- 1Department of Gastroenterology, Affiliated Hospital of Guilin Medical University, Guilin, China
- 2Department of Gastroenterology, The First Affiliated Hospital of Guangxi Medical University, Nanning, China
- 3Department of Oncology, Affiliated Hospital of Guilin Medical University, Guilin, China
- 4Department of Pathology, Affiliated Hospital of Guilin Medical University, Guilin, China
Liver cancer is the fifth most common malignant tumor in terms of incidence and the third leading cause of cancer-related mortality globally. Hepatocellular carcinoma (HCC) is the most common type of primary liver cancer. Although great progress has been made in surgical techniques, hepatic artery chemoembolization, molecular targeting and immunotherapy, the prognosis of liver cancer patients remains very poor. N6-methyladenosine (m6A) is the most abundant internal RNA modification in eukaryotic cells and regulates various stages of the RNA life cycle. Many studies have reported that the abnormal expression of m6A-related regulators in HCC represent diagnostic and prognostic markers and potential therapeutic targets. In this review, firstly, we introduce the latest research on m6A-related regulators in detail. Next, we summarize the mechanism of each regulator in the pathogenesis and progression of HCC. Finally, we summarize the potential diagnostic, prognostic and therapeutic value of the regulators currently reported in HCC.
Introduction
Liver cancer is the fifth most common malignant tumor in terms of incidence and the third leading cause of cancer-related mortality globally (1). Among all primary liver cancers, hepatocellular carcinoma (HCC) is the most common, accounting for about 75–85% (1). Risk factors for liver cancer include viral infection (hepatitis B and C), fatty liver, heavy drinking, smoking, obesity, diabetes and aflatoxin contamination of food (2). Most patients are not candidates for curative treatments (such as surgical resection or liver transplantation) at diagnosis because of the extent or distribution of the tumor, underlying liver function, or medical comorbidities (3). The tyrosine multikinase inhibitors sorafenib and regorafenib were the first drugs approved as first- and second-line treatments, respectively, for advanced liver cancer. Although regorafenib can improve the survival rate (from 7.8 to 10.6 months) among patients whose tumors have progressed during sorafenib treatment, the side effects include impairment of liver function and performance status (PS) (4, 5). Worse still, the response rate among HCC patients to nivolumab, a programmed cell death protein-1 (PD-1) immune checkpoint inhibitor, is ≤20% (6). Thus, there is an urgent need for earlier diagnosis and new effective therapies.
Among the more than 170 types of RNA modification, m6A is the most abundant internal RNA modification in eukaryotic cells and it is related to almost every step of RNA metabolism (7). The modification is involved in all events in the entire life cycle of RNA molecules, including splicing, transportation, degradation, stability and translation (8). Consensus motif analyses revealed that m6A modification sites, known as DRACH motifs (R = G or A; H = A, C, or U; A is converted to m6A), in the transcriptome are not randomly distributed. Instead, they occur in coding sequences (CDS), 3’-untranslated regions (3’-UTRs) and the regions around stop codons (9, 10). RNA m6A modification is dynamically and reversibly regulated by methyltransferases (“writers”) and demethylases (“erasers”). These modifications are recognized by a group of binding proteins (“readers”) that recognize specific m6A-modified positions and subsequently regulate RNA functions (11).
The functions of m6A modification in mammals include the regulation of tissue development, circadian rhythm, DNA damage response, sex determination, T cell homeostasis and tumorigenesis (12, 13). There is a mounting number of studies showing that m6A dysregulation is critical in a variety of human cancers, including HCC (14–19). Furthermore, several m6A-related regulators have shown clinical value as biomarkers or therapeutic targets in HCC (20–24). Researches shown that m6A modification related to the etiology of HCC, including viral hepatitis and non- alcoholic fatty liver disease (NAFLD) (25).
Here, we summarized the physiological functions of m6A-related regulators and the potential role of m6A modification in HCC.
Regulators of m6A Modification
The m6A-related regulators, comprising writers, erasers and readers, cooperatively maintain the dynamic and reversible balance of m6A methylation (12). Summaries of the functions of m6A-related regulators in RNA metabolism are shown in Figure 1 and Table 1.
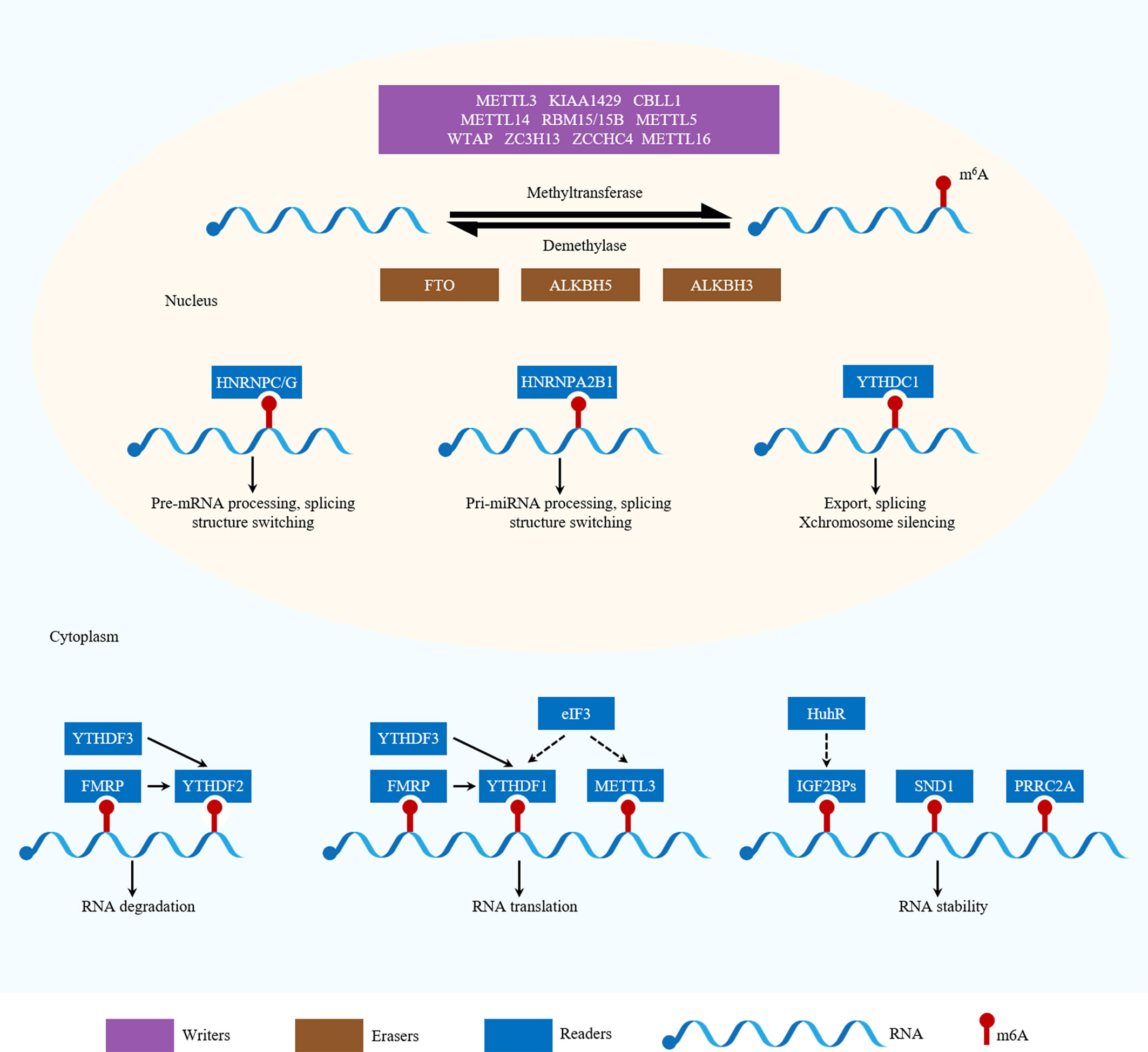
Figure 1 Functions of m6A-related regulators in RNA metabolism. m6A is catalyzed by writers and removed by erasers. METTL3, METTL14, WTAP, KIAA1429, RBM15/15B, ZC3H13, CBLL1, METTL5, ZCCHC4 and METTL16 are writers. ALKBH5, ALKBH3 and FTO are erasers. YTHDF1, YTHDF2, YTHDF3, YTHDC1, YTHDC2, hnRNPC/G, hnRNPA2B1, FMRP, eIF3, IGF2BPs, SND1 and PRRC2A are readers. Different readers binding to m6A sites can produce different biological effects.
Writers
Writers, including methyltransferase-like 3 (METTL3), methyltransferase-like 14 (METTL14) and Wilm’s tumor 1-associated protein (WTAP), are the major components of the methyltransferase complex (MTC) in the nucleus, which is responsible for the m6A methylation process (11). METTL3 is the core catalytic enzyme for transferring methyl groups to N6 positions, while METTL14 is vital for structural stabilization of the METTL3–METTL14 complex and is involved in RNA substrate recognition (26). However, a few studies have shown that METTL3 can also act as an m6A cytoplasmic reader. METTL3 associates with ribosomes or directly and promotes the translation of certain mRNAs, including Epidermal growth factor receptor (EGFR) and the Hippo pathway effector Transcriptional co-activator with PDZ-binding motif (TAZ), independently of its methyltransferase activity or downstream m6A reader proteins (27). It does this by recruiting eukaryotic translation initiation factor 3 subunit H (eIF3h) to the translation initiation complex, thus promoting cancer cell growth, survival and invasion (28). As a regulatory subunit, WTAP ensures METTL3–METTL14 localization to the nuclear speckle and promotes catalytic activity. Additionally, WTAP may regulate MTC recruitment to mRNA targets (29).
Other identified writers include KIAA1429 (also known as Vir-like m6A methyltransferase-associated [VIRMA]), RNA-binding motif protein 15 and 15B (RBM15 and RMB15B), zinc finger CCCH domain-containing protein 13 (ZC3H13) and Cbl proto-oncogene-like 1 (CBLL1; also known as HAKAI). KIAA1429 preferentially mediates mRNA methylation in 3′-UTRs and near stop codons and is associated with alternative polyadenylation (30). RMB15 and RMB15B, two other WTAP interactors, can recruit the MTC to specific sites, thus mediating m6A methylation of the long non-coding RNA (lncRNA) X-inactive-specific transcript (XIST) (31). ZC3H13 and CBLL1, in concert with additional cofactors, help to regulate nuclear m6A methylation (32). Furthermore, methyltransferase-like 16 (METTL16) was proposed to act as an independent mRNA methyltransferase in 2017 (33). It induces m6A modification in the 3’-UTR of mRNAs and at A43 in the U6 small nuclear RNA (snRNA), regulating tumorigenesis by targeting pre-mRNAs and non-coding RNAs (ncRNAs) (33, 34). Recently, it has been reported that methyltransferase-like 5 (METTL5) and zinc finger CCCH domain- containing protein 4 (ZCCHC4) are responsible for modifying 18S rRNA (35)and 28S rRNA (36), respectively. METTL5 is stabilized by transfer RNA (tRNA) methyltransferase 112 (TRMT112) (35). In addition, phosphorylated CTD- interacting factor 1 (PCIF1) mediates the N6, 2’-O-dimethyladenosine (m6Am) modification, an evolutionarily conserved mRNA modification (37). This involves catalyzing m6A methylation of 2-O-methylated adenines at the 5’ ends of mRNA (38). Furthermore, methyltransferase-like 4 (METTL4) has been identified as a novel internal m6Am methyltransferase that targets U2 snRNA, which regulates pre-mRNA splicing (39).
Erasers
It was not until 2011 that scientists discovered that m6A modification could be reversed by RNA demethylases (also known as m6A erasers), which drew the attention of the broader academic community (40). These RNA demethylases include fat mass and obesity-associated protein (FTO) and AlkB homolog 5 (ALKBH5). ALKBH5 catalyzes the direct removal of m6A modifications, while FTO can sequentially oxidize m6A to N6-hydroxymethyladenosine (hm6A) and N6-formyladenosine (f6A), which are moderately stable and can later be hydrolyzed to adenine (40, 41). In addition, recent studies have shown that AlkB homolog 3 (ALKBH3) may serve as a novel demethylase that reverses m6A modifications (42). m6A-modified mammalian tRNA has been identified as a novel ALKBH3 substrate, highlighting a novel role for ALKBH3 in tumor progression via RNA demethylation and subsequent promotion of protein synthesis (43).
Readers
M6A readers recognize and bind to m6A sites, altering the destinies of their target RNAs. They can also influence mRNA by destabilizing its structure, which can affect the binding of diverse RNA-binding proteins. Pull-down assays using a methylated probe and quantitative protein mass spectrometry assays have identified multiple m6A-binding proteins. The functions of m6A modification, which include regulating RNA splicing, export, degradation, stability and translation, are mainly exerted via the recruitment of these m6A readers. There are three classes of these proteins.
The class I m6A readers are YTH domain-containing proteins (YTH domain- containing family protein 1/2/3 [YTHDF1/2/3] and YTH domain-containing1/2 [YTHDC1/2]). YTHDF2 was the first identified and is the most studied m6A reader. Nuclear YTHDF2 binds to m6A sites in the 5’-UTR, prevents demethylation by FTO and thereby promotes cap-independent translation during the heat shock response (45). Cytoplasmic YTHDF2 promotes degradation of its target mRNAs partly by recruiting the CCR4-NOT deadenylase complex (46). Independently, YTHDF1 binding promotes the translation of m6A-modified mRNAs by recruiting the eukaryotic initiation factor 3 (eIF3) translation initiation complex (44). YTHDF3, in synergy with YTHDF1, facilitates translation and it also affects YTHDF2-mediated mRNA decay (47). All three YTHDF proteins function cooperatively in fundamental biological pathways (72). Moreover, nuclear YTHDC1 has been reported to regulate mRNA splicing, expedite mRNA export (48) and accelerate the decay of certain mRNAs (49). Additionally, this protein preferentially recognizes m6A residues in the lncRNA XIST and is required for XIST-mediated transcriptional silencing (31). Furthermore, YTHDC2 can be both nuclear and cytosolic (50–52). It was found to interact with RNA helicase to positively regulate translation elongation in an m6A-dependent manner (50). Additionally, YTHDC2 can mediate mRNA degradation by recruiting the 5′–3′ exoribonuclease XRN1 (51).
The class II m6A readers are three heterogeneous nuclear ribonucleoproteins (hnRNPs): hnRNPC, hnRNPG and hnRNPA2B1. These proteins can remodel the local RNA structure in an m6A-dependent manner and, consequently, modulate nearby RNA–protein interactions, in a mechanism known as an “m6A-switch”. hnRNPC regulates pre-mRNA processing and alternative splicing (53), modulating the alternative splicing of nearby exons (54). In contrast, hnRNPA2B1 is a nuclear reader that recognizes primary (pri)-miRNA m6A modifications and subsequently stimulates miRNA processing and alternative splicing (55, 56).
The class III m6A readers are insulin-like growth factor 2 mRNA-binding proteins (IGF2BPs), including IGF2BP1-3. These proteins are a distinct family of cytoplasmic m6A readers. They recognize the GG(m6A)C consensus sequence via their K homology (KH) domains and enhance the stability and translation of their target mRNAs under normal and stress conditions (57). These proteins function by recruiting RNA stabilizers, such as Hu antigen R (HuR), which is an indirect m6A effector with a preference for less m6A-modified transcripts, in order to maintain mRNA stability (58–60). Specifically, by promoting the expression of serum response factor (SRF) in an m6A-dependent manner via impairing the miRNA-dependent decay of the SRF mRNA, IGF2BP1 promotes SRF-dependent transcription in cancer, thereby enhancing tumor cell growth and invasion. At the post-transcriptional level, IGF2BP1 maintains the expression of multiple SRF target genes, including PDZ and LIM domain 7 (PDLIM7) and forkhead box K1 (FOXK1), which further enhances tumor cell growth and invasion (61). IGF2BP2 regulates the m6A-modified lncRNA DANCR to promote cancer stemness-like properties and cancer pathogenesis (62). Lastly, IGF2BP3 regulates RNA stability, RNA degradation, RNA localization and miRNA biogenesis, but the exact molecular processes underlying these functions have only begun to be elucidated (60, 63). Furthermore, several novel m6A readers have been identified, including fragile X mental retardation protein (FMRP), leucine-rich pentatricopeptide-repeat containing (LRPPRC), the eIF3 complex, staphylococcal nuclease domain-containing protein 1 (SND1), proline rich coiled-coil 2A (PRRC2A), and ribosomes. FMRP contains three KH domains and one arginine–glycine–glycine (RGG) domain and has been shown to prefer m6A-containing RNA, repressing target mRNA translation and maintaining target mRNA stability, likely by interacting with YTHDF1 and YTHDF2 (64, 65). LRPPRC is indispensable for maintaining the pool of non-translated transcripts and for regulation of mitochondrial translation (66, 67). The eIF3 complex is sufficient to recruit the 43S complex to initiate translation in the absence of the cap-binding factor eIF4E (68) and eIF3h also interacts with cytoplasmic METTL3 to bring about enhanced cap-dependent translation, the formation of densely packed polyribosomes and oncogenic transformation (28). SND1 is reported to be an m6A reader that stabilizes open reading frame 50 (ORF50) RNA and is essential for Kaposi’s sarcoma associated herpesvirus (KSHV) replication (69). PRRC2A plays an important role in oligodendrocyte specification by stabilizing m6A-modified oligodendrocyte lineage transcription factor 2 (Olig2) mRNA (70). Lastly, ribosomes may also act as m6A readers. Single-molecule ribosome translocation experiments have shown that an mRNA molecule containing a single-base m6A modification can change the translation dynamics by ribosomes in Escherichia coli (e.g., acting as a barrier to translation elongation) (71). However, the effects of this mechanism on the stability and/or translation of m6A-modified mRNA need to be further investigated.
In summary, the intricate interactions between m6A modifications and RNA-binding proteins can regulate mRNA expression at multiple levels. Further investigation of these proteins and their dynamic roles in cancer biology is necessary to deepen our understanding of RNA methylation. The findings may provide novel insights into the mechanisms of cancer pathogenesis and potential therapeutic strategies.
Implications of m6A Modification in HCC
Owing to advances in RNA sequencing, a growing number of studies have shown that m6A modification plays an important role in human cancer progression. Genes encoding m6A-related regulators can act as oncogenes or tumor suppressor genes. The expression of these regulators can be affected by various factors, such as tumor heterogeneity, the presence of hypoxic microenvironments and post-translational modifications, which affect their function in cancer. The specific roles of m6A-related regulators in HCC are summarized in Figure 2 and Table 2.
Writers and HCC
As the most important component of the m6A MTC, the role of METTL3 in HCC has been widely reported. METTL3 is upregulated in HCC and high METTL3 expression is associated with poor HCC prognosis. It can promote the growth, migration and invasion of cancer cells both in vitro and in vivo, serving as an oncogene through various mechanisms (19, 20, 73, 74). First, Chen et al. reported that METTL3 facilitates HCC tumorigenicity, growth and lung metastasis in vivo in an m6A-YTHDF2-dependent manner by promoting the degradation of suppressor of cytokine signaling 2 (SOCS2) mRNA (19). Second, Lin et al. found that METTL3 regulates cancer cell epithelial–mesenchymal transition (EMT) by upregulating Snail (a key transcription factor in EMT) through the m6A-YTHDF1 pathway (73). Third, Zuo et al. showed that METTL3 enhances the expression and stability of LINC00958, which targets miR-3619-5p in order to upregulate hepatoma-derived growth factor (HDGF), thereby facilitating HCC lipogenesis and progression (75). Fourth, Chen et al. demonstrated that METTL3 overexpression markedly reduces RAD52 motif 1 (RDM1) mRNA expression via m6A modification; RDM1 knockdown increases HCC cell proliferation, colony formation and the cell population at the G2/M phase, as RDM1 serves as a tumor suppressor in HCC (76). Fifth, Lin et al. reported that METTL3 is involved in the regulation of glycolysis in HCC via regulating mTORC1 activity (104). Sixth, Qiao et al. found that METTL3 promotes vasculogenic mimicry in HCC via the Hippo pathway by facilitating the translation of Yes-associated protein 1 (YAP1) mRNA (74). Seventh, Xu et al. demonstrated that METTL3 can be SUMOylated by the small ubiquitin-like modifier SUMO and subsequently controls Snail mRNA homeostasis in an m6A methyltransferase activity-dependent manner, thus promoting HCC progression (77). Moreover, METTL3 is significantly downregulated in human sorafenib-resistant HCC. Depletion of METTL3 in mouse xenograft models increases sorafenib resistance in HCC by decreasing the stability of FOXO3 mRNA (without METTL3 depletion, METTL3 causes m6A modification of FOXO3 mRNA, increasing its stability via a YTHDF1-dependent mechanism) (105).
Similarly, WTAP is highly expressed in HCC tissue and is an independent predictor of survival in HCC patients (78, 106). WTAP suppresses ETS proto-oncogene 1 (ETS1) in an m6A-HuR-dependent manner, which upregulates the cell cycle regulators p21 and p27 to promote the G2/M phase in HCC cells, thus increasing proliferation (78). KIAA1429 is elevated in HCC and high KIAA1429 expression is associated with poor HCC prognosis (79, 80). It promotes HCC cell migration and invasion by altering the m6A modification of inhibitor of DNA binding 2 (ID2) and GATA binding protein 3 (GATA3) mRNA (79, 80). Relatedly, circ_KIAA1429 (hsa_ circ_0084922) was upregulated in HCC cells and tissues. Circ_KIAA1429, which maintains zinc finger E-box binding homeobox 1 (ZEB1) expression via an m6A-YTHDF3-ZEB1 mechanism in HCC, can facilitate HCC cell migration, invasion and EMT (93). Furthermore, RBM15B was also reported to be dramatically upregulated in human HCC (19).
Unlike other m6A methyltransferases, METTL14 was downregulated in HCC, and low METTL14 protein expression is associated with shorter overall survival (OS) and recurrence-free survival (17, 92, 107). Ma et al. (17) reported that METTL14 suppressed HCC invasion and metastasis by interacting with the micro-processor protein DiGeorge syndrome key region gene 8 (DGCR8) and positively promoting primary miR-126 maturation in an m6A-dependent manner. Likewise, Liu et al. (108) and Li et al. (109) analyzed data from The Cancer Genome Atlas (TCGA) and Gene Expression Omnibus (GEO) databases and found that METTL14 was significantly downregulated in HCC. METTL14 may inhibit HCC progression by altering the m6A modification of cysteine sulfonic acid deacidiase (CSAD), glutamic-oxaloacetic transaminase 2 (GOT2) and SOCS2 (109). In contrast, Chen et al. (19) reported that METTL14 was unchanged in HCC in their study, but METTL14 knockdown significantly suppressed Huh-7 cell proliferation, migration and colony formation (19).
Given the paradoxical expression patterns and roles of METTL14 in prior studies of HCC, Zhang et al. (110) analyzed paired HCC and normal samples in multiple microarray datasets. Unexpectedly, their results indicated that METTL14 may have a variety of roles as opposed to only being a tumor suppressor or promoter in hepatocarcinogenesis. They ascribed the contradictory findings regarding the METTL14 expression pattern and role in HCC samples compared to normal samples to the heterogeneity of HCC cell lines and clinical samples, the versatility of the METTL3-METTL14 heterocomplex, and m6A-independent effects of METTL14. Additionally, METTL16 is a newly identified RNA methyltransferase that operates independently of the MTC. Wang et al. (111) carried out a bioinformatics analysis using TCGA data and reported that METTL16 was downregulated in HCC and that low METTL16 expression was associated with poor OS and disease-free survival. Another new m6A methyltransferase, ZCCHC4, was overexpressed in HCC, and ZCCHC4 knockout eliminated m6A modification of 28S rRNA, inhibited HepG2 cell proliferation and reduced the tumor size in a xenograft mouse model (81).
In summary, these results reveal that m6A writers, including those in the MTC, regulate HCC progression. Except for METTL16 and METTL14, the m6A writers are upregulated in liver cancer tissues and act as oncogenes. Further investigations are required to resolve the contradictions.
Erasers and HCC
The expression and role of FTO in liver cancer is controversial. Li et al. (82) reported that upregulated FTO was associated with poor HCC prognosis and demonstrated that FTO can induce HCC tumorigenesis by regulating pyruvate kinase M2 (PKM2) in an m6A modification-dependent manner. However, in addition to its oncogenic function, FTO also suppresses HCC progression. Ma et al. (17) and Hou et al. (92) reported that FTO was downregulated in liver cancer tissues. More specifically, Ma et al. (17) observed that METTL14 and FTO were downregulated in cancer tissues compared to adjacent tissues and there was a correlation between them. Although both proteins are expressed in the nucleus, there is little interaction; METTL14 silencing or overexpression had no significant effect on FTO expression. Despite this, Ma et al. suggested that the eventual FTO downregulation in liver cancer with reduced METTL14 expression may involve a compensatory feedback mechanism. Later, Liu et al. (83) reported that FTO is negatively regulated by the deacetylase SIRT1 via RANBP2-mediated SUMOylation; its downregulation reduces the expression of its target gene guanine nucleotide-binding protein G(O) subunit alpha (GNAO1), thereby promoting the progression of liver cancer. Moreover, a subsequent study revealed that FTO might target Cul4a mRNA to downregulate CUL4A protein, thereby presumably blocking HCC cell cycle progression and proliferation (84).
ALKBH5, another m6A demethylase, was downregulated in HCC, and decreased ALKBH5 expression was an independent prognostic factor associated with poor survival in HCC patients (85). ALKBH5-mediated m6A demethylation suppresses proliferation and invasion by suppressing IGF2BP1-mediated LY6/PLAUR domain-containing 1 (LYPD1) RNA stability (85). Additionally, Liu et al. (112) reported that ALKBH5 inhibits autophagy in sorafenib-treated HCC cells and can be inhibited by an important circRNA known as cIARS (hsa_circ_0008367). Moreover, recent research has identified another m6A demethylase, ALKBH3 (43). Wang et al. (86) reported that ALKBH3 was overexpressed in HCC and high ALKBH3 expression in HCC tissues decreased OS and disease-free survival in HCC patients. ALKBH3 knockdown inhibited human HCC cell proliferation in vitro and xenograft tumor formation in vivo, presumably through p21/p27-mediated cell cycle arrest at the G1 phase (86).
In summary, these results suggest that m6A erasers are involved in regulating HCC progression, but some of the conclusions are contradictory, suggesting a complex role of m6A modification in HCC.
Readers and HCC
Like writers and erasers, multiple m6A readers have also been implicated in liver cancer. Many studies have reported upregulation of YTHDF1 in liver cancer tissues and its overexpression is associated with poor HCC prognosis. It promotes HCC cell proliferation and metastasis both in vitro and in vivo (21, 88, 113–115). Regarding the underlying mechanism, Ding et al. (87) found that high expression of G-protein alpha-subunit (GNAS) promotes lipopolysaccharide-induced HCC cell growth and invasion by interacting with signal transducer and activator of transcription 3 (STAT3) in an m6A-YTHDF1-dependent manner. Additionally, Lin et al. (73) reported that YTHDF1 mediates m6A-induced translation of Snail mRNA, which triggers polysome-mediated translation and regulates EMT in cancer cells. Bian et al. (21) identified YTHDF1 as an HCC oncogene that facilitates EMT and AKT/GSK3β/β-catenin signaling. Liu et al. (88) revealed that YTHDF1 can act as an oncogene by mediating the m6A-dependent acceleration of the translation of FZD5 mRNA, which is involved in the WNT/β-catenin pathway. Nevertheless, there are conflicting opinions on YTHDF1 expression in liver cancer tissues, as Hou et al. (92) assessed YTHDF1 mRNA levels in 51 paired HCC and paracancerous tissues by qRT-PCR and found no significant difference between the two tissue types. Furthermore, the role of YTHDF2 in liver cancer is controversial. Yang et al. (89) found that miR-145 modulates m6A levels by targeting the 3’-UTR of YTHDF2 mRNA in HCC cells. As miR-145 is frequently downregulated in HCC and targets YTHDF2, upregulated YTHDF2 in HCC appears to be closely related to the malignancy of HCC. Additionally, Zhang et al. (90) reported that YTHDF2 was a predictor of poor HCC prognosis and it promotes the liver cancer stem cell phenotype and metastasis by upregulating octamer-binding transcription factor-4 (OCT4) in an m6A-dependent manner. Nevertheless, YTHDF2 may also act as a tumor suppressor, as Zhong et al. (91) and Hou et al. (92) reported that it was downregulated by hypoxia in HCC. The former study found that YTHDF2 directly binds to the m6A modification site of EGFR mRNA 3’-UTR to promote mRNA degradation, thereby suppressing HCC cell proliferation and tumor growth (91). The latter study found that YTHDF2 was downregulated in HCC by hypoxia-inducible factor (HIF)-2α, which reduced the degradation of m6A-containing interleukin 11 (IL11) and serpin family E member 2 (SERPINE2) mRNAs, leading to inflammation-mediated malignancy and disruption of vascular normalization (92). Moreover, another YTH domain protein, YTHDF3, is upregulated in HCC and increases ZEB1 mRNA stability in an m6A-dependent manner (93). ZEB1 is the downstream target of circ_KIAA1429 and its upregulation leads to HCC metastasis. In summary, these contradictory functions may be related to the tumor heterogeneity and/or the small sample sizes used.
The roles of the three hnRNPs, which are class II m6A readers, in liver cancer have rarely been studied. However, trichostatin A-induced lncRNA-uc002mbe.2 directly bound to hnRNPA2B1 and promoted its degradation. This hnRNPA2B1 downregulation contributed to AKT deactivation and p21 upregulation, resulting in liver cancer cell apoptosis and the inhibition of proliferation in vitro and in vivo (116).
Regarding class III m6A readers, IGF2BPs are highly expressed in HCC, playing oncogenic roles in HepG2 cells by enhancing MYC mRNA stability and post-transcriptionally upregulating target gene expression (59). Consistently, IGF2BP1 upregulates SRF in an m6A-dependent manner by decreasing the miRNA-mediated decay of SRF mRNA, which subsequently leads to increased PDLIM7 and FOXK1 translation. This results in tumor cell growth and invasion, leading to a poor OS in liver cancer patients (61). Gong et al. (117) reported that inhibition of fatty acid synthase (FASN) decreases IGF2BP1 expression, along with HIF-1α activity, thereby suppressing HCC cell migration and invasion. Moreover, He et al. (118) demonstrated that IGF2BP1 interacts with glioma-associated oncogene homologue 1 (GLI1) mRNA, which is involved in HCC progression; the liver-specific lncRNA LINC01093 disrupts this interaction. The above studies suggest that IGF2BP1 upregulation plays an oncogenic role. However, it has also been reported that IGF2BP1 downregulation can promote tumor progression (94, 119). Geis et al. (94) identified and verified IGFBP1 as a target gene of the transcription factor HIF-2α, and silencing of IGF2BP1 significantly enhanced the potential of HepG2 cells to induce lymphangiogenesis. In addition, Nielson et al. (119) reported that hepatitis B virus (HBV) suppresses IGF2BP1 secretion to facilitate pro-survival and anti-apoptotic insulin-like growth factor (IGF)-1 activity. Adding recombinant IGF2BP1 reversed the anti-apoptotic effect in HepG2 cells.
Regarding IGF2BP2, it was upregulated in HCC patients compared to healthy controls, and IGF2BP2 expression was positively associated with decreased tumor differentiation and increased size, metastasis and portal vein infiltration in HCC (95). Moreover, exogenous IGF2BP2 promoted the integrin β1/FAK/Erk/Elk1/EGR1 pathway, which stimulated HCC cell proliferation (95). Furthermore, Liu et al. (96) showed that HBV X protein (HBx) in HepG2 cells downregulated miR-216b and thereby upregulated IGF2BP2, which activated the downstream insulin-like growth factor 2 (IGF-2), PI3K/AKT and MAPK/ERK signaling pathways, thus promoting cell proliferation and invasion. Regarding IGF2BP3, it has been reported to be a downstream oncogenic effector of LIN28B, whose overexpression drives liver tumorigenesis in murine models (97). In contrast, Wang et al. (98) reported that sanguinarine can upregulate IGF2BP3 in Hep3B cells and thereby promote apoptosis. Similarly, it was shown that IGFBP3 can inhibit HCC cell proliferation by suppressing transcription of early growth response protein 1 (EGR1) and its target genes basic fibroblast growth factor (bFGF) and platelet-derived growth factor (PDGF) (99).
Increasing numbers of new m6A readers are being identified. In eukaryotes, the eIF3 complex, composed of 13 subunits from eIF3a to eIF3m, is the largest and most complex translation initiation factor. eIFs play major roles in the initiation step of protein translation (100). M6A modifications in the 5′-UTR of transcripts can directly recruit eIF3, contributing to the assembly of translation initiation complexes on eIF3-specialized mRNAs (68). Golob-Schwarzl et al. (120) reported that various eIF3 subunits were significantly increased in chronic HBV-associated HCC. Recently, eIF3a has been recognized as a proto-oncogene, which is overexpressed in HCC and linked to HCC tumorigenesis (100, 101, 121). Heo et al. (121) reported that eIF3a is significantly upregulated in HCC tissue compared to normal tissue in mice and patients and combined detection of anti-eIF3a autoantibody and alpha-fetoprotein (AFP) in patient sera improved the accuracy of HCC diagnosis. Additionally, Miao et al. (100) found that eIF3a regulates cellular glycolysis by increasing HIF-1α protein expression via internal ribosomal entry site (IRES)-dependent translation and eIF3a predicts poor HCC prognosis. Moreover, Chen et al. (101) demonstrated that eIF3a had an oncogenic role and was upregulated and negatively correlated with miR-875-5p expression in HCC tissues; eIF3a knockdown inhibited HCC cell proliferation, motility and EMT. Similarly, Yue et al. (122) revealed that eIF3b was upregulated in liver cancer tissues and it had promising prognostic value, as high eIF3b expression was generally associated with shorter OS and relapse-free survival. Lastly, eIF3c has also been reported to be an oncogene in liver cancer. Li et al. (123) found that eIF3c was upregulated during HCC progression and associated with poor survival in TCGA datasets. Lee et al. (124) also reported that the expression of eIF3c in HCC cells significantly increased extracellular exosome secretion and these eIF3c-enhanced exosomes were oncogenic and potentiated tumor angiogenesis.
FMRP also plays an oncogenic role in HCC. Recently, Zhu et al. (102) reported that FMRP increases cellular stemness in HCC via its target gene Cell division cycle and apoptosis regulator 1 (CCAR1), which assists in Wnt/β-catenin pathway activation. Additionally, SND1, a subunit of the RNA-induced silencing complex (RISC), has been implicated as an oncogene in HCC (103, 125, 126). First, SND1 can regulate cellular cholesterol distribution and homeostasis in HCC cells (126) and promote tumor-initiating cell (TIC) formation via the Akt and NF-κB signaling pathways in Alb/SND1 mice (125). Second, Cui et al. (103) analyzed Human Protein Atlas (HPA) and TCGA data and found that SND1 was significantly upregulated in liver cancer patients. SND1 is an anti-apoptotic factor in HCC cells and positively regulates lncRNA UCA1 expression via the transcriptional activator MYB (103). LRPPRC, a novel regulator of m6A modification, was revealed has prognostic value in HCC patients. High levels of LRPPRC are beneficial to the OS, but the precise molecular mechanisms remain elusive (127).
To sum up, m6A modification can affect the fate of mRNAs by recruiting m6A readers. The various m6A readers have different effects on HCC. However, the roles of YTHDF2, IGF2BP1 and IGF2BP3 in HCC are controversial and more studies are needed to further explore these factors.
Clinical Applications of m6A Modification in HCC
As RNA methylation plays an extensive regulatory role in HCC, RNA methylation profiling has the potential to be used as a clinical tool. The diagnosis and prognostic value of m6A-related regulators has been reported in several studies. METTL3 and YTHDF1 are both overexpressed in HCC in several studies. As METTL3/YTHDF1 overexpression is associated with poor HCC prognosis, they may be prognostic markers and therapeutic targets (19–21). Additionally, KIAA1429 was considerably upregulated in HCC tissues, and high KIAA1429 expression was associated with poor HCC prognosis (79). Another study found that eIF3b was highly expressed in liver cancer tissues and had promising diagnostic and prognostic value (122).
In addition to having diagnostic and prognostic value, m6A modification and m6A-related regulators may also be useful for developing treatments. Recently, progress has been made regarding experimental therapies targeting m6A-related mechanisms. The natural product rhein was the first identified FTO inhibitor; it exerts good inhibitory activity against FTO and increases m6A modification levels (128). However, it is not a selective FTO inhibitor, as it can also bind to a different part of the active site in AlkB than it binds to in FTO (129). Later, R-2-hydroxyglutarate (R-2HG) (130) and meclofenamic acid (MA) (131) were identified as FTO inhibitors and confirmed to inhibit tumor cell growth and induce apoptosis. Lu et al. (132) reported that curcumin can affect METTL3, METTL14, ALKBH5, FTO and YTHDF2 expression and subsequently increase m6A modification, thereby inhibiting lipopolysaccharide-induced liver injury and lipid metabolism disorders in piglets. Hou et al. (92) demonstrated that YTHDF2 downregulation in HCC is regulated by HIF-2α, and an HIF-2α antagonist (PT2385) can upregulate YTHDF2 in vitro (without changing its cytosolic distribution) and repress liver cancer. Furthermore, to treat HCC, Zuo et al. (75) developed novel PEGylated poly (lactic-co-glycolic acid) nanoparticles (PLGA-PEG NPs) loaded with si-LINC00958 targeting LINC00958, which is otherwise upregulated and stabilized by METTL3-mediated m6A modification, which promotes HCC progression. The PLGA-PEG NPs exhibited controllable drug release, excellent cellular uptake and precise tumor-targeting capacity. Moreover, tumor growth was significantly inhibited and the OS was remarkably prolonged in HCC-bearing mice injected with the PLGA-PEG NPs. Additionally, the pathological and blood test results indicated that there were no significant adverse effects in the mice. Lastly, Wang et al. (98) reported that sanguinarine can upregulate IGF2BP3 and thereby promote Hep3B cell apoptosis and inhibit proliferation, invasion and migration.
These studies suggest that HCC-related changes in the expression of m6A-related regulators may allow them to be used as HCC biomarkers and therapeutic targets. However, more studies are needed to determine the value of m6A-related regulators in early HCC diagnosis and the prediction of HCC prognosis and to assess their potential as therapeutic targets.
Conclusions and Perspectives
In summary, increasing attention has been paid to the roles of m6A modification and dysregulated m6A-related regulators in HCC. In this review, we focused on the function of m6A RNA modification in HCC. Some of the studies discussed have reported contrasting results on the expression patterns or functions of the various m6A-related regulators. For example, most of the proteins that comprise the MTC are upregulated and promote HCC progression; however, METTL14 is decreased and can suppress liver cancer cell metastasis. Interestingly, an m6A-associated regulator can perform multiple biological functions via various target genes in HCC. The roles of FTO, YTHDF2, IGF2BP1 and IGF2BP3 in liver cancer are controversial. Some studies have reported that they are highly expressed in tumor tissues and are oncogenes (61, 82, 89, 90, 97). However, other studies have reported that they are downregulated in tumor tissues and serve as tumor suppressor genes (83, 84, 91, 92, 94, 98). The contradictory results may be related to cancer heterogeneity, cell background, the targeting specificity of the m6A-related regulators and small sample sizes. In addition, some researchers have explored targeted HCC therapies related to m6A modification. For example, the HIF-2α antagonist PT2385 can upregulate YTHDF2, thus inhibiting liver cancer, while si-LINC00958-loaded PLGA-PEG NPs exhibited controllable release, excellent cellular drug uptake and precise tumor-targeting capacity in HCC. However, few studies have focused on such targeted cancer therapies.
In the future, we intend to conduct the following research (1): screen for gene expression that can be used for early diagnosis and prognosis using a large sample of liver cancer cases (2), elucidate the mechanisms underlying the conflicting effects of different/the same m6A-related regulators in liver cancer and (3) develop specific inhibitors of m6A-related regulators for treating liver cancer. It is exciting that the recent development of m6A sequencing and editing tools will greatly facilitate m6A research at the single-nucleotide level, thus advancing the field.
Author Contributions
NQ drafted the manuscript. XB constructed the figures. LM,QZ, and XX constructed the tables. FW, FH, and YS collected the references. BL revised the manuscript. XZ managed the article design, reviewed the manuscript, and provided funding support. All authors contributed to the article and approved the submitted version.
Funding
This study was supported by grants from the National Natural Science Foundation of China (81802478) and Guangxi Natural Science Foundation (2018GXNSFBA050062).
Conflict of Interest
The authors declare that the research was conducted in the absence of any commercial or financial relationships that could be construed as a potential conflict of interest.
Publisher’s Note
All claims expressed in this article are solely those of the authors and do not necessarily represent those of their affiliated organizations, or those of the publisher, the editors and the reviewers. Any product that may be evaluated in this article, or claim that may be made by its manufacturer, is not guaranteed or endorsed by the publisher.
Acknowledgments
We sincerely thank Professor Haixing Jiang and Professor Shanyu Qin from the Department of Gastroenterology, the First Affiliated Hospital of Guangxi Medical University, for their contributions to this article.
Abbreviations
RBM15/15B, RNA-binding motif protein 15; CBLL1/HAKAI, Cbl proto-oncogene-like 1; METTL16, methyltransferase-like 16; METTL5, methyltransferase-like 5; ZCCHC4, zinc finger CCCH domain-containing protein 4; ALKBH3, AlkB homolog 3; hnRNP, heterogeneous nuclear ribonucleoprotein; IGF2BP, Insulin-like growth factor 2 mRNA-binding protein; HuR, Hu antigen R; FMRP, fragile X mental retardation protein; SND1, Staphylococcal nuclease domain-containing protein 1; PRRC2A, proline rich coiled-coil 2A; LRPPRC, leucine-rich pentatricopeptide-repeat containing.
References
1. Sung H, Ferlay J, Siegel RL, Laversanne M, Soerjomataram I, Jemal A, et al. Global Cancer Statistics 2020: GLOBOCAN Estimates of Incidence and Mortality Worldwide for 36 Cancers in 185 Countries. CA Cancer J Clin (2021) . 71:209–49. doi: 10.3322/caac.21660
2. Center MM, Jemal A. International Trends in Liver Cancer Incidence Rates. Cancer Epidemiol Biomarkers Prev (2011) 20:2362–8. doi: 10.1158/1055-9965.EPI-11-0643
3. Maluccio M, Covey A. Recent Progress in Understanding, Diagnosing, and Treating Hepatocellular Carcinoma. CA Cancer J Clin (2012) 62:394–9. doi: 10.3322/caac.21161
4. Reig M, da Fonseca LG, Faivre S. New Trials and Results in Systemic Treatment of HCC. J Hepatol (2018) 69:525–33. doi: 10.1016/j.jhep.2018.03.028
5. Forner A, Reig M, Bruix J. Hepatocellular Carcinoma. Lancet (2018) 391:1301–14. doi: 10.1016/s0140-6736(18)30010-2
6. El-Khoueiry AB, Sangro B, Yau T, Crocenzi TS, Kudo M, Hsu C, et al. Nivolumab in Patients With Advanced Hepatocellular Carcinoma (CheckMate 040): An Open-Label, non-Comparative, Phase 1/2 Dose Escalation and Expansion Trial. Lancet (2017) 389:2492–502. doi: 10.1016/S0140-6736(17)31046-2
7. Desrosiers R, Friderici K, Rottman F. Identification of Methylated Nucleosides in Messenger RNA From Novikoff Hepatoma Cells. Proc Natl Acad Sci U S A (1974) 71:3971–5. doi: 10.1073/pnas.71.10.3971
8. Dai D, Wang H, Zhu L, Jin H, Wang X. N6-Methyladenosine Links RNA Metabolism to Cancer Progression. Cell Death Dis (2018) 9:124. doi: 10.1038/s41419-017-0129-x
9. Meyer KD, Saletore Y, Zumbo P, Elemento O, Mason CE, Jaffrey SR. Comprehensive Analysis of mRNA Methylation Reveals Enrichment in 3' UTRs and Near Stop Codons. Cell (2012) 149:1635–46. doi: 10.1016/j.cell.2012.05.003
10. Dominissini D, Moshitch-Moshkovitz S, Schwartz S, Salmon-Divon M, Ungar L, Osenberg S, et al. Topology of the Human and Mouse M6a RNA Methylomes Revealed by M6a-Seq. Nature (2012) 485:201–6. doi: 10.1038/nature11112
11. Meyer KD, Jaffrey SR. Rethinking M(6)A Readers, Writers, and Erasers. Annu Rev Cell Dev Biol (2017) 33:319–42. doi: 10.1146/annurev-cellbio-100616-060758
12. Pan Y, Ma P, Liu Y, Li W, Shu Y. Multiple Functions of M(6)A RNA Methylation in Cancer. J Hematol Oncol (2018) 11:48. doi: 10.1186/s13045-018-0590-8
13. Liu J, Harada BT, He C. Regulation of Gene Expression by N(6)-Methyladenosine in Cancer. Trends Cell Biol (2019) 29:487–99. doi: 10.1016/j.tcb.2019.02.008
14. Lin R, Zhan M, Yang L, Wang H, Shen H, Huang S, et al. Deoxycholic Acid Modulates the Progression of Gallbladder Cancer Through N(6)-Methyladenosine-Dependent microRNA Maturation. Oncogene (2020) 39:4983–5000. doi: 10.1038/s41388-020-1349-6
15. Shi Y, Fan S, Wu M, Zuo Z, Li X, Jiang L, et al. YTHDF1 Links Hypoxia Adaptation and non-Small Cell Lung Cancer Progression. Nat Commun (2019) 10:4892. doi: 10.1038/s41467-019-12801-6
16. Li J, Meng S, Xu M, Wang S, He L, Xu X, et al. Downregulation of N-Methyladenosine Binding YTHDF2 Protein Mediated by miR-493-3p Suppresses Prostate Cancer by Elevating N-Methyladenosine Levels. Oncotarget (2018) 9:3752–64. doi: 10.18632/oncotarget.23365
17. Ma JZ, Yang F, Zhou CC, Liu F, Yuan JH, Wang F, et al. METTL14 Suppresses the Metastatic Potential of Hepatocellular Carcinoma by Modulating N(6) -Methyladenosine-Dependent Primary MicroRNA Processing. Hepatol (Baltimore Md) (2017) 65:529–43. doi: 10.1002/hep.28885
18. Barbieri I, Tzelepis K, Pandolfini L, Shi J, Millan-Zambrano G, Robson SC, et al. Promoter-Bound METTL3 Maintains Myeloid Leukaemia by M(6)A-Dependent Translation Control. Nature (2017) 552:126–31. doi: 10.1038/nature24678
19. Chen M, Wei L, Law C-T, Tsang FH-C, Shen J, Cheng CL-H, et al. RNA N6-Methyladenosine Methyltransferase-Like 3 Promotes Liver Cancer Progression Through YTHDF2-Dependent Posttranscriptional Silencing of SOCS2. Hepatol (Baltimore Md) (2018) 67:2254–70. doi: 10.1002/hep.29683
20. Liu G-M, Zeng H-D, Zhang C-Y, Xu J-W. Identification of METTL3 as an Adverse Prognostic Biomarker in Hepatocellular Carcinoma. Dig Dis Sci (2021) 66:1110–26. doi: 10.1007/s10620-020-06260-z
21. Bian S, Ni W, Zhu M, Song Q, Zhang J, Ni R, et al. Identification and Validation of the N6-Methyladenosine RNA Methylation Regulator YTHDF1 as a Novel Prognostic Marker and Potential Target for Hepatocellular Carcinoma. Front Mol Biosci (2020) 7:604766. doi: 10.3389/fmolb.2020.604766
22. Sivasudhan E, Blake N, Lu ZL, Meng J, Rong R. Dynamics of M6a RNA Methylome on the Hallmarks of Hepatocellular Carcinoma. Front Cell Dev Biol (2021) 9:642443. doi: 10.3389/fcell.2021.642443
23. Pan XY, Huang C, Li J. The Emerging Roles of M(6)A Modification in Liver Carcinogenesis. Int J Biol Sci (2021) 17:271–84. doi: 10.7150/ijbs.50003
24. Chen M, Wong CM. The Emerging Roles of N6-Methyladenosine (M6a) Deregulation in Liver Carcinogenesis. Mol Cancer (2020) 19:44. doi: 10.1186/s12943-020-01172-y
25. Lu J, Qian J, Yin S, Zhou L, Zheng S, Zhang W. Mechanisms of RNA N(6)-Methyladenosine in Hepatocellular Carcinoma: From the Perspectives of Etiology. Front Oncol (2020) 10:1105. doi: 10.3389/fonc.2020.01105
26. Wang X, Feng J, Xue Y, Guan Z, Zhang D, Liu Z, et al. Structural Basis of N(6)-Adenosine Methylation by the METTL3-METTL14 Complex. Nature (2016) 534:575–8. doi: 10.1038/nature18298
27. Lin S, Choe J, Du P, Triboulet R, Gregory RI. The M(6)A Methyltransferase METTL3 Promotes Translation in Human Cancer Cells. Mol Cell (2016) 62:335–45. doi: 10.1016/j.molcel.2016.03.021
28. Choe J, Lin S, Zhang W, Liu Q, Wang L, Ramirez-Moya J, et al. mRNA Circularization by METTL3-Eif3h Enhances Translation and Promotes Oncogenesis. Nature (2018) 561:556–60. doi: 10.1038/s41586-018-0538-8
29. Ping XL, Sun BF, Wang L, Xiao W, Yang X, Wang WJ, et al. Mammalian WTAP is a Regulatory Subunit of the RNA N6-Methyladenosine Methyltransferase. Cell Res (2014) 24:177–89. doi: 10.1038/cr.2014.3
30. Yue Y, Liu J, Cui X, Cao J, Luo G, Zhang Z, et al. VIRMA Mediates Preferential M(6)A mRNA Methylation in 3'UTR and Near Stop Codon and Associates With Alternative Polyadenylation. Cell Discov (2018) 4:10. doi: 10.1038/s41421-018-0019-0
31. Patil DP, Chen CK, Pickering BF, Chow A, Jackson C, Guttman M, et al. M(6)A RNA Methylation Promotes XIST-Mediated Transcriptional Repression. Nature (2016) 537:369–73. doi: 10.1038/nature19342
32. Wen J, Lv R, Ma H, Shen H, He C, Wang J, et al. Zc3h13 Regulates Nuclear RNA M(6)A Methylation and Mouse Embryonic Stem Cell Self-Renewal. Mol Cell (2018) 69:1028–38.e6. doi: 10.1016/j.molcel.2018.02.015
33. Warda AS, Kretschmer J, Hackert P, Lenz C, Urlaub H, Hobartner C, et al. Human METTL16 is a N(6)-Methyladenosine (M(6)A) Methyltransferase That Targets pre-mRNAs and Various non-Coding RNAs. EMBO Rep (2017) 18:2004–14. doi: 10.15252/embr.201744940
34. Pendleton KE, Chen B, Liu K, Hunter OV, Xie Y, Tu BP, et al. The U6 snRNA M(6)A Methyltransferase METTL16 Regulates SAM Synthetase Intron Retention. Cell (2017) 169:824–35.e14. doi: 10.1016/j.cell.2017.05.003
35. van Tran N, Ernst FGM, Hawley BR, Zorbas C, Ulryck N, Hackert P, et al. The Human 18S rRNA M6a Methyltransferase METTL5 is Stabilized by TRMT112. Nucleic Acids Res (2019) 47:7719–33. doi: 10.1093/nar/gkz619
36. Ren W, Lu J, Huang M, Gao L, Li D, Wang GG, et al. Structure and Regulation of ZCCHC4 in M(6)A-Methylation of 28S rRNA. Nat Commun (2019) 10:5042. doi: 10.1038/s41467-019-12923-x
37. Sendinc E, Valle-Garcia D, Dhall A, Chen H, Henriques T, Navarrete-Perea J, et al. PCIF1 Catalyzes M6am mRNA Methylation to Regulate Gene Expression. Mol Cell (2019) 75:620–30.e9. doi: 10.1016/j.molcel.2019.05.030
38. Mauer J, Luo X, Blanjoie A, Jiao X, Grozhik AV, Patil DP, et al. Reversible Methylation of M(6)Am in the 5' Cap Controls mRNA Stability. Nature (2017) 541:371–5. doi: 10.1038/nature21022
39. Chen H, Gu L, Orellana EA, Wang Y, Guo J, Liu Q, et al. METTL4 is an snRNA M(6)Am Methyltransferase That Regulates RNA Splicing. Cell Res (2020) 30:544–7. doi: 10.1038/s41422-019-0270-4
40. Jia G, Fu Y, Zhao X, Dai Q, Zheng G, Yang Y, et al. N6-Methyladenosine in Nuclear RNA is a Major Substrate of the Obesity-Associated FTO. Nat Chem Biol (2011) 7:885–7. doi: 10.1038/nchembio.687
41. Zheng G, Dahl JA, Niu Y, Fedorcsak P, Huang CM, Li CJ, et al. ALKBH5 is a Mammalian RNA Demethylase That Impacts RNA Metabolism and Mouse Fertility. Mol Cell (2013) 49:18–29. doi: 10.1016/j.molcel.2012.10.015
42. Zhou Z, Lv J, Yu H, Han J, Yang X, Feng D, et al. Mechanism of RNA Modification N6-Methyladenosine in Human Cancer. Mol Cancer (2020) 19:104. doi: 10.1186/s12943-020-01216-3
43. Ueda Y, Ooshio I, Fusamae Y, Kitae K, Kawaguchi M, Jingushi K, et al. AlkB Homolog 3-Mediated tRNA Demethylation Promotes Protein Synthesis in Cancer Cells. Sci Rep (2017) 7:42271. doi: 10.1038/srep42271
44. Wang X, Zhao BS, Roundtree IA, Lu Z, Han D, Ma H, et al. N(6)-Methyladenosine Modulates Messenger RNA Translation Efficiency. Cell (2015) 161:1388–99. doi: 10.1016/j.cell.2015.05.014
45. Zhou J, Wan J, Gao X, Zhang X, Jaffrey SR, Qian SB. Dynamic M(6)A mRNA Methylation Directs Translational Control of Heat Shock Response. Nature (2015) 526:591–4. doi: 10.1038/nature15377
46. Du H, Zhao Y, He J, Zhang Y, Xi H, Liu M, et al. YTHDF2 Destabilizes M(6)A-Containing RNA Through Direct Recruitment of the CCR4-NOT Deadenylase Complex. Nat Commun (2016) 7:12626. doi: 10.1038/ncomms12626
47. Wang X, Lu Z, Gomez A, Hon GC, Yue Y, Han D, et al. N6-Methyladenosine-Dependent Regulation of Messenger RNA Stability. Nature (2014) 505:117–20. doi: 10.1038/nature12730
48. Roundtree IA, Luo GZ, Zhang Z, Wang X, Zhou T, Cui Y, et al. YTHDC1 Mediates Nuclear Export of N(6)-Methyladenosine Methylated mRNAs. Elife (2017) 6:e31311. doi: 10.7554/eLife.31311
49. Shima H, Matsumoto M, Ishigami Y, Ebina M, Muto A, Sato Y, et al. S-Adenosylmethionine Synthesis Is Regulated by Selective N(6)-Adenosine Methylation and mRNA Degradation Involving METTL16 and YTHDC1. Cell Rep (2017) 21:3354–63. doi: 10.1016/j.celrep.2017.11.092
50. Mao Y, Dong L, Liu XM, Guo J, Ma H, Shen B, et al. M(6)A in mRNA Coding Regions Promotes Translation via the RNA Helicase-Containing YTHDC2. Nat Commun (2019) 10:5332. doi: 10.1038/s41467-019-13317-9
51. Kretschmer J, Rao H, Hackert P, Sloan KE, Hobartner C, Bohnsack MT. The M(6)A Reader Protein YTHDC2 Interacts With the Small Ribosomal Subunit and the 5'-3' Exoribonuclease XRN1. RNA (2018) 24:1339–50. doi: 10.1261/rna.064238.117
52. Wojtas MN, Pandey RR, Mendel M, Homolka D, Sachidanandam R, Pillai RS. Regulation of M(6)A Transcripts by the 3'–>5' RNA Helicase YTHDC2 Is Essential for a Successful Meiotic Program in the Mammalian Germline. Mol Cell (2017) 68:374–87.e12. doi: 10.1016/j.molcel.2017.09.021
53. Liu N, Dai Q, Zheng G, He C, Parisien M, Pan T. N(6)-Methyladenosine-Dependent RNA Structural Switches Regulate RNA-Protein Interactions. Nature (2015) 518:560–4. doi: 10.1038/nature14234
54. Liu N, Zhou KI, Parisien M, Dai Q, Diatchenko L, Pan T. N6-Methyladenosine Alters RNA Structure to Regulate Binding of a Low-Complexity Protein. Nucleic Acids Res (2017) 45:6051–63. doi: 10.1093/nar/gkx141
55. Chen Z, Chen X, Lei T, Gu Y, Gu J, Huang J, et al. Integrative Analysis of NSCLC Identifies LINC01234 as an Oncogenic lncRNA That Interacts With HNRNPA2B1 and Regulates miR-106b Biogenesis. Mol Ther (2020) 28:1479–93. doi: 10.1016/j.ymthe.2020.03.010
56. Alarcon CR, Goodarzi H, Lee H, Liu X, Tavazoie S, Tavazoie SF. HNRNPA2B1 Is a Mediator of M(6)A-Dependent Nuclear RNA Processing Events. Cell (2015) 162:1299–308. doi: 10.1016/j.cell.2015.08.011
57. Huang H, Weng H, Sun W, Qin X, Shi H, Wu H, et al. Publisher Correction: Recognition of RNA N(6)-Methyladenosine by IGF2BP Proteins Enhances mRNA Stability and Translation. Nat Cell Biol (2020) 22:1288. doi: 10.1038/s41556-020-00580-y
58. Wang Y, Li Y, Toth JI, Petroski MD, Zhang Z, Zhao JC. N6-Methyladenosine Modification Destabilizes Developmental Regulators in Embryonic Stem Cells. Nat Cell Biol (2014) 16:191–8. doi: 10.1038/ncb2902
59. Huang H, Weng H, Sun W, Qin X, Shi H, Wu H, et al. Recognition of RNA N(6)-Methyladenosine by IGF2BP Proteins Enhances mRNA Stability and Translation. Nat Cell Biol (2018) 20:285–95. doi: 10.1038/s41556-018-0045-z
60. Bell JL, Wachter K, Muhleck B, Pazaitis N, Kohn M, Lederer M, et al. Insulin-Like Growth Factor 2 mRNA-Binding Proteins (IGF2BPs): Post-Transcriptional Drivers of Cancer Progression? Cell Mol Life Sci (2013) 70:2657–75. doi: 10.1007/s00018-012-1186-z
61. Muller S, Glass M, Singh AK, Haase J, Bley N, Fuchs T, et al. IGF2BP1 Promotes SRF-Dependent Transcription in Cancer in a M6a- and miRNA-Dependent Manner. Nucleic Acids Res (2019) 47:375–90. doi: 10.1093/nar/gky1012
62. Hu X, Peng WX, Zhou H, Jiang J, Zhou X, Huang D, et al. IGF2BP2 Regulates DANCR by Serving as an N6-Methyladenosine Reader. Cell Death Differ (2020) 27:1782–94. doi: 10.1038/s41418-019-0461-z
63. Mancarella C, Scotlandi K. IGF2BP3 From Physiology to Cancer: Novel Discoveries, Unsolved Issues, and Future Perspectives. Front Cell Dev Biol (2019) 7:363. doi: 10.3389/fcell.2019.00363
64. Zhang F, Kang Y, Wang M, Li Y, Xu T, Yang W, et al. Fragile X Mental Retardation Protein Modulates the Stability of its M6a-Marked Messenger RNA Targets. Hum Mol Genet (2018) 27:3936– 50. doi: 10.1093/hmg/ddy292
65. Edupuganti RR, Geiger S, Lindeboom RGH, Shi H, Hsu PJ, Lu Z, et al. N(6)-Methyladenosine (M(6)A) Recruits and Repels Proteins to Regulate mRNA Homeostasis. Nat Struct Mol Biol (2017) 24:870–8. doi: 10.1038/nsmb.3462
66. Ruzzenente B, Metodiev MD, Wredenberg A, Bratic A, Park CB, Cámara Y, et al. LRPPRC is Necessary for Polyadenylation and Coordination of Translation of Mitochondrial mRNAs. EMBO J (2012) 31:443–56. doi: 10.1038/emboj.2011.392
67. Arguello AE, DeLiberto AN, Kleiner RE. RNA Chemical Proteomics Reveals the N-Methyladenosine (Ma)-Regulated Protein-RNA Interactome. J Am Chem Soc (2017) 139:17249–52. doi: 10.1021/jacs.7b09213
68. Meyer KD, Patil DP, Zhou J, Zinoviev A, Skabkin MA, Elemento O, et al. 5' UTR M(6)A Promotes Cap-Independent Translation. Cell (2015) 163:999–1010. doi: 10.1016/j.cell.2015.10.012
69. Baquero-Perez B, Antanaviciute A, Yonchev ID, Carr IM, Wilson SA, Whitehouse A. The Tudor SND1 Protein is an M(6)A RNA Reader Essential for Replication of Kaposi's Sarcoma-Associated Herpesvirus. Elife (2019) 8:e47261. doi: 10.7554/eLife.47261
70. Wu R, Li A, Sun B, Sun JG, Zhang J, Zhang T, et al. A Novel M(6)A Reader Prrc2a Controls Oligodendroglial Specification and Myelination. Cell Res (2019) 29:23–41. doi: 10.1038/s41422-018-0113-8
71. Choi J, Ieong KW, Demirci H, Chen J, Petrov A, Prabhakar A, et al. N(6)-Methyladenosine in mRNA Disrupts tRNA Selection and Translation-Elongation Dynamics. Nat Struct Mol Biol (2016) 23:110–5. doi: 10.1038/nsmb.3148
72. Shi H, Wang X, Lu Z, Zhao BS, Ma H, Hsu PJ, et al. YTHDF3 Facilitates Translation and Decay of N(6)-Methyladenosine-Modified RNA. Cell Res (2017) 27:315–28. doi: 10.1038/cr.2017.15
73. Lin X, Chai G, Wu Y, Li J, Chen F, Liu J, et al. RNA M(6)A Methylation Regulates the Epithelial Mesenchymal Transition of Cancer Cells and Translation of Snail. Nat Commun (2019) 10:2065. doi: 10.1038/s41467-019-09865-9
74. Qiao K, Liu Y, Xu Z, Zhang H, Zhang H, Zhang C, et al. RNA M6a Methylation Promotes the Formation of Vasculogenic Mimicry in Hepatocellular Carcinoma via Hippo Pathway. Angiogenesis (2021) 24:83–96. doi: 10.1007/s10456-020-09744-8
75. Zuo X, Chen Z, Gao W, Zhang Y, Wang J, Wang J, et al. M6A-Mediated Upregulation of LINC00958 Increases Lipogenesis and Acts as a Nanotherapeutic Target in Hepatocellular Carcinoma. J Gastroenterol Hepatol (2020) 13:5. doi: 10.1186/s13045-019-0839-x
76. Chen SL, Liu LL, Wang CH, Lu SX, Yang X, He YF, et al. Loss of RDM1 Enhances Hepatocellular Carcinoma Progression via P53 and Ras/Raf/ERK Pathways. Mol Oncol (2020) 14:373–86. doi: 10.1002/1878-0261.12593
77. Xu H, Wang H, Zhao W, Fu S, Li Y, Ni W, et al. SUMO1 Modification of Methyltransferase-Like 3 Promotes Tumor Progression via Regulating Snail mRNA Homeostasis in Hepatocellular Carcinoma. Theranostics (2020) 10:5671–86. doi: 10.7150/thno.42539
78. Chen Y, Peng C, Chen J, Chen D, Yang B, He B, et al. WTAP Facilitates Progression of Hepatocellular Carcinoma via M6a-HuR-Dependent Epigenetic Silencing of ETS1. Mol Cancer (2019) 18:127. doi: 10.1186/s12943-019-1053-8
79. Lan T, Li H, Zhang D, Xu L, Liu H, Hao X, et al. KIAA1429 Contributes to Liver Cancer Progression Through N6-Methyladenosine-Dependent Post-Transcriptional Modification of GATA3. Mol Cancer (2019) 18:186–. doi: 10.1186/s12943-019-1106-z
80. Cheng X, Li M, Rao X, Zhang W, Li X, Wang L, et al. KIAA1429 Regulates the Migration and Invasion of Hepatocellular Carcinoma by Altering M6a Modification of ID2 mRNA. OncoTargets Ther (2019) 12:3421–8. doi: 10.2147/ott.S180954
81. Ma H, Wang X, Cai J, Dai Q, Natchiar SK, Lv R, et al. N(6-)Methyladenosine Methyltransferase ZCCHC4 Mediates Ribosomal RNA Methylation. Nat Chem Biol (2019) 15:88–94. doi: 10.1038/s41589-018-0184-3
82. Li J, Zhu L, Shi Y, Liu J, Lin L, Chen X. M6a Demethylase FTO Promotes Hepatocellular Carcinoma Tumorigenesis via Mediating PKM2 Demethylation. Am J Transl Res (2019) 11:6084–92.
83. Liu X, Liu J, Xiao W, Zeng Q, Bo H, Zhu Y, et al. SIRT1 Regulates N(6) -Methyladenosine RNA Modification in Hepatocarcinogenesis by Inducing RANBP2-Dependent FTO SUMOylation. Hepatol (Baltimore Md) (2020) 72:2029–50. doi: 10.1002/hep.31222
84. Mittenbühler MJ, Saedler K, Nolte H, Kern L, Zhou J, Qian SB, et al. Hepatic FTO is Dispensable for the Regulation of Metabolism But Counteracts HCC Development In Vivo. Mol Metab (2020) 42:101085. doi: 10.1016/j.molmet.2020.101085
85. Chen Y, Zhao Y, Chen J, Peng C, Zhang Y, Tong R, et al. ALKBH5 Suppresses Malignancy of Hepatocellular Carcinoma via M(6)A-Guided Epigenetic Inhibition of LYPD1. Mol Cancer (2020) 19:123. doi: 10.1186/s12943-020-01239-w
86. Wang Q, Wang G, Wang Y, Liu C, He X. Association of AlkB Homolog 3 Expression With Tumor Recurrence and Unfavorable Prognosis in Hepatocellular Carcinoma. J Gastroenterol Hepatol (2018). doi: 10.1111/jgh.14117
87. Ding H, Zhang X, Su Y, Jia C, Dai C. GNAS Promotes Inflammation-Related Hepatocellular Carcinoma Progression by Promoting STAT3 Activation. Cell Mol Biol Lett (2020) 25:8. doi: 10.1186/s11658-020-00204-1
88. Liu X, Qin J, Gao T, Li C, He B, Pan B, et al. YTHDF1 Facilitates the Progression of Hepatocellular Carcinoma by Promoting FZD5 mRNA Translation in an M6a-Dependent Manner. Mol Ther Nucleic Acids (2020) 22:750–65. doi: 10.1016/j.omtn.2020.09.036
89. Yang Z, Li J, Feng G, Gao S, Wang Y, Zhang S, et al. MicroRNA-145 Modulates N(6)-Methyladenosine Levels by Targeting the 3'-Untranslated mRNA Region of the N(6)-Methyla- Denosine Binding YTH Domain Family 2 Protein. J Biol Chem (2017) 292:3614–23. doi: 10.1074/jbc.M116.749689
90. Zhang C, Huang S, Zhuang H, Ruan S, Zhou Z, Huang K, et al. YTHDF2 Promotes the Liver Cancer Stem Cell Phenotype and Cancer Metastasis by Regulating OCT4 Expression via M6a RNA Methylation. Oncogene (2020) 39:4507–18. doi: 10.1038/s41388-020-1303-7
91. Zhong L, Liao D, Zhang M, Zeng C, Li X, Zhang R, et al. YTHDF2 Suppresses Cell Proliferation and Growth via Destabilizing the EGFR mRNA in Hepatocellular Carcinoma. Cancer Lett (2019) 442:252–61. doi: 10.1016/j.canlet.2018.11.006
92. Hou J, Zhang H, Liu J, Zhao Z, Wang J, Lu Z, et al. YTHDF2 Reduction Fuels Inflammation and Vascular Abnormalization in Hepatocellular Carcinoma. Mol Cancer (2019) 18:163. doi: 10.1186/s12943-019-1082-3
93. Wang M, Yang Y, Yang J, Yang J, Han S. Circ_KIAA1429 Accelerates Hepatocellular Carcinoma Advancement Through the Mechanism of M(6)A-YTHDF3-Zeb1. Hepatology (Baltimore, Md) (2020) 257:118082. doi: 10.1016/j.lfs.2020.118082
94. Geis T, Popp R, Hu J, Fleming I, Henke N, Dehne N, et al. HIF-2α Attenuates Lymphangiogenesis by Up-Regulating IGFBP1 in Hepatocellular Carcinoma. Biol Cell (2015) 107:175–88. doi: 10.1111/boc.201400079
95. Ma Y, Cui D, Zhang Y, Han CC, Wei W. Insulin-Like Growth Factor Binding Protein-2 Promotes Proliferation and Predicts Poor Prognosis in Hepatocellular Carcinoma. OncoTargets Ther (2020) 13:5083–92. doi: 10.2147/ott.S249527
96. Liu FY, Zhou SJ, Deng YL, Zhang ZY, Zhang EL, Wu ZB, et al. MiR-216b is Involved in Pathogenesis and Progression of Hepatocellular Carcinoma Through HBx-miR-216b-IGF2BP2 Signaling Pathway. Cell Death Dis (2015) 6:e1670. doi: 10.1038/cddis.2015.46
97. Nguyen LH, Robinton DA, Seligson MT, Wu L, Li L, Rakheja D, et al. Lin28b is Sufficient to Drive Liver Cancer and Necessary for its Maintenance in Murine Models. J Gastroenterol Hepatol (2014) 26:248–61. doi: 10.1016/j.ccr.2014.06.018
98. Wang H, Wang H, Li K, Li S, Sun B. IGFBP-3 Is the Key Target of Sanguinarine in Promoting Apoptosis in Hepatocellular Carcinoma. Cancer Manage Res (2020) 12:1007–15. doi: 10.2147/cmar.S234291
99. Ma Y, Han CC, Li Y, Wang Y, Wei W. Insulin-Like Growth Factor-Binding Protein-3 Inhibits IGF-1-Induced Proliferation of Human Hepatocellular Carcinoma Cells by Controlling bFGF and PDGF Autocrine/Paracrine Loops. Biochem Biophys Res Commun (2016) 478:964–9. doi: 10.1016/j.bbrc.2016.08.059
100. Miao B, Wei C, Qiao Z, Han W, Chai X, Lu J, et al. Eif3a Mediates HIF1α-Dependent Glycolytic Metabolism in Hepatocellular Carcinoma Cells Through Translational Regulation. Am J Cancer Res (2019) 9:1079–90.
101. Chen T, Sun L, Yao B, Wang L, Wang Y, Niu Y, et al. MicroRNA−875−5p Inhibits Tumor Growth and Metastasis of Hepatocellular Carcinoma by Targeting Eukaryotic Translation Initiation Factor 3 Subunit a. Oncol Rep (2020) 44:2067–79. doi: 10.3892/or.2020.7743
102. Zhu YJ, Zheng B, Luo GJ, Ma XK, Lu XY, Lin XM, et al. Circular RNAs Negatively Regulate Cancer Stem Cells by Physically Binding FMRP Against CCAR1 Complex in Hepatocellular Carcinoma. Theranostics (2019) 9:3526–40. doi: 10.7150/thno.32796
103. Cui X, Zhao C, Yao X, Qian B, Su C, Ren Y, et al. SND1 Acts as an Anti-Apoptotic Factor via Regulating the Expression of lncRNA UCA1 in Hepatocellular Carcinoma. RNA Biol (2018) 15:1364–75. doi: 10.1080/15476286.2018.1534525
104. Lin Y, Wei X, Jian Z, Zhang X. METTL3 Expression is Associated With Glycolysis Metabolism and Sensitivity to Glycolytic Stress in Hepatocellular Carcinoma. Cancer Med (2020) 9:2859–67. doi: 10.1002/cam4.2918
105. Lin Z, Niu Y, Wan A, Chen D, Liang H, Chen X, et al. RNA M(6) A Methylation Regulates Sorafenib Resistance in Liver Cancer Through FOXO3-Mediated Autophagy. EMBO J (2020) 39:e103181. doi: 10.15252/embj.2019103181
106. Sera T, Hiasa Y, Mashiba T, Tokumoto Y, Hirooka M, Konishi I, et al. Wilms' Tumour 1 Gene Expression is Increased in Hepatocellular Carcinoma and Associated With Poor Prognosis. Eur J Cancer (2008) 44:600–8. doi: 10.1016/j.ejca.2008.01.008
107. Zhou T, Ren Z, Chen C. METTL14 as a Predictor of Postoperative Survival Outcomes of Patients With Hepatocellular Carcinoma. Nan Fang Yi Ke Da Xue Xue Bao (2020) 40:567–72. doi: 10.12122/j.issn.1673-4254.2020.04.19
108. Liu X, Qin J, Gao T, Li C, Chen X, Zeng K, et al. Analysis of METTL3 and METTL14 in Hepatocellular Carcinoma. Aging (2020) 12:21638–59. doi: 10.18632/aging.103959
109. Li Z, Li F, Peng Y, Fang J, Zhou J. Identification of Three M6a-Related mRNAs Signature and Risk Score for the Prognostication of Hepatocellular Carcinoma. Cancer Med (2020) 9:1877–89. doi: 10.1002/cam4.2833
110. Zhang BH, Yan LN, Yang JY. Pending Role of METTL14 in Liver Cancer. Hepatobiliary Surg Nutr (2019) 8:669–70. doi: 10.21037/hbsn.2019.10.16
111. Wang P, Wang X, Zheng L, Zhuang C. Gene Signatures and Prognostic Values of M6a Regulators in Hepatocellular Carcinoma. Front Genet (2020) 11:540186. doi: 10.3389/fgene.2020.540186
112. Liu Z, Wang Q, Wang X, Xu Z, Wei X, Li J. Circular RNA cIARS Regulates Ferroptosis in HCC Cells Through Interacting With RNA Binding Protein ALKBH5. Cell Death Discov (2020) 6:72. doi: 10.1038/s41420-020-00306-x
113. Qu N, Qin S, Zhang X, Bo X, Liu Z, Tan C, et al. Multiple M(6)A RNA Methylation Modulators Promote the Malignant Progression of Hepatocellular Carcinoma and Affect its Clinical Prognosis. BMC Cancer (2020) 20:165. doi: 10.1186/s12885-020-6638-5
114. Zhao X, Chen Y, Mao Q, Jiang X, Jiang W, Chen J, et al. Overexpression of YTHDF1 is Associated With Poor Prognosis in Patients With Hepatocellular Carcinoma. Cancer Biomarkers Sect A Dis Markers (2018) 21:859–68. doi: 10.3233/CBM-170791
115. Zhao Z, Yang L, Fang S, Zheng L, Wu F, Chen W, et al. The Effect of M6a Methylation Regulatory Factors on the Malignant Progression and Clinical Prognosis of Hepatocellular Carcinoma. Front Oncol (2020) 10:1435. doi: 10.3389/fonc.2020.01435
116. Chen T, Gu C, Xue C, Yang T, Zhong Y, Liu S, et al. LncRNA-Uc002mbe.2 Interacting With Hnrnpa2b1 Mediates AKT Deactivation and P21 Up-Regulation Induced by Trichostatin in Liver Cancer Cells. Front Pharmacol (2017) 8:669. doi: 10.3389/fphar.2017.00669
117. Gong J, Shen S, Yang Y, Qin S, Huang L, Zhang H, et al. Inhibition of FASN Suppresses Migration, Invasion and Growth in Hepatoma Carcinoma Cells by Deregulating the HIF-1α/IGFBP1 Pathway. Int J Oncol (2017) 50:883–92. doi: 10.3892/ijo.2017.3867
118. He J, Zuo Q, Hu B, Jin H, Wang C, Cheng Z, et al. A Novel, Liver-Specific Long Noncoding RNA LINC01093 Suppresses HCC Progression by Interaction With IGF2BP1 to Facilitate Decay of GLI1 mRNA. Cancer Lett (2019) 450:98–109. doi: 10.1016/j.canlet.2019.02.033
119. Nielsen KO, Mirza AH, Kaur S, Jacobsen KS, Winther TN, Glebe D, et al. Hepatitis B Virus Suppresses the Secretion of Insulin-Like Growth Factor Binding Protein 1 to Facilitate Anti-Apoptotic IGF-1 Effects in HepG2 Cells. Exp Cell Res (2018) 370:399–408. doi: 10.1016/j.yexcr.2018.07.002
120. Golob-Schwarzl N, Krassnig S, Toeglhofer AM, Park YN, Gogg-Kamerer M, Vierlinger K, et al. New Liver Cancer Biomarkers: PI3K/AKT/mTOR Pathway Members and Eukaryotic Translation Initiation Factors. Eur J Cancer (2017) 83:56–70. doi: 10.1016/j.ejca.2017.06.003
121. Heo CK, Hwang HM, Lee HJ, Kwak SS, Yoo JS, Yu DY, et al. Serum Anti-EIF3A Autoantibody as a Potential Diagnostic Marker for Hepatocellular Carcinoma. Sci Rep (2019) 9:11059. doi: 10.1038/s41598-019-47365-4
122. Yue Q, Meng L, Jia B, Han W. Expression of Eukaryotic Translation Initiation Factor 3 Subunit B in Liver Cancer and its Prognostic Significance. Exp Ther Med (2020) 20:436–46. doi: 10.3892/etm.2020.8726
123. Li T, Li S, Chen D, Chen B, Yu T, Zhao F, et al. Transcriptomic Analyses of RNA-Binding Proteins Reveal Eif3c Promotes Cell Proliferation in Hepatocellular Carcinoma. Cancer Sci (2017) 108:877–85. doi: 10.1111/cas.13209
124. Lee HY, Chen CK, Ho CM, Lee SS, Chang CY, Chen KJ, et al. EIF3C-Enhanced Exosome Secretion Promotes Angiogenesis and Tumorigenesis of Human Hepatocellular Carcinoma. Oncotarget (2018) 9:13193–205. doi: 10.18632/oncotarget.24149
125. Jariwala N, Rajasekaran D, Mendoza RG, Shen XN, Siddiq A, Akiel MA, et al. Oncogenic Role of SND1 in Development and Progression of Hepatocellular Carcinoma. Cancer Res (2017) 77:3306–16. doi: 10.1158/0008-5472.Can-17-0298
126. Navarro-Imaz H, Rueda Y, Fresnedo O. SND1 Overexpression Deregulates Cholesterol Homeostasis in Hepatocellular Carcinoma. Biochim Biophys Acta (2016) 1861:988–96. doi: 10.1016/j.bbalip.2016.05.011
127. Li W, Dai Y, Shi B, Yue F, Zou J, Xu G, et al. LRPPRC Sustains Yap-P27-Mediated Cell Ploidy and P62-HDAC6-Mediated Autophagy Maturation and Suppresses Genome Instability and Hepatocellular Carcinomas. Oncogene (2020) 39:3879–92. doi: 10.1038/s41388-020-1257-9
128. Chen B, Ye F, Yu L, Jia G, Huang X, Zhang X, et al. Development of Cell-Active N6-Methyladenosine RNA Demethylase FTO Inhibitor. J Am Chem Soc (2012) 134:17963–71. doi: 10.1021/ja3064149
129. Li Q, Huang Y, Liu X, Gan J, Chen H, Yang CG. Rhein Inhibits AlkB Repair Enzymes and Sensitizes Cells to Methylated DNA Damage. J Biol Chem (2016) 291:11083–93. doi: 10.1074/jbc.M115.711895
130. Su R, Dong L, Li C, Nachtergaele S, Wunderlich M, Qing Y, et al. R-2hg Exhibits Anti-Tumor Activity by Targeting FTO/m(6)A/MYC/CEBPA Signaling. Cell (2018) 172:90–105.e23. doi: 10.1016/j.cell.2017.11.031
131. Huang Y, Yan J, Li Q, Li J, Gong S, Zhou H, et al. Meclofenamic Acid Selectively Inhibits FTO Demethylation of M6a Over ALKBH5. Nucleic Acids Res (2015) 43:373–84. doi: 10.1093/nar/gku1276
Keywords: hepatocellular carcinoma, N6-methyladenosine m6A, writers, readers, erasers
Citation: Qu N, Bo X, Li B, Ma L, Wang F, Zheng Q, Xiao X, Huang F, Shi Y and Zhang X (2021) Role of N6-Methyladenosine (m6A) Methylation Regulators in Hepatocellular Carcinoma. Front. Oncol. 11:755206. doi: 10.3389/fonc.2021.755206
Received: 08 August 2021; Accepted: 22 September 2021;
Published: 07 October 2021.
Edited by:
Tianbao Li, Geneis (Beijing) Co. Ltd, ChinaReviewed by:
Chun-I Wang, Chang Gung University, TaiwanMin Yu, Guangdong Provincial People’s Hospital, China
Copyright © 2021 Qu, Bo, Li, Ma, Wang, Zheng, Xiao, Huang, Shi and Zhang. This is an open-access article distributed under the terms of the Creative Commons Attribution License (CC BY). The use, distribution or reproduction in other forums is permitted, provided the original author(s) and the copyright owner(s) are credited and that the original publication in this journal is cited, in accordance with accepted academic practice. No use, distribution or reproduction is permitted which does not comply with these terms.
*Correspondence: Xuemei Zhang, emhhbmd4dWVtMjAyMTA5QDE2My5jb20=