- 1Department of Radiation Oncology, Fudan University Shanghai Cancer Center, Shanghai, China
- 2Department of Oncology, Shanghai Medical College, Fudan University, Shanghai, China
- 3Department of Orthopedics, TongRen Hospital, School of Medicine Shanghai Jiao Tong University, Shanghai, China
Brain metastasis of non-small cell lung cancer is associated with poor survival outcomes and poses rough clinical challenges. At the era of immunotherapy, it is urgent to perform a comprehensive study uncovering the specific immune microenvironment of brain metastases of NSCLC. The immune microenvironment of brain is distinctly different from microenvironments of extracranial lesions. In this review, we summarized the process of brain metastases across the barrier and revealed that brain is not completely immune-privileged. We comprehensively described the specific components of immune microenvironment for brain metastases such as central nervous system-derived antigen-presenting cells, microglia and astrocytes. Besides, the difference of immune microenvironment between brain metastases and primary foci of lung was particularly demonstrated.
Background
Brain metastases are the most common type intracranial tumors, which are commonly metastasized from lung cancer (1). Approximately 50% of brain metastases originate from non-small cell lung cancer (NSCLC). During the progression of NSCLC, about one third of patients may develop brain metastases (1, 2). Current therapeutic strategies for brain metastases of NSCLC are largely limited, and the prognosis is relatively poor because of the specific anatomic and physiologic features of the central nervous system (CNS). Moreover, comprehensive researches on brain metastases of NSCLC are significantly lacked. Immunotherapy has been rapidly adopted for the treatment of NSCLC (3, 4). Recent small-scale clinical studies have shown that some of NSCLC patients can be benefited from immune checkpoint inhibitors (5). However, due to genetic differences between brain metastases and primary tumors, as well as the difference in tumor microenvironment, the response of intracranial and extracranial lesions to systemic immunotherapy may differ a lot. In addition, the difficulty in collecting intracranial tissues increases the challenge in clarifying the molecular mechanism of brain metastases (6). Therefore, it is urgent to carry out an in-depth exploration on the immune microenvironment of brain metastases, aiming to guide clinical treatment.
The Process of Brain Metastases
Cancer metastasis is one of the most significant characteristics of malignant tumors, which is a multistep cell-biological process, called the invasion-metastasis cascade (7). During metastatic progression, tumor cells detach from their primary lesions (locally invasive and intravasate), translocate systemically (survive in the circulation, arrest at a distant tissue and extravasate), and finally form the metastases in the foreign microenvironment of distant organs (Figure 1) (7). Although the circulating tumor cells (CTCs) in the hematogenous circulation could disseminate to a variety of secondary loci, it is noticed that the metastases of a certain type of carcinoma could only form in particular target organs (7). In 1889, Stephen Paget proposed the well-known “seed-and-soil” hypothesis of metastases that metastases only develop at those organ sites (“soils”), in which the newly “seeded” metastatic tumor cells are suitably growing (8). The nervous system is one of the most preferential and frequent metastatic sites of NSCLC (9). CTCs infiltrate through the blood circulation at brain capillaries with a slower flow rate, where they interact with microvascular endothelial cells and secrete cytokines. Then, tumor cells with a strong invasiveness ability circulate through the bloodstream, the brain lymphatics or the CSF, and survive and thrive in the parenchymal, leptomeningeal, or epidural areas, thus leading to brain metastases.
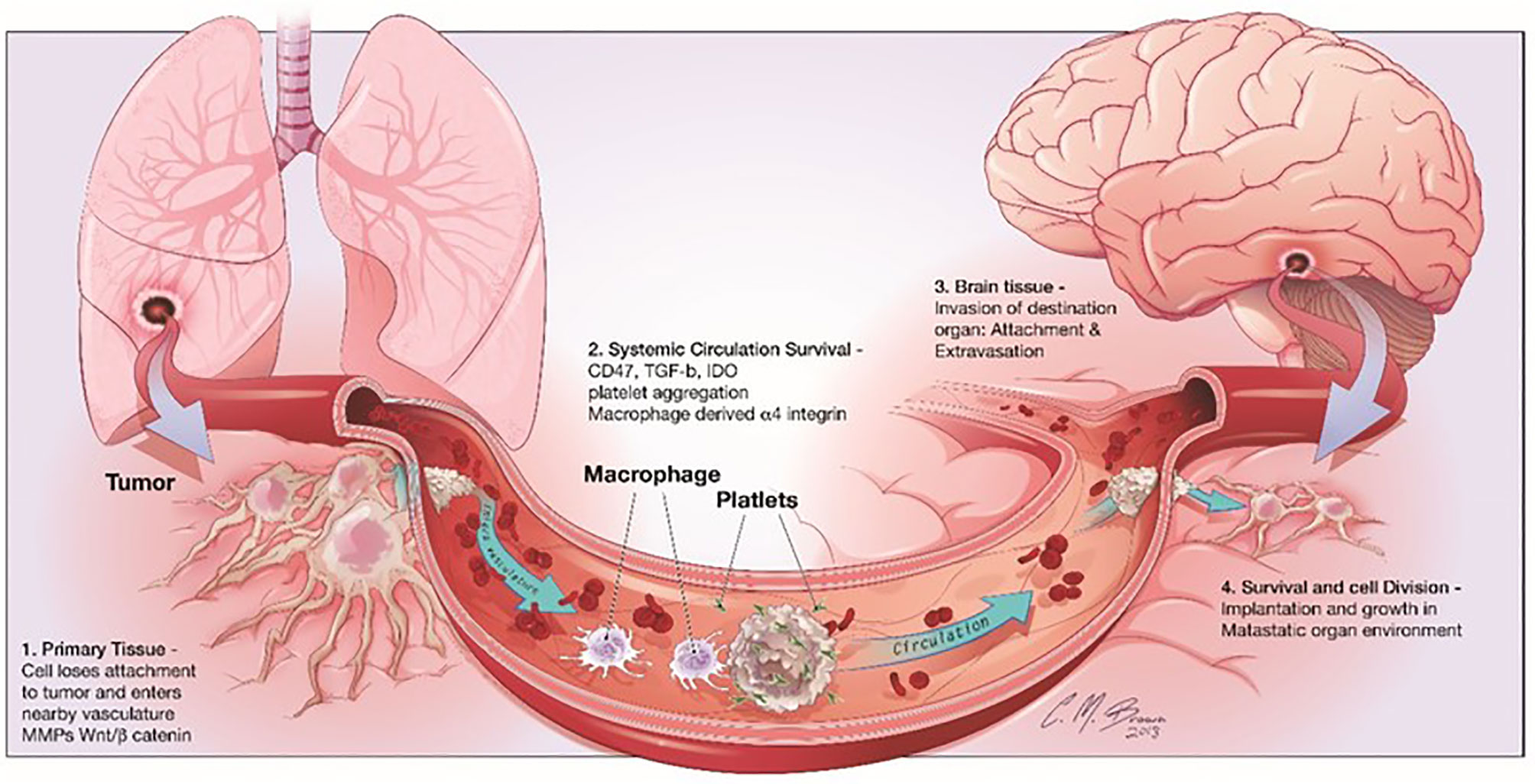
Figure 1 Steps involved in brain metastasis. Brain metastasis cascade involves four major steps: 1) Detachment of the metastatic cell from the primary cancer, 2) Survival in systemic circulation, 3) Invasion in the brain parenchyma and 4) Survival in the CNS microenvironment.
The Molecular Mechanism of Brain Metastases of NSCLC
The molecular mechanisms underlying brain metastases of NSCLC remain largely unknown due to the lack of in vitro models that simulate the complex structure and microenvironment of CNS. A previous study established a multi-organ microfluidic bionic chip platform to recapitulate the process of brain metastases. It is found that AKR1B10 can promote the extravasation of lung cancer cells through the blood–brain barrier (BBB) and then induce brain metastases (10). Through comparing genomic sequencing data of a large number of brain metastases and primary lung adenocarcinomas, three novel metastatic drivers with significantly higher amplification frequencies are identified, including MYC, YAP1, and MMP13. Overexpression of them increases the incidence of brain metastases (11, 12). Besides, in vitro and in vivo experiments demonstrated that cell adhesion molecule 2 (CADMA2), long noncoding RNA MALAT1, and microRNA-330-3p promote the development of brain metastases by inducing epithelial-mesenchymal transition (EMT) in NSCLC (13–15). The transmembrane cell adhesion protein ADAM9 is able to promote lung cancer metastases to the brain by a plasminogen activator-based pathway (16). Moreover, activated leukocyte cell adhesion molecule (ALCAM), the tubulin-detyrosinating activity of VASH1, lysophosphatidylcholine acyltransferase 1 (LPCAT1), and the TAZ-AXL-ABL2 feed-forward signaling axis are also essential for the formation of brain metastases from NSCLC (17–20). As for immune-related mechanism, tumor-induced peripheral immunosuppression might promote brain metastases in patients with NSCLC (21). Patients with brain metastatic lung carcinoma exhibit a profound systemic immunosuppression with increased myeloid-derived suppressor cells, regulatory T cell populations, peripheral monocyte PD-L1, myeloid-derived suppressor cells (MDSCs), and regulatory T cells compared to early stage pre-metastatic patients and healthy controls, accompanied by less reactive T cells and worse survival (21).
Incompletely Immune Privileged CNS
There are three main barriers in CNS, including the blood–brain barrier (BBB), blood–cerebrospinal fluid (BCSFB) barrier, and the blood– tumor barrier (BTB) (22). In the normal brain, BBB and BCSFB are the initial gatekeepers of CNS, which are formed by the tight junctions of the endothelial cells of capillaries and connective tissues and responsible for protecting CNS from a massive inflammation (brain edema) (23). CTCs could cross the barrier by the transendothelial migration. When the micrometastases (<1 mm) are formed, the BBB still functions normally to protect the micrometastases escaping from the effective anti-cancer drugs, such as water-soluble agents and macromolecules (23, 24). The formation of metastatic tumor in the brain leads to neo-angiogenesis, vascular remodeling, and changes of surface molecules, such as overexpression of the pericyte protein desmin and deficiency of physiological TJ protein (25–27). The changed neurovascular-tumor unit is known as BTB, which is featured by increased permeability, promoting tumor growth, and changing the delivery of anti-cancer agents. Meanwhile, dynamic angiogenesis differs from lesions and regions of the same lesion during metastatic progression (24). As a result, there is a significant heterogeneous permeability in brain metastases, leading to a non-uniform and suboptimal drug distribution and thus promotes drug resistance (28).
CNS is not completely immune-privileged. In the last century, the CNS has been considered as the immune-privileged organ because of the existence of BBB and BCSFB, where immune cells in the blood circulation system are blocked. However, along with the explorations on lymphatic system in the brain and lymphatic ducts of meninges, this conception has been overthrown (29). Experimental data also showed that tumor-infiltrating T lymphocytes and other blood-borne immune cells are observed in the brain metastases (30). Besides, a connection between the blood-borne immune cells and immune components in the brain exists. The specific immune cells of the CNS are able to pass through the endolymphatic system into the cerebrospinal fluid, which further infiltrate to the olfactory bulb, olfactory nerve, cribriform plate, nasal mucosa, and finally reach the deep cervical lymph nodes. Although the BBB limits the penetration of immune cells, they can pass through the tapetum lucidum and lymphatic channels of cerebrospinal fluid (Figure 2) (31). Besides, macrophages and CD4-positive memory T cells are residents in the ventricle, pia mater, and perivascular space, which are important for immune monitoring of the CNS (31).
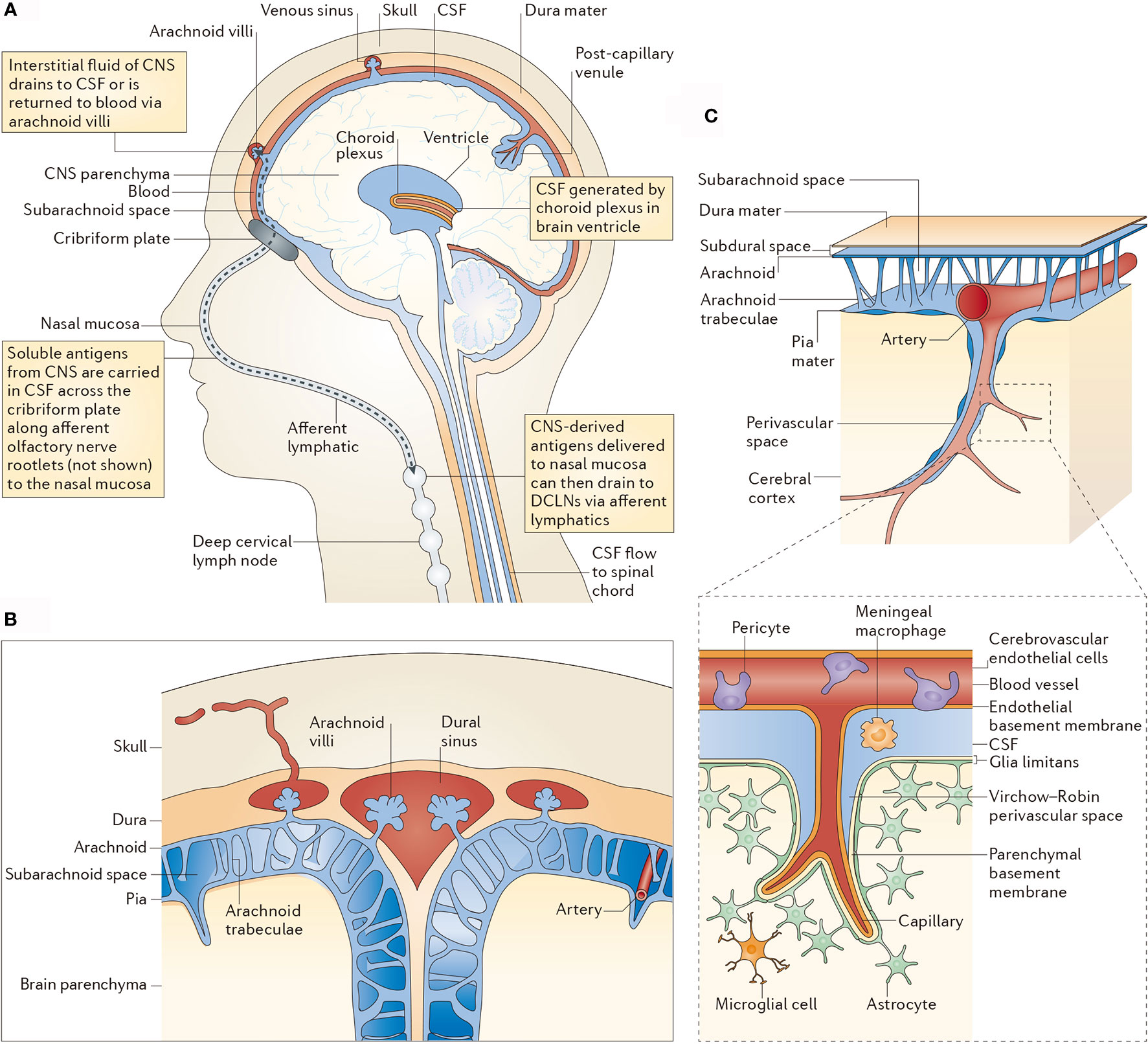
Figure 2 CSF-mediated drainage of interstitial fluid and CNS antigens to deep cervical lymph nodes. (A) A human head in midline sagittal section, showing revelant anatomical structures [namely the ventricle, choroid plexus, central nervous system (CNS) parenchyma, lymphatics and deep cervical lymph nodes (DCLNs)] in schematic form. (B) Arachnoid granulations in relation to the subarachnoid space and brain parenchyma. (C) Subpial vasculature in relation to subarachnoid space and brain parenchyma, indicating the anatomy discussed in the main text. The inset shows the cellular components of cerebral capillaries, the glia limitans and the basement membranes in relation to the perivascular space. CSF, cerebrospinal fluid.
Specific Components of Immune Microenvironment For Brain Metastases
CNS-Derived Antigen-Presenting Cells
According to previous studies, CD11c-expressing cells are found as the residents in the juxta vascular parenchyma (32). They are not only recruited from the blood to parenchyma but also derived from an intraneural precursor in situ (32). Apart from the CD11c-expressing cells, perivascular and ventricular macrophages, as well as epiplexus cells of the choroid plexus and meninges constitute a main population of antigen-presenting cells (APCs) (CNS-derived APCs) (31). Although they are located outside the parenchyma, they can sample the contents of tumor cells in the parenchyma through the circulating CSF. They express MHC-II molecules, co-stimulatory molecules, and can present antigens to elicit priming and proliferation of CD4+ T cells to activate the adaptive immune response (32).
Microglia
Microglia is another main population of APCs in CNS, playing a vital role in the immune response of CNS. It is the only one type of immune cells existed in healthy CNS parenchyma and unique in CNS. Microglial cells can respond rapidly. As the main resident immune cells in the CNS, microglia is extremely heterogeneous. Microglia can lyse tumor cells by secreting NO and sheltering the brain from the metastatic cell colonization (33). Despite their anti-tumor ability, microglial have shown their tumor-promoting effect. A previous study showed that in brain metastases initiated from lung cancer, a dense accumulation of activated microglia tightly “encapsulate” brain metastases. Microglia can respond rapidly to the metastatic lung cancer cells in the brain and lead to migration and proliferation (34). Since the morphology and molecular markers of activated microglia like MHC-II molecules, CD40 and other co-stimulatory molecules are similar to those of blood-borne macrophages, it is difficult to distinguish the two types of cells (35). Thus, they are classified into the mononuclear-macrophage system, named as microglia-macrophages. Compared with the blood-borne macrophages, microglia accounts for the minority. They express low levels of the accessory molecules required for efficient antigen presentation and present a weak antigen-presenting activity (31, 36–38). According to their functional differences, microglia can be divided into M1-like and M2-like phenotypes based on the polarization (39). As is known, M1 macrophages can either engulf tumor cells or function as APCs to provoke activate CD8+ T cells and the adaptive immune response, thus killing tumor cells. On the contrary, M2 macrophages are immunosuppressive that promote tumor growth by secreting growth factors or facilitating angiogenesis (39). A large population of microglia-macrophages in intracranial tumors are usually similar to M2 macrophages, called M2-like phenotypes. They induce tumor invasion and angiogenesis by interacting with tumor cells (28). At present, microglia in brain metastases of NSCLC have been rarely reported (34, 39). Thus, targeting microglia with M2 macrophages or inhibiting signaling pathways that activate astrocytes might provide novel ideas for immunotherapy of brain metastases of NSCLC.
Astrocytes
Astrocytes are another glial type in CNS besides microglia, accounting for 30% of cells in CNS (40). Responding to injuries or tumors, astrocytes are activated into a reactive state that are responsible for the repair and the formation of glial scars (41). Astrocytes limit metastases without entering in the lesions in the early stage, and in turn, the inflammatory environment caused by brain metastases can activate astrocytes that further promotes tumor growth (42). Lung cancer cells employ protocadherin 7 (PCDH7) to engage astrocytes and promote the establishment of carcinoma-astrocyte gap (43). These channels allow the transfer of cGAMP from cancer cells to astrocytes, thus activating the STING pathway and promoting inflammatory cytokines released by astrocytes, including interferon-α (IFNα) and tumor necrosis factor (TNF), which is an innate immune response pathway to support tumor growth and chemoresistance (43). Besides, latest evidences have shown that activated astrocytes infiltrating to surrounding tissues are capable of triggering metastasis, in which the STAT3 signaling pathway is a key link for inhibiting intracranial metastases (44).
Other Immune Cells
There exist a small proportion of monocytes in the CSF that comprise about 5% of cells in CSF. They derive from a minority population of CCR1+/CCR5+ monocytes in the blood circulation and are activated and retained in the CNS. For further activation, myeloid monocytes down-regulate CCR1, whereas microglia up-regulate CCR5 (40, 45).
An early study demonstrated that cerebrospinal fluid (CSF) from healthy individuals contains 1,000 to 3,000 leukocytes/ml, which predominantly consist of activated central memory T cells, suggesting that they might be involved in CNS immune surveillance (46). Moreover, it is noticed that CD8+ tissue-resident memory T (Trm) cells have been discovered in the CNS after brain viral infection (46, 47). Although the role of Trm cells in brain immune surveillance as emerged, it is not clear whether they are infiltrated during the brain metastases. To depict the components in immune microenvironment in brain metastases, the overview of immune cells and tumor cells interaction were depicted in Figure 3.
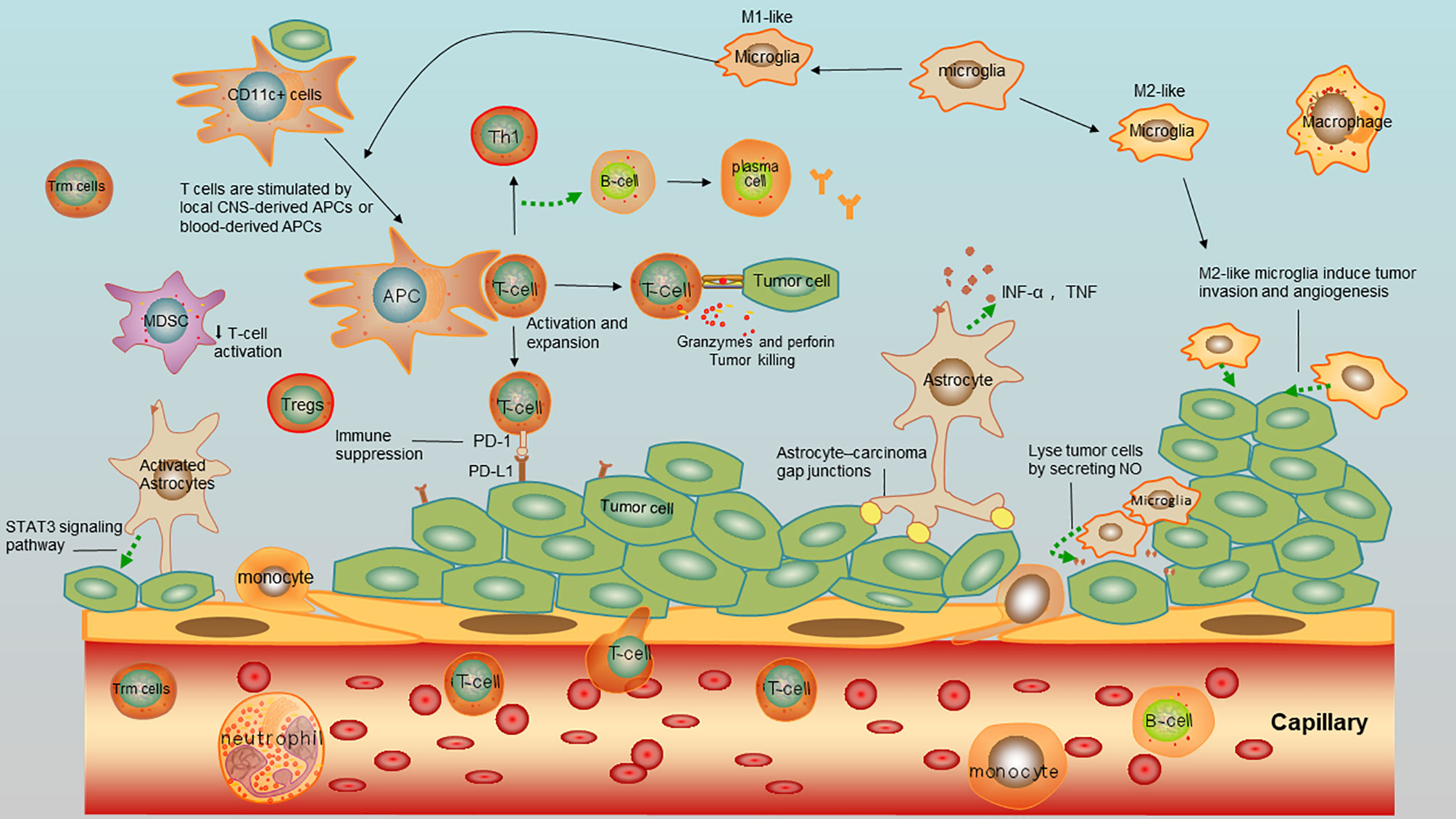
Figure 3 Immnue microenvirment of brain metastases of NSCLC. Tumor cells interact with tumor-infiltrating lymphocytes (TILS), antigen-presenting cells (APCs), astrocytes, microglia, myeloid derived suppressor cells (MDSCs), and macrophages.
Tumor-Infiltrating Lymphocytes (TILS) in Brain Metastases
As is known, TILs are the main component of tumor immune microenvironment and the key subtype of cells involved in the immune response. TILs are subtyped into two categories based on surface molecules, showing anti-cancer function and cancer-promoting effect on the immune escape, respectively (48). During the process of tumor metastasis to the brain, the damaged BBB with increased permeability allows the peripheral lymphatic system passing through the CNS. As a result, TILs can be detected around brain metastases. There are three infiltrating models of TILs, including matrix infiltration, peritumoral infiltration, and diffuse infiltration. Brain metastases of NSCLC are mainly infiltrated as the former two models (49).
TILS for Brain Metastases Prognosis
TILs in brain metastases are positively correlated to the prognosis (12). An immunohistochemical analysis involving 61 specimens of brain metastases of lung cancer showed better overall survival in patients with higher ratios of CD3+ T cells, CD8+ T cells, and CD45+ T cells (memory T cells) than those with lower ratios of the three subtypes of T cells (30). Consistently, another study analyzing 25 pairs of primary NSCLC specimens and brain metastases of NSCLC found that the overall density of CD8+ T cells in the parenchyma of brain metastases is higher than that in primary foci, and patients with a lower number of CD8+ TILs in the matrix present a worse prognosis (50).
Comparison Of TILs in Metastases From in Primary FOCI
TILs in brain metastases differ from those in primary foci of lung, and those in the former present a stronger immunosuppressive phenotype in the tumor microenvironment (12). In an analysis of immune gene expression profile involving 78 pairs of primary NSCLC specimens and brain metastases, a total of 161 differentially expressed genes are detected (51). Compared with primary NSCLC specimens, an attenuated antigen presentation function of dendritic cells, reduced lymphocyte extravasation and down-regulated vascular cell adhesion molecule 1 (VCAM1) are examined in brain metastases, indicating that the immunosuppressive microenvironment is more pronounced in brain metastases than that of primary foci (51). Moreover, they estimated the infiltrating level of immune cell subpopulations and lower infiltrating levels of dendritic cells, Th1 cells, and CD8+T cells are examined in brain metastases than those of primary foci. In addition, the overall ratio of infiltrating lymphocytes in brain metastases is lower than that of the primary foci, but that of macrophages is higher, especially M2 macrophages (51).
Comparison of T Cell Receptor Characteristics in Primary Foci and Brain Metastases
It is found that both primary foci and brain metastases share the majority of tumor-associated antigens. However, the density of T cells and T-cell richness in brain metastases are significantly lower than those of primary foci (51). To further investigate T cell phenotypes, T cell clones are assessed by T cell receptor (TCR)-β sequence. In the process of TCR rearrangement, a highly variable region that recognizes antigenic peptides is formed, called complementarity determining region 3 (CDR3). TCR-β-CDR3 sequence contributes to recognize the diversity of TCR, and determines T cell clonality and abundance (52). To compare characteristics of TCR in primary foci and brain metastases, the TCR-β sequencing analysis is performed in 39 pairs of NSCLC specimens and brain metastases (51). No significant difference in the clonality among brain metastases, pulmonary primary tumors, and normal tissues is found. However, the density of T cells and clonal abundance of T cells are significantly lower in brain metastases than those of primary foci (51). Furthermore, the dominant T cell colonies are analyzed, which are shared in most brain metastases and paired primary foci, and the median ratio of shared colonies in brain metastases reaches 100%. Subsequently, the clonal proliferation of T cells in brain metastases is analyzed. Effective clonal proliferation of T cells is found in 64% of brain metastases, with a median frequency of 11.2%. In most cases, for brain metastases and primary tumors, there are tumor-associated antigens. T cell clone amplification can be detected in brain metastases, but insufficient T cell infiltrations and the diversity of TCR in metastases indicate less abundance of T cells in brain metastases (51). An analysis involving 20 cases of brain metastases of lung adenocarcinoma and primary foci consistently identified that T cell colonies and the diversity of T cells are fewer in brain metastases compared with those of primary foci (53).
Comparison of Tumor Mutational Burden Between Primary Foci and Brain Metastases
Although tumor mutational burden (TMB) level is higher in brain metastases, the novel antigen levels do not increase and are similar to those of primary foci. Therefore, using TMB as a single immunotherapy biomarker is not reliable. Mansfield et al. further detected TMB in brain metastases of lung adenocarcinoma and primary foci (53). The average TMB of 13 cases of brain metastases of lung adenocarcinoma and primary foci is 24.9 and 12.5, respectively, indicating more non-identical mutations in intracranial lesions, which are favorable to the immune response. Later, peptides with a strong affinity to MHC are selected from the mutated sequence as new tumor antigen candidates. However, it is found that the number of new tumor antigens does not increase, which may be attributed to the limitation of current methods for predicting new antigens or short mutations generated by non-identical mutations that only a small part of them can be recognized by the immune system. Therefore, some lung adenocarcinoma patients with a relatively high TMB do not respond to immunotherapy. Moreover, it is also suggested that the use of TMB as a single immunotherapy biomarker in either primary foci or brain metastases is not reliable.
Expression Levels of PD-1 and PD-L1 in Brain Metastases
PD-1 is mainly expressed in immune cells, including activated T cells, monocytes, and dendritic cells. After binding to PD-L1, PD-1 inactivates the cytotoxic T cells that recognize tumor cells, thus leading to the immune escape. PD-L1 is mainly expressed in tumor cells and immune cells, including T cells, B cells, macrophages, and dendritic cells (54).
PD-1 and PD-L1 are differentially expressed between brain metastases and primary foci of lung. Mansfield et al. (55) analyzed pathological samples of 73 cases of brain metastases of lung adenocarcinoma and primary foci. The positive expression of PD-L1 is detected in 39% of brain metastasis samples, while the inconsistency rate of positive expression of PD-L1 in paired cancer samples reaches 14%, and that in immune cells is 26%. It is suggested that the spatial heterogeneity of PD-L1 expression in intracranial and extracranial lesions should be taken into consideration. Notably, down-regulation of PD-L1 or loss of PD-L1 can be detected in a considerable number of intracranial lesions compared with that of primary foci. A large number of brain metastases are non-immune responded (both PD-L1 and TILs are negative).
Therapeutic strategies do not influence expression levels of PD-1/PD-L1 in brain metastases and primary foci. Preoperative radiotherapy, chemotherapy, and hormone therapy generally do not alter expression level of PD-L1 in tumor cells and immune cells of primary foci and brain metastases (56). Up-regulation of PD-1 in immune cells of brain metastases is only detected in 2/61 patients who receive preoperative radiotherapy prior to primary foci resection. Nevertheless, the small sample size limits the reliability of the conclusion that requires to be validated in large-sample studies (56).
Influences of PD-1/PD-L1 levels on therapeutic efficacy of ICI medication in brain metastases need to be further explored as well (12). Currently, a phase III clinical trial of PD-1 inhibitor in the treatment of brain metastases showed that patients with PD-L1 expression ≥ 1% in stromal/immune cells have a longer overall survival than those with PD-L1 <1% (5). Median OS is numerically higher in those with PD-L1 expression ≥ 1% in tumor cells, although no significant difference is obtainable (5). At present, there are multiple studies on immunotherapy for patients with brain metastases, and the therapeutic functions of PD-L1 are waiting to be revealed (12).
Conclusions
As one of the most protected organs in the body, the brain is still prone to be the distant metastatic organ of NSCLC. Compared with extracranial tumors, the immune microenvironment of intracranial tumors is unique and highly specific. Specific immune cells in the immune microenvironment of intracranial tumors mainly include microglia and astrocytes, showing heterogeneous properties. Compared to the primary foci of lung, the immune microenvironment of brain metastases is overall immunosuppressed. More comprehensive and detailed studies are required to pave the way for developing new immunotherapeutic strategies by targeting their immunosuppressive properties, thus controlling brain metastases of NSCLC.
Author Contributions
XZ, PL, and LL were major contributor in writing the manuscript. All authors contributed to the article and approved the submitted version.
Conflict of Interest
The authors declare that the research was conducted in the absence of any commercial or financial relationships that could be construed as a potential conflict of interest.
References
1. Arvold ND, Lee EQ, Mehta MP, Margolin K, Alexander BM, Lin NU, et al. Updates in the Management of Brain Metastases. Neuro Oncol (2016) 18(8):1043–65. doi: 10.1093/neuonc/now127
2. Barnholtz-Sloan JS, Sloan AE, Davis FG, Vigneau FD, Lai P, Sawaya RE. Incidence Proportions of Brain Metastases in Patients Diagnosed (1973 to 2001) in the Metropolitan Detroit Cancer Surveillance System. J Clin Oncol (2004) 22(14):2865–72. doi: 10.1200/JCO.2004.12.149
3. Borghaei H, Paz-Ares L, Horn L, Spigel DR, Steins M, Ready NE, et al. Nivolumab Versus Docetaxel in Advanced Nonsquamous Non-Small-Cell Lung Cancer. N Engl J Med (2015) 373(17):1627–39. doi: 10.1056/NEJMoa1507643
4. Herbst RS, Baas P, Kim DW, Felip E, Perez-Gracia JL, Han JY, et al. Pembrolizumab Versus Docetaxel for Previously Treated, PD-L1-Positive, Advanced Non-Small-Cell Lung Cancer (KEYNOTE-010): A Randomised Controlled Trial. Lancet (2016) 387(10027):1540–50. doi: 10.1016/S0140-6736(15)01281-7
5. Goldberg SB, Schalper KA, Gettinger SN, Mahajan A, Herbst RS, Chiang AC, et al. Pembrolizumab for Management of Patients With NSCLC and Brain Metastases: Long-Term Results and Biomarker Analysis From a non-Randomised, Open-Label, Phase 2 Trial. Lancet Oncol (2020) 21(5):655–63. doi: 10.1016/S1470-2045(20)30111-X
6. Boire A, Brastianos PK, Garzia L, Valiente M. Brain Metastasis. Nat Rev Cancer (2020) 20(1):4–11. doi: 10.1038/s41568-019-0220-y
7. Valastyan S, Weinberg RA. Tumor Metastasis: Molecular Insights and Evolving Paradigms. Cell (2011) 147(2):275–92. doi: 10.1016/j.cell.2011.09.024
8. Fidler IJ. The Pathogenesis of Cancer Metastasis: The ‘Seed and Soil’ Hypothesis Revisited. Nat Rev Cancer (2003) 3(6):453–8. doi: 10.1038/nrc1098
9. Riihimaki M, Hemminki A, Fallah M, Thomsen H, Sundquist K, Sundquist J, et al. Metastatic Sites and Survival in Lung Cancer. Lung Cancer (2014) 86(1):78–84. doi: 10.1016/j.lungcan.2014.07.020
10. Liu W, Song J, Du X, Zhou Y, Li Y, Li R, et al. AKR1B10 (Aldo-Keto Reductase Family 1 B10) Promotes Brain Metastasis of Lung Cancer Cells in a Multi-Organ Microfluidic Chip Model. Acta Biomater (2019) 91:195–208. doi: 10.1016/j.actbio.2019.04.053
11. Shih DJH, Nayyar N, Bihun I, Dagogo-Jack I, Gill CM, Aquilanti E, et al. Genomic Characterization of Human Brain Metastases Identifies Drivers of Metastatic Lung Adenocarcinoma. Nat Genet (2020) 52(4):371–7.
12. Vilarino N, Bruna J, Bosch-Barrera J, Valiente M, Nadal E. Immunotherapy in NSCLC Patients With Brain Metastases. Understanding Brain Tumor Microenvironment and Dissecting Outcomes From Immune Checkpoint Blockade in the Clinic. Cancer Treat Rev (2020) 89:102067. doi: 10.1016/j.ctrv.2020.102067
13. Dai L, Zhao J, Yin J, Fu W, Chen G. Cell Adhesion Molecule 2 (CADM2) Promotes Brain Metastasis by Inducing Epithelial-Mesenchymal Transition (EMT) in Human Non-Small Cell Lung Cancer. Ann Transl Med (2020) 8(7):465. doi: 10.21037/atm.2020.03.85
14. Shen L, Chen L, Wang Y, Jiang X, Xia H, Zhuang Z. Long Noncoding RNA MALAT1 Promotes Brain Metastasis by Inducing Epithelial-Mesenchymal Transition in Lung Cancer. J Neurooncol (2015) 121(1):101–8. doi: 10.1007/s11060-014-1613-0
15. Wei C, Zhang R, Cai Q, Gao X, Tong F, Dong J, et al. MicroRNA-330-3p Promotes Brain Metastasis and Epithelial-Mesenchymal Transition Via GRIA3 in Non-Small Cell Lung Cancer. Aging (Albany NY) (2019) 11(17):6734–61. doi: 10.18632/aging.102201
16. Lin CY, Chen HJ, Huang CC, Lai LC, Lu TP, Tseng GC, et al. ADAM9 Promotes Lung Cancer Metastases to Brain by a Plasminogen Activator-Based Pathway. Cancer Res (2014) 74(18):5229–43. doi: 10.1158/0008-5472.CAN-13-2995
17. Wang H, Deng Q, Lv Z, Ling Y, Hou X, Chen Z, et al. N6-Methyladenosine Induced miR-143-3p Promotes the Brain Metastasis of Lung Cancer Via Regulation of VASH1. Mol Cancer (2019) 18(1):181. doi: 10.1186/s12943-019-1108-x
18. Munsterberg J, Loreth D, Brylka L, Werner S, Karbanova J, Gandrass M, et al. ALCAM Contributes to Brain Metastasis Formation in Non-Small-Cell Lung Cancer Through Interaction With the Vascular Endothelium. Neuro Oncol (2020) 22(7):955–66. doi: 10.1093/neuonc/noaa028
19. Wei C, Dong X, Lu H, Tong F, Chen L, Zhang R, et al. LPCAT1 Promotes Brain Metastasis of Lung Adenocarcinoma by Up-Regulating PI3K/AKT/MYC Pathway. J Exp Clin Cancer Res (2019) 38(1):95. doi: 10.1186/s13046-019-1092-4
20. Hoj JP, Mayro B, Pendergast AM. A TAZ-AXL-ABL2 Feed-Forward Signaling Axis Promotes Lung Adenocarcinoma Brain Metastasis. Cell Rep (2019) 29(11):3421–34.e8. doi: 10.1016/j.celrep.2019.11.018
21. Li YD, Lamano JB, Lamano JB, Quaggin-Smith J, Veliceasa D, Kaur G, et al. Tumor-Induced Peripheral Immunosuppression Promotes Brain Metastasis in Patients With Non-Small Cell Lung Cancer. Cancer Immunol Immunother (2019) 68(9):1501–13. doi: 10.1007/s00262-019-02384-y
22. Lun MP, Monuki ES, Lehtinen MK. Development and Functions of the Choroid Plexus-Cerebrospinal Fluid System. Nat Rev Neurosci (2015) 16(8):445–57. doi: 10.1038/nrn3921
23. Banks WA. From Blood-Brain Barrier to Blood-Brain Interface: New Opportunities for CNS Drug Delivery. Nat Rev Drug Discov (2016) 15(4):275–92. doi: 10.1038/nrd.2015.21
24. Sprowls SA, Arsiwala TA, Bumgarner JR, Shah N, Lateef SS, Kielkowski BN, et al. Improving CNS Delivery to Brain Metastases by Blood-Tumor Barrier Disruption. Trends Cancer (2019) 5(8):495–505. doi: 10.1016/j.trecan.2019.06.003
25. Lockman PR, Mittapalli RK, Taskar KS, Rudraraju V, Gril B, Bohn KA, et al. Heterogeneous Blood-Tumor Barrier Permeability Determines Drug Efficacy in Experimental Brain Metastases of Breast Cancer. Clin Cancer Res (2010) 16(23):5664–78. doi: 10.1158/1078-0432.CCR-10-1564
26. Lyle LT, Lockman PR, Adkins CE, Mohammad AS, Sechrest E, Hua E, et al. Alterations in Pericyte Subpopulations Are Associated With Elevated Blood-Tumor Barrier Permeability in Experimental Brain Metastasis of Breast Cancer. Clin Cancer Res (2016) 22(21):5287–99. doi: 10.1158/1078-0432.CCR-15-1836
27. Hendricks BK, Cohen-Gadol AA, Miller JC. Novel Delivery Methods Bypassing the Blood-Brain and Blood-Tumor Barriers. Neurosurg Focus (2015) 38(3):E10. doi: 10.3171/2015.1.FOCUS14767
28. Soffietti R, Ahluwalia M, Lin N, Ruda R. Management of Brain Metastases According to Molecular Subtypes. Nat Rev Neurol (2020) 16(10):557–74. doi: 10.1038/s41582-020-0391-x
29. Korn T, Kallies A. T Cell Responses in the Central Nervous System. Nat Rev Immunol (2017) 17(3):179–94. doi: 10.1038/nri.2016.144
30. Berghoff AS, Fuchs E, Ricken G, Mlecnik B, Bindea G, Spanberger T, et al. Density of Tumor-Infiltrating Lymphocytes Correlates With Extent of Brain Edema and Overall Survival Time in Patients With Brain Metastases. Oncoimmunology (2016) 5(1):e1057388. doi: 10.1080/2162402X.2015.1057388
31. Ransohoff RM, Engelhardt B. The Anatomical and Cellular Basis of Immune Surveillance in the Central Nervous System. Nat Rev Immunol (2012) 12(9):623–35. doi: 10.1038/nri3265
32. Prodinger C, Bunse J, Kruger M, Schiefenhovel F, Brandt C, Laman JD, et al. CD11c-Expressing Cells Reside in the Juxtavascular Parenchyma and Extend Processes Into the Glia Limitans of the Mouse Nervous System. Acta Neuropathol (2011) 121(4):445–58. doi: 10.1007/s00401-010-0774-y
33. Brantley EC, Guo L, Zhang C, Lin Q, Yokoi K, Langley RR, et al. Nitric Oxide-Mediated Tumoricidal Activity of Murine Microglial Cells. Transl Oncol (2010) 3(6):380–8. doi: 10.1593/tlo.10208
34. He BP, Wang JJ, Zhang X, Wu Y, Wang M, Bay BH, et al. Differential Reactions of Microglia to Brain Metastasis of Lung Cancer. Mol Med (2006) 12(7-8):161–70. doi: 10.2119/2006-00033.He
35. Utz SG, See P, Mildenberger W, Thion MS, Silvin A, Lutz M, et al. Early Fate Defines Microglia and Non-Parenchymal Brain Macrophage Development. Cell (2020) 181(3):557–73.e18. doi: 10.1016/j.cell.2020.03.021
36. Ransohoff RM, Perry VH. Microglial Physiology: Unique Stimuli, Specialized Responses. Annu Rev Immunol (2009) 27(119-45). doi: 10.1146/annurev.immunol.021908.132528
37. Ransohoff RM, Cardona AE. The Myeloid Cells of the Central Nervous System Parenchyma. Nature (2010) 468(7321):253–62. doi: 10.1038/nature09615
38. Hanisch UK, Kettenmann H. Microglia: Active Sensor and Versatile Effector Cells in the Normal and Pathologic Brain. Nat Neurosci (2007) 10(11):1387–94. doi: 10.1038/nn1997
39. Yuan A, Hsiao YJ, Chen HY, Chen HW, Ho CC, Chen YY, et al. Opposite Effects of M1 and M2 Macrophage Subtypes on Lung Cancer Progression. Sci Rep (2015) 5:14273. doi: 10.1038/srep14273
40. Liddelow SA, Barres BA. Reactive Astrocytes: Production, Function, and Therapeutic Potential. Immunity (2017) 46(6):957–67. doi: 10.1016/j.immuni.2017.06.006
41. Sofroniew MV. Molecular Dissection of Reactive Astrogliosis and Glial Scar Formation. Trends Neurosci (2009) 32(12):638–47. doi: 10.1016/j.tins.2009.08.002
42. Anderson MA, Ao Y, Sofroniew MV. Heterogeneity of Reactive Astrocytes. Neurosci Lett (2014) 565:23–9. doi: 10.1016/j.neulet.2013.12.030
43. Chen Q, Boire A, Jin X, Valiente M, Er EE, Lopez–Soto A, et al. Carcinoma-Astrocyte Gap Junctions Promote Brain Metastasis by cGAMP Transfer. Nature (2016) 533(7604):493–8. doi: 10.1038/nature18268
44. Priego N, Zhu L, Monteiro C, Mulders M, Wasilewski D, Bindeman W, et al. STAT3 Labels a Subpopulation of Reactive Astrocytes Required for Brain Metastasis. Nat Med (2018) 24(7):1024–35. doi: 10.1158/1538-7445.AM2019-2746
45. Trebst C, Sorensen TL, Kivisakk P, Cathcart MK, Hesselgesser J, Horuk R, et al. CCR1+/CCR5+ Mononuclear Phagocytes Accumulate in the Central Nervous System of Patients With Multiple Sclerosis. Am J Pathol (2001) 159(5):1701–10. doi: 10.1016/S0002-9440(10)63017-9
46. Kivisakk P, Mahad DJ, Callahan MK, Trebst C, Tucky B, Wei T, et al. Human Cerebrospinal Fluid Central Memory CD4+ T Cells: Evidence for Trafficking Through Choroid Plexus and Meninges Via P-Selectin. Proc Natl Acad Sci USA (2003) 100(14):8389–94. doi: 10.1073/pnas.1433000100
47. Wakim LM, Woodward-Davis A, Bevan MJ. Memory T Cells Persisting Within the Brain After Local Infection Show Functional Adaptations to Their Tissue of Residence. Proc Natl Acad Sci USA (2010) 107(42):17872–9. doi: 10.1073/pnas.1010201107
48. Schreiber RD, Old LJ, Smyth MJ. Cancer Immunoediting: Integrating Immunity’s Roles in Cancer Suppression and Promotion. Science (2011) 331(6024):1565–70. doi: 10.1126/science.1203486
49. Harter PN, Bernatz S, Scholz A, Zeiner PS, Zinke J, Kiyose M, et al. Distribution and Prognostic Relevance of Tumor-Infiltrating Lymphocytes (Tils) and PD-1/PD-L1 Immune Checkpoints in Human Brain Metastases. Oncotarget (2015) 6(38):40836–49. doi: 10.18632/oncotarget.5696
50. Zhou J, Gong Z, Jia Q, Wu Y, Yang ZZ, Zhu B. Programmed Death Ligand 1 Expression and CD8(+) Tumor-Infiltrating Lymphocyte Density Differences Between Paired Primary and Brain Metastatic Lesions in Non-Small Cell Lung Cancer. Biochem Biophys Res Commun (2018) 498(4):751–7. doi: 10.1016/j.bbrc.2018.03.053
51. Kudo Y, Haymaker C, Zhang J, Reuben A, Duose DY, Fujimoto J, et al. Suppressed Immune Microenvironment and Repertoire in Brain Metastases From Patients With Resected Non-Small-Cell Lung Cancer. Ann Oncol (2019) 30(9):1521–30. doi: 10.1093/annonc/mdz207
52. Liu P, Liu D, Yang X, Gao J, Chen Y, Xiao X, et al. Characterization of Human AlphabetaTCR Repertoire and Discovery of D-D Fusion in TCRbeta Chains. Protein Cell (2014) 5(8):603–15. doi: 10.1007/s13238-014-0060-1
53. Mansfield AS, Ren H, Sutor S, Sarangi V, Nair A, Davila J, et al. Contraction of T Cell Richness in Lung Cancer Brain Metastases. Sci Rep (2018) 8(1):2171. doi: 10.1038/s41598-018-20622-8
54. Cha JH, Chan LC, Li CW, Hsu JL, Hung MC. Mechanisms Controlling Pd-L1 Expression in Cancer. Mol Cell (2019) 76(3):359–70. doi: 10.1016/j.molcel.2019.09.030
55. Mansfield AS, Aubry MC, Moser JC, Harrington SM, Dronca RS, Park SS, et al. Temporal and Spatial Discordance of Programmed Cell Death-Ligand 1 Expression and Lymphocyte Tumor Infiltration Between Paired Primary Lesions and Brain Metastases in Lung Cancer. Ann Oncol (2016) 27(10):1953–8. doi: 10.1093/annonc/mdw289
Keywords: immune microenvironment, brain metastases, non-small cell lung cancer, immune therapeutics, brain metastases of non-small cell lung cancer
Citation: Luo L, Liu P, Zhao K, Zhao W and Zhang X (2021) The Immune Microenvironment in Brain Metastases of Non-Small Cell Lung Cancer. Front. Oncol. 11:698844. doi: 10.3389/fonc.2021.698844
Received: 22 April 2021; Accepted: 28 May 2021;
Published: 14 July 2021.
Edited by:
Kevin J. Ni, St George Hospital, AustraliaReviewed by:
Xiaoxia Chen, Shanghai Pulmonary Hospital, ChinaTianqing Chu, Shanghai Jiaotong University, China
Copyright © 2021 Luo, Liu, Zhao, Zhao and Zhang. This is an open-access article distributed under the terms of the Creative Commons Attribution License (CC BY). The use, distribution or reproduction in other forums is permitted, provided the original author(s) and the copyright owner(s) are credited and that the original publication in this journal is cited, in accordance with accepted academic practice. No use, distribution or reproduction is permitted which does not comply with these terms.
*Correspondence: Xiaofei Zhang, emh4Zl8xMDE3QDE2My5jb20=; Weixin Zhao, end4MjFAMTI2LmNvbQ==
†These authors share first authorship