- 1Department of Radiation Oncology, Brigham and Women’s Hospital, Dana-Farber Cancer Institute, Boston Children’s Hospital, Harvard Medical School, Boston, MA, United States
- 2Albany Medical College, Albany, NY, United States
- 3Department of Radiation Oncology, Dana-Farber Cancer Institute, Boston, MA, United States
Cancer cells rewire their metabolism to promote cell proliferation, invasion, and metastasis. Alterations in the lactate pathway have been characterized in diverse cancers, correlate with outcomes, and lead to many downstream effects, including decreasing oxidative stress, promoting an immunosuppressive tumor microenvironment, lipid synthesis, and building chemo- or radio-resistance. Radiotherapy is a key modality of treatment for many cancers and approximately 50% of patients with cancer will receive radiation for cure or palliation; thus, overcoming radio-resistance is important for improving outcomes. Growing research suggests that important molecular controls of the lactate pathway may serve as novel therapeutic targets and in particular, radiosensitizers. In this mini-review, we will provide an overview of lactate metabolism in cancer, discuss three important contributors to lactate metabolism (lactate dehydrogenase, monocarboxylate transporters, and mitochondrial pyruvate carrier), and present data that inhibition of these three pathways can lead to radiosensitization. Future research is needed to further understand critical regulators of lactate metabolism and explore clinical safety and efficacy of inhibitors of lactate dehydrogenase, monocarboxylate transporters, and mitochondrial pyruvate carrier alone and in combination with radiation.
Introduction
At the most fundamental level, cells must be self-reliant—producing sufficient ATP and biosynthetic compounds to fuel their ongoing survival and proliferation (1). However, the ability of tumor cells to reprogram their metabolic activity in order to promote their own survival is one of the defining hallmarks of cancer (2). Dating all the way back to the 1920s and the pioneering work of Otto Warburg, lactate has long been identified as a major player in cancer metabolism (3). In his work, Dr. Warburg noted that many cancer cells uptake large amounts of glucose and preferentially produce lactate through glycolytic pathways, even in the presence of oxygen (4). This phenomenon has been observed across many different neoplasms and serves as the basis for tumor detection using glucose tracers with positron emission tomography (PET) (5–9). Recent studies have further shown that lactate plays a critical role in fueling tumor progression, remodeling the tumor microenvironment (TME), and inducing treatment resistance (10). Even more so, research has begun to reveal how lactate metabolism may be used to influence the radiosensitivity of tumors (11–13). This mini-review will provide a general overview of lactate metabolism and its role within diverse cancers, and specifically, summarize recent studies that suggest an interplay between lactate metabolism and response to radiation.
Lactate Pathway
In normal human cellular physiology, glucose serves as a major source of lactate production (Figure 1). Glucose is most commonly taken into cells via facilitated diffusions through glucose transporter proteins (GLUT) (14). Once inside, glucose is phosphorylated to glucose-6-phosphate by hexokinase, effectively entrapping it in the cell (15). In the cytoplasm, glucose-6-phosphate is routed through several oxygen-independent glycolytic reactions to generate two ATP and two molecules of pyruvate, among other products (15). Under normal aerobic conditions, the majority of this pyruvate is then transported into the mitochondria via either mitochondrial pyruvate carrier (MPC) or after conversion to lactate via monocarboxylate transporters (MCTs), and undergoes oxidative phosphorylation, generating another 32 to 34 ATP per glucose molecule via the tricarboxylic acid cycle and electron transport chain (16, 17). However, under anaerobic conditions, cells are unable to rely on oxidative phosphorylation to balance their redox state, and pyruvate is preferentially converted to lactic acid through an enzymatic reaction catalyzed by cytosolic lactate dehydrogenase (LDH) (18). Within cells, lactic acid almost completely dissociates to lactate and H+, and lactic acid accumulation leads to acidification of the cytoplasm and potent inhibition of further glycolysis (18, 19). As such, proper physiological functioning depends on the efflux of lactate out of the cell, and transport across mitochondrial and cellular membranes requires MCTs. Once outside of a glycolytic cell, the excreted lactate is ultimately destined for one of several possible fates. Under physiological conditions, tissues like the heart, brain, and skeletal muscles can use lactate as a fuel source, while the liver can convert circulating lactate into glucose through the Cori cycle (20, 21). Indeed, a growing body of literature has shown that such “shuttling of lactate” between organs plays an important role in the overall regulation of metabolism (22).
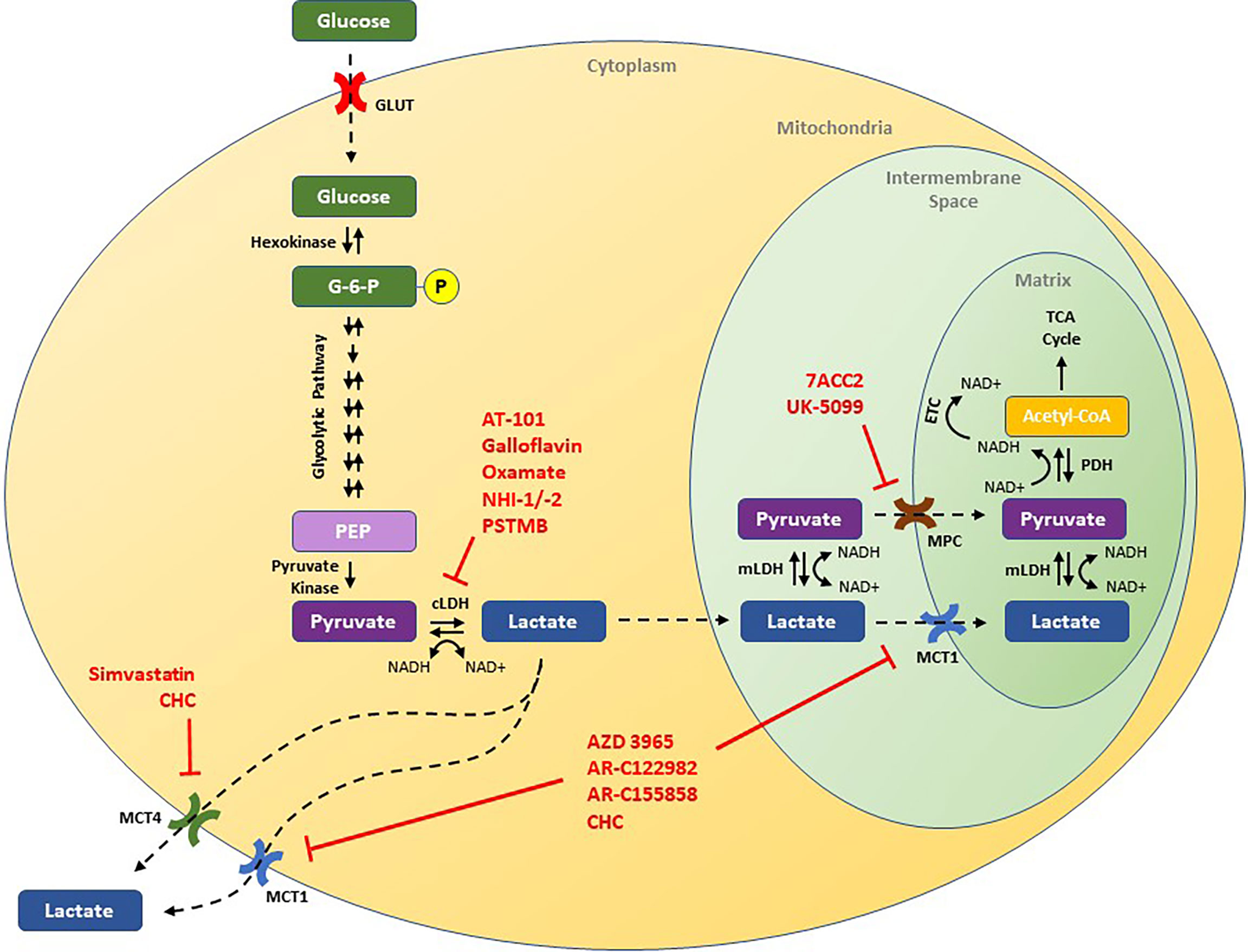
Figure 1 Schematic of the lactate pathway and illustration of novel therapeutic strategies that have been shown to decrease tumor growth in preclinical studies. Drugs discussed in this review are presented in red and are shown by their putative target of action. ECT, electron transport chain; G-6-P, glucose-6-phosphate; GLUT, glucose transporter; cLDH, cytosolic lactate dehydrogenase; mLDH, mitochondrial lactate dehydrogenase; MCT1, monocarboxylate transporter 1; MCT4, monocarboxylate transporter 4; MPC, mitochondrial pyruvate carrier; NAD+, nicotinamide adenine dinucleotide (oxidized); NADH, nicotinamide adenine dinucleotide (reduced); PEP, phosphoenolpyruvate; PDH, pyruvate dehydrogenase.
Lactate and Cancer
In blood and healthy tissues, the physiological concentration of lactate is roughly 1.0 to 3.0 mmol/L. However, in cancer cells, lactate concentrations may be up to an order of magnitude higher. Such high concentrations of lactate have been shown to arise primarily from enhanced rates of glycolysis (23). Interestingly, despite its inherent inefficiency in terms of ATP production, high rates of glycolysis have been observed in many cancer cells, even under fully aerobic conditions and with intact oxidative phosphorylation function (23). There are two major theories regarding the preferential dependence of cancer cells on glycolysis. First, the rate of ATP production through glycolysis is much more rapid than oxidative phosphorylation allowing cells to meet changing energy requirements, and second, glycolysis produces many intermediate biosynthetic molecules required by rapidly proliferating cells (24, 25). Regardless of teleology, there are a number of adaptive enzymatic alterations that lead to this so-called, “Warburg phenotype,” including changes in the function of hexokinase 2 (HK2), pyruvate kinase type M2 (PKM2), GLUT1, LDH, MCTs, and pyruvate dehydrogenase (PDH) (26–32). The resulting high concentrations of lactate have been further implicated in a wide range of tumoral aberrations, including changes in the TME, immune suppression, and metastasis—where increasing tumoral lactate concentrations are associated with an increased risk of metastatic dissemination (10, 33–39).
Radiotherapy is a curative treatment modality in diverse cancer types, including breast cancer (40, 41), head and neck cancers (42), brain cancer (43), and many pediatric solid tumors (44–46). Furthermore, radiotherapy remains an essential option for palliation such that approximately 50% of patients with cancer will receive radiation treatments during their disease course (47, 48). Recent studies have found that high rates of glycolysis and the build-up of lactate likely contribute to radioresistance in many tumor types through diverse mechanisms including antioxidant protective effects and promoting an immunosuppressive TME (39, 49–53). Interestingly, through impacts on several cellular processes including LDH and PDH, radiation itself can also promote lactate production, which, in turn, may drive a degree of radioresistance (39, 54–56). Within the extensive cellular machinery involved with lactate metabolism, three promising targets, LDH, MCT1/4, and MPC, have been shown to modulate radiosensitivity.
Lactate Dehydrogenase (LDH)
LDH is a nicotinamide adenine dinucleotide (NAD+) oxidoreductase enzyme that catalyzes the conversion between pyruvate and lactate (57). While constitutively active in aerobic conditions, LDH expression is upregulated in hypoxic environments via HIF-1α (58). LDH is a tetrameric enzyme comprised of 2 subunits that can combine in any of 5 combinations. The most common subtype, known as LDHA, preferentially reduces pyruvate to lactate, and is frequently over-expressed in many tumors (10). In addition, LDHA has been shown to catalyze a number of “non-canonical” reactions, including the formation of an “onco-metabolite”—2-hydroxyglutarate—in acidic and anaerobic environments, thus promoting oncogenesis (59–65). Recent studies demonstrate that 2-hydroxyglutarate can promote a transcriptional program of genes that regulate proliferation and growth by inhibiting histone demethylation and TET-mediated DNA demethylation (59–65). Clinically, increased tumoral LDHA expression is associated with poorer clinical outcomes and recent studies suggest it may serve as a prognostic biomarker (66–68).
Knockdown studies of both PKM2 and LDHA have been shown to reduce ATP production, inhibit cell growth, decrease invasiveness, and induce oxidative stress and radiosensitivity in cancer cells (39, 69, 70). Several clinical and pre-clinical studies have further analyzed the effects of both selective and non-selective inhibitors of LDHA on cancer cells. AT-101, a naturally occurring compound derived from cottonseed, is an oral non-selective inhibitor of LDH that additionally inhibits the anti-apoptotic proteins Bcl-2, Bcl-xL, Bcl-W, and Mcl-1 while simultaneously stimulating pro-apoptotic signaling (71, 72). A study of AT-101 monotherapy in 23 men with metastatic castrate-resistant prostate cancer showed that a dose of 20 mg/day was well tolerated and led to a >50% decrease in PSA in roughly 9% of patients (71). Heist and colleagues found that while AT-101 administered concurrently with topotecan was safe for patients with small cell lung cancer (SCLC) who had failed prior platinum-based chemotherapy, this regimen failed to show significant activity with only 8% of patients experiencing a partial response (73). Similarly, Baggstrom et al. failed to demonstrate efficacy in patients with chemo-sensitive recurrent SCLC (74). However, in pre-clinical studies, FX11—a derivative of AT-101 that selectively inhibits LDHA over LDHB—effectively inhibited tumorigenesis in vivo using human lymphoma and pancreatic tumor xenograft models (38). When combined with a small molecule inhibitor of NAD+ synthesis, FX11 was further able to induce tumor regression in the lymphoma xenograft model (38). Another study found that both galloflavin, a polyphenol inhibitor of LDH, and oxamate, a competitive analogue of pyruvate, disrupted the heat shock response in cultured hepatocellular carcinoma (HCC) cells and induced cellular senescence (75). Furthermore, two recent studies found that inhibition of LDHA in glioblastoma cell lines with either oxamate or the selective inhibitors, NHI-1 and NHI-2, improved chemotherapy and radiation sensitivity and triggered apoptosis and differentiation of cancer stem cells (76, 77). More recently, PSTMB—a novel allosteric inhibitor of LDH—was found to reduce cellular proliferation in in vitro models of lung cancer, breast cancer, melanoma, HCC, and colon cancer (78). In cultured colon cancer cells, PSTMB reduced LDH activity in both aerobic and anaerobic conditions without altering LDH expression, and increased reactive oxygen species (ROS) formation (78).
Despite clinical and pre-clinical interest in LDH inhibitors, there have been few studies of these agents in combination with radiotherapy. Koukourakis, et al. assessed the effects of LDH blockade on the treatment sensitivity of 2 glioblastoma cell lines, U87MG and the more radio-resistant T98G. Silencing LDHA gene expression or inhibiting LDH with oxamate led to enhanced sensitivity to both radiation and temozolomide, with more pronounced effects observed in the T98G cell line (76). Another study by Zhai and colleagues showed that oxamate increased radiation sensitivity primarily by enhancing mitochondrial ROS generation, which in turn promoted apoptosis in two nasopharyngeal cancer cell lines (79). Yang et al. found that radiotherapy increased lactate concentrations in the TME which then led to localized immunosuppression via MDSCs in murine models with explanted human pancreatic cancer cells, and administration of the selective LDHA inhibitor GSK2837808A concurrently with radiation improved antitumoral T-cell response and reduced tumor progression (39). These results suggest that lactate is at least partially responsible for the observed radiotherapy-induced immunosuppression. In a different tact, Judge et al. observed that high lactate concentrations activated latent TGF-β, leading to excessive fibrosis and found that increased LDHA expression correlated with higher rates of pulmonary fibrosis in patients treated with radiotherapy (80). Treatment with AT-101 four weeks after exposing C57BL/6 mice to total-body and thoracic radiation showed significantly decreased TGF-β expression and rates of pulmonary fibrosis (80). Two early phase clinical trials are examining the safety of concurrent chemoradiation with AT-101 in glioblastoma and esophageal and esophagogastric junction cancers (Table 1). Overall, these results highlight many of the potential advantages of LDH inhibitors in combination with radiotherapy; however, significant work still remains in order to determine clinical utility.
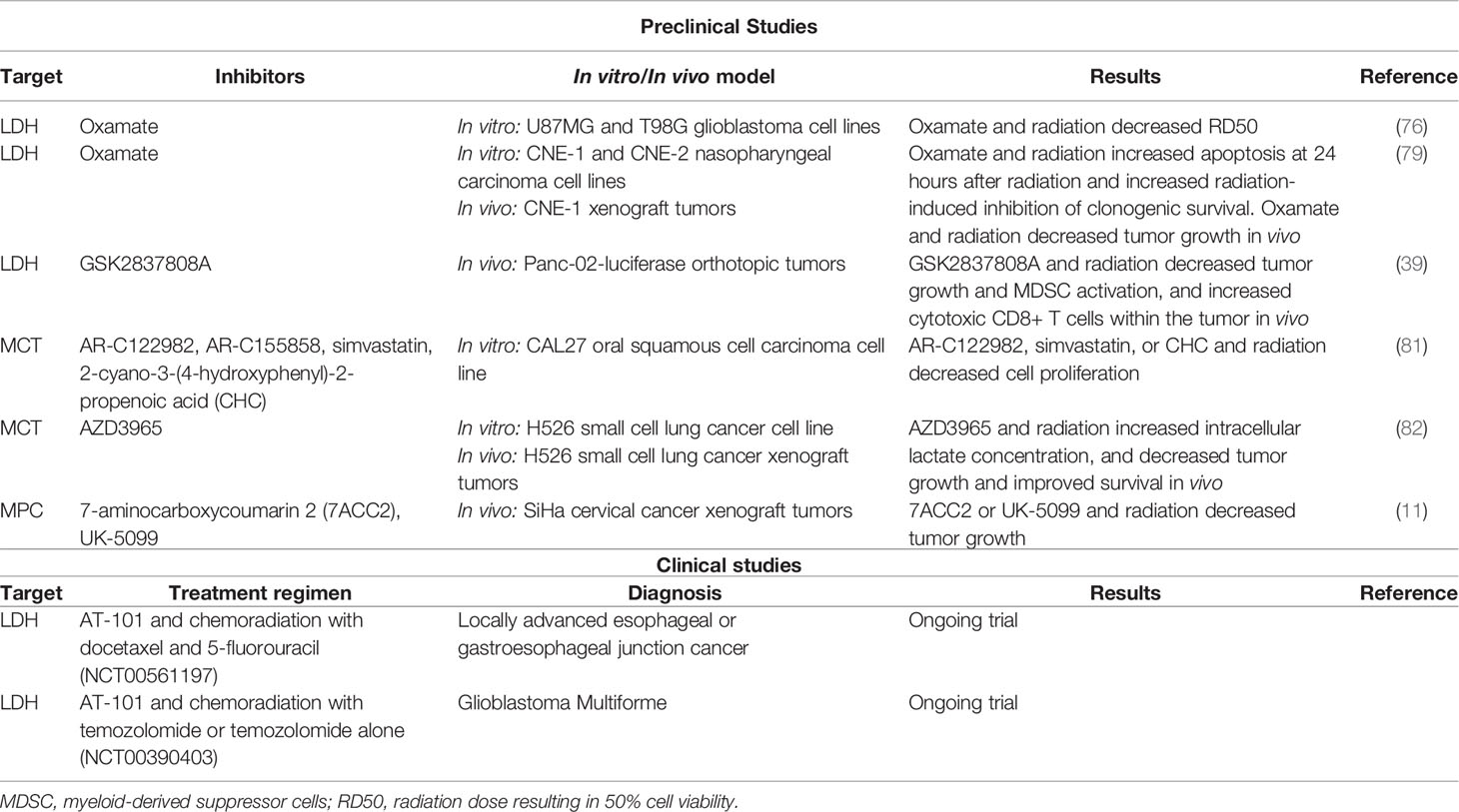
Table 1 Preclinical studies and clinical trials exploring lactate pathway targets with radiotherapy in cancer.
Monocarboxylate Transporters
MCTs constitute 14 isoforms of membrane transport proteins that aid in the absorption and efflux of a wide range of biological compounds (83). Ubiquitously expressed, MCT1 primarily mediates import of lactate along with other monocarboxylates (84–87). On the other hand, MCT4 expression is regulated by hypoxia through a HIF-1α-dependent mechanism. MCT4 has lower affinity for lactate compared to MCT1, and predominantly participates in lactate efflux (84–87). MCT1 and MCT4 are overexpressed in many cancer types, and their upregulation correlates with worse overall prognosis (17, 88–91). MCT1 and MCT4 may help not only maintain metabolic balance for oxidative and glycolytic cancer cells, respectively, but may also promote an immunosuppressive milieu by increasing the acidity of the TME secondary to the accumulation of lactate (92, 93). Increased acidity has been found to decrease CD8+ T-cell cytotoxicity and CD8+ T-cell-mediated cytokine release; and induce macrophage polarization to an immunosuppressive M2 state (3, 94). Thus, studies have investigated MCTs as a therapeutic target and in particular, a radiosensitizer, in various cancers (81, 82, 89, 95–98).
Numerous studies have found that knockdown or inhibition of MCT1 and/or MCT4 decreases lactate levels and tumor cell growth, migration, and invasion in vitro and in vivo for diverse cancers, including bladder cancer, breast cancer, colon cancer, glioblastoma, and liver cancer (89, 95–100). Interestingly, in a model of Burkitt’s lymphoma, a selective small molecule inhibitor of MCT1, AZD3965, also decreased lipid biosynthesis after lactate build-up, and specifically, levels of phosphocholine were significantly decreased by inhibition of choline kinase α expression and de novo phosphocholine synthesis. Furthermore, in the TME, AZD3965-treated tumors also displayed greater interaction with dendritic cells—increasing tumor antigen presentation—and natural killer cells—leading to direct killing of tumor cells (101).
Two studies have examined the combination of MCT inhibition and radiation in tumor models. Brandstetter et al. studied the effects of multiple MCT inhibitors with or without radiation on the oral squamous cell carcinoma (SCC) cell line CAL27 in vitro. Specifically, they analyzed MCT1 inhibitors, AR-C122982 and AR-C155858, the MCT4 inhibitor, simvastatin, and the non-specific MCT inhibitor, 2-cyano-3-(4-hydroxyphenyl)-2-propenoic acid (CHC) (81). Though the MCT inhibitors differed in their potency and efficacy, treatment decreased cell proliferation, viability, and wound healing (81). The combination of radiation with specific MCT inhibitors further resulted in enhanced anti-proliferative activity (81). Currently, it is not known whether these other pathways may further contribute to the effects seen in this study.
Many studies have found that AZD3965, a selective small molecule inhibitor of MCT1, is effective in inhibiting tumor growth in many different preclinical models of cancer (82, 99–104). Two studies demonstrated that AZD3965 inhibited bidirectional lactate transport leading to both accumulation of intracellular lactate (with greater effects observed in hypoxic conditions), and antitumor activity in SCLC models in vitro and in xenograft models (82, 99). Bola et al. found that radiation alone did not affect intracellular lactate in H526 SCLC cells, but when delivered in combination with AZD3965, intracellular lactate concentration significantly increased (82). Furthermore, compared with radiation alone, AZD3965 for seven days with concurrent radiation delivered on days 3-5 significantly decreased tumor growth and improved survival with one mouse showing no tumor recurrence (82). Unfortunately, MCT1 inhibitors are ineffective in tumor cells that highly express MCT4, which suggests that MCT4 expression may serve as a biomarker for patient selection and predictor of response to anti-MCT1 therapy (98, 99).
Of the MCT1 inhibitors, AZD3965 has entered early phase clinical trials. Preliminary results from a phase I study investigating the safety of AZD3965 in patients with refractory advanced solid malignancies found that AZD3965 was well tolerated. While the most common side effects were nausea and fatigue, patients also experienced expected on-target effects of retinal electroretinographic changes that were dose-limiting at 20 mg daily and increased urinary ketones (105). One patient had exacerbation of previously undiagnosed tumor-associated lactic acidosis, which was dose-limiting (105). Future research is still needed to explore the tolerability and efficacy of AZD3965 and other MCT inhibitors alone and in combination with radiation.
Mitochondrial Pyruvate Carrier
MPC is formed by two proteins encoded by genes MPC1 and MPC2. It transports pyruvate from the cytoplasm into mitochondria, and sits at the crossroads of glycolysis, mitochondrial oxidative phosphorylation, and lactate production (106, 107). In highly glycolytic tumors, decreased MPC expression can lead to aerobic glycolysis and shunting to glutaminolysis, ultimately leading to greater tumor proliferation. On the other hand, tumors that are more dependent on oxidative phosphorylation may be more sensitive to alterations in MPC-mediated pyruvate transport (106). Recent studies have also found that lactate accumulation in tumors can promote the synthesis of intermediates of the tricarboxylic acid cycle, further supporting cell proliferation (12, 108, 109). Thus, there is growing interest in MPC as a regulator of both oxidative phosphorylation and lactate production in tumorigenesis.
Recently, 7-aminocarboxycoumarin 2 (7ACC2) was identified as a novel MPC inhibitor that led to downstream reductions in lactate influx and delays in tumor growth within in vitro models of cervical cancer, colorectal cancer, breast cancer, hypopharyngeal SCC, and pancreatic cancer (11, 110–112). Corbet et al. found that 7ACC2 blocked MPC activity, thereby inhibiting pyruvate metabolism and subsequently blocking lactate influx consistent with another known MPC inhibitor, UK-5099 (11). In a spheroid model using FaDu hypopharyngeal SCC cells, treatment with MPC inhibitors produced cytotoxic effects and led to decreased hypoxia in the spheroids (11). In SiHa cervical cancer xenograft models, the combination of 7AAC2 with radiation using either 16 Gy in one fraction or 20 Gy in five fractions, led to significantly decreased tumor growth compared with 7AAC2 or radiation monotherapy. Similar results were also observed in vivo using shRNA targeting MPC1 or UK-5099 (11). These preclinical data suggest that MPC represents a novel target warranting further clinical investigation both alone and in combination with radiation.
Future Directions
Many preclinical studies have identified multiple targets within the lactate metabolic pathway that play a role in radiosensitization, and future research is ongoing to identify novel targets for lactate metabolism. Studies exploring safety of these targets are still needed, particularly for patients at risk for metabolic acidosis either from co-morbidities or prior cancer therapy. Furthermore, it remains important to note that different solid tumors may have unique alterations in lactate metabolism and intratumoral metabolic heterogeneity may also cause differential response to inhibition of lactate metabolism (10, 108, 113, 114). These characteristics are important considerations for future studies and increasingly support identifying tumor types in which harnessing radiosensitizing properties through lactate metabolism inhibition has the greatest therapeutic benefit. For example, additional imaging techniques, such as 13C magnetic resonance spectroscopy, can better provide dynamic imaging of lactate metabolic reprogramming (115–117). A recent clinical trial explored de-escalation of radiation to 30 Gy for patients with human papillomavirus-associated oropharyngeal tumors who had no hypoxia at baseline using dynamic fluorine-18-labeled fluoromisonidazole PET or resolution of hypoxia during intratreatment PET; while patients with persistent hypoxia received 70 Gy (118). Identifying patients with hypoxic tumors or tumors with specific alterations of lactate metabolism may allow for improved patient selection for future clinical trials involving radiation and inhibitors of lactate metabolic pathways.
Conclusion
A growing body of evidence has shown that, in addition to its use as a fuel source, lactate also promotes tumor growth. Interestingly, elevated lactate levels and lactate-mediated downstream pathways can cause changes in transcriptional programming (59–65), tumor immune microenvironment (10, 39), lipid synthesis (101), among others (3). The effects of these downstream changes, particularly with regards to decreasing the levels of ROS, can contribute to radio-resistance (3). Recent studies have found that inhibitors of LDH, MCT, and MPC can serve as radiosensitizers in models of glioblastoma, pancreatic cancer, SCLC and cervical cancer (11, 39, 76, 82). There remains limited clinical investigation of these inhibitors with radiation as only two early phase clinical trials are studying AT-101 in combination with radiation (Table 1). Future research is needed to understand the mechanisms by which regulators of lactate metabolism promote tumorigenesis, identify tumor subtypes that are uniquely dependent on lactate pathways, and to further explore targeted inhibitors of this pathway in preclinical and clinical studies.
Author Contributions
KL, SP, DH-K, and MM contributed to the conception and design of this study. KL, EE, and MM wrote the first draft of the manuscript. All authors contributed to the article and approved the submitted version.
Conflict of Interest
The authors declare that the research was conducted in the absence of any commercial or financial relationships that could be construed as a potential conflict of interest.
Publisher’s Note
All claims expressed in this article are solely those of the authors and do not necessarily represent those of their affiliated organizations, or those of the publisher, the editors and the reviewers. Any product that may be evaluated in this article, or claim that may be made by its manufacturer, is not guaranteed or endorsed by the publisher.
References
1. Hanahan D, Weinberg RA. The Hallmarks of Cancer. Cell (2000) 100(1):57–70. doi: 10.1016/S0092-8674(00)81683-9
2. Hanahan D, Weinberg RA. Hallmarks of Cancer: The Next Generation. Cell (2011) 144(5):646–74. doi: 10.1016/j.cell.2011.02.013
3. Hirschhaeuser F, Sattler UG, Mueller-Klieser W. Lactate: A Metabolic Key Player in Cancer. Cancer Res (2011) 71(22):6921–5. doi: 10.1158/0008-5472.CAN-11-1457
4. Warburg O, Wind F, Negelein E. The Metabolism of Tumors in the Body. J Gen Physiol (1927) 8(6):519–30. doi: 10.1085/jgp.8.6.519
5. Jiao L, Zhang HL, Li DD, Yang KL, Tang J, Li X, et al. Regulation of Glycolytic Metabolism by Autophagy in Liver Cancer Involves Selective Autophagic Degradation of HK2 (Hexokinase 2). Autophagy (2018) 14(4):671–84. doi: 10.1080/15548627.2017.1381804
6. Liu Y, Murray-Stewart T, Casero RA Jr., Kagiampakis I, Jin L, Zhang J, et al. Targeting Hexokinase 2 Inhibition Promotes Radiosensitization in HPV16 E7-Induced Cervical Cancer and Suppresses Tumor Growth. Int J Oncol (2017) 50(6):2011–23. doi: 10.3892/ijo.2017.3979
7. Marshall MJ, Neal FE, Goldberg DM. Isoenzymes of Hexokinase, 6-Phosphogluconate Dehydrogenase, Phosphoglucomutase and Lactate Dehydrogenase in Uterine Cancer. Br J Cancer (1979) 40(3):380–90. doi: 10.1038/bjc.1979.192
8. Wadsak W, Mitterhauser M. Basics and Principles of Radiopharmaceuticals for PET/Ct. Eur J Radiol (2010) 73(3):461–9. doi: 10.1016/j.ejrad.2009.12.022
9. Liberti MV, Locasale JW. The Warburg Effect: How Does it Benefit Cancer Cells? Trends Biochem Sci (2016) 41(3):211–8. doi: 10.1016/j.tibs.2015.12.001
10. de la Cruz-Lopez KG, Castro-Munoz LJ, Reyes-Hernandez DO, Garcia-Carranca A, Manzo-Merino J. Lactate in the Regulation of Tumor Microenvironment and Therapeutic Approaches. Front Oncol (2019) 9:1143. doi: 10.3389/fonc.2019.01143
11. Corbet C, Bastien E, Draoui N, Doix B, Mignion L, Jordan BF, et al. Interruption of Lactate Uptake by Inhibiting Mitochondrial Pyruvate Transport Unravels Direct Antitumor and Radiosensitizing Effects. Nat Commun (2018) 9(1):1208. doi: 10.1038/s41467-018-03525-0
12. Linden CV, Corbet C. Killing Two Birds With One Stone: Blocking the Mitochondrial Pyruvate Carrier to Inhibit Lactate Uptake by Cancer Cells and Radiosensitize Tumors. Mol Cell Oncol (2018) 5(4):e1465016. doi: 10.1080/23723556.2018.1465016
13. Mathupala SP, Colen CB, Parajuli P, Sloan AE. Lactate and Malignant Tumors: A Therapeutic Target at the End Stage of Glycolysis. J Bioenerg Biomembr (2007) 39(1):73–7. doi: 10.1007/s10863-006-9062-x
14. Navale AM, Paranjape AN. Glucose Transporters: Physiological and Pathological Roles. Biophys Rev (2016) 8(1):5–9. doi: 10.1007/s12551-015-0186-2
15. Lincet H, Icard P. How do Glycolytic Enzymes Favour Cancer Cell Proliferation by Nonmetabolic Functions? Oncogene (2015) 34(29):3751–9. doi: 10.1038/onc.2014.320
16. McCommis KS, Finck BN. Mitochondrial Pyruvate Transport: A Historical Perspective and Future Research Directions. Biochem J (2015) 466(3):443–54. doi: 10.1042/BJ20141171
17. Payen VL, Mina E, Van Hee VF, Porporato PE, Sonveaux P. Monocarboxylate Transporters in Cancer. Mol Metab (2020) 33:48–66. doi: 10.1016/j.molmet.2019.07.006
18. Rogatzki MJ, Ferguson BS, Goodwin ML, Gladden LB. Lactate is Always the End Product of Glycolysis. Front Neurosci (2015) 9:22. doi: 10.3389/fnins.2015.00022
19. Trivedi B, Danforth WH. Effect of pH on the Kinetics of Frog Muscle Phosphofructokinase. J Biol Chem (1966) 241(17):4110–2. doi: 10.1016/S0021-9258(18)99819-4
20. Gladden LB. Lactate Metabolism: A New Paradigm for the Third Millennium. J Physiol (2004) 558(Pt 1):5–30. doi: 10.1113/jphysiol.2003.058701
21. Neufeld EF, Ginsburg V. Carbohydrate Metabolism. Annu Rev Biochem (1965) 34:297–312. doi: 10.1146/annurev.bi.34.070165.001501
22. Brooks GA. Cell-Cell and Intracellular Lactate Shuttles. J Physiol (2009) 587(Pt 23):5591–600. doi: 10.1113/jphysiol.2009.178350
23. Yu L, Chen X, Sun X, Wang L, Chen S. The Glycolytic Switch in Tumors: How Many Players Are Involved? J Cancer (2017) 8(17):3430–40. doi: 10.7150/jca.21125
24. Hamanaka RB, Chandel NS. Targeting Glucose Metabolism for Cancer Therapy. J Exp Med (2012) 209(2):211–5. doi: 10.1084/jem.20120162
25. Locasale JW, Cantley LC. Altered Metabolism in Cancer. BMC Biol (2010) 8:88. doi: 10.1186/1741-7007-8-88
26. Fantin VR, St-Pierre J, Leder P. Attenuation of LDH-A Expression Uncovers a Link Between Glycolysis, Mitochondrial Physiology, and Tumor Maintenance. Cancer Cell (2006) 9(6):425–34. doi: 10.1016/j.ccr.2006.04.023
27. Mayer A, Schmidt M, Seeger A, Serras AF, Vaupel P, Schmidberger H. GLUT-1 Expression Is Largely Unrelated to Both Hypoxia and the Warburg Phenotype in Squamous Cell Carcinomas of the Vulva. BMC Cancer (2014) 14:760. doi: 10.1186/1471-2407-14-760
28. Shim H, Dolde C, Lewis BC, Wu CS, Dang G, Jungmann RA, et al. C-Myc Transactivation of LDH-A: Implications for Tumor Metabolism and Growth. Proc Natl Acad Sci USA (1997) 94(13):6658–63. doi: 10.1073/pnas.94.13.6658
29. Wang L, Wang J, Xiong H, Wu F, Lan T, Zhang Y, et al. Co-Targeting Hexokinase 2-Mediated Warburg Effect and ULK1-Dependent Autophagy Suppresses Tumor Growth of PTEN- and TP53-Deficiency-Driven Castration-Resistant Prostate Cancer. EBioMedicine (2016) 7:50–61. doi: 10.1016/j.ebiom.2016.03.022
30. Wang L, Xiong H, Wu F, Zhang Y, Wang J, Zhao L, et al. Hexokinase 2-Mediated Warburg Effect Is Required for PTEN- and P53-Deficiency-Driven Prostate Cancer Growth. Cell Rep (2014) 8(5):1461–74. doi: 10.1016/j.celrep.2014.07.053
31. Wong N, Ojo D, Yan J, Tang D. PKM2 Contributes to Cancer Metabolism. Cancer Lett (2015) 356(2 Pt A):184–91. doi: 10.1016/j.canlet.2014.01.031
32. de Bari L, Atlante A. Including the Mitochondrial Metabolism of L-Lactate in Cancer Metabolic Reprogramming. Cell Mol Life Sci (2018) 75(15):2763–76. doi: 10.1007/s00018-018-2831-y
33. Brizel DM, Schroeder T, Scher RL, Walenta S, Clough RW, Dewhirst MW, et al. Elevated Tumor Lactate Concentrations Predict for an Increased Risk of Metastases in Head-and-Neck Cancer. Int J Radiat Oncol Biol Phys (2001) 51(2):349–53. doi: 10.1016/S0360-3016(01)01630-3
34. Lee DC, Sohn HA, Park ZY, Oh S, Kang YK, Lee KM, et al. A Lactate-Induced Response to Hypoxia. Cell (2015) 161(3):595–609. doi: 10.1016/j.cell.2015.03.011
35. Walenta S, Salameh A, Lyng H, Evensen JF, Mitze M, Rofstad EK, et al. Correlation of High Lactate Levels in Head and Neck Tumors With Incidence of Metastasis. Am J Pathol (1997) 150(2):409–15.
36. Walenta S, Wetterling M, Lehrke M, Schwickert G, Sundfor K, Rofstad EK, et al. High Lactate Levels Predict Likelihood of Metastases, Tumor Recurrence, and Restricted Patient Survival in Human Cervical Cancers. Cancer Res (2000) 60(4):916–21.
37. Doherty JR, Cleveland JL. Targeting Lactate Metabolism for Cancer Therapeutics. J Clin Invest (2013) 123(9):3685–92. doi: 10.1172/JCI69741
38. Le A, Cooper CR, Gouw AM, Dinavahi R, Maitra A, Deck LM, et al. Inhibition of Lactate Dehydrogenase A Induces Oxidative Stress and Inhibits Tumor Progression. Proc Natl Acad Sci USA (2010) 107(5):2037–42. doi: 10.1073/pnas.0914433107
39. Yang X, Lu Y, Hang J, Zhang J, Zhang T, Huo Y, et al. Lactate-Modulated Immunosuppression of Myeloid-Derived Suppressor Cells Contributes to the Radioresistance of Pancreatic Cancer. Cancer Immunol Res (2020) 8(11):1440–51. doi: 10.1158/2326-6066.CIR-20-0111
40. Early Breast Cancer Trialists’ Collaborative G, Darby S, McGale P, Correa C, Taylor C, Arriagada R, et al. Effect of Radiotherapy After Breast-Conserving Surgery on 10-Year Recurrence and 15-Year Breast Cancer Death: Meta-Analysis of Individual Patient Data for 10,801 Women in 17 Randomised Trials. Lancet (2011) 378(9804):1707–16. doi: 10.1016/S0140-6736(11)61629-2
41. Ebctcg, McGale P, Taylor C, Correa C, Cutter D, Duane F, et al. Effect of Radiotherapy After Mastectomy and Axillary Surgery on 10-Year Recurrence and 20-Year Breast Cancer Mortality: Meta-Analysis of Individual Patient Data for 8135 Women in 22 Randomised Trials. Lancet (2014) 383(9935):2127–35. doi: 10.1016/S0140-6736(14)60488-8
42. Yeh SA. Radiotherapy for Head and Neck Cancer. Semin Plast Surg (2010) 24(2):127–36. doi: 10.1055/s-0030-1255330
43. Stupp R, Mason WP, van den Bent MJ, Weller M, Fisher B, Taphoorn MJ, et al. Radiotherapy Plus Concomitant and Adjuvant Temozolomide for Glioblastoma. N Engl J Med (2005) 352(10):987–96. doi: 10.1056/NEJMoa043330
44. Haas-Kogan DA, Devine CA, Liu KX, Terezakis SA. A Cautionary Tale: Risks of Radiation Therapy De-Escalation in Pediatric Malignancies. J Clin Oncol (2017) 35(22):2471–2. doi: 10.1200/JCO.2017.73.3485
45. Liu KX, Naranjo A, Zhang FF, DuBois SG, Braunstein SE, Voss SD, et al. Prospective Evaluation of Radiation Dose Escalation in Patients With High-Risk Neuroblastoma and Gross Residual Disease After Surgery: A Report From the Children’s Oncology Group ANBL0532 Study. J Clin Oncol (2020). 38(24):2741–52. JCO1903316. doi: 10.1200/JCO.19.03316
46. Merchant TE, Bendel AE, Sabin ND, Burger PC, Shaw DW, Chang E, et al. Conformal Radiation Therapy for Pediatric Ependymoma, Chemotherapy for Incompletely Resected Ependymoma, and Observation for Completely Resected, Supratentorial Ependymoma. J Clin Oncol (2019) 37(12):974–83. doi: 10.1200/JCO.18.01765
47. Barnett GC, West CM, Dunning AM, Elliott RM, Coles CE, Pharoah PD, et al. Normal Tissue Reactions to Radiotherapy: Towards Tailoring Treatment Dose by Genotype. Nat Rev Cancer (2009) 9(2):134–42. doi: 10.1038/nrc2587
48. Delaney G, Jacob S, Featherstone C, Barton M. The Role of Radiotherapy in Cancer Treatment: Estimating Optimal Utilization From a Review of Evidence-Based Clinical Guidelines. Cancer (2005) 104(6):1129–37. doi: 10.1002/cncr.21324
49. Sattler UG, Meyer SS, Quennet V, Hoerner C, Knoerzer H, Fabian C, et al. Glycolytic Metabolism and Tumour Response to Fractionated Irradiation. Radiother Oncol (2010) 94(1):102–9. doi: 10.1016/j.radonc.2009.11.007
50. Grotius J, Dittfeld C, Huether M, Mueller-Klieser W, Baumann M, Kunz-Schughart LA. Impact of Exogenous Lactate on Survival and Radioresponse of Carcinoma Cells In Vitro. Int J Radiat Biol (2009) 85(11):989–1001. doi: 10.3109/09553000903242156
51. Quennet V, Yaromina A, Zips D, Rosner A, Walenta S, Baumann M, et al. Tumor Lactate Content Predicts for Response to Fractionated Irradiation of Human Squamous Cell Carcinomas in Nude Mice. Radiother Oncol (2006) 81(2):130–5. doi: 10.1016/j.radonc.2006.08.012
52. Shimura T, Noma N, Sano Y, Ochiai Y, Oikawa T, Fukumoto M, et al. AKT-Mediated Enhanced Aerobic Glycolysis Causes Acquired Radioresistance by Human Tumor Cells. Radiother Oncol (2014) 112(2):302–7. doi: 10.1016/j.radonc.2014.07.015
53. Groussard C, Morel I, Chevanne M, Monnier M, Cillard J, Delamarche A. Free Radical Scavenging and Antioxidant Effects of Lactate Ion: An In Vitro Study. J Appl Physiol (1985) (2000) 89(1):169–75. doi: 10.1152/jappl.2000.89.1.169
54. Dittmann K, Mayer C, Paasch A, Huber S, Fehrenbacher B, Schaller M, et al. Nuclear EGFR Renders Cells Radio-Resistant by Binding mRNA Species and Triggering a Metabolic Switch to Increase Lactate Production. Radiother Oncol (2015) 116(3):431–7. doi: 10.1016/j.radonc.2015.08.016
55. Dittmann K, Mayer C, Rodemann HP. EP-2064: Radiation Induces Metabolic Switch to Lactate Production to Support Tumour Cell Survival. Radiother Oncol (2016) 119:S974. doi: 10.1016/S0167-8140(16)33315-1
56. Tang L, Wei F, Wu Y, He Y, Shi L, Xiong F, et al. Role of Metabolism in Cancer Cell Radioresistance and Radiosensitization Methods. J Exp Clin Cancer Res (2018) 37(1):87. doi: 10.1186/s13046-018-0758-7
57. Adeva-Andany M, Lopez-Ojen M, Funcasta-Calderon R, Ameneiros-Rodriguez E, Donapetry-Garcia C, Vila-Altesor M, et al. Comprehensive Review on Lactate Metabolism in Human Health. Mitochondrion (2014) 17:76–100. doi: 10.1016/j.mito.2014.05.007
58. Mauceri HJ, Sutton HG, Darga TE, Kocherginsky M, Kochanski J, Weichselbaum RR, et al. Everolimus Exhibits Efficacy as a Radiosensitizer in a Model of non-Small Cell Lung Cancer. Oncol Rep (2012) 27(5):1625–9. doi: 10.3892/or.2012.1666
59. Dang L, White DW, Gross S, Bennett BD, Bittinger MA, Driggers EM, et al. Cancer-Associated IDH1 Mutations Produce 2-Hydroxyglutarate. Nature (2009) 462(7274):739–44. doi: 10.1038/nature08617
60. Chowdhury R, Yeoh KK, Tian YM, Hillringhaus L, Bagg EA, Rose NR, et al. The Oncometabolite 2-Hydroxyglutarate Inhibits Histone Lysine Demethylases. EMBO Rep (2011) 12(5):463–9. doi: 10.1038/embor.2011.43
61. Figueroa ME, Abdel-Wahab O, Lu C, Ward PS, Patel J, Shih A, et al. Leukemic IDH1 and IDH2 Mutations Result in a Hypermethylation Phenotype, Disrupt TET2 Function, and Impair Hematopoietic Differentiation. Cancer Cell (2010) 18(6):553–67. doi: 10.1016/j.ccr.2010.11.015
62. Turcan S, Rohle D, Goenka A, Walsh LA, Fang F, Yilmaz E, et al. IDH1 Mutation is Sufficient to Establish the Glioma Hypermethylator Phenotype. Nature (2012) 483(7390):479–83. doi: 10.1038/nature10866
63. Xu W, Yang H, Liu Y, Yang Y, Wang P, Kim SH, et al. Oncometabolite 2-Hydroxyglutarate Is a Competitive Inhibitor of Alpha-Ketoglutarate-Dependent Dioxygenases. Cancer Cell (2011) 19(1):17–30. doi: 10.1016/j.ccr.2010.12.014
64. Linster CL, Van Schaftingen E, Hanson AD. Metabolite Damage and its Repair or Pre-Emption. Nat Chem Biol (2013) 9(2):72–80. doi: 10.1038/nchembio.1141
65. Ye D, Guan KL, Xiong Y. Metabolism, Activity, and Targeting of D- and L-2-Hydroxyglutarates. Trends Cancer (2018) 4(2):151–65. doi: 10.1016/j.trecan.2017.12.005
66. Fu Y, Lan T, Cai H, Lu A, Yu W. Meta-Analysis of Serum Lactate Dehydrogenase and Prognosis for Osteosarcoma. Med (Baltimore) (2018) 97(19):e0741. doi: 10.1097/MD.0000000000010741
67. Gan J, Wang W, Yang Z, Pan J, Zheng L, Yin L. Prognostic Value of Pretreatment Serum Lactate Dehydrogenase Level in Pancreatic Cancer Patients: A Meta-Analysis of 18 Observational Studies. Med (Baltimore) (2018) 97(46):e13151. doi: 10.1097/MD.0000000000013151
68. Zhang Z, Li Y, Yan X, Song Q, Wang G, Hu Y, et al. Pretreatment Lactate Dehydrogenase may Predict Outcome of Advanced non Small-Cell Lung Cancer Patients Treated With Immune Checkpoint Inhibitors: A Meta-Analysis. Cancer Med (2019) 8(4):1467–73. doi: 10.1002/cam4.2024
69. Hou XM, Yuan SQ, Zhao D, Liu XJ, Wu XA. LDH-A Promotes Malignant Behavior via Activation of Epithelial-to-Mesenchymal Transition in Lung Adenocarcinoma. Biosci Rep (2019) 39(1):1–10. doi: 10.1042/BSR20181476
70. Meng MB, Wang HH, Guo WH, Wu ZQ, Zeng XL, Zaorsky NG, et al. Targeting Pyruvate Kinase M2 Contributes to Radiosensitivity of Non-Small Cell Lung Cancer Cells In Vitro and In Vivo. Cancer Lett (2015) 356(2 Pt B):985–93. doi: 10.1016/j.canlet.2014.11.016
71. Liu G, Kelly WK, Wilding G, Leopold L, Brill K, Somer B. An Open-Label, Multicenter, Phase I/II Study of Single-Agent AT-101 in Men With Castrate-Resistant Prostate Cancer. Clin Cancer Res (2009) 15(9):3172–6. doi: 10.1158/1078-0432.CCR-08-2985
72. Meng Y, Tang W, Dai Y, Wu X, Liu M, Ji Q, et al. Natural BH3 Mimetic (-)-Gossypol Chemosensitizes Human Prostate Cancer via Bcl-xL Inhibition Accompanied by Increase of Puma and Noxa. Mol Cancer Ther (2008) 7(7):2192–202. doi: 10.1158/1535-7163.MCT-08-0333
73. Heist RS, Fain J, Chinnasami B, Khan W, Molina JR, Sequist LV, et al. Phase I/II Study of AT-101 With Topotecan in Relapsed and Refractory Small Cell Lung Cancer. J Thorac Oncol (2010) 5(10):1637–43. doi: 10.1097/JTO.0b013e3181e8f4dc
74. Baggstrom MQ, Qi Y, Koczywas M, Argiris A, Johnson EA, Millward MJ, et al. A Phase II Study of AT-101 (Gossypol) in Chemotherapy-Sensitive Recurrent Extensive-Stage Small Cell Lung Cancer. J Thorac Oncol (2011) 6(10):1757–60. doi: 10.1097/JTO.0b013e31822e2941
75. Manerba M, Di Ianni L, Govoni M, Roberti M, Recanatini M, Di Stefano G. LDH Inhibition Impacts on Heat Shock Response and Induces Senescence of Hepatocellular Carcinoma Cells. Eur J Pharm Sci (2017) 105:91–8. doi: 10.1016/j.ejps.2017.05.015
76. Koukourakis M, Tsolou A, Pouliliou S, Lamprou I, Papadopoulou M, Ilemosoglou M, et al. Blocking LDHA Glycolytic Pathway Sensitizes Glioblastoma Cells to Radiation and Temozolomide. Biochem Biophys Res Commun (2017) 491(4):932–8. doi: 10.1016/j.bbrc.2017.07.138
77. Daniele S, Giacomelli C, Zappelli E, Granchi C, Trincavelli ML, Minutolo F, et al. Lactate Dehydrogenase-A Inhibition Induces Human Glioblastoma Multiforme Stem Cell Differentiation and Death. Sci Rep (2015) 5:15556. doi: 10.1038/srep15556
78. Kim EY, Chung TW, Han CW, Park SY, Park KH, Jang SB, et al. A Novel Lactate Dehydrogenase Inhibitor, 1-(Phenylseleno)-4-(Trifluoromethyl) Benzene, Suppresses Tumor Growth Through Apoptotic Cell Death. Sci Rep (2019) 9(1):3969. doi: 10.1038/s41598-019-40617-3
79. Zhai X, Yang Y, Wan J, Zhu R, Wu Y. Inhibition of LDH-A by Oxamate Induces G2/M Arrest, Apoptosis and Increases Radiosensitivity in Nasopharyngeal Carcinoma Cells. Oncol Rep (2013) 30(6):2983–91. doi: 10.3892/or.2013.2735
80. Judge JL, Lacy SH, Ku WY, Owens KM, Hernady E, Thatcher TH, et al. The Lactate Dehydrogenase Inhibitor Gossypol Inhibits Radiation-Induced Pulmonary Fibrosis. Radiat Res (2017) 188(1):35–43. doi: 10.1667/RR14620.1
81. Brandstetter G, Blatt S, Goldschmitt J, Taylor L, Heymann P, Al-Nawas B, et al. Targeted Sensitization of Tumor Cells for Radiation Through Monocarboxylate Transporters 1 and 4 Inhibition In Vitro. Clin Oral Investig (2021) 25(1):295–310. doi: 10.1007/s00784-020-03364-8
82. Bola BM, Chadwick AL, Michopoulos F, Blount KG, Telfer BA, Williams KJ, et al. Inhibition of Monocarboxylate Transporter-1 (MCT1) by AZD3965 Enhances Radiosensitivity by Reducing Lactate Transport. Mol Cancer Ther (2014) 13(12):2805–16. doi: 10.1158/1535-7163.MCT-13-1091
83. Jones RS, Morris ME. Monocarboxylate Transporters: Therapeutic Targets and Prognostic Factors in Disease. Clin Pharmacol Ther (2016) 100(5):454–63. doi: 10.1002/cpt.418
84. Ullah MS, Davies AJ, Halestrap AP. The Plasma Membrane Lactate Transporter MCT4, But Not MCT1, Is Up-Regulated by Hypoxia Through a HIF-1alpha-Dependent Mechanism. J Biol Chem (2006) 281(14):9030–7. doi: 10.1074/jbc.M511397200
85. Juel C, Halestrap AP. Lactate Transport in Skeletal Muscle - Role and Regulation of the Monocarboxylate Transporter. J Physiol (1999) 517(Pt 3):633–42. doi: 10.1111/j.1469-7793.1999.0633s.x
86. Halestrap AP, Meredith D. The SLC16 Gene Family-From Monocarboxylate Transporters (MCTs) to Aromatic Amino Acid Transporters and Beyond. Pflugers Arch (2004) 447(5):619–28. doi: 10.1007/s00424-003-1067-2
87. Garcia CK, Goldstein JL, Pathak RK, Anderson RG, Brown MS. Molecular Characterization of a Membrane Transporter for Lactate, Pyruvate, and Other Monocarboxylates: Implications for the Cori Cycle. Cell (1994) 76(5):865–73. doi: 10.1016/0092-8674(94)90361-1
88. Kim Y, Choi JW, Lee JH, Kim YS. Expression of Lactate/H(+) Symporters MCT1 and MCT4 and Their Chaperone CD147 Predicts Tumor Progression in Clear Cell Renal Cell Carcinoma: Immunohistochemical and The Cancer Genome Atlas Data Analyses. Hum Pathol (2015) 46(1):104–12. doi: 10.1016/j.humpath.2014.09.013
89. Zhang G, Zhang Y, Dong D, Wang F, Ma X, Guan F, et al. MCT1 Regulates Aggressive and Metabolic Phenotypes in Bladder Cancer. J Cancer (2018) 9(14):2492–501. doi: 10.7150/jca.25257
90. Latif A, Chadwick AL, Kitson SJ, Gregson HJ, Sivalingam VN, Bolton J, et al. Monocarboxylate Transporter 1 (MCT1) Is an Independent Prognostic Biomarker in Endometrial Cancer. BMC Clin Pathol (2017) 17:27. doi: 10.1186/s12907-017-0067-7
91. Simoes-Sousa S, Granja S, Pinheiro C, Fernandes D, Longatto-Filho A, Laus AC, et al. Prognostic Significance of Monocarboxylate Transporter Expression in Oral Cavity Tumors. Cell Cycle (2016) 15(14):1865–73. doi: 10.1080/15384101.2016.1188239
92. Perez-Escuredo J, Van Hee VF, Sboarina M, Falces J, Payen VL, Pellerin L, et al. Monocarboxylate Transporters in the Brain and in Cancer. Biochim Biophys Acta (2016) 1863(10):2481–97. doi: 10.1016/j.bbamcr.2016.03.013
93. Park SJ, Smith CP, Wilbur RR, Cain CP, Kallu SR, Valasapalli S, et al. An Overview of MCT1 and MCT4 in GBM: Small Molecule Transporters With Large Implications. Am J Cancer Res (2018) 8(10):1967–76.
94. Romero-Garcia S, Moreno-Altamirano MM, Prado-Garcia H, Sanchez-Garcia FJ. Lactate Contribution to the Tumor Microenvironment: Mechanisms, Effects on Immune Cells and Therapeutic Relevance. Front Immunol (2016) 7:52. doi: 10.3389/fimmu.2016.00052
95. Colen CB, Shen Y, Ghoddoussi F, Yu P, Francis TB, Koch BJ, et al. Metabolic Targeting of Lactate Efflux by Malignant Glioma Inhibits Invasiveness and Induces Necrosis: An In Vivo Study. Neoplasia (2011) 13(7):620–32. doi: 10.1593/neo.11134
96. Benjamin D, Robay D, Hindupur SK, Pohlmann J, Colombi M, El-Shemerly MY, et al. Dual Inhibition of the Lactate Transporters MCT1 and MCT4 Is Synthetic Lethal With Metformin Due to NAD+ Depletion in Cancer Cells. Cell Rep (2018) 25(11):3047–58 e4. doi: 10.1016/j.celrep.2018.11.043
97. Hong CS, Graham NA, Gu W, Espindola Camacho C, Mah V, Maresh EL, et al. MCT1 Modulates Cancer Cell Pyruvate Export and Growth of Tumors That Co-Express MCT1 and MCT4. Cell Rep (2016) 14(7):1590–601. doi: 10.1016/j.celrep.2016.01.057
98. Le Floch R, Chiche J, Marchiq I, Naiken T, Ilc K, Murray CM, et al. CD147 Subunit of Lactate/H+ Symporters MCT1 and Hypoxia-Inducible MCT4 is Critical for Energetics and Growth of Glycolytic Tumors. Proc Natl Acad Sci USA (2011) 108(40):16663–8. doi: 10.1073/pnas.1106123108
99. Polanski R, Hodgkinson CL, Fusi A, Nonaka D, Priest L, Kelly P, et al. Activity of the Monocarboxylate Transporter 1 Inhibitor AZD3965 in Small Cell Lung Cancer. Clin Cancer Res (2014) 20(4):926–37. doi: 10.1158/1078-0432.CCR-13-2270
100. Noble RA, Bell N, Blair H, Sikka A, Thomas H, Phillips N, et al. Inhibition of Monocarboxyate Transporter 1 by AZD3965 as a Novel Therapeutic Approach for Diffuse Large B-Cell Lymphoma and Burkitt Lymphoma. Haematologica (2017) 102(7):1247–57. doi: 10.3324/haematol.2016.163030
101. Beloueche-Babari M, Casals Galobart T, Delgado-Goni T, Wantuch S, Parkes HG, Tandy D, et al. Monocarboxylate Transporter 1 Blockade With AZD3965 Inhibits Lipid Biosynthesis and Increases Tumour Immune Cell Infiltration. Br J Cancer (2020) 122(6):895–903. doi: 10.1038/s41416-019-0717-x
102. Beloueche-Babari M, Wantuch S, Casals Galobart T, Koniordou M, Parkes HG, Arunan V, et al. MCT1 Inhibitor AZD3965 Increases Mitochondrial Metabolism, Facilitating Combination Therapy and Noninvasive Magnetic Resonance Spectroscopy. Cancer Res (2017) 77(21):5913–24. doi: 10.1158/0008-5472.CAN-16-2686
103. Curtis NJ, Mooney L, Hopcroft L, Michopoulos F, Whalley N, Zhong H, et al. Pre-Clinical Pharmacology of AZD3965, A Selective Inhibitor of MCT1: DLBCL, NHL and Burkitt’s Lymphoma Anti-Tumor Activity. Oncotarget (2017) 8(41):69219–36. doi: 10.18632/oncotarget.18215
104. Guan X, Rodriguez-Cruz V, Morris ME. Cellular Uptake of MCT1 Inhibitors AR-C155858 and AZD3965 and Their Effects on MCT-Mediated Transport of L-Lactate in Murine 4t1 Breast Tumor Cancer Cells. AAPS J (2019) 21(2):13. doi: 10.1208/s12248-018-0279-5
105. Plummer R, Halford S, Jones P, Wedge S, Hirschberg S, Veal G, et al. A First-in-Human First-in-Class (FIC) Trial of the Monocarboxylate Transporter 1 (MCT1) Inhibitor AZD3965 in Patients With Advanced Solid Tumours. Ann Oncol (2018) 29:iii9. doi: 10.1093/annonc/mdy048.008
106. Rauckhorst AJ, Taylor EB. Mitochondrial Pyruvate Carrier Function and Cancer Metabolism. Curr Opin Genet Dev (2016) 38:102–9. doi: 10.1016/j.gde.2016.05.003
107. Kane DA. Lactate Oxidation at the Mitochondria: A Lactate-Malate-Aspartate Shuttle at Work. Front Neurosci (2014) 8:366. doi: 10.3389/fnins.2014.00366
108. Faubert B, Li KY, Cai L, Hensley CT, Kim J, Zacharias LG, et al. Lactate Metabolism in Human Lung Tumors. Cell (2017) 171(2):358–71.e9. doi: 10.1016/j.cell.2017.09.019
109. Hui S, Ghergurovich JM, Morscher RJ, Jang C, Teng X, Lu W, et al. Glucose Feeds the TCA Cycle via Circulating Lactate. Nature (2017) 551(7678):115–8. doi: 10.1038/nature24057
110. Draoui N, Schicke O, Fernandes A, Drozak X, Nahra F, Dumont A, et al. Synthesis and Pharmacological Evaluation of Carboxycoumarins as a New Antitumor Treatment Targeting Lactate Transport in Cancer Cells. Bioorg Med Chem (2013) 21(22):7107–17. doi: 10.1016/j.bmc.2013.09.010
111. Draoui N, Schicke O, Seront E, Bouzin C, Sonveaux P, Riant O, et al. Antitumor Activity of 7-Aminocarboxycoumarin Derivatives, a New Class of Potent Inhibitors of Lactate Influx But Not Efflux. Mol Cancer Ther (2014) 13(6):1410–8. doi: 10.1158/1535-7163.MCT-13-0653
112. Sandforth L, Ammar N, Dinges LA, Rocken C, Arlt A, Sebens S, et al. Impact of the Monocarboxylate Transporter-1 (MCT1)-Mediated Cellular Import of Lactate on Stemness Properties of Human Pancreatic Adenocarcinoma Cells Dagger. Cancers (Basel) (2020) 12(3):1–21. doi: 10.3390/cancers12030581
113. Naik A, Decock J. Lactate Metabolism and Immune Modulation in Breast Cancer: A Focused Review on Triple Negative Breast Tumors. Front Oncol (2020) 10:598626. doi: 10.3389/fonc.2020.598626
114. Hensley CT, Faubert B, Yuan Q, Lev-Cohain N, Jin E, Kim J, et al. Metabolic Heterogeneity in Human Lung Tumors. Cell (2016) 164(4):681–94. doi: 10.1016/j.cell.2015.12.034
115. Brender JR, Kishimoto S, Merkle H, Reed G, Hurd RE, Chen AP, et al. Dynamic Imaging of Glucose and Lactate Metabolism by (13)C-MRS Without Hyperpolarization. Sci Rep (2019) 9(1):3410. doi: 10.1038/s41598-019-38981-1
116. Macdonald EB, Begovatz P, Barton GP, Erickson-Bhatt S, Inman DR, Cox BL, et al. Hyperpolarized (13)C Magnetic Resonance Spectroscopic Imaging of Pyruvate Metabolism in Murine Breast Cancer Models of Different Metastatic Potential. Metabolites (2021) 11(5):1–18. doi: 10.3390/metabo11050274
117. van Heijster FHA, Heskamp S, Breukels V, Veltien A, Franssen GM, Jansen K, et al. Pyruvate-Lactate Exchange and Glucose Uptake in Human Prostate Cancer Cell Models. A Study in Xenografts and Suspensions by Hyperpolarized [1-(13) C]pyruvate MRS and [(18) F]FDG-PET. NMR BioMed (2020) 33(10):e4362. doi: 10.1002/nbm.4362
Keywords: lactate metabolism, Warburg phenomenon, radiation therapy, radiosensitization, synergistic effects
Citation: Liu KX, Everdell E, Pal S, Haas-Kogan DA and Milligan MG (2021) Harnessing Lactate Metabolism for Radiosensitization. Front. Oncol. 11:672339. doi: 10.3389/fonc.2021.672339
Received: 25 February 2021; Accepted: 12 July 2021;
Published: 23 July 2021.
Edited by:
Timothy James Kinsella, Warren Alpert Medical School of Brown University, United StatesReviewed by:
DeeDee Smart, National Cancer Institute, United StatesM. Saeed Sheikh, Upstate Medical University, United States
Copyright © 2021 Liu, Everdell, Pal, Haas-Kogan and Milligan. This is an open-access article distributed under the terms of the Creative Commons Attribution License (CC BY). The use, distribution or reproduction in other forums is permitted, provided the original author(s) and the copyright owner(s) are credited and that the original publication in this journal is cited, in accordance with accepted academic practice. No use, distribution or reproduction is permitted which does not comply with these terms.
*Correspondence: Michael G. Milligan, bWljaGFlbF9taWxsaWdhbkBkZmNpLmhhcnZhcmQuZWR1