- 1Department of Pharmaceutical Sciences, College of Pharmacy, OSU, Corvallis, OR, United States
- 2John A. Burns School of Medicine at the University of Hawai’i at Mānoa, Honolulu, HI, United States
- 3Department of Dermatology, University of Alabama at Birmingham, Birmingham, AL, United States
- 4Cancer Chemoprevention Program, Comprehensive Cancer Center, University of Alabama at Birmingham, Birmingham, AL, United States
- 5Knight Cancer Institute, Oregon Health & Science University (OHSU), Portland, OR, United States
- 6Department of Biochemistry and Biophysics, Oregon State University (OSU), Corvallis, OR, United States
- 7Linus Pauling Science Center, Oregon State University (OSU), Corvallis, OR, United States
- 8Department of Dermatology, Oregon Health & Science University (OHSU), Portland, OR, United States
Melanoma is the malignant transformation of melanocytes and represents the most lethal form of skin cancer. While early-stage melanoma localized to the skin can be cured with surgical excision, metastatic melanoma often requires a multi-pronged approach and even then can exhibit treatment resistance. Understanding the molecular mechanisms involved in the pathogenesis of melanoma could lead to novel diagnostic, prognostic, and therapeutic strategies to ultimately decrease morbidity and mortality. One emerging candidate that may have value as both a prognostic marker and in a therapeutic context is the vitamin D receptor (VDR). VDR is a nuclear steroid hormone receptor activated by 1,25 dihydroxy-vitamin D3 [calcitriol, 1,25(OH)2D3]. While 1,25 dihydroxy-vitamin D3 is typically thought of in relation to calcium metabolism, it also plays an important role in cell proliferation, differentiation, programmed-cell death as well as photoprotection. This review discusses the role of VDR in the crosstalk between keratinocytes and melanocytes during melanomagenesis and summarizes the clinical data regarding VDR polymorphisms, VDR as a prognostic marker, and potential uses of vitamin D and its analogs as an adjuvant treatment for melanoma.
Introduction
The worldwide incidence of melanoma has steadily increased over the past several decades with the annual incidence rising as rapidly as 4-6% in certain regions (1). In 2021, it is estimated that approximately 106,110 new melanomas will be diagnosed in the United States alone (2). While the incidence of melanoma is greatest in older adult populations, peaking at the sixth decade of life in the United States, it is also one of the most common malignancies found in adolescent and young adult populations (1, 3, 4). In addition to being a relatively ubiquitous cancer, melanoma is the most lethal skin cancer resulting in 9,008 deaths per year in the United States between the years of 2012-2016 (1).
Cutaneous melanoma results from the malignant transformation of predominantly melanocytes (5). Since these pigment producing cells are generally confined to the epidermis of the skin the appearance of vertical growth or Breslow thickness play key roles in determining the aggressiveness of the tumor and its likelihood of metastasis (6). For instance, a stage 0 melanoma is confined only to the epidermis and does not involve nearby dermis or spread to lymph nodes and distant organs. Whereas any melanoma that involves distant metastases is classified as a stage IV tumor.
In early-stage melanoma surgical excision is often curative when the tumor is localized to the skin (1). However, following progression to metastatic melanoma treatment becomes more complex and may include inhibition of metastasis, immunotherapy, targeted inhibition of the mitogen-activated protein kinase (MAPK) pathway, and/or radiation therapy (7, 8). Despite initial improvements these treatments are not fully effective and the cancer is terminal in many cases (9). Reversing this trend is the challenge ahead of melanoma investigators and clinicians, where a more thorough understanding of the molecular mechanisms involved in the pathogenesis of melanoma could lead to novel diagnostic, prognostic, and therapeutic strategies, ultimately resulting in a decreased mortality rate.
One emerging candidate for both targeted therapy and prediction of prognosis is the vitamin-D-receptor (VDR) (10–13). VDR is a nuclear steroid hormone receptor that is found in several organs, including the skin (14). VDR is activated by 1,25 dihydroxy-vitamin D3 (calcitriol, 1,25(OH)2D3) which, in addition of regulating body calcium metabolism, is involved in many pleiotropic activities including regulation of cell proliferation, differentiation, and programmed cell death as well as in photoprotection (12, 13, 15–21).
Activation of Vitamin D
In the canonical pathway of the activation of vitamin D to 1,25(OH)2D3 involves sequential hydroxylations at C25 by CYP2R1 and CYP27A1 and at C1α by CYP27B1 occurring, respectively, in the liver and kidney (22, 23) and in peripheral organs including skin (24). In alternative pathway (non-canonical) vitamin D is activated by CYP11A1 through sequential hydroxylations of it side chain with additional metabolism by other CYP enzymes (23, 25–28). In addition, CYP11A1 is expressed in immune cells, raising a possibility that CYP11A1-derived vitamin D metabolites can be produced in immune cells to regulate their function in a cell autonomous manner (29). While 1,25(OH)2D3 exerts its phenotypic activity through activation of the VDR (30–35) and to some degree through non-genomic action on 1,25D3-MARRS receptor (36, 37), the CYP11A1-derived vitamin D metabolites, in addition on acting on the VDR (13, 38–41), can also interact with alternative nuclear receptors including retinoic acid receptors (RORs) (41, 42), aryl hydrocarbon receptor (AhR) (43) and liver X receptors (LXR) (44). It should be noted that 1,25(OH)2D3 can also act as an agonist on the AhR and LXRs (see Figures 1 and 2 for details).
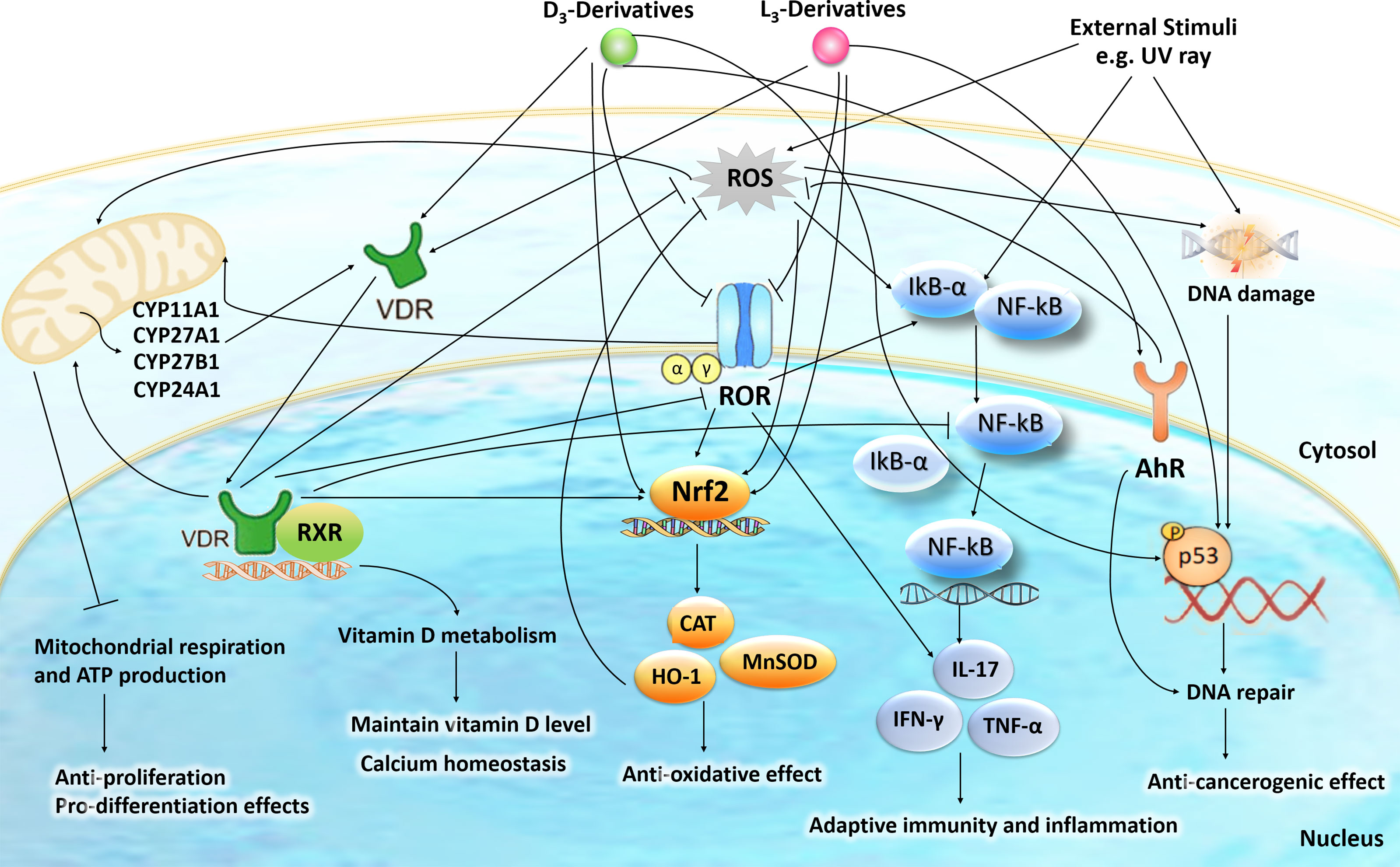
Figure 1 The intracellular action of vitamin D3 (D3)- and lumisterol (L3)-hydroxyderivatives in photoprotection against UVR. Signal transduction includes the activation of nuclear receptors such as vitamin D receptor (VDR), retinoic acid orphan receptor (ROR)α/γ, and aryl hydrocarbon receptor (AhR) and the direct action of D3- and L3-hydroxyderivatives on mitochondrial processes. The nuclear receptors activities are linked with the transcriptional master regulators NRF2 (nuclear factor erythroid-derived 2-like 2), p53 and NFκB (nuclear factor kappa-light-chain-enhancer of activated B cells) to coordinate anti-oxidative, DNA repair, anti-inflammatory, and antiproliferative as well as anti-carcinogenesis mechanisms. The figure is reprinted from (45) with a permission from the publisher.
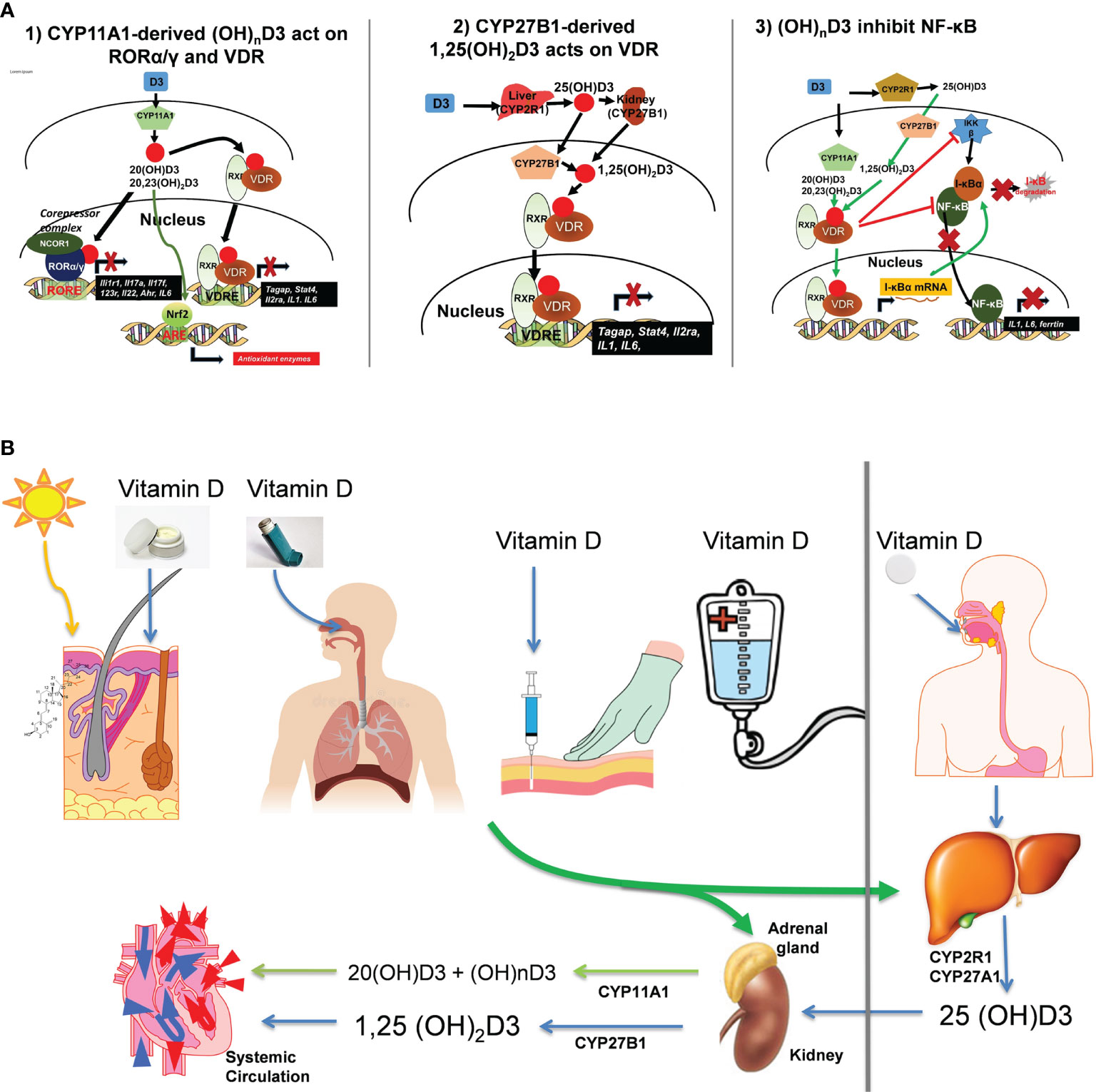
Figure 2 (A) Mechanism of action of canonical and non-canonical vitamin D-hydroxyderivatives. Vitamin D signaling in mononuclear cells downregulates inflammatory genes and suppresses oxidative stress. VDR, vitamin D receptor; RXR, retinoid X receptor; ROR, retinoic acid orphan receptor, ROR, ROR response element; ARE, antioxidant response element; VDRE, vitamin D response element; NRF2, nuclear factor erythroid-derived 2-like 2. (B) Different routes of vitamin D delivery will impact vitamin D activation pattern. The figure is reprinted from (46) with a permission from the publisher.
VDR in the Crosstalk Between Keratinocytes and Melanocytes in Melanomagenesis
Under normal physiological conditions, melanocyte homeostasis is maintained by paracrine, autocrine, and direct cell-cell communication between melanocytes and adjacent keratinocytes that comprise epidermal melanin units (47, 48). During melanomagenesis, melanocytes begin to downregulate expression of adhesion molecules, such as E-cadherin, enabling an epithelial-mesenchymal transition that severs transforming melanoma cells from the regulatory activity of adjacent keratinocytes. This process then enables the tumor to take control of its epidermal microenvironment (49). It is known that Wnt/β-catenin signaling is a key regulator of melanocyte-keratinocyte adhesion and interactions; however, the exact role it plays is complicated. Some studies indicate activation of Wnt/β-catenin signaling is associated with decreased melanoma cell proliferation and that loss of this signaling pathway might induce melanomegenesis (50). Indeed, Wnt/β-catenin signaling is important for melanocyte differentiation via activation of MITF expression and posttranslational processing (51). On the other hand, others have shown that Wnt/β-catenin signaling is essential for metastatic melanoma cell survival and its inhibition leads to reduced proliferation, migration, and invasion (52). These differing observations could result from differing influences of canonical (β-catenin dependent) or non-canonical Wnt signaling on melanomas during disease progression (53). Of note, active forms of vitamin D inhibit Wnt/β-catenin signaling in squamous cell carcinoma (54). Recently, there is evidence that points towards an inverse relationship with VDR expression and Wnt/β-catenin signaling in primary melanomas which yields reduced proliferation and immune response evasion (11). It could be that differences in VDR expression contribute to how Wnt/β-catenin signaling influences melanomas. The complex changes in vitamin D signaling and their roles in melanoma development, progression, and therapy have been also discussed recently (12, 55).
Also important in the crosstalk within the epidermal melanin unit is that VDR heterodimerizes with other nuclear receptors including retinoid X receptors (RXRs). We have previously shown that in a VDR null (VDR-/-) mouse model topically treated with the carcinogen 12-dimethyl-benz[a]anthracene (DMBA)-12-O-tetradecanoylphorbol-13 acetate (TPA) resulted in numerous melanocytic growths. In that same study, a separate mouse model harboring a conditional tissue-specific keratin 14 promoter-driven cre-mediated epidermal RXRα knockout (RXRαep-/- mice) also exhibited melanocytic growths (10). These data indicated that both absence of VDR and keratinocytic RXRα knockout stimulated melanocytic growth following tumor promoting treatment. This observation was further explored in additional mouse models in which keratinocytic RXRα knockout was combined with two melanomagenic mutational backgrounds (RXRαep-/-|CDk4R24C/R24C and RXRαep-/-|Tyr-NRASQ61K) and exposed to acute neonatal UVB irradiation in combination with adult chronic UVB doses. These mice exhibited increased melanocytic growth as had been seen previously. They also had elevated malignant melanocytic tumors and increased metastasis to the draining lymph nodes concurrent with a loss in skin expression of PTEN and P53 tumor suppressors (29). To further explore the contribution of keratinocytic RXRα towards melanomagenesis we generated a mouse model that combined the previous background mutations to generate a highly conducive mutational landscape (RXRαep-/-|Tyr-NRASQ61K|CDk4R24C/R24C). With this mouse model we observed the formation of spontaneous melanomas in the absence of UVB when keratinocytic RXRα was ablated. Following acute neonatal UVB irradiation, melanomas in adult keratinocytic RXRα ablated mice had increased radial and vertical growth phases, increased proliferation, increased angiogenesis, reduced apoptosis, and increased metastasis to the draining lymph nodes. We also noted in the tumor adjacent normal skin irradiated with UVB that there was increased expression of activated AKT, p21, and cyclin D1 with reduced expression of pro-apoptotic marker BAX (30).
A significantly higher percent of cells from benign human nevi samples exhibited nuclear localization and strong expression of RXRα (P < 0.0001) compared to melanomas (with or without metastasis) (31). In the same report, primary human melanoma samples exhibited significantly higher cytoplasmic expression of RXRα compared to the nevi (P = 0.018) or the melanomas with metastasis and in metastasis samples (P = 0.004). The nuclear vs cytoplasmic expression of transcription factor such as RXRα could be critical for regulating their target gene expression by limiting its interaction with their heterodimeric partners and cytoplasmic localization could be essential to mediate the non-genomic actions of RXRα. A previous study by Boehm et al. also showed decreased expression of RXRα in human melanocytic tumors (32). Above results argue for its anticancerogenic role and suggest a cell-autonomous role of melanocytic RXRα in melanoma suppression.
Interestingly, strong nuclear expression of RXRα is also reported in epidermal keratinocytes of normal human skin and we reported for the first time that its expression is reduced or lost in skin keratinocytes adjacent to melanocytic tumors during melanoma progression in humans (33) suggesting a non-cell autonomous role of keratinocytic RXRα in suppressing melanoma progression. In contrast, cytoplasmic intensity of RXRβ did not differ significantly between groups of nevi and melanoma. Although, cytoplasmic expression of RXRβ was significantly reduced in human metastasis samples compared to the human melanoma samples (31) indicating a role of RXRβ in mediating melanoma metastasis.
We have also shown that both VDR and keratinocytic RXRα contribute towards photoprotection of melanocytes against UVB radiation in vivo using preclinical studies in mice models. Using the RXRαep-/- mouse model subjected to acute neonatal UVB irradiation, we demonstrated that the absence of keratinocytic RXRα resulted in increased DNA damage, proliferation, and migration of melanocytes in vivo. We then confirmed these results ex vivo using primary melanocytes which exhibited increased growth in conditioned media generated from culturing isolated RXRα knockout keratinocytes. This was explained by increased expression of keratinocyte secreted growth factors ET-1, FGF2, and SCF in the skin of RXRαep-/- mice following UVB irradiation (34) underscoring a “non-cell autonomous” role of keratinocytic RXRα in UV-induced melanocyte homeostasis.
Interestingly, mice with melanocyte-specific ablation of RXRα and RXRβ (RXRαmel-/- | RXRβmel-/-) attract a reduced number of IFN-γ secreting immune cells than in wild-type mice following acute UVR, via altered expression of chemoattractive and chemorepulsive chemokines/cytokines. Reduced IFN-γ in the microenvironment modifies UVR-induced apoptosis, and due to this, the survival of dermal fibroblasts is significantly decreased in mice lacking RXRα/β (35). Results demonstrate that melanocytic-RXRs in a “non-cell autonomous” manner modulate post-UVR survival of dermal fibroblasts highlighting a role in immune surveillance, while independently in a “cell autonomous” manner regulate post-UVR melanocyte survival (35).
We have also demonstrated that melanocytic VDR also affords photoprotective properties in a different mouse model in which melanocytic VDR was ablated (VDRmel-/-). When knockout mice were subjected to acute neonatal UVB irradiation they exhibited fewer differentiated melanocytes with reduced proliferation, reduced apoptosis, and increased DNA damage (36).
Interestingly active forms of vitamin D3 show photoprotective activities in both melanocytes and keratinocytes (37–43) through various mechanisms also including the VDR (40, 44, 56, 57).
Altogether, above data highlight the importance of nuclear receptor signaling in melanocytes driven by VDR and its principal heterodimer partners RXRα and RXRβ in the regulation of melanocyte homeostasis and melanomagenesis in the skin and tumor microenvironment. Our data further underscores a non-cell autonomous role of RXRα both in keratinocytes and melanocytes of the skin in controlling melanocyte homeostasis and melanomagenesis.
Vitamin D Receptor Polymorphisms in Melanoma
The VDR gene is located on chromosome 12q13.11 and has 11 exons (58). Over 600 single nucleotide polymorphisms have been identified in the VDR gene including FokI (C/T-rs2228570, previously named rs10735810), TaqI (rs731236), BsmI (rs1544410), and ApaI (rs7975232) which are the most commonly analyzed in relation to melanoma (5). Cdx2 (rs11568820), EcoRV (rs4516035), BglI (rs739837) have also been studied in this context, but to a lesser extent.
The FokI polymorphism (C/T-rs2228570, previously named rs10735810) is located on exon 2 of the VDR gene (5). This polymorphism creates a new start codon 10 base pairs upstream from the usual start codon, leading to a longer VDR protein that is less active compared to the shorter protein variant. The shorter protein variant is 424 amino acids and corresponds to the C nucleotide allele or F allele, and the longer 427 amino acid variant corresponds to the F allele (59, 60). The TaqI polymorphism (rs731236) is located at codon 352 of exon 9 of the VDR gene, and functions as a restriction fragment length polymorphism (5). It creates a silent codon change of ATT to ATC, which both code for isoleucine (5, 61).
The BsmI polymorphism (rs1544410) also acts as a restriction fragment length polymorphism that results in a silent mutation (5, 61). It is located in intron 8 at the 3rd end of the VDR gene, thus it may affect VDR gene expression and mRNA stability (60). The ApaI polymorphism is located near the BsmI polymorphism, and thus, may have similar effects (5, 61). The Cdx2 (rs11568820) polymorphism is located in the promoter region of the VDR gene, and results in an adenine replacing a guanine (5, 61). The EcoRV polymorphism (rs4516035) is also located in the promoter region of the VDR gene, and is thought to play a role in the anticancer immune response (5, 62). Lastly, the BglI polymorphism (rs739837) is located near the stop codon in exon 9 (5).
A 2020 meta-analysis calculated the odds ratios and 95% confidence intervals for the dominant and recessive models for 7 VDR gene polymorphisms (63). The dominant model (Bb + BB vs. bb) of Bsml (rs1544410) showed a statistically significant 15% risk reduction in malignant melanoma incidence for carriers of the rarer allele B. Carriers of the rarer allele f (Ff + ff vs. FF) of FokI (rs2228570) were shown to be 22% more likely to develop malignant melanoma. Additionally, for ApaI (rs7975232), there is a 20% higher risk of melanoma for carriers of the rarer a allele (Aa + aa vs. AA). No significant association between melanoma risk and the other investigated VDR polymorphisms, which included TaqI (rs731236), A-1012G (rs4516035), Cdx2 (rs11568820), and BglI (rs739837), was found.
VDR Expression as a Prognostic Biomarker
One cohort-study assessed the relationship between VDR expression and prognostic factors in Central European cohort of melanoma patients (64, 65). VDR expression was quantified immunohistochemically in 69 cutaneous melanomas and compared to the tumors’ pTNM (pathological tumor, node, metastasis) stage, ulceration, and tumor-infiltrating lymphocytes. pTNM staging is based on the tumor (i.e., Breslow thickness, ulceration), spread to nearby lymph nodes, and distant metastases. The higher the tumor’s stage, the worse the prognosis.
Strongest and highest VDR expression was detected in the nuclei of epidermal keratinocytes for normal uninvolved skin compared to melanocytic lesions. For “nuclear localization”, VDR expression decreased in the following order: normal skin > melanocytic nevi > primary melanomas = metastases (64). For “cytoplasmic localization”, VDR expression decreased in the order: normal skin = melanocytic nevi > primary melanomas = metastases. Reduction in VDR expression with the development of the pigmented lesions was more evident in the nuclei than in the cell-cytoplasm suggesting a cell-autonomous role of canonical VDR signaling in the melanocytes during melanoma progression and metastasis (64).
Interestingly, VDR expression in the basal and supra-basal keratinocytes of the skin epidermis surrounding the melanocytic tumors was markedly lower in comparison to normal skin without any skin lesions, which also suggests a non-cell autonomous role of keratinocytic VDR in melanomagenesis (64). Further, high VDR expression both in primary and metastatic melanomas was a factor that favorably influenced the OS in melanoma cohort.
In melanoma, ulceration contributes to the tumor of pTNM staging, and is a hallmark of more aggressive tumors. Whereas, the presence of tumor-infiltrating lymphocytes in melanoma is associated with a favorable prognosis. Less advanced melanomas, like those with fewer than three lymph node metastases and those without distant metastases, had the strongest VDR expression (64, 65). Whereas tumors with indicators of poor prognosis like ulceration or non-brisk or absence of tumor-infiltrating lymphocytes, showed significantly lower VDR expression. Most importantly patients with metastatic disease and VDR-/- melanomas had the poorest probability of survival (64, 65). Interestingly, the expression of activating vitamin D enzyme CYP27B1 was inversely correlated with melanoma progression and overall and disease-free survival times and such correlation was amplified by a concomitant decrease in the VDR expression (55, 65, 66). While CYP24A1 levels were high in nevi and early-stage melanomas in comparison to normal epidermis, its level decreased during melanoma progression similarly to CYP27B1 and VDR (67). These findings indicate that vitamin D signaling system including VDR expression plays an important role in melanoma prognosis and may also be used as an additional prognostic biomarker. Similar trend was reported for ocular melanoma (68). Importantly, recent experimental studies have shown that knocking out of the VDR in melanoma cells increase their malignant behavior and decreases responsiveness to active form of vitamin D indicating that the VDR can serve as the melanoma tumor suppressor gene (69), which is consistent with the role of the VDR as the tumor suppressor gene in the skin as originally proposed by Bikle (44). Of note, defects in VDR lead to increased malignant behavior in other tumors including bladder, ovarian, lung and breast cancers, lymphomas (70–75).
There was a reverse correlation between melanin content and expression of the VDR and CYP27B1 as well as of RORα and γ in human melanoma samples (64, 66, 76). RORα and γ, alternative receptors for vitamin D-hydroxyderivatives, are expressed at lower levels in melanomas than in nevi and their expression decreases during melanoma progression, with lowest expression found in stage III and IV melanomas and in metastases (76). Interestingly, the expression of VDR as wells as of RORs was related to the HIF1α activity, which also affected FoxP3 expression in metastatic melanoma (77). Of note, melanogenesis can stimulate HIF1α expression and anaerobic glycolysis in melanoma cells (78) explaining in part the correlation between defects in VDR expression and signaling and defective responses to vitamin D in pigmented melanoma cells (64, 79, 80).
A separate study conducted by Muralidhar et al. analyzed 703 primary melanoma transcriptomes to better understand the role of vitamin D-VDR signaling (11). They found that VDR expression was independently protective against melanoma-related death in both primary and metastatic disease. VDR expression was shown to be inversely related to Wnt/β-catenin signaling, suggesting a mechanism for the anti-proliferative effects of vitamin D-VDR signaling. Additionally, increased VDR expression was associated with the upregulation of pathways involving the antitumor immune response as demonstrated by a greater abundance of tumor-infiltrating lymphocytes. This study further supports VDR’s utility as a prognostic biomarker, especially in those patients considering immunotherapy. It also establishes a causal relationship between vitamin D-VDR signaling and melanoma survival, suggesting that this mechanism could serve as a target for pharmacologic agents.
In addition to its generalized expression, the subcellular localization of VDR to the nucleus also could be beneficial as a biomarker for melanoma progression. Hutchinson et al. studied 34 benign nevi, 149 metastatic melanomas, and 44 matched metastases via immunohistology for the subcellular localization of VDR and phosphorylated ERK (p-ERK) as an indicator of MAPK activation (81). They found that as melanomas progressed, they exhibited reduced nuclear localization of VDR and increased cytoplasmic localization. Overall, expression of VDR decreased from benign nevi to metastatic melanoma and further decreased in metastasizing primary tumors. When they observed VDR localization in malignant melanomas known to have metastasized and compared them to those known to not have metastasized within five years, they saw nuclear VDR was reduced while there was no difference in cytoplasmic localization. They also found increased p-ERK consistent with cytoplasmic localization of VDR likely a result of the known mechanism of MAPK inhibition of VDR signaling when it is heterodimerized to RXRα via phosphorylation of serine 260 (82). These observations highlight the need for more research on the usefulness of VDR nuclear localization as a prognosticator for metastasizing melanomas.
Serum Vitamin D Levels and Prognosis
As part of the Leeds Melanoma Cohort, Newton-Bishop et al. reported an association between higher 25-hydroxyvitamin D3 serum levels at time of melanoma diagnosis and lower Breslow thickness (p value= .002) (83). Higher 25-hydroxyvitamin D3 levels were also found to be associated with increased survival independent of Breslow thickness. Several other studies have confirmed an association between higher serum vitamin D levels at diagnosis and better prognosis in melanoma (84–86). However, a more recent study asserts that rather than high levels of vitamin D being protective a deficiency in vitamin D (<25 nmol/L) actually shortens patient survival time from melanoma in a VDR-dependent manner (11).
Additionally, an observational single center study with estimated study completion date of January 2021, not yet published, is investigating the response to treatment with anti-programmed death 1 (PD-1) therapy in relation to serum vitamin D levels in 40 advanced melanoma patients (ClinicalTrials.gov Identifier: NCT03197636) (87). Serum levels of vitamin D will be measured at baseline, 3, and 6 weeks after initiation of treatment with anti-PD1 therapy followed by three years of observational follow-up. Response to treatment will be assessed at each visit within the study period and at follow-up.
Vitamin D, VDR and Immunotherapy
The issue of interference of active forms of vitamin D on immunotherapy deserves special attention, especially that immunotherapy represents the promising therapeutic approach against melanoma (88–95). In this context, inhibitory role of vitamin D in the adaptive immune responses (96, 97) requires explanation. Although it inhibits T cell responses in autoimmune responses (98), the evidence that it acts as an immunosuppressor is missing. On the opposite, it is inhibiting proinflammatory responses through VDR mediated inhibition of NFκβ and inverse agonism on RORγ and inhibition of oxidative stress through activation of NRF2-dependent pathways (45, 46, 57, 99). However, it is unclear to which degree, how, and whether it will inhibit anti-tumor T-cell responses. On the other hand, vitamin D activates the innate immune system (96, 97), which plays an important role in anti-tumor activity (100–106). Therefore, the actions of active forms of vitamin D can be defined as immunoregulatory, with their full definitions requiring future careful studies.
Vitamin D and Its Analogs in the Treatment of Melanoma
Several studies are investigating the use of vitamin D or its analogs as adjuvant treatment in melanoma patients with an understanding that different delivery routes will influence vit D activation (Figures 2B and see below).
One report that utilized data from the Women’s Health initiative (WHI) calcium/vitamin D randomized controlled trial, studied the effects of calcium and low-dose vitamin D on the risk of non-melanoma and melanoma skin cancers in post-menopausal women (107). Women ages 50-79 years (N=36,282) were randomly assigned to receive 1,000 mg of elemental calcium plus 400 IU of vitamin D3 daily or placebo for a mean follow-up period of seven years. Non-melanoma and melanoma skin cancer diagnoses were self-reported annually. The study concluded that the treatment group and control group showed no significant difference in the incidence of melanoma or non-melanoma skin cancers. However, women on the calcium/vitamin D regiment with a history of non-melanoma skin cancer had a reduced risk of melanoma as opposed to those receiving placebo (hazard ratio 0.43; 95% confidence interval: 0.21 to 0.90: P(interaction) =.038). It was also noted that this difference was not seen in women that did not have a history of non-melanoma skin cancer.
In 2010, the Australia and New Zealand Melanoma Trials Group conducted a pilot randomized placebo-controlled phase II trial, Mel-D, to investigate the safety and efficacy of adjuvant high-dose vitamin D administration in patients with cutaneous melanoma that had initially been treated with wide excision (Australian New Zealand Clinical Trials Registry #ACTRN12609000351213) (108, 109). The adjuvant treatment included an oral loading dose of 500,000 IU Vitamin D followed by a once monthly oral dose of 50,000 IU Vitamin D for two years. Patients in this study reportedly experienced an improvement in progression-free survival and overall survival.
The ongoing study, VidMe, is a multicenter randomized placebo-controlled phase III trial intended to examine the efficacy and long-term safety of high-dose vitamin D supplementation in 500 patients with melanoma (ClinicalTrials.gov Identifier: NCT01748448) (110, 111). Once a month, participants will either receive 100,000 IU of vitamin D or placebo (Arachidis oleum raffinatum). This study’s primary endpoint is relapse-free survival. They also plan to assess the expression of VDR in the primary tumor and its possible correlation with relapse. Secondarily, vitamin D levels at diagnosis will be correlated with melanoma site, subtype, and stage at diagnosis. Vitamin D levels will continue to be monitored after supplementation to determine if serum levels depend on the genetic variability of the vitamin D pathway. Additionally, they plan to investigate whether VDR immunoreactivity correlates with stage at diagnosis.
Vitamin D analogs have also exhibited promising photoprotective and anticancer properties (13, 57, 112) indicating their possible application to counteracting skin cancer, including melanomas. The anti-melanoma activity of the non-calcemic analog, 20(OH)D3, was shown in a preclinical in vivo model (113). 20(OH)D3 is non-calcemic but possesses similar antiproliferative activity in vitro when compared to 1,25(OH)2D3. Skobowiat et al. demonstrated decreased colony formation both in the monolayer and soft agar conditions when cells were treated with 20(OH)D3. 20(OH)D3 was also shown to inhibit melanoma cells in transwell migration and spheroid toxicity. Additionally, 20(OH)D3 decreased melanoma tumor growth in immunocompromised mice without obvious signs of toxicity. These results suggest that 20(OH)D3 is likely effective and safe, and thus, should undergo further preclinical testing as an antimelanoma therapy.
Therefore, cellular expression of RXRs and VDR in addition to their sub-cellular localization could be used as a prognostic biomarker for melanoma progression in humans. While vitamin D3 and its analogs are currently being explored in pre-clinical and clinical settings as a possible adjuvant therapy in the treatment of melanoma (107, 108, 110, 111, 113), in those individuals with decreased or dysfunctional VDR and RXR expression, vitamin D supplementation is unlikely to be beneficial. Thus, there is a need for a novel therapy that increases and/or restores functional VDR and RXR expression in conjunction with the supplementation of vit D or its analogs. Similarly, the in vivo anti-melanoma effects of the novel vit D analogs need to be established and the underlying mechanisms of action need to be deciphered.
Author Contributions
All authors have contributed intellectually for the preparation of the manuscript. All authors contributed to the article and approved the submitted version.
Funding
Research reported in this publication was supported in part by National Institute of Environmental Health Sciences (NIEHS) of the National Institutes of Health (NIH) under the award number 1R01ES016629-01A1 (PI :AI), the OSU/OHSU College of Pharmacy Pilot Project Grant (PI :AI), NIH grants to ATS including 1R01AR073004-01A1, R01AR071189-01A1, and a VA merit grant [no. 1I01BX004293-01A1 (PI ATS)], and a Training grant from the National Center for Complementary and Integrative Health (NCCIH) of the National Institutes of Health under award number T32AT010131.
Conflict of Interest
The authors declare that the research was conducted in the absence of any commercial or financial relationships that could be construed as a potential conflict of interest.
Publisher’s Note
All claims expressed in this article are solely those of the authors and do not necessarily represent those of their affiliated organizations, or those of the publisher, the editors and the reviewers. Any product that may be evaluated in this article, or claim that may be made by its manufacturer, is not guaranteed or endorsed by the publisher.
References
1. Matthews NH, Li W-Q, Qureshi AA, Weinstock MA, Cho E. “Epidemiology of Melanoma”, In: Cutaneous Melanoma: Etiology and Therapy. Brisbane (AU: Codon Publications. Available at: http://www.ncbi.nlm.nih.gov/books/NBK481862/ (Accessed February 17, 2021).
2. Siegel RL, Miller KD, Fuchs HE, Jemal A. Cancer Statistics, 2021. CA Cancer J Clin (2021) 71:7–33. doi: 10.3322/caac.21654
3. Watson M, Geller AC, Tucker MA, Guy GP, Weinstock MA. Melanoma Burden and Recent Trends Among Non-Hispanic Whites Aged 15–49 Years, United States. Prev Med (2016) 91:294–8. doi: 10.1016/j.ypmed.2016.08.032
4. Ballantine KR, Watson H, Macfarlane S, Winstanley M, Corbett RP, Spearing R, et al. Small Numbers, Big Challenges: Adolescent and Young Adult Cancer Incidence and Survival in New Zealand. J Adolesc Young Adult Oncol (2017) 6:277–85. doi: 10.1089/jayao.2016.0074
5. Vasilovici AF, Grigore LE, Ungureanu L, Fechete O, Candrea E, Trifa AP, et al. Vitamin D Receptor Polymorphisms and Melanoma. Oncol Lett (2019) 17:4162–9. doi: 10.3892/ol.2018.9733
6. Uong A, Zon LI. Melanocytes in Development and Cancer. J Cell Physiol (2010) 222:38–41. doi: 10.1002/jcp.21935
7. Davis LE, Shalin SC, Tackett AJ. Current State of Melanoma Diagnosis and Treatment. Cancer Biol Ther (2019) 20:1366–79. doi: 10.1080/15384047.2019.1640032
8. Domingues B, Lopes JM, Soares P, Pópulo H. Melanoma Treatment in Review. ImmunoTargets Ther (2018) 7:35–49. doi: 10.2147/ITT.S134842
9. Slominski AT, Carlson JA. Melanoma Resistance: A Bright Future for Academicians and a Challenge for Patient Advocates. Mayo Clin Proc (2014) 89:429–33. doi: 10.1016/j.mayocp.2014.02.009
10. Indra AK, Castaneda E, Antal MC, Jiang M, Messaddeq N, Meng X, et al. Malignant Transformation of DMBA/TPA-Induced Papillomas and Nevi in the Skin of Mice Selectively Lacking Retinoid-X-Receptor Alpha in Epidermal Keratinocytes. J Invest Dermatol (2007) 127:1250–60. doi: 10.1038/sj.jid.5700672
11. Muralidhar S, Filia A, Nsengimana J, Poźniak J, O’Shea SJ, Diaz JM, et al. Vitamin D-VDR Signaling Inhibits Wnt/β-Catenin-Mediated Melanoma Progression and Promotes Antitumor Immunity. Cancer Res (2019) 79:5986–98. doi: 10.1158/0008-5472.CAN-18-3927
12. Slominski AT, Brozyna AA, Zmijewski MA, Jozwicki W, Jetten AM, Mason RS, et al. Vitamin D Signaling and Melanoma: Role of Vitamin D and Its Receptors in Melanoma Progression and Management. Lab Invest (2017) 97:706–24. doi: 10.1038/labinvest.2017.3
13. Brozyna AA, Hoffman RM, Slominski AT. Relevance of Vitamin D in Melanoma Development, Progression and Therapy. Anticancer Res (2020) 40:473–89. doi: 10.21873/anticanres.13976
14. Stumpf WE, Sar M, Reid FA, Tanaka Y, DeLuca HF. Target Cells for 1,25-Dihydroxyvitamin D3 in Intestinal Tract, Stomach, Kidney, Skin, Pituitary, and Parathyroid. Science (1979) 206:1188–90. doi: 10.1126/science.505004
15. Orlow I, Roy P, Reiner AS, Yoo S, Patel H, Paine S, et al. Vitamin D Receptor Polymorphisms in Patients With Cutaneous Melanoma. Int J Cancer J Int Cancer (2012) 130:405–18. doi: 10.1002/ijc.26023
16. Colston K, Colston MJ, Feldman D. 1,25-Dihydroxyvitamin D3 and Malignant Melanoma: The Presence of Receptors and Inhibition of Cell Growth in Culture. Endocrinology (1981) 108:1083–6. doi: 10.1210/endo-108-3-1083
17. Evans SR, Houghton AM, Schumaker L, Brenner RV, Buras RR, Davoodi F, et al. Vitamin D Receptor and Growth Inhibition by 1,25-Dihydroxyvitamin D3 in Human Malignant Melanoma Cell Lines. J Surg Res (1996) 61:127–33. doi: 10.1006/jsre.1996.0092
18. Ranson M, Posen S, Mason RS. Human Melanocytes as a Target Tissue for Hormones: In Vitro Studies With 1 Alpha-25, Dihydroxyvitamin D3, Alpha-Melanocyte Stimulating Hormone, and Beta-Estradiol. J Invest Dermatol (1988) 91:593–8. doi: 10.1111/1523-1747.ep12477126
20. Bouillon R, Marcocci C, Carmeliet G, Bikle D, White JH, Dawson-Hughes B, et al. Skeletal and Extraskeletal Actions of Vitamin D: Current Evidence and Outstanding Questions. Endocr Rev (2018) 40:1109–51. doi: 10.1210/er.2018-00126
21. Bikle DD. The Vitamin D Receptor as Tumor Suppressor in Skin. Adv Exp Med Biol (2020) 1268:285–306. doi: 10.1007/978-3-030-46227-7_14
22. Jenkinson C. The Vitamin D Metabolome: An Update on Analysis and Function. Cell Biochem Funct (2019) 37:408–23. doi: 10.1002/cbf.3421
23. Tuckey RC, Cheng CYS, Slominski AT. The Serum Vitamin D Metabolome: What We Know and What Is Still to Discover. J Steroid Biochem Mol Biol (2019) 186:4–21. doi: 10.1016/j.jsbmb.2018.09.003
24. Bikle D, Christakos S. New Aspects of Vitamin D Metabolism and Action - Addressing the Skin as Source and Target. Nat Rev Endocrinol (2020) 16:234–52. doi: 10.1038/s41574-019-0312-5
25. Guryev O, Carvalho RA, Usanov S, Gilep A, Estabrook RW. A Pathway for the Metabolism of Vitamin D3: Unique Hydroxylated Metabolites Formed During Catalysis With Cytochrome P450scc (Cyp11a1. Proc Natl Acad Sci USA (2003) 100:14754–9. doi: 10.1073/pnas.2336107100
26. Slominski A, Semak I, Zjawiony J, Wortsman J, Li W, Szczesniewski A, et al. The Cytochrome P450scc System Opens an Alternate Pathway of Vitamin D3 Metabolism. FEBS J (2005) 272:4080–90. doi: 10.1111/j.1742-4658.2005.04819.x
27. Slominski AT, Kim TK, Li W, Postlethwaite A, Tieu EW, Tang EK, et al. Detection of Novel CYP11A1-Derived Secosteroids in the Human Epidermis and Serum and Pig Adrenal Gland. Sci Rep (2015) 5:14875. doi: 10.1038/srep14875
28. Slominski AT, Li W, Kim TK, Semak I, Wang J, Zjawiony JK, et al. Novel Activities of CYP11A1 and Their Potential Physiological Significance. J Steroid Biochem Mol Biol (2015) 151:25–37. doi: 10.1016/j.jsbmb.2014.11.010
29. Coleman DJ, Chagani S, Hyter S, Sherman AM, Löhr CV, Liang X, et al. Loss of Keratinocytic Rxrα Combined With Activated CDK4 or Oncogenic NRAS Generates UVB-Induced Melanomas via Loss of P53 and PTEN in the Tumor Microenvironment. Mol Cancer Res MCR (2015) 13:186–96. doi: 10.1158/1541-7786.MCR-14-0164
30. Chagani S, Wang R, Carpenter EL, Löhr CV, Ganguli-Indra G, Indra AK. Ablation of Epidermal Rxrα in Cooperation With Activated CDK4 and Oncogenic NRAS Generates Spontaneous and Acute Neonatal UVB Induced Malignant Metastatic Melanomas. BMC Cancer (2017) 17:736. doi: 10.1186/s12885-017-3714-6
31. Chakravarti N, Lotan R, Diwan AH, Warneke CL, Johnson MM, Prieto VG. Decreased Expression of Retinoid Receptors in Melanoma: Entailment in Tumorigenesis and Prognosis. Clin Cancer Res (2007) 13:4817–24. doi: 10.1158/1078-0432.ccr-06-3026
32. Boehm N, Samama B, Cribier B, Rochette-Egly C. Retinoic-Acid Receptor Beta Expression in Melanocytes. Eur J Dermatol EJD (2004) 14:19–23.
33. Hyter S, Bajaj G, Liang X, Barbacid M, Ganguli-Indra G, Indra AK. Loss of Nuclear Receptor Rxrα in Epidermal Keratinocytes Promotes the Formation of Cdk4-Activated Invasive Melanomas. Pigment Cell Melanoma Res (2010) 23:635–48. doi: 10.1111/j.1755-148X.2010.00732.x
34. Wang Z, Coleman DJ, Bajaj G, Liang X, Ganguli-Indra G, Indra AK. Rxrα Ablation in Epidermal Keratinocytes Enhances UVR-Induced DNA Damage, Apoptosis, and Proliferation of Keratinocytes and Melanocytes. J Invest Dermatol (2011) 131:177–87. doi: 10.1038/jid.2010.290
35. Coleman DJ, Garcia G, Hyter S, Jang HS, Chagani S, Liang X, et al. Retinoid-X-Receptors (α/β) in Melanocytes Modulate Innate Immune Responses and Differentially Regulate Cell Survival Following UV Irradiation. PloS Genet (2014) 10:e1004321. doi: 10.1371/journal.pgen.1004321
36. Chagani S, Kyryachenko S, Yamamoto Y, Kato S, Ganguli-Indra G, Indra AK. In Vivo Role of Vitamin D Receptor Signaling in UVB-Induced DNA Damage and Melanocyte Homeostasis. J Invest Dermatol (2016) 136:2108–11. doi: 10.1016/j.jid.2016.06.004
37. Gordon-Thomson C, Tongkao-On W, Song EJ, Carter SE, Dixon KM, Mason RS. Protection From Ultraviolet Damage and Photocarcinogenesis by Vitamin D Compounds. Adv Exp Med Biol (2014) 810:303–28. doi: 10.1007/978-1-4939-0437-2_17
38. Slominski AT, Janjetovic Z, Kim TK, Wasilewski P, Rosas S, Hanna S, et al. Novel Non-Calcemic Secosteroids That Are Produced by Human Epidermal Keratinocytes Protect Against Solar Radiation. J Steroid Biochem Mol Biol (2015) 148:52–63. doi: 10.1016/j.jsbmb.2015.01.014
39. Tongkao-On W, Carter S, Reeve VE, Dixon KM, Gordon-Thomson C, Halliday GM, et al. CYP11A1 in Skin: An Alternative Route to Photoprotection by Vitamin D Compounds. J Steroid Biochem Mol Biol (2015) 148:72–8. doi: 10.1016/j.jsbmb.2014.11.015
40. Rybchyn MS, De Silva WGM, Sequeira VB, Mccarthy BY, Dilley AV, Dixon KM, et al. Enhanced Repair of UV-Induced DNA Damage by 1,25-Dihydroxyvitamin D3 in Skin Is Linked to Pathways That Control Cellular Energy. J Invest Dermatol (2018) 138:1146–56. doi: 10.1016/j.jid.2017.11.037
41. Chaiprasongsuk A, Janjetovic Z, Kim TK, Jarrett SG, D’orazio JA, Holick MF, et al. Protective Effects of Novel Derivatives of Vitamin D3 and Lumisterol Against UVB-Induced Damage in Human Keratinocytes Involve Activation of Nrf2 and P53 Defense Mechanisms. Redox Biol (2019) 24:101206. doi: 10.1016/j.redox.2019.101206
42. Chaiprasongsuk A, Janjetovic Z, Kim TK, Schwartz CJ, Tuckey RC, Tang EKY, et al. Hydroxylumisterols, Photoproducts of Pre-Vitamin D3, Protect Human Keratinocytes Against UVB-Induced Damage. Int J Mol Sci (2020) 21:9374. doi: 10.3390/ijms21249374
43. Chaiprasongsuk A, Janjetovic Z, Kim TK, Tuckey RC, Li W, Raman C, et al. CYP11A1-Derived Vitamin D3 Products Protect Against UVB-Induced Inflammation and Promote Keratinocytes Differentiation. Free Radic Biol Med (2020) 155:87–98. doi: 10.1016/j.freeradbiomed.2020.05.016
44. Bikle DD. Vitamin D Receptor, a Tumor Suppressor in Skin. Can J Physiol Pharmacol (2015) 93:349–54. doi: 10.1139/cjpp-2014-0367
45. Slominski AT, Chaiprasongsuk A, Janjetovic Z, Kim TK, Stefan J, Slominski RM, et al. Photoprotective Properties of Vitamin D and Lumisterol Hydroxyderivatives. Cell Biochem Biophys (2020) 78(2):165–80. doi: 10.1007/s12013-020-00913-6
46. Slominski RM, Stefan J, Athar M, Holick MF, Jetten AM, Raman C, et al. COVID-19 and Vitamin D: A Lesson From the Skin. Exp Dermatol (2020) 29(9):885–90. doi: 10.1111/exd.14170
47. Slominski A, Tobin DJ, Shibahara S, Wortsman J. Melanin Pigmentation in Mammalian Skin and Its Hormonal Regulation. Physiol Rev (2004) 84:1155–228. doi: 10.1152/physrev.00044.2003
48. Brandner JM, Haass NK. Melanoma’s Connections to the Tumour Microenvironment. Pathol (Phila) (2013) 45:443–52. doi: 10.1097/PAT.0b013e328363b3bd
49. Gurzu S, Beleaua MA, Jung I. The Role of Tumor Microenvironment in Development and Progression of Malignant Melanomas - a Systematic Review. Romanian J Morphol Embryol Rev Roum Morphol Embryol (2018) 59:23–8.
50. Chien AJ, Moore EC, Lonsdorf AS, Kulikauskas RM, Rothberg BG, Berger AJ, et al. Activated Wnt/beta-Catenin Signaling in Melanoma Is Associated With Decreased Proliferation in Patient Tumors and a Murine Melanoma Model. Proc Natl Acad Sci USA (2009) 106:1193–8. doi: 10.1073/pnas.0811902106
51. Vance KW, Goding CR. The Transcription Network Regulating Melanocyte Development and Melanoma. Pigment Cell Res (2004) 17:318–25. doi: 10.1111/j.1600-0749.2004.00164.x
52. Sinnberg T, Menzel M, Ewerth D, Sauer B, Schwarz M, Schaller M, et al. β-Catenin Signaling Increases During Melanoma Progression and Promotes Tumor Cell Survival and Chemoresistance. PloS One (2011) 6:e23429. doi: 10.1371/journal.pone.0023429
53. Gajos-Michniewicz A, Czyz M. WNT Signaling in Melanoma. Int J Mol Sci (2020) 21:E4852. doi: 10.3390/ijms21144852
54. Oak ASW, Bocheva G, Kim T-K, Brożyna AA, Janjetovic Z, Athar M, et al. Noncalcemic Vitamin D Hydroxyderivatives Inhibit Human Oral Squamous Cell Carcinoma and Down-Regulate Hedgehog and WNT/β-Catenin Pathways. Anticancer Res (2020) 40:2467–74. doi: 10.21873/anticanres.14216
55. Slominski AT, Brozyna AA, Skobowiat C, Zmijewski MA, Kim TK, Janjetovic Z, et al. On the Role of Classical and Novel Forms of Vitamin D in Melanoma Progression and Management. J Steroid Biochem Mol Biol (2018) 177:159–70. doi: 10.1016/j.jsbmb.2017.06.013
56. Bikle DD, Oda Y, Tu CL, Jiang Y. Novel Mechanisms for the Vitamin D Receptor (VDR) in the Skin and in Skin Cancer. J Steroid Biochem Mol Biol (2015) 148:47–51. doi: 10.1016/j.jsbmb.2014.10.017
57. Slominski AT, Brozyna AA, Zmijewski MA, Janjetovic Z, Kim TK, Slominski RM, et al. The Role of Classical and Novel Forms of Vitamin D in the Pathogenesis and Progression of Non-Melanoma Skin Cancers. (2020) 1268:257–83. doi: 10.1007/978-3-030-46227-7_13
58. Miyamoto K, Kesterson RA, Yamamoto H, Taketani Y, Nishiwaki E, Tatsumi S, et al. Structural Organization of the Human Vitamin D Receptor Chromosomal Gene and Its Promoter. Mol Endocrinol Baltim Md (1997) 11:1165–79. doi: 10.1210/mend.11.8.9951
59. Saijo T, Ito M, Takeda E, Huq AH, Naito E, Yokota I, et al. A Unique Mutation in the Vitamin D Receptor Gene in Three Japanese Patients With Vitamin D-Dependent Rickets Type II: Utility of Single-Strand Conformation Polymorphism Analysis for Heterozygous Carrier Detection. Am J Hum Genet (1991) 49:668–73.
60. Uitterlinden AG, Fang Y, Van Meurs JBJ, Pols HAP, Van Leeuwen JPTM. Genetics and Biology of Vitamin D Receptor Polymorphisms. Gene (2004) 338:143–56. doi: 10.1016/j.gene.2004.05.014
61. Hustmyer FG, DeLuca HF, Peacock M. ApaI, BsmI, EcoRV and TaqI Polymorphisms at the Human Vitamin D Receptor Gene Locus in Caucasians, Blacks and Asians. Hum Mol Genet (1993) 2:487. doi: 10.1093/hmg/2.4.487
62. Halsall JA, Osborne JE, Potter L, Pringle JH, Hutchinson PE. A Novel Polymorphism in the 1A Promoter Region of the Vitamin D Receptor Is Associated With Altered Susceptibilty and Prognosis in Malignant Melanoma. Br J Cancer (2004) 91:765–70. doi: 10.1038/sj.bjc.6602006
63. Birke M, Schöpe J, Wagenpfeil S, Vogt T, Reichrath J. Association of Vitamin D Receptor Gene Polymorphisms With Melanoma Risk: A Meta-Analysis and Systematic Review. Anticancer Res (2020) 40:583–95. doi: 10.21873/anticanres.13988
64. Brozyna AA, Jozwicki W, Janjetovic Z, Slominski AT. Expression of Vitamin D Receptor Decreases During Progression of Pigmented Skin Lesions. Hum Pathol (2011) 42:618–31. doi: 10.1016/j.humpath.2010.09.014
65. Brozyna AA, Jozwicki W, Slominski AT. Decreased VDR Expression in Cutaneous Melanomas as Marker of Tumor Progression: New Data and Analyses. Anticancer Res (2014) 34:2735–43.
66. Brozyna AA, Jozwicki W, Janjetovic Z, Slominski AT. Expression of the Vitamin D-Activating Enzyme 1alpha-Hydroxylase (CYP27B1) Decreases During Melanoma Progression. Hum Pathol (2013) 44:374–87. doi: 10.1016/j.humpath.2012.03.031
67. Brozyna AA, Jochymski C, Janjetovic Z, Jozwicki W, Tuckey RC, Slominski AT. CYP24A1 Expression Inversely Correlates With Melanoma Progression: Clinic-Pathological Studies. Int J Mol Sci (2014) 15:19000–17. doi: 10.3390/ijms151019000
68. Markiewicz A, Brozyna AA, Podgorska E, Elas M, Urbanska K, Jetten AM, et al. Vitamin D Receptors (VDR), Hydroxylases CYP27B1 and CYP24A1 and Retinoid-Related Orphan Receptors (ROR) Level in Human Uveal Tract and Ocular Melanoma With Different Melanization Levels. Sci Rep (2019) 9:9142. doi: 10.1038/s41598-019-45161-8
69. Podgorska E, Kim TK, Janjetovic Z, Urbanska K, Tuckey RC, Bae S, et al. Knocking Out the Vitamin D Receptor Enhances Malignancy and Decreases Responsiveness to Vitamin D3 Hydroxyderivatives in Human Melanoma Cells. Cancers Basel (2021) 13:3111. doi: 10.3390/cancers13133111
70. Srinivasan M, Parwani AV, Hershberger PA, Lenzner DE, Weissfeld JL. Nuclear Vitamin D Receptor Expression Is Associated With Improved Survival in Non-Small Cell Lung Cancer. J Steroid Biochem Mol Biol (2011) 123:30–6. doi: 10.1016/j.jsbmb.2010.10.002
71. Jozwicki W, Brozyna AA, Siekiera J, Slominski AT. Expression of Vitamin D Receptor (VDR) Positively Correlates With Survival of Urothelial Bladder Cancer Patients. Int J Mol Sci (2015) 16:24369–86. doi: 10.3390/ijms161024369
72. Al-Azhri J, Zhang Y, Bshara W, Zirpoli G, Mccann SE, Khoury T, et al. Tumor Expression of Vitamin D Receptor and Breast Cancer Histopathological Characteristics and Prognosis. Clin Cancer Res (2017) 23:97–103. doi: 10.1158/1078-0432.CCR-16-0075
73. Atoum M, Alzoughool F. Vitamin D and Breast Cancer: Latest Evidence and Future Steps. Breast Cancer Auckl (2017) 11:1178223417749816. doi: 10.1177/1178223417749816
74. Gascoyne DM, Lyne L, Spearman H, Buffa FM, Soilleux EJ, Banham AH. Vitamin D Receptor Expression in Plasmablastic Lymphoma and Myeloma Cells Confers Susceptibility to Vitamin D. Endocrinology (2017) 158:503–15. doi: 10.1210/en.2016-1802
75. Brozyna AA, Kim TK, Zablocka M, Jozwicki W, Yue J, Tuckey RC, et al. Association Among Vitamin D, Retinoic Acid-Related Orphan Receptors, and Vitamin D Hydroxyderivatives in Ovarian Cancer. Nutrients (2020) 12:3541. doi: 10.3390/nu12113541
76. Brozyna AA, Jozwicki W, Skobowiat C, Jetten A, Slominski AT. RORalpha and RORgamma Expression Inversely Correlates With Human Melanoma Progression. Oncotarget (2016) 7:63261–82. doi: 10.18632/oncotarget.11211
77. Brozyna AA, Jozwicki W, Jetten AM, Slominski AT. On the Relationship Between VDR, RORalpha and RORgamma Receptors Expression and HIF1-Alpha Levels in Human Melanomas. Exp Dermatol (2019) 28:1036–43. doi: 10.1111/exd.14002
78. Slominski A, Kim TK, Brozyna AA, Janjetovic Z, Brooks DL, Schwab LP, et al. The Role of Melanogenesis in Regulation of Melanoma Behavior: Melanogenesis Leads to Stimulation of HIF-1alpha Expression and HIF-Dependent Attendant Pathways. Arch Biochem Biophys (2014) 563:79–93. doi: 10.1016/j.abb.2014.06.030
79. Janjetovic Z, Brozyna AA, Tuckey RC, Kim TK, Nguyen MN, Jozwicki W, et al. High Basal NF-kappaB Activity in Nonpigmented Melanoma Cells Is Associated With an Enhanced Sensitivity to Vitamin D3 Derivatives. Br J Cancer (2011) 105:1874–84. doi: 10.1038/bjc.2011.458
80. Brozyna AA, Jozwicki W, Carlson JA, Slominski AT. Melanogenesis Affects Overall and Disease-Free Survival in Patients With Stage III and IV Melanoma. Hum Pathol (2013) 44:2071–4. doi: 10.1016/j.humpath.2013.02.022
81. Hutchinson PE, Halsall JA, Popovici S, Papadogeorgakis E, Osborne JE, Powley IR, et al. Compromised Vitamin D Receptor Signalling in Malignant Melanoma Is Associated With Tumour Progression and Mitogen-Activated Protein Kinase Activity. Melanoma Res (2018) 28:410–22. doi: 10.1097/CMR.0000000000000475
82. Solomon C, White JH, Kremer R. Mitogen-Activated Protein Kinase Inhibits 1,25-Dihydroxyvitamin D3-Dependent Signal Transduction by Phosphorylating Human Retinoid X Receptor Alpha. J Clin Invest (1999) 103:1729–35. doi: 10.1172/JCI6871
83. Newton-Bishop JA, Beswick S, Randerson-Moor J, Chang Y-M, Affleck P, Elliott F, et al. Serum 25-Hydroxyvitamin D3 Levels Are Associated With Breslow Thickness at Presentation and Survival From Melanoma. J Clin Oncol Off J Am Soc Clin Oncol (2009) 27:5439–44. doi: 10.1200/JCO.2009.22.1135
84. Fang S, Sui D, Wang Y, Liu H, Chiang Y-J, Ross MI, et al. Association of Vitamin D Levels With Outcome in Patients With Melanoma After Adjustment For C-Reactive Protein. J Clin Oncol Off J Am Soc Clin Oncol (2016) 34:1741–7. doi: 10.1200/JCO.2015.64.1357
85. Saiag P, Aegerter P, Vitoux D, Lebbé C, Wolkenstein P, Dupin N, et al. Prognostic Value of 25-Hydroxyvitamin D3 Levels at Diagnosis and During Follow-Up in Melanoma Patients. JNCI J Natl Cancer Inst (2015) 107:djv264. doi: 10.1093/jnci/djv264
86. Gambichler T, Bindsteiner M, Höxtermann S, Kreuter A. Serum 25-Hydroxyvitamin D Serum Levels in a Large German Cohort of Patients With Melanoma. Br J Dermatol (2013) 168:625–8. doi: 10.1111/j.1365-2133.2012.11212.x
87. Schmidt H. An Observational Study Design to Detect If Co-Stimulatory Markers and Vitamin D Status in Anti-PD-1 Treated Advanced Melanoma Patients Can Predict Treatment Outcome (2020). clinicaltrials.gov. Available at: https://clinicaltrials.gov/ct2/show/results/NCT03197636 (Accessed July 1, 2021).
88. Fillon M. Fecal Microbiota Transplants may Aid Melanoma Immunotherapy Resistance. CA Cancer J Clin (2021) 71:285–6. doi: 10.3322/caac.21676
89. Trojaniello C, Luke JJ, Ascierto PA. Therapeutic Advancements Across Clinical Stages in Melanoma, With a Focus on Targeted Immunotherapy. Front Oncol (2021) 11:670726. doi: 10.3389/fonc.2021.670726
90. Puglisi R, Bellenghi M, Pontecorvi G, Pallante G, Carè A, Mattia G. Biomarkers for Diagnosis, Prognosis and Response to Immunotherapy in Melanoma. Cancers (2021) 13:2875. doi: 10.3390/cancers13122875
91. Burzi L, Alessandrini AM, Quaglino P, Piraccini BM, Dika E, Ribero S. Cutaneous Events Associated With Immunotherapy of Melanoma: A Review. J Clin Med (2021) 10:3047. doi: 10.3390/jcm10143047
92. Naik PP. Current Trends of Immunotherapy in the Treatment of Cutaneous Melanoma: A Review. Dermatol Ther (2021). doi: 10.1007/s13555-021-00583-z
93. Rohatgi A, Kirkwood JM. Beyond PD-1: The Next Frontier for Immunotherapy in Melanoma. Front Oncol (2021) 11:640314. doi: 10.3389/fonc.2021.640314
94. Buchanan T, Amouzegar A, Luke JJ. Next-Generation Immunotherapy Approaches in Melanoma. Curr Oncol Rep (2021) 23:116. doi: 10.1007/s11912-021-01104-z
95. Moreira A, Heinzerling L, Bhardwaj N, Friedlander P. Current Melanoma Treatments: Where Do We Stand? Cancers (2021) 13:E221. doi: 10.3390/cancers13020221
96. Chun RF, Liu PT, Modlin RL, Adams JS, Hewison M. Impact of Vitamin D on Immune Function: Lessons Learned From Genome-Wide Analysis. Front Physiol (2014) 5:151. doi: 10.3389/fphys.2014.00151
97. Hewison M. An Update on Vitamin D and Human Immunity. Clin Endocrinol (Oxf) (2012) 76:315–25. doi: 10.1111/j.1365-2265.2011.04261.x
98. Postlethwaite AE, Tuckey RC, Kim T-K, Li W, Bhattacharya SK, Myers LK, et al. 20s-Hydroxyvitamin D3, a Secosteroid Produced in Humans, Is Anti-Inflammatory and Inhibits Murine Autoimmune Arthritis. Front Immunol (2021) 12:678487. doi: 10.3389/fimmu.2021.678487
99. Slominski RM, Raman C, Elmets C, Jetten AM, Slominski A, Tuckey RC. The Significance of CYP11A1 Expression in Skin Physiology and Pathology. Mol Cell Endocrinol (2021) 530:111238. doi: 10.1016/j.mce.2021.111238
100. Jacquelot N, Belz GT. Type 2 Innate Lymphoid Cells: A Novel Actor in Anti-Melanoma Immunity. Oncoimmunology (2021) 10:1943168. doi: 10.1080/2162402X.2021.1943168
101. Wang H, Zhang L, Yang L, Liu C, Zhang Q, Zhang L. Targeting Macrophage Anti-Tumor Activity to Suppress Melanoma Progression. Oncotarget (2017) 8:18486–96. doi: 10.18632/oncotarget.14474
102. Marzagalli M, Ebelt ND, Manuel ER. Unraveling the Crosstalk Between Melanoma and Immune Cells in the Tumor Microenvironment. Semin Cancer Biol (2019) 59:236–50. doi: 10.1016/j.semcancer.2019.08.002
103. Vacca P, Pietra G, Tumino N, Munari E, Mingari MC, Moretta L. Exploiting Human NK Cells in Tumor Therapy. Front Immunol (2019) 10:3013. doi: 10.3389/fimmu.2019.03013
104. Gonzalez-Gugel E, Saxena M, Bhardwaj N. Modulation of Innate Immunity in the Tumor Microenvironment. Cancer Immunol Immunother CII (2016) 65:1261–8. doi: 10.1007/s00262-016-1859-9
105. Atherton MJ, Morris JS, McDermott MR, Lichty BD. Cancer Immunology and Canine Malignant Melanoma: A Comparative Review. Vet Immunol Immunopathol (2016) 169:15–26. doi: 10.1016/j.vetimm.2015.11.003
106. Navarini-Meury AA, Conrad C. Melanoma and Innate Immunity–Aactive Inflammation or Just Erroneous Attraction? Melanoma as the Source of Leukocyte-Attracting Chemokines. Semin Cancer Biol (2009) 19:84–91. doi: 10.1016/j.semcancer.2008.10.012
107. Tang JY, Fu T, Leblanc E, Manson JE, Feldman D, Linos E, et al. Calcium Plus Vitamin D Supplementation and the Risk of Nonmelanoma and Melanoma Skin Cancer: Post Hoc Analyses of the Women’s Health Initiative Randomized Controlled Trial. J Clin Oncol Off J Am Soc Clin Oncol (2011) 29:3078–84. doi: 10.1200/JCO.2011.34.5967
108. Saw RP, Armstrong BK, Mason RS, Morton RL, Shannon KF, Spillane AJ, et al. Adjuvant Therapy With High Dose Vitamin D Following Primary Treatment of Melanoma at High Risk of Recurrence: A Placebo Controlled Randomised Phase II Trial (ANZMTG 02.09 Mel-D). BMC Cancer (2014) 14:780. doi: 10.1186/1471-2407-14-780
109. Stucci LS, D’Oronzo S, Tucci M, Macerollo A, Ribero S, Spagnolo F, et al. Vitamin D in Melanoma: Controversies and Potential Role in Combination With Immune Check-Point Inhibitors. Cancer Treat Rev (2018) 69:21–8. doi: 10.1016/j.ctrv.2018.05.016
110. De Smedt J, Van Kelst S, Boecxstaens V, Stas M, Bogaerts K, Vanderschueren D, et al. Vitamin D Supplementation in Cutaneous Malignant Melanoma Outcome (ViDMe): A Randomized Controlled Trial. BMC Cancer (2017) 17:562. doi: 10.1186/s12885-017-3538-4
111. Universitaire Ziekenhuizen Leuven. Vitamin D Supplementation in Cutaneous Malignant Melanoma Outcome (2020). clinicaltrials.gov. Available at: https://clinicaltrials.gov/ct2/show/NCT01748448 (Accessed July 1, 2021).
112. Slominski AT, Kim TK, Janjetovic Z, Brozyna AA, Zmijewski MA, Xu H, et al. Differ Overlapping Eff Of (2018) 19:E3072. doi: 10.3390/ijms19103072
Keywords: melanoma, vitamin D receptor (VDR), vitamin D3 metabolite, therapy, pathogenesis, tumor microenvironment, polymorphisms, heterodimers
Citation: Becker AL, Carpenter EL, Slominski AT and Indra AK (2021) The Role of the Vitamin D Receptor in the Pathogenesis, Prognosis, and Treatment of Cutaneous Melanoma. Front. Oncol. 11:743667. doi: 10.3389/fonc.2021.743667
Received: 19 July 2021; Accepted: 15 September 2021;
Published: 06 October 2021.
Edited by:
Vijayasaradhi Setaluri, University of Wisconsin-Madison, United StatesReviewed by:
Sanjay Premi, Moffitt Cancer Center & Research Institute, United StatesChandra K. Singh, University of Wisconsin-Madison, United States
Copyright © 2021 Becker, Carpenter, Slominski and Indra. This is an open-access article distributed under the terms of the Creative Commons Attribution License (CC BY). The use, distribution or reproduction in other forums is permitted, provided the original author(s) and the copyright owner(s) are credited and that the original publication in this journal is cited, in accordance with accepted academic practice. No use, distribution or reproduction is permitted which does not comply with these terms.
*Correspondence: Arup K. Indra, YXJ1cC5pbmRyYUBvcmVnb25zdGF0ZS5lZHU=