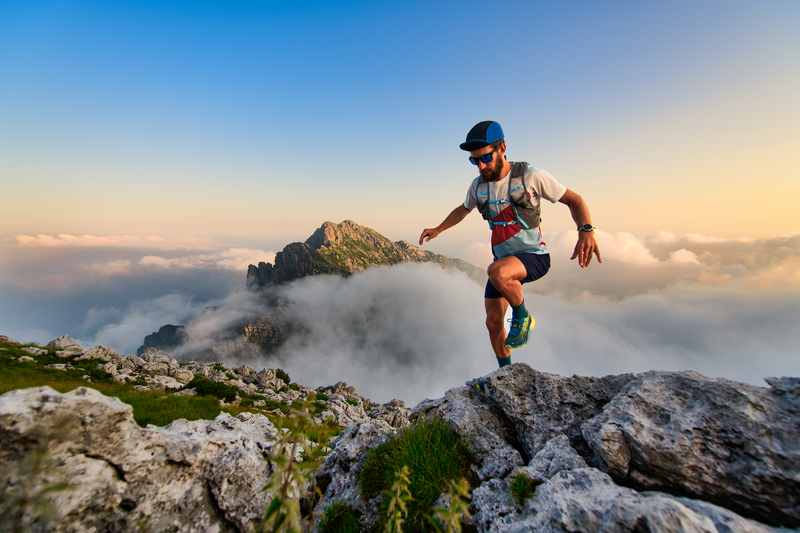
95% of researchers rate our articles as excellent or good
Learn more about the work of our research integrity team to safeguard the quality of each article we publish.
Find out more
REVIEW article
Front. Oncol. , 05 November 2021
Sec. Cancer Molecular Targets and Therapeutics
Volume 11 - 2021 | https://doi.org/10.3389/fonc.2021.758884
This article is part of the Research Topic The Role of Extracellular Vesicles in Diagnosis, Prognosis And Treatment Of Patients with Gastrointestinal Cancer View all 3 articles
Cancer cell-derived extracellular vesicles (CEVs), a novel type of therapeutic agent in cancer treatment, can be prepared from the autocrine secretion of various cancer cells, the direct extraction of cancer cells and the combination of cancer cell-derived membranes with advanced materials. With various bioactive molecules, exosomes are produced by cells for intercellular communication. Although cancer cell-derived exosomes are known to inhibit tumor apoptosis and promote the progression of cancer, researchers have developed various innovative strategies to prepare anti-tumor vesicles from cancer cells. With current strategies for anti-tumor vesicles, four different kinds of CEVs are classified including irradiated CEVs, advanced materials combined CEVs, chemotherapeutic drugs loaded CEVs and genetically engineered CEVs. In this way, CEVs can not only be the carriers for anti-tumor drugs to the target tumor area but also act as immune-active agents. Problems raised in the strategies mainly concerned with the preparation, efficacy and application. In this review, we classified and summarized the current strategies for utilizing the anti-tumor potential of CEVs. Additionally, the challenges and the prospects of this novel agent have been discussed.
Extracellular vesicles (EVs) are phospholipid bilayer membrane-coated vesicles that are generated by cells for intercellular communication (1, 2). EVs can be classified into exosomes, microvesicles and apoptotic bodies (3, 4). The exosomes biogenesis was showed in Figure 1. The classification of EVs is mainly based on their biogenesis. Ranging from 100 nm to 5 μm, apoptotic bodies were generated and secreted by the cells undergoing apoptosis with outward blebbing of the plasma membrane (5, 6). With a diameter from 50 nm to 1 μm, microvesicles generated by budding from cellular membranes after activation, shear, or physical stress (7). Exosomes are defined as the vesicles with a size of 30-150 nm, and are released from cells undergoing fusion of an endocytic compartment multivesicular body with the plasma membrane (8). EVs are inherently loaded with cargoes, including various bioactive molecules, such as nucleic acids, proteins, and lipids for cell-to-cell signaling (9). Therefore, the short/long distant exchange of bioactive factors in cells is closely associated with the transfer of EVs in health and disease. Regarding the EVs derived from cancer cells, EVs are naturally the key players in tumor progression, tumor metastasis, immunosuppression, and drug resistance (10–15). The presence of pernicious products in vesicles limits, to a great extent, the exploration of the therapeutic value in Cancer cell-derived extracellular vesicles (CEVs) but still fail to cover the latent anti-tumor capacity of vesicles.
In the past decade, there has been extensive research done on CEVs, which has facilitated their use in the development of novel therapeutic agents for cancer therapy. Several in vitro, in vivo, and clinical trials have been conducted using CEVs-based strategies to obtain CEVs with effective anti-tumor activity. One of the primary advantages of CEVs includes their high targetability to the tumor. Additionally, they are known to preferentially target their homologous tumor cells (16–20). The specific accumulation of CEVs in the tumor area implies that CEVs could be used for drug delivery for potential targeted therapy. Thus, recent studies investigated the effects of drug-loaded CEVs and found excellent effectiveness against cancer (21–26). Additionally, oncolytic viruses can also be packed into CEVs to protect them from neutralizing antibodies in the serum, allowing them to function as oncolytic agents (27). The parent cancer cells, which secrete CEVs, heavily determine the constituents, determining the essential quality of CEVs. Studies have revealed genetically engineered cancer cells, which generate genetically engineered CEVs, resulting in efficient anti-tumor immunity and improved anti-tumor efficacy (28–33). Additionally, cancer cells were also irradiated to prepare CEVs to enhance the safety and anti-tumor efficacy of CEVs. With a potential role in exosome-mediated bystander effects (34), these irradiated CEVs could inhibit the progression of the tumor (25, 35).
Several studies have indicated that EVs from cancer can promote tumorigenesis and metastasis; thus, the EVs derived from immune cells, such as dendritic cells, natural killer cells, and CAR-T cells, have been used for cancer therapy (36–40). The in-depth analysis of CEVs, as well as the exploration of therapeutic EVs of immune cells, have provided indications for the role of CEVs in cancer therapy. In this review, we provide a comprehensive overview of the current strategies, challenges, and prospects for the use of CEVs in cancer treatment.
Previous studies have shown that radiotherapy, one of the primary treatments in cancer therapy, can cause a radiation-induced bystander effect (RIBE), which is a unique reaction triggered by irradiated cells or tissues, leading to the induction of apoptosis in unexposed cells (41–45). Similar to RIBE, the ionizing radiation-exposed cancer cell vaccines were tested, and their efficacy was confirmed in clinical trials (46–48). Researchers showed that the underlying mechanism of irradiated tumor cell vaccine in cancer therapy involved the activation of dendritic cells and T cells (49–51). Hence, radiation exposure was used in the preparation of CEVs. Figure 2 illustrates the mechanism involved in the preparation of irradiated CEVs.
Figure 2 The current strategies for the preparation and anti-tumor effect of irradiated cancer cells-derived extracellular vesicles.
In a recent study on irradiated CEVs, the researchers irradiated the cancer cells with a single dose of 20 Gy by 6-MV X-rays to generate EVs (35). The results showed the irradiated CEVs could induce the ferroptosis of cancer cells. Additionally, the treatment with irradiated CEVs in the mouse model induced the transformation of M2 tumor-associated macrophages into M1 tumor-associated macrophages. For CEVs loaded with chemotherapeutic agents, the process of irradiation was able to improve the efficacy in the cancer treatment (51). Another study showed that compared with the PBS group, CEVs of A549 lung cancer promoted the progression of cancer and malignant pleural effusion, while the irradiated CEVs group showed no significant change in the cancer progression (25). Additionally, the irradiated and drug-loaded CEVs of A549 lung cancer inhibited the progression of cancer and malignant pleural effusion in the clinical tests (25, 52).
Although the anti-tumor efficacy of irradiated CEVs was triggered by the promotion of tumor antigen (53), the detailed mechanism of the RIBE is unclear and the presentation of tumor antigen may associate with the therapeutic Dexosomes (one kind of exosomes secreted form Dendritic cells) (54). Further studies are required to explore the underlying mechanism of irradiated CEVs against cancer.
There has been an extensive increase in the use of advanced materials as biomimetic carriers in the field of cancer therapy. Nanoparticles serve as stable carriers to protect antigens from rapid clearance and degradation in the serum, achieving a stronger immune response (55–57). In a study, PLGA microspheres were coated with CEVs, and the results showed an increase in phagocytosis in macrophages and dendritic cells (58). Consequently, treatment with coated CEVs caused an enhanced cytokine release in antigen-presenting cells. Figure 3 illustrates the mechanism involved in the coating of CEVs with advanced materials.
Figure 3 The current strategies for the preparation and anti-tumor effect of advanced materials combined cancer cells-derived extracellular vesicles.
When therapeutic materials, such as photothermal materials and cytotoxic agents, are combined, CEVs serve as carriers to accumulate in tumor cells leading to cytotoxicity. The pristine zinc oxide nanocrystals enclosed with CEVs could be engulfed by cancer cells, exhibiting toxicity (59). Also, photothermal therapy was designed to combine with CEVs, based on its direct cytotoxic effect and ability to increase the susceptibility of cancer cells to chemotherapy. CEVs were loaded with photothermal Bi2Se3 and chemotherapeutic doxorubicin hydrochloride (21). With good biosafety and feasibility, the CEVs could disperse well in the tumor area, exhibiting photothermal effect.
Most studies on CEVs for advanced materials combined with biomimetic EVs were performed using membranes of cancer cells. The design of cancer cell membrane-coated nanoparticles is based on the homologous target for targeted drug delivery (18, 60–63). The membrane is extracted from cancer cells mostly via freeze-thawing and centrifugation. The photothermal materials loaded-nanoparticles with cancer cell membrane were assembled by the co-extrusion of their mixture to generate the biomimetic CEVs (18, 63). The membrane from hybrid cells of cancer and dendritic cells were extracted and combined with photothermal nanoparticles against cancer to enhance the antigen-presenting function of biomimetic EVs, resulting in a promising anti-tumor effect (16).
CEVs possess the properties of homologous tumor targeting and favorable compatibility for drugs. Figure 4 illustrates the mechanism involved in the loading of chemotherapeutic drugs into CEVs. The drug-loaded CEVs are mostly prepared by the co-incubation of drugs with cancer cells, resulting in the phagocytosis of drugs. As the EVs are processed and generated, CEVs are released and packed with chemotherapeutic drugs in the supernatant of cancer cells (21–23, 25, 51, 64). In other studies, the drug-loaded CEVs were mainly prepared in two steps: the first step involved the isolation of CEVs, and the second step involved drug loading (19, 26, 65–67). The first step of isolation was conducted mainly via the traditional ultracentrifugation. In a study of doxorubicin-loaded cell-derived nanovesicles for the generation of CEVs (66), U937 lymphoma cells were sequentially extruded through filters using 10 and 8 μm filter membranes, followed by purification using Sephadex G50 size-exclusion column. After collecting CEVs, the second step of drug loading was performed via the direct incubation of CEVs with chemotherapeutic agents at 37°C (26, 66) or 22°C (67). However, compared with incubation at room temperature, a better efficiency of drug loading and release was observed at 37°C (66). Additionally, the co-extrusion of the mixture of chemotherapeutic drugs and CEVs also exhibited efficient drug loading and anti-cancer activity (19). The sonication of the mixture of catalase and EVs of macrophages showed the highest loading efficiency and good releasing efficiency, among other methods of direct incubation, freeze-thawing, and co-extrusion (68).
Figure 4 The current strategies for the preparation and anti-tumor effect of chemotherapeutic drugs loaded cancer cells-derived extracellular vesicles.
Additionally, oncolytic virotherapy was also used in combination with CEVs against cancer. Oncolytic adenovirus infected- A549 lung cancer cells could release EVs containing bioactive adenovirus particles (27). The CEVs can protect adenovirus from the clearance in the serum, resulting in improved anti-cancer efficacy. Although viruses combined with CEVs could exhibit therapeutic efficiency, upregulated levels of CLTA-4 and PD-1 were detected after the administration of the virus for the cancer therapy (69–71), and the PD-L1 of cancer EVs also promoted tumorigenesis (72). Thus, further studies are required to design combined therapy, including immunotherapeutic drugs with EVs, by constructing CEVs with immune checkpoint inhibitors.
In clinical experiments, chemotherapeutic drugs-loaded CEVs achieved promising outcomes in lung cancer patients with malignant pleural effusion (MPE). Cisplatin-loaded CEVs were prepared from the supernatant of the mixture of A549 cells and Cisplatin (52). The injections of cisplatin-loaded CEVs were administered intrathoracically four times, and the results exhibited a 95% reduction in tumor cells in the MPE. In another clinical study with methotrexate-loaded CEVs (25), autologous tumor cells were prepared from patients’ MPE and incubated with chemotherapeutic methotrexate after ultraviolet irradiation. The methotrexate-loaded CEVs were collected by ultracentrifugation. Autologous CEVs were administered intrapleurally six times. This therapy could effectively stimulate CD4+ T cells and showed a good safety profile of CEVs, exhibiting\ promising reduction in tumor cells and CD163+ macrophages.
CEVs are the final products generated and prepared from cancer cells. Therefore, the construction of a genetically modified CEVs requires a genetically engineered cancer cell line. Thus, various design strategies were designed by transfecting therapeutic genes into cancer cells to alter the inherent characteristics of CEVs for cancer therapy (28–31, 33). Figure 5 illustrates the design strategies for genetically engineered CEVs.
Figure 5 The current strategies for the preparation and anti-tumor effect of genetically engineered cancer cells-derived extracellular vesicles.
The miRNA content of the exosomes is also known to promote the progression of cancer (73), such as miRNA-15b (74) and miRNA-21 (75), while exosomal miRNA-22 (76), miRNA-142 (77), and miRNA-155 (31, 78) have been shown to exert therapeutic effects against cancer. A study showed that after transfecting DNA vectors expressing miRNA-155 and miRNA-125b2 into pancreatic cancer cells, the altered CEVs had upregulated miRNA levels, as detected by the ExoQuick-TC exosome precipitation solution (31). The results showed that the genetically engineered CEVs promoted the reprogramming of macrophages to the M1 phenotype. Additionally, miRNA-21 is known to enhance chemotherapy resistance; thus, a combination of silencing such miRNA and CEVs showed improved chemotherapy sensitivity. Also, treatment with anti-miRNA-21-loaded CEVs and doxorubicin arrested the growth of cancer cells (30).
Also, CEVs are known to naturally possess the proteins that present in the corresponding cancer cells, such as immunosuppressive PD-L1 (72) and prostate-specific antigen (PSA) (79). In a study, B16BL6 cells were transfected with the plasmid expressing streptavidin and lactadherin, followed by ultracentrifugation to isolate the CEVs to endow them with increased capacity of tumor antigen presentation (33). In another study, the engineered EVs derived from Expi293 cells expressing anti-CD3 and anti-HER2 antibodies exhibited a potent anti-tumor effect for targeted immunotherapy. Additionally, the therapeutic proteins in CEVs could be overexpressed via transfection to boost the therapeutic efficacy of inherent proteins in CEVs. An efficient induction of anti-tumor immunity was observed after treatment with CEVs that overexpressed Rab27a (28), which is known to serve as an important regulator of exosomes (80) and antigen presenters (81). It is noteworthy that the genetically engineered CEVs which had experienced irradiation could serve as a much more effective medicine against cancer (82).
Although gradient ultracentrifugation provides excellent purity for the preparation of CEVs, sequential ultracentrifugation is more commonly used as it is more convenient and provides a larger volume. The polymer precipitation technique is easy to perform and efficient; however, it has a complicated clean-up process. Additionally, novel strategies, such as microfluidics-based techniques and immunoaffinity capture, are also suitable for the isolation of CEVs; however, they are expensive.
Thus, new techniques are required for the innovative generation of self-designed CEVs for further analysis of the anti-tumor potential of CEVs. A sequential extrusion strategy was used for the artificial synthesis of CEVs. CEVs were prepared after extrusion through 10 and 8 μm membrane filters (66). Additionally, the process of freeze-thawing of cancer cells and co-extrusion with cancer cells and nanoparticles were used to generate CEVs with the cancer cell membrane and advanced nanoparticles (18, 63).
Before CEVs reach the tumor area, the number of intact EVs determine the therapeutic efficacy of CEVs. The efficacy of CEVs is largely dependent on the stable construction and good targetability after preparation and preservation.
Since the EVs derived from cancer cells can be important promotors of tumor progression, tumor metastasis, immunosuppression, and drug resistance (10–14, 83), irradiation is used for the preparation of therapeutic CEVs to reduce vicious side effects (25, 35, 49, 51, 53, 84). Cancer cells are prone to apoptosis after irradiation, which positively influences the construction of cancer cells membrane and the membrane of CEVs, leading to a damaged stability of CEVs. Besides, the resistance against radiotherapy maybe induced via the communication of irradiated exosomes (85). Additionally, the pH of the reserve solution plays a controversial role in the preservation of CEVs (86, 87), although EVs retain their quality for 16 days at -80°C (88). Studies showed the core-shell structure to construct biomimetic EVs (60, 61) and the hydrogel encapsulated exosomes (89) can result in enhanced stability.
Although the cargo carried by the EVs play a vital role in exerting a suitable therapeutic effect, their efficacy is also determined by their targetability and the effective functioning in the tumor. For the efficacious transport of CEVs to the tumor, the targetability of CEVs could be enhanced via the modification of tumor-targeted-aptamers (62) and peptides (84) on EVs. Along with specific accumulation, the absorption and the anti-tumor activity in the tumor area are also crucial, especially for drug-loaded CEVs. After homologous tumor targeting, the pH-sensitive fusogenic peptides-modified CEVs exerted a membrane-lytic activity in the tumor microenvironment to enhance the effective delivery and release of the cargoes (33).
CD47, on EVs in cancer patients, carries a “don’t eat me” signal (90); thus, CEVs might overexpress CD47 in their parent cancer cells (91–93) to escape the phagocytosis and rapid clearance of macrophages in the serum. Thus, several studies intravenously administered CEVs (19, 21, 23, 26, 51). However, numerous phospholipid bilayer membrane-coated CEVs were also degraded and accumulated in the lung and liver (19, 21). Therefore, an intrapleural administration was adopted to treat lung cancer patients with MPE to avoid unnecessary clearance (25, 52).
In the bright side, the newest researches showed the CEVs were able to be used for the trace of the early stage of neoplastic transformation (94) as well as the intracranial tumor may also can apply the CEVs because they can penetrate the blood-brain barrier (BBB) and have an extraordinarily high brain delivery efficiency (95).
In the clinical experiments, the drug resistance of tumor-repopulating cells can be reversed after the application of CEVs (52). Besides, the autologous chemotherapeutic drugs-loaded CEVs can increase release of IL-2 and IFN-γ and reduce of CD163+ macrophages in malignant pleural effusion (25). Nevertheless, the side effect of transient decrease in the absolute numbers of lymphoid cells could be observed.
However, the low production of CEVs remains a challenge for clinical application. Compared with the CEVs from MPE, the autologous tumor cell-derived extracellular vesicles are not always available in patients with solid tumors for the preparation of CEVs. There is an urgent need for the development of an innovative strategy for the large-scale production of CEVs.
Despite the predetermined notions regarding the role of CEVs based on their contents, the therapeutic role of phospholipid bilayer membrane-coated EVs prepared from cancer cells was explored. The CEVs have shown excellent potential as anti-cancer agents. The strategies employ diverse generation, application, and therapeutic mechanisms. The main strategies for CEVs generation have been summarized in four categories, including irradiated CEVs, advanced materials-combined CEVs, chemotherapeutic drug-loaded CEVs, and genetically engineered CEVs (Table 1). Along with the increasing challenges and advantages of CEVs for cancer therapy, the exploration of CEVs is becoming more and more necessary. This review also discussed and categorized the future perspectives and the top three challenges for the preparation, efficacy, and application of CEVs.
Table 1 The summative evaluation for the preparation, therapeutic mechanism and challenges of cancer cells-derived extracellular vesicles.
WL was in charge of all the writing and graphics. X-DC was in charge of the design and instruction. All authors contributed to the article and approved the submitted version.
The authors declare that the research was conducted in the absence of any commercial or financial relationships that could be construed as a potential conflict of interest.
All claims expressed in this article are solely those of the authors and do not necessarily represent those of their affiliated organizations, or those of the publisher, the editors and the reviewers. Any product that may be evaluated in this article, or claim that may be made by its manufacturer, is not guaranteed or endorsed by the publisher.
1. Meldolesi J. Exosomes and Ectosomes in Intercellular Communication. Curr Biol (2018) 28:R435–44. doi: 10.1016/j.cub.2018.01.059
2. Maacha S, Bhat AA, Jimenez L, Raza A, Haris M, Uddin S, et al. Extracellular Vesicles-Mediated Intercellular Communication: Roles in the Tumor Microenvironment and Anti-Cancer Drug Resistance. Mol Cancer (2019) 18:55. doi: 10.1186/s12943-019-0965-7
3. van Niel G, D’Angelo G, Raposo G. Shedding Light on the Cell Biology of Extracellular Vesicles. Nat Rev Mol Cell Biol (2018) 19:213–28. doi: 10.1038/nrm.2017.125
4. Lotvall J, Hill AF, Hochberg F, Buzas EI, Di Vizio D, Gardiner C, et al. Minimal Experimental Requirements for Definition of Extracellular Vesicles and Their Functions: A Position Statement From the International Society for Extracellular Vesicles. J Extracell Vesicles (2014) 3:26913. doi: 10.3402/jev.v3.26913
5. Liu D, Kou X, Chen C, Liu S, Liu Y, Yu W, et al. Circulating Apoptotic Bodies Maintain Mesenchymal Stem Cell Homeostasis and Ameliorate Osteopenia via Transferring Multiple Cellular Factors. Cell Res (2018) 28:918–33. doi: 10.1038/s41422-018-0070-2
6. Dou G, Tian R, Liu X, Yuan P, Ye Q, Liu J, et al. Chimeric Apoptotic Bodies Functionalized With Natural Membrane and Modular Delivery System for Inflammation Modulation. Sci Adv (2020) 6:eaba2987. doi: 10.1126/sciadv.aba2987
7. Tkach M, Thery C. Communication by Extracellular Vesicles: Where We Are and Where We Need to Go. Cell (2016) 164:1226–32. doi: 10.1016/j.cell.2016.01.043
8. Agrahari V, Agrahari V, Burnouf PA, Chew CH, Burnouf T. Extracellular Microvesicles as New Industrial Therapeutic Frontiers. Trends Biotechnol (2019) 37:707–29. doi: 10.1016/j.tibtech.2018.11.012
9. Mathieu M, Martin-Jaular L, Lavieu G, Thery C. Specificities of Secretion and Uptake of Exosomes and Other Extracellular Vesicles for Cell-to-Cell Communication. Nat Cell Biol (2019) 21:9–17. doi: 10.1038/s41556-018-0250-9
10. Whiteside TL. Exosome and Mesenchymal Stem Cell Cross-Talk in the Tumor Microenvironment. Semin Immunol (2018) 35:69–79. doi: 10.1016/j.smim.2017.12.003
11. Ozawa PMM, Alkhilaiwi F, Cavalli IJ, Malheiros D, de Souza Fonseca Ribeiro EM, Cavalli LR. Extracellular Vesicles From Triple-Negative Breast Cancer Cells Promote Proliferation and Drug Resistance in Non-Tumorigenic Breast Cells. Breast Cancer Res Treat (2018) 172:713–23. doi: 10.1007/s10549-018-4925-5
12. Ma R, Ji T, Chen D, Dong W, Zhang H, Yin X, et al. Tumor Cell-Derived Microparticles Polarize M2 Tumor-Associated Macrophages for Tumor Progression. Oncoimmunology (2016) 5:e1118599. doi: 10.1080/2162402X.2015.1118599
13. Tadokoro H, Hirayama A, Kudo R, Hasebe M, Yoshioka Y, Matsuzaki J, et al. Adenosine Leakage From Perforin-Burst Extracellular Vesicles Inhibits Perforin Secretion by Cytotoxic T-Lymphocytes. PloS One (2020) 15:e0231430. doi: 10.1371/journal.pone.0231430
14. Yamada N, Kuranaga Y, Kumazaki M, Shinohara H, Taniguchi K, Akao Y. Colorectal Cancer Cell-Derived Extracellular Vesicles Induce Phenotypic Alteration of T Cells Into Tumor-Growth Supporting Cells With Transforming Growth Factor-Beta1-Mediated Suppression. Oncotarget (2016) 7:27033–43. doi: 10.18632/oncotarget.7041
15. Ahmadi M, Rezaie J. Tumor Cells Derived-Exosomes as Angiogenenic Agents: Possible Therapeutic Implications. J Transl Med (2020) 18:249. doi: 10.1186/s12967-020-02426-5
16. Liu WL, Zou MZ, Liu T, Zeng JY, Li X, Yu WY, et al. Expandable Immunotherapeutic Nanoplatforms Engineered From Cytomembranes of Hybrid Cells Derived From Cancer and Dendritic Cells. Adv Mater (2019) 31:e1900499. doi: 10.1002/adma.201900499
17. Wang D, Dong H, Li M, Cao Y, Yang F, Zhang K, et al. Erythrocyte-Cancer Hybrid Membrane Camouflaged Hollow Copper Sulfide Nanoparticles for Prolonged Circulation Life and Homotypic-Targeting Photothermal/Chemotherapy of Melanoma. ACS Nano (2018) 12:5241–52. doi: 10.1021/acsnano.7b08355
18. Chen Z, Zhao P, Luo Z, Zheng M, Tian H, Gong P, et al. Cancer Cell Membrane-Biomimetic Nanoparticles for Homologous-Targeting Dual-Modal Imaging and Photothermal Therapy. ACS Nano (2016) 10:10049–57. doi: 10.1021/acsnano.6b04695
19. Qiao L, Hu S, Huang K, Su T, Li Z, Vandergriff A, et al. Tumor Cell-Derived Exosomes Home to Their Cells of Origin and can be Used as Trojan Horses to Deliver Cancer Drugs. Theranostics (2020) 10:3474–87. doi: 10.7150/thno.39434
20. Emam SE, Abu Lila AS, Elsadek NE, Ando H, Shimizu T, Okuhira K, et al. Cancer Cell-Type Tropism Is One of Crucial Determinants for the Efficient Systemic Delivery of Cancer Cell-Derived Exosomes to Tumor Tissues. Eur J Pharm Biopharm (2019) 145:27–34. doi: 10.1016/j.ejpb.2019.10.005
21. Wang D, Yao Y, He J, Zhong X, Li B, Rao S, et al. Engineered Cell-Derived Microparticles Bi2Se3/DOX@MPs for Imaging Guided Synergistic Photothermal/Low-Dose Chemotherapy of Cancer. Adv Sci (Weinh) (2020) 7:1901293. doi: 10.1002/advs.201901293
22. Xu P, Tang K, Ma J, Zhang H, Wang D, Zhu L, et al. Chemotherapeutic Tumor Microparticles Elicit a Neutrophil Response Targeting Malignant Pleural Effusions. Cancer Immunol Res (2020) 8:1193–205. doi: 10.1158/2326-6066.CIR-19-0789
23. Peng J, Zhao J, Zhao Y, Wu P, Gou L, Fu S, et al. HeLa Cell-Derived Paclitaxel-Loaded Microparticles Efficiently Inhibit the Growth of Cervical Carcinoma. Int J Nanomedicine (2020) 15:6409–20. doi: 10.2147/IJN.S246659
24. Qiu Y, Sun J, Qiu J, Chen G, Wang X, Mu Y, et al. Antitumor Activity of Cabazitaxel and MSC-TRAIL Derived Extracellular Vesicles in Drug-Resistant Oral Squamous Cell Carcinoma. Cancer Manag Res (2020) 12:10809–20. doi: 10.2147/CMAR.S277324
25. Guo M, Wu F, Hu G, Chen L, Xu J, Xu P, et al. Autologous Tumor Cell-Derived Microparticle-Based Targeted Chemotherapy in Lung Cancer Patients With Malignant Pleural Effusion. Sci Transl Med (2019) 11(474):eaat5690. doi: 10.1126/scitranslmed.aat5690
26. Ingato D, Edson JA, Zakharian M, Kwon YJ. Cancer Cell-Derived, Drug-Loaded Nanovesicles Induced by Sulfhydryl-Blocking for Effective and Safe Cancer Therapy. ACS Nano (2018) 12:9568–77. doi: 10.1021/acsnano.8b05377
27. Ran L, Tan X, Li Y, Zhang H, Ma R, Ji T, et al. Delivery of Oncolytic Adenovirus Into the Nucleus of Tumorigenic Cells by Tumor Microparticles for Virotherapy. Biomaterials (2016) 89:56–66. doi: 10.1016/j.biomaterials.2016.02.025
28. Li W, Mu D, Tian F, Hu Y, Jiang T, Han Y, et al. Exosomes Derived From Rab27aoverexpressing Tumor Cells Elicit Efficient Induction of Antitumor Immunity. Mol Med Rep (2013) 8:1876–82. doi: 10.3892/mmr.2013.1738
29. Shi X, Cheng Q, Hou T, Han M, Smbatyan G, Lang JE, et al. Genetically Engineered Cell-Derived Nanoparticles for Targeted Breast Cancer Immunotherapy. Mol Ther (2020) 28:536–47. doi: 10.1016/j.ymthe.2019.11.020
30. Bose RJC, Uday Kumar S, Zeng Y, Afjei R, Robinson E, Lau K, et al. Tumor Cell-Derived Extracellular Vesicle-Coated Nanocarriers: An Efficient Theranostic Platform for the Cancer-Specific Delivery of Anti-miR-21 and Imaging Agents. ACS Nano (2018) 12:10817–32. doi: 10.1021/acsnano.8b02587
31. Su MJ, Aldawsari H, Amiji M. Pancreatic Cancer Cell Exosome-Mediated Macrophage Reprogramming and the Role of MicroRNAs 155 and 125b2 Transfection Using Nanoparticle Delivery Systems. Sci Rep (2016) 6:30110. doi: 10.1038/srep30110
32. Purushothaman A, Bandari SK, Chandrashekar DS, Jones RJ, Lee HC, Weber DM, et al. Chondroitin Sulfate Proteoglycan Serglycin Influences Protein Cargo Loading and Functions of Tumor-Derived Exosomes. Oncotarget (2017) 8:73723–32. doi: 10.18632/oncotarget.20564
33. Morishita M, Takahashi Y, Nishikawa M, Ariizumi R, Takakura Y. Enhanced Class I Tumor Antigen Presentation via Cytosolic Delivery of Exosomal Cargos by Tumor-Cell-Derived Exosomes Displaying a pH-Sensitive Fusogenic Peptide. Mol Pharm (2017) 14:4079–86. doi: 10.1021/acs.molpharmaceut.7b00760
34. Jabbari N, Karimipour M, Khaksar M, Akbariazar E, Heidarzadeh M, Mojarad B, et al. Tumor-Derived Extracellular Vesicles: Insights Into Bystander Effects of Exosomes After Irradiation. Lasers Med Sci (2020) 35:531–45. doi: 10.1007/s10103-019-02880-8
35. Wan C, Sun Y, Tian Y, Lu L, Dai X, Meng J, et al. Irradiated Tumor Cell-Derived Microparticles Mediate Tumor Eradication via Cell Killing and Immune Reprogramming. Sci Adv (2020) 6:eaay9789. doi: 10.1126/sciadv.aay9789
36. Li S, Wu Y, Ding F, Yang J, Li J, Gao X, et al. Engineering Macrophage-Derived Exosomes for Targeted Chemotherapy of Triple-Negative Breast Cancer. Nanoscale (2020) 12:10854–62. doi: 10.1039/D0NR00523A
37. Besse B, Charrier M, Lapierre V, Dansin E, Lantz O, Planchard D, et al. Dendritic Cell-Derived Exosomes as Maintenance Immunotherapy After First Line Chemotherapy in NSCLC. Oncoimmunology (2016) 5:e1071008. doi: 10.1080/2162402X.2015.1071008
38. Sun H, Shi K, Qi K, Kong H, Zhang J, Dai S, et al. Natural Killer Cell-Derived Exosomal miR-3607-3p Inhibits Pancreatic Cancer Progression by Targeting IL-26. Front Immunol (2019) 10:2819. doi: 10.3389/fimmu.2019.02819
39. Farcas M, Inngjerdingen M. Natural Killer Cell-Derived Extracellular Vesicles in Cancer Therapy. Scand J Immunol (2020) 92:e12938. doi: 10.1111/sji.12938
40. Tang XJ, Sun XY, Huang KM, Zhang L, Yang ZS, Zou DD, et al. Therapeutic Potential of CAR-T Cell-Derived Exosomes: A Cell-Free Modality for Targeted Cancer Therapy. Oncotarget (2015) 6:44179–90. doi: 10.18632/oncotarget.6175
41. Mothersill C, Seymour C. Radiation-Induced Bystander Effects: Past History and Future Directions. Radiat Res (2001) 155:759–67. doi: 10.1667/0033-7587(2001)155[0759:RIBEPH]2.0.CO;2
42. Karam J, Constanzo J, Pichard A, Gros L, Chopineau J, Morille M, et al. Rapid Communication: Insights Into the Role of Extracellular Vesicles During Auger Radioimmunotherapy. Int J Radiat Biol (2021) 1–10. doi: 10.1080/09553002.2021.1955999
43. Nakaoka A, Nakahana M, Inubushi S, Akasaka H, Salah M, Fujita Y, et al. Exosome-Mediated Radiosensitizing Effect on Neighboring Cancer Cells via Increase in Intracellular Levels of Reactive Oxygen Species. Oncol Rep (2021) 45(4):13. doi: 10.3892/or.2021.7964
44. Prise KM, O’Sullivan JM. Radiation-Induced Bystander Signalling in Cancer Therapy. Nat Rev Cancer (2009) 9:351–60. doi: 10.1038/nrc2603
45. Rzeszowska-Wolny J, Przybyszewski WM, Widel M. Ionizing Radiation-Induced Bystander Effects, Potential Targets for Modulation of Radiotherapy. Eur J Pharmacol (2009) 625:156–64. doi: 10.1016/j.ejphar.2009.07.028
46. Bang OY, Chung JW, Lee MJ, Kim SJ, Cho YH, Kim GM, et al. Cancer Cell-Derived Extracellular Vesicles Are Associated With Coagulopathy Causing Ischemic Stroke via Tissue Factor-Independent Way: The OASIS-CANCER Study. PloS One (2016) 11:e0159170. doi: 10.1371/journal.pone.0159170
47. Hunn MK, Farrand KJ, Broadley KW, Weinkove R, Ferguson P, Miller RJ, et al. Vaccination With Irradiated Tumor Cells Pulsed With an Adjuvant That Stimulates NKT Cells Is an Effective Treatment for Glioma. Clin Cancer Res (2012) 18:6446–59. doi: 10.1158/1078-0432.CCR-12-0704
48. Mortara L, Frangione V, Castellani P, De Lerma Barbaro A, Accolla RS. Irradiated CIITA-Positive Mammary Adenocarcinoma Cells Act as a Potent Anti-Tumor-Preventive Vaccine by Inducing Tumor-Specific CD4+ T Cell Priming and CD8+ T Cell Effector Functions. Int Immunol (2009) 21:655–65. doi: 10.1093/intimm/dxp034
49. Fang C, Mo F, Liu L, Du J, Luo M, Men K, et al. Oxidized Mitochondrial DNA Sensing by STING Signaling Promotes the Antitumor Effect of an Irradiated Immunogenic Cancer Cell Vaccine. Cell Mol Immunol (2020) 18:2211–23. doi: 10.1038/s41423-020-0456-1
50. Stary V, Wolf B, Unterleuthner D, List J, Talic M, Laengle J, et al. Short-Course Radiotherapy Promotes Pro-Inflammatory Macrophages via Extracellular Vesicles in Human Rectal Cancer. J Immunother Cancer (2020) 8(2):e000667. doi: 10.1136/jitc-2020-000667
51. Sun Y, Zheng Z, Zhang H, Yu Y, Ma J, Tang K, et al. Chemotherapeutic Tumor Microparticles Combining Low-Dose Irradiation Reprogram Tumor-Promoting Macrophages Through a Tumor-Repopulating Cell-Curtailing Pathway. Oncoimmunology (2017) 6:e1309487. doi: 10.1080/2162402X.2017.1309487
52. Ma J, Zhang Y, Tang K, Zhang H, Yin X, Li Y, et al. Reversing Drug Resistance of Soft Tumor-Repopulating Cells by Tumor Cell-Derived Chemotherapeutic Microparticles. Cell Res (2016) 26:713–27. doi: 10.1038/cr.2016.53
53. Lin W, Xu Y, Chen X, Liu J, Weng Y, Zhuang Q, et al. Radiation-Induced Small Extracellular Vesicles as “Carriages” Promote Tumor Antigen Release and Trigger Antitumor Immunity. Theranostics (2020) 10:4871–84. doi: 10.7150/thno.43539
54. Nikfarjam S, Rezaie J, Kashanchi F, Jafari R. Dexosomes as a Cell-Free Vaccine for Cancer Immunotherapy. J Exp Clin Cancer Res (2020) 39:258. doi: 10.1186/s13046-020-01781-x
55. Xiang J, Xu L, Gong H, Zhu W, Wang C, Xu J, et al. Antigen-Loaded Upconversion Nanoparticles for Dendritic Cell Stimulation, Tracking, and Vaccination in Dendritic Cell-Based Immunotherapy. ACS Nano (2015) 9:6401–11. doi: 10.1021/acsnano.5b02014
56. Limongi T, Canta M, Racca L, Ancona A, Tritta S, Vighetto V, et al. Improving Dispersal of Therapeutic Nanoparticles in the Human Body. Nanomedicine (Lond) (2019) 14:797–801. doi: 10.2217/nnm-2019-0070
57. Villa CH, Dao T, Ahearn I, Fehrenbacher N, Casey E, Rey DA, et al. Single-Walled Carbon Nanotubes Deliver Peptide Antigen Into Dendritic Cells and Enhance IgG Responses to Tumor-Associated Antigens. ACS Nano (2011) 5:5300–11. doi: 10.1021/nn200182x
58. Jung H, Jang HE, Kang YY, Song J, Mok H. PLGA Microspheres Coated With Cancer Cell-Derived Vesicles for Improved Internalization Into Antigen-Presenting Cells and Immune Stimulation. Bioconjug Chem (2019) 30:1690–701. doi: 10.1021/acs.bioconjchem.9b00240
59. Dumontel B, Susa F, Limongi T, Canta M, Racca L, Chiodoni A, et al. ZnO Nanocrystals Shuttled by Extracellular Vesicles as Effective Trojan Nano-Horses Against Cancer Cells. Nanomedicine (Lond) (2019) 14:2815–33. doi: 10.2217/nnm-2019-0231
60. Nie D, Dai Z, Li J, Yang Y, Xi Z, Wang J, et al. Cancer-Cell-Membrane-Coated Nanoparticles With a Yolk-Shell Structure Augment Cancer Chemotherapy. Nano Lett (2020) 20:936–46. doi: 10.1021/acs.nanolett.9b03817
61. Chen M, Chen M, He J. Cancer Cell Membrane Cloaking Nanoparticles for Targeted Co-Delivery of Doxorubicin and PD-L1 siRNA. Artif Cells Nanomed Biotechnol (2019) 47:1635–41. doi: 10.1080/21691401.2019.1608219
62. Luo C, Hu X, Peng R, Huang H, Liu Q, Tan W. Biomimetic Carriers Based on Giant Membrane Vesicles for Targeted Drug Delivery and Photodynamic/Photothermal Synergistic Therapy. ACS Appl Mater Interfaces (2019) 11:43811–9. doi: 10.1021/acsami.9b11223
63. Fang RH, Hu CM, Luk BT, Gao W, Copp JA, Tai Y, et al. Cancer Cell Membrane-Coated Nanoparticles for Anticancer Vaccination and Drug Delivery. Nano Lett (2014) 14:2181–8. doi: 10.1021/nl500618u
64. Cocozza F, Menay F, Tsacalian R, Elisei A, Sampedro P, Soria I, et al. Cyclophosphamide Enhances the Release of Tumor Exosomes That Elicit a Specific Immune Response In Vivo in a Murine T-Cell Lymphoma. Vaccine (2019) 37:1565–76. doi: 10.1016/j.vaccine.2019.02.004
65. Song J, Jung H, You G, Mok H. Cancer-Cell-Derived Hybrid Vesicles From MCF-7 and HeLa Cells for Dual-Homotypic Targeting of Anticancer Drugs. Macromol Biosci (2021) 21:e2100067. doi: 10.1002/mabi.202100067
66. Goh WJ, Lee CK, Zou S, Woon EC, Czarny B, Pastorin G. Doxorubicin-Loaded Cell-Derived Nanovesicles: An Alternative Targeted Approach for Anti-Tumor Therapy. Int J Nanomedicine (2017) 12:2759–67. doi: 10.2147/IJN.S131786
67. Saari H, Lazaro-Ibanez E, Viitala T, Vuorimaa-Laukkanen E, Siljander P, Yliperttula M. Microvesicle- and Exosome-Mediated Drug Delivery Enhances the Cytotoxicity of Paclitaxel in Autologous Prostate Cancer Cells. J Control Release (2015) 220:727–37. doi: 10.1016/j.jconrel.2015.09.031
68. Haney MJ, Klyachko NL, Zhao Y, Gupta R, Plotnikova EG, He Z, et al. Exosomes as Drug Delivery Vehicles for Parkinson’s Disease Therapy. J Control Release (2015) 207:18–30. doi: 10.1016/j.jconrel.2015.03.033
69. Rosato PC, Wijeyesinghe S, Stolley JM, Nelson CE, Davis RL, Manlove LS, et al. Virus-Specific Memory T Cells Populate Tumors and can be Repurposed for Tumor Immunotherapy. Nat Commun (2019) 10:567. doi: 10.1038/s41467-019-08534-1
70. Goepfert K, Dinsart C, Rommelaere J, Foerster F, Moehler M. Rational Combination of Parvovirus H1 With CTLA-4 and PD-1 Checkpoint Inhibitors Dampens the Tumor Induced Immune Silencing. Front Oncol (2019) 9:425. doi: 10.3389/fonc.2019.00425
71. Oseledchyk A, Ricca JM, Gigoux M, Ko B, Redelman-Sidi G, Walther T, et al. Lysis-Independent Potentiation of Immune Checkpoint Blockade by Oncolytic Virus. Oncotarget (2018) 9:28702–16. doi: 10.18632/oncotarget.25614
72. Kim DH, Kim H, Choi YJ, Kim SY, Lee JE, Sung KJ, et al. Exosomal PD-L1 Promotes Tumor Growth Through Immune Escape in Non-Small Cell Lung Cancer. Exp Mol Med (2019) 51:1–13. doi: 10.1038/s12276-019-0295-2
73. Caponnetto F, Dalla E, Mangoni D, Piazza S, Radovic S, Ius T, et al. The miRNA Content of Exosomes Released From the Glioma Microenvironment Can Affect Malignant Progression. Biomedicines (2020) 8(12):564. doi: 10.3390/biomedicines8120564
74. Li J, Xue J, Ling M, Sun J, Xiao T, Dai X, et al. MicroRNA-15b in Extracellular Vesicles From Arsenite-Treated Macrophages Promotes the Progression of Hepatocellular Carcinomas by Blocking the LATS1-Mediated Hippo Pathway. Cancer Lett (2021) 497:137–53. doi: 10.1016/j.canlet.2020.10.023
75. Xiao L, He Y, Peng F, Yang J, Yuan C. Endometrial Cancer Cells Promote M2-Like Macrophage Polarization by Delivering Exosomal miRNA-21 Under Hypoxia Condition. J Immunol Res (2020) 2020:9731049. doi: 10.1155/2020/9731049
76. Konishi H, Hayashi M, Taniguchi K, Nakamura M, Kuranaga Y, Ito Y, et al. The Therapeutic Potential of Exosomal miR-22 for Cervical Cancer Radiotherapy. Cancer Biol Ther (2020) 21:1128–35. doi: 10.1080/15384047.2020.1838031
77. Khani AT, Sharifzad F, Mardpour S, Hassan ZM, Ebrahimi M. Tumor Extracellular Vesicles Loaded With Exogenous Let-7i and miR-142 can Modulate Both Immune Response and Tumor Microenvironment to Initiate a Powerful Anti-Tumor Response. Cancer Lett (2020) 501:200–9. doi: 10.1016/j.canlet.2020.11.014
78. Mohamed AA, Omar AAA, El-Awady RR, Hassan SMA, Eitah WMS, Ahmed R, et al. MiR-155 and MiR-665 Role as Potential Non-Invasive Biomarkers for Hepatocellular Carcinoma in Egyptian Patients With Chronic Hepatitis C Virus Infection. J Transl Int Med (2020) 8:32–40. doi: 10.2478/jtim-2020-0006
79. Logozzi M, Angelini DF, Iessi E, Mizzoni D, Di Raimo R, Federici C, et al. Increased PSA Expression on Prostate Cancer Exosomes in In Vitro Condition and in Cancer Patients. Cancer Lett (2017) 403:318–29. doi: 10.1016/j.canlet.2017.06.036
80. Ostrowski M, Carmo NB, Krumeich S, Fanget I, Raposo G, Savina A, et al. Rab27a and Rab27b Control Different Steps of the Exosome Secretion Pathway. Nat Cell Biol (2010) 12:19–30; sup pp 1-13. doi: 10.1038/ncb2000
81. Cruz FM, Colbert JD, Rock KL. The GTPase Rab39a Promotes Phagosome Maturation Into MHC-I Antigen-Presenting Compartments. EMBO J (2020) 39:e102020. doi: 10.15252/embj.2019102020
82. Jella KK, Nasti TH, Li Z, Lawson DH, Switchenko JM, Ahmed R, et al. Exosome-Containing Preparations From Postirradiated Mouse Melanoma Cells Delay Melanoma Growth In Vivo by a Natural Killer Cell-Dependent Mechanism. Int J Radiat Oncol Biol Phys (2020) 108:104–14. doi: 10.1016/j.ijrobp.2020.06.016
83. Jabbari N, Akbariazar E, Feqhhi M, Rahbarghazi R, Rezaie J. Breast Cancer-Derived Exosomes: Tumor Progression and Therapeutic Agents. J Cell Physiol (2020) 235:6345–56. doi: 10.1002/jcp.29668
84. Ye Z, Zhang T, He W, Jin H, Liu C, Yang Z, et al. Methotrexate-Loaded Extracellular Vesicles Functionalized With Therapeutic and Targeted Peptides for the Treatment of Glioblastoma Multiforme. ACS Appl Mater Interfaces (2018) 10:12341–50. doi: 10.1021/acsami.7b18135
85. Jabbari N, Nawaz M, Rezaie J. Ionizing Radiation Increases the Activity of Exosomal Secretory Pathway in MCF-7 Human Breast Cancer Cells: A Possible Way to Communicate Resistance Against Radiotherapy. Int J Mol Sci (2019) 20(15):3649. doi: 10.3390/ijms20153649
86. Cheng Y, Zeng Q, Han Q, Xia W. Effect of Ph, Temperature and Freezing-Thawing on Quantity Changes and Cellular Uptake of Exosomes. Protein Cell (2019) 10:295–9. doi: 10.1007/s13238-018-0529-4
87. Zhao Y, Chen K, Li H, Wu H. Effect of pH on the Isolation of Urinary Exosome. Int Urol Nephrol (2017) 49:165–9. doi: 10.1007/s11255-016-1408-7
88. Richter M, Fuhrmann K, Fuhrmann G. Evaluation of the Storage Stability of Extracellular Vesicles. J Vis Exp (2019) 147. doi: 10.3791/59584
89. Akbari A, Jabbari N, Sharifi R, Ahmadi M, Vahhabi A, Seyedzadeh SJ, et al. Free and Hydrogel Encapsulated Exosome-Based Therapies in Regenerative Medicine. Life Sci (2020) 249:117447. doi: 10.1016/j.lfs.2020.117447
90. Kang KW, Kim H, Hur W, Jung JH, Jeong SJ, Shin H, et al. A Proteomic Approach to Understand the Clinical Significance of Acute Myeloid Leukemia-Derived Extracellular Vesicles Reflecting Essential Characteristics of Leukemia. Mol Cell Proteomics (2020) 20:100017. doi: 10.1074/mcp.RA120.002169
91. Lian S, Xie R, Ye Y, Xie X, Li S, Lu Y, et al. Simultaneous Blocking of CD47 and PD-L1 Increases Innate and Adaptive Cancer Immune Responses and Cytokine Release. EBioMedicine (2019) 42:281–95. doi: 10.1016/j.ebiom.2019.03.018
92. Lu Y, Liang H, Yu T, Xie J, Chen S, Dong H, et al. Isolation and Characterization of Living Circulating Tumor Cells in Patients by Immunomagnetic Negative Enrichment Coupled With Flow Cytometry. Cancer (2015) 121:3036–45. doi: 10.1002/cncr.29444
93. Kamerkar S, LeBleu VS, Sugimoto H, Yang S, Ruivo CF, Melo SA, et al. Exosomes Facilitate Therapeutic Targeting of Oncogenic KRAS in Pancreatic Cancer. Nature (2017) 546:498–503. doi: 10.1038/nature22341
94. Garofalo M, Villa A, Brunialti E, Crescenti D, Dell’Omo G, Kuryk L, et al. Cancer-Derived EVs Show Tropism for Tissues at Early Stage of Neoplastic Transformation. Nanotheranostics (2021) 5:1–7. doi: 10.7150/ntno.47226
Keywords: cancer cell-derived extracellular vesicles, cancer therapy, nanomedicine, drug delivery, advanced materials
Citation: Lin W and Cai X-D (2021) Current Strategies for Cancer Cell-Derived Extracellular Vesicles for Cancer Therapy. Front. Oncol. 11:758884. doi: 10.3389/fonc.2021.758884
Received: 15 August 2021; Accepted: 14 October 2021;
Published: 05 November 2021.
Edited by:
Xiangbing Meng, The University of Iowa, United StatesReviewed by:
Elinaz Akbariazar, Islamic Azad University North Tehran Branch, IranCopyright © 2021 Lin and Cai. This is an open-access article distributed under the terms of the Creative Commons Attribution License (CC BY). The use, distribution or reproduction in other forums is permitted, provided the original author(s) and the copyright owner(s) are credited and that the original publication in this journal is cited, in accordance with accepted academic practice. No use, distribution or reproduction is permitted which does not comply with these terms.
*Correspondence: Xing-Dong Cai, Y3hkMTk3OTA5MjBAc2luYS5jb20=
Disclaimer: All claims expressed in this article are solely those of the authors and do not necessarily represent those of their affiliated organizations, or those of the publisher, the editors and the reviewers. Any product that may be evaluated in this article or claim that may be made by its manufacturer is not guaranteed or endorsed by the publisher.
Research integrity at Frontiers
Learn more about the work of our research integrity team to safeguard the quality of each article we publish.