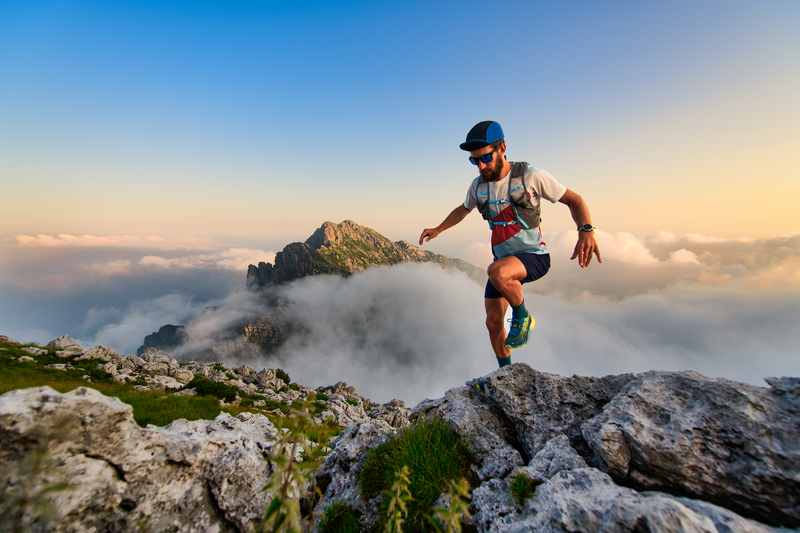
94% of researchers rate our articles as excellent or good
Learn more about the work of our research integrity team to safeguard the quality of each article we publish.
Find out more
REVIEW article
Front. Oncol. , 17 November 2021
Sec. Cancer Immunity and Immunotherapy
Volume 11 - 2021 | https://doi.org/10.3389/fonc.2021.738323
Photodynamic therapy (PDT) is a low invasive antitumor therapy with fewer side effects. On the other hand, immunotherapy also has significant clinical applications in the treatment of cancer. Both therapies, on their own, have some limitations and are incapable of meeting the demands of the current cancer treatment. The efficacy of PDT and immunotherapy against tumor metastasis and tumor recurrence may be improved by combination strategies. In this review, we discussed the possibility that PDT could be used to activate immune responses by inducing immunogenic cell death or generating cancer vaccines. Furthermore, we explored the latest advances in PDT antitumor therapy in combination with some immunotherapy such as immune adjuvants, inhibitors of immune suppression, and immune checkpoint blockade.
Cancers are chronologic diseases that seriously threaten human life. Many strategies have been developed for cancer treatment, including chemotherapy, radiotherapy, surgery, and targeted therapy, which are found to be effective for some malignant tumors (1, 2). However, metastasis, recurrence, heterogeneity, resistance to chemotherapy and radiotherapy, and avoidance of immunological surveillance are the most common reasons for cancer treatment failure. Therefore, new therapies with targeted and less invasive features are needed for cancer treatment. In 1903, Tappeiner and Jesionek used white light and eosin to treat skin tumors, setting a photodynamic therapy (PDT) model to treat tumors (3). PDT is a minimally invasive therapy that generates cytotoxic reactive oxygen species (ROS) through a light source, molecular oxygen, and organic macrocycles called photosensitizers (PSs) (4, 5). Currently, nanomaterials are widely used as PS carriers due to their better cytocompatibility, lower cytotoxicity, and excellent tumor targeting compared with traditional small-molecule PSs (6, 7). The enhanced permeability and retention (EPR) effect-based nanomedicine has been widely used for tumor targeting during the past decades. PDT only produces ROS in the tumor after local irradiation with excitation light, making it less invasive and confined. Furthermore, PDT has shown promising results in the diagnosis and treatment of cancers, such as breast, colorectal, and skin cancers, because of the mechanism of action of PSs, which does not cause drug tolerance (8–10). PDT can also induce immunogenic cell death (ICD), promote the release of tumor-associated antigens (TAAs) from tumor cell remnants, and increase the proliferation, activation, and infiltration of antigen-presenting cells and antigen-specific T cells (11–13).
Tumor immunotherapy is an innovative therapy that modulates the immune microenvironment and activates the immune system. It depends on autoimmune functions to kill cancer cells and tumor tissues (14, 15). This approach has the advantage of producing long-term immunological memory effects while causing no harm to normal tissues or cells (16). In recent years, with the discovery of tumor immune checkpoint molecules, such as cytotoxic T lymphocyte (CTL)-associated protein-4 (CTLA-4), programmed cell death protein-1 (PD-1), and its ligand PD-L1, important breakthroughs have been made in the study of antitumor immune mechanisms, and immunotherapy has become a promising tumor treatment (17, 18). Currently, the main strategies in cancer immunotherapy include tumor vaccines, adoptive cellular immunotherapy (ACI), and immune checkpoint blockade (ICB) therapy (19–21). However, immune drugs alone are not effective for all patients. Drug resistance or adverse effects, including skin rashes, itching, diarrhea, pneumonia, and thyroid malfunction, may occur in some people (22). In addition, immune side effects, including the cascade of inflammatory mediators, hematopoietic system dysfunction, and organ toxicity, also limit the optimization of immunotherapy methods (23–25). Therefore, it is needed to develop appropriate combined cancer therapies to enhance the effectiveness of immunotherapy and to reduce side effects. It has been revealed that combining PDT with antitumor immunotherapy not only can improve the PDT-induced antitumor immune response but also can promote the proliferation and activation of immune memory cells, inhibit tumor metastasis, and prevent tumor recurrence (26, 27).
In this review, we explain the principles and damage mechanisms of PDT and discuss the immune response induced by PDT. We also summarize the combined treatment strategies of PDT and some immunotherapies, such as immune adjuvants, inhibitors of immune suppression, and ICB for cancer. We believe that this combination therapy strategy will be further developed and functionalized to meet the application in biomedicine, thereby making remarkable contributions to human health.
The exact mechanism of PDT has not yet been elucidated. However, the widely accepted theory is based on photophysical principles and guided by the Jablonski Diagram. The Jablonski Diagram clarifies the different electronic states of molecules and the process of their transitions, which are considered as the basic principles for designing phototherapeutic reagents (28, 29). As shown in Figure 1, PS is excited by light irradiation, and the electronic state changes from the ground state (S0) to the singlet excited state (Sn) and then relaxes to the lowest energy level of the singlet excited state (S1) through internal conversion (IC). The PS at the S1 state can consume energy in three ways, resulting in diverse outcomes (30, 31). 1). The molecules at the S1 state emit a photon with a longer wavelength to S0. The photon emission process can be applied to fluorescence imaging. 2) S1 state molecules release heat by colliding with each other and relax to the S0 state in a non-radiative manner, which is often used in photothermal therapy. 3) The PS at the S1 state transitions to the lowest energy level of the triplet state (T1) through intersystem crossing (ISC) (32–34). The molecules at the T1 state can relax to the S0 state by emitting photons. This phenomenon is called phosphorescence. In addition, molecules in the T1 state can also perform two different types of PDT by generating free radicals or singlet oxygen (35–37). In type I reaction, T1 state chemicals form free radicals by directly interacting with endogenous substrates, such as cell membranes or biological macromolecules, and subsequently react with oxygen to produce ROS (38). In the type II reaction, T1 state molecules directly transfer energy to oxygen molecules in the surrounding environment to generate singlet oxygen, which may oxidize the macromolecular cellular components, resulting in cellular death through either apoptosis or necrosis (39, 40). However, it should be noted that most PSs exert their antitumor effects by causing cell damage through the generation of singlet oxygen from the type II reaction (41). Singlet oxygen can act on protein sulfhydryl and amino groups to denature proteins and reduce enzymatic activity in cells (42, 43). Singlet oxygen can also alter the structure and function of cell membranes, mitochondrial membranes, and DNA molecules (44, 45).
Figure 1 The photophysical mechanism and the two classic types of photodynamic therapy (PDT). The photosensitizer absorbs light energy to jump from the ground state to the excited state, which then leads to a long-lived excited triplet state through intersystem crossing and finally generate reactive oxygen species through type I reaction or type II reaction.
The tumor damage mechanism caused by PDT mainly includes the following types. 1) Direct killing effect of ROS on tumor cells, including apoptosis, necrosis, and autophagy. 2) PSs target the vascular system to form thrombi, causing hypoxic infarction of tumor tissues. 3) Tumor cells that undergo apoptosis or necrosis release inflammatory factors, which trigger an inflammatory response that leads to an antitumor immune response (46–48) (Figure 2). It is worth mentioning that apoptosis, autophagy, and cell cycle arrest after PDT may occur simultaneously during a single treatment session. Sasnauskiene et al. (49) found that the degree of oxidative stress damage to cells is dose-dependent. Cells showed increased autophagy and cell cycle arrest but no apoptosis when the cytotoxic dose was increased to 50%. However, the cells displayed significant apoptosis, autophagy, and cell cycle arrest when the cytotoxic dose was greater than 70%. The damage to blood vessels by PDT is based on the characteristics of tumor tissue with wide vascular gaps and poor integrity, which are conducive to PS aggregation (50). After photoactivation, PS enrichment in tumor vascular endothelial cells causes many physiological responses, including platelet aggregation and vasoconstriction, which lead to tumor vascular blockage, ischemia, and hypoxia (51, 52). The direct ablation effect of PDT on tumor cells also releases inflammatory mediators, thereby recruiting a variety of white blood cells, such as neutrophils, macrophages, and dendritic cells (DCs) (53). These white blood cells will then activate the immune cascade to further suppress the tumor (54, 55).
Figure 2 The tumor damage mechanism caused by photodynamic therapy (PDT). PDT generates reactive oxygen species (ROS) to induce cell apoptosis and necrosis; photosensitizers target the vascular system to form thrombi, causing hypoxic infarction of tumor tissues; apoptotic and necrotic tumor cells recruit a variety of white blood cells.
The current elaboration on the mechanism of PDT antitumor immune response tends to focus on PDT-induced oxidative stress in tumor cells, causing ICD and release of TAAs and damage-associated molecular patterns (DAMPs) (56, 57). ICD is a specific cell death mode that translocates calreticulin (CRT) to the cell surface and releases high mobility group box 1 protein (HMGB1), adenosine triphosphate (ATP), and heat shock proteins (HSPs) to the extracellular surface (58, 59). Inflammation-related signaling pathways, the release of immune-related cytokines, neutrophil infiltration, and the complement cascade are all triggered by these DAMPs (60, 61). DCs are specialized antigen-presenting cells that link the innate immune response to the adaptive immune response, taking up TAAs, binding DAMPs through pattern recognition receptors (PRRs), and processing antigens as they migrate to lymph nodes and mature. The antigens are presented to T cells for proliferation and differentiation into CTLs, which exert antitumor immune effects (62–64).
After the determination of the important role of immunogenic DAMPs in the PDT-mediated antitumor immune response, many studies have been conducted to improve the ICD triggered by PDT. Deng et al. (65) designed reduction-sensitive Ds-sP nanocarriers loaded with an efficient endoplasmic reticulum (ER)-targeting PS TCPP-TER. The unique ER targeting ability of PS TCPP-TER results in an elevated level of oxidative stress in the ER of tumor cells, which in turn releases more DAMPs and enhances the immune effect. This strategy can effectively address the problems of short ROS half-life and limited intracellular diffusion depth. However, a hypoxic tumor microenvironment (TME) can limit the efficacy of PDT and reduce the efficiency of ICD induction (66). Therefore, increasing the oxygen content of tumor tissues is essential to improve the efficiency of PDT treatment. Liang and colleagues (67) developed gold nanocages (AuNCs) with hollow structures and coated them with a layer of manganese dioxide to synthesize core-shell nanoparticles (AuNC@MnO2). In the acidic microenvironment of tumor tissue rich in H2O2, manganese dioxide reacts as follows: MnO2 + H2O2 + 2H+→Mn2+ + 2H2O + O2↑ generates a large amount of oxygen to promote the accumulation of ROS in the tumor and enhances the efficacy of PDT by improving tumor hypoxia to achieve ICD. The released oxygen and Mn2+ can provide fluorescence (FL)/photoacoustic (PA)/magnetic resonance multimodal imaging function to evaluate the integration of tumor diagnosis and treatment. In short, the induction of ICD by enhanced PDT to promote antitumor immune response is a promising tumor treatment strategy (Figure 3).
Figure 3 Photodynamic therapy (PDT)-induced immune response. PDT induces immunogenic cell death and promotes the release of calreticulin (CRT) and high mobility group box 1 protein (HMGB1) from tumor cells; tumor cell lysates and antigens are used as cancer vaccines to cause a series of immune cascades.
Tumor cell lysates and TAAs produced by PDT can also induce specific immune responses and are more effective than tumor cell lysates produced by ionizing radiation and ultraviolet rays (68). Similar to the inoculation mechanism of conventional vaccines that directly introduce microorganisms into the body to produce protective antibodies, cancer vaccines stimulate the activation of the body’s immune system through tumor cell death (69, 70). DCs have played a major role in the development of cancer vaccine therapy as critical mediators of antigen presentation, reversing a major component of tumor-mediated immune suppression (71, 72). Tumor residues after PDT can be used as a cancer vaccine to dramatically increase DC activation and release inflammatory cytokines to boost immune response in a mouse breast cancer model, according to a study using chlorin e6 (Ce6) as a PS (73). A promising tumor treatment strategy is to use PDT-treated tumor cells as a DC vaccine to develop a PDT-DC vaccination that can more efficiently destroy tumors and trigger a powerful antitumor immune response (74, 75).
lAntigens produced by PDT ablation of tumors may have insufficient immunogenicity as a DC vaccine and are limited to immunosuppressed “cold” tumors (76). Korbelik made a vaccine against SCCVII cells using PDT to destroy the cells and used this vaccine in the SCCVII tumor model to show that it inhibits tumors growth (77). However, the levels of splenic myeloid-derived suppressor cells (MDSCs) were significantly enhanced. Therefore, the immune adjuvant N-dihydrogalactochitosan (GC) was added to the PDT vaccine group to reduce the number of MDSCs (precursors of DCs, macrophages, and granulocytes) and alleviate immunosuppression (78). Ni et al. (79) used the amphipathic 4T1 breast cancer cell membrane to load PS Ce6 and the chemotherapeutic drug, i.e., doxorubicin hydrochloride (Dox), and coated the cell membrane surface with calcium carbonate to construct nanodrug delivery systems. Ce6-based PDT and Dox cause DNA damage, induce tumor ICD, and release TAA. The ROS generated during this process is expected to form in situ PDT-DC vaccination by mimicking inflammatory mechanisms to recruit DCs. In PDT-DC vaccinated mice, the growth of both primary 4T1 and untreated distant tumors was suppressed, indicating the establishment of an efficient immune response. Moreover, serum levels of inflammatory cytokines in mice increased continuously, peaking and then stabilizing the day after vaccination. It provides a novel antitumor combination therapy for improving the immunogenicity of the PDT-DC vaccine by introducing adjuvants or chemotherapeutic drugs. This therapy enhances body-specific immune responses, eliminates tumors, and builds long-term immunological memory.
Immune adjuvants are chemicals that boost the cellular or humoral immune response to an antigen (80, 81). Vaccines, which are one of the most successful medicinal discoveries against a variety of infectious diseases, occasionally require a molecule in conjugation to boost the immune response (82). It is therefore expedient to co-administer these with an adjuvant to ensure a high-quality/high-quantity, memory-enhanced antibody response. In chronological order of appearance, the first immune adjuvant to be used clinically was Alum, followed by the development of oil-in-water emulsions and toll-like receptor (TLR) agonists (83, 84). TLR agonists are currently being used as immune adjuvants to activate TLR signaling pathways and boost immunological responses and are found to be promising agents for cancer treatment (85, 86). This review focuses on summarizing the strategies of TLR agonists in combination with PDT.
TLRs are one of the PRRs that are expressed by a wide range of immune cells and have received more attention. To date, 13 different TLRs (TLR1–13) have been identified in mammals (87). The TLR7 agonist imiquimod (R837) is a synthetic imidazoquinoline-like molecule, approved by the US Food and Drug Administration (FDA) as a single drug and commonly used in the treatment of various skin diseases, including basal cell carcinomas (88). R837 interacts with TLR7 on the DC surface and endosomes that results in the stimulation of DC maturation and release of pro-inflammatory cytokines through elevated expression of co-stimulatory molecules (89). PDT using the PS 5-aminolevulinic acid (ALA) in combination with imiquimod cream has been proven to be useful in the treatment of squamous cell carcinoma of the skin (90). This combination therapy ameliorates the poor oncogenic effect caused by insufficient local penetration of the PS into the tumor. Because of the limitations of topical use of imiquimod cream, this agent can only be used for superficial skin cancer treatment. If tumors in internal organs of the body are to be destroyed, R837 must be delivered locally to the tumor through blood circulation. R837, being a small-molecule, is diffused after local injection, and few of them eventually reach the tumor site (91). Furthermore, R837 causes direct cell death by inducing autophagy and has concentration-dependent cytotoxicity (92–94). The use of nanomaterials to encapsulate immune adjuvants and decrease their harmful effects is a viable technique for addressing such issues. Xu et al. (95) used the hydrophobic region between UCNP and PEG to load the PS chlorin e6 (Ce6) and the TLR7 agonist R837. The results showed that TAA released from PDT-killed tumor cells and R837 induced DC maturation and released cytokines related to innate and adaptive immunity, such as TNF-α and IL-12, by upregulating the expression of co-stimulatory molecules such as CD80 and CD86. Resiquimod (R848), a second-generation derivative of R837, shares a similar structure and properties with R837. However, in contrast to R837, R848 can be used as an agonist of both TLR7 and TLR8 (96, 97). Many studies on R848 immunotherapy, both alone and in combination with chemotherapy and photothermal therapy, have been reported, suggesting that R848 can enhance immunity and improve anticancer therapeutic effectiveness (98–100). However, studies on the combined application of R848 with PDT have not been reported, which may be a promising direction for future research.
Other TLR agonists have been used in PDT immunotherapy to enhance the immune response. According to the reported study, the combination of PDT and TLR5 agonist flagellin (FlaB-Vax) effectively inhibited bilateral melanoma in mice, enhanced TME tumor antigen cross-presentation, and promoted tumor CD8+ T-cell infiltration and systemic IFN-γ secretion (101). CpG oligodeoxynucleotides (CpG ODN) are synthetic DNA fragments that function as TLR9 agonists by interacting with DC-expressed TLR9 and enhancing antigen-specific immune responses (102). Ni and co-workers (103) used the cationic PS 5,10,15,20-tetra(p-benzoato)porphyrin (TBP) to adsorb the anion CpG to achieve efficient PDT and effective delivery of CpG. In a mouse breast cancer model, the combination of PDT and CpG was found to provide excellent tumor suppression, with about 97% of tumors being eliminated. Cai et al. (104) designed a metal–organic framework (MOF) nanoparticle formed by the self-assembly of the PS H2TCPP and zirconium ions. The porous internal structure of MOF was used to load the TLR9 agonist CpG ODN. When compared with the control and the treatment group alone, the combination of CpG ODN and PDT dramatically increased the expression of MHC-II, CD317, and co-stimulatory molecules including CD80/CD86. Remarkably, CpG ODN also reduced the immunosuppressive activity of MDSCs and improved the tumor immunosuppressive microenvironment (105). However, CpG ODN is still mostly used as an immunostimulant in PDT immunotherapy, and there is a significant research gap in improving immunosuppression.
Many immune adjuvants such as glycated chitosan (GC), lactobacillus BCG, mycobacterial cell wall extract (MCWE), complete Freund (CF) adjuvant, and incomplete Freund (IF) adjuvant can stimulate the immune response similar to that of TLR agonists (106). Previously, it was demonstrated that GC, a water-soluble compound synthesized from galactose and chitosan, was shown to stimulate TNF-α secretion by macrophages and induce tumor-specific immune responses (107). Cai’s group (108) found the highest levels of apoptotic and inflammatory responses and the highest infiltration of immune cells in tumors in the synergistic treatment group of PDT and GC. Additionally, mice treated with a combination of PDT and GC had a significantly better survival rate in the EMT6 mammary tumor and 4T1 metastatic mammary tumor models. In short, PDT adjuvant by immune adjuvant has promising research potential because of its ability to inhibit tumor metastasis and recurrence.
PDT can activate the immune system to some extent, but the intensity of the PDT-induced immune response may not be sufficient to destroy the tumors or prevent their metastasis and recurrence due to the immunosuppressive effect of the TME and immune escape of tumor cells (109, 110) (Figure 4). Moreover, PDT causes local inflammation and immunosuppression due to contact hypersensitivity (CHS) (111). Therefore, it is necessary to explore suitable immune inhibitors to inhibit tumor immunosuppressive signals and enhance PDT-induced immune responses.
Figure 4 Tumor immunosuppressive environment after photodynamic therapy (PDT). Tumor tissues secrete vascular endothelial growth factor (VEGF) to promote the proliferation of myeloid-derived suppressor cells (MDSCs); the high expression of indoleamine 2,3-dioxygenase (IDO) in tumor cells promotes the recruitment of Treg cells and inhibits the activation of CD8+ T cells through tryptophan metabolism.
Immunosuppressive cells such as MDSCs and regulatory T cells (Tregs) in the TME suppress the antitumor immune responses and promote tumor progression and invasion (112, 113). Specifically, MDSCs suppress T-cell function through multiple mechanisms, including production of nitric oxide and immunosuppressive metabolites, secretion of immunosuppressive cytokines such as TGF-β and IL-10, and upregulation of cyclo-oxygenase 2 (Cox2) and prostaglandin E2 (PGE2) (114, 115), while Tregs suppress T-cell function through CTL antigen 4 to inhibit the expression of DC co-stimulatory molecules including CD80 and CD86 (116). Recently, it has been found that PDT vaccination significantly increased MDSC and Treg levels, while low doses of GC and cyclophosphamide were found to reduce the elevated levels of immunosuppressive cells (77, 117). The findings demonstrated the feasibility of using small-molecule inhibitors to weaken immunosuppressive cells and alter the immunosuppressive TME, thereby enhancing the PDT-DC vaccine-induced immune responses.
Apart from their role in tumor development and immune escape, the reprogramming of tumor cells’ metabolism influences immune cell metabolism (118). Tryptophan (Trp) metabolism in T cells is mediated by tumor cells through an elevated level of indoleamine 2,3-dioxygenase (IDO) expression, which converts Trp to kynurenine (Kyn) (119). Lack of Trp inhibits CTL activation, while abnormal accumulation of Kyn recruits Treg to suppress effector T-cell function (120, 121). Reducing the level of immunosuppression by PDT nanoparticles loaded with IDO inhibitors is a more appropriate strategy. Zhao et al. (122) constructed self-delivery photo-immune stimulators (iPSs) through non-covalent interactions between the PS Ce6 and IDO inhibitor, i.e., NLG919. They first demonstrated that iPSs can promote DC maturation by inducing apoptosis and ICD in CT26 cells via PDT and cellular release of CRT and HMGB1. Following PDT treatment with iPSs, increased CD4+/CD8+ T-cell infiltration was observed in the mouse CT26 tumor tissues, while a significant decrease in Kyn/Trp ratio was observed in the serum. Additionally, transcriptomic analysis of mouse tumor tissues demonstrated that iPS-based PDT could effectively stimulate the tumor immune microenvironment and enhance tumor immunotherapy efficacy. A variety of PSs and IDO inhibitors, such as self-assembled nanoparticles, are being developed extensively. Yang’s group (123) designed pH-responsive nanovesicles (pRNVs) as carriers to synthesize PRNVS/HPPH/IND smart nanoparticles by encapsulating the PS HPPH and the IDO inhibitor indoximod (IND) through hydrophobic interactions. They found that PDT treatment of the nanoparticles inhibits mouse melanoma growth, and the release of IND stimulates CD8+ T cells to destroy distant tumors by increasing P-S6K phosphorylation.
Tumor tissues secrete large amounts of vascular endothelial growth factor (VEGF) to promote the proliferation of immunosuppressive cells and inhibit DC maturation by NF-κB pathway activation (124, 125). Excess VEGF leads to abnormalities in tumor vascular structure and function, exacerbating the hypoxic state of the TME and affecting the efficacy of PDT therapy (126). Zhou et al. (127) developed a self-assembled nanoplatform containing the PS Ce6, the VEGF receptor (VEGFR) inhibitor axitinib (AXT), and the IDO inhibitor dextro-1-methyl tryptophan (1MT) to alleviate immunosuppression by promoting vascular normalization and improving the tumor hypoxic microenvironment, thereby enhancing PDT immunotherapy. According to the obtained results, the enhanced PDT immunotherapy has significant effects on both primary melanoma and lung metastases in mice.
The PS-based PDT induces tumor ICD and stimulates immune activation, while the inhibitor of immune suppression promotes PDT-induced immune response by weakening tumor immune escape (128). It is about the immunosuppressive TME of MDSCs and Tregs and immunosuppressive molecules like IDO-1 and VEGF. PDT should be combined with compounds that can inhibit MDSCs and/or Tregs and IDO or VEGF.
Various immunosuppressive mechanisms can impair the efficiency of antitumor immunotherapy during tumor progression. For example, immune checkpoint molecules are considered to be the primary anticancer immunotherapy targets, as they have a negative immunomodulatory effect (129). The development of target-specific antibodies to block the underlined immune checkpoints is a hot topic in immunotherapy. However, due to the low tumor immunogenicity, the response rate of some patients to ICB therapy is unsatisfactory (130). The efficiency of ICB therapy can be improved by enhancing tumor immunogenicity and sensitivity through PDT-mediated ICD induction (131, 132). Herein, three immune checkpoints, including CTLA-4, PD-1/PD-L1, and CD47, have been described, followed by summarizing their combined treatment strategies along with PDT.
CTLA-4 is an immune checkpoint receptor expressed on Tregs and other activated T cells (133). It binds CD80 and CD86 ligands on DCs, weakening T-cell activation and inability to perform normal immune functions (134). It is considered the first immune checkpoint receptor to be used clinically for cancer immunotherapy. Additionally, ipilimumab, an anti-CTLA-4 monoclonal antibody used to treat metastatic melanoma, has been approved by the FDA (135). The combination of anti-CTLA-4 antibody and PDT is essential for the eradication of systemic tumors and may be an effective therapeutic strategy for advanced cancers (136). Wang et al. (137) developed bullet-shaped magnetic mesoporous organosilica nanoparticles (M-MONs) with Fe3O4 at the head and a mesoporous silica framework at the tail. Next, the redox/pH dual-responsive M-MONs@Ce6 nanoparticles were developed with M-MONs (pores size of ~3.8 nm) and loaded with the PS Ce6. M-MONs@Ce6 induced more severe ICD by simultaneous PDT and magnetothermal treatment under the combined action of laser and alternating-current magnetic field (ACMF), releasing DAMPs to trigger specific immune responses and significantly inhibiting the growth of mouse and human breast cancers. In a mouse model of breast cancer with lung metastasis, the PDT+anti-CTLA-4 antibody treatment group and the magnetothermal treatment+anti-CTLA-4 antibody treatment group showed an inhibitory effect on lung metastatic tumor. This inhibitory effect was further enhanced when the two treatments were combined, accompanied by an increase in CTL and a decrease in Treg. Furthermore, the nanoparticles used for PDT treatment had no severe side effects when combined with ICB treatment, indicating that this is a safe and effective strategy for the treatment of metastatic cancer.
Following the success of CTLA-4 checkpoint blockade in antitumor immunotherapy, more consideration has been paid to the exploration of new immune checkpoints. PD-1, also known as CD279, is an immunosuppressive signaling molecule highly expressed on tumor-specific T cells. PD-1 binds to PD-L1 (PD-1 ligand) present on the tumor cells. Consequently, the inhibition of T-cell proliferation and activation, elevated levels of T-cell apoptosis, and reduced cytokine secretion were observed (138, 139). PD-1/PD-L1 signaling promotes tumor immune escape and severely affects the efficacy of cancer immunotherapy (140). For this reason, several anti-PD-1/PD-L1 antibodies have been developed for restoring T-cell viability and promoting antitumor immune response (141). It has been reported that PDT significantly increases tumor PD-L1 levels, while the majority of recruited CD8+ T cells express PD-1, emphasizing the importance of a combined anti-PD-1/PD-L1 antibody therapeutic strategy (142, 143). Liu et al. (144) used PS-g-PEG micelles to encapsulate the PS, i.e., BDP-I-N to improve its water solubility, followed by modifying the micelles with functional groups to attach anti-PD-L1 antibodies that result in the synthesis of BDP-I-N-anti-PD-L1 multifunctional nanoparticles. Unlike the conventional therapeutic strategy to achieve the combination with PDT by intravenous injection of anti-PD-1/PD-L1 antibodies, this work assembled anti-PD-L1 antibodies and PSs into nanoparticles and accomplished the efficient accumulation in tumor tissues through the active targeting of immune checkpoint antibodies and the EPR effect of nanoparticles. In vivo results demonstrate that BDP-I-N-anti-PD-L1 nanoparticles eliminate MC38 mouse colon tumors by synergistic action of PDT and ICB, generate immune memory to prevent tumor recurrence, and have an excellent biosafety profile.
Anti-PD-1/PD-L1 antibodies have demonstrated excellent efficacy in tumor immunotherapy; however, the high cost of these antibodies adds to the financial burden of cancer patients (145). To address the high cost of anti-PD-L1 antibodies, Zhang and colleagues (146) extracted PD-1-expressing HEK293T cell membranes to replace anti-PD-L1 antibodies to bind PD-L1 on 4T1 cells. They developed PDT-mediated PFTBA@HSA-DVMS (PHD) nanoemulsions against hypoxic tumors by wrapping the oxygen supply agent perfluorotributylamine (PFTBA) in human serum albumin (HSA), followed by loading it with the PS sinoporphyrin sodium (DVDMS). In addition, they developed PHD@PM nanoplatform by encapsulating PHD nanoemulsion inside PD-1-expressing cell membranes to realize the combination of PDT and ICB. Their work demonstrates that PHD@PM nanoplatform is an innovative therapeutic platform. This platform has high clinical application because of its low cost, high biocompatibility, and active targeting. Furthermore, it has the ability to improve TME, which is hypoxic and immunosuppressive.
Aside from the two most widely studied immune checkpoints mentioned above, one of the current hot spots in cancer ICB therapy is targeting the CD47–SIRP signaling axis. CD47 is a membrane protein expressed by almost all cells, while signal-regulating protein α (SIRPα) is only expressed by myeloid cells such as macrophages and monocytes (147, 148). CD47 on the surface of tumor cells binds to SIRPα on macrophages releasing a “don’t eat me” signal that inhibits macrophage phagocytosis and thus promotes tumor immune escape (149). A reported study has revealed that thrombospondin-1 (TSP-1) in the TSP-1/CD47/SIRP-α signal axis could significantly improve treatment outcomes by blocking it, laying the groundwork for the clinical use of PDT cancer vaccines (150). Chang et al. (151) designed Cu2O@CaCO3 nanoparticles for the target-specific treatment of colorectal cancer (CRC). In the acidic microenvironment of CRC, the CaCO3 shell decomposed to release the PS precursor Cu2O. Exposed Cu2O reacted with endogenous H2S in the CRC, producing Cu31S16 and ROS to achieve PDT under 1,064-nm laser radiation. It was found that the oxidative stress induced by Cu2O@CaCO3 nanoparticles could also promote macrophages from immunosuppressed M2 phenotype to immune-activated M1 phenotype. Combined treatment with anti-CD47 antibody resulted in improved phagocytosis of macrophages, promoted antigen presentation, and induced antitumor immune response by T cells, achieving effective inhibition of CRC metastasis and recurrence. Furthermore, recent studies have also indicated that the combination of PDT and CD47 monoclonal antibodies may have the potential for the treatment of human bladder cancer (152).
The combination of PDT and ICB can effectively inhibit tumor metastasis and recurrence compared with the individual therapeutic effect of PDT. Moreover, the combination strategy also improves the failure of ICB treatment due to insufficient immunogenicity of tumor cells. Current studies have identified several new immune checkpoints, such as V-domain Ig suppressor of T-cell activation (VISTA), T-cell immunoglobulin and ITIM domain (TIGIT), and T-cell immunoglobulin and mucin-domain containing-3 (TIM-3) (153, 154). Taken together, the combination of immune checkpoints and PDT may be a promising direction for future research.
Figure 5 shows the combined treatment strategies of different types of PDT immunotherapy. These strategies exert significant effects in cancer treatment mainly by enhancing the anticancer immune response or by reducing the suppression of the immune system. The specific details of the combination of PDT and different types of immunotherapies are summarized in Table 1.
Figure 5 Schematic overview of synergized photodynamic immunotherapy. Photodynamic therapy enhances the antitumor immune response, thus killing primary and distant tumors in combination with different immunotherapeutic strategies (immune adjuvants, immune inhibitors and immune checkpoint blockade).
In this review, we have explained the classification and photophysical mechanism of PDT based on the Jablonski Diagram. By inducing ICD, PDT has been shown to successfully activate the immune response. However, due to insufficient immunogenicity or immunosuppression, the immune reaction induced by a single PDT is greatly restricted. Therefore, PDT with other immunotherapies has been integrated to solve such problems. However, optimizing the in vivo safety assessment is still a challenge and needs further research to enhance the efficiency of PDT immunotherapy for effective tumor treatment.
Immunotherapy has been extensively studied in clinical trials, but clinical studies on PDT and its effects on the human immune system are very rare. Although a large number of PSs have been developed and used for PDT in animal studies, Photofrin and aminolevulinic acid (ALA) are the few two used in clinical research. And only a few of these studies have investigated on the effect of PDT on the human immune system (155, 156). Determining the relationship between PDT and immune response in clinical research and combining it with immunotherapy will be a major focus for future research. We hope that PDT immunotherapy can be proven to be an excellent cancer treatment in clinical trials.
YH contributed to the conception and design of the review. JHu and PW wrote the manuscript. LG, ZZ, JHe, LZ, and YZ revised the manuscript. All authors contributed to the article and approved the submitted version.
This work was supported by the National Natural Science Foundation of China (No. 82072340), the Major national science and technology projects-Major new drug creation (2019ZX09301-132), Changjiang Scholars and Innovative Research Team in University (No. IRT_15R13), and Guangxi Science and Technology Base and Talent Special Project (No. AD17129003).
The authors declare that the research was conducted in the absence of any commercial or financial relationships that could be construed as a potential conflict of interest.
All claims expressed in this article are solely those of the authors and do not necessarily represent those of their affiliated organizations, or those of the publisher, the editors and the reviewers. Any product that may be evaluated in this article, or claim that may be made by its manufacturer, is not guaranteed or endorsed by the publisher.
1. Wang P, Sun S, Ma H, Sun S, Zhao D, Wang S, et al. Treating Tumors With Minimally Invasive Therapy: A Review. Materials Sci Eng C Materials Biol Appl (2020) 108:110198. doi: 10.1016/j.msec.2019.110198
2. Xu X, Lu H, Lee R. Near Infrared Light Triggered Photo/Immuno-Therapy Toward Cancers. Front Bioeng Biotechnol (2020) 8:488. doi: 10.3389/fbioe.2020.00488
3. Von Tappeiner H. Therapeutische Versuche Mit Fluoreszierenden Stoffen. Munch Med Wochenschr (1903) 1:2042–4.
4. Nguyen VN, Yan Y, Zhao J, Yoon J. Heavy-Atom-Free Photosensitizers: From Molecular Design to Applications in the Photodynamic Therapy of Cancer. Acc Chem Res (2021) 54(1):207–20. doi: 10.1021/acs.accounts.0c00606
5. Wang ZX, Peng HM, Shi W, Gan L, Zhong LP, He J, et al. Application of Photodynamic Therapy in Cancer: Challenges and Advancements. Biocell (2021) 45(3):489–500. doi: 10.32604/biocell.2021.014439
6. Liu H, Yao J, Guo H, Cai X, Jiang Y, Lin M, et al. Tumor Microenvironment-Responsive Nanomaterials as Targeted Delivery Carriers for Photodynamic Anticancer Therapy. Front Chem (2020) 8:758. doi: 10.3389/fchem.2020.00758
7. Hou X, Tao Y, Pang Y, Li X, Jiang G, Liu Y. Nanoparticle-Based Photothermal and Photodynamic Immunotherapy for Tumor Treatment. Int J Cancer (2018) 143(12):3050–60. doi: 10.1002/ijc.31717
8. Dolatkhah M, Hashemzadeh N, Barar J, Adibkia K, Aghanejad A, Barzegar-Jalali M, et al. Graphene-Based Multifunctional Nanosystems for Simultaneous Detection and Treatment of Breast Cancer. Colloids Surf B Biointerfaces (2020) 193:111104. doi: 10.1016/j.colsurfb.2020.111104
9. Kawczyk-Krupka A, Bugaj AM, Latos W, Zaremba K, Wawrzyniec K, Sieron A. Photodynamic Therapy in Colorectal Cancer Treatment: The State of the Art in Clinical Trials. Photodiagnosis Photodyn Ther (2015) 12(3):545–53. doi: 10.1016/j.pdpdt.2015.04.004
10. Champeau M, Vignoud S, Mortier L, Mordon S. Photodynamic Therapy for Skin Cancer: How to Enhance Drug Penetration? J Photochem Photobiol B (2019) 197:111544. doi: 10.1016/j.jphotobiol.2019.111544
11. Panzarini E, Inguscio V, Dini L. Immunogenic Cell Death: Can It be Exploited in PhotoDynamic Therapy for Cancer? BioMed Res Int (2013) 2013:482160. doi: 10.1155/2013/482160
12. Showalter A, Limaye A, Oyer JL, Igarashi R, Kittipatarin C, Copik AJ, et al. Cytokines in Immunogenic Cell Death: Applications for Cancer Immunotherapy. Cytokine (2017) 97:123–32. doi: 10.1016/j.cyto.2017.05.024
13. Turubanova VD, Balalaeva IV, Mishchenko TA, Catanzaro E, Alzeibak R, Peskova NN, et al. Immunogenic Cell Death Induced by a New Photodynamic Therapy Based on Photosens and Photodithazine. J Immunother Cancer (2019) 7(1):350. doi: 10.1186/s40425-019-0826-3
14. Farhood B, Najafi M, Mortezaee K. CD8(+) Cytotoxic T Lymphocytes in Cancer Immunotherapy: A Review. J Cell Physiol (2019) 234(6):8509–21. doi: 10.1002/jcp.27782
15. Tan S, Li D, Zhu X. Cancer Immunotherapy: Pros, Cons and Beyond. Biomed pharmacotherapy (2020) 124:109821. doi: 10.1016/j.biopha.2020.109821
16. Chen Q, Xu L, Liang C, Wang C, Peng R, Liu Z. Photothermal Therapy With Immune-Adjuvant Nanoparticles Together With Checkpoint Blockade for Effective Cancer Immunotherapy. Nat Commun (2016) 7:13193. doi: 10.1038/ncomms13193
17. Topalian SL, Drake CG, Pardoll DM. Immune Checkpoint Blockade: A Common Denominator Approach to Cancer Therapy. Cancer Cell (2015) 27(4):450–61. doi: 10.1016/j.ccell.2015.03.001
18. Li B, Chan HL, Chen P. Immune Checkpoint Inhibitors: Basics and Challenges. Curr Med Chem (2019) 26(17):3009–25. doi: 10.2174/0929867324666170804143706
19. Yang Y. Cancer Immunotherapy: Harnessing the Immune System to Battle Cancer. J Clin Invest (2015) 125(9):3335–7. doi: 10.1172/JCI83871
20. Choi Y, Yoon H, Kim J, Yang S, Lee J, Choi J, et al. Doxorubicin-Loaded PLGA Nanoparticles for Cancer Therapy: Molecular Weight Effect of PLGA in Doxorubicin Release for Controlling Immunogenic Cell Death. Pharmaceutics (2020) 12(12):1165. doi: 10.3390/pharmaceutics12121165
21. Choi Y, Lee S, Kim K, Kim S, Chung Y, Lee C. Studying Cancer Immunotherapy Using Patient-Derived Xenografts (PDXs) in Humanized Mice. Exp Mol Med (2018) 50(8):1–9. doi: 10.1038/s12276-018-0115-0
22. Costa R, Talamantes S, Helenoswki I, Carneiro B, Chae Y, Gradishar W, et al. Analyses of Selected Safety Endpoints in Phase 1 and Late-Phase Clinical Trials of Anti-PD-1 and PD-L1 Inhibitors: Prediction of Immune-Related Toxicities. Oncotarget (2017) 8(40):67782–9. doi: 10.18632/oncotarget.18847
23. Sade-Feldman M, Jiao YJ, Chen JH, Rooney MS, Barzily-Rokni M, Eliane JP, et al. Resistance to Checkpoint Blockade Therapy Through Inactivation of Antigen Presentation. Nat Commun (2017) 8(1):1136. doi: 10.1038/s41467-017-01062-w
24. Johnson DB, Balko JM, Compton ML, Chalkias S, Gorham J, Xu Y, et al. Fulminant Myocarditis With Combination Immune Checkpoint Blockade. N Engl J Med (2016) 375(18):1749–55. doi: 10.1056/NEJMoa1609214
25. Pollack MH, Betof A, Dearden H, Rapazzo K, Valentine I, Brohl AS, et al. Safety of Resuming Anti-PD-1 in Patients With Immune-Related Adverse Events (irAEs) During Combined Anti-CTLA-4 and Anti-PD1 in Metastatic Melanoma. Ann Oncol (2018) 29(1):250–5. doi: 10.1093/annonc/mdx642
26. Kleinovink J, van Driel P, Snoeks T, Prokopi N, Fransen M, Cruz L, et al. Combination of Photodynamic Therapy and Specific Immunotherapy Efficiently Eradicates Established Tumors. Clin Cancer Res an Off J Am Assoc Cancer Res (2016) 22(6):1459–68. doi: 10.1158/1078-0432.Ccr-15-0515
27. Rajendrakumar SK, Uthaman S, Cho CS, Park IK. Nanoparticle-Based Phototriggered Cancer Immunotherapy and Its Domino Effect in the Tumor Microenvironment. Biomacromolecules (2018) 19(6):1869–87. doi: 10.1021/acs.biomac.8b00460
28. Dash BS, Das S, Chen JP. Photosensitizer-Functionalized Nanocomposites for Light-Activated Cancer Theranostics. Int J Mol Sci (2021) 22(13):6658. doi: 10.3390/ijms22136658
29. Juzenas P, Chen W, Sun Y, Coelho M, Generalov R, Generalova N, et al. Quantum Dots and Nanoparticles for Photodynamic and Radiation Therapies of Cancer. Advanced Drug delivery Rev (2008) 60(15):1600–14. doi: 10.1016/j.addr.2008.08.004
30. Huang X, Sun X, Wang W, Shen Q, Shen Q, Tang X, et al. Nanoscale Metal-Organic Frameworks for Tumor Phototherapy. J materials Chem B (2021) 9(18):3756–77. doi: 10.1039/d1tb00349f
31. Korupalli C, Kalluru P, Nuthalapati K, Kuthala N, Thangudu S, Vankayala R. Recent Advances of Polyaniline-Based Biomaterials for Phototherapeutic Treatments of Tumors and Bacterial Infections. Bioeng (Basel) (2020) 7(3):94. doi: 10.3390/bioengineering7030094
32. Jun J, Chenoweth D, Petersson E. Rational Design of Small Molecule Fluorescent Probes for Biological Applications. Organic biomolecular Chem (2020) 18(30):5747–63. doi: 10.1039/d0ob01131b
33. Chen R, Wu R, Zhang G, Gao Y, Xiao L, Jia S. Electron Transfer-Based Single Molecule Fluorescence as a Probe for Nano-Environment Dynamics. Sensors (Basel Switzerland) (2014) 14(2):2449–67. doi: 10.3390/s140202449
34. Feng G, Zhang GQ, Ding D. Design of Superior Phototheranostic Agents Guided by Jablonski Diagrams. Chem Soc Rev (2020) 49(22):8179–234. doi: 10.1039/d0cs00671h
35. Zhao Q, Zhou X, Cao T, Zhang K, Yang L, Liu S, et al. Fluorescent/phosphorescent Dual-Emissive Conjugated Polymer Dots for Hypoxia Bioimaging. Chem Sci (2015) 6(3):1825–31. doi: 10.1039/c4sc03062a
36. Zhao B, He Y. Recent Advances in the Prevention and Treatment of Skin Cancer Using Photodynamic Therapy. Expert Rev Anticancer Ther (2010) 10(11):1797–809. doi: 10.1586/era.10.154
37. Martins TD, Lima E, Boto RE, Ferreira D, Fernandes JR, Almeida P, et al. Red and Near-Infrared Absorbing DicyanomethyleneSquaraine Cyanine Dyes: PhotophysicochemicalProperties and Anti-Tumor Photosensitizing Effects. Materials (Basel) (2020) 13(9):2083. doi: 10.3390/ma13092083
38. Zhang J, Jiang C, Figueiro Longo JP, Azevedo RB, Zhang H, Muehlmann LA. An Updated Overview on the Development of New Photosensitizers for Anticancer Photodynamic Therapy. Acta Pharm Sin B (2018) 8(2):137–46. doi: 10.1016/j.apsb.2017.09.003
39. Abrahamse H, Hamblin MR. New Photosensitizers for Photodynamic Therapy. Biochem J (2016) 473(4):347–64. doi: 10.1042/BJ20150942
40. Castano A, Demidova T, Hamblin M. Mechanisms in Photodynamic Therapy: Part Two-Cellular Signaling, Cell Metabolism and Modes of Cell Death. Photodiagnosis Photodynamic Ther (2005) 2(1):1–23. doi: 10.1016/s1572-1000(05)00030-x
41. Fujita Y, Sasayama T, Tanaka K, Kyotani K, Nagashima H, Kohta M, et al. DWI for Monitoring the Acute Response of Malignant Gliomas to Photodynamic Therapy. AJNR Am J Neuroradiol (2019) 40(12):2045–51. doi: 10.3174/ajnr.A6300
42. Hsieh YJ, Chien KY, Yang IF, Lee IN, Wu CC, Huang TY, et al. Oxidation of Protein-Bound Methionine in Photofrin-Photodynamic Therapy-Treated Human Tumor Cells Explored by Methionine-Containing Peptide Enrichment and Quantitative Proteomics Approach. Sci Rep (2017) 7(1):1370. doi: 10.1038/s41598-017-01409-9
43. Qiu H, Tan M, Ohulchanskyy TY, Lovell JF, Chen G. Recent Progress in Upconversion Photodynamic Therapy. Nanomaterials (Basel) (2018) 8(5):344–62. doi: 10.3390/nano8050344
44. Di Mascio P, Martinez GR, Miyamoto S, Ronsein GE, Medeiros MHG, Cadet J. Singlet Molecular Oxygen Reactions With Nucleic Acids, Lipids, and Proteins. Chem Rev (2019) 119(3):2043–86. doi: 10.1021/acs.chemrev.8b00554
45. Liang J, Wu P, Tan C, Jiang Y. White Light-Induced Cell Apoptosis by a Conjugated Polyelectrolyte Through Singlet Oxygen Generation. RSC Adv (2018) 8(17):9218–22. doi: 10.1039/c8ra00774h
46. van Straten D, Mashayekhi V, de Bruijn HS, Oliveira S, Robinson DJ. Oncologic Photodynamic Therapy: Basic Principles, Current Clinical Status and Future Directions. Cancers (Basel) (2017) 9(2):19–73. doi: 10.3390/cancers9020019
47. Meng Z, Hou W, Zhou H, Zhou L, Chen H, Wu C. Therapeutic Considerations and Conjugated Polymer-Based Photosensitizers for Photodynamic Therapy. Macromol Rapid Commun (2018) 39(5):1700614. doi: 10.1002/marc.201700614
48. Donohoe C, Senge MO, Arnaut LG, Gomes-da-Silva LC. Cell Death in Photodynamic Therapy: From Oxidative Stress to Anti-Tumor Immunity. Biochim Biophys Acta Rev Cancer (2019) 1872(2):188308. doi: 10.1016/j.bbcan.2019.07.003
49. Sasnauskiene A, Kadziauskas J, Vezelyte N, Jonusiene V, Kirveliene V. Apoptosis, Autophagy and Cell Cycle Arrest Following Photodamage to Mitochondrial Interior. Apoptosis (2009) 14(3):276–86. doi: 10.1007/s10495-008-0292-8
50. Zhang H, Ye Z, Yuan Z, Luo Z, Jin H, Qian Q. New Strategies for the Treatment of Solid Tumors With CAR-T Cells. Int J Biol Sci (2016) 12(6):718–29. doi: 10.7150/ijbs.14405
51. Baluk P, Hashizume H, McDonald DM. Cellular Abnormalities of Blood Vessels as Targets in Cancer. Curr Opin Genet Dev (2005) 15(1):102–11. doi: 10.1016/j.gde.2004.12.005
52. Ji S, Zhang B, Wang X, Shi H, Yu L, Wang X. Effects of Statin Therapy on Mean Platelet Volume in Patients With Risk of Cardiovascular Diseases: A Systematic Review and Meta-Analysis. Biosci Rep (2019) 39(7):BSR20190180. doi: 10.1042/bsr20190180
53. Nath S, Obaid G, Hasan T. The Course of Immune Stimulation by Photodynamic Therapy: Bridging Fundamentals of Photochemically Induced Immunogenic Cell Death to the Enrichment of T-Cell Repertoire. Photochem Photobiol (2019) 95(6):1288–305. doi: 10.1111/php.13173
54. Sai D, Lee J, Nguyen D, Kim Y. Tailoring Photosensitive ROS for Advanced Photodynamic Therapy. Exp Mol Med (2021) 53(4):495–504. doi: 10.1038/s12276-021-00599-7
55. Huang Y, Tanaka M, Vecchio D, Garcia-Diaz M, Chang J, Morimoto Y, et al. Photodynamic Therapy Induces an Immune Response Against a Bacterial Pathogen. Expert Rev Clin Immunol (2012) 8(5):479–94. doi: 10.1586/eci.12.37
56. Beltran Hernandez I, Angelier ML, Del Buono D’Ondes T, Di Maggio A, Yu Y, Oliveira S. The Potential of Nanobody-Targeted Photodynamic Therapy to Trigger Immune Responses. Cancers (Basel) (2020) 12(4):978–94. doi: 10.3390/cancers12040978
57. Li W, Yang J, Luo L, Jiang M, Qin B, Yin H, et al. Targeting Photodynamic and Photothermal Therapy to the Endoplasmic Reticulum Enhances Immunogenic Cancer Cell Death. Nat Commun (2019) 10(1):3349. doi: 10.1038/s41467-019-11269-8
58. Shang T, Yu X, Han S, Yang B. Nanomedicine-Based Tumor Photothermal Therapy Synergized Immunotherapy. Biomater Sci (2020) 8(19):5241–59. doi: 10.1039/d0bm01158d
59. Garg AD, Agostinis P. ER Stress, Autophagy and Immunogenic Cell Death in Photodynamic Therapy-Induced Anti-Cancer Immune Responses. Photochem Photobiol Sci (2014) 13(3):474–87. doi: 10.1039/c3pp50333j
60. Pizova K, Tomankova K, Daskova A, Binder S, Bajgar R, Kolarova H. Photodynamic Therapy for Enhancing Antitumour Immunity. Biomed papers (2012) 156(2):93–102. doi: 10.5507/bp.2012.056
61. Radogna F, Diederich M. Stress-Induced Cellular Responses in Immunogenic Cell Death: Implications for Cancer Immunotherapy. Biochem Pharmacol (2018) 153:12–23. doi: 10.1016/j.bcp.2018.02.006
62. Wculek SK, Cueto FJ, Mujal AM, Melero I, Krummel MF, Sancho D. Dendritic Cells in Cancer Immunology and Immunotherapy. Nat Rev Immunol (2020) 20(1):7–24. doi: 10.1038/s41577-019-0210-z
63. Yap XZ, Lundie RJ, Beeson JG, O’Keeffe M. Dendritic Cell Responses and Function in Malaria. Front Immunol (2019) 10:357. doi: 10.3389/fimmu.2019.00357
64. Garg AD, Krysko DV, Vandenabeele P, Agostinis P. DAMPs and PDT-Mediated Photo-Oxidative Stress: Exploring the Unknown. Photochem Photobiol Sci (2011) 10(5):670–80. doi: 10.1039/c0pp00294a
65. Deng H, Zhou Z, Yang W, Lin LS, Wang S, Niu G, et al. Endoplasmic Reticulum Targeting to Amplify Immunogenic Cell Death for Cancer Immunotherapy. Nano Lett (2020) 20(3):1928–33. doi: 10.1021/acs.nanolett.9b05210
66. Alzeibak R, Mishchenko TA, Shilyagina NY, Balalaeva IV, Vedunova MV, Krysko DV. Targeting Immunogenic Cancer Cell Death by Photodynamic Therapy: Past, Present and Future. J Immunother Cancer (2021) 9(1):e001926. doi: 10.1136/jitc-2020-001926
67. Liang R, Liu L, He H, Chen Z, Han Z, Luo Z, et al. Oxygen-Boosted Immunogenic Photodynamic Therapy With Gold Nanocages@Manganese Dioxide to Inhibit Tumor Growth and Metastases. Biomaterials (2018) 177:149–60. doi: 10.1016/j.biomaterials.2018.05.051
68. Zhang Q, Li L. Photodynamic Combinational Therapy in Cancer Treatment. J BUON (2018) 23(3):561–7.
69. Morse MA, Gwin WR 3rd, Mitchell DA. Vaccine Therapies for Cancer: Then and Now. Target Oncol (2021) 16(2):121–52. doi: 10.1007/s11523-020-00788-w
70. Hwang HS, Shin H, Han J, Na K. Combination of Photodynamic Therapy (PDT) and Anti-Tumor Immunity in Cancer Therapy. J Pharm Investig (2018) 48(2):143–51. doi: 10.1007/s40005-017-0377-x
71. Kaneno R, Shurin G, Tourkova I, Shurin M. Chemomodulation of Human Dendritic Cell Function by Antineoplastic Agents in Low Noncytotoxic Concentrations. J Trans Med (2009) 7:58. doi: 10.1186/1479-5876-7-58
72. Koya T, Date I, Kawaguchi H, Watanabe A, Sakamoto T, Togi M, et al. Dendritic Cells Pre-Pulsed With Wilms’ Tumor 1 in Optimized Culture for Cancer Vaccination. Pharmaceutics (2020) 12(4):305. doi: 10.3390/pharmaceutics12040305
73. Pan H, Shi H, Fu P, Shi P, Yang J. Liposomal Dendritic Cell Vaccine in Breast Cancer Immunotherapy. ACS Omega (2021) 6(5):3991–8. doi: 10.1021/acsomega.0c05924
74. Yang W, Zhu G, Wang S, Yu G, Yang Z, Lin L, et al. In Situ Dendritic Cell Vaccine for Effective Cancer Immunotherapy. ACS Nano (2019) 13(3):3083–94. doi: 10.1021/acsnano.8b08346
75. Wang Y, Gong N, Ma C, Zhang Y, Tan H, Qing G, et al. An Amphiphilic Dendrimer as a Light-Activable Immunological Adjuvant for in Situ Cancer Vaccination. Nat Commun (2021) 12(1):4964. doi: 10.1038/s41467-021-25197-z
76. Beltrán Hernández I, Yu Y, Ossendorp F, Korbelik M, Oliveira S. Preclinical and Clinical Evidence of Immune Responses Triggered in Oncologic Photodynamic Therapy: Clinical Recommendations. J Clin Med (2020) 9(2):333–58. doi: 10.3390/jcm9020333
77. Korbelik M, Banath J, Zhang W, Gallagher P, Hode T, Lam SSK, et al. N-Dihydrogalactochitosan as Immune and Direct Antitumor Agent Amplifying the Effects of Photodynamic Therapy and Photodynamic Therapy-Generated Vaccines. Int Immunopharmacol (2019) 75:105764. doi: 10.1016/j.intimp.2019.105764
78. Bruger AM, Dorhoi A, Esendagli G, Barczyk-Kahlert K, van der Bruggen P, Lipoldova M, et al. How to Measure the Immunosuppressive Activity of MDSC: Assays, Problems and Potential Solutions. Cancer Immunol Immunother (2019) 68(4):631–44. doi: 10.1007/s00262-018-2170-8
79. Ni J, Song J, Wang B, Hua H, Zhu H, Guo X, et al. Dendritic Cell Vaccine for the Effective Immunotherapy of Breast Cancer. BioMed Pharmacother (2020) 126:110046. doi: 10.1016/j.biopha.2020.110046
80. Circelli L, Tornesello M, Buonaguro FM, Buonaguro L. Use of Adjuvants for Immunotherapy. Hum Vaccin Immunother (2017) 13(8):1774–7. doi: 10.1080/21645515.2017.1321725
81. Tan K, Li R, Huang X, Liu Q. Outer Membrane Vesicles: Current Status and Future Direction of These Novel Vaccine Adjuvants. Front Microbiol (2018) 9:783. doi: 10.3389/fmicb.2018.00783
82. Flatz L, Hegazy A, Bergthaler A, Verschoor A, Claus C, Fernandez M, et al. Development of Replication-Defective Lymphocytic Choriomeningitis Virus Vectors for the Induction of Potent CD8+ T Cell Immunity. Nat Med (2010) 16(3):339–45. doi: 10.1038/nm.2104
83. Speir M, Authier-Hall A, Brooks C, Farrand K, Compton B, Anderson R, et al. Glycolipid-Peptide Conjugate Vaccines Enhance CD8 T Cell Responses Against Human Viral Proteins. Sci Rep (2017) 7(1):14273. doi: 10.1038/s41598-017-14690-5
84. Zhang N, Channappanavar R, Ma C, Wang L, Tang J, Garron T, et al. Identification of an Ideal Adjuvant for Receptor-Binding Domain-Based Subunit Vaccines Against Middle East Respiratory Syndrome Coronavirus. Cell Mol Immunol (2016) 13(2):180–90. doi: 10.1038/cmi.2015.03
85. Wang Y, Zhang S, Li H, Wang H, Zhang T, Hutchinson MR, et al. Small-Molecule Modulators of Toll-Like Receptors. Accounts Chem Res (2020) 53(5):1046–55. doi: 10.1021/acs.accounts.9b00631
86. Bahmani B, Gong H, Luk B, Haushalter K, DeTeresa E, Previti M, et al. Intratumoral Immunotherapy Using Platelet-Cloaked Nanoparticles Enhances Antitumor Immunity in Solid Tumors. Nat Commun (2021) 12(1):1999. doi: 10.1038/s41467-021-22311-z
87. Vijay K. Toll-Like Receptors in Immunity and Inflammatory Diseases: Past, Present, and Future. Int Immunopharmacol (2018) 59:391–412. doi: 10.1016/j.intimp.2018.03.002
88. Patinote C, Karroum NB, Moarbess G, Cirnat N, Kassab I, Bonnet PA, et al. Agonist and Antagonist Ligands of Toll-Like Receptors 7 and 8: Ingenious Tools for Therapeutic Purposes. Eur J Med Chem (2020) 193:112238. doi: 10.1016/j.ejmech.2020.112238
89. Kim H, Griffith TS, Panyam J. Poly(d,l-Lactide-Co-Glycolide) Nanoparticles as Delivery Platforms for TLR7/8 Agonist-Based Cancer Vaccine. J Pharmacol Exp Ther (2019) 370(3):715–24. doi: 10.1124/jpet.118.254953
90. Bhatta AK, Wang P, Keyal U, Zhao Z, Ji J, Zhu L, et al. Therapeutic Effect of Imiquimod Enhanced ALA-PDT on Cutaneous Squamous Cell Carcinoma. Photodiagnosis Photodyn Ther (2018) 23:273–80. doi: 10.1016/j.pdpdt.2018.07.010
91. Wang L, He Y, He T, Liu G, Lin C, Li K, et al. Lymph Node-Targeted Immune-Activation Mediated by Imiquimod-Loaded Mesoporous Polydopamine Based-Nanocarriers. Biomaterials (2020) 255:120208. doi: 10.1016/j.biomaterials.2020.120208
92. Kang S-J, Tak J-H, Cho J-H, Lee H-J, Jung Y-J. Stimulation of the Endosomal TLR Pathway Enhances Autophagy-Induced Cell Death in Radiotherapy of Breast Cancer. Genes Genomics (2010) 32(6):599–606. doi: 10.1007/s13258-010-0139-x
93. Chuang KC, Chang CR, Chang SH, Huang SW, Chuang SM, Li ZY, et al. Imiquimod-Induced ROS Production Disrupts the Balance of Mitochondrial Dynamics and Increases Mitophagy in Skin Cancer Cells. J Dermatol Sci (2020) 98(3):152–62. doi: 10.1016/j.jdermsci.2020.03.009
94. Cho J, Lee H, Ko H, Yoon B, Choe J, Kim K, et al. The TLR7 Agonist Imiquimod Induces Anti-Cancer Effects via Autophagic Cell Death and Enhances Anti-Tumoral and Systemic Immunity During Radiotherapy for Melanoma. Oncotarget (2017) 8(15):24932–48. doi: 10.18632/oncotarget.15326
95. Xu J, Xu L, Wang C, Yang R, Zhuang Q, Han X, et al. Near-Infrared-Triggered Photodynamic Therapy With Multitasking Upconversion Nanoparticles in Combination With Checkpoint Blockade for Immunotherapy of Colorectal Cancer. ACS Nano (2017) 11(5):4463–74. doi: 10.1021/acsnano.7b00715
96. Frega G, Wu Q, Le Naour J, Vacchelli E, Galluzzi L, Kroemer G, et al. Trial Watch: Experimental TLR7/TLR8 Agonists for Oncological Indications. Oncoimmunology (2020) 9(1):1796002. doi: 10.1080/2162402X.2020.1796002
97. Yang Y, Csakai A, Jiang S, Smith C, Tanji H, Huang J, et al. Tetrasubstituted Imidazoles as Incognito Toll-Like Receptor 8 a(Nta)Gonists. Nat Commun (2021) 12(1):4351. doi: 10.1038/s41467-021-24536-4
98. Zhang H, Tang WL, Kheirolomoom A, Fite BZ, Wu B, Lau K, et al. Development of Thermosensitive Resiquimod-Loaded Liposomes for Enhanced Cancer Immunotherapy. J Control Release (2021) 330:1080–94. doi: 10.1016/j.jconrel.2020.11.013
99. Figueiredo P, Lepland A, Scodeller P, Fontana F, Torrieri G, Tiboni M, et al. Peptide-Guided Resiquimod-Loaded Lignin Nanoparticles Convert Tumor-Associated Macrophages From M2 to M1 Phenotype for Enhanced Chemotherapy. Acta Biomater (2020) S1742-7061(20):30561–74. doi: 10.1016/j.actbio.2020.09.038
100. Chen PM, Pan WY, Wu CY, Yeh CY, Korupalli C, Luo PK, et al. Modulation of Tumor Microenvironment Using a TLR-7/8 Agonist-Loaded Nanoparticle System That Exerts Low-Temperature Hyperthermia and Immunotherapy for in Situ Cancer Vaccination. Biomaterials (2020) 230:119629. doi: 10.1016/j.biomaterials.2019.119629
101. Hwang HS, Cherukula K, Bang YJ, Vijayan V, Moon MJ, Thiruppathi J, et al. Combination of Photodynamic Therapy and a Flagellin-Adjuvanted Cancer Vaccine Potentiated the Anti-PD-1-Mediated Melanoma Suppression. Cells (2020) 9(11):2432–50. doi: 10.3390/cells9112432
102. Bode C, Zhao G, Steinhagen F, Kinjo T, Klinman DM. CpG DNA as a Vaccine Adjuvant. Expert Rev Vaccines (2011) 10(4):499–511. doi: 10.1586/erv.10.174
103. Ni K, Luo T, Lan G, Culbert A, Song Y, Wu T, et al. A Nanoscale Metal-Organic Framework to Mediate Photodynamic Therapy and Deliver CpG Oligodeoxynucleotides to Enhance Antigen Presentation and Cancer Immunotherapy. Angew Chem Int Ed Engl (2020) 59(3):1108–12. doi: 10.1002/anie.201911429
104. Cai Z, Xin F, Wei Z, Wu M, Lin X, Du X, et al. Photodynamic Therapy Combined With Antihypoxic Signaling and CpG Adjuvant as an In Situ Tumor Vaccine Based on Metal-Organic Framework Nanoparticles to Boost Cancer Immunotherapy. Adv Healthc Mater (2020) 9(1):e1900996. doi: 10.1002/adhm.201900996
105. Shirota Y, Shirota H, Klinman DM. Intratumoral Injection of CpG Oligonucleotides Induces the Differentiation and Reduces the Immunosuppressive Activity of Myeloid-Derived Suppressor Cells. J Immunol (2012) 188(4):1592–9. doi: 10.4049/jimmunol.1101304
106. Shen L, Zhou T, Fan Y, Chang X, Wang Y, Sun J, et al. Recent Progress in Tumor Photodynamic Immunotherapy. Chin Chem Lett (2020) 31(7):1709–16. doi: 10.1016/j.cclet.2020.02.007
107. Zhou F, Song S, Chen WR, Xing D. Immunostimulatory Properties of Glycated Chitosan. J Xray Sci Technol (2011) 19(2):285–92. doi: 10.3233/XST-2011-0293
108. Cai X, Feng J, Chen F, Guo C, Sun L, Li L. Synergistic Effect of Glycated Chitosan and Photofrin Photodynamic Therapy on Different Breast Tumor Model. Photodiagnosis Photodyn Ther (2020) 31:101842. doi: 10.1016/j.pdpdt.2020.101842
109. Anzengruber F, Avci P, de Freitas LF, Hamblin MR. T-Cell Mediated Anti-Tumor Immunity After Photodynamic Therapy: Why Does it Not Always Work and How can We Improve it? Photochem Photobiol Sci (2015) 14(8):1492–509. doi: 10.1039/c4pp00455h
110. Dang J, He H, Chen D, Yin L. Manipulating Tumor Hypoxia Toward Enhanced Photodynamic Therapy (PDT). Biomater Sci (2017) 5(8):1500–11. doi: 10.1039/c7bm00392g
111. Mroz P, Hamblin MR. The Immunosuppressive Side of PDT. Photochem Photobiol Sci (2011) 10(5):751–8. doi: 10.1039/c0pp00345j
112. Liu Y, Cao X. Immunosuppressive Cells in Tumor Immune Escape and Metastasis. J Mol Med (Berl) (2016) 94(5):509–22. doi: 10.1007/s00109-015-1376-x
113. Jo Y, Ali LA, Shim JA, Lee BH, Hong C. Innovative CAR-T Cell Therapy for Solid Tumor; Current Duel Between CAR-T Spear and Tumor Shield. Cancers (Basel) (2020) 12(8):2087. doi: 10.3390/cancers12082087
114. Condamine T, Gabrilovich DI. Molecular Mechanisms Regulating Myeloid-Derived Suppressor Cell Differentiation and Function. Trends Immunol (2011) 32(1):19–25. doi: 10.1016/j.it.2010.10.002
115. Thommen D, Schumacher T. T Cell Dysfunction in Cancer. Cancer Cell (2018) 33(4):547–62. doi: 10.1016/j.ccell.2018.03.012
116. Ohue Y, Nishikawa H. Regulatory T (Treg) Cells in Cancer: Can Treg Cells be a New Therapeutic Target? Cancer Sci (2019) 110(7):2080–9. doi: 10.1111/cas.14069
117. Korbelik M, Banath J, Saw KM. Immunoregulatory Cell Depletion Improves the Efficacy of Photodynamic Therapy-Generated Cancer Vaccines. Int J Mol Sci (2015) 16(11):27005–14. doi: 10.3390/ijms161126008
118. Lee J, Choi S, Jung N, Song J, Seo H, Lee H, et al. The Effect of the Tumor Microenvironment and Tumor-Derived Metabolites on Dendritic Cell Function. J Cancer (2020) 11(4):769–75. doi: 10.7150/jca.38785
119. Zhou B, Gao Y, Zhang P, Chu Q. Acquired Resistance to Immune Checkpoint Blockades: The Underlying Mechanisms and Potential Strategies. Front Immunol (2021) 12:693609. doi: 10.3389/fimmu.2021.693609
120. Cheong JE, Ekkati A, Sun L. A Patent Review of IDO1 Inhibitors for Cancer. Expert Opin Ther Pat (2018) 28(4):317–30. doi: 10.1080/13543776.2018.1441290
121. Wachowska M, Stachura J, Tonecka K, Fidyt K, Braniewska A, Sas Z, et al. Inhibition of IDO Leads to IL-6-Dependent Systemic Inflammation in Mice When Combined With Photodynamic Therapy. Cancer Immunol Immunother (2020) 69(6):1101–12. doi: 10.1007/s00262-020-02528-5
122. Zhao LP, Zheng RR, Huang JQ, Chen XY, Deng FA, Liu YB, et al. Self-Delivery Photo-Immune Stimulators for Photodynamic Sensitized Tumor Immunotherapy. ACS Nano (2020) 14(12):17100–13. doi: 10.1021/acsnano.0c06765
123. Yang W, Zhang F, Deng H, Lin L, Wang S, Kang F, et al. Smart Nanovesicle-Mediated Immunogenic Cell Death Through Tumor Microenvironment Modulation for Effective Photodynamic Immunotherapy. ACS Nano (2020) 14(1):620–31. doi: 10.1021/acsnano.9b07212
124. Gabrilovich DI, Ostrand-Rosenberg S, Bronte V. Coordinated Regulation of Myeloid Cells by Tumours. Nat Rev Immunol (2012) 12(4):253–68. doi: 10.1038/nri3175
125. Mo Z, Yu F, Han S, Yang S, Wu L, Li P, et al. New Peptide MY1340 Revert the Inhibition Effect of VEGF on Dendritic Cells Differentiation and Maturation via Blocking VEGF-NRP-1 Axis and Inhibit Tumor Growth In Vivo. Int Immunopharmacol (2018) 60:132–40. doi: 10.1016/j.intimp.2018.04.025
126. Huang Y, Kim BYS, Chan CK, Hahn SM, Weissman IL, Jiang W. Improving Immune-Vascular Crosstalk for Cancer Immunotherapy. Nat Rev Immunol (2018) 18(3):195–203. doi: 10.1038/nri.2017.145
127. Zhou Y, Ren X, Hou Z, Wang N, Jiang Y, Luan Y. Engineering a Photosensitizer Nanoplatform for Amplified Photodynamic Immunotherapy via Tumor Microenvironment Modulation. Nanoscale Horiz (2021) 6(2):120–31. doi: 10.1039/d0nh00480d
128. Ding D, Zhong H, Liang R, Lan T, Zhu X, Huang S, et al. Multifunctional Nanodrug Mediates Synergistic Photodynamic Therapy and MDSCs-Targeting Immunotherapy of Colon Cancer. Advanced Sci (Weinheim Baden-Wurttemberg Germany) (2021) 8(14):e2100712. doi: 10.1002/advs.202100712
129. Aversa I, Malanga D, Fiume G, Palmieri C. Molecular T-Cell Repertoire Analysis as Source of Prognostic and Predictive Biomarkers for Checkpoint Blockade Immunotherapy. Int J Mol Sci (2020) 21(7):2378. doi: 10.3390/ijms21072378
130. Olivo Pimentel V, Marcus D, van der Wiel A, Lieuwes N, Biemans R, Lieverse R, et al. Releasing the Brakes of Tumor Immunity With Anti-PD-L1 and Pushing Its Accelerator With L19-IL2 Cures Poorly Immunogenic Tumors When Combined With Radiotherapy. J immunotherapy Cancer (2021) 9(3):e001764. doi: 10.1136/jitc-2020-001764
131. Cramer GM, Moon EK, Cengel KA, Busch TM. Photodynamic Therapy and Immune Checkpoint Blockade(Dagger). Photochem Photobiol (2020) 96(5):954–61. doi: 10.1111/php.13300
132. Ni K, Luo T, Nash GT, Lin W. Nanoscale Metal-Organic Frameworks for Cancer Immunotherapy. Acc Chem Res (2020) 53(9):1739–48. doi: 10.1021/acs.accounts.0c00313
133. Cheng X, Zhao G, Zhao Y. Combination Immunotherapy Approaches for Pancreatic Cancer Treatment. Can J Gastroenterol Hepatol (2018) 2018:6240467. doi: 10.1155/2018/6240467
134. Van Coillie S, Wiernicki B, Xu J. Molecular and Cellular Functions of CTLA-4. Adv Exp Med Biol (2020) 1248:7–32. doi: 10.1007/978-981-15-3266-5_2
135. Lipson EJ, Drake CG. Ipilimumab: An Anti-CTLA-4 Antibody for Metastatic Melanoma. Clin Cancer Res (2011) 17(22):6958–62. doi: 10.1158/1078-0432.CCR-11-1595
136. Kleinovink JW, Fransen MF, Lowik CW, Ossendorp F. Photodynamic-Immune Checkpoint Therapy Eradicates Local and Distant Tumors by CD8(+) T Cells. Cancer Immunol Res (2017) 5(10):832–8. doi: 10.1158/2326-6066.CIR-17-0055
137. Wang Z, Zhang F, Shao D, Chang Z, Wang L, Hu H, et al. Janus Nanobullets Combine Photodynamic Therapy and Magnetic Hyperthermia to Potentiate Synergetic Anti-Metastatic Immunotherapy. Adv Sci (Weinh) (2019) 6(22):1901690. doi: 10.1002/advs.201901690
138. Han Y, Liu D, Li L. PD-1/PD-L1 Pathway: Current Researches in Cancer. Am J Cancer Res (2020) 10(3):727–42.
139. Drakes M, Czerlanis C, Stiff P. Immune Checkpoint Blockade in Gynecologic Cancers: State of Affairs. Cancers (2020) 12(11):3301. doi: 10.3390/cancers12113301
140. Brahmer J, Reckamp K, Baas P, Crinò L, Eberhardt W, Poddubskaya E, et al. Nivolumab Versus Docetaxel in Advanced Squamous-Cell Non-Small-Cell Lung Cancer. New Engl J Med (2015) 373(2):123–35. doi: 10.1056/NEJMoa1504627
141. Ribas A, Wolchok J. Cancer Immunotherapy Using Checkpoint Blockade. Sci (New York NY) (2018) 359(6382):1350–5. doi: 10.1126/science.aar4060
142. Bao R, Wang Y, Lai J, Zhu H, Zhao Y, Li S, et al. Enhancing Anti-PD-1/PD-L1 Immune Checkpoint Inhibitory Cancer Therapy by CD276-Targeted Photodynamic Ablation of Tumor Cells and Tumor Vasculature. Mol Pharm (2019) 16(1):339–48. doi: 10.1021/acs.molpharmaceut.8b00997
143. Li X, Jeon YH, Kwon N, Park JG, Guo T, Kim HR, et al. In Vivo-Assembled Phthalocyanine/Albumin Supramolecular Complexes Combined With a Hypoxia-Activated Prodrug for Enhanced Photodynamic Immunotherapy of Cancer. Biomaterials (2021) 266:120430. doi: 10.1016/j.biomaterials.2020.120430
144. Liu Q, Tian J, Tian Y, Sun Q, Sun D, Wang F, et al. Near-Infrared-II Nanoparticles for Cancer Imaging of Immune Checkpoint Programmed Death-Ligand 1 and Photodynamic/Immune Therapy. ACS Nano (2021) 15(1):515–25. doi: 10.1021/acsnano.0c05317
145. Pierik A, Leemans C, Brakenhoff R. Resection Margins in Head and Neck Cancer Surgery: An Update of Residual Disease and Field Cancerization. Cancers (2021) 13(11):2635. doi: 10.3390/cancers13112635
146. Zhang Y, Liao Y, Tang Q, Lin J, Huang P. Biomimetic Nanoemulsion for Synergistic Photodynamic-Immunotherapy Against Hypoxic Breast Tumor. Angew Chem Int Ed Engl (2021) 60(19):10647–53. doi: 10.1002/anie.202015590
147. Waters W, Palmer M, Nonnecke B, Thacker T, Estes D, Larsen M, et al. Signal Regulatory Protein Alpha (SIRPalpha) Cells in the Adaptive Response to ESAT-6/CFP-10 Protein of Tuberculous Mycobacteria. PloS One (2009) 4(7):e6414. doi: 10.1371/journal.pone.0006414
148. Zhang S, Dehn S, DeBerge M, Rhee K, Hudson B, Thorp E. Phagocyte-Myocyte Interactions and Consequences During Hypoxic Wound Healing. Cell Immunol (2014) 291:65–73. doi: 10.1016/j.cellimm.2014.04.006
149. Matlung HL, Szilagyi K, Barclay NA, van den Berg TK. The CD47-SIRPalpha Signaling Axis as an Innate Immune Checkpoint in Cancer. Immunol Rev (2017) 276(1):145–64. doi: 10.1111/imr.12527
150. Zheng Y, Zou F, Wang J, Yin G, Le V, Fei Z, et al. Photodynamic Therapy-Mediated Cancer Vaccination Enhances Stem-Like Phenotype and Immune Escape, Which can be Blocked by Thrombospondin-1 Signaling Through CD47 Receptor Protein. J Biol Chem (2015) 290(14):8975–86. doi: 10.1074/jbc.M114.624965
151. Chang M, Hou Z, Jin D, Zhou J, Wang M, Wang M, et al. Colorectal Tumor Microenvironment-Activated Bio-Decomposable and Metabolizable Cu2O@CaCO3 Nanocomposites for Synergistic Oncotherapy. Adv Mater (2020) 32(43):e2004647. doi: 10.1002/adma.202004647
152. Bou Kheir G, Aoun F, Roumeguere T. CD47 Targeted Near-Infrared Photo-Immunotherapy: A Promising Tool Combining Monoclonal Antibodies and Photodynamics for Treating Human Bladder Cancer. Transl Androl Urol (2019) 8(6):779–80. doi: 10.21037/tau.2019.07.10
153. Qin S, Xu L, Yi M, Yu S, Wu K, Luo S. Novel Immune Checkpoint Targets: Moving Beyond PD-1 and CTLA-4. Mol Cancer (2019) 18(1):155. doi: 10.1186/s12943-019-1091-2
154. Belliere J, Mazieres J, Meyer N, Chebane L, Despas F. Renal Complications Related to Checkpoint Inhibitors: Diagnostic and Therapeutic Strategies. Diagnostics (Basel Switzerland) (2021) 11(7):1187. doi: 10.3390/diagnostics11071187
155. Reginato E, Lindenmann J, Langner C, Schweintzger N, Bambach I, Smolle-Jüttner F, et al. Photodynamic Therapy Downregulates the Function of Regulatory T Cells in Patients With Esophageal Squamous Cell Carcinoma. Photochemical photobiological Sci Off J Eur Photochemistry Assoc Eur Soc Photobiol (2014) 13(9):1281–9. doi: 10.1039/c4pp00186a
Keywords: photodynamic therapy, immunotherapy, combination, tumor, nanoparticle
Citation: Hua J, Wu P, Gan L, Zhang Z, He J, Zhong L, Zhao Y and Huang Y (2021) Current Strategies for Tumor Photodynamic Therapy Combined With Immunotherapy. Front. Oncol. 11:738323. doi: 10.3389/fonc.2021.738323
Received: 08 July 2021; Accepted: 27 October 2021;
Published: 17 November 2021.
Edited by:
Alessandro Poggi, San Martino Hospital (IRCCS), ItalyReviewed by:
Fernando Lucas Primo, São Paulo State University, BrazilCopyright © 2021 Hua, Wu, Gan, Zhang, He, Zhong, Zhao and Huang. This is an open-access article distributed under the terms of the Creative Commons Attribution License (CC BY). The use, distribution or reproduction in other forums is permitted, provided the original author(s) and the copyright owner(s) are credited and that the original publication in this journal is cited, in accordance with accepted academic practice. No use, distribution or reproduction is permitted which does not comply with these terms.
*Correspondence: Yong Huang, aHVhbmd5b25nNTAzQDEyNi5jb20=
Disclaimer: All claims expressed in this article are solely those of the authors and do not necessarily represent those of their affiliated organizations, or those of the publisher, the editors and the reviewers. Any product that may be evaluated in this article or claim that may be made by its manufacturer is not guaranteed or endorsed by the publisher.
Research integrity at Frontiers
Learn more about the work of our research integrity team to safeguard the quality of each article we publish.