that SNHG1 is positively related to advanced clinical stage, distant metastasis, and poor survival of OS patients. In addition, SNHG1 facilitates cell proliferation, migration, and invasion via activating PI3K/AKT and Wnt/β-catenin pathways by sequestrating various miRNAs (341, 342, 372). Besides, SNHG12 promotes metastasis by increasing angiomotin (373), Notch2 (374) and IGF1R (375) through sponging miRNA-195-5p. There are two research groups that independently demonstrated the role of lncRNA DANCR in OS progression and possible underlying mechanisms. As a competitive endogenous lncRNA, DANCR either increases rho-associated protein kinase 1 (ROCK1) through decoying miRNA-335-5p and miRNA-1972 (376), or enhances AXL/PI3K/Akt signaling pathway by sponging miRNA-33a-5p (377), and thereby promotes OS metastasis.
Circular RNA
CircRNAs are a newly identified class of endogenous noncoding RNAs characterized by closed-loop structures. Unlike typical linear RNA, circRNAs do not contain 5′-3′ polarity or polyadenylated tail and is thus resistant to enzyme degradation (378, 379). Similar to lncRNAs, circRNAs can competitively bind miRNAs as sponges to indirectly regulate gene expression. Currently, increasing evidence indicates that circRNAs have an important role in many biological behaviors and diseases, particularly in tumorigenesis and tumor progression. The dysregulation of some circRNAs has also been identified in OS metastatic process (380). Almost all circRNAs, like lncRNAs, act as sponges of miRNAs to disinhibit specific prometastatic pathways that normally are inhibited by miRNAs in OS. However, it is not always the case. It has been reported that low expression of circ-HIPK3 is observed in OS cell lines, tissues, and plasm and associated with lung metastasis (381). Overexpression of circ-HIPK3 can inhibit proliferation, migration, and invasion in OS cells. These findings indicate that circ-HIPK3 may have great value in clinical practice as a tumor suppressor. Intriguingly, circ-HIPK3 plays the opposite role in tumor occurrence and progression in other tumors, which strongly suggests that whether a single circRNA acts as an oncogene or a tumor suppressor gene depends on tumor types and which miRNA it sponges (382–386). In addition, circRNAs hsa_circ_0002052 and circ-ITCH are also found to be downregulated in OS cell lines and tissues. Functional studies further confirm that their overexpression can suppress OS cell progressiveness by inhibiting Wnt/β-catenin pathway via targeting miRNA-1205/APC2 axis (387), and by suppressing PTEN/PI3K/AKT and Sp1 pathways via sponging miRNA-22 (388), respectively. So far, the knowledge and research on circRNAs in OS metastasis are much less than that of miRNAs or lncRNAs. Nevertheless, the special annular structure gives circRNAs unique advantages with stronger stability and higher abundance.
In addition to tumor tissues, collecting noncoding RNAs from blood is convenient in clinical practice. Notably, a number of studies have reported that exosomes could carry noncoding RNAs (212, 213) and even transport them into OS cells (210), thus foster OS metastasis. With the deeper understanding of the relationship between noncoding RNAs and clinicalpathological characteristics, noncoding RNAs will help early diagnosis of metastasis, monitoring of treatment, and prediction of prognosis in OS patients. Although there is no therapy targeting noncoding RNAs used for OS yet, further research will make significant progress to develop novel therapeutic strategies and improve outcomes for OS patients with metastasis.
Discussion
In conclusion, metastasis is the most important factor resulting in treatment failure and the leading cause of death in OS patients. The 5-year survival rate for patients with metastasis remains only about 20% despite the use of aggressive surgery and intensive chemotherapy. Approximately 20% of patients present detectable metastasis at their first visit to hospital. However, almost all patients with localized disease are assumed to have micrometastasis already and nearly half of them will progress to clinical metastasis.
Although there have been great advances in the research of OS metastasis in the past few decades, the underlying mechanism is not yet clearly elucidated. Generally, the formation of new blood vessels by endothelial cells is a prerequisite for both primary and metastatic tumor growth. For OS, publications suggested that OS cell could facilitate endothelial cell proliferation (389) and several reagents have been tested to suppress angiogenesis of endothelial cells by directly acting on OS cells (390, 391). Although seemingly inconsistent with tumor angiogenesis, OS cells induce contact-dependent endothelial apoptosis, which may contribute to tumor invasion across vascular barrier during metastasis (29). Therefore, the role of endothelial cells and the interaction between them and OS cells are complex, perhaps playing a dual role, or changing dynamically with the stage and site of OS metastasis.
Lung is the most common site of OS metastasis; however, the underlying mechanism of this apparent organotropism remains to be elucidated. Recently, research found that secreted extracellular vesicles, specifically exosomes, would prepopulate in a particular organ, making it more suitable for tumor metastasis. Metastatic organotropism is one of the prominent characteristics of OS, and more investigations are necessary to reveal the potential mechanisms. In addition, novel therapy targeting those molecules that direct OS cells to lung may yield encouraging outcomes in OS patients. Also, immunotherapy has occupied an important position in the field of tumor therapy. However, no immunotherapy has made a breakthrough in metastatic osteosarcoma so far. The role of immune system in OS development and progression may differ from other tumors. Efforts should be made to explore the unique immune niche of OS metastasis, supporting performance of clinical transformation. In addition to external environmental and genetic factors, physical stimuli and modulation significantly influence OS metastatic process. The interaction of metastatic OS cells with their microenvironment, where they encounter dynamic mechanical forces, definitely affects OS cell invasiveness and to some extent determines the preferred metastatic site. However, it is not fully understood how OS cells specifically respond and adapt to their mechanical surroundings. Besides, the substrate stiffness and fluid flow shear stress may also modify the response of OS to therapy. Thus, the research on the fluid flow and/or substrate pressure can provide a novel consideration for developing anti-metastasis therapy.
Herein, we summarize the key regulatory molecules, signaling pathways, and dysregulated noncoding RNAs during metastatic process and hope to reveal potential druggable targets. The innovative technologies have ushered OS metastasis research into new era, such as multi-omics, liquid biopsy, tissue engineering, bioluminescent imaging, and so on. The OS research community should create biological banks and online databases to store and share precious specimens and relevant research results. Bioinformatics is an effective approach to reanalyze the raw data and explore the most valuable information. Moreover, there is a complex regulatory network between microenvironment, angiogenesis, osteoclast, metabolism, immunity, drug resistance, and OS metastasis. Based on emerging technologies and methods, it is expected to depict this network and expand our understanding of metastasis biology.
The standard drug development platform and evaluation system are necessary for clinical translation of promising drugs specifically in rare diseases like OS. As such, it is essential to establish various cell lines and biological models including animal models and bioengineering models that will facilitate research on metastasis. The models especially canine model that faithfully recapitulate metastasis development as in humans are prerequisites for clinical trials of candidate drugs. In addition, we should pay more attention to the effect of drugs on micrometastasis since some drugs uniquely reduce subclinical lesions (392–394). The novel drug delivery strategy and routes may allow conventional drugs to achieve unexpected efficiency while decreasing systemic toxicity (395–397).
The early diagnosis of metastasis and detection of circulating OS cells or micrometastasis will be the hotspots of future research and lead to breakthrough in improving survival of metastatic patients. A sensitive immunomagnetic detection assay has been successfully used to detect micrometastases from bone marrow and peripheral blood (398, 399). Molecular imaging with specific molecular probes are also attempted to track OS cells, such as CXCR4-targeted near-infrared fluorescence imaging (400) and ssDNA aptamer LP-16 targeting metastatic OS cells (401), which both allow detection of OS microlesions.
Drug therapy may be the only option to completely eliminate micrometastasis or inoperable lesions. The increasing studies have identified more and more biomarkers that are of predictive value for metastasis and prognosis in OS patients. However, individualized comprehensive molecular profiling of OS patients has not significantly changed the therapeutic prospects of advanced osteosarcoma. Therefore, more work is needed to better classify patients and further propel personalized management for specific patients. In short, only overcoming metastasis can effectively improve the survival of patients with osteosarcoma.
Author Contributions
The manuscript was drafted by GS. YG created the diagrams. YY was the guarantor of the entire manuscript for designing and supervising the entire study. HW checked and approved it. All authors contributed to the article and approved the submitted version.
Funding
This study was supported by the National Natural Science Foundation of China (Grant No. 51537004).
Conflict of Interest
The authors declare that the research was conducted in the absence of any commercial or financial relationships that could be construed as a potential conflict of interest.
Publisher’s Note
All claims expressed in this article are solely those of the authors and do not necessarily represent those of their affiliated organizations, or those of the publisher, the editors and the reviewers. Any product that may be evaluated in this article, or claim that may be made by its manufacturer, is not guaranteed or endorsed by the publisher.
References
1. Bielack S, Jurgens H, Jundt G, Kevric M, Kuhne T, Reichardt P, et al. Osteosarcoma: The COSS Experience. Cancer Treat Res (2009) 152:289–308. doi: 10.1007/978-1-4419-0284-9_15
2. Mirabello L, Troisi RJ, Savage SA. Osteosarcoma Incidence and Survival Rates From 1973 to 2004: Data From the Surveillance, Epidemiology, and End Results Program. Cancer (2009) 115(7):1531–43. doi: 10.1002/cncr.24121
3. Whelan J, McTiernan A, Cooper N, Wong YK, Francis M, Vernon S, et al. Incidence and Survival of Malignant Bone Sarcomas in England 1979-2007. Int J Cancer (2012) 131(4):E508–17. doi: 10.1002/ijc.26426
4. Stiller CA, Craft AW, Corazziari I, Group EW. Survival of Children With Bone Sarcoma in Europe Since 1978: Results From the EUROCARE Study. Eur J Cancer (2001) 37(6):760–6. doi: 10.1016/s0959-8049(01)00004-1
5. Bielack SS, Kempf-Bielack B, Delling G, Exner GU, Flege S, Helmke K, et al. Prognostic Factors in High-Grade Osteosarcoma of the Extremities or Trunk: An Analysis of 1,702 Patients Treated on Neoadjuvant Cooperative Osteosarcoma Study Group Protocols. J Clin Oncol (2002) 20(3):776–90. doi: 10.1200/JCO.2002.20.3.776
6. Kager L, Zoubek A, Potschger U, Kastner U, Flege S, Kempf-Bielack B, et al. Primary Metastatic Osteosarcoma: Presentation and Outcome of Patients Treated on Neoadjuvant Cooperative Osteosarcoma Study Group Protocols. J Clin Oncol (2003) 21(10):2011–8. doi: 10.1200/JCO.2003.08.132
7. Mialou V, Philip T, Kalifa C, Perol D, Gentet JC, Marec-Berard P, et al. Metastatic Osteosarcoma at Diagnosis: Prognostic Factors and Long-Term Outcome–the French Pediatric Experience. Cancer (2005) 104(5):1100–9. doi: 10.1002/cncr.21263
8. Kaste SC, Pratt CB, Cain AM, Jones-Wallace DJ, Rao BN. Metastases Detected at the Time of Diagnosis of Primary Pediatric Extremity Osteosarcoma at Diagnosis: Imaging Features. Cancer (1999) 86(8):1602–8. doi: 10.1002/(sici)1097-0142(19991015)86:8<1602::aid-cncr31>3.0.co;2-r
9. Harting MT, Blakely ML. Management of Osteosarcoma Pulmonary Metastases. Semin Pediatr Surg (2006) 15(1):25–9. doi: 10.1053/j.sempedsurg.2005.11.005
10. Bacci G, Rocca M, Salone M, Balladelli A, Ferrari S, Palmerini E, et al. High Grade Osteosarcoma of the Extremities With Lung Metastases at Presentation: Treatment With Neoadjuvant Chemotherapy and Simultaneous Resection of Primary and Metastatic Lesions. J Surg Oncol (2008) 98(6):415–20. doi: 10.1002/jso.21140
11. Isakoff MS, Bielack SS, Meltzer P, Gorlick R. Osteosarcoma: Current Treatment and a Collaborative Pathway to Success. J Clin Oncol (2015) 33(27):3029–35. doi: 10.1200/JCO.2014.59.4895
12. Gorlick R, Anderson P, Andrulis I, Arndt C, Beardsley GP, Bernstein M, et al. Biology of Childhood Osteogenic Sarcoma and Potential Targets for Therapeutic Development: Meeting Summary. Clin Cancer Res (2003) 9(15):5442–53.
13. PosthumaDeBoer J, Witlox MA, Kaspers GJ, van Royen BJ. Molecular Alterations as Target for Therapy in Metastatic Osteosarcoma: A Review of Literature. Clin Exp Metastasis (2011) 28(5):493–503. doi: 10.1007/s10585-011-9384-x
14. Khanna C, Khan J, Nguyen P, Prehn J, Caylor J, Yeung C, et al. Metastasis-Associated Differences in Gene Expression in a Murine Model of Osteosarcoma. Cancer Res (2001) 61(9):3750–9.
15. Hynes RO. Metastatic Potential: Generic Predisposition of the Primary Tumor or Rare, Metastatic Variants-or Both? Cell (2003) 113(7):821–3. doi: 10.1016/s0092-8674(03)00468-9
16. Khanna C, Wan X, Bose S, Cassaday R, Olomu O, Mendoza A, et al. The Membrane-Cytoskeleton Linker Ezrin is Necessary for Osteosarcoma Metastasis. Nat Med (2004) 10(2):182–6. doi: 10.1038/nm982
17. Zucchini C, Rocchi A, Manara MC, De Sanctis P, Capanni C, Bianchini M, et al. Apoptotic Genes as Potential Markers of Metastatic Phenotype in Human Osteosarcoma Cell Lines. Int J Oncol (2008) 32(1):17–31. doi: 10.3892/ijo.32.1.17
18. Weiss L. Metastatic Inefficiency. Adv Cancer Res (1990) 54:159–211. doi: 10.1016/s0065-230x(08)60811-8
19. Cameron MD, Schmidt EE, Kerkvliet N, Nadkarni KV, Morris VL, Groom AC, et al. Temporal Progression of Metastasis in Lung: Cell Survival, Dormancy, and Location Dependence of Metastatic Inefficiency. Cancer Res (2000) 60(9):2541–6.
20. Tsuru A, Setoguchi T, Matsunoshita Y, Nagao-Kitamoto H, Nagano S, Yokouchi M, et al. Hairy/enhancer-Of-Split Related With YRPW Motif Protein 1 Promotes Osteosarcoma Metastasis via Matrix Metallopeptidase 9 Expression. Br J Cancer (2015) 112(7):1232–40. doi: 10.1038/bjc.2015.84
21. Liu JF, Chen PC, Chang TM, Hou CH. Thrombospondin-2 Stimulates MMP-9 Production and Promotes Osteosarcoma Metastasis via the PLC, PKC, C-Src and NF-kappaB Activation. J Cell Mol Med (2020) 24(21):12826–39. doi: 10.1111/jcmm.15874
22. Osaki M, Takeshita F, Sugimoto Y, Kosaka N, Yamamoto Y, Yoshioka Y, et al. MicroRNA-143 Regulates Human Osteosarcoma Metastasis by Regulating Matrix Metalloprotease-13 Expression. Mol Ther (2011) 19(6):1123–30. doi: 10.1038/mt.2011.53
23. Hirahata M, Osaki M, Kanda Y, Sugimoto Y, Yoshioka Y, Kosaka N, et al. PAI-1, a Target Gene of miR-143, Regulates Invasion and Metastasis by Upregulating MMP-13 Expression of Human Osteosarcoma. Cancer Med (2016) 5(5):892–902. doi: 10.1002/cam4.651
24. Ingvarsen SZ, Gardsvoll H, van Putten S, Norregaard KS, Krigslund O, Meilstrup JA, et al. Tumor Cell MT1-MMP is Dispensable for Osteosarcoma Tumor Growth, Bone Degradation and Lung Metastasis. Sci Rep (2020) 10(1):19138. doi: 10.1038/s41598-020-75995-6
25. Zhang M, Zhang J, Zhou Q. Elevated Expression of microRNA-328-3p Suppresses Aggressive Malignant Behaviors via Targeting Matrix Metalloprotease 16 in Osteosarcoma. Onco Targets Ther (2019) 12:2063–70. doi: 10.2147/OTT.S195022
26. Kane SE, Gottesman MM. The Role of Cathepsin L in Malignant Transformation. Semin Cancer Biol (1990) 1(2):127–36.
27. Han XG, Li Y, Mo HM, Li K, Lin D, Zhao CQ, et al. TIMP3 Regulates Osteosarcoma Cell Migration, Invasion, and Chemotherapeutic Resistances. Tumour Biol (2016) 37(7):8857–67. doi: 10.1007/s13277-015-4757-4
28. Cheng HL, Hsieh MJ, Yang JS, Lin CW, Lue KH, Lu KH, et al. Nobiletin Inhibits Human Osteosarcoma Cells Metastasis by Blocking ERK and JNK-Mediated MMPs Expression. Oncotarget (2016) 7(23):35208–23. doi: 10.18632/oncotarget.9106
29. McEwen A, Emmanuel C, Medbury H, Leick A, Walker DM, Zoellner H. Induction of Contact-Dependent Endothelial Apoptosis by Osteosarcoma Cells Suggests a Role for Endothelial Cell Apoptosis in Blood-Borne Metastasis. J Pathol (2003) 201(3):395–403. doi: 10.1002/path.1457
30. Bian ZY, Fan QM, Li G, Xu WT, Tang TT. Human Mesenchymal Stem Cells Promote Growth of Osteosarcoma: Involvement of Interleukin-6 in the Interaction Between Human Mesenchymal Stem Cells and Saos-2. Cancer Sci (2010) 101(12):2554–60. doi: 10.1111/j.1349-7006.2010.01731.x
31. Xu WT, Bian ZY, Fan QM, Li G, Tang TT. Human Mesenchymal Stem Cells (hMSCs) Target Osteosarcoma and Promote Its Growth and Pulmonary Metastasis. Cancer Lett (2009) 281(1):32–41. doi: 10.1016/j.canlet.2009.02.022
32. Kawano M, Tanaka K, Itonaga I, Iwasaki T, Miyazaki M, Ikeda S, et al. Dendritic Cells Combined With Anti-GITR Antibody Produce Antitumor Effects in Osteosarcoma. Oncol Rep (2015) 34(4):1995–2001. doi: 10.3892/or.2015.4161
33. Kawano M, Itonaga I, Iwasaki T, Tsumura H. Enhancement of Antitumor Immunity by Combining Anti-Cytotoxic T Lymphocyte Antigen-4 Antibodies and Cryotreated Tumor Lysate-Pulsed Dendritic Cells in Murine Osteosarcoma. Oncol Rep (2013) 29(3):1001–6. doi: 10.3892/or.2013.2224
34. Kawano M, Itonaga I, Iwasaki T, Tsuchiya H, Tsumura H. Anti-TGF-Beta Antibody Combined With Dendritic Cells Produce Antitumor Effects in Osteosarcoma. Clin Orthop Relat Res (2012) 470(8):2288–94. doi: 10.1007/s11999-012-2299-2
35. Fernandez L, Valentin J, Zalacain M, Leung W, Patino-Garcia A, Perez-Martinez A. Activated and Expanded Natural Killer Cells Target Osteosarcoma Tumor Initiating Cells in an NKG2D-NKG2DL Dependent Manner. Cancer Lett (2015) 368(1):54–63. doi: 10.1016/j.canlet.2015.07.042
36. Norregaard KS, Jurgensen HJ, Gardsvoll H, Engelholm LH, Behrendt N, Soe K. Osteosarcoma and Metastasis Associated Bone Degradation-A Tale of Osteoclast and Malignant Cell Cooperativity. Int J Mol Sci 22(13):6865. doi: 10.3390/ijms22136865
37. Tan K, Goldstein D, Crowe P, Yang JL. Uncovering a Key to the Process of Metastasis in Human Cancers: A Review of Critical Regulators of Anoikis. J Cancer Res Clin Oncol (2013) 139(11):1795–805. doi: 10.1007/s00432-013-1482-5
38. Wirtz D, Konstantopoulos K, Searson PC. The Physics of Cancer: The Role of Physical Interactions and Mechanical Forces in Metastasis. Nat Rev Cancer (2011) 11(7):512–22. doi: 10.1038/nrc3080
39. Follain G, Herrmann D, Harlepp S, Hyenne V, Osmani N, Warren SC, et al. Fluids and Their Mechanics in Tumour Transit: Shaping Metastasis. Nat Rev Cancer (2020) 20(2):107–24. doi: 10.1038/s41568-019-0221-x
40. Matsubara T, Diresta GR, Kakunaga S, Li D, Healey JH. Additive Influence of Extracellular Ph, Oxygen Tension, and Pressure on Invasiveness and Survival of Human Osteosarcoma Cells. Front Oncol (2013) 3199:199. doi: 10.3389/fonc.2013.00199
41. Coughlin TR, Sana A, Voss K, Gadi A, Basu-Roy U, Curtin CM, et al. The Effect of Fluid Flow Shear Stress and Substrate Stiffness on Yes-Associated Protein (YAP) Activity and Osteogenesis in Murine Osteosarcoma Cells. Cancers (Basel) (2021) 13(13):3128. doi: 10.3390/cancers13133128
42. Zachos TA, Aiken SW, DiResta GR, Healey JH. Interstitial Fluid Pressure and Blood Flow in Canine Osteosarcoma and Other Tumors. Clin Orthop Relat Res (2001) 385):230–6. doi: 10.1097/00003086-200104000-00034
43. Nathan SS, DiResta GR, Casas-Ganem JE, Hoang BH, Sowers R, Yang R, et al. Elevated Physiologic Tumor Pressure Promotes Proliferation and Chemosensitivity in Human Osteosarcoma. Clin Cancer Res (2005) 11(6):2389–97. doi: 10.1158/1078-0432.CCR-04-2048
44. Fidler IJ. Metastasis: Quantitative Analysis of Distribution and Fate of Tumor Emboli Labeled With 125 I-5-Iodo-2'-Deoxyuridine. J Natl Cancer Inst (1970) 45(4):773–82.
45. Holmgren L, O'Reilly MS, Folkman J. Dormancy of Micrometastases: Balanced Proliferation and Apoptosis in the Presence of Angiogenesis Suppression. Nat Med (1995) 1(2):149–53. doi: 10.1038/nm0295-149
46. Almog N, Ma L, Raychowdhury R, Schwager C, Erber R, Short S, et al. Transcriptional Switch of Dormant Tumors to Fast-Growing Angiogenic Phenotype. Cancer Res (2009) 69(3):836–44. doi: 10.1158/0008-5472.CAN-08-2590
47. Szegezdi E, Logue SE, Gorman AM, Samali A. Mediators of Endoplasmic Reticulum Stress-Induced Apoptosis. EMBO Rep (2006) 7(9):880–5. doi: 10.1038/sj.embor.7400779
48. Yan M, Ni J, Song D, Ding M, Huang J. Activation of Unfolded Protein Response Protects Osteosarcoma Cells From Cisplatin-Induced Apoptosis Through NF-kappaB Pathway. Int J Clin Exp Pathol (2015) 8(9):10204–15.
49. Chaiyawat P, Sungngam P, Teeyakasem P, Sirikaew N, Klangjorhor J, Settakorn J, et al. Protein Profiling of Osteosarcoma Tissue and Soft Callus Unveils Activation of the Unfolded Protein Response Pathway. Int J Oncol (2019) 54(5):1704–18. doi: 10.3892/ijo.2019.4737
50. Lizardo MM, Morrow JJ, Miller TE, Hong ES, Ren L, Mendoza A, et al. Upregulation of Glucose-Regulated Protein 78 in Metastatic Cancer Cells Is Necessary for Lung Metastasis Progression. Neoplasia (2016) 18(11):699–710. doi: 10.1016/j.neo.2016.09.001
51. Yarapureddy S, Abril J, Foote J, Kumar S, Asad O, Sharath V, et al. ATF6alpha Activation Enhances Survival Against Chemotherapy and Serves as a Prognostic Indicator in Osteosarcoma. Neoplasia (2019) 21(6):516–32. doi: 10.1016/j.neo.2019.02.004
52. Chambers AF, Groom AC, MacDonald IC. Dissemination and Growth of Cancer Cells in Metastatic Sites. Nat Rev Cancer (2002) 2(8):563–72. doi: 10.1038/nrc865
53. Weibel ER. Lung Morphometry: The Link Between Structure and Function. Cell Tissue Res (2017) 367(3):413–26. doi: 10.1007/s00441-016-2541-4
54. Liu Y, Cao X. Characteristics and Significance of the Pre-Metastatic Niche. Cancer Cell (2016) 30(5):668–81. doi: 10.1016/j.ccell.2016.09.011
55. Hood JL, San RS, Wickline SA. Exosomes Released by Melanoma Cells Prepare Sentinel Lymph Nodes for Tumor Metastasis. Cancer Res (2011) 71(11):3792–801. doi: 10.1158/0008-5472.CAN-10-4455
56. Zhang H, Deng T, Liu R, Bai M, Zhou L, Wang X, et al. Exosome-Delivered EGFR Regulates Liver Microenvironment to Promote Gastric Cancer Liver Metastasis. Nat Commun (2017) 8:15016. doi: 10.1038/ncomms15016
57. Costa-Silva B, Aiello NM, Ocean AJ, Singh S, Zhang H, Thakur BK, et al. Pancreatic Cancer Exosomes Initiate Pre-Metastatic Niche Formation in the Liver. Nat Cell Biol (2015) 17(6):816–26. doi: 10.1038/ncb3169
58. Zhou CF, Ma J, Huang L, Yi HY, Zhang YM, Wu XG, et al. Cervical Squamous Cell Carcinoma-Secreted Exosomal miR-221-3p Promotes Lymphangiogenesis and Lymphatic Metastasis by Targeting VASH1. Oncogene (2019) 38(8):1256–68. doi: 10.1038/s41388-018-0511-x
59. Guo Y, Ji X, Liu J, Fan D, Zhou Q, Chen C, et al. Effects of Exosomes on Pre-Metastatic Niche Formation in Tumors. Mol Cancer (2019) 18(1):39. doi: 10.1186/s12943-019-0995-1
60. Wortzel I, Dror S, Kenific CM, Lyden D. Exosome-Mediated Metastasis: Communication From a Distance. Dev Cell (2019) 49(3):347–60. doi: 10.1016/j.devcel.2019.04.011
61. Chicon-Bosch M, Tirado OM. Exosomes in Bone Sarcomas: Key Players in Metastasis. Cells 9(1):241. doi: 10.3390/cells9010241
62. Hoshino A, Costa-Silva B, Shen TL, Rodrigues G, Hashimoto A, Tesic Mark M, et al. Tumour Exosome Integrins Determine Organotropic Metastasis. Nature (2015) 527(7578):329–35. doi: 10.1038/nature15756
63. Wang J, Zhang H, Sun X, Wang X, Ren T, Huang Y, et al. Exosomal PD-L1 and N-Cadherin Predict Pulmonary Metastasis Progression for Osteosarcoma Patients. J Nanobiotechnol (2020) 18(1):151. doi: 10.1186/s12951-020-00710-6
64. Zhang H, Wang J, Ren T, Huang Y, Yu Y, Chen C, et al. LncRNA CASC15 is Upregulated in Osteosarcoma Plasma Exosomes and CASC15 Knockdown Inhibits Osteosarcoma Progression by Regulating miR-338-3p/RAB14 Axis. Onco Targets Ther (2020) 13:12055–66. doi: 10.2147/OTT.S282053
65. Araki Y, Aiba H, Yoshida T, Yamamoto N, Hayashi K, Takeuchi A, et al. Osteosarcoma-Derived Small Extracellular Vesicles Enhance Tumor Metastasis and Suppress Osteoclastogenesis by miR-146a-5p. Front Oncol (2021) 11:667109. doi: 10.3389/fonc.2021.667109
66. Wang L, Wu J, Song S, Chen H, Hu Y, Xu B, et al. Plasma Exosome-Derived Sentrin SUMO-Specific Protease 1: A Prognostic Biomarker in Patients With Osteosarcoma. Front Oncol (2021) 11:625109. doi: 10.3389/fonc.2021.625109
67. Macklin R, Wang H, Loo D, Martin S, Cumming A, Cai N, et al. Extracellular Vesicles Secreted by Highly Metastatic Clonal Variants of Osteosarcoma Preferentially Localize to the Lungs and Induce Metastatic Behaviour in Poorly Metastatic Clones. Oncotarget (2016) 7(28):43570–87. doi: 10.18632/oncotarget.9781
68. Fan TM, Roberts RD, Lizardo MM. Understanding and Modeling Metastasis Biology to Improve Therapeutic Strategies for Combating Osteosarcoma Progression. Front Oncol (2020) 10:13. doi: 10.3389/fonc.2020.00013
69. Ren L, Hong SH, Chen QR, Briggs J, Cassavaugh J, Srinivasan S, et al. Dysregulation of Ezrin Phosphorylation Prevents Metastasis and Alters Cellular Metabolism in Osteosarcoma. Cancer Res (2012) 72(4):1001–12. doi: 10.1158/0008-5472.CAN-11-0210
70. Han G, Wang Y, Bi W, Jia J, Wang W, Xu M. Effects of Vascular Endothelial Growth Factor Expression on Pathological Characteristics and Prognosis of Osteosarcoma. Clin Exp Med (2016) 16(4):577–84. doi: 10.1007/s10238-015-0382-1
71. Jia SF, Guan H, Duan X, Kleinerman ES. VEGF165 is Necessary to the Metastatic Potential of Fas(-) Osteosarcoma Cells But Will Not Rescue the Fas(+) Cells. J Exp Ther Oncol (2008) 7(2):89–97.
72. Wan X, Kim SY, Guenther LM, Mendoza A, Briggs J, Yeung C, et al. Beta4 Integrin Promotes Osteosarcoma Metastasis and Interacts With Ezrin. Oncogene (2009) 28(38):3401–11. doi: 10.1038/onc.2009.206
73. Gao YS, Mei J, Tong TL, Hu M, Xue HM, Cai XS. Inhibitory Effects of VEGF-siRNA Mediated by Adenovirus on Osteosarcoma-Bearing Nude Mice. Cancer Biother Radiopharm (2009) 24(2):243–7. doi: 10.1089/cbr.2008.0544
74. Ren L, Hong SH, Cassavaugh J, Osborne T, Chou AJ, Kim SY, et al. The Actin-Cytoskeleton Linker Protein Ezrin is Regulated During Osteosarcoma Metastasis by PKC. Oncogene (2009) 28(6):792–802. doi: 10.1038/onc.2008.437
75. Folkman J. Role of Angiogenesis in Tumor Growth and Metastasis. Semin Oncol (2002) 29(6 Suppl 16):15–8. doi: 10.1053/sonc.2002.37263
76. Lafleur EA, Koshkina NV, Stewart J, Jia SF, Worth LL, Duan X, et al. Increased Fas Expression Reduces the Metastatic Potential of Human Osteosarcoma Cells. Clin Cancer Res (2004) 10(23):8114–9. doi: 10.1158/1078-0432.CCR-04-0353
77. Ek ET, Dass CR, Contreras KG, Choong PF. Pigment Epithelium-Derived Factor Overexpression Inhibits Orthotopic Osteosarcoma Growth, Angiogenesis and Metastasis. Cancer Gene Ther (2007) 14(7):616–26. doi: 10.1038/sj.cgt.7701044
78. Gordon N, Kleinerman ES. Aerosol Therapy for the Treatment of Osteosarcoma Lung Metastases: Targeting the Fas/FasL Pathway and Rationale for the Use of Gemcitabine. J Aerosol Med Pulm Drug Deliv (2010) 23(4):189–96. doi: 10.1089/jamp.2009.0812
79. Ek ET, Dass CR, Contreras KG, Choong PF. Inhibition of Orthotopic Osteosarcoma Growth and Metastasis by Multitargeted Antitumor Activities of Pigment Epithelium-Derived Factor. Clin Exp Metastasis (2007) 24(2):93–106. doi: 10.1007/s10585-007-9062-1
80. Dhupkar P, Gordon N, Stewart J, Kleinerman ES. Anti-PD-1 Therapy Redirects Macrophages From an M2 to an M1 Phenotype Inducing Regression of OS Lung Metastases. Cancer Med (2018) 7(6):2654–64. doi: 10.1002/cam4.1518
81. Ek ET, Dass CR, Contreras KG, Choong PF. PEDF-Derived Synthetic Peptides Exhibit Antitumor Activity in an Orthotopic Model of Human Osteosarcoma. J Orthop Res (2007) 25(12):1671–80. doi: 10.1002/jor.20434
82. Lussier DM, O'Neill L, Nieves LM, McAfee MS, Holechek SA, Collins AW, et al. Enhanced T-Cell Immunity to Osteosarcoma Through Antibody Blockade of PD-1/PD-L1 Interactions. J Immunother (2015) 38(3):96–106. doi: 10.1097/CJI.0000000000000065
83. Tang Q, Lu J, Zou C, Shao Y, Chen Y, Narala S, et al. CDH4 is a Novel Determinant of Osteosarcoma Tumorigenesis and Metastasis. Oncogene (2018) 37(27):3617–30. doi: 10.1038/s41388-018-0231-2
84. Gvozdenovic A, Arlt MJ, Campanile C, Brennecke P, Husmann K, Li Y, et al. CD44 Enhances Tumor Formation and Lung Metastasis in Experimental Osteosarcoma and is an Additional Predictor for Poor Patient Outcome. J Bone Miner Res (2013) 28(4):838–47. doi: 10.1002/jbmr.1817
85. Dass CR, Khachigian LM, Choong PF. C-Jun Is Critical for the Progression of Osteosarcoma: Proof in an Orthotopic Spontaneously Metastasizing Model. Mol Cancer Res (2008) 6(8):1289–92. doi: 10.1158/1541-7786.MCR-08-0036
86. Gvozdenovic A, Arlt MJ, Campanile C, Brennecke P, Husmann K, Born W, et al. Silencing of CD44 Gene Expression in Human 143-B Osteosarcoma Cells Promotes Metastasis of Intratibial Tumors in SCID Mice. PloS One (2013) 8(4):e60329. doi: 10.1371/journal.pone.0060329
87. Dass CR, Khachigian LM, Choong PF. C-Jun Knockdown Sensitizes Osteosarcoma to Doxorubicin. Mol Cancer Ther (2008) 7(7):1909–12. doi: 10.1158/1535-7163.MCT-08-0086
88. Xu JF, Pan XH, Zhang SJ, Zhao C, Qiu BS, Gu HF, et al. CD47 Blockade Inhibits Tumor Progression Human Osteosarcoma in Xenograft Models. Oncotarget (2015) 6(27):23662–70. doi: 10.18632/oncotarget.4282
89. Dass CR, Friedhuber AM, Khachigian LM, Dunstan DE, Choong PF. Downregulation of C-Jun Results in Apoptosis-Mediated Anti-Osteosarcoma Activity in an Orthotopic Model. Cancer Biol Ther (2008) 7(7):1033–6. doi: 10.4161/cbt.7.7.6037
90. Manara MC, Bernard G, Lollini PL, Nanni P, Zuntini M, Landuzzi L, et al. CD99 Acts as an Oncosuppressor in Osteosarcoma. Mol Biol Cell (2006) 17(4):1910–21. doi: 10.1091/mbc.e05-10-0971
91. Sabile AA, Arlt MJ, Muff R, Bode B, Langsam B, Bertz J, et al. Cyr61 Expression in Osteosarcoma Indicates Poor Prognosis and Promotes Intratibial Growth and Lung Metastasis in Mice. J Bone Miner Res (2012) 27(1):58–67. doi: 10.1002/jbmr.535
92. Adhikari AS, Agarwal N, Wood BM, Porretta C, Ruiz B, Pochampally RR, et al. CD117 and Stro-1 Identify Osteosarcoma Tumor-Initiating Cells Associated With Metastasis and Drug Resistance. Cancer Res (2010) 70(11):4602–12. doi: 10.1158/0008-5472.CAN-09-3463
93. Habel N, Vilalta M, Bawa O, Opolon P, Blanco J, Fromigue O. Cyr61 Silencing Reduces Vascularization and Dissemination of Osteosarcoma Tumors. Oncogene (2015) 34(24):3207–13. doi: 10.1038/onc.2014.232
94. He A, Yang X, Huang Y, Feng T, Wang Y, Sun Y, et al. CD133(+) CD44(+) Cells Mediate in the Lung Metastasis of Osteosarcoma. J Cell Biochem (2015) 116(8):1719–29. doi: 10.1002/jcb.25131
95. Habel N, Stefanovska B, Carene D, Patino-Garcia A, Lecanda F, Fromigue O. CYR61 Triggers Osteosarcoma Metastatic Spreading via an IGF1Rbeta-Dependent EMT-Like Process. BMC Cancer (2019) 19(1):62. doi: 10.1186/s12885-019-5282-4
96. Zhang Y, Ma Q, Liu T, Guan G, Zhang K, Chen J, et al. Interleukin-6 Suppression Reduces Tumour Self-Seeding by Circulating Tumour Cells in a Human Osteosarcoma Nude Mouse Model. Oncotarget (2016) 7(1):446–58. doi: 10.18632/oncotarget.6371
97. Tu B, Du L, Fan QM, Tang Z, Tang TT. STAT3 Activation by IL-6 From Mesenchymal Stem Cells Promotes the Proliferation and Metastasis of Osteosarcoma. Cancer Lett (2012) 325(1):80–8. doi: 10.1016/j.canlet.2012.06.006
98. Kularatne SA, Deshmukh V, Ma J, Tardif V, Lim RK, Pugh HM, et al. A CXCR4-Targeted Site-Specific Antibody-Drug Conjugate. Angew Chem Int Ed Engl (2014) 53(44):11863–7. doi: 10.1002/anie.201408103
99. Zhang Z, Wang F, Li Q, Zhang H, Cui Y, Ma C, et al. CD151 Knockdown Inhibits Osteosarcoma Metastasis Through the GSK-3beta/Beta-Catenin/MMP9 Pathway. Oncol Rep (2016) 35(3):1764–70. doi: 10.3892/or.2015.4517
100. Neklyudova O, Arlt MJ, Brennecke P, Thelen M, Gvozdenovic A, Kuzmanov A, et al. Altered CXCL12 Expression Reveals a Dual Role of CXCR4 in Osteosarcoma Primary Tumor Growth and Metastasis. J Cancer Res Clin Oncol (2016) 142(8):1739–50. doi: 10.1007/s00432-016-2185-5
101. Itoh H, Kadomatsu T, Tanoue H, Yugami M, Miyata K, Endo M, et al. TET2-Dependent IL-6 Induction Mediated by the Tumor Microenvironment Promotes Tumor Metastasis in Osteosarcoma. Oncogene (2018) 37(22):2903–20. doi: 10.1038/s41388-018-0160-0
102. Dang H, Wu W, Wang B, Cui C, Niu J, Chen J, et al. CXCL5 Plays a Promoting Role in Osteosarcoma Cell Migration and Invasion in Autocrine- and Paracrine-Dependent Manners. Oncol Res (2017) 25(2):177–86. doi: 10.3727/096504016X14732772150343
103. Wang M, Wang L, Ren T, Xu L, Wen Z. IL-17a/IL-17RA Interaction Promoted Metastasis of Osteosarcoma Cells. Cancer Biol Ther (2013) 14(2):155–63. doi: 10.4161/cbt.22955
104. Du L, Han XG, Tu B, Wang MQ, Qiao H, Zhang SH, et al. CXCR1/Akt Signaling Activation Induced by Mesenchymal Stem Cell-Derived IL-8 Promotes Osteosarcoma Cell Anoikis Resistance and Pulmonary Metastasis. Cell Death Dis (2018) 9(7):714. doi: 10.1038/s41419-018-0745-0
105. Segaliny AI, Mohamadi A, Dizier B, Lokajczyk A, Brion R, Lanel R, et al. Interleukin-34 Promotes Tumor Progression and Metastatic Process in Osteosarcoma Through Induction of Angiogenesis and Macrophage Recruitment. Int J Cancer (2015) 137(1):73–85. doi: 10.1002/ijc.29376
106. Brennecke P, Arlt MJ, Campanile C, Husmann K, Gvozdenovic A, Apuzzo T, et al. CXCR4 Antibody Treatment Suppresses Metastatic Spread to the Lung of Intratibial Human Osteosarcoma Xenografts in Mice. Clin Exp Metastasis (2014) 31(3):339–49. doi: 10.1007/s10585-013-9632-3
107. Akiyama T, Choong PF, Dass CR. RANK-Fc Inhibits Malignancy via Inhibiting ERK Activation and Evoking Caspase-3-Mediated Anoikis in Human Osteosarcoma Cells. Clin Exp Metastasis (2010) 27(4):207–15. doi: 10.1007/s10585-010-9319-y
108. Zhang P, Dong L, Yan K, Long H, Yang TT, Dong MQ, et al. CXCR4-Mediated Osteosarcoma Growth and Pulmonary Metastasis is Promoted by Mesenchymal Stem Cells Through VEGF. Oncol Rep (2013) 30(4):1753–61. doi: 10.3892/or.2013.2619
109. Akiyama T, Dass CR, Shinoda Y, Kawano H, Tanaka S, Choong PF. Systemic RANK-Fc Protein Therapy is Efficacious Against Primary Osteosarcoma Growth in a Murine Model via Activity Against Osteoclasts. J Pharm Pharmacol (2010) 62(4):470–6. doi: 10.1211/jpp.62.04.0009
110. Goguet-Surmenian E, Richard-Fiardo P, Guillemot E, Benchetrit M, Gomez-Brouchet A, Buzzo P, et al. CXCR7-Mediated Progression of Osteosarcoma in the Lungs. Br J Cancer (2013) 109(6):1579–85. doi: 10.1038/bjc.2013.482
111. Lamoureux F, Picarda G, Rousseau J, Gourden C, Battaglia S, Charrier C, et al. Therapeutic Efficacy of Soluble Receptor Activator of Nuclear Factor-Kappa B-Fc Delivered by Nonviral Gene Transfer in a Mouse Model of Osteolytic Osteosarcoma. Mol Cancer Ther (2008) 7(10):3389–98. doi: 10.1158/1535-7163.MCT-08-0497
112. de Nigris F, Rossiello R, Schiano C, Arra C, Williams-Ignarro S, Barbieri A, et al. Deletion of Yin Yang 1 Protein in Osteosarcoma Cells on Cell Invasion and CXCR4/angiogenesis and Metastasis. Cancer Res (2008) 68(6):1797–808. doi: 10.1158/0008-5472.CAN-07-5582
113. Picarda G, Lamoureux F, Geffroy L, Delepine P, Montier T, Laud K, et al. Preclinical Evidence That Use of TRAIL in Ewing's Sarcoma and Osteosarcoma Therapy Inhibits Tumor Growth, Prevents Osteolysis, and Increases Animal Survival. Clin Cancer Res (2010) 16(8):2363–74. doi: 10.1158/1078-0432.CCR-09-1779
114. Gozo MC, Jia D, Aspuria PJ, Cheon DJ, Miura N, Walts AE, et al. FOXC2 Augments Tumor Propagation and Metastasis in Osteosarcoma. Oncotarget (2016) 7(42):68792–802. doi: 10.18632/oncotarget.11990
115. Cao Y, Zhou Z, de Crombrugghe B, Nakashima K, Guan H, Duan X, et al. Osterix, a Transcription Factor for Osteoblast Differentiation, Mediates Antitumor Activity in Murine Osteosarcoma. Cancer Res (2005) 65(4):1124–8. doi: 10.1158/0008-5472.CAN-04-2128
116. Brennecke P, Arlt MJ, Muff R, Campanile C, Gvozdenovic A, Husmann K, et al. Expression of the Chemokine Receptor CXCR7 in CXCR4-Expressing Human 143B Osteosarcoma Cells Enhances Lung Metastasis of Intratibial Xenografts in SCID Mice. PloS One (2013) 8(9):e74045. doi: 10.1371/journal.pone.0074045
117. Lamora A, Talbot J, Bougras G, Amiaud J, Leduc M, Chesneau J, et al. Overexpression of Smad7 Blocks Primary Tumor Growth and Lung Metastasis Development in Osteosarcoma. Clin Cancer Res (2014) 20(19):5097–112. doi: 10.1158/1078-0432.CCR-13-3191
118. Perissinotto E, Cavalloni G, Leone F, Fonsato V, Mitola S, Grignani G, et al. Involvement of Chemokine Receptor 4/Stromal Cell-Derived Factor 1 System During Osteosarcoma Tumor Progression. Clin Cancer Res (2005) 11(2 Pt 1):490–7.
119. Endo-Munoz L, Cumming A, Rickwood D, Wilson D, Cueva C, Ng C, et al. Loss of Osteoclasts Contributes to Development of Osteosarcoma Pulmonary Metastases. Cancer Res (2010) 70(18):7063–72. doi: 10.1158/0008-5472.CAN-09-4291
120. Rodriguez Calleja L, Jacques C, Lamoureux F, Baud'huin M, Tellez Gabriel M, Quillard T, et al. DeltaNp63alpha Silences a miRNA Program to Aberrantly Initiate a Wound-Healing Program That Promotes TGFbeta-Induced Metastasis. Cancer Res (2016) 76(11):3236–51. doi: 10.1158/0008-5472.CAN-15-2317
121. Gross AC, Cam H, Phelps DA, Saraf AJ, Bid HK, Cam M, et al. IL-6 and CXCL8 Mediate Osteosarcoma-Lung Interactions Critical to Metastasis. JCI Insight 3(16):e99791. doi: 10.1172/jci.insight.99791
122. Gvozdenovic A, Boro A, Meier D, Bode-Lesniewska B, Born W, Muff R, et al. Targeting Alphavbeta3 and Alphavbeta5 Integrins Inhibits Pulmonary Metastasis in an Intratibial Xenograft Osteosarcoma Mouse Model. Oncotarget (2016) 7(34):55141–54. doi: 10.18632/oncotarget.10461
123. Bid HK, Roberts RD, Cam M, Audino A, Kurmasheva RT, Lin J, et al. DeltaNp63 Promotes Pediatric Neuroblastoma and Osteosarcoma by Regulating Tumor Angiogenesis. Cancer Res (2014) 74(1):320–9. doi: 10.1158/0008-5472.CAN-13-0894
124. Li R, Shi Y, Zhao S, Shi T, Zhang G. NF-kappaB Signaling and Integrin-Beta1 Inhibition Attenuates Osteosarcoma Metastasis via Increased Cell Apoptosis. Int J Biol Macromol (2019) 123:1035–43. doi: 10.1016/j.ijbiomac.2018.11.003
125. Li Y, Liao Q, Li K, Zhong D, Weng X, Mi M. Knockdown of Endothelin A Receptor Expression Inhibits Osteosarcoma Pulmonary Metastasis in an Orthotopic Xenograft Mouse Model. Mol Med Rep (2012) 5(6):1391–5. doi: 10.3892/mmr.2012.842
126. Pourebrahim R, Zhang Y, Liu B, Gao R, Xiong S, Lin PP, et al. Integrative Genome Analysis of Somatic P53 Mutant Osteosarcomas Identifies Ets2-Dependent Regulation of Small Nucleolar RNAs by Mutant P53 Protein. Genes Dev (2017) 31(18):1847–57. doi: 10.1101/gad.304972.117
127. Zhang D, Jiang F, Wang X, Li G. Knockdown of SALL4 Inhibits Proliferation, Migration, and Invasion in Osteosarcoma Cells. Oncol Res (2017) 25(5):763–71. doi: 10.3727/096504016X14772402056137
128. Zhang Y, Hu Q, Li G, Li L, Liang S, Zhang Y, et al. ONZIN Upregulation by Mutant P53 Contributes to Osteosarcoma Metastasis Through the CXCL5-MAPK Signaling Pathway. Cell Physiol Biochem (2018) 48(3):1099–111. doi: 10.1159/000491976
129. Yong BC, Lu JC, Xie XB, Su Q, Tan PX, Tang QL, et al. LDOC1 Regulates Wnt5a Expression and Osteosarcoma Cell Metastasis and is Correlated With the Survival of Osteosarcoma Patients. Tumour Biol (2017) 39(2):1010428317691188. doi: 10.1177/1010428317691188
130. Luther GA, Lamplot J, Chen X, Rames R, Wagner ER, Liu X, et al. IGFBP5 Domains Exert Distinct Inhibitory Effects on the Tumorigenicity and Metastasis of Human Osteosarcoma. Cancer Lett (2013) 336(1):222–30. doi: 10.1016/j.canlet.2013.05.002
131. Wang J, Ni J, Song D, Ding M, Huang J, Li W, et al. MAT1 Facilitates the Lung Metastasis of Osteosarcoma Through Upregulation of AKT1 Expression. Life Sci (2019) 234:116771. doi: 10.1016/j.lfs.2019.116771
132. Su Y, Wagner ER, Luo Q, Huang J, Chen L, He BC, et al. Insulin-Like Growth Factor Binding Protein 5 Suppresses Tumor Growth and Metastasis of Human Osteosarcoma. Oncogene (2011) 30(37):3907–17. doi: 10.1038/onc.2011.97
133. Zeng H, Zhang JM, Du Y, Wang J, Ren Y, Li M, et al. Crosstalk Between ATF4 and MTA1/HDAC1 Promotes Osteosarcoma Progression. Oncotarget (2016) 7(6):7329–42. doi: 10.18632/oncotarget.6940
134. Wang S, Zhang D, Han S, Gao P, Liu C, Li J, et al. Fibulin-3 Promotes Osteosarcoma Invasion and Metastasis by Inducing Epithelial to Mesenchymal Transition and Activating the Wnt/beta-Catenin Signaling Pathway. Sci Rep (2017) 7(1):6215. doi: 10.1038/s41598-017-06353-2
135. Lu J, Song G, Tang Q, Zou C, Han F, Zhao Z, et al. IRX1 Hypomethylation Promotes Osteosarcoma Metastasis via Induction of CXCL14/NF-kappaB Signaling. J Clin Invest (2015) 125(5):1839–56. doi: 10.1172/JCI78437
136. Zhang D, Wang S, Chen J, Liu H, Lu J, Jiang H, et al. Fibulin-4 Promotes Osteosarcoma Invasion and Metastasis by Inducing Epithelial to Mesenchymal Transition via the PI3K/Akt/mTOR Pathway. Int J Oncol (2017) 50(5):1513–30. doi: 10.3892/ijo.2017.3921
137. Manara MC, Baldini N, Serra M, Lollini PL, De Giovanni C, Vaccari M, et al. Reversal of Malignant Phenotype in Human Osteosarcoma Cells Transduced With the Alkaline Phosphatase Gene. Bone (2000) 26(3):215–20. doi: 10.1016/s8756-3282(99)00266-5
138. Zhang G, Li M, Jin J, Bai Y, Yang C. Knockdown of S100A4 Decreases Tumorigenesis and Metastasis in Osteosarcoma Cells by Repression of Matrix Metalloproteinase-9. Asian Pac J Cancer Prev (2011) 12(8):2075–80.
139. Li YJ, Dong BK, Fan M, Jiang WX. BTG2 Inhibits the Proliferation and Metastasis of Osteosarcoma Cells by Suppressing the PI3K/AKT Pathway. Int J Clin Exp Pathol (2015) 8(10):12410–8.
140. Fujiwara M, Kashima TG, Kunita A, Kii I, Komura D, Grigoriadis AE, et al. Stable Knockdown of S100A4 Suppresses Cell Migration and Metastasis of Osteosarcoma. Tumour Biol (2011) 32(3):611–22. doi: 10.1007/s13277-011-0160-y
141. Chen MW, Wu XJ. SLC25A22 Promotes Proliferation and Metastasis of Osteosarcoma Cells via the PTEN Signaling Pathway. Technol Cancer Res Treat (2018) 17:1533033818811143. doi: 10.1177/1533033818811143
142. Qin Y, Ye J, Zhao F, Hu S, Wang S. TRIM2 Regulates the Development and Metastasis of Tumorous Cells of Osteosarcoma. Int J Oncol (2018) 53(4):1643–56. doi: 10.3892/ijo.2018.4494
143. Chien MH, Lee WJ, Yang YC, Tan P, Pan KF, Liu YC, et al. N-Alpha-Acetyltransferase 10 Protein Promotes Metastasis by Stabilizing Matrix Metalloproteinase-2 Protein in Human Osteosarcomas. Cancer Lett (2018) 433:86–98. doi: 10.1016/j.canlet.2018.06.033
144. Chen Y, Guo Y, Yang H, Shi G, Xu G, Shi J, et al. TRIM66 Overexpresssion Contributes to Osteosarcoma Carcinogenesis and Indicates Poor Survival Outcome. Oncotarget (2015) 6(27):23708–19. doi: 10.18632/oncotarget.4291
145. Li Y, Nakka M, Kelly AJ, Lau CC, Krailo M, Barkauskas DA, et al. P27 Is a Candidate Prognostic Biomarker and Metastatic Promoter in Osteosarcoma. Cancer Res (2016) 76(13):4002–11. doi: 10.1158/0008-5472.CAN-15-3189
146. Cao J, Wang Y, Dong R, Lin G, Zhang N, Wang J, et al. Hypoxia-Induced WSB1 Promotes the Metastatic Potential of Osteosarcoma Cells. Cancer Res (2015) 75(22):4839–51. doi: 10.1158/0008-5472.CAN-15-0711
147. Endo-Munoz L, Cai N, Cumming A, Macklin R, Merida de Long L, Topkas E, et al. Progression of Osteosarcoma From a Non-Metastatic to a Metastatic Phenotype Is Causally Associated With Activation of an Autocrine and Paracrine uPA Axis. PloS One (2015) 10(8):e0133592. doi: 10.1371/journal.pone.0133592
148. Fukaya Y, Ishiguro N, Senga T, Ichigotani Y, Sohara Y, Tsutsui M, et al. A Role for PI3K-Akt Signaling in Pulmonary Metastatic Nodule Formation of the Osteosarcoma Cell Line, LM8. Oncol Rep (2005) 14(4):847–52. doi: 10.3892/or.14.4.847
149. Shi QM, Luo J, Wu K, Yin M, Gu YR, Cheng XG. High Level of alphaB-Crystallin Contributes to the Progression of Osteosarcoma. Oncotarget (2016) 7(8):9007–16. doi: 10.18632/oncotarget.6928
150. Liu JF, Tsao YT, Hou CH. Amphiregulin Enhances Intercellular Adhesion Molecule-1 Expression and Promotes Tumor Metastasis in Human Osteosarcoma. Oncotarget (2015) 6(38):40880–95. doi: 10.18632/oncotarget.5679
151. Lv YF, Yan GN, Meng G, Zhang X, Guo QN. Enhancer of Zeste Homolog 2 Silencing Inhibits Tumor Growth and Lung Metastasis in Osteosarcoma. Sci Rep (2015) 5:12999. doi: 10.1038/srep12999
152. Guo YS, Zhao R, Ma J, Cui W, Sun Z, Gao B, et al. Betaig-H3 Promotes Human Osteosarcoma Cells Metastasis by Interacting With Integrin Alpha2beta1 and Activating PI3K Signaling Pathway. PloS One (2014) 9(3):e90220. doi: 10.1371/journal.pone.0090220
153. Baglio SR, Lagerweij T, Perez-Lanzon M, Ho XD, Leveille N, Melo SA, et al. Blocking Tumor-Educated MSC Paracrine Activity Halts Osteosarcoma Progression. Clin Cancer Res (2017) 23(14):3721–33. doi: 10.1158/1078-0432.CCR-16-2726
154. Ren K, Zhang J, Gu X, Wu S, Shi X, Ni Y, et al. Migration-Inducing Gene-7 Independently Predicts Poor Prognosis of Human Osteosarcoma and Is Associated With Vasculogenic Mimicry. Exp Cell Res (2018) 369(1):80–9. doi: 10.1016/j.yexcr.2018.05.008
155. Liu J, Luo B, Zhao M. Bmi1targeting Suppresses Osteosarcoma Aggressiveness Through the NFkappaB Signaling Pathway. Mol Med Rep (2017) 16(6):7949–58. doi: 10.3892/mmr.2017.7660
156. Han W, Liu J. Epigenetic Silencing of the Wnt Antagonist APCDD1 by Promoter DNA Hyper-Methylation Contributes to Osteosarcoma Cell Invasion and Metastasis. Biochem Biophys Res Commun (2017) 491(1):91–7. doi: 10.1016/j.bbrc.2017.07.049
157. El-Naggar AM, Clarkson PW, Negri GL, Turgu B, Zhang F, Anglesio MS, et al. HACE1 is a Potential Tumor Suppressor in Osteosarcoma. Cell Death Dis (2019) 10(1):21. doi: 10.1038/s41419-018-1276-4
158. Yue B, Ren QX, Su T, Wang LN, Zhang L. ERK5 Silencing Inhibits Invasion of Human Osteosarcoma Cell via Modulating the Slug/MMP-9 Pathway. Eur Rev Med Pharmacol Sci (2014) 18(18):2640–7.
159. Sun W, Wang W, Lei J, Li H, Wu Y. Actin-Like Protein 6A is a Novel Prognostic Indicator Promoting Invasion and Metastasis in Osteosarcoma. Oncol Rep (2017) 37(4):2405–17. doi: 10.3892/or.2017.5473
160. Contaldo C, Myers TJ, Zucchini C, Manara MC, Chiodoni C, Colombo MP, et al. Expression Levels of Insulin Receptor Substrate-1 Modulate the Osteoblastic Differentiation of Mesenchymal Stem Cells and Osteosarcoma Cells. Growth Factors (2014) 32(1):41–52. doi: 10.3109/08977194.2013.870168
161. Zhao Z, Wu MS, Zou C, Tang Q, Lu J, Liu D, et al. Downregulation of MCT1 Inhibits Tumor Growth, Metastasis and Enhances Chemotherapeutic Efficacy in Osteosarcoma Through Regulation of the NF-kappaB Pathway. Cancer Lett (2014) 342(1):150–8. doi: 10.1016/j.canlet.2013.08.042
162. Levings PP, McGarry SV, Currie TP, Nickerson DM, McClellan S, Ghivizzani SC, et al. Expression of an Exogenous Human Oct-4 Promoter Identifies Tumor-Initiating Cells in Osteosarcoma. Cancer Res (2009) 69(14):5648–55. doi: 10.1158/0008-5472.CAN-08-3580
163. Wang Z, Cao CJ, Huang LL, Ke ZF, Luo CJ, Lin ZW, et al. EFEMP1 Promotes the Migration and Invasion of Osteosarcoma via MMP-2 With Induction by AEG-1 via NF-kappaB Signaling Pathway. Oncotarget (2015) 6(16):14191–208. doi: 10.18632/oncotarget.3691
164. Arlt MJ, Kuzmanov A, Snedeker JG, Fuchs B, Silvan U, Sabile AA. Fascin-1 Enhances Experimental Osteosarcoma Tumor Formation and Metastasis and is Related to Poor Patient Outcome. BMC Cancer (2019) 19(1):83. doi: 10.1186/s12885-019-5303-3
165. Zandueta C, Ormazabal C, Perurena N, Martinez-Canarias S, Zalacain M, Julian MS, et al. Matrix-Gla Protein Promotes Osteosarcoma Lung Metastasis and Associates With Poor Prognosis. J Pathol (2016) 239(4):438–49. doi: 10.1002/path.4740
166. Long F, Cai X, Luo W, Chen L, Li K. Role of Aldolase A in Osteosarcoma Progression and Metastasis: In Vitro and In Vivo Evidence. Oncol Rep (2014) 32(5):2031–7. doi: 10.3892/or.2014.3473
167. Xu L, Xia C, Sheng F, Sun Q, Xiong J, Wang S. CEP55 Promotes the Proliferation and Invasion of Tumour Cells via the AKT Signalling Pathway in Osteosarcoma. Carcinogenesis (2018) 39(4):623–31. doi: 10.1093/carcin/bgy017
168. Ma C, Zhang Z, Cui Y, Yuan H, Wang F. Silencing FAT10 Inhibits Metastasis of Osteosarcoma. Int J Oncol (2016) 49(2):666–74. doi: 10.3892/ijo.2016.3549
169. Ren L, Mendoza A, Zhu J, Briggs JW, Halsey C, Hong ES, et al. Characterization of the Metastatic Phenotype of a Panel of Established Osteosarcoma Cells. Oncotarget (2015) 6(30):29469–81. doi: 10.18632/oncotarget.5177
170. Niinaka Y, Harada K, Fujimuro M, Oda M, Haga A, Hosoki M, et al. Silencing of Autocrine Motility Factor Induces Mesenchymal-to-Epithelial Transition and Suppression of Osteosarcoma Pulmonary Metastasis. Cancer Res (2010) 70(22):9483–93. doi: 10.1158/0008-5472.CAN-09-3880
171. Yu W, Zhang Z, Min D, Yang Q, Du X, Tang L, et al. Mediator of RNA Polymerase II Transcription Subunit 19 Promotes Osteosarcoma Growth and Metastasis and Associates With Prognosis. Eur J Cancer (2014) 50(6):1125–36. doi: 10.1016/j.ejca.2014.01.030
172. Zhang Y, Cheng H, Li W, Wu H, Yang Y. Highly-Expressed P2X7 Receptor Promotes Growth and Metastasis of Human HOS/MNNG Osteosarcoma Cells via PI3K/Akt/GSK3beta/beta-Catenin and mTOR/HIF1alpha/VEGF Signaling. Int J Cancer (2019) 145(4):1068–82. doi: 10.1002/ijc.32207
173. Hou CH, Yang RS, Tsao YT. Connective Tissue Growth Factor Stimulates Osteosarcoma Cell Migration and Induces Osteosarcoma Metastasis by Upregulating VCAM-1 Expression. Biochem Pharmacol (2018) 155:71–81. doi: 10.1016/j.bcp.2018.06.015
174. Zhao SJ, Jiang YQ, Xu NW, Li Q, Zhang Q, Wang SY, et al. SPARCL1 Suppresses Osteosarcoma Metastasis and Recruits Macrophages by Activation of Canonical WNT/beta-Catenin Signaling Through Stabilization of the WNT-Receptor Complex. Oncogene (2018) 37(8):1049–61. doi: 10.1038/onc.2017.403
175. McManus M, Kleinerman E, Yang Y, Livingston JA, Mortus J, Rivera R, et al. Hes4: A Potential Prognostic Biomarker for Newly Diagnosed Patients With High-Grade Osteosarcoma. Pediatr Blood Cancer (2017) 64(5):10. doi: 10.1002/pbc.26318
176. Dai H, Lv YF, Yan GN, Meng G, Zhang X, Guo QN. RanBP9/TSSC3 Complex Cooperates to Suppress Anoikis Resistance and Metastasis via Inhibiting Src-Mediated Akt Signaling in Osteosarcoma. Cell Death Dis (2016) 7(12):e2572. doi: 10.1038/cddis.2016.436
177. Zhang P, Yang Y, Zweidler-McKay PA, Hughes DP. Critical Role of Notch Signaling in Osteosarcoma Invasion and Metastasis. Clin Cancer Res (2008) 14(10):2962–9. doi: 10.1158/1078-0432.CCR-07-1992
178. Brun J, Dieudonne FX, Marty C, Muller J, Schule R, Patino-Garcia A, et al. FHL2 Silencing Reduces Wnt Signaling and Osteosarcoma Tumorigenesis In Vitro and In Vivo. PloS One (2013) 8(1):e55034. doi: 10.1371/journal.pone.0055034
179. Hughes DP. How the NOTCH Pathway Contributes to the Ability of Osteosarcoma Cells to Metastasize. Cancer Treat Res (2009) 152:479–96. doi: 10.1007/978-1-4419-0284-9_28
180. Cheng Q, Yin G. Cullin-1 Regulates MG63 Cell Proliferation and Metastasis and Is a Novel Prognostic Marker of Osteosarcoma. Int J Biol Markers (2017) 32(2):e202–9. doi: 10.5301/jbm.5000247
181. Zhang Y, Zvi YS, Batko B, Zaphiros N, O'Donnell EF, Wang J, et al. Down-Regulation of Skp2 Expression Inhibits Invasion and Lung Metastasis in Osteosarcoma. Sci Rep (2018) 8(1):14294. doi: 10.1038/s41598-018-32428-9
182. Morrow JJ, Mendoza A, Koyen A, Lizardo MM, Ren L, Waybright TJ, et al. mTOR Inhibition Mitigates Enhanced mRNA Translation Associated With the Metastatic Phenotype of Osteosarcoma Cells In Vivo. Clin Cancer Res (2016) 22(24):6129–41. doi: 10.1158/1078-0432.CCR-16-0326
183. Zhang N, Xie T, Xian M, Wang YJ, Li HY, Ying MD, et al. SIRT1 Promotes Metastasis of Human Osteosarcoma Cells. Oncotarget (2016) 7(48):79654–69. doi: 10.18632/oncotarget.12916
184. Zhang F, Yan T, Guo W, Sun K, Wang S, Bao X, et al. Novel Oncogene COPS3 Interacts With Beclin1 and Raf-1 to Regulate Metastasis of Osteosarcoma Through Autophagy. J Exp Clin Cancer Res (2018) 37(1):135. doi: 10.1186/s13046-018-0791-6
185. Lv YF, Dai H, Yan GN, Meng G, Zhang X, Guo QN. Downregulation of Tumor Suppressing STF cDNA 3 Promotes Epithelial-Mesenchymal Transition and Tumor Metastasis of Osteosarcoma by the Wnt/GSK-3beta/Beta-Catenin/Snail Signaling Pathway. Cancer Lett (2016) 373(2):164–73. doi: 10.1016/j.canlet.2016.01.046
186. Techavichit P, Gao Y, Kurenbekova L, Shuck R, Donehower LA, Yustein JT. Secreted Frizzled-Related Protein 2 (Sfrp2) Promotes Osteosarcoma Invasion and Metastatic Potential. BMC Cancer (2016) 16(1):869. doi: 10.1186/s12885-016-2909-6
187. Zhao GS, Gao ZR, Zhang Q, Tang XF, Lv YF, Zhang ZS, et al. TSSC3 Promotes Autophagy via Inactivating the Src-Mediated PI3K/Akt/mTOR Pathway to Suppress Tumorigenesis and Metastasis in Osteosarcoma, and Predicts a Favorable Prognosis. J Exp Clin Cancer Res (2018) 37(1):188. doi: 10.1186/s13046-018-0856-6
188. Ma J, Gao W, Gao J. sCLU as Prognostic Biomarker and Therapeutic Target in Osteosarcoma. Bioengineered (2019) 10(1):229–39. doi: 10.1080/21655979.2019.1621136
189. Jin PY, Lu HJ, Tang Y, Fan SH, Zhang ZF, Wang Y, et al. The Effect of DNA-PKcs Gene Silencing on Proliferation, Migration, Invasion and Apoptosis, and In Vivo Tumorigenicity of Human Osteosarcoma MG-63 Cells. BioMed Pharmacother (2017) 96:1324–34. doi: 10.1016/j.biopha.2017.11.079
190. Hou CH, Lin FL, Tong KB, Hou SM, Liu JF. Transforming Growth Factor Alpha Promotes Osteosarcoma Metastasis by ICAM-1 and PI3K/Akt Signaling Pathway. Biochem Pharmacol (2014) 89(4):453–63. doi: 10.1016/j.bcp.2014.03.010
191. Tieken C, Verboom MC, Ruf W, Gelderblom H, Bovee JV, Reitsma PH, et al. Tissue Factor Associates With Survival and Regulates Tumour Progression in Osteosarcoma. Thromb Haemost (2016) 115(5):1025–33. doi: 10.1160/TH15-07-0541
192. Chang H, Dong T, Ma X, Zhang T, Chen Z, Yang Z, et al. Spondin 1 Promotes Metastatic Progression Through Fak and Src Dependent Pathway in Human Osteosarcoma. Biochem Biophys Res Commun (2015) 464(1):45–50. doi: 10.1016/j.bbrc.2015.05.092
193. Yu L, Liu S, Guo W, Zhang B, Liang Y, Feng Q. Upregulation of Mad2 Facilitates In Vivo and In Vitro Osteosarcoma Progression. Oncol Rep (2012) 28(6):2170–6. doi: 10.3892/or.2012.2032
194. El-Naggar AM, Veinotte CJ, Cheng H, Grunewald TG, Negri GL, Somasekharan SP, et al. Translational Activation of HIF1alpha by YB-1 Promotes Sarcoma Metastasis. Cancer Cell (2015) 27(5):682–97. doi: 10.1016/j.ccell.2015.04.003
195. Rubin EM, Guo Y, Tu K, Xie J, Zi X, Hoang BH. Wnt Inhibitory Factor 1 Decreases Tumorigenesis and Metastasis in Osteosarcoma. Mol Cancer Ther (2010) 9(3):731–41. doi: 10.1158/1535-7163.MCT-09-0147
196. Hu X, Liu Y, Qin C, Pan Z, Luo J, Yu A, et al. Up-Regulated Isocitrate Dehydrogenase 1 Suppresses Proliferation, Migration and Invasion in Osteosarcoma: In Vitro and In Vivo. Cancer Lett (2014) 346(1):114–21. doi: 10.1016/j.canlet.2013.12.020
197. Cantiani L, Manara MC, Zucchini C, De Sanctis P, Zuntini M, Valvassori L, et al. Caveolin-1 Reduces Osteosarcoma Metastases by Inhibiting C-Src Activity and Met Signaling. Cancer Res (2007) 67(16):7675–85. doi: 10.1158/0008-5472.CAN-06-4697
198. Severe N, Dieudonne FX, Marty C, Modrowski D, Patino-Garcia A, Lecanda F, et al. Targeting the E3 Ubiquitin Casitas B-Lineage Lymphoma Decreases Osteosarcoma Cell Growth and Survival and Reduces Tumorigenesis. J Bone Miner Res (2012) 27(10):2108–17. doi: 10.1002/jbmr.1667
199. Zhang W, Zhao JM, Lin J, Hu CZ, Zhang WB, Yang WL, et al. Adaptive Fibrogenic Reprogramming of Osteosarcoma Stem Cells Promotes Metastatic Growth. Cell Rep (2018) 24(5):1266–1277 e5. doi: 10.1016/j.celrep.2018.06.103
200. Koshkina NV, Khanna C, Mendoza A, Guan H, DeLauter L, Kleinerman ES. Fas-Negative Osteosarcoma Tumor Cells Are Selected During Metastasis to the Lungs: The Role of the Fas Pathway in the Metastatic Process of Osteosarcoma. Mol Cancer Res (2007) 5(10):991–9. doi: 10.1158/1541-7786.MCR-07-0007
201. Gordon N, Kleinerman ES. The Role of Fas/FasL in the Metastatic Potential of Osteosarcoma and Targeting This Pathway for the Treatment of Osteosarcoma Lung Metastases. Cancer Treat Res (2009) 152:497–508. doi: 10.1007/978-1-4419-0284-9_29
202. Huang G, Nishimoto K, Yang Y, Kleinerman ES. Participation of the Fas/FasL Signaling Pathway and the Lung Microenvironment in the Development of Osteosarcoma Lung Metastases. Adv Exp Med Biol (2014) 804:203–17. doi: 10.1007/978-3-319-04843-7_11
203. Gordon N, Koshkina NV, Jia SF, Khanna C, Mendoza A, Worth LL, et al. Corruption of the Fas Pathway Delays the Pulmonary Clearance of Murine Osteosarcoma Cells, Enhances Their Metastatic Potential, and Reduces the Effect of Aerosol Gemcitabine. Clin Cancer Res (2007) 13(15 Pt 1):4503–10. doi: 10.1158/1078-0432.CCR-07-0313
204. Koshkina N, Yang Y, Kleinerman ES. The Fas/FasL Signaling Pathway: Its Role in the Metastatic Process and as a Target for Treating Osteosarcoma Lung Metastases. Adv Exp Med Biol (2020) 1258:177–87. doi: 10.1007/978-3-030-43085-6_12
205. Rodriguez CO Jr., Crabbs TA, Wilson DW, Cannan VA, Skorupski KA, Gordon N, et al. Aerosol Gemcitabine: Preclinical Safety and In Vivo Antitumor Activity in Osteosarcoma-Bearing Dogs. J Aerosol Med Pulm Drug Deliv (2010) 23(4):197–206. doi: 10.1089/jamp.2009.0773
206. Ruivo CF, Adem B, Silva M, Melo SA. The Biology of Cancer Exosomes: Insights and New Perspectives. Cancer Res (2017) 77(23):6480–8. doi: 10.1158/0008-5472.CAN-17-0994
207. Bao Q, Gong L, Wang J, Wen J, Shen Y, Zhang W. Extracellular Vesicle RNA Sequencing Reveals Dramatic Transcriptomic Alterations Between Metastatic and Primary Osteosarcoma in a Liquid Biopsy Approach. Ann Surg Oncol (2018) 25(9):2642–51. doi: 10.1245/s10434-018-6642-z
208. Jerez S, Araya H, Thaler R, Charlesworth MC, Lopez-Solis R, Kalergis AM, et al. Proteomic Analysis of Exosomes and Exosome-Free Conditioned Media From Human Osteosarcoma Cell Lines Reveals Secretion of Proteins Related to Tumor Progression. J Cell Biochem (2017) 118(2):351–60. doi: 10.1002/jcb.25642
209. Kok VC, Yu CC. Cancer-Derived Exosomes: Their Role in Cancer Biology and Biomarker Development. Int J Nanomed (2020) 15:8019–36. doi: 10.2147/IJN.S272378
210. Zhao W, Qin P, Zhang D, Cui X, Gao J, Yu Z, et al. Long non-Coding RNA PVT1 Encapsulated in Bone Marrow Mesenchymal Stem Cell-Derived Exosomes Promotes Osteosarcoma Growth and Metastasis by Stabilizing ERG and Sponging miR-183-5p. Aging (Albany NY) (2019) 11(21):9581–96. doi: 10.18632/aging.102406
211. Wang Y, Chu Y, Li K, Zhang G, Guo Z, Wu X, et al. Exosomes Secreted by Adipose-Derived Mesenchymal Stem Cells Foster Metastasis and Osteosarcoma Proliferation by Increasing COLGALT2 Expression. Front Cell Dev Biol (2020) 8:353. doi: 10.3389/fcell.2020.00353
212. Liu W, Long Q, Zhang W, Zeng D, Hu B, Liu S, et al. miRNA-221-3p Derived From M2-Polarized Tumor-Associated Macrophage Exosomes Aggravates the Growth and Metastasis of Osteosarcoma Through SOCS3/JAK2/STAT3 Axis. Aging (Albany NY) (2021) 13(15):19760–75. doi: 10.18632/aging.203388
213. Zhang H, Yu Y, Wang J, Han Y, Ren T, Huang Y, et al. Macrophages-Derived Exosomal lncRNA LIFR-AS1 Promotes Osteosarcoma Cell Progression via miR-29a/NFIA Axis. Cancer Cell Int (2021) 21(1):192. doi: 10.1186/s12935-021-01893-0
214. Balkwill F. Cancer and the Chemokine Network. Nat Rev Cancer (2004) 4(7):540–50. doi: 10.1038/nrc1388
215. Li B, Wang Z, Wu H, Xue M, Lin P, Wang S, et al. Epigenetic Regulation of CXCL12 Plays a Critical Role in Mediating Tumor Progression and the Immune Response In Osteosarcoma. Cancer Res (2018) 78(14):3938–53. doi: 10.1158/0008-5472.CAN-17-3801
216. Benslimane-Ahmim Z, Pereira J, Lokajczyk A, Dizier B, Galy-Fauroux I, Fischer AM, et al. Osteoprotegerin Regulates Cancer Cell Migration Through SDF-1/CXCR4 Axis and Promotes Tumour Development by Increasing Neovascularization. Cancer Lett (2017) 395:11–9. doi: 10.1016/j.canlet.2017.02.032
217. Liao YX, Fu ZZ, Zhou CH, Shan LC, Wang ZY, Yin F, et al. AMD3100 Reduces CXCR4-Mediated Survival and Metastasis of Osteosarcoma by Inhibiting JNK and Akt, But Not P38 or Erk1/2, Pathways in In Vitro and Mouse Experiments. Oncol Rep (2015) 34(1):33–42. doi: 10.3892/or.2015.3992
218. Georges S, Chesneau J, Hervouet S, Taurelle J, Gouin F, Redini F, et al. A Disintegrin And Metalloproteinase 12 Produced by Tumour Cells Accelerates Osteosarcoma Tumour Progression and Associated Osteolysis. Eur J Cancer (2013) 49(9):2253–63. doi: 10.1016/j.ejca.2013.02.020
219. Ory B, Baud'huin M, Verrecchia F, Royer BB, Quillard T, Amiaud J, et al. Blocking HSP90 Addiction Inhibits Tumor Cell Proliferation, Metastasis Development, and Synergistically Acts With Zoledronic Acid to Delay Osteosarcoma Progression. Clin Cancer Res (2016) 22(10):2520–33. doi: 10.1158/1078-0432.CCR-15-1925
220. Akiyama T, Dass CR, Choong PF. Novel Therapeutic Strategy for Osteosarcoma Targeting Osteoclast Differentiation, Bone-Resorbing Activity, and Apoptosis Pathway. Mol Cancer Ther (2008) 7(11):3461–9. doi: 10.1158/1535-7163.MCT-08-0530
221. Costa-Rodrigues J, Fernandes A, Fernandes MH. Reciprocal Osteoblastic and Osteoclastic Modulation in Co-Cultured MG63 Osteosarcoma Cells and Human Osteoclast Precursors. J Cell Biochem (2011) 112(12):3704–13. doi: 10.1002/jcb.23295
222. Saharinen P, Eklund L, Pulkki K, Bono P, Alitalo K. VEGF and Angiopoietin Signaling in Tumor Angiogenesis and Metastasis. Trends Mol Med (2011) 17(7):347–62. doi: 10.1016/j.molmed.2011.01.015
223. Zetter BR. Angiogenesis and Tumor Metastasis. Annu Rev Med (1998) 49:407–24. doi: 10.1146/annurev.med.49.1.407
224. Wang X, Hu Z, Wang Z, Cui Y, Cui X. Angiopoietin-Like Protein 2 is an Important Facilitator of Tumor Proliferation, Metastasis, Angiogenesis and Glycolysis in Osteosarcoma. Am J Transl Res (2019) 11(10):6341–55.
225. Wang G, Sun M, Jiang Y, Zhang T, Sun W, Wang H, et al. Anlotinib, a Novel Small Molecular Tyrosine Kinase Inhibitor, Suppresses Growth and Metastasis via Dual Blockade of VEGFR2 and MET in Osteosarcoma. Int J Cancer (2019) 145(4):979–93. doi: 10.1002/ijc.32180
226. Broadhead ML, Choong PF, Dass CR. Efficacy of Continuously Administered PEDF-Derived Synthetic Peptides Against Osteosarcoma Growth and Metastasis. J BioMed Biotechnol (2012) 2012:230298. doi: 10.1155/2012/230298
227. Xie L, Ji T, Guo W. Anti-Angiogenesis Target Therapy for Advanced Osteosarcoma (Review). Oncol Rep (2017) 38(2):625–36. doi: 10.3892/or.2017.5735
228. Liu Y, Huang N, Liao S, Rothzerg E, Yao F, Li Y, et al. Current Research Progress in Targeted Anti-Angiogenesis Therapy for Osteosarcoma. Cell Prolif (2021) 54(9):e13102. doi: 10.1111/cpr.13102
229. Lehuede C, Dupuy F, Rabinovitch R, Jones RG, Siegel PM. Metabolic Plasticity as a Determinant of Tumor Growth and Metastasis. Cancer Res (2016) 76(18):5201–8. doi: 10.1158/0008-5472.CAN-16-0266
230. Giang AH, Raymond T, Brookes P, de Mesy Bentley K, Schwarz E, O'Keefe R, et al. Mitochondrial Dysfunction and Permeability Transition in Osteosarcoma Cells Showing the Warburg Effect. J Biol Chem (2013) 288(46):33303–11. doi: 10.1074/jbc.M113.507129
231. Liberti MV, Locasale JW. The Warburg Effect: How Does it Benefit Cancer Cells? Trends Biochem Sci (2016) 41(3):211–8. doi: 10.1016/j.tibs.2015.12.001
232. Hua Y, Qiu Y, Zhao A, Wang X, Chen T, Zhang Z, et al. Dynamic Metabolic Transformation in Tumor Invasion and Metastasis in Mice With LM-8 Osteosarcoma Cell Transplantation. J Proteome Res (2011) 10(8):3513–21. doi: 10.1021/pr200147g
233. Roy J, Dibaeinia P, Fan TM, Sinha S, Das A. Global Analysis of Osteosarcoma Lipidomes Reveal Altered Lipid Profiles in Metastatic Versus Nonmetastatic Cells. J Lipid Res (2019) 60(2):375–87. doi: 10.1194/jlr.M088559
234. Kort WJ, Hulsmann WC, Stehman TE. Modulation of Metastatic Ability by Inhibition of Cholesterol Synthesis. Clin Exp Metastasis (1989) 7(5):517–23. doi: 10.1007/BF01753812
235. Luo X, Cheng C, Tan Z, Li N, Tang M, Yang L, et al. Emerging Roles of Lipid Metabolism in Cancer Metastasis. Mol Cancer (2017) 16(1):76. doi: 10.1186/s12943-017-0646-3
236. Ren L, Hong ES, Mendoza A, Issaq S, Tran Hoang C, Lizardo M, et al. Metabolomics Uncovers a Link Between Inositol Metabolism and Osteosarcoma Metastasis. Oncotarget (2017) 8(24):38541–53. doi: 10.18632/oncotarget.15872
237. Zhao H, Wu Y, Chen Y, Liu H. Clinical Significance of Hypoxia-Inducible Factor 1 and VEGF-A in Osteosarcoma. Int J Clin Oncol (2015) 20(6):1233–43. doi: 10.1007/s10147-015-0848-x
238. Guan G, Zhang Y, Lu Y, Liu L, Shi D, Wen Y, et al. The HIF-1alpha/CXCR4 Pathway Supports Hypoxia-Induced Metastasis of Human Osteosarcoma Cells. Cancer Lett (2015) 357(1):254–64. doi: 10.1016/j.canlet.2014.11.034
239. Kunz M, Moeller S, Koczan D, Lorenz P, Wenger RH, Glocker MO, et al. Mechanisms of Hypoxic Gene Regulation of Angiogenesis Factor Cyr61 in Melanoma Cells. J Biol Chem (2003) 278(46):45651–60. doi: 10.1074/jbc.M301373200
240. Liapis V, Labrinidis A, Zinonos I, Hay S, Ponomarev V, Panagopoulos V, et al. Hypoxia-Activated Pro-Drug TH-302 Exhibits Potent Tumor Suppressive Activity and Cooperates With Chemotherapy Against Osteosarcoma. Cancer Lett (2015) 357(1):160–9. doi: 10.1016/j.canlet.2014.11.020
241. Abu-Remaileh M, Aqeilan RI. Tumor Suppressor WWOX Regulates Glucose Metabolism via HIF1alpha Modulation. Cell Death Differ (2014) 21(11):1805–14. doi: 10.1038/cdd.2014.95
242. Gaudio E, Palamarchuk A, Palumbo T, Trapasso F, Pekarsky Y, Croce CM, et al. Physical Association With WWOX Suppresses C-Jun Transcriptional Activity. Cancer Res (2006) 66(24):11585–9. doi: 10.1158/0008-5472.CAN-06-3376
243. Del Mare S, Aqeilan RI. Tumor Suppressor WWOX Inhibits Osteosarcoma Metastasis by Modulating RUNX2 Function. Sci Rep 5:12959. doi: 10.1038/srep12959
244. Ozanne BW, Spence HJ, McGarry LC, Hennigan RF. Transcription Factors Control Invasion: AP-1 the First Among Equals. Oncogene (2007) 26(1):1–10. doi: 10.1038/sj.onc.1209759
245. Leaner VD, Chick JF, Donninger H, Linniola I, Mendoza A, Khanna C, et al. Inhibition of AP-1 Transcriptional Activity Blocks the Migration, Invasion, and Experimental Metastasis of Murine Osteosarcoma. Am J Pathol (2009) 174(1):265–75. doi: 10.2353/ajpath.2009.071006
246. Kunita A, Kashima TG, Ohazama A, Grigoriadis AE, Fukayama M. Podoplanin Is Regulated by AP-1 and Promotes Platelet Aggregation and Cell Migration in Osteosarcoma. Am J Pathol (2011) 179(2):1041–9. doi: 10.1016/j.ajpath.2011.04.027
247. Weekes D, Kashima TG, Zandueta C, Perurena N, Thomas DP, Sunters A, et al. Regulation of Osteosarcoma Cell Lung Metastasis by the C-Fos/AP-1 Target FGFR1. Oncogene (2016) 35(22):2852–61. doi: 10.1038/onc.2015.344
248. Chano T, Avnet S, Kusuzaki K, Bonuccelli G, Sonveaux P, Rotili D, et al. Tumour-Specific Metabolic Adaptation to Acidosis is Coupled to Epigenetic Stability in Osteosarcoma Cells. Am J Cancer Res (2016) 6(4):859–75.
249. Avnet S, Di Pompo G, Chano T, Errani C, Ibrahim-Hashim A, Gillies RJ, et al. Cancer-Associated Mesenchymal Stroma Fosters the Stemness of Osteosarcoma Cells in Response to Intratumoral Acidosis via NF-kappaB Activation. Int J Cancer (2017) 140(6):1331–45. doi: 10.1002/ijc.30540
250. Avnet S, Chano T, Massa A, Bonuccelli G, Lemma S, Falzetti L, et al. Acid Microenvironment Promotes Cell Survival of Human Bone Sarcoma Through the Activation of cIAP Proteins and NF-kappaB Pathway. Am J Cancer Res (2019) 9(6):1127–44.
251. Orrenius S, Gogvadze V, Zhivotovsky B. Mitochondrial Oxidative Stress: Implications for Cell Death. Annu Rev Pharmacol Toxicol (2007) 47:143–83. doi: 10.1146/annurev.pharmtox.47.120505.105122
252. Ott M, Gogvadze V, Orrenius S, Zhivotovsky B. Mitochondria, Oxidative Stress and Cell Death. Apoptosis (2007) 12(5):913–22. doi: 10.1007/s10495-007-0756-2
253. Qiu H, Orr FW, Jensen D, Wang HH, McIntosh AR, Hasinoff BB, et al. Arrest of B16 Melanoma Cells in the Mouse Pulmonary Microcirculation Induces Endothelial Nitric Oxide Synthase-Dependent Nitric Oxide Release That Is Cytotoxic to the Tumor Cells. Am J Pathol (2003) 162(2):403–12. doi: 10.1016/S0002-9440(10)63835-7
254. Poderoso JJ, Helfenberger K, Poderoso C. The Effect of Nitric Oxide on Mitochondrial Respiration. Nitric Oxide (2019) 88:61–72. doi: 10.1016/j.niox.2019.04.005
255. Ridnour LA, Thomas DD, Mancardi D, Espey MG, Miranda KM, Paolocci N, et al. The Chemistry of Nitrosative Stress Induced by Nitric Oxide and Reactive Nitrogen Oxide Species. Putting Perspective on Stressful Biological Situations. Biol Chem (2004) 385(1):1–10. doi: 10.1515/BC.2004.001
256. Winterbourn CC. Revisiting the Reactions of Superoxide With Glutathione and Other Thiols. Arch Biochem Biophys (2016) 595:68–71. doi: 10.1016/j.abb.2015.11.028
257. Buddingh EP, Kuijjer ML, Duim RA, Burger H, Agelopoulos K, Myklebost O, et al. Tumor-Infiltrating Macrophages are Associated With Metastasis Suppression in High-Grade Osteosarcoma: A Rationale for Treatment With Macrophage Activating Agents. Clin Cancer Res (2011) 17(8):2110–9. doi: 10.1158/1078-0432.CCR-10-2047
258. Dumars C, Ngyuen JM, Gaultier A, Lanel R, Corradini N, Gouin F, et al. Dysregulation of Macrophage Polarization is Associated With the Metastatic Process in Osteosarcoma. Oncotarget (2016) 7(48):78343–54. doi: 10.18632/oncotarget.13055
259. Han Q, Shi H, Liu F. CD163(+) M2-Type Tumor-Associated Macrophage Support the Suppression of Tumor-Infiltrating T Cells in Osteosarcoma. Int Immunopharmacol (2016) 34:101–6. doi: 10.1016/j.intimp.2016.01.023
260. Hingorani P, Maas ML, Gustafson MP, Dickman P, Adams RH, Watanabe M, et al. Increased CTLA-4(+) T Cells and an Increased Ratio of Monocytes With Loss of Class II (CD14(+) HLA-DR(lo/neg)) Found in Aggressive Pediatric Sarcoma Patients. J Immunother Cancer 3:35. doi: 10.1186/s40425-015-0082-0
261. Gomez-Brouchet A, Illac C, Gilhodes J, Bouvier C, Aubert S, Guinebretiere JM, et al. CD163-Positive Tumor-Associated Macrophages and CD8-Positive Cytotoxic Lymphocytes are Powerful Diagnostic Markers for the Therapeutic Stratification of Osteosarcoma Patients: An Immunohistochemical Analysis of the Biopsies Fromthe French OS2006 Phase 3 Trial. Oncoimmunology (2017) 6(9):e1331193. doi: 10.1080/2162402X.2017.1331193
262. Pahl JH, Kwappenberg KM, Varypataki EM, Santos SJ, Kuijjer ML, Mohamed S, et al. Macrophages Inhibit Human Osteosarcoma Cell Growth After Activation With the Bacterial Cell Wall Derivative Liposomal Muramyl Tripeptide in Combination With Interferon-Gamma. J Exp Clin Cancer Res (2014) 33:27. doi: 10.1186/1756-9966-33-27
263. Shao XJ, Xiang SF, Chen YQ, Zhang N, Cao J, Zhu H, et al. Inhibition of M2-Like Macrophages by All-Trans Retinoic Acid Prevents Cancer Initiation and Stemness in Osteosarcoma Cells. Acta Pharmacol Sin (2019) 40(10):1343–50. doi: 10.1038/s41401-019-0262-4
264. Zhou Q, Xian M, Xiang S, Xiang D, Shao X, Wang J, et al. All-Trans Retinoic Acid Prevents Osteosarcoma Metastasis by Inhibiting M2 Polarization of Tumor-Associated Macrophages. Cancer Immunol Res (2017) 5(7):547–59. doi: 10.1158/2326-6066.CIR-16-0259
265. Koirala P, Roth ME, Gill J, Piperdi S, Chinai JM, Geller DS, et al. Immune Infiltration and PD-L1 Expression in the Tumor Microenvironment are Prognostic in Osteosarcoma. Sci Rep (2016) 6:30093. doi: 10.1038/srep30093
266. Fritzsching B, Fellenberg J, Moskovszky L, Sapi Z, Krenacs T, Machado I, et al. CD8(+)/FOXP3(+)-Ratio in Osteosarcoma Microenvironment Separates Survivors From Non-Survivors: A Multicenter Validated Retrospective Study. Oncoimmunology (2015) 4(3):e990800. doi: 10.4161/2162402X.2014.990800
267. Sundara YT, Kostine M, Cleven AH, Bovee JV, Schilham MW, Cleton-Jansen AM. Increased PD-L1 and T-Cell Infiltration in the Presence of HLA Class I Expression in Metastatic High-Grade Osteosarcoma: A Rationale for T-Cell-Based Immunotherapy. Cancer Immunol Immunother (2017) 66(1):119–28. doi: 10.1007/s00262-016-1925-3
268. Chen C, Xie L, Ren T, Huang Y, Xu J, Guo W. Immunotherapy for Osteosarcoma: Fundamental Mechanism, Rationale, and Recent Breakthroughs. Cancer Lett (2021) 500:1–10. doi: 10.1016/j.canlet.2020.12.024
269. Yang JX, Rastetter RH, Wilhelm D. Non-Coding RNAs: An Introduction. Adv Exp Med Biol (2016) 886:13–32. doi: 10.1007/978-94-017-7417-8_2
270. Gao Y, Luo LH, Li S, Yang C. miR-17 Inhibitor Suppressed Osteosarcoma Tumor Growth and Metastasis via Increasing PTEN Expression. Biochem Biophys Res Commun (2014) 444(2):230–4. doi: 10.1016/j.bbrc.2014.01.061
271. Ding J, Sha L, Shen P, Huang M, Cai Q, Li J. MicroRNA-18a Inhibits Cell Growth and Induces Apoptosis in Osteosarcoma by Targeting MED27. Int J Oncol (2018) 53(1):329–38. doi: 10.3892/ijo.2018.4374
272. Sun Z, Liu Q, Hong H, Zhang H, Zhang T. miR-19 Promotes Osteosarcoma Progression by Targeting SOCS6. Biochem Biophys Res Commun (2018) 495(1):1363–9. doi: 10.1016/j.bbrc.2017.10.002
273. Xin M, Qiao Z, Li J, Liu J, Song S, Zhao X, et al. miR-22 Inhibits Tumor Growth and Metastasis by Targeting ATP Citrate Lyase: Evidence in Osteosarcoma, Prostate Cancer, Cervical Cancer and Lung Cancer. Oncotarget (2016) 7(28):44252–65. doi: 10.18632/oncotarget.10020
274. He Y, Meng C, Shao Z, Wang H, Yang S. MiR-23a Functions as a Tumor Suppressor in Osteosarcoma. Cell Physiol Biochem (2014) 34(5):1485–96. doi: 10.1159/000366353
275. Chen B, Liu J, Qu J, Song Y, Li Y, Pan S. MicroRNA-25 Suppresses Proliferation, Migration, and Invasion of Osteosarcoma by Targeting SOX4. Tumour Biol (2017) 39(7):1010428317703841. doi: 10.1177/1010428317703841
276. Lu J, Song G, Tang Q, Yin J, Zou C, Zhao Z, et al. MiR-26a Inhibits Stem Cell-Like Phenotype and Tumor Growth of Osteosarcoma by Targeting Jagged1. Oncogene (2017) 36(2):231–41. doi: 10.1038/onc.2016.194
277. Zhang R, Yan S, Wang J, Deng F, Guo Y, Li Y, et al. MiR-30a Regulates the Proliferation, Migration, and Invasion of Human Osteosarcoma by Targeting Runx2. Tumour Biol (2016) 37(3):3479–88. doi: 10.1007/s13277-015-4086-7
278. Tao J, Cong H, Wang H, Zhang D, Liu C, Chu H, et al. MiR-30a-5p Inhibits Osteosarcoma Cell Proliferation and Migration by Targeting FOXD1. Biochem Biophys Res Commun (2018) 503(2):1092–7. doi: 10.1016/j.bbrc.2018.06.121
279. Zhao Y, Tu MJ, Yu YF, Wang WP, Chen QX, Qiu JX, et al. Combination Therapy With Bioengineered miR-34a Prodrug and Doxorubicin Synergistically Suppresses Osteosarcoma Growth. Biochem Pharmacol (2015) 98(4):602–13. doi: 10.1016/j.bcp.2015.10.015
280. Liu Q, Song Y, Duan X, Chang Y, Guo J. MiR-92a Inhibits the Progress of Osteosarcoma Cells and Increases the Cisplatin Sensitivity by Targeting Notch1. BioMed Res Int (2018) 2018:9870693. doi: 10.1155/2018/9870693
281. Yu B, Jiang K, Zhang J. MicroRNA-124 Suppresses Growth and Aggressiveness of Osteosarcoma and Inhibits TGF-Beta-Mediated AKT/GSK-3beta/SNAIL-1 Signaling. Mol Med Rep (2018) 17(5):6736–44. doi: 10.3892/mmr.2018.8637
282. Liu LH, Li H, Li JP, Zhong H, Zhang HC, Chen J, et al. miR-125b Suppresses the Proliferation and Migration of Osteosarcoma Cells Through Down-Regulation of STAT3. Biochem Biophys Res Commun (2011) 416(1-2):31–8. doi: 10.1016/j.bbrc.2011.10.117
283. Bao Y, Peng L, Ma J, Liu K, Li W. Decreased miR-134 Expression and its Tumor-Suppressive Function in Human Osteosarcoma. Genet Mol Res (2015) 14(4):16771–81. doi: 10.4238/2015.December.14.4
284. Li ZM, Zhang HY, Wang YX, Wang WB. MicroRNA-137 Is Downregulated in Human Osteosarcoma and Regulates Cell Proliferation and Migration Through Targeting FXYD6. J Drug Target (2016) 24(2):102–10. doi: 10.3109/1061186X.2015.1057149
285. Shi YK, Guo YH. MiR-139-5p Suppresses Osteosarcoma Cell Growth and Invasion Through Regulating DNMT1. Biochem Biophys Res Commun (2018) 503(2):459–66. doi: 10.1016/j.bbrc.2018.04.124
286. Gu R, Sun YF, Wu MF, Liu JB, Jiang JL, Wang SH, et al. Biological Roles of microRNA-140 in Tumor Growth, Migration, and Metastasis of Osteosarcoma In Vivo and In Vitro. Tumour Biol (2016) 37(1):353–60. doi: 10.1007/s13277-015-3801-8
287. Xiao Q, Huang L, Zhang Z, Chen X, Luo J, Zhang Z, et al. Overexpression of miR-140 Inhibits Proliferation of Osteosarcoma Cells via Suppression of Histone Deacetylase 4. Oncol Res (2017) 25(2):267–75. doi: 10.3727/096504016X14732510786564
288. Wang W, Zhou X, Wei M. MicroRNA-144 Suppresses Osteosarcoma Growth and Metastasis by Targeting ROCK1 and ROCK2. Oncotarget (2015) 6(12):10297–308. doi: 10.18632/oncotarget.3305
289. Li Y, Liu J, Liu ZZ, Wei WB. MicroRNA-145 Inhibits Tumour Growth and Metastasis in Osteosarcoma by Targeting Cyclin-Dependent Kinase, CDK6. Eur Rev Med Pharmacol Sci (2016) 20(24):5117–25.
290. Yang H, Peng Z, Da Z, Li X, Cheng Y, Tan B, et al. MicroRNA-148a Acts as a Tumor Suppressor in Osteosarcoma via Targeting Rho-Associated Coiled-Coil Kinase. Oncol Res (2017) 25(8):1231–43. doi: 10.3727/096504017X14850134190255
291. Zhou H, Zhang M, Yuan H, Zheng W, Meng C, Zhao D. MicroRNA-154 Functions as a Tumor Suppressor in Osteosarcoma by Targeting Wnt5a. Oncol Rep (2016) 35(3):1851–8. doi: 10.3892/or.2015.4495
292. Jiang C, Fang X, Zhang H, Wang X, Li M, Jiang W, et al. Triptolide Inhibits the Growth of Osteosarcoma by Regulating microRNA-181a via Targeting PTEN Gene In Vivo and Vitro. Tumour Biol (2017) 39(4):1010428317697556. doi: 10.1177/1010428317697556
293. Zhang Z, Zhang W, Mao J, Xu Z, Fan M. miR-186-5p Functions as a Tumor Suppressor in Human Osteosarcoma by Targeting Foxk1. Cell Physiol Biochem (2019) 52(3):553–64. doi: 10.33594/000000039
294. Pan L, Meng L, Liang F, Cao L. Mir188 Suppresses Tumor Progression by Targeting SOX4 in Pediatric Osteosarcoma. Mol Med Rep (2018) 18(1):441–6. doi: 10.3892/mmr.2018.8997
295. Pu Y, Zhao F, Cai W, Meng X, Li Y, Cai S. MiR-193a-3p and miR-193a-5p Suppress the Metastasis of Human Osteosarcoma Cells by Down-Regulating Rab27B and SRR, Respectively. Clin Exp Metastasis (2016) 33(4):359–72. doi: 10.1007/s10585-016-9783-0
296. Li M, Shen Y, Wang Q, Zhou X. MiR-204-5p Promotes Apoptosis and Inhibits Migration of Osteosarcoma via Targeting EBF2. Biochimie (2019) 158:224–32. doi: 10.1016/j.biochi.2018.12.003
297. Jiang Z, Jiang C, Yu C, Fang J. MicroRNA-208b Inhibits Human Osteosarcoma Progression by Targeting ROR2. Tumour Biol (2017) 39(6):1010428317705751. doi: 10.1177/1010428317705751
298. Liu C, Tang X. Downregulation of microRNA-210 Inhibits Osteosarcoma Growth In Vitro and In Vivo. Mol Med Rep (2015) 12(3):3674–80. doi: 10.3892/mmr.2015.3880
299. Luo XJ, Tang DG, Gao TL, Zhang YL, Wang M, Quan ZX, et al. MicroRNA-212 Inhibits Osteosarcoma Cells Proliferation and Invasion by Down-Regulation of Sox4. Cell Physiol Biochem (2014) 34(6):2180–8. doi: 10.1159/000369661
300. Xu Z, Wang T. miR-214 Promotes the Proliferation and Invasion of Osteosarcoma Cells Through Direct Suppression of LZTS1. Biochem Biophys Res Commun (2014) 449(2):190–5. doi: 10.1016/j.bbrc.2014.04.140
301. Sun B, Yang M, Li M, Wang F. The microRNA-217 Functions as a Tumor Suppressor and is Frequently Downregulated in Human Osteosarcoma. BioMed Pharmacother (2015) 71:58–63. doi: 10.1016/j.biopha.2015.02.014
302. Jiang W, Liu J, Xu T, Yu X. MiR-329 Suppresses Osteosarcoma Development by Downregulating Rab10. FEBS Lett (2016) 590(17):2973–81. doi: 10.1002/1873-3468.12337
303. He F, Fang L, Yin Q. miR-363 Acts as a Tumor Suppressor in Osteosarcoma Cells by Inhibiting PDZD2. Oncol Rep (2019) 41(5):2729–38. doi: 10.3892/or.2019.7078
304. Xu SY, Xu PF, Gao TT. MiR-372-3p Inhibits the Growth and Metastasis of Osteosarcoma Cells by Targeting FXYD6. Eur Rev Med Pharmacol Sci (2018) 22(1):62–9. doi: 10.26355/eurrev_201801_14101
305. Li Z, Shen J, Chan MT, Wu WK. MicroRNA-379 Suppresses Osteosarcoma Progression by Targeting PDK1. J Cell Mol Med (2017) 21(2):315–23. doi: 10.1111/jcmm.12966
306. Zhao D, Jia P, Wang W, Zhang G. VEGF-Mediated Suppression of Cell Proliferation and Invasion by miR-410 in Osteosarcoma. Mol Cell Biochem 400(1-2):87–95. doi: 10.1007/s11010-014-2265-2
307. Yang G, Zhang C, Wang N, Chen J. miR-425-5p Decreases LncRNA MALAT1 and TUG1 Expressions and Suppresses Tumorigenesis in Osteosarcoma via Wnt/beta-Catenin Signaling Pathway. Int J Biochem Cell Biol (2019) 111:42–51. doi: 10.1016/j.biocel.2019.04.004
308. Yuan J, Lang J, Liu C, Zhou K, Chen L, Liu Y. The Expression and Function of miRNA-451 in Osteosarcoma. Med Oncol (2015) 32(1):324. doi: 10.1007/s12032-014-0324-x
309. Yuan W, Wang D, Liu Y, Tian D, Wang Y, Zhang R, et al. Mir494 Inhibits Cell Proliferation and Metastasis via Targeting of CDK6 in Osteosarcoma. Mol Med Rep (2017) 16(6):8627–34. doi: 10.3892/mmr.2017.7709
310. Qi NN, Tian S, Li X, Wang FL, Liu B. Up-Regulation of microRNA-496 Suppresses Proliferation, Invasion, Migration and In Vivo Tumorigenicity of Human Osteosarcoma Cells by Targeting Eif4e. Biochimie (2019) 163:1–11. doi: 10.1016/j.biochi.2019.04.017
311. Pang PC, Shi XY, Huang WL, Sun K. miR-497 as a Potential Serum Biomarker for the Diagnosis and Prognosis of Osteosarcoma. Eur Rev Med Pharmacol Sci (2016) 20(18):3765–9.
312. Cai W, Xu Y, Yin J, Zuo W, Su Z. Mir5905p Suppresses Osteosarcoma Cell Proliferation and Invasion via Targeting KLF5. Mol Med Rep (2018) 18(2):2328–34. doi: 10.3892/mmr.2018.9173
313. Liu K, Sun X, Zhang Y, Liu L, Yuan Q. MiR-598: A Tumor Suppressor With Biomarker Significance in Osteosarcoma. Life Sci (2017) 188:141–8. doi: 10.1016/j.lfs.2017.09.003
314. Ma C, Zhan C, Yuan H, Cui Y, Zhang Z. MicroRNA-603 Functions as an Oncogene by Suppressing BRCC2 Protein Translation in Osteosarcoma. Oncol Rep (2016) 35(6):3257–64. doi: 10.3892/or.2016.4718
315. Wang H, Xing D, Ren D, Feng W, Chen Y, Zhao Z, et al. MicroRNA643 Regulates the Expression of ZEB1 and Inhibits Tumorigenesis in Osteosarcoma. Mol Med Rep (2017) 16(4):5157–64. doi: 10.3892/mmr.2017.7273
316. Zhang L, Wang Y, Zhang L, Xia X, Chao Y, He R, et al. ZBTB7A, a miR-663a Target Gene, Protects Osteosarcoma From Endoplasmic Reticulum Stress-Induced Apoptosis by Suppressing LncRNA GAS5 Expression. Cancer Lett (2019) 448:105–16. doi: 10.1016/j.canlet.2019.01.046
317. Liu Y, Wang Y, Yang H, Zhao L, Song R, Tan H, et al. MicroRNA873 Targets HOXA9 to Inhibit the Aggressive Phenotype of Osteosarcoma by Deactivating the Wnt/betacatenin Pathway. Int J Oncol (2019) 54(5):1809–20. doi: 10.3892/ijo.2019.4735
318. Ghosh T, Varshney A, Kumar P, Kaur M, Kumar V, Shekhar R, et al. MicroRNA-874-Mediated Inhibition of the Major G1/S Phase Cyclin, CCNE1, is Lost in Osteosarcomas. J Biol Chem (2017) 292(52):21264–81. doi: 10.1074/jbc.M117.808287
319. Zhong L, Zheng C, Fang H, Xu M, Chen B, Li C. MicroRNA-1270 is Associated With Poor Prognosis and its Inhibition Yielded Anticancer Mechanisms in Human Osteosarcoma. IUBMB Life (2018) 70(7):625–32. doi: 10.1002/iub.1753
320. Yuan H, Gao Y. MicroRNA-1908 is Upregulated in Human Osteosarcoma and Regulates Cell Proliferation and Migration by Repressing PTEN Expression. Oncol Rep (2015) 34(5):2706–14. doi: 10.3892/or.2015.4242
321. Shi D, Wu F, Mu S, Hu B, Zhong B, Gao F, et al. LncRNA AFAP1-AS1 Promotes Tumorigenesis and Epithelial-Mesenchymal Transition of Osteosarcoma Through RhoC/ROCK1/p38MAPK/Twist1 Signaling Pathway. J Exp Clin Cancer Res (2019) 38(1):375. doi: 10.1186/s13046-019-1363-0
322. Li R, Liu S, Li Y, Tang Q, Xie Y, Zhai R. Long Noncoding RNA AFAP1AS1 Enhances Cell Proliferation and Invasion in Osteosarcoma Through Regulating Mir46955p/TCF4betacatenin Signaling. Mol Med Rep (2018) 18(2):1616–22. doi: 10.3892/mmr.2018.9131
323. Lu L, Dai Z, Luo Q, Lv G. The Long Noncoding RNA Cancer Susceptibility Candidate 2 Inhibits Tumor Progression in Osteosarcoma. Mol Med Rep (2018) 17(1):1947–53. doi: 10.3892/mmr.2017.8080
324. Zhao W, Zhang D, Qin P, Zhang J, Cui X, Gao J, et al. Long non-Coding RNA EPIC1 Inhibits Viability and Invasion of Osteosarcoma Cells by Promoting MEF2D Ubiquitylation. Int J Biol Macromol (2019) 128:566–73. doi: 10.1016/j.ijbiomac.2019.01.156
325. Kun-Peng Z, Chun-Lin Z, Xiao-Long M. Antisense lncRNA FOXF1-AS1 Promotes Migration and Invasion of Osteosarcoma Cells Through the FOXF1/MMP-2/-9 Pathway. Int J Biol Sci (2017) 13(9):1180–91. doi: 10.7150/ijbs.21722
326. Sun J, Wang X, Fu C, Wang X, Zou J, Hua H, et al. Long Noncoding RNA FGFR3-AS1 Promotes Osteosarcoma Growth Through Regulating its Natural Antisense Transcript FGFR3. Mol Biol Rep (2016) 43(5):427–36. doi: 10.1007/s11033-016-3975-1
327. Ren Z, Hu Y, Li G, Kang Y, Liu Y, Zhao H. HIF-1alpha Induced Long Noncoding RNA FOXD2-AS1 Promotes the Osteosarcoma Through Repressing P21. BioMed Pharmacother (2019) 117:109104. doi: 10.1016/j.biopha.2019.109104
328. Ye K, Wang S, Zhang H, Han H, Ma B, Nan W. Long Noncoding RNA GAS5 Suppresses Cell Growth and Epithelial-Mesenchymal Transition in Osteosarcoma by Regulating the miR-221/ARHI Pathway. J Cell Biochem (2017) 118(12):4772–81. doi: 10.1002/jcb.26145
329. Qu Y, Zheng S, Kang M, Dong R, Zhou H, Zhao D, et al. Knockdown of Long non-Coding RNA HOXD-AS1 Inhibits the Progression of Osteosarcoma. BioMed Pharmacother (2018) 98:899–906. doi: 10.1016/j.biopha.2018.01.024
330. Wang B, Su Y, Yang Q, Lv D, Zhang W, Tang K, et al. Overexpression of Long Non-Coding RNA HOTAIR Promotes Tumor Growth and Metastasis in Human Osteosarcoma. Mol Cells (2015) 38(5):432–40. doi: 10.14348/molcells.2015.2327
331. Gu Z, Hou Z, Zheng L, Wang X, Wu L, Zhang C. Long Noncoding RNA LINC00858 Promotes Osteosarcoma Through Regulating miR-139-CDK14 Axis. Biochem Biophys Res Commun (2018) 503(2):1134–40. doi: 10.1016/j.bbrc.2018.06.131
332. Zhang B, Yu L, Han N, Hu Z, Wang S, Ding L, et al. LINC01116 Targets miR-520a-3p and Affects IL6R to Promote the Proliferation and Migration of Osteosarcoma Cells Through the Jak-Stat Signaling Pathway. BioMed Pharmacother (2018) 107:270–82. doi: 10.1016/j.biopha.2018.07.119
333. Han Z, Shi L. Long non-Coding RNA LUCAT1 Modulates Methotrexate Resistance in Osteosarcoma via miR-200c/ABCB1 Axis. Biochem Biophys Res Commun (2018) 495(1):947–53. doi: 10.1016/j.bbrc.2017.11.121
334. Chen Y, Huang W, Sun W, Zheng B, Wang C, Luo Z, et al. LncRNA MALAT1 Promotes Cancer Metastasis in Osteosarcoma via Activation of the PI3K-Akt Signaling Pathway. Cell Physiol Biochem (2018) 51(3):1313–26. doi: 10.1159/000495550
335. Dong Y, Liang G, Yuan B, Yang C, Gao R, Zhou X. MALAT1 Promotes the Proliferation and Metastasis of Osteosarcoma Cells by Activating the PI3K/Akt Pathway. Tumour Biol (2015) 36(3):1477–86. doi: 10.1007/s13277-014-2631-4
336. Duan G, Zhang C, Xu C, Xu C, Zhang L, Zhang Y. Knockdown of MALAT1 Inhibits Osteosarcoma Progression via Regulating the Mir34a/Cyclin D1 Axis. Int J Oncol (2019) 54(1):17–28. doi: 10.3892/ijo.2018.4600
337. Li J, Wu QM, Wang XQ, Zhang CQ. Long Noncoding RNA Mir210hg Sponges miR-503 to Facilitate Osteosarcoma Cell Invasion and Metastasis. DNA Cell Biol (2017) 36(12):1117–25. doi: 10.1089/dna.2017.3888
338. Hu Y, Yang Q, Wang L, Wang S, Sun F, Xu D, et al. Knockdown of the Oncogene lncRNA NEAT1 Restores the Availability of miR-34c and Improves the Sensitivity to Cisplatin in Osteosarcoma. Biosci Rep (2018) 38(3):BSR20180375. doi: 10.1042/BSR20180375
339. Ye H, Lin J, Yao X, Li Y, Lin X, Lu H. Overexpression of Long Non-Coding RNA NNT-AS1 Correlates With Tumor Progression and Poor Prognosis in Osteosarcoma. Cell Physiol Biochem (2018) 45(5):1904–14. doi: 10.1159/000487966
340. Zhu KP, Ma XL, Zhang CL. LncRNA ODRUL Contributes to Osteosarcoma Progression Through the miR-3182/MMP2 Axis. Mol Ther (2017) 25(10):2383–93. doi: 10.1016/j.ymthe.2017.06.027
341. Wang J, Cao L, Wu J, Wang Q. Long non-Coding RNA SNHG1 Regulates NOB1 Expression by Sponging miR-326 and Promotes Tumorigenesis in Osteosarcoma. Int J Oncol (2018) 52(1):77–88. doi: 10.3892/ijo.2017.4187
342. Jiang Z, Jiang C, Fang J. Up-Regulated lnc-SNHG1 Contributes to Osteosarcoma Progression Through Sequestration of miR-577 and Activation of WNT2B/Wnt/beta-Catenin Pathway. Biochem Biophys Res Commun (2018) 495(1):238–45. doi: 10.1016/j.bbrc.2017.11.012
343. Deng Y, Zhao F, Zhang Z, Sun F, Wang M. Long Noncoding RNA SNHG7 Promotes the Tumor Growth and Epithelial-To-Mesenchymal Transition via Regulation of miR-34a Signals in Osteosarcoma. Cancer Biother Radiopharm (2018) 33(9):365–72. doi: 10.1089/cbr.2018.2503
344. Yang G, Song R, Wang L, Wu X. Knockdown of Long non-Coding RNA TP73-AS1 Inhibits Osteosarcoma Cell Proliferation and Invasion Through Sponging miR-142. BioMed Pharmacother (2018) 103:1238–45. doi: 10.1016/j.biopha.2018.04.146
345. Wang H, Yu Y, Fan S, Luo L. Knockdown of Long Noncoding RNA TUG1 Inhibits the Proliferation and Cellular Invasion of Osteosarcoma Cells by Sponging miR-153. Oncol Res (2018) 26(5):665–73. doi: 10.3727/096504017X14908298412505
346. Yang C, Wu K, Wang S, Wei G. Long non-Coding RNA XIST Promotes Osteosarcoma Progression by Targeting YAP via miR-195-5p. J Cell Biochem (2018) 119(7):5646–56. doi: 10.1002/jcb.26743
347. Zhang R, Xia T. Long non-Coding RNA XIST Regulates PDCD4 Expression by Interacting With miR-21-5p and Inhibits Osteosarcoma Cell Growth and Metastasis. Int J Oncol (2017) 51(5):1460–70. doi: 10.3892/ijo.2017.4127
348. Liu G, Huang K, Jie Z, Wu Y, Chen J, Chen Z, et al. CircFAT1 Sponges miR-375 to Promote the Expression of Yes-Associated Protein 1 in Osteosarcoma Cells. Mol Cancer (2018) 17(1):170. doi: 10.1186/s12943-018-0917-7
349. Bartel DP. MicroRNAs: Genomics, Biogenesis, Mechanism, and Function. Cell (2004) 116(2):281–97. doi: 10.1016/s0092-8674(04)00045-5
350. Pasquinelli AE, Hunter S, Bracht J. MicroRNAs: A Developing Story. Curr Opin Genet Dev (2005) 15(2):200–5. doi: 10.1016/j.gde.2005.01.002
351. Kim VN, Nam JW. Genomics of microRNA. Trends Genet (2006) 22(3):165–73. doi: 10.1016/j.tig.2006.01.003
352. Mao JH, Zhou RP, Peng AF, Liu ZL, Huang SH, Long XH, et al. microRNA-195 Suppresses Osteosarcoma Cell Invasion and Migration In Vitro by Targeting FASN. Oncol Lett (2012) 4(5):1125–9. doi: 10.3892/ol.2012.863
353. Cai H, Zhao H, Tang J, Wu H. Serum miR-195 is a Diagnostic and Prognostic Marker for Osteosarcoma. J Surg Res (2015) 194(2):505–10. doi: 10.1016/j.jss.2014.11.025
354. Lian F, Cui Y, Zhou C, Gao K, Wu L. Identification of a Plasma four-microRNA Panel as Potential Noninvasive Biomarker for Osteosarcoma. PloS One (2015) 10(3):e0121499. doi: 10.1371/journal.pone.0121499
355. Salah Z, Arafeh R, Maximov V, Galasso M, Khawaled S, Abou-Sharieha S, et al. miR-27a and miR-27a* Contribute to Metastatic Properties of Osteosarcoma Cells. Oncotarget (2015) 6(7):4920–35. doi: 10.18632/oncotarget.3025
356. Jones KB, Salah Z, Del Mare S, Galasso M, Gaudio E, Nuovo GJ, et al. miRNA Signatures Associate With Pathogenesis and Progression of Osteosarcoma. Cancer Res (2012) 72(7):1865–77. doi: 10.1158/0008-5472.CAN-11-2663
357. Pan W, Wang H, Jianwei R, Ye Z. MicroRNA-27a Promotes Proliferation, Migration and Invasion by Targeting MAP2K4 in Human Osteosarcoma Cells. Cell Physiol Biochem (2014) 33(2):402–12. doi: 10.1159/000356679
358. Dai N, Zhong ZY, Cun YP, Qing Y, Chen C, Jiang P, et al. Alteration of the microRNA Expression Profile in Human Osteosarcoma Cells Transfected With APE1 siRNA. Neoplasma (2013) 60(4):384–94. doi: 10.4149/neo_2013_050
359. Zhang Y, Hu H, Song L, Cai L, Wei R, Jin W. Epirubicin-Mediated Expression of miR-302b is Involved in Osteosarcoma Apoptosis and Cell Cycle Regulation. Toxicol Lett (2013) 222(1):1–9. doi: 10.1016/j.toxlet.2013.06.242
360. Li Y, Zhang J, Zhang L, Si M, Yin H, Li J. Diallyl Trisulfide Inhibits Proliferation, Invasion and Angiogenesis of Osteosarcoma Cells by Switching on Suppressor microRNAs and Inactivating of Notch-1 Signaling. Carcinogenesis (2013) 34(7):1601–10. doi: 10.1093/carcin/bgt065
361. Chen Y, Zhu X, Zhang X, Liu B, Huang L. Nanoparticles Modified With Tumor-Targeting scFv Deliver siRNA and miRNA for Cancer Therapy. Mol Ther (2010) 18(9):1650–6. doi: 10.1038/mt.2010.136
362. Varshney J, Subramanian S. MicroRNAs as Potential Target in Human Bone and Soft Tissue Sarcoma Therapeutics. Front Mol Biosci (2015) 2:31. doi: 10.3389/fmolb.2015.00031
363. Qi P, Du X. The Long non-Coding RNAs, a New Cancer Diagnostic and Therapeutic Gold Mine. Mod Pathol (2013) 26(2):155–65. doi: 10.1038/modpathol.2012.160
364. Yang Z, Li X, Yang Y, He Z, Qu X, Zhang Y. Long Noncoding RNAs in the Progression, Metastasis, and Prognosis of Osteosarcoma. Cell Death Dis (2016) 7(9):e2389. doi: 10.1038/cddis.2016.272
365. Ji P, Diederichs S, Wang W, Boing S, Metzger R, Schneider PM, et al. MALAT-1, a Novel Noncoding RNA, and Thymosin Beta4 Predict Metastasis and Survival in Early-Stage non-Small Cell Lung Cancer. Oncogene (2003) 22(39):8031–41. doi: 10.1038/sj.onc.1206928
366. Gao KT, Lian D. Long non-Coding RNA MALAT1 is an Independent Prognostic Factor of Osteosarcoma. Eur Rev Med Pharmacol Sci (2016) 20(17):3561–5.
367. Zhu L, Liu J, Ma S, Zhang S. Long Noncoding RNA MALAT-1 Can Predict Metastasis and a Poor Prognosis: A Meta-Analysis. Pathol Oncol Res (2015) 21(4):1259–64. doi: 10.1007/s12253-015-9960-5
368. Liu M, Yang P, Mao G, Deng J, Peng G, Ning X, et al. Long non-Coding RNA MALAT1 as a Valuable Biomarker for Prognosis in Osteosarcoma: A Systematic Review and Meta-Analysis. Int J Surg (2019) 72:206–13. doi: 10.1016/j.ijsu.2019.11.004
369. Huo Y, Li Q, Wang X, Jiao X, Zheng J, Li Z, et al. MALAT1 Predicts Poor Survival in Osteosarcoma Patients and Promotes Cell Metastasis Through Associating With EZH2. Oncotarget (2017) 8(29):46993–7006. doi: 10.18632/oncotarget.16551
370. Fang D, Yang H, Lin J, Teng Y, Jiang Y, Chen J, et al. 17beta-Estradiol Regulates Cell Proliferation, Colony Formation, Migration, Invasion and Promotes Apoptosis by Upregulating miR-9 and Thus Degrades MALAT-1 in Osteosarcoma Cell MG-63 in an Estrogen Receptor-Independent Manner. Biochem Biophys Res Commun (2015) 457(4):500–6. doi: 10.1016/j.bbrc.2014.12.114
371. Hu Q, Li S, Chen C, Zhu M, Chen Y, Zhao Z. 17betaestradiol Treatment Drives Sp1 to Upregulate MALAT1 Expression and Epigenetically Affects Physiological Processes in U2OS Cells. Mol Med Rep (2017) 15(3):1335–42. doi: 10.3892/mmr.2017.6115
372. Deng R, Zhang J, Chen J. lncRNA SNHG1 Negatively Regulates Mirna1013p to Enhance the Expression of ROCK1 and Promote Cell Proliferation, Migration and Invasion in Osteosarcoma. Int J Mol Med (2019) 43(3):1157–66. doi: 10.3892/ijmm.2018.4039
373. Ruan W, Wang P, Feng S, Xue Y, Li Y. Long non-Coding RNA Small Nucleolar RNA Host Gene 12 (SNHG12) Promotes Cell Proliferation and Migration by Upregulating Angiomotin Gene Expression in Human Osteosarcoma Cells. Tumour Biol (2016) 37(3):4065–73. doi: 10.1007/s13277-015-4256-7
374. Zhou S, Yu L, Xiong M, Dai G. LncRNA SNHG12 Promotes Tumorigenesis and Metastasis in Osteosarcoma by Upregulating Notch2 by Sponging miR-195-5p. Biochem Biophys Res Commun (2018) 495(2):1822–32. doi: 10.1016/j.bbrc.2017.12.047
375. Xu N, Xu J, Zuo Z, Liu Y, Yan F, Han C. Downregulation of lncRNA SNHG12 Reversed IGF1R-Induced Osteosarcoma Metastasis and Proliferation by Targeting miR-195-5p. Gene (2020) 726:144145. doi: 10.1016/j.gene.2019.144145
376. Wang Y, Zeng X, Wang N, Zhao W, Zhang X, Teng S, et al. Long Noncoding RNA DANCR, Working as a Competitive Endogenous RNA, Promotes ROCK1-Mediated Proliferation and Metastasis via Decoying of miR-335-5p and miR-1972 in Osteosarcoma. Mol Cancer (2018) 17(1):89. doi: 10.1186/s12943-018-0837-6
377. Jiang N, Wang X, Xie X, Liao Y, Liu N, Liu J, et al. lncRNA DANCR Promotes Tumor Progression and Cancer Stemness Features in Osteosarcoma by Upregulating AXL via miR-33a-5p Inhibition. Cancer Lett (2017) 405:46–55. doi: 10.1016/j.canlet.2017.06.009
378. Jeck WR, Sorrentino JA, Wang K, Slevin MK, Burd CE, Liu J, et al. Circular RNAs are Abundant, Conserved, and Associated With ALU Repeats. RNA (2013) 19(2):141–57. doi: 10.1261/rna.035667.112
379. Ebbesen KK, Kjems J, Hansen TB. Circular RNAs: Identification, Biogenesis and Function. Biochim Biophys Acta (2016) 1859(1):163–8. doi: 10.1016/j.bbagrm.2015.07.007
380. Wang C, Ren M, Zhao X, Wang A, Wang J. Emerging Roles of Circular RNAs in Osteosarcoma. Med Sci Monit (2018) 24:7043–50. doi: 10.12659/MSM.912092
381. Xiao-Long M, Kun-Peng Z, Chun-Lin Z. Circular RNA Circ_HIPK3 is Down-Regulated and Suppresses Cell Proliferation, Migration and Invasion in Osteosarcoma. J Cancer (2018) 9(10):1856–62. doi: 10.7150/jca.24619
382. Zheng Q, Bao C, Guo W, Li S, Chen J, Chen B, et al. Circular RNA Profiling Reveals an Abundant Circhipk3 That Regulates Cell Growth by Sponging Multiple miRNAs. Nat Commun 7:11215. doi: 10.1038/ncomms11215
383. Li Y, Zheng F, Xiao X, Xie F, Tao D, Huang C, et al. CircHIPK3 Sponges miR-558 to Suppress Heparanase Expression in Bladder Cancer Cells. EMBO Rep (2017) 18(9):1646–59. doi: 10.15252/embr.201643581
384. Chen G, Shi Y, Liu M, Sun J. Circhipk3 Regulates Cell Proliferation and Migration by Sponging miR-124 and Regulating AQP3 Expression in Hepatocellular Carcinoma. Cell Death Dis (2018) 9(2):175. doi: 10.1038/s41419-017-0204-3
385. Kai D, Yannian L, Yitian C, Dinghao G, Xin Z, Wu J. Circular RNA HIPK3 Promotes Gallbladder Cancer Cell Growth by Sponging microRNA-124. Biochem Biophys Res Commun (2018) 503(2):863–9. doi: 10.1016/j.bbrc.2018.06.088
386. Zeng K, Chen X, Xu M, Liu X, Hu X, Xu T, et al. CircHIPK3 Promotes Colorectal Cancer Growth and Metastasis by Sponging miR-7. Cell Death Dis (2018) 9(4):417. doi: 10.1038/s41419-018-0454-8
387. Wu Z, Shi W, Jiang C. Overexpressing Circular RNA Hsa_Circ_0002052 Impairs Osteosarcoma Progression via Inhibiting Wnt/beta-Catenin Pathway by Regulating miR-1205/APC2 Axis. Biochem Biophys Res Commun (2018) 502(4):465–71. doi: 10.1016/j.bbrc.2018.05.184
388. Ren C, Liu J, Zheng B, Yan P, Sun Y, Yue B. The Circular RNA Circ-ITCH Acts as a Tumour Suppressor in Osteosarcoma via Regulating miR-22. Artif Cells Nanomed Biotechnol (2019) 47(1):3359–67. doi: 10.1080/21691401.2019.1649273
389. de Nigris F, Mancini FP, Schiano C, Infante T, Zullo A, Minucci PB, et al. Osteosarcoma Cells Induce Endothelial Cell Proliferation During Neo-Angiogenesis. J Cell Physiol (2013) 228(4):846–52. doi: 10.1002/jcp.24234
390. Yamanegi K, Kawabe M, Futani H, Nishiura H, Yamada N, Kato-Kogoe N, et al. Sodium Valproate, a Histone Deacetylase Inhibitor, Modulates the Vascular Endothelial Growth Inhibitor-Mediated Cell Death in Human Osteosarcoma and Vascular Endothelial Cells. Int J Oncol (2015) 46(5):1994–2002. doi: 10.3892/ijo.2015.2924
391. Vimalraj S, Saravanan S, Raghunandhakumar S, Anuradha D. Melatonin Regulates Tumor Angiogenesis via miR-424-5p/VEGFA Signaling Pathway in Osteosarcoma. Life Sci (2020) 256:118011. doi: 10.1016/j.lfs.2020.118011
392. Bulut G, Hong SH, Chen K, Beauchamp EM, Rahim S, Kosturko GW, et al. Small Molecule Inhibitors of Ezrin Inhibit the Invasive Phenotype of Osteosarcoma Cells. Oncogene (2012) 31(3):269–81. doi: 10.1038/onc.2011.245
393. Sleeman J, Steeg PS. Cancer Metastasis as a Therapeutic Target. Eur J Cancer (2010) 46(7):1177–80. doi: 10.1016/j.ejca.2010.02.039
395. Liang C, Li F, Wang L, Zhang ZK, Wang C, He B, et al. Tumor Cell-Targeted Delivery of CRISPR/Cas9 by Aptamer-Functionalized Lipopolymer for Therapeutic Genome Editing of VEGFA in Osteosarcoma. Biomaterials (2017) 147:68–85. doi: 10.1016/j.biomaterials.2017.09.015
396. Chou AJ, Gupta R, Bell MD, Riewe KO, Meyers PA, Gorlick R. Inhaled Lipid Cisplatin (ILC) in the Treatment of Patients With Relapsed/Progressive Osteosarcoma Metastatic to the Lung. Pediatr Blood Cancer (2013) 60(4):580–6. doi: 10.1002/pbc.24438
397. Dow S, Elmslie R, Kurzman I, MacEwen G, Pericle F, Liggitt D. Phase I Study of Liposome-DNA Complexes Encoding the Interleukin-2 Gene in Dogs With Osteosarcoma Lung Metastases. Hum Gene Ther (2005) 16(8):937–46. doi: 10.1089/hum.2005.16.937
398. Bruland OS, Hoifodt H, Saeter G, Smeland S, Fodstad O. Hematogenous Micrometastases in Osteosarcoma Patients. Clin Cancer Res (2005) 11(13):4666–73. doi: 10.1158/1078-0432.CCR-05-0165
399. Bruland OS, Hoifodt H, Hall KS, Smeland S, Fodstad O. Bone Marrow Micrometastases Studied by an Immunomagnetic Isolation Procedure in Extremity Localized non-Metastatic Osteosarcoma Patients. Cancer Treat Res (2009) 152:509–15. doi: 10.1007/978-1-4419-0284-9_30
400. Guan G, Lu Y, Zhu X, Liu L, Chen J, Ma Q, et al. CXCR4-Targeted Near-Infrared Imaging Allows Detection of Orthotopic and Metastatic Human Osteosarcoma in a Mouse Model. Sci Rep (2015) 5:15244. doi: 10.1038/srep15244
Keywords: osteosarcoma, metastasis, microenvironment, metabolism, noncoding RNAs
Citation: Sheng G, Gao Y, Yang Y and Wu H (2021) Osteosarcoma and Metastasis. Front. Oncol. 11:780264. doi: 10.3389/fonc.2021.780264
Received: 20 September 2021; Accepted: 16 November 2021;
Published: 10 December 2021.
Edited by:
Chi-Kong Li, The Chinese University of Hong Kong, Hong Kong SAR, ChinaReviewed by:
Oscar M. Tirado, Institut d’Investigacio Biomedica de Bellvitge (IDIBELL), SpainDominique Heymann, Université de Nantes, France
Copyright © 2021 Sheng, Gao, Yang and Wu. This is an open-access article distributed under the terms of the Creative Commons Attribution License (CC BY). The use, distribution or reproduction in other forums is permitted, provided the original author(s) and the copyright owner(s) are credited and that the original publication in this journal is cited, in accordance with accepted academic practice. No use, distribution or reproduction is permitted which does not comply with these terms.
*Correspondence: Yong Yang, dGp5YW5neW9uZ0BodXN0LmVkdS5jbg==; Hua Wu, d3VodWFAaHVzdC5lZHUuY24=