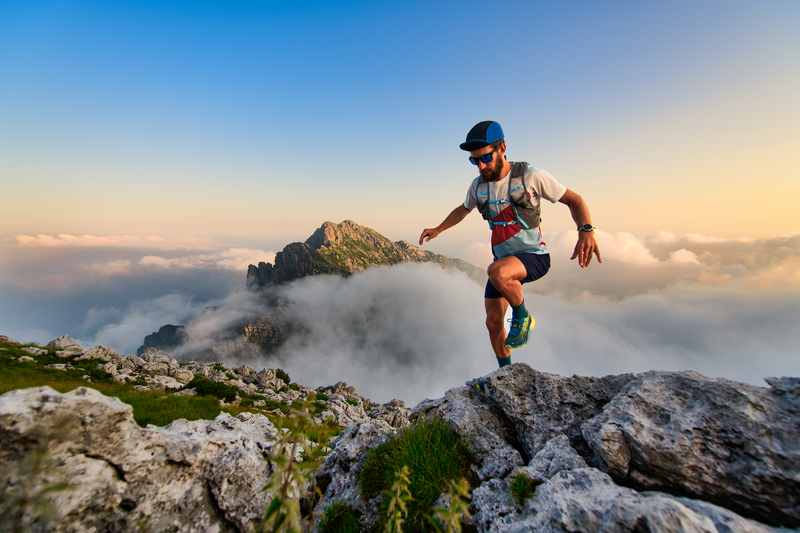
94% of researchers rate our articles as excellent or good
Learn more about the work of our research integrity team to safeguard the quality of each article we publish.
Find out more
REVIEW article
Front. Oncol. , 27 August 2021
Sec. Cancer Immunity and Immunotherapy
Volume 11 - 2021 | https://doi.org/10.3389/fonc.2021.682325
This article is part of the Research Topic The Clinical Application of Neoantigens View all 11 articles
Somatic mutation-derived neoantigens, expressed only on tumor cells, may elicit antitumor T-cell responses in cancer immunotherapies with minimal immune tolerance. Neoantigens can be identified by multiple bioinformatics technologies, mainly based on whole-exome sequencing. Personalized cancer vaccines and adoptive T cell therapies are two primary treatment modalities targeting neoantigens, and both of them have shown promising therapeutic effects. This review, summarizes the history of neoantigen-related tumor control, introduces recent neoantigen screening and identification methods, and discusses the role of neoantigen in cancer immunotherapies. Moreover, we propose the challenges of targeting neoantigens for cancer treatment.
Immunotherapy has revolutionized the management of cancer treatment. Targeting the immune inhibitory regulators PD-1, PD-L1 or CTLA-4 is a common way to promote antitumor immune responses. However, immunotherapy boosting the immune system to destroy cancer cells has shown durable clinical responses in patients with various malignancies, but only in a subset of patients (1). Moreover, the non-antigen-specific simulation can activate global T cells and damage the human body, leading to immune-related adverse events, even fatal events (2). Thus substantial efforts are needed to explore more specific and powerful immunotherapy approaches, either alone or in combination.
As a genetic disease, cancer results from the accumulation of DNA damage and genetic alterations (3). These non-synonymous somatic mutations generate neoepitopes, which can be recognized by endogenous T cells as non-self-proteins and induce an antitumor immune response (1, 4). In most cases, neoantigens arise from single nucleotide mutations (SNV), gene fusion, alternative splicing, intron retention, insertion, and deletions. Other sources of neoantigens including post-translational modifications and endogenous retrovirus-associated tumors (5). The presentation of neoantigens is tumor-specific and able to elicit T cell-mediated antitumor immunity (4). Therefore, neoantigen is an ideal immunotherapy candidate. Two primary neoantigen-based therapeutic modalities have been explored in clinical practices: personalized vaccines and adoptive cell therapy (ACT) (6). Here we review the history of neoantigens about tumor control and introduce recent neoantigen screening and identification methods. We also discuss the role of neoantigen in cancer immunotherapies and its current challenges.
The foundation of cancer immunology can date back to the middle of the twentieth. Gross (7) reported that transplantable tumors could produce active immunity against syngeneic mice. Another research showed that carcinogen-induced tumors had antigenic properties that the immune system could identify (8). In 1988, De Plaen et al. (9) discovered the first neoantigen recognized by cytolytic T lymphocytes (CTLs) in a methylcholanthrene (MCA)-induced mouse tumor model. The recognized neoantigen also could be identified in a cDNA library. Then, CD4+ T cells stimulated tumor-specific immunity and inhibited tumor growth after recognizing neoantigens in mice (10). In 1996, mutated neoantigens recognized by tumor-infiltrating lymphocytes (TILs) and CTLs were found in human melanoma (11) and renal cell carcinoma (12), respectively. Several years later, Rosenberg and his colleagues (13) revealed nearly complete regression in a melanoma patient after receiving autologous tumor-reactive TILs. T cells reactivity against neoantigen was proved to predominate cellular antitumor response in a long-term surviving melanoma patient (14). A detailed analysis in a melanoma patient with complete tumor regression after adoptive TILs transfer indicated that tumor-reactive T cells could be persistent and relevant for tumor regression (15). Collectively, neoantigen has the potential to be the target of antitumor immunity.
Advances in next-generation sequencing (NGS) technologies have provided access to compare mutations in the normal and tumor genome in 2008 (16). Then NGS was used to demonstrate tumor-specific antigens in an immunogenic mouse model, which opened a new dimension for antigenic targets of cancer immunity (17). By using NGS, Castle et al. (18) assessed the antitumoral activity of the neoantigen vaccine in the B16 melanoma tumor model. Consequently, the vaccine-elicited T-cell immunogenicity showed protective effects, providing new insights into immunogenic neoantigen-based vaccine treatment in human cancers (18). NGS also contributed to identify neoantigens recognized by TILs in melanoma patients (19, 20) and suggested the feasibility of neoantigen-specific T cell reactivity analysis in human cancer immunotherapy (20). Neoantigen was identified as the target of checkpoint blockade immunotherapies in a sarcomas mouse model (21). Concurrently, the adoptive transfer of neoantigen-specific CD4+ T cells achieved tumor regression in a metastatic epithelial cancer (22).
Further investigations found that neoantigen burden was associated with immune checkpoint inhibitors’ therapeutic responses in melanoma (23) and lung cancer patients (24). In 2015, Beatriz’s team first reported that vaccination with neoantigen could augment T cell immunity in patients with advanced melanoma, demonstrating the efficacy and feasibility of a personalized dendritic cell (DC)vaccine (25). A subsequent study revealed that ACT targeting mutant KRAS neoantigen mediated antitumor immune system, leading to regression in patients with metastatic colon cancer (26). Furthermore, the correlation between clonal neoantigen burden and response to immune checkpoint blockade was defined, rendering it is possible to target clonal neoantigens in T cell therapies (27). Ott et al. treated melanoma patients with a peptide vaccine targeting 20 predicted personal tumor neoantigens (28). Consistent with the reactive T cells responses, four patients had no recurrent diseases, and two patients had complete tumor regression after anti-PD-1 was administrated to recurrence (28). Then individualized RNA mutanome vaccine also induced T cell infiltration and killed autologous tumor cells, resulting in tumor regression and durable survival (29). The personalized neoantigen vaccine and nivolumab combination proved safe and immunogenicity in advanced solid tumors (30).
With the advances of NGS, it has become feasible to acquire genomic changes in some kinds of tumors, which provided the basis of neoantigen identification (31). Thus, the current process of predicting candidate neoantigens mainly includes three parts: 1) identify tumor-specific mutations using whole-genome sequencing (WGS), whole-exome sequencing (WES), or RNA-seq; 2) predict major histocompatibility complex (MHC) types, which in humans are called human leukocyte antigen (HLA) and neoantigen presentation; 3) prioritize and select candidate neoantigens (4, 32).
WES should be performed to map the cancer mutanome from the tumor and paired normal tissue. Combining RNA-seq can determine whether the mutation is expressed in tumors and infer its relative frequency overlapped with exome-based variants (4, 33). The minimum requirements for immunogenic and potential therapeutic mutations include: 1) the mutant peptide must be processed and presented by MHC molecules, and 2) the presented peptide-MHC complex must be recognized by endogenous T cells (31). Antigen processing and presentation are complex but different processes for MHC class I and II molecules (34). Peptides binding to MHC-I molecules are usually of a small length of 8-10 residues, while MHC-II molecules could bind to longer peptides with a length of 11-20 amino acids (34–36). Several tools depend on NGS data from WES, WGS, or RNA-seq can be used to predict HLA alleles, including Optitype (37) and Polysolver (38) for class I alleles, seq2HLA (39), Athlates (40), HLAScan (41), HLAProfiler (42), PHLAT (43), and ArcasHLA (44) for both class I and II HLA alleles. Following this, computational algorithms [for example, NetMHC (45), NetMHCpan (46, 47), and MHCflurry (48)] have been developed to predict MHC binding affinity. The criteria of binding affinities (IC50) are defined as high, intermediate to weak, and non-binder with IC50 <150, 150-500, or >500 nM, respectively (the commonly described binding affinity threshold) (49). The prioritization of candidate neoantigens can be achieved by the predicted binding affinities alone or in combination with the mutant expression level (50). On the other hand, mass spectrometry (MS) based immunopeptidome has enabled the discovery of thousands of MHC-associated neoantigens (51), and the combination of MS and WES is a powerful weapon to predict immunogenic tumor mutations (52). Compared to MS, T cell-based assays can directly detect whether an MHC-presented neoantigen has been recognized by T cells (4, 32). A variety of methodologies [e.g., fluorescently labeled HLA tetramers or multimers (53), the enzyme-linked immunosorbent spot (ELISpot) (54)] that stimulate and test neoantigen-reactive T cells have been reported. However, these cell-based assays are currently costly, time-consuming, and technically challenging (4).
The predicted tumor-specific neoantigens are relevant targets for clinical personalized immunotherapies, either as a vaccine or a cellular therapy product.
Personalized vaccines can be formulated as synthetic long peptide (SLP), DNA, RNA, DC, and viral and bacteria (55). Results from several clinical trials using neoantigen vaccines in patients with melanoma or glioblastoma are encouraging (25, 28, 29, 56, 57). Carreno et al. (25) first found that the DC vaccine augmented pre-existing neoantigen-specific immunity and induced previously undetected neoantigen induced T cell responses in three advanced melanoma patients. Neoantigens are processed and presented by HLA-A*02:01 molecules. Two subsequent clinical studies published in 2017 confirmed the potential of personalized neoantigen vaccine in treating patients with melanoma (28, 29). Ott et al. vaccinated six patients with SLP targeting up to 20 predicted tumor neoantigens (NCT01970358). Four of the six patients experienced no tumor recurrence in the following 25 months after vaccination, and another two patients with recurrence achieved complete tumor regression after received anti-PD-1 therapy (28).
Further analysis indicated that eight high-risk patients had durable neoantigens-induced T cell responses. It is encouraging that almost four years after being treated with vaccines, all are alive, and six have no active disease (58). Sahin et al. generated the first individualized RNA mutanome vaccines in 13 patients with stage melanoma (NCT02035956). Eight patients remained tumor-free within the follow-up period. Two of five patients with metastatic disease achieved objective responses, while one presented a complete response to RNA vaccine combined with PD-1 inhibitor (29).
Notably, two recent clinical trials emphasized the significance of the tailored vaccines in treating glioblastoma (56, 57). First, Keskin et al. treated glioblastoma patients with an individualized multi-epitope vaccine (NCT02287428), leading to increased neoantigen-specific CD4+ and CD8+ T cells responses in peripheral blood (56). Second, in another clinical trial, Hilf et al. reported that the combination of personalized vaccine and standard of care (NCT02149225) could induce sustained CD8+ T cells responses and predominantly CD4+ Th1 cell responses in glioblastoma patients (57).
Those encouraging results indicated that the personalized neoantigen vaccine approach is feasible for immunologically “cold” tumors with a low tumor mutation burden (56). Another early DC-based vaccine study in ovarian cancer has shown promising clinical outcomes without serious adverse events (59). Vaccination upregulated T cells responses against neoantigens, therefore elicited a broad antitumor immunity. In line with previous studies, the neoantigen-based EpiGVAX vaccine improved antitumor immunity in colorectal cancer (60). Recently, Ott et al. conducted a clinical trial that combined personalized neoantigen-based vaccine (NEO-PV-01) with nivolumab in patients with advanced melanoma, non-small cell lung cancer, and urothelial cancer (NCT02897765) (30). T cells responsive to neoantigens were detected in all vaccinated patients, while no serious adverse events were observed. The increasing T cells induced by this approach could control tumor growth and kill tumor cells, leading to potential clinical benefits (30).
To date, dozens of clinical trials investigating personalized neoantigen-based vaccines alone or in combination with checkpoint inhibitors are underway in various cancers (Table 1).
Another neoantigen-targeted treatment approach is adoptive cell therapy (ACT). Natural or modified T cells are expanded ex vivo and infused into patients to enhance T cell responses and kill tumor cells. Adoptive T cell therapies include the adoptive transfer of TILs, or of T cells genetically engineered to express a T cell receptor (TCR), or a chimeric antigen receptor (CAR), as well as other immune cells like natural killer cells (61).
Personal TILs could recognize neoepitopes derived from somatic mutations were identified in gastrointestinal cancers (62). Several studies have shown that the adoptive transfer of T cells specific against oncogenic mutations could mediate tumor regression in metastatic cholangiocarcinoma (22), colorectal cancer (26), cervical cancer (63), and breast cancer (64). In 2014, Rosenberg et al. administrated neoantigen-reactive CD4+ TILs in a patient with metastatic cholangiocarcinoma (NCT01174121), resulting in complete tumor regression (22). This finding evidenced that CD4+ T cells against neoantigens can be used to mediate epithelial cancer regression. Then a patient with metastatic colorectal cancer was found to have CD8+ T cells in TILs which could specifically target mutant KRAS G12D (NCT01174121) (26). After infusion of the HLA-C*08:02–restricted TILs, all lung metastases’ objective regression was observed. One lesion that progressed nine months later was proven to have the loss of chromosome 6 encoding the HLA-C*08:02 MHC class I molecule (26). Additionally, therapeutic TILs against mutant neoantigens induced immunodominant antitumor T cell responses instead of against human papillomavirus (HPV) antigens, resulting in complete tumor regression in virally associated cervical cancer (63). Successful adoptive therapy was also found in patients with metastatic breast cancer (NCT01174121) (64). After received TILs against four neoantigens (SLC3A2, KIAA0368, CADPS2, and CTSB), the patient achieved durable tumor regression over 22 months (64). All these works supported that the adoptive transfer of neoantigen-based TILs played a vital role in immunotherapies.
Table 2 showed selected ongoing adoptive T cell therapy studies.
Despite recent advances, many challenges remain for the application of personalized neoantigen-based vaccine or adoptive cell transfer.
A critical issue that needs to be addressed is the expensive and time-consuming manufacturing. Although the cost of genome sequencing has decreased (65), it remains costly to identify neoantigens and process good manufacturing practices. The overall timeline from acquiring the patient’s sample to vaccine administration was about 3 to 5 months (34). Reducing production turnaround time is urgent, especially for patients with metastatic disease. These cell-based experiments also are difficult to standardize and require significant numbers of cells. Thus high-throughput and unbiased computational strategies may need to select neoantigens (4). An additional obstacle could be neoantigens prediction and validation. Despite the current computational neoantigen prediction algorithms and experimental validation approaches (tetramers or multimers, ELISpot) used to prioritize neoantigens, some efforts are still needed to pursue further optimization. Strategies include better predict MHC-peptide binding and develop big datasets and new algorithms (4). In addition, tumor heterogeneity is common and can be caused by several factors, including 1) spontaneous mutations during tumor progression, 2) tumor microenvironments regulation or neoantigen loss (66), and 3) multiple lesions or even an individual tumor originated from different subclones (61). Tumor heterogeneity can reduce the accuracy of antigen clone prediction in heterogeneous tumor masses. Therefore, it is vital to analyze beneficial mutations carefully. In addition, fully personalized immunotherapy targeting multiple clonal neoantigens may also need to overcome the obstacle of tumor heterogeneity (4, 61). Another challenge is to define the reliable immune biomarkers to predict antitumor immunity and even survival benefit. Although immune-related response criteria (irRC) attempt to evaluate immunotherapeutic effects in clinical practice, they may still not fully reflect all the characteristics of clinical responses (67). Furthermore, the T cell responses induced by neoantigens-based therapies may not directly be translated into durable clinical responses. Thus, it is plausible to identify immune response biomarkers in a systematic pattern.
Emerging evidence reveals that tumor neoantigens play an essential role in antitumor immunity and successful cancer immunotherapies. Both personalized neoantigen-based vaccines and ACT approaches have shown encouraging antitumor results. Decreased production turnaround time, reduced manufacturing cost, better detection of immunogenic neoantigens, improved computational algorithms, and effective treatment biomarkers are expected to add the feasibility, affordability, and momentum of neoantigen targeting therapies. Neoantigen-based therapies have the potential to turn “cold” tumors into “hot” ones. Therefore, it’s warranted to explore the combinatorial approaches with other immunotherapies, including checkpoint blockade therapies or conventional treatments, including chemoradiotherapies, kinase inhibitors, anti-angiogenesis therapies, et al. (68). Thus, it’s plausible to think that neoantigen-based tailored therapies can be widely performed in various cancers soon.
YZ: Conceptualization, writing the original draft. JL: Funding acquisition, writing review, and editing. All authors contributed to the article and approved the submitted version.
This work was supported by the Sichuan Science and Technology Department Key Research and Development Project (2019YFS0539), the 1.3.5 Project for Disciplines of Excellence, West China Hospital, Sichuan University (ZYJC18022), and the National Clinical Research Center for Geriatrics (West China Hospital, Z2018B12).
The authors declare that the research was conducted in the absence of any commercial or financial relationships that could be construed as a potential conflict of interest.
All claims expressed in this article are solely those of the authors and do not necessarily represent those of their affiliated organizations, or those of the publisher, the editors and the reviewers. Any product that may be evaluated in this article, or claim that may be made by its manufacturer, is not guaranteed or endorsed by the publisher.
1. De Mattos-Arruda L, Blanco-Heredia J, Aguilar-Gurrieri C, Carrillo J, Blanco J. New Emerging Targets in Cancer Immunotherapy: The Role of Neoantigens. ESMO Open (2020) 4(Suppl 3):e000684. doi: 10.1136/esmoopen-2020-000684.
2. Wang DY, Salem JE, Cohen JV, Chandra S, Menzer C, Ye F, et al. Fatal Toxic Effects Associated With Immune Checkpoint Inhibitors: A Systematic Review and Meta-Analysis. JAMA Oncol (2018) 4(12):1721–8. doi: 10.1001/jamaoncol.2018.3923
3. Linnemann C, Mezzadra R, Schumacher TN. TCR Repertoires of Intratumoral T-Cell Subsets. Immunol Rev (2014) 257(1):72–82. doi: 10.1111/imr.12140
4. De Mattos-Arruda L, Vazquez M, Finotello F, Lepore R, Porta E, Hundal J, et al. Neoantigen Prediction and Computational Perspectives Towards Clinical Benefit: Recommendations From the ESMO Precision Medicine Working Group. Ann Oncol (2020) 31(8):978–90. doi: 10.1016/j.annonc.2020.05.008
5. Srivastava RM, Purohit TA, Chan TA. Diverse Neoantigens and the Development of Cancer Therapies. Semin Radiat Oncol (2020) 30(2):113–28. doi: 10.1016/j.semradonc.2019.12.001
6. Jiang T, Shi T, Zhang H, Hu J, Song Y, Wei J, et al. Tumor Neoantigens: From Basic Research to Clinical Applications. J Hematol Oncol (2019) 12(1):93. doi: 10.1186/s13045-019-0787-5
7. Gross L. Intradermal Immunization of C3H Mice Against a Sarcoma That Originated in an Animal of the Same Line. Cancer Res (1943) 3(5):326–33.
8. Foley EJ. Antigenic Properties of Methylcholanthrene-Induced Tumors in Mice of the Strain of Origin. Cancer Res (1953) 13(12):835–7.
9. De Plaen E, Lurquin C, Van Pel A, Mariamé B, Szikora JP, Wölfel T, et al. Immunogenic (Tum-) Variants of Mouse Tumor P815: Cloning of the Gene of Tum- Antigen P91A and Identification of the Tum- Mutation. Proc Natl Acad Sci USA (1988) 85(7):2274–8. doi: 10.1073/pnas.85.7.2274
10. Monach PA, Meredith SC, Siegel CT, Schreiber H. A Unique Tumor Antigen Produced by a Single Amino Acid Substitution. Immunity (1995) 2(1):45–59. doi: 10.1016/1074-7613(95)90078-0
11. Robbins PF, El-Gamil M, Li YF, Kawakami Y, Loftus D, Appella E, et al. A Mutated Beta-Catenin Gene Encodes a Melanoma-Specific Antigen Recognized by Tumor Infiltrating Lymphocytes. J Exp Med (1996) 183(3):1185–92. doi: 10.1084/jem.183.3.1185
12. Brändle D, Brasseur F, Weynants P, Boon T, Van den Eynde B. A Mutated HLA-A2 Molecule Recognized by Autologous Cytotoxic T Lymphocytes on a Human Renal Cell Carcinoma. J Exp Med (1996) 183(6):2501–8. doi: 10.1084/jem.183.6.2501
13. Huang J, El-Gamil M, Dudley ME, Li YF, Rosenberg SA, Robbins PF. T Cells Associated With Tumor Regression Recognize Frameshifted Products of the CDKN2A Tumor Suppressor Gene Locus and a Mutated HLA Class I Gene Product. J Immunol (2004) 172(10):6057–64. doi: 10.4049/jimmunol.172.10.6057
14. Lennerz V, Fatho M, Gentilini C, Frye RA, Lifke A, Ferel D, et al. The Response of Autologous T Cells to a Human Melanoma Is Dominated by Mutated Neoantigens. Proc Natl Acad Sci USA (2005) 102(44):16013–8. doi: 10.1073/pnas.0500090102
15. Zhou J, Dudley ME, Rosenberg SA, Robbins PF. Persistence of Multiple Tumor-Specific T-Cell Clones Is Associated With Complete Tumor Regression in a Melanoma Patient Receiving Adoptive Cell Transfer Therapy. J Immunother (2005) 28(1):53–62. doi: 10.1097/00002371-200501000-00007
16. Ley TJ, Mardis ER, Ding L, Fulton B, McLellan MD, Chen K, et al. DNA Sequencing of a Cytogenetically Normal Acute Myeloid Leukaemia Genome. Nature (2008) 456(7218):66–72. doi: 10.1038/nature07485
17. Matsushita H, Vesely MD, Koboldt DC, Rickert CG, Uppaluri R, Magrini VJ, et al. Cancer Exome Analysis Reveals a T-Cell-Dependent Mechanism of Cancer Immunoediting. Nature (2012) 482(7385):400–4. doi: 10.1038/nature10755
18. Castle JC, Kreiter S, Diekmann J, Löwer M, van de Roemer N, de Graaf J, et al. Exploiting the Mutanome for Tumor Vaccination. Cancer Res (2012) 72(5):1081–91. doi: 10.1158/0008-5472.CAN-11-3722
19. Robbins PF, Lu YC, El-Gamil M, Li YF, Gross C, Gartner J, et al. Mining Exomic Sequencing Data to Identify Mutated Antigens Recognized by Adoptively Transferred Tumor-Reactive T Cells. Nat Med (2013) 19(6):747–52. doi: 10.1038/nm.3161
20. van Rooij N, van Buuren MM, Philips D, Velds A, Toebes M, Heemskerk B, et al. Tumor Exome Analysis Reveals Neoantigen-Specific T-Cell Reactivity in an Ipilimumab-Responsive Melanoma. J Clin Oncol (2013) 31(32):e439–42. doi: 10.1200/JCO.2012.47.7521
21. Gubin MM, Zhang X, Schuster H, Caron E, Ward JP, Noguchi T, et al. Checkpoint Blockade Cancer Immunotherapy Targets Tumour-Specific Mutant Antigens. Nature (2014) 515(7528):577–81. doi: 10.1038/nature13988
22. Tran E, Turcotte S, Gros A, Robbins PF, Lu YC, Dudley ME, et al. Cancer Immunotherapy Based on Mutation-Specific CD4+ T Cells in a Patient With Epithelial Cancer. Science (2014) 344(6184):641–5. doi: 10.1126/science.1251102
23. Chan TA, Wolchok JD, Snyder A. Genetic Basis for Clinical Response to CTLA-4 Blockade in Melanoma. N Engl J Med (2015) 373(20):1984. doi: 10.1056/NEJMc1508163
24. Rizvi NA, Hellmann MD, Snyder A, Kvistborg P, Makarov V, Havel JJ, et al. Cancer Immunology. Mutational Landscape Determines Sensitivity to PD-1 Blockade in Non-Small Cell Lung Cancer. Science (2015) 348(6230):124–8. doi: 10.1126/science.aaa1348
25. Carreno BM, Magrini V, Becker-Hapak M, Kaabinejadian S, Hundal J, Petti AA, et al. Cancer Immunotherapy. A Dendritic Cell Vaccine Increases the Breadth and Diversity of Melanoma Neoantigen-Specific T Cells. Science (2015) 348(6236):803–8. doi: 10.1126/science.aaa3828
26. Tran E, Robbins PF, Lu YC, Prickett TD, Gartner JJ, Jia L, et al. T-Cell Transfer Therapy Targeting Mutant KRAS in Cancer. N Engl J Med (2016) 375(23):2255–62. doi: 10.1056/NEJMoa1609279
27. McGranahan N, Furness AJ, Rosenthal R, Ramskov S, Lyngaa R, Saini SK, et al. Clonal Neoantigens Elicit T Cell Immunoreactivity and Sensitivity to Immune Checkpoint Blockade. Science (2016) 351(6280):1463–9. doi: 10.1126/science.aaf1490
28. Ott PA, Hu Z, Keskin DB, Shukla SA, Sun J, Bozym DJ, et al. An Immunogenic Personal Neoantigen Vaccine for Patients With Melanoma. Nature (2017) 547(7662):217–21. doi: 10.1038/nature22991
29. Sahin U, Derhovanessian E, Miller M, Kloke BP, Simon P, Löwer M, et al. Personalized RNA Mutanome Vaccines Mobilize Poly-Specific Therapeutic Immunity Against Cancer. Nature (2017) 547(7662):222–6. doi: 10.1038/nature23003
30. Ott PA, Hu-Lieskovan S, Chmielowski B, Govindan R, Naing A, Bhardwaj N, et al. A Phase Ib Trial of Personalized Neoantigen Therapy Plus Anti-PD-1 in Patients With Advanced Melanoma, Non-Small Cell Lung Cancer, or Bladder Cancer. Cell (2020) 183(2):347–62.e24. doi: 10.1016/j.cell.2020.08.053
31. Schumacher TN, Schreiber RD. Neoantigens in Cancer Immunotherapy. Science (2015) 348(6230):69–74. doi: 10.1126/science.aaa4971
32. Schumacher TN, Scheper W, Kvistborg P. Cancer Neoantigens. Annu Rev Immunol (2019) 37:173–200. doi: 10.1146/annurev-immunol-042617-053402
33. Wang Z, Cao YJ. Adoptive Cell Therapy Targeting Neoantigens: A Frontier for Cancer Research. Front Immunol (2020) 11:176. doi: 10.3389/fimmu.2020.00176
34. Li L, Goedegebuure SP, Gillanders WE. Preclinical and Clinical Development of Neoantigen Vaccines. Ann Oncol (2017) 28(suppl_12):xii11–7. doi: 10.1093/annonc/mdx681
35. Josephs TM, Grant EJ, Gras S. Molecular Challenges Imposed by MHC-I Restricted Long Epitopes on T Cell Immunity. Biol Chem (2017) 398(9):1027–36. doi: 10.1515/hsz-2016-0305
36. Rock KL, Reits E, Neefjes J. Present Yourself! By MHC Class I and MHC Class II Molecules. Trends Immunol (2016) 37(11):724–37. doi: 10.1016/j.it.2016.08.010
37. Szolek A, Schubert B, Mohr C, Sturm M, Feldhahn M, Kohlbacher O. Optitype: Precision HLA Typing From Next-Generation Sequencing Data. Bioinformatics (2014) 30(23):3310–6. doi: 10.1093/bioinformatics/btu548
38. Shukla SA, Rooney MS, Rajasagi M, Tiao G, Dixon PM, Lawrence MS, et al. Comprehensive Analysis of Cancer-Associated Somatic Mutations in Class I HLA Genes. Nat Biotechnol (2015) 33(11):1152–8. doi: 10.1038/nbt.3344
39. Boegel S, Löwer M, Schäfer M, Bukur T, de Graaf J, Boisguérin V, et al. HLA Typing From RNA-Seq Sequence Reads. Genome Med (2012) 4(12):102. doi: 10.1186/gm403
40. Liu C, Yang X, Duffy B, Mohanakumar T, Mitra RD, Zody MC, et al. ATHLATES: Accurate Typing of Human Leukocyte Antigen Through Exome Sequencing. Nucleic Acids Res (2013) 41(14):e142. doi: 10.1093/nar/gkt481
41. Ka S, Lee S, Hong J, Cho Y, Sung J, Kim HN, et al. Hlascan: Genotyping of the HLA Region Using Next-Generation Sequencing Data. BMC Bioinf (2017) 18(1):258. doi: 10.1186/s12859-017-1671-3
42. Buchkovich ML, Brown CC, Robasky K, Chai S, Westfall S, Vincent BG, et al. Hlaprofiler Utilizes K-Mer Profiles to Improve HLA Calling Accuracy for Rare and Common Alleles in RNA-Seq Data. Genome Med (2017) 9(1):86. doi: 10.1186/s13073-017-0473-6
43. Bai Y, Wang D, Fury W. PHLAT: Inference of High-Resolution HLA Types From RNA and Whole Exome Sequencing. Methods Mol Biol (2018) 1802:193–201. doi: 10.1007/978-1-4939-8546-3_13
44. Orenbuch R, Filip I, Comito D, Shaman J, Pe'er I, Rabadan R. Arcashla: High-Resolution HLA Typing From Rnaseq. Bioinformatics (2020) 36(1):33–40. doi: 10.1093/bioinformatics/btz474
45. Andreatta M, Nielsen M. Gapped Sequence Alignment Using Artificial Neural Networks: Application to the MHC Class I System. Bioinformatics (2016) 32(4):511–7. doi: 10.1093/bioinformatics/btv639
46. Nielsen M, Andreatta M. Netmhcpan-3.0; Improved Prediction of Binding to MHC Class I Molecules Integrating Information From Multiple Receptor and Peptide Length Datasets. Genome Med (2016) 8(1):33. doi: 10.1186/s13073-016-0288-x
47. Jurtz V, Paul S, Andreatta M, Marcatili P, Peters B, Nielsen M. Netmhcpan-4.0: Improved Peptide-MHC Class I Interaction Predictions Integrating Eluted Ligand and Peptide Binding Affinity Data. J Immunol (2017) 199(9):3360–8. doi: 10.4049/jimmunol.1700893
48. O’Donnell TJ, Rubinsteyn A, Bonsack M, Riemer AB, Laserson U, Hammerbacher J, et al. Mhcflurry: Open-Source Class I MHC Binding Affinity Prediction. Cell Syst (2018) 7(1):129–32.e4. doi: 10.1016/j.cels.2018.05.014
49. Rajasagi M, Shukla SA, Fritsch EF, Keskin DB, DeLuca D, Carmona E, et al. Systematic Identification of Personal Tumor-Specific Neoantigens in Chronic Lymphocytic Leukemia. Blood (2014) 124(3):453–62. doi: 10.1182/blood-2014-04-567933
50. Kreiter S, Vormehr M, van de Roemer N, Diken M, Löwer M, Diekmann J, et al. Mutant MHC Class II Epitopes Drive Therapeutic Immune Responses to Cancer. Nature (2015) 520(7549):692–6. doi: 10.1038/nature14426
51. Bassani-Sternberg M, Coukos G. Mass Spectrometry-Based Antigen Discovery for Cancer Immunotherapy. Curr Opin Immunol (2016) 41:9–17. doi: 10.1016/j.coi.2016.04.005
52. Yadav M, Jhunjhunwala S, Phung QT, Lupardus P, Tanguay J, Bumbaca S, et al. Predicting Immunogenic Tumour Mutations by Combining Mass Spectrometry and Exome Sequencing. Nature (2014) 515(7528):572–6. doi: 10.1038/nature14001
53. Hadrup SR, Bakker AH, Shu CJ, Andersen RS, van Veluw J, Hombrink P, et al. Parallel Detection of Antigen-Specific T-Cell Responses by Multidimensional Encoding of MHC Multimers. Nat Methods (2009) 6(7):520–6. doi: 10.1038/nmeth.1345
54. Slota M, Lim JB, Dang Y, Disis ML. Elispot for Measuring Human Immune Responses to Vaccines. Expert Rev Vaccines (2011) 10(3):299–306. doi: 10.1586/erv.10.169
55. Sahin U, Türeci Ö. Personalized Vaccines for Cancer Immunotherapy. Science (2018) 359(6382):1355–60. doi: 10.1126/science.aar7112
56. Keskin DB, Anandappa AJ, Sun J, Tirosh I, Mathewson ND, Li S, et al. Neoantigen Vaccine Generates Intratumoral T Cell Responses in Phase Ib Glioblastoma Trial. Nature (2019) 565(7738):234–9. doi: 10.1038/s41586-018-0792-9
57. Hilf N, Kuttruff-Coqui S, Frenzel K, Bukur V, Stevanović SA, outtefangeas C, et al. Actively Personalized Vaccination Trial for Newly Diagnosed Glioblastoma. Nature (2019) 565(7738):240–5. doi: 10.1038/s41586-018-0810-y
58. Hu Z, Leet DE, Allesøe RL, Oliveira G, Li S, Luoma AM, et al. Personal Neoantigen Vaccines Induce Persistent Memory T Cell Responses and Epitope Spreading in Patients With Melanoma. Nat Med (2021). 27(3):515–25. doi: 10.1038/s41591-020-01206-4
59. Tanyi JL, et al. Personalized Cancer Vaccine Effectively Mobilizes Antitumor T Cell Immunity in Ovarian Cancer. Sci Transl Med (2018) 10(436):eaao5931. doi: 10.1126/scitranslmed.aao5931
60. Kim VM, Pan X, Soares KC, Azad NS, Ahuja N, Gamper CJ, et al. Neoantigen-Based Epigvax Vaccine Initiates Antitumor Immunity in Colorectal Cancer. JCI Insight (2020) 5(9):e136368. doi: 10.1172/jci.insight.136368.
61. Yamamoto TN, Kishton RJ, Restifo NP. Developing Neoantigen-Targeted T Cell-Based Treatments for Solid Tumors. Nat Med (2019) 25(10):1488–99. doi: 10.1038/s41591-019-0596-y
62. Tran E, Ahmadzadeh M, Lu YC, Gros A, Turcotte S, Robbins PF, et al. Immunogenicity of Somatic Mutations in Human Gastrointestinal Cancers. Science (2015) 350(6266):1387–90. doi: 10.1126/science.aad1253
63. Stevanović S, Pasetto A, Helman SR, Gartner JJ, Prickett TD, Howie B, et al. Landscape of Immunogenic Tumor Antigens in Successful Immunotherapy of Virally Induced Epithelial Cancer. Science (2017) 356(6334):200–5. doi: 10.1126/science.aak9510
64. Zacharakis N, Chinnasamy H, Black M, Xu H, Lu YC, Zheng Z, et al. Immune Recognition of Somatic Mutations Leading to Complete Durable Regression in Metastatic Breast Cancer. Nat Med (2018) 24(6):724–30. doi: 10.1038/s41591-018-0040-8
65. Li L, Goedegebuure P, Mardis ER, Ellis MJ, Zhang X, Herndon JM, et al. Cancer Genome Sequencing and Its Implications for Personalized Cancer Vaccines. Cancers (Basel) (2011) 3(4):4191–211. doi: 10.3390/cancers3044191
66. Han XJ, Ma XL, Yang L, Wei YQ, Peng Y, Wei XW. Progress in Neoantigen Targeted Cancer Immunotherapies. Front Cell Dev Biol (2020) 8:728. doi: 10.3389/fcell.2020.00728
67. Wolchok JD, Hoos A, O'Day S, Weber JS, Hamid O, Lebbé C, et al. Guidelines for the Evaluation of Immune Therapy Activity in Solid Tumors: Immune-Related Response Criteria. Clin Cancer Res (2009) 15(23):7412–20. doi: 10.1158/1078-0432.CCR-09-1624
Keywords: neoantigen, immunotherapy, vaccine, adoptive cell therapy, personalized treatment
Citation: Zhu Y and Liu J (2021) The Role of Neoantigens in Cancer Immunotherapy. Front. Oncol. 11:682325. doi: 10.3389/fonc.2021.682325
Received: 18 March 2021; Accepted: 10 August 2021;
Published: 27 August 2021.
Edited by:
Min Cheng, Weifang Medical University, ChinaReviewed by:
Stéphanie Corgnac, Institut Gustave Roussy, FranceCopyright © 2021 Zhu and Liu. This is an open-access article distributed under the terms of the Creative Commons Attribution License (CC BY). The use, distribution or reproduction in other forums is permitted, provided the original author(s) and the copyright owner(s) are credited and that the original publication in this journal is cited, in accordance with accepted academic practice. No use, distribution or reproduction is permitted which does not comply with these terms.
*Correspondence: Jiyan Liu, bGl1aml5YW4xOTcyQDE2My5jb20=
Disclaimer: All claims expressed in this article are solely those of the authors and do not necessarily represent those of their affiliated organizations, or those of the publisher, the editors and the reviewers. Any product that may be evaluated in this article or claim that may be made by its manufacturer is not guaranteed or endorsed by the publisher.
Research integrity at Frontiers
Learn more about the work of our research integrity team to safeguard the quality of each article we publish.