- Brain Metastasis Group, Molecular Oncology Programme, Spanish National Cancer Research Centre (CNIO), Madrid, Spain
Uncovering the complexity of the microenvironment that emerges in brain disorders is key to identify potential vulnerabilities that might help challenging diseases affecting this organ. Recently, genomic and proteomic analyses, especially at the single cell level, have reported previously unrecognized diversity within brain cell types. The complexity of the brain microenvironment increases during disease partly due to the immune infiltration from the periphery that contributes to redefine the brain connectome by establishing a new crosstalk with resident brain cell types. Within the rewired brain ecosystem, glial cell subpopulations are emerging hubs modulating the dialogue between the Immune System and the Central Nervous System with important consequences in the progression of brain tumors and other disorders. Single cell technologies are crucial not only to define and track the origin of disease-associated cell types, but also to identify their molecular similarities and differences that might be linked to specific brain injuries. These altered molecular patterns derived from reprogramming the healthy brain into an injured organ, might provide a new generation of therapeutic targets to challenge highly prevalent and lethal brain disorders that remain incurable with unprecedented specificity and limited toxicities. In this perspective, we present the most relevant clinical and pre-clinical work regarding the characterization of the heterogeneity within different components of the microenvironment in the healthy and injured brain with a special interest on single cell analysis. Finally, we discuss how understanding the diversity of the brain microenvironment could be exploited for translational purposes, particularly in primary and secondary tumors affecting the brain.
Introduction
The brain microenvironment represents a complex habitat that notably differs from the microenvironment associated with other tumors (1). In addition to the still incomplete understanding of brain homeostasis and the structural heterogeneity of this organ, the presence of any insult, such as a tumor, might contribute to amplify the pre-existing diversity within the microenvironment.
Imaging, genomic and proteomic analyses have been valuable tools for dissecting inter- and intra-regional heterogeneity within the brain. Initially applied to uncover neuronal subtypes across brain regions (2–5), single-cell RNA sequencing (scRNAseq), single-nucleus RNA sequencing (snRNAseq), mass cytometry (CyTOF) and spatial transcriptomics, have also proved to be a powerful tool beyond non-neuronal cells, revolutionizing the way we interrogate cancer-associated heterogeneity. Recently, the principles of scRNAseq have been expanded to elucidate in vivo networks based on cell-to-single cell interactions (6–8). These studies are dramatically expanding the complexity of the brain that should be translated into comprehensive pharmacologic approaches overcoming initial technical difficulties associated with this organ (9).
Although the characterization of altered molecular pathways within the brain microenvironment at the single cell level in brain tumors, especially in brain metastasis, is still limited, in this perspective we take advantage of findings obtained from other contexts (Figure 1) to discuss how exploiting heterogeneity could be translated into novel therapeutic strategies also for brain tumors.
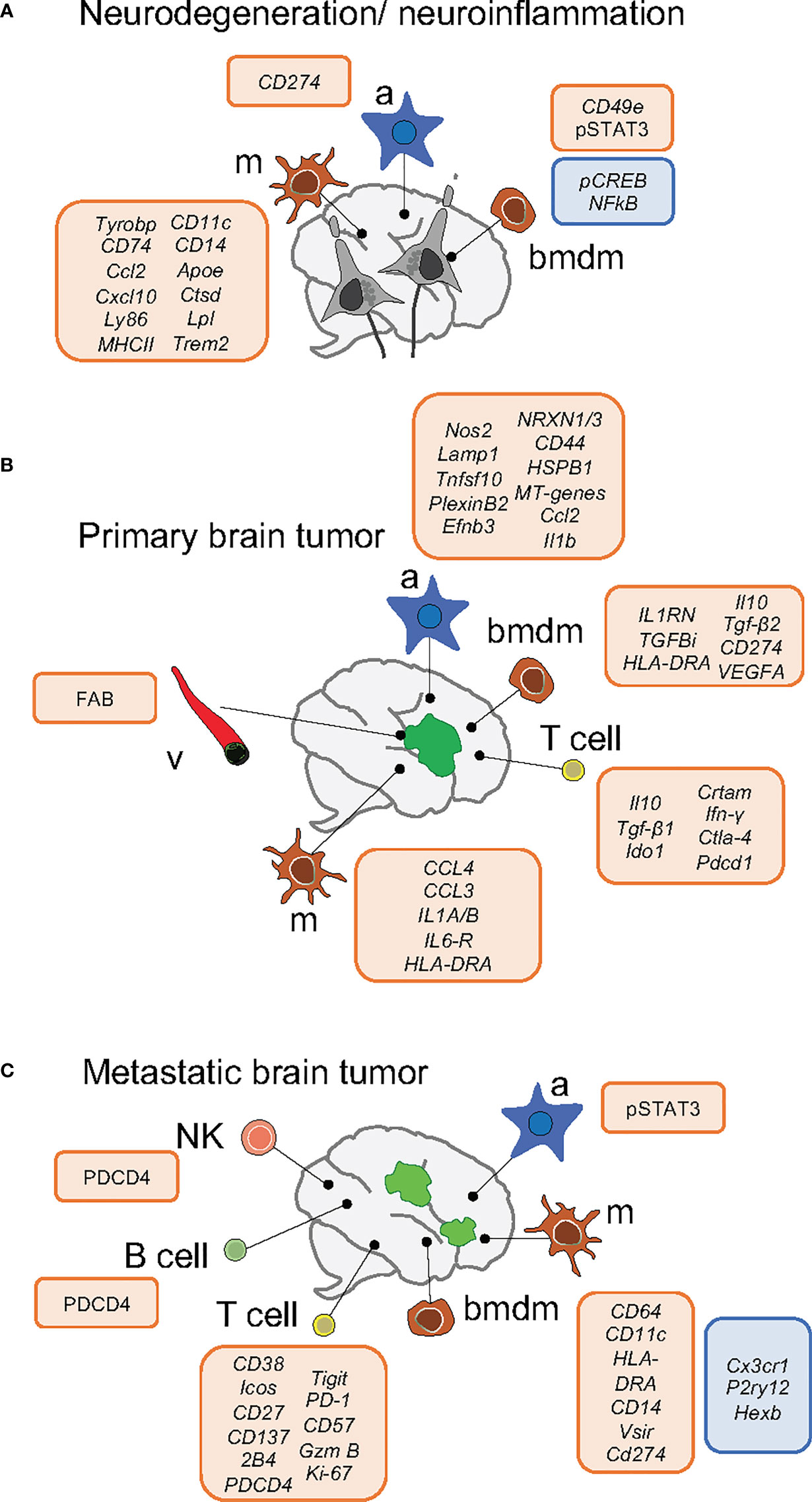
Figure 1 Schema of key markers deregulated within the brain microenvironment in neurodegeneration/neuroinflammation (A) and primary (B) and secondary (C) brain tumors. Upregulation is indicated by the box in red and downregulation by the box in blue. Neurodegeneration/neuroinflammation comprises the following brain disorders: Alzheimer, Huntington disease, Amyotrophic Lateral Sclerosis and Experimental Autoimmune Encephalomyelitis. a, astrocytes; m, microglia; bmdm, bone marrow-derived macrophages; v, vasculature.
Diversity of Macrophages Within the Brain Microenvironment
Health and Aging
scRNA-seq approaches have uncovered specific transcriptomic profiles that distinguish brain microglia and macrophages (2, 10–13). Additionally, different microglial states have been found at embryonic and early postnatal time points (14–16), while aging modulates inflammatory and interferon response signatures in microglia (14), as detailed in Table 1.
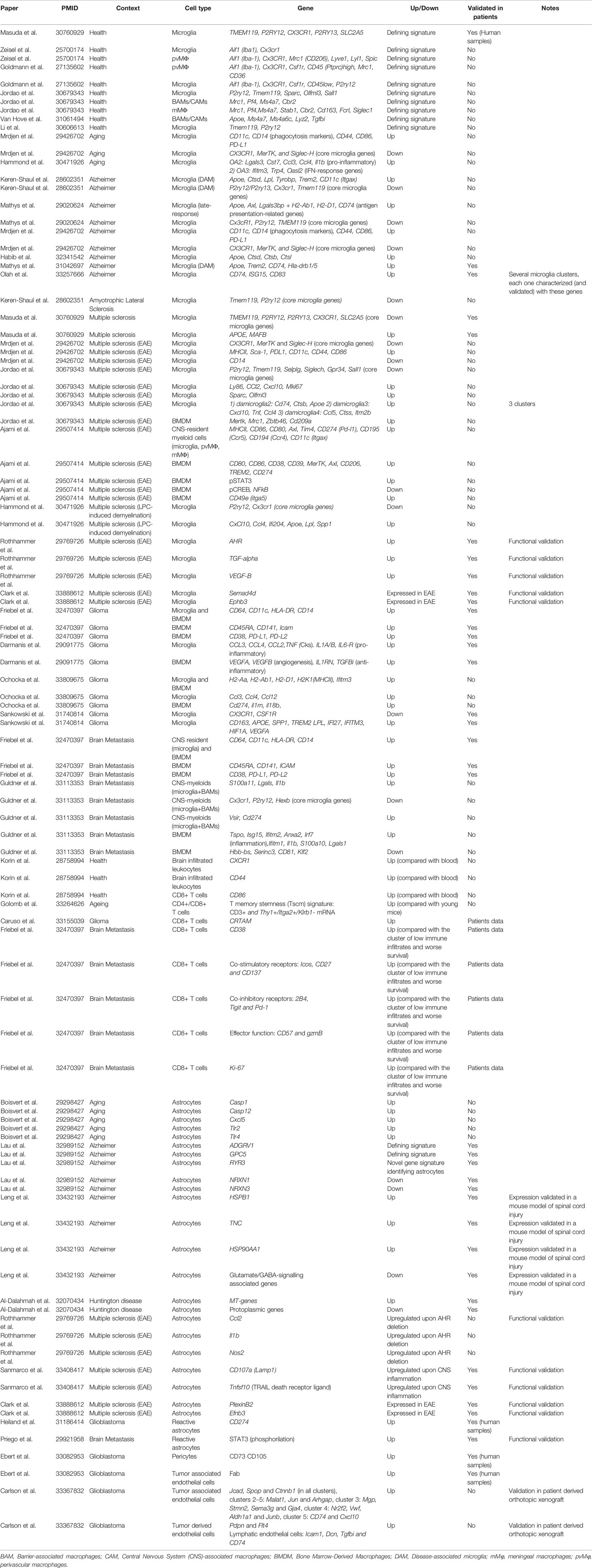
Table 1 Key signatures and markers found in microglia/macrophages, T cells, astrocytes and endothelial cells subpopulations within the brain in preclinical models and/or patients of brain disorders and primary and secondary brain tumors.
Brain Disorders
During Alzheimer disease (AD), disease-associated microglia (DAM) and late-response microglia are defined by the expression of genes related to lipid metabolism and phagocytosis (ApoE, Lpl, Trem2, Tyrobp, Ctsd) and interferon response (17, 18). By combining CyTOF with lineage tracing models Mrdjen et al. identified a subset of microglia during AD characterized by the upregulation of phagocytic markers CD11c and CD14. However, the specific functional contribution of DAMs during AD remains unclear (17, 19). During Experimental Autoimmune Encephalomyelitis (EAE) microglia showed a similar signature, except for decreased CD14 and increased MHCII and Sca-1 expression (11). In the same line, Ajami et al. identified two CNS-resident myeloid populations increased in frequency during EAE, Amyotrophic Lateral Sclerosis (ALS) and Huntington’s disease (HD) (20) and Jordao et al. described four disease-associated microglia in EAE (Table 1 details defining gene signatures). Peripheral monocyte populations present in the EAE model, but absent in AD and HD, express CD49e and show higher expression of pSTAT3 and lower of pCREB and NFκ-B in comparison to resident myeloid cells.
Remarkably, high-throughput technological pipelines are now available to profile novel cell-to-cell interactions at a single cell level. Clark et al. combines molecular barcoding, viral tracing and scRNASeq in vivo (RABID-seq) to map the microglia-astrocyte crosstalk during EAE, being responsible of inducing a pro-inflammatory microenvironment through two main axes: Sema4D-PlexinB2 and Ephrin-B3/EphB3 (8).
As summarized in Table 1, analysis of human and mouse microglia suggests high correlation in their transcriptomic profiles (i.e. upregulation of Apoe) and highlight the broader heterogeneity of human microglia (15, 21–23).
Brain Tumors
Recent sc-RNAseq analysis found that the interaction of tumor-associated macrophages (TAMs) and glioma cells occurs mainly through CXCL chemokines and their receptors (24). Furthermore, scRNA-seq analysis of CD11b+ myeloid cells isolated from murine experimental GL261 gliomas unveiled that activated microglia and BMDM significantly change their transcriptional networks, with upregulation of MHCII related proteins (25). In glioma patients, TAM BMDM invade the tumor core displaying an anti-inflammatory and pro-angiogenic phenotype, expressing immunosuppressive cytokines (i.e. Il10 and Tgfβ2) and markers of active phagocytosis (CD93). Meanwhile, microglia located in the surrounding space is characterized by the expression of pro-inflammatory molecules (i.e. CCL4, CCL3, IL1A/B) (25–27).
Recently this heterogeneity has also been addressed in brain metastasis in comparison to gliomas. Friebel et al. found that, while the glioma microenvironment is predominantly composed by activated microglia, brain metastases are characterized by the infiltration of BMDM (28). Similarly, Guldner et al. identified myeloid clusters characterized by the expression of complement genes, while BMDMs express higher levels of inflammatory genes (S100a11, Lgals, Il1b) in brain metastasis. Furthermore, it was shown that loss of Cx3cr1 in CNS-myeloid cells triggers upregulation of Cxcl10, which in turn drives an immunosuppressive pro-metastatic microenvironment through PD-L1 and VISTA. Interestingly, co-inhibition of both molecules reduced the brain metastatic burden (29).
Lymphocytes and Natural Killer Cells Heterogeneity Within the Brain Microenvironment
Health and Ageing
Applying CyTOF to the naïve mouse brain, Korine et al. found that CD4+ and CD8+ infiltrating T cells express markers of memory T cells (CD44+CD62L-) and could be characterized by the increased expression of CD86 and CX3CR1 in comparison to their blood counterparts. Indeed, CD44 was suggested to be a general marker for brain infiltrating immune populations (30). Brain B cells and NK cells, which are found in lower numbers than in peripheral blood, particularly IgM+ B cells, are also defined by CX3CR1 expression (30). In the aged brain, using cellular indexing of transcriptomes and epitopes by sequencing (CITE-seq), T cells were found to express a T cell memory stemness signature characterize by CD3+ and Thy1+/Itga2+/Klrb1- mRNA expression and additional gene signatures associated with chemotaxis and ribosomal proteins, including Ly6a and Dusp2 expression. These findings suggest that organismal aging correlates with the enrichment of specific lymphocytes populations within the brain (31).
Brain Tumors
Single-cell transcriptomics uncovered a gene signature in glioma composed by immune effector molecules and inhibitory feedback mechanisms (genes such as Ifn-γ, Ctla-4, Pdcd1, IL-10, Tgf-β1 or Ido1) that lead to the reprogramming of T cells subsets that become unable to target the cancer cells (32). A more specific dissection of the crosstalk between glioma cells and T cells in patients was achieved by applying single-cell Tumor-Host Interaction (scTHI) analysis of scRNA sequencing data. In particular, Caruso et al. found that the cross-talk between CD8+ T cells and tumor cells included components belonging to major histocompatibility complex Class I, chemokines, interleukins, IFN-γ and TNF. This study also described paracrine interactions with myeloid cells involving immune checkpoint genes, TNF family members and chemoattractant chemokine ligands, such as CXCR6 receptor on T cells and its ligand CXCL16 secreted by macrophages that are upregulated in glioma (7).
Cy-TOF of surgical resections have characterized the lymphocyte landscape in primary and secondary brain tumor entities (28).Compared to primary brain tumors, metastases favor T and B cell infiltration and T regulatory cells (T regs) present higher accumulation in brain metastasis and IDH1 wt gliomas. Moreover, CD8+ T cells present an increased expression of co-stimulatory and co-inhibitory receptors, the activation marker CD38 and effector and proliferation functions in metastases, while glioma samples show less activation (28). The activation/exhaustion phenotypic state of T cells in metastatic tumors could explain their favorable clinical response to immune checkpoint inhibitors compared to those of primary origin.
Recent papers shed light on the stromal and immune landscape in human multiple sclerosis and brain tumors, focusing on the analysis by scRNAseq and set enrichment analysis of cerebrospinal fluid (CSF) leukocytes (33), and CSF from patients (34, 35). Specifically, Rubio-Perez et al. have described an inflammatory status independently of the primary tumor source of the metastasis and a cluster characterized by active proliferation of T cells. Noteworthy, identical T cell receptor sequences between the CSF and the metastatic lesions were detected in 66.7% of patients, indicating a partial connection of the immune profiles from both compartments (34). This work suggests the potential value of CSF to characterize the immune microenvironment and T cells subclonal evolution in brain metastasis to monitor patients during tumor progression or treatment.
Astrocytes Diversity Within the Brain Microenvironment
Health and Aging
Different studies have shown that astrocytic transcriptome heterogeneity encompasses well-recognized astrocyte functions and happens both between and within brain regions (9, 36). In aged brains, cerebellar astrocytes were characterized by the upregulation of inflammatory factors that can damage synapses (caspase-1 and -12, Cxcl5) and key inflammasome receptors Tlr2 and 4. This demonstrates that dependency of the glial cell type correlates with more severe or less synaptic dysfunction (37).
Brain Disorders
Astrocytes can be rapidly activated in response to various insults, by a process known as “reactive astrogliosis” which aims to limit the damage that occurs locally. Three states of reactive astrocytes (RAs) can be found in HD, defined by different levels of GFAP, metallothionein (MT) genes and quiescent protoplasmic genes. The upregulation of MTs by RAs could be a protective response to combat oxidative stress, which is characteristic of the HD brain (38). Interestingly, astrocytes in AD were found to express a unique and novel signature (Adgrv1, Gpc5 and Ryr3 genes). Down-regulated genes in AD-astrocytes are associated mainly with synaptic signaling (i.e. NRXN1 and NRXN3) and glutamate secretion (39). An independent study, showed that high GFAP astrocytes from AD, which lose homeostatic functions, also express pan-astrocytes and reactive markers such as CD44, HSPB1, TNC and HSP90AA1 (40). Remarkably, some recent studies emphasize the gut-brain axis as an important player during the course of CNS disease that fine-tunes inflammation and neurodegeneration. In EAE, the deletion of aryl hydrocarbon receptor (AHR) in microglia, upregulated the expression of genes in astrocytes associated with inflammation and neurodegeneration (Ccl2, Il1b and Nos2) (41). A later study described a subset of LAMP1+ astrocytes limiting inflammation, driven by IFNγ produced by meningeal natural killer cells, which is modulated by the commensal flora in mice (42). Notably, Clark et al. use the RABID-seq technology to identify pro-inflammatory astrocytes connected to T cells that exhibited high TNFα signaling via NF-κB (8).
Brain Tumors
In malignant brain tumors, knowledge related to astrocyte function and crosstalk to other components of the environment requires further investigation. Tumor-occupying astrocytes analyzed in three glioblastoma patients revealed similarities to highly proliferative astrocyte precursor cells from fetal brains (43). JAK/STAT pathway activation and CD274 expression was present in RAs, in a set of de-novo and recurrent glioblastoma specimens, inducing immunological cold tumor environment (44). Notably, in the context of brain metastasis, a pro-metastatic program driven by STAT3 signaling in a subpopulation of RAs surrounding metastatic lesions promotes an immunosuppressive microenvironment, being an interesting target (45).
Heterogeneity of Endothelial Cells Within the Brain Microenvironment
Health
The lack of a molecular understanding of the constituent cell types of the brain vasculature could be solved by using single cell approaches. In murine models, single-cell transcriptomics distinguished different molecular signatures and phenotypic changes in endothelial and mural cells (46). Moreover, brain-specific endothelial transcripts have been identified, mainly cell surface transporters and intracellular enzymes (47).
Brain Tumors
In a glioblastoma mouse model, single cell sequencing identified three separated clusters of brain endothelium with a distinct molecular signature, differentiating tumor associated vessels and tumor derived endothelial cells (detailed in Table 1) (48). Moreover, in human samples, heterogeneity was reported within pericytes and endothelial cells (49). These pioneer studies describe molecular inter and intra-heterogeneity within the primary brain tumor vasculature.
In human brain metastasis patients, clusters of endothelial cells have been identified using the marker CLDN5+, being in higher proportion in melanoma than in breast cancer brain metastasis (50).
Therapeutic Strategies Exploiting the Heterogeneity Within the Brain Microenvironment
Uncovering functional and molecular diversity of glial and brain immune cells in preclinical models and patients affected by disease has a remarkable translational potential, including brain tumors. However, an important effort in the field is needed to validate the contribution of disease-associated alterations and cellular cross-talk between the various reactive states described.
Brain Disorders
Ajami et al. proposed the surface marker CD49e found in peripheral monocytes, to be a therapeutic target in EAE since the treatment with anti-CD49e antibody significantly reduced disease severity (20). Interestingly, in the treatment of brain neurodegeneration, targeted immunotherapies may be used against B cell clusters responsible for disease-specific antibody production (51). Mapping the cross-talk between identified cell populations that shape the local microenvironment in brain disorders is key to uncover potential targets (8). Clark et al. have shown that in a EAE model, inactivating the interaction between Sema4d-Plxnb2 or Ephb3-Efnb3 in microglia-astrocytes, respectively, ameliorates the disease (8). Interestingly, as a proof of concept in traumatic brain injury models (mTBI), Arneson et al. focused on the thyroid hormone pathway based on its differential expression across cell types in mTBI. Injecting T4 immediately after the damage improved cognitive deficits in a mouse model of concussive injury (52). In addition, specific gene expression programs related to endosome, plasma membrane, mitochondrion and autophagy have been shown to be relevant for the progression of neurodegeneration in humans, especially when enriched in neurons and microglia (53, 54). This finding emphasizes the emerging vulnerability of dysfunctional bioenergetics for brain disorders.
Brain Tumors
Understanding the diversity within the microenvironment of clinically-relevant experimental models of brain tumors will help to identify altered pathways not essential for brain homeostasis. To illustrate this point, using single cell transcriptomics in the DNp53-PDGFB glioma model, Weng et al. have been able to identify the RNA-binding protein Zfp36l1 to be necessary for malignant oligodendrocyte-astrocyte lineage transition and glioma growth (55). In human primary and secondary tumors, candidate immunosuppressive molecules could be used to potentiate immunotherapy by designing customized strategies for brain tumors. For instance, by using single-cell gene expression Caruso et al. found TLR2 to be exclusively upregulated in glioma-associated microglia and CRTAM receptor in CD8+ T cells, confirming previous studies (56, 57) that have proposed these molecules as targets for adjuvant immunotherapies in glioma. Additionally, the same scRNAseq study defined ligand-receptor interactions between the microenvironment and cancer cells such as HBEGF-EGFR, MIF-CD74 and CD11B/CD18-CD90, that could be potential targets given their role in immunosuppression. FAB, identified mainly in endothelial cells and pericytes by scRNAseq, has been proposed as a potential antigen for (CAR)‐T cells therapy to target tumor cells and tumor associated vessels in glioblastoma (49).
Intrinsic properties of cancer cells could indirectly influence response to therapy by modulating the brain microenvironment. Whether the mutational status of cancer cells could influence the brain microenvironment in secondary brain tumors, as it does in primary brain tumors (58–62), is still unexplored. However changes in the immune infiltrate have been reported depending on the primary origin of brain metastases. For example, melanoma brain metastasis present higher frequencies of T cells than carcinoma brain metastasis, except for Tregs (28, 61). Notably, tumor location influences microenvironmental landscapes (63, 64). Meningiomas have higher TAM infiltration and less presence of T regs than gliomas (64), while in secondary brain tumors, scRNAseq revealed a distinct immune-suppressed T-cell microenvironment in leptomeningeal metastasis compared with brain metastasis derived from melanoma (63).
Furthermore, a deep knowledge of immune diversity induced by the presence of tumor cells is critical to predict immunotherapeutic outcomes since it might help to explain the different response to checkpoint inhibitors reported in primary and secondary brain tumors. For instance, Close et al. suggest that the presence of immune signatures with anti-tumor effector functions (i.e. granzyme B or IFN-γ) in a subset of patients with GBM will predispose to better benefit from combination immunotherapies (32). In addition, immune evasion signatures have been defined and novel targets, such as CDK4/6, have been proposed to overcome the resistance to immune checkpoint blockade in cancer metastatic to the brain (65, 66). scRNAseq of a melanoma brain metastasis patient found PDCD4 to be expressed on CD8 Tcells, NK cells, B cells and mast cells, associated with cytotoxicity (Gzm expression), suggesting a role to potentiate immune response (67). Other very interesting tools are predictive studies of brain metastatic tumors to classify patients into potential good or bad responders to immunotherapy. This approach is able to determine specific molecules as targets for adjuvant immunotherapies according to the immune profile, which allows to narrow down candidates to specific biomarkers. For instance, the expression of CD74 in the microenvironment of brain metastases fulfilled the in silico criteria (68). Uncovering functional and dysfunctional CD8+ T cell activation states in brain tumors is key to establish more accurate immune signatures to stratify patients. Transposase-accessible chromatin sequencing (ATAC-seq) and RNA-seq could be applied to preclinical models of brain metastasis and human data to achieve this goal, as it has been done for hepatocarcinoma and melanoma (69). Other important aspect to consider is the reprogramming of the brain immune landscape by therapy. i.e. TMZ in primary tumors (60) and WBRT in brain metastasis (70).
Finally, due to the limited availability of brain tissue from patients, profiling the mutational landscape and evolutionary patterns of tumor and microenvironment using non-invasive biopsies, could be key to establish predictive biomarkers of therapeutic response. In this sense, pioneer studies using CSF as a relative non-invasive surrogate to be processed by single-cell techniques could help to define the heterogeneity of the immune microenvironment and its link to clinically meaningful correlations (34, 35). Analysis of this liquid biopsy in patients with positive and negative local responses to immunotherapy has started to be explored (61). Moreover, considering the important role of meningeal lymphatics in regulating brain tumor immunity, other plausible source of cancer-derived material is the regional lymphatic drainage (71), although in-depth analysis is needed to characterize the immune landscape in this liquid biopsy.
Discussion
In conclusion, the data reviewed lays a firm foundation for considering vulnerabilities generated in the brain metastasis microenvironment relevant to predict and improve responses to immune based therapies that are effective only in a limited percentage of patients, especially when asymptomatic (72, 73). Overall, we consider a key aspect to embrace the emerging complexity and to dissect functionally relevant hubs within the local microenvironment, providing the avenues to transform the clinical management of brain metastasis patients within the years to come.
Data Availability Statement
The original contributions presented in the study are included in the article/supplementary material. Further inquiries can be directed to the corresponding author.
Author Contributions
LÁ-E, AP-A, MV, and NP conceptualized and wrote the manuscript. All authors contributed to the article and approved the submitted version.
Funding
Research in the Brain Metastasis Group is supported by MINECO (SAF2017-89643-R) (MV), Fundació La Marató de TV3 (141) (MV), Fundación Ramón Areces (CIVP19S8163) (MV), Worldwide Cancer Research (19–0177) (MV), H2020-FETOPEN (828972) (MV), Cancer Research Institute (Clinic and Laboratory Integration Program CRI Award 2018 (54545) (MV), AECC (Coordinated Translational Groups 2017 (GCTRA16015SEOA) (MV), LAB AECC 2019 (LABAE19002VALI) (MV), ERC CoG (864759) (MV), La Caixa INPhINIT Fellowship (LCF/BQ/DI19/11730044) (AP-A), MINECO-Severo Ochoa PhD Fellowship (BES-2017-081995) (LA-E), AECC Postdoctoral Fellowship (POSTD19016PRIE) (NP). MV is an EMBO YIP investigator (4053).
Conflict of Interest
The authors declare that the research was conducted in the absence of any commercial or financial relationships that could be construed as a potential conflict of interest.
Publisher’s Note
All claims expressed in this article are solely those of the authors and do not necessarily represent those of their affiliated organizations, or those of the publisher, the editors and the reviewers. Any product that may be evaluated in this article, or claim that may be made by its manufacturer, is not guaranteed or endorsed by the publisher.
Acknowledgments
The authors want to thank the members of the Brain Metastasis Group for their comments on the manuscript.
References
1. Boire A, Brastianos PK, Garzia L, Valiente M. Brain Metastasis. Nat Rev Cancer (2020) 20:4–11. doi: 10.1038/s41568-019-0220-y
2. Zeisel A, Muñoz-Manchado AB, Codeluppi S, Lönnerberg P, La Manno G, Juréus A, et al. Brain Structure. Cell Types in the Mouse Cortex and Hippocampus Revealed by Single-Cell RNA-Seq. Science (2015) 347:1138–42. doi: 10.1126/science.aaa1934
3. Llorens-Bobadilla E, Zhao S, Baser A, Saiz-Castro G, Zwadlo K, Martin-Villalba A. Single-Cell Transcriptomics Reveals a Population of Dormant Neural Stem Cells That Become Activated Upon Brain Injury. Cell Stem Cell (2015) 17:329–40. doi: 10.1016/j.stem.2015.07.002
4. Prasad JA, Balwani AH, Johnson EC, Miano JD, Sampathkumar V, De Andrade V, et al. A Three-Dimensional Thalamocortical Dataset for Characterizing Brain Heterogeneity. Sci Data (2020) 7:358. doi: 10.1038/s41597-020-00692-y
5. Lake BB, Chen S, Sos BC, Fan J, Kaeser GE, Yung YC, et al. Integrative Single-Cell Analysis of Transcriptional and Epigenetic States in the Human Adult Brain. Nat Biotechnol (2018) 36:70–80. doi: 10.1038/nbt.4038
6. Pasqual G, Chudnovskiy A, Tas JMJ, Agudelo M, Schweitzer LD, Cui A, et al. Monitoring T Cell-Dendritic Cell Interactions In Vivo by Intercellular Enzymatic Labelling. Nature (2018) 553:496–500. doi: 10.1038/nature25442
7. Caruso FP, Garofano L, D’Angelo F, Yu K, Tang F, Yuan J, et al. A Map of Tumor-Host Interactions in Glioma at Single-Cell Resolution. Gigascience (2020) 9(10). doi: 10.1093/gigascience/giaa109
8. Clark IC, Gutiérrez-Vázquez C, Wheeler MA, Li Z, Rothhammer V, Linnerbauer M, et al. Barcoded Viral Tracing of Single-Cell Interactions in Central Nervous System Inflammation. Science (2021) 372(6540). doi: 10.1126/science.abf1230
9. Batiuk MY, Martirosyan A, Wahis J, de Vin F, Marneffe C, Kusserow C, et al. Identification of Region-Specific Astrocyte Subtypes at Single Cell Resolution. Nat Commun (2020) 11:1220. doi: 10.1038/s41467-019-14198-8
10. Goldmann T, Wieghofer P, Jordão MJC, Prutek F, Hagemeyer N, Frenzel K, et al. Origin, Fate and Dynamics of Macrophages at Central Nervous System Interfaces. Nat Immunol (2016) 17:797–805. doi: 10.1038/ni.3423
11. Mrdjen D, Pavlovic A, Hartmann FJ, Schreiner B, Utz SG, Leung BP, et al. High-Dimensional Single-Cell Mapping of Central Nervous System Immune Cells Reveals Distinct Myeloid Subsets in Health, Aging, and Disease. Immunity (2018) 48:380–95.e6. doi: 10.1016/j.immuni.2018.01.011
12. Jordão MJC, Sankowski R, Brendecke SM, Sagar, Locatelli G, Tai Y-H, et al. Single-Cell Profiling Identifies Myeloid Cell Subsets With Distinct Fates During Neuroinflammation. Science (2019) 363(6425). doi: 10.1126/science.aat7554
13. Van Hove H, Martens L, Scheyltjens I, De Vlaminck K, Pombo Antunes AR, De Prijck S, et al. A Single-Cell Atlas of Mouse Brain Macrophages Reveals Unique Transcriptional Identities Shaped by Ontogeny and Tissue Environment. Nat Neurosci (2019) 22:1021–35. doi: 10.1038/s41593-019-0393-4
14. Hammond TR, Dufort C, Dissing-Olesen L, Giera S, Young A, Wysoker A, et al. Single-Cell RNA Sequencing of Microglia Throughout the Mouse Lifespan and in the Injured Brain Reveals Complex Cell-State Changes. Immunity (2019) 50:253–71.e6. doi: 10.1016/j.immuni.2018.11.004
15. Masuda T, Sankowski R, Staszewski O, Böttcher C, Amann L, Sagar, et al. Spatial and Temporal Heterogeneity of Mouse and Human Microglia at Single-Cell Resolution. Nature (2019) 566:388–92. doi: 10.1038/s41586-019-0924-x
16. Li Q, Cheng Z, Zhou L, Darmanis S, Neff NF, Okamoto J, et al. Developmental Heterogeneity of Microglia and Brain Myeloid Cells Revealed by Deep Single-Cell RNA Sequencing. Neuron (2019) 101:207–23.e10. doi: 10.1016/j.neuron.2018.12.006
17. Keren-Shaul H, Spinrad A, Weiner A, Matcovitch-Natan O, Dvir-Szternfeld R, Ulland TK, et al. A Unique Microglia Type Associated With Restricting Development of Alzheimer’s Disease. Cell (2017) 169:1276–90.e17. doi: 10.1016/j.cell.2017.05.018
18. Mathys H, Davila-Velderrain J, Peng Z, Gao F, Mohammadi S, Young JZ, et al. Single-Cell Transcriptomic Analysis of Alzheimer’s Disease. Nature (2019) 570:332–7. doi: 10.1038/s41586-019-1195-2
19. Krasemann S, Madore C, Cialic R, Baufeld C, Calcagno N, El Fatimy R, et al. The TREM2-APOE Pathway Drives the Transcriptional Phenotype of Dysfunctional Microglia in Neurodegenerative Diseases. Immunity (2017) 47:566–81.e9. doi: 10.1016/j.immuni.2017.08.008
20. Ajami B, Samusik N, Wieghofer P, Ho PP, Crotti A, Bjornson Z, et al. Single-Cell Mass Cytometry Reveals Distinct Populations of Brain Myeloid Cells in Mouse Neuroinflammation and Neurodegeneration Models. Nat Neurosci (2018) 21:541–51. doi: 10.1038/s41593-018-0100-x
21. Gosselin D, Skola D, Coufal NG, Holtman IR, Schlachetzki JCM, Sajti E, et al. An Environment-Dependent Transcriptional Network Specifies Human Microglia Identity. Science (2017) 356(6344). doi: 10.1126/science.aal3222
22. Böttcher C, Schlickeiser S, Sneeboer MAM, Kunkel D, Knop A, Paza E, et al. Human Microglia Regional Heterogeneity and Phenotypes Determined by Multiplexed Single-Cell Mass Cytometry. Nat Neurosci (2019) 22:78–90. doi: 10.1038/s41593-018-0290-2
23. Sankowski R, Böttcher C, Masuda T, Geirsdottir L, Sagar, Sindram E, et al. Mapping Microglia States in the Human Brain Through the Integration of High-Dimensional Techniques. Nat Neurosci (2019) 22:2098–110. doi: 10.1038/s41593-019-0532-y
24. Yu K, Hu Y, Wu F, Guo Q, Qian Z, Hu W, et al. Surveying Brain Tumor Heterogeneity by Single-Cell RNA Sequencing of Multi-Sector Biopsies. Natl Sci Rev (2020) 7(8):1306–18. doi: 10.1093/nsr/nwaa099
25. Ochocka N, Segit P, Walentynowicz KA, Wojnicki K, Cyranowski S, Swatler J, et al. Single-Cell RNA Sequencing Reveals Functional Heterogeneity of Glioma-Associated Brain Macrophages. Nat Commun (2021) 12:1151. doi: 10.1038/s41467-021-21407-w
26. Müller S, Kohanbash G, Liu SJ, Alvarado B, Carrera D, Bhaduri A, et al. Single-Cell Profiling of Human Gliomas Reveals Macrophage Ontogeny as a Basis for Regional Differences in Macrophage Activation in the Tumor Microenvironment. Genome Biol (2017) 18:234. doi: 10.1186/s13059-017-1362-4
27. Darmanis S, Sloan SA, Croote D, Mignardi M, Chernikova S, Samghababi P, et al. Single-Cell RNA-Seq Analysis of Infiltrating Neoplastic Cells at the Migrating Front of Human Glioblastoma. Cell Rep (2017) 21:1399–410. doi: 10.1016/j.celrep.2017.10.030
28. Friebel E, Kapolou K, Unger S, Núñez NG, Utz S, Rushing EJ, et al. Single-Cell Mapping of Human Brain Cancer Reveals Tumor-Specific Instruction of Tissue-Invading Leukocytes. Cell (2020) 181:1626–42.e20. doi: 10.1016/j.cell.2020.04.055
29. Guldner IH, Wang Q, Yang L, Golomb SM, Zhao Z, Lopez JA, et al. CNS-Native Myeloid Cells Drive Immune Suppression in the Brain Metastatic Niche Through Cxcl10. Cell (2020) 183:1234–48.e25. doi: 10.1016/j.cell.2020.09.064
30. Korin B, Ben-Shaanan TL, Schiller M, Dubovik T, Azulay-Debby H, Boshnak NT, et al. High-Dimensional, Single-Cell Characterization of the Brain’s Immune Compartment. Nat Neurosci (2017) 20:1300–9. doi: 10.1038/nn.4610
31. Golomb SM, Guldner IH, Zhao A, Wang Q, Palakurthi B, Aleksandrovic EA, et al. Multi-Modal Single-Cell Analysis Reveals Brain Immune Landscape Plasticity During Aging and Gut Microbiota Dysbiosis. Cell Rep (2020) 33:108438. doi: 10.1016/j.celrep.2020.108438
32. Close HJ, Stead LF, Nsengimana J, Reilly KA, Droop A, Wurdak H, et al. Expression Profiling of Single Cells and Patient Cohorts Identifies Multiple Immunosuppressive Pathways and an Altered NK Cell Phenotype in Glioblastoma. Clin Exp Immunol (2020) 200:33–44. doi: 10.1111/cei.13403
33. Schafflick D, Xu CA, Hartlehnert M, Cole M, Schulte-Mecklenbeck A, Lautwein T, et al. Integrated Single Cell Analysis of Blood and Cerebrospinal Fluid Leukocytes in Multiple Sclerosis. Nat Commun (2020) 11:247. doi: 10.1038/s41467-019-14118-w
34. Rubio-Perez C, Planas-Rigol E, Trincado JL, Bonfill-Teixidor E, Arias A, Marchese D, et al. Immune Cell Profiling of the Cerebrospinal Fluid Enables the Characterization of the Brain Metastasis Microenvironment. Nat Commun (2021) 12:1503. doi: 10.1038/s41467-021-21789-x
35. Miller AM, Shah RH, Pentsova EI, Pourmaleki M, Briggs S, Distefano N, et al. Tracking Tumour Evolution in Glioma Through Liquid Biopsies of Cerebrospinal Fluid. Nature (2019) 565:654–8. doi: 10.1038/s41586-019-0882-3
36. Bayraktar OA, Bartels T, Holmqvist S, Kleshchevnikov V, Martirosyan A, Polioudakis D, et al. Astrocyte Layers in the Mammalian Cerebral Cortex Revealed by a Single-Cell in Situ Transcriptomic Map. Nat Neurosci (2020) 23:500–9. doi: 10.1038/s41593-020-0602-1
37. Boisvert MM, Erikson GA, Shokhirev MN, Allen NJ. The Aging Astrocyte Transcriptome From Multiple Regions of the Mouse Brain. Cell Rep (2018) 22:269–85. doi: 10.1016/j.celrep.2017.12.039
38. Al-Dalahmah O, Sosunov AA, Shaik A, Ofori K, Liu Y, Vonsattel JP, et al. Single-Nucleus RNA-Seq Identifies Huntington Disease Astrocyte States. Acta Neuropathol Commun (2020) 8:19. doi: 10.1186/s40478-020-0880-6
39. Lau S-F, Cao H, Fu AKY, Ip NY. Single-Nucleus Transcriptome Analysis Reveals Dysregulation of Angiogenic Endothelial Cells and Neuroprotective Glia in Alzheimer’s Disease. Proc Natl Acad Sci U S A (2020) 117:25800–9. doi: 10.1073/pnas.2008762117
40. Leng K, Li E, Eser R, Piergies A, Sit R, Tan M, et al. Molecular Characterization of Selectively Vulnerable Neurons in Alzheimer’s Disease. Nat Neurosci (2021) 24:276–87. doi: 10.1038/s41593-020-00764-7
41. Rothhammer V, Borucki DM, Tjon EC, Takenaka MC, Chao C-C, Ardura-Fabregat A, et al. Microglial Control of Astrocytes in Response to Microbial Metabolites. Nature (2018) 557:724–8. doi: 10.1038/s41586-018-0119-x
42. Sanmarco LM, Wheeler MA, Gutiérrez-Vázquez C, Polonio CM, Linnerbauer M, Pinho-Ribeiro FA, et al. Gut-Licensed Ifnγ+ NK Cells Drive LAMP1+TRAIL+ Anti-Inflammatory Astrocytes. Nature (2021) 590:473–9. doi: 10.1038/s41586-020-03116-4
43. Zhang Y, Sloan SA, Clarke LE, Caneda C, Plaza CA, Blumenthal PD, et al. Purification and Characterization of Progenitor and Mature Human Astrocytes Reveals Transcriptional and Functional Differences With Mouse. Neuron (2016) 89:37–53. doi: 10.1016/j.neuron.2015.11.013
44. Henrik Heiland D, Ravi VM, Behringer SP, Frenking JH, Wurm J, Joseph K, et al. Tumor-Associated Reactive Astrocytes Aid the Evolution of Immunosuppressive Environment in Glioblastoma. Nat Commun (2019) 10:2541. doi: 10.1038/s41467-019-10493-6
45. Priego N, Zhu L, Monteiro C, Mulders M, Wasilewski D, Bindeman W, et al. STAT3 Labels a Subpopulation of Reactive Astrocytes Required for Brain Metastasis. Nat Med (2018) 24:1024–35. doi: 10.1038/s41591-018-0044-4
46. Vanlandewijck M, He L, Mäe MA, Andrae J, Ando K, Del Gaudio F, et al. A Molecular Atlas of Cell Types and Zonation in the Brain Vasculature. Nature (2018) 554:475–80. doi: 10.1038/nature25739
47. Seaman S, Stevens J, Yang MY, Logsdon D, Graff-Cherry C, St Croix B. Genes That Distinguish Physiological and Pathological Angiogenesis. Cancer Cell (2007) 11:539–54. doi: 10.1016/j.ccr.2007.04.017
48. Carlson JC, Cantu Gutierrez M, Lozzi B, Huang-Hobbs E, Turner WD, Tepe B, et al. Identification of Diverse Tumor Endothelial Cell Populations in Malignant Glioma. Neuro Oncol (2021) 23:932–44. doi: 10.1093/neuonc/noaa297
49. Ebert LM, Yu W, Gargett T, Toubia J, Kollis PM, Tea MN, et al. Endothelial, Pericyte and Tumor Cell Expression in Glioblastoma Identifies Fibroblast Activation Protein (FAP) as an Excellent Target for Immunotherapy. Clin Transl Immunol (2020) 9:e1191. doi: 10.1002/cti2.1191
50. Dankner M, Caron M, Al-Saadi T, Yu W, Ouellet V, Ezzeddine R, et al. Invasive Growth Associated With Cold-Inducible RNA-Binding Protein Expression Drives Recurrence of Surgically Resected Brain Metastases. Neuro Oncol (2021) noab002. doi: 10.1093/neuonc/noab002
51. Zou A, Ramanathan S, Dale RC, Brilot F. Single-Cell Approaches to Investigate B Cells and Antibodies in Autoimmune Neurological Disorders. Cell Mol Immunol (2021) 18:294–306. doi: 10.1038/s41423-020-0510-z
52. Arneson D, Zhang G, Ying Z, Zhuang Y, Byun HR, Ahn IS, et al. Single Cell Molecular Alterations Reveal Target Cells and Pathways of Concussive Brain Injury. Nat Commun (2018) 9:3894. doi: 10.1038/s41467-018-06222-0
53. Capurro A, Bodea L-G, Schaefer P, Luthi-Carter R, Perreau VM. Computational Deconvolution of Genome Wide Expression Data From Parkinson’s and Huntington’s Disease Brain Tissues Using Population-Specific Expression Analysis. Front Neurosci (2014) 8:441. doi: 10.3389/fnins.2014.00441
54. Skene NG, Grant SGN. Identification of Vulnerable Cell Types in Major Brain Disorders Using Single Cell Transcriptomes and Expression Weighted Cell Type Enrichment. Front Neurosci (2016) 10:16. doi: 10.3389/fnins.2016.00016
55. Weng Q, Wang J, Wang J, He D, Cheng Z, Zhang F, et al. Single-Cell Transcriptomics Uncovers Glial Progenitor Diversity and Cell Fate Determinants During Development and Gliomagenesis. Cell Stem Cell (2019) 24:707–23.e8. doi: 10.1016/j.stem.2019.03.006
56. Hu F, Dzaye OD, Hahn A, Yu Y, Scavetta RJ, Dittmar G, et al. Glioma-Derived Versican Promotes Tumor Expansion via Glioma-Associated Microglial/Macrophages Toll-Like Receptor 2 Signaling. Neuro Oncol (2015) 17:200–10. doi: 10.1093/neuonc/nou324
57. Boles KS, Barchet W, Diacovo T, Cella M, Colonna M. The Tumor Suppressor TSLC1/NECL-2 Triggers NK-Cell and CD8+ T-Cell Responses Through the Cell-Surface Receptor CRTAM. Blood (2005) 106:779–86. doi: 10.1182/blood-2005-02-0817
58. Gargini R, Segura-Collar B, Herránz B, García-Escudero V, Romero-Bravo A, Núñez FJ, et al. The IDH-TAU-EGFR Triad Defines the Neovascular Landscape of Diffuse Gliomas. Sci Transl Med (2020) 12(527). doi: 10.1126/scitranslmed.aax1501
59. Miroshnikova YA, Mouw JK, Barnes JM, Pickup MW, Lakins JN, Kim Y, et al. Tissue Mechanics Promote IDH1-Dependent HIF1α-Tenascin C Feedback to Regulate Glioblastoma Aggression. Nat Cell Biol (2016) 18:1336–45. doi: 10.1038/ncb3429
60. Kohanbash G, Carrera DA, Shrivastav S, Ahn BJ, Jahan N, Mazor T, et al. Isocitrate Dehydrogenase Mutations Suppress STAT1 and CD8+ T Cell Accumulation in Gliomas. J Clin Invest (2017) 127:1425–37. doi: 10.1172/JCI90644
61. Klemm F, Maas RR, Bowman RL, Kornete M, Soukup K, Nassiri S, et al. Interrogation of the Microenvironmental Landscape in Brain Tumors Reveals Disease-Specific Alterations of Immune Cells. Cell (2020) 181:1643–60.e17. doi: 10.1016/j.cell.2020.05.007
62. Wang Q, Hu B, Hu X, Kim H, Squatrito M, Scarpace L, et al. Tumor Evolution of Glioma-Intrinsic Gene Expression Subtypes Associates With Immunological Changes in the Microenvironment. Cancer Cell (2017) 32:42–56.e6. doi: 10.1016/j.ccell.2017.06.003
63. Smalley I, Chen Z, Phadke M, Li J, Yu X, Wyatt C, et al. Single-Cell Characterization of the Immune Microenvironment of Melanoma Brain and Leptomeningeal Metastases. Clin Cancer Res (2021) 27:4109–25. doi: 10.1158/1078-0432.CCR-21-1694
64. Domingues P, González-Tablas M, Otero Á, Pascual D, Miranda D, Ruiz L, et al. Tumor Infiltrating Immune Cells in Gliomas and Meningiomas. Brain Behav Immun (2016) 53:1–15. doi: 10.1016/j.bbi.2015.07.019
65. Jerby-Arnon L, Shah P, Cuoco MS, Rodman C, Su M-J, Melms JC, et al. A Cancer Cell Program Promotes T Cell Exclusion and Resistance to Checkpoint Blockade. Cell (2018) 175:984–97.e24. doi: 10.1016/j.cell.2018.09.006
66. Zhao J, Chen AX, Gartrell RD, Silverman AM, Aparicio L, Chu T, et al. Immune and Genomic Correlates of Response to Anti-PD-1 Immunotherapy in Glioblastoma. Nat Med (2019) 25:462–9. doi: 10.1038/s41591-019-0349-y
67. Tran TT, Rane CK, Zito CR, Weiss SA, Jessel S, Lucca L, et al. Clinical Significance of PDCD4 in Melanoma by Subcellular Expression and in Tumor-Associated Immune Cells. Cancers (Basel) (2021) 13(5):1049. doi: 10.3390/cancers13051049
68. García-Mulero S, Alonso MH, Pardo J, Santos C, Sanjuan X, Salazar R, et al. Lung Metastases Share Common Immune Features Regardless of Primary Tumor Origin. J Immunother Cancer (2020) 8(1):e000491. doi: 10.1136/jitc-2019-000491
69. Pritykin Y, van der Veeken J, Pine AR, Zhong Y, Sahin M, Mazutis L, et al. A Unified Atlas of CD8 T Cell Dysfunctional States in Cancer and Infection. Mol Cell (2021) 81:2477–93.e10. doi: 10.1016/j.molcel.2021.03.045
70. Niesel K, Schulz M, Anthes J, Alekseeva T, Macas J, Salamero-Boix A, et al. The Immune Suppressive Microenvironment Affects Efficacy of Radio-Immunotherapy in Brain Metastasis. EMBO Mol Med (2021) 13:e13412. doi: 10.15252/emmm.202013412
71. García-Silva S, Benito-Martín A, Sánchez-Redondo S, Hernández-Barranco A, Ximénez-Embún P, Nogués L, et al. Use of Extracellular Vesicles From Lymphatic Drainage as Surrogate Markers of Melanoma Progression and BRAFV600E Mutation. J Exp Med (2019) 216:1061–70. doi: 10.1084/jem.20181522
72. Tawbi HA, Forsyth PA, Algazi A, Hamid O, Hodi FS, Moschos SJ, et al. Combined Nivolumab and Ipilimumab in Melanoma Metastatic to the Brain. N Engl J Med (2018) 379:722–30. doi: 10.1056/NEJMoa1805453
73. Goldberg SB, Schalper KA, Gettinger SN, Mahajan A, Herbst RS, Chiang AC, et al. Pembrolizumab for Management of Patients With NSCLC and Brain Metastases: Long-Term Results and Biomarker Analysis From a non-Randomised, Open-Label, Phase 2 Trial. Lancet Oncol (2020) 21:655–63. doi: 10.1016/S1470-2045(20)30111-X
Keywords: brain, brain metastasis, microenvironment, heterogeneity, single-cell analysis
Citation: Álvaro-Espinosa L, de Pablos-Aragoneses A, Valiente M and Priego N (2021) Brain Microenvironment Heterogeneity: Potential Value for Brain Tumors. Front. Oncol. 11:714428. doi: 10.3389/fonc.2021.714428
Received: 25 May 2021; Accepted: 16 August 2021;
Published: 01 September 2021.
Edited by:
Frits Thorsen, University of Bergen, NorwayReviewed by:
Monika Vishnoi, Houston Methodist Research Institute, United StatesSivan Izraely, Tel Aviv University, Israel
Copyright © 2021 Álvaro-Espinosa, de Pablos-Aragoneses, Valiente and Priego. This is an open-access article distributed under the terms of the Creative Commons Attribution License (CC BY). The use, distribution or reproduction in other forums is permitted, provided the original author(s) and the copyright owner(s) are credited and that the original publication in this journal is cited, in accordance with accepted academic practice. No use, distribution or reproduction is permitted which does not comply with these terms.
*Correspondence: Neibla Priego, bnByaWVnb0BjbmlvLmVz