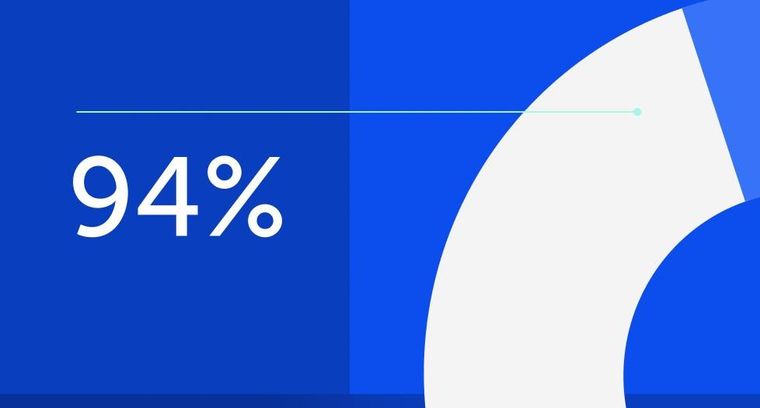
94% of researchers rate our articles as excellent or good
Learn more about the work of our research integrity team to safeguard the quality of each article we publish.
Find out more
REVIEW article
Front. Oncol., 26 July 2021
Sec. Women's Cancer
Volume 11 - 2021 | https://doi.org/10.3389/fonc.2021.654428
This article is part of the Research TopicMetabolic Abnormalities and Breast Cancer: Challenges from Bench to BedsideView all 19 articles
Breast cancer (BC) is the most frequent cancer among women worldwide and is the leading cause of cancer-related deaths in women. Cancer cells with stem cell-like features and tumor-initiating potential contribute to drug resistance, tumor recurrence, and metastasis. To achieve better clinical outcomes, it is crucial to eradicate both bulk BC cells and breast cancer stem cells (BCSCs). Salinomycin, a monocarboxylic polyether antibiotic isolated from Streptomyces albus, can precisely kill cancer stem cells (CSCs), particularly BCSCs, by various mechanisms, including apoptosis, autophagy, and necrosis. There is increasing evidence that salinomycin can inhibit cell proliferation, invasion, and migration in BC and reverse the immune-inhibitory microenvironment to prevent tumor growth and metastasis. Therefore, salinomycin is a promising therapeutic drug for BC. In this review, we summarize established mechanisms by which salinomycin protects against BC and discuss its future clinical applications.
According to the International Agency for Research on Cancer of the World Health Organization, the number of new breast cancer (BC) cases increased to 2.26 million in 2020, exceeding the number of lung cancer cases (2.21 million in 2020) for the first time; thus, BC has officially replaced lung cancer as the most common cancer worldwide. BC accounts for 1/4 of female cancer cases and 1/6 of female cancer-related deaths. In most countries, it is now the greatest threat to the health of women.
Owing to the limitations of current therapies, many patients die from metastasis, recurrence, and drug resistance (1, 2). In the last two decades, studies have revealed that breast cancer stem cells (BCSCs), a small subpopulation of cells, have been shown to have the ability to self-renew and differentiate into tumor cells. The clinical relevance of BCSCs has been a focus of many studies (3, 4), which have demonstrated that these cells are resistant to conventional chemotherapy and radiation treatment and are very likely to be the origin of cancer relapse and metastasis (5–9). Hence, to improve clinical outcomes, it is crucial to eradicate both bulk BC cells and BCSCs.
Salinomycin is a monocarboxylic polyether antibiotic derived from Streptomyces albus (10). Its chemical structure is shown in Figure 1. It functions on different biological membranes and shows high affinity for positive ions, especially potassium, interfering with the balance of ion concentrations between the inside and outside of cells, thereby affecting osmotic pressure and eventually leading to germ cell disruption (12). At the end of the last decade, the preferential toxicity towards cancer stem cells (CSCs) has been reported by Gupta and colleagues (13). In addition to BC, salinomycin can also selectively kill CSCs in many other types of cancers, such as ovarian, lung, prostate, and colorectal cancers (14–19). The multiple function of salinomycin against CSCs and its molecular mechanism have been intensively studied in many types of cancer cells, with these studies extensively reviewed in several publications (11, 20–22). These reviews have also described the chemical properties of salinomycin in detail and summarized its role in cancer cells and CSCs. Extensive studies on BC have established that salinomycin inhibits cell proliferation, invasion, and migration, modulates cell death, and reverses the immune-inhibitory microenvironment to prevent tumor growth and metastasis (13, 16, 23–26). Moreover, salinomycin can be used to kill BC cells with drug resistance (27, 28). Therefore, salinomycin is expected to be a potent therapeutic drug for BC.
Figure 1 Chemical structure of Salinomycin, edit from (11).
In this review, we summarize our current understanding of the mechanisms by which salinomycin inhibits BC, including its precise effects on BCSC proliferation, invasion, migration, and apoptosis. Furthermore, we discuss its potential applications in BC therapy, including the potential utility of nanocarrier-based delivery systems and combination therapies.
CSCs are tumor cells with stem cell characteristics and initiate cancer formation. They form and maintain heterogeneous tumor entities through self-renewal and asymmetric division (29). Compared with highly differentiated tumor cells, CSCs have a lower degree of differentiation, stronger drug metabolism, and the ability to repair drug-induced damage (30). They can renew themselves through epigenetic changes, acquire new mutations, and quickly adapt to the environment, thereby forming a new tumor entity with molecular characteristics very differently from those of the initial tumor. Therefore, CSCs are also considered to be the source of tumor metastasis and drug resistance (31). If therapies only target differentiated tumor cells and cannot effectively eliminate CSCs, tumor recurrence will be inevitable. Hence, effective inhibition of differentiated tumor cells and simultaneous and specific elimination of CSCs are the focus of current research in the field of tumor therapy.
Salinomycin is over 100 times more effective against BCSCs than paclitaxel, the traditional chemotherapy drug for the treatment of BC (26). Salinomycin increases the apoptosis of BC mammosphere cells, which is accompanied by downregulation of Bcl-2 expression, and decreases their migration capacity, which is accompanied by downregulation of c-Myc and Snail expression (23). Furthermore, extensive studies on BCSCs have established that salinomycin decreases CSC population (32–34). Global gene expression analyses have shown that salinomycin inhibits the expression of BCSC genes (13). In addition, consistent with previous findings in prostate cancer, salinomycin reduces aldehyde dehydrogenase activity and the expression of MYC, AR, and ERG; it induces oxidative stress and inhibits nuclear factor (NF)-κB activity (35). In BC cells, salinomycin influences stem cell signaling, such as Wnt and Hedgehog signaling, or ALDH1 activity, to suppress mammosphere formation, induce cell apoptosis, or inhibit cell proliferation (34, 36–39). Further, salinomycin effectively inhibits mammary tumor growth in vivo. In BC xenograft tumors, it significantly reduces tumor growth, which is accompanied by decreased PTCH, SMO, Gli1, and Gli2 expression (23).
Although the exact mechanisms underlying the effects of salinomycin on BCSCs are not fully understood, recent studies have provided insights into its molecular mechanisms and modes of action. The effect of salinomycin on BCSCs and BC cells through various mechanisms of action and its molecular targets have been summarized in this review (see Tables 1, 2 and Figure 2).
Figure 2 Mechanisms of the anticancer activity of salinomycin in breast cancer (BC) cells. Salinomycin inhibits cell proliferation in BC cells through the Hedgehog and Wnt signaling pathways. Additionally, salinomycin is capable of inhibiting Wnt signaling by blocking the phosphorylation of LRP6 or by ER stress, which leads to apoptosis in BC cells. Moreover, salinomycin induces the apoptosis of BC cells by increasing DNA damage or intracellular ROS level, thereby influencing the p53 and caspase cascade separately. Salinomycin also induces autophagy by increasing intracellular ROS level, which is accompanied by MAPK signaling pathway activation. Furthermore, salinomycin can affect the cell membrane potential and reduce the level of ATP to induce mitophagy and mitoptosis. Moreover, salinomycin inhibits angiogenesis through the VEGF signaling pathway.
Salinomycin and its derivatives have multiple functions in BC cells. We review evidence for its precise effects on various cellular processes in BC and the mechanisms underlying these effects.
Three major cell death pathways have been described: apoptosis, autophagy, and necrosis (48). Cancer cell death induced by salinomycin is achieved by multiple mechanisms (13, 16, 24). In fact, accumulating evidence shows that apoptosis and autophagic cell death can both occur, with crosstalk between the two pathways. Furthermore, both pathways may eventually lead to necrosis (secondary necrosis), depending on the stimulus and cell type (49).
Apoptosis is the major cell death pathway contributing to the removal of unnecessary and harmful cells during embryonic development, tissue homeostasis, and immune regulation. Apoptosis is mainly executed by intracellular cysteine proteases called caspases, which may be activated by two principal pathways: death receptor-dependent (extrinsic) and -independent (intrinsic or mitochondrial) pathways (50).
Salinomycin induces apoptosis in CSCs of different origins (18, 51, 52). However, the precise mechanisms vary substantially and are tightly correlated with the origin of the CSCs (26, 53–55).
Salinomycin increases DNA breaks in BC cells as well as the expression of phosphorylated p53 and γH2AX in Hs578T cells. It was indicated that salinomycin increases DNA damage, and this effect plays an important role in increasing apoptosis (26). Furthermore, salinomycin induces the expression of the pro-apoptotic protein Bax but inhibits the expression of the anti-apoptotic protein Bcl-2 in MDA-MB231 cells, suggesting that an increase in the Bax/Bcl-2 ratio may be involved in salinomycin-induced apoptosis (46). The same result was shown in cisplatin-resistant MCF7DDP cells; in these cells, salinomycin abrogated nuclear translocation of NF-κB and caused a concurrent reduction in NF-κB-regulated expression of pro-survival proteins (27). Moreover, salinomycin reportedly activates a distinct apoptotic pathway that is not accompanied by cell cycle arrest and is independent of the tumor suppressor protein p53, caspase activation, the CD95/CD95L system, and proteasome (54). Interestingly, Yusra Al Dhaheri et al. reported that combination of salinomycin with paclitaxel or docetaxel can synergistically increase apoptosis in the BC cell line MDA-MB-231, which is not sensitive enough to salinomycin, providing a new insight for the clinical application of salinomycin (53).
These results suggest that the induction of apoptotic or non-apoptotic cell death by salinomycin in cancer cells depends on the particular cell type and that the detailed mechanisms underlying salinomycin-induced cell death in cancer cells remain to be fully elucidated.
Autophagy is the catabolic process that regulates the degradation of a cell’s own components via the lysosomal machinery, characterized by the appearance of large autophagic vacuoles in the cytoplasm. It can be considered a physiological process used for the recycling of damaged organelles and energy supply during “lean times,” leading to cell death if extensively activated. At low levels, autophagy promotes cell survival by removing damaged proteins and organelles while supplying extra energy; however, the excessive and long-term upregulation of autophagy eventually results in the destruction of essential proteins and organelles beyond a certain threshold, resulting in cell death. Salinomycin reportedly induces and inhibits autophagy (56).
On the one hand, a substantial autophagic response to salinomycin (substantially stronger than the response to the commonly used autophagy inducer rapamycin) has been detected in BC cells (SKBR3 and MDA-MB-468 cells) and to a lesser degree in normal human dermal fibroblasts (16). Salinomycin strongly decreases ATP levels in BC cells in a time-dependent manner. Conversely, following treatment with salinomycin, human normal dermal fibroblasts exhibit decreased mitochondrial mass, although they are largely resistant to salinomycin-induced ATP depletion. Autophagy caused by salinomycin has a protective effect on various cancer cell lines, but experiments have shown that by blocking the expression of ATG7 with siRNA (41), this protective effect can be reversed. Therefore, the combined use of autophagy blockers in the clinic will be more effective.
On the other hand, the inhibitory effect of salinomycin on autophagy may explain its ability to block the degradation of LC3 and long-lived proteins. The suppressive effect of salinomycin on autophagic flux is relatively higher in the ALDH(+) population in HMLER cells than in the ALDH(−) population, and this differential effect is correlated with an increase in apoptosis in the ALDH(+) population. ATG7 depletion accelerates the proapoptotic capacity of salinomycin in the ALDH(+) population (41). Similarly, Pellegrini et al. found that CSC-like cells have greater sensitivity to autophagy inhibition than that of cells not expressing CSC markers (32).
Different from apoptosis, necrosis is another type of cell death that is typically not associated with the activation of caspases. It is characterized by the swelling of the endoplasmic reticulum (ER), mitochondria, and cytoplasm, with the subsequent rupture of the plasma membrane and cell lysis. Necrotic cell death is the result of interplay between several signaling cascades. The determinants of necrosis are mainly RIP3, calcium, and mitochondria. RIP3 interacts with RIP1 and binds to several enzymes involved in carbohydrate and glutamine metabolism. Calcium controls the activation of PLA, calpains, and NOS, inducing a series of events leading to necrotic cell death.
According to Xipell et al., in glioblastoma cells, salinomycin induces substantial ER stress, triggering the unfolded protein response and an aberrant autophagic flux that culminates in necrosis due to mitochondria and lysosomal alterations (57). However, the mechanism underlying necrosis in BC has not been revealed. It may occur as a consequence of massive cell injury or in response to extreme changes in physiological conditions.
Treatment with salinomycin hampers the proliferation of BC cells, and this effect is mediated by different mechanisms. For example, salinomycin inhibits proliferation in MDA-MB-231 cells in concentration- and time-dependent manners; in particular, it blocks the G1-to-S phase transition by the downregulation of genes downstream of the Hedgehog signaling pathway (38). In addition, salinomycin blocks the proliferation of BC cells by suppressing cyclin D1 expression and the GSK3β-mediated inhibition of the Wnt signaling pathway (36). It also suppresses BCSC proliferation, concomitant with the downregulation of cyclin D1 and increased p27(kip1) nuclear accumulation (34). Furthermore, an interesting study has shown that at low concentrations, salinomycin could induce transient G1 arrest at early time points and G2 arrest at late time points as well as senescence, in addition to inducing an enlarged cell morphology, the upregulation of p21 protein, and increases in histone H3 and H4 hyperacetylation and SA-β-Gal activity (44).
ROS, which are products of normal metabolism and xenobiotic exposure, can be beneficial or harmful to cells and tissues depending on their concentration. ER stress can be an initiator of apoptosis and occurs when unfolded proteins accumulate; ER Golgi transport is inhibited, or the Ca2+ equilibrium is disrupted in cells. Excessive ROS production triggers oxidative modification of cellular macromolecules, inhibits protein function, and promotes cell death.
The elevated oxidative stress and mitochondrial membrane depolarization in response to salinomycin-mediated apoptosis were first reported in prostate cancer cells (55). Salinomycin can induce an increase in intracellular ROS levels, accompanied by decreased mitochondrial membrane potential, causing ER damage, with increased levels of Ca2+ being released into the cytoplasm; this process is regulated by Bcl-2 and Bax/Bak, further activating caspase-3 and the cleavage of PARP-1, ultimately leading to mitochondrial apoptosis. Moreover, Bcl-2, Bax, and Bak are also localized in the ER; thus, the ER serves as an important organelle for apoptotic control that is further enhanced by the mitochondria ER connection.
In BC cell lines (MCF-7, T47D, and MDA-MB-453), salinomycin induces ROS formation, causing JNK activation and induction of the transcription factor JUN. Salinomycin-mediated cell death was partially inhibited by N-acetyl-cysteine (NAC), a free radical scavenger, suggesting ROS formation contributes to the toxicity of salinomycin (45). This has been observed in MDA-MB-231 cells, in which salinomycin-mediated ROS production leads to mitochondrial dysfunction, and NAC attenuates salinomycin-induced apoptosis and autophagy. This result seems to conflict with the fact that the acceleration of apoptosis induced by salinomycin is related to autophagy inhibition. NAC thereby makes the function of salinomycin more complex. This indicates that the crosstalk between two different physiological responses (autophagy and apoptosis) induced by salinomycin might play a pivotal role in the determination of the fate of cancer cells (46).
Furthermore, ionomycin (AM5), a synthetic derivative of salinomycin, exhibits potent and selective activity against BCSCs in vitro and in vivo by accumulating and sequestering iron in lysosomes. Iron-mediated ROS production reportedly promotes lysosomal membrane permeabilization, thereby activating a cell death pathway consistent with ferroptosis (40). These findings highlight the importance of iron homeostasis in BCSCs, suggesting both iron- and iron-mediated processes to be potential therapeutic targets in BC.
Epithelial-to-mesenchymal transition (EMT) is the major cause of BC invasion and metastasis. In addition to substantial inhibitory effects on invasion and metastasis in BC, as determined by single-cell tracking, salinomycin significantly reduces the metastatic tumor burden in mice (58). Furthermore, various migration-related parameters in MDA-MB-231 cells are significantly lower after salinomycin treatment than in control cells, and the effects of salinomycin are concentration-dependent (59). Further, salinomycin inhibits the TGF-β1-induced EMT phenotypic transition and activation of Smad (p-Smad2/3 and Snail1) and non-Smad (β-catenin, p-p38, and MAPK) signaling molecules, which cooperatively regulate EMT induction. Importantly, these findings were confirmed in a series of BC specimens, in which there were strong correlations among levels of E-cadherin and β-catenin and the lymph node metastatic potential of BC (60).
The characteristics of CSCs have been associated with angiogenesis. It has been reported that the expression of several CSC biomarkers correlates with that of angiogenesis markers, and some stem cell signaling pathways (e.g., Notch) are used by both CSCs and by pro-angiogenic factors (61). This indicates that treatment linked to anti-angiogenic therapy and targeted at CSCs may be effective in the treatment of many malignant tumors, including BC. The antiangiogenic and anticancer efficacies of salinomycin in BC have been explored. In particular, salinomycin interrupts HIF-1α/VEGF signaling to inhibit VEGF-induced angiogenesis and BC growth. Moreover, it inhibits the expression of the pro-angiogenic cell surface marker CD31, thereby disrupting endothelial tubulogenesis, thereby interrupting endothelial tubulogenesis, decreases the binding of HIF-1α to the HRE sequence in human BC cells and suppresses neovascularization in a chick chorioallantoic membrane and a Matrigel plug-implanted mouse model (47). Salinomycin inhibits BC growth and tumor angiogenesis in mice based on bioluminescence and immunofluorescence imaging analyses. It also suppresses serum VEGFA levels in tumor-bearing mice and induces caspase-dependent apoptosis in BC cells (47).
Salinomycin could play a role in modulating the immune microenvironment in BC. It may exert these modulatory effects by two different mechanisms. First, at insufficient concentrations for direct antitumor activity, it could effectively stimulate M1 type macrophages and limit tumor growth and metastasis. In a previous study, the intratumoral injection of salinomycin increased the proportion of CD86 cells and decreased CD206 cells in transplant 4T1 tumors, thereby preventing tumor growth and pulmonary metastasis (62). Second, salinomycin could potently reverse tumor immune tolerance via the repression of IDO1 enzymatic activity. Moreover, it suppresses and inhibits IFN-γ-induced activation of the Janus kinase/signal transducer and activator of transcription (JAK/STAT) pathway and the nuclear factor NF-κB pathway, respectively, by inhibiting IκB degradation and NF-κB phosphorylation. Furthermore, it restores the proliferation of T cells co-cultured with IFN-γ-treated BC cells and potentiates the antitumor activity of cisplatin in vivo (63).
Salinomycin is a membrane ionophore that facilitates ion flux through the cytoplasmic and mitochondrial membranes. It works as a mobile carrier and discharges K+ rapidly (64–66). However, recent studies showed that salinomycin physically targets the lysosomal compartment, and not the ER, mitochondria, or the Golgi apparatus in CSCs (40, 67). It then accumulates in the lysosomal compartment in an endocytosis-independent manner (40). In the lysosomal compartment, salinomycin can interact with iron(II) and inhibit the effective translocation of the metal into the cytosol, thereby blocking the release of iron from lysosomes and resulting in lysosomal iron accumulation. The elevated iron level in lysosomes promotes enhanced ROS reaction via Fenton chemistry (68), possibly through lysosomal degradation of ferritin and the release of additional soluble redox-active iron, subsequently inducing lysosomal membrane permeabilization (LMP) (69). Compared with normal cells, cancer cells are sensitive to LMP through a variety of mechanisms, such as altered lysosomal localization, decreased LAMP-1 and LAMP-2 level (70), increased lysosomal size, altered heat shock protein 70 localization, and elevated sphingosine level (71–74). Particularly, CSCs contain significantly higher levels of iron, which either directly alter their differentiation or cause them to be selected for proliferation in favor of a subpopulation exhibiting a pronounced CSC phenotype.
Therefore, in CSCs, salinomycin can trigger a much stronger ROS reaction and severe LMP. Salinomycin has been shown to activate distinct cell responses, including autophagy, apoptosis, and necrosis. This is likely linked to the extent of LMP in various cancer cells in response to salinomycin. Lysosomes are intrinsically heterogeneous, and not all lysosomes are permeabilized simultaneously in response to lysosomal stress (75, 76). Thus, damage to a small proportion of lysosomes can be fixed by activating lysophagy and other endolysosomal damage response mechanisms, thereby ensuring cell survival. However, when majority of lysosomes are damaged or autophagy is inhibited, the damaged lysosomes can no longer be eliminated by lysophagy, causing cell death. This point has not been addressed by previous investigations on salinomycin, and thus requires clarification. In some cancer cells, salinomycin triggered LMP and resulted in the translocation of cathepsins from lysosomes to the cytosol, where they induced the proteolytic activation of substrates, such as Bid and Bax, which in turn, promoted mitochondrial outer membrane permeabilization and caspase activation (77–80). This cell death can be inhibited by blocking cathepsin activity with protease inhibitors or by increasing the activity of iron chelators and endogenous cathepsin inhibitors, such as serpins and cystatins (81). However, under some circumstances, complete lysosomal rupture can also lead to uncontrolled necrosis. Ferroptosis is a regulated cell death pathway. A recent publication showed that salinomycin can kill CSCs by sequestering iron in lysosomes via a process triggered by severe lipid peroxidation related to ROS production and iron availability, and such cell death could be partially prevented by the ferroptosis inhibitor, ferrostatin-1, whereas the apoptosis and necrosis inhibitors Z-VAD-FMK and necrostatin-1, respectively, could not influence the cell death profiles (67). The possible molecular mechanisms underlying the effect of salinomycin through the lysosomal compartment on BC cells have been summarized in this review (see Figure 3).
Figure 3 Molecular mechanisms underlying the effects of salinomycin through the lysosomal compartment. Salinomycin is enriched in the lysosome of breast cancer tumor cells, leading to imbalance in Fe+ metabolism, excessive accumulation of Fe ions, and degradation of ferritin, which causes the release of a large amount of free radicals and ultimately induces LMP. Depending on the degree of damage to the lysosome, different responses will be triggered. If the damage to the lysosome is minor, the stress sensor and response mechanisms, including autophagy, will be activated to ensure cell survival. However, when the degree of LMP is very high, the damage cannot be repaired. As a result, special cathepsins will be released from the lysosome into the cytoplasm, causing the disruption of mitochondrial membrane integrity, leading to apoptosis. In the case of severe damage to the lysosome, the release of enzymes from the lysosome can also cause uncontrolled cell death, such as necrosis edit from (69).
As a potent therapeutic agent against cancer, salinomycin is still in the initial phase of the preclinical stage. Up to date, there is still no registered clinical trial about salinomycin. Two individual cases have been described in the literature as follows: one describing a 40-year-old female patient with metastatic (bone and subcutaneous) invasive ductal BC and another describing an 82-year-old female patient with advanced and metastatic (pelvic lymphatic metastasis) squamous cell carcinoma of the vulva. Both cases were treated systemically with intravenous administration of salinomycin (200–250 μg/kg salinomycin every second day), which partially regressed metastasis for 3 weeks and showed only minor adverse effects, as opposed to the severe adverse effects commonly observed with conventional chemotherapy (24). These findings confirm the safety and efficacy of salinomycin for selective clinical use. However, the potential toxicity of salinomycin may need to be studied in larger clinical trials.
Tumors are composed of heterogenous cell populations with various mutations and/or phenotypes. Therefore, it is generally ineffective to use a single drug to prevent cancer progression. For the complete eradication of cancers, several drugs with distinct mechanisms but complementary anticancer activities (i.e., combination therapies) are often used to enhance the antitumor efficacy and minimize the risk of developing drug resistance. The recent development of innovative delivery methods using biocompatible nanocarriers has enabled studies of various combinations. In particular, combination therapy with salinomycin can achieve promising results, and selective delivery via biocompatible nanocarriers provides a novel therapeutic approach (82–85). The effects of various combination strategies with salinomycin on BC cells have been summarized in Table 3.
Salinomycin and dasatinib (a Src kinase inhibitor) have a synergistic effect in BC cells via the horizontal suppression of multiple pathways (96). This effect possibly involves the promotion of cell cycle arrest at the G1/S phase through both the estrogen-mediated S phase entry and BRCA1 and DNA damage response pathways.
Additionally, salinomycin increases cancer cell sensitivity to the apoptotic effects of doxorubicin (DOX) or etoposide (ETO). pH2AX, pBRCA1, p53BP1, and pChk1 levels substantially increase in response to co-treatment with salinomycin and either DOX or ETO. The level of the anti-apoptotic p21 protein increases in response to DOX or ETO but decreases in response to salinomycin, which increases proteasome activity. These results indicate that the ability of salinomycin to sensitize cancer cells to DOX or ETO is associated with an increase in DNA damage and a decrease in p21 levels. Accordingly, salinomycin-based chemotherapy may be beneficial for patients with cancer receiving DOX or ETO (26).
The development of innovative delivery systems has significantly improved the efficacy of combination therapy (100). For example, Gao et al. (87) found that the synergistic effects of salinomycin and docetaxel could be effectively maintained in vivo by the co-encapsulation of these agents in PLGA/TPGS nanoparticles, providing a promising strategy for BC treatment.
Moreover, combining therapies targeting CSCs with conventional chemotherapy may be a useful strategy for cancer treatment (83). A single-walled carbon nanotube (SWCNT) drug delivery system combining paclitaxel, salinomycin, and biocompatible CD44 antibody-conjugated SWCNTs via a hydrazone linker had better effects than did individual drug-conjugated nanocarriers or free drug suspensions in both in vitro and in vivo assays (85). Moreover, another study has shown that an elastin-like polypeptide-salinomycin nanoparticle has a long release half-life in vitro and results in a lower CSC frequency in 4T1 orthotopic tumors than in untreated tumors or free salinomycin-treated tumors (84). Furthermore, HER2 is overexpressed in both breast CSCs and cancer cells and can also be utilized for targeted delivery systems. Salinomycin-loaded polymer-lipid hybrid anti-HER2 nanoparticles (Sali-NP-HER2) were developed to target both HER2-positive breast CSCs and cancer cells, with a high delivery efficacy and low untargeted toxicity (91).
Simultaneous eradication of both CSCs and cancer cells is necessary for optimizing therapeutic efficacy (101). The combination of salinomycin and traditional therapies has proven to be effective in BC; however, the proximal mechanisms underlying the effects of salinomycin on CSCs remain unclear and should be a focus of future studies.
The potency of tumor initiation and drug resistance of BCSCs are key factors contributing to the metastasis and recurrence of BC and thus increasing mortality. Salinomycin in combination with traditional chemotherapy and targeted therapy can kill both common cancer cells and CSCs, providing a very promising clinical strategy to treat BC. Salinomycin has multiple functions in the regulation of cellular processes in BC cells. Importantly, it functions in the regulation of cell death by a highly complex mechanism involving multiple pathways and interactions among several cell death-related biological processes. Its effects may be mediated by Bax/Bak, the activation of mitoptosis, irreversible deterioration of mitochondrial structure, or other apoptogenic factors associated with apoptosis released from mitochondria. Owing to the limited research focused on the mechanisms of action of salinomycin, further studies of its precise effects on apoptosis and autophagy, as well as the interactions among mitoptosis, mitophagy, ferroptosis, and necrosis are needed. In addition, suitable methods for the identification and isolation of CSCs are lacking despite their key importance for studies of the effects of salinomycin and the development of CSC-targeted therapy. Furthermore, a better understanding of the different characteristics of somatic stem cells and CSCs, such as the signaling pathways that regulate self-renewal and cell fate, would be important for the design of new strategies targeting CSCs.
HW, HZ, and FC: conceptualization. HW, HZ, and CC wrote the manuscript. YZ, ZW, and FC: manuscript review and critical comments. All authors contributed to the article and approved the submitted version.
This review article was supported by the National Natural Science Foundation of China (no. 81802961), the Shanghai Yangpu District Health and Family Planning Commission Fund for Hao Yi Shi Training Project (2020-2023), and the Natural Science Foundation of Shanghai (grant no. 18ZR1436000).
The authors declare that the research was conducted in the absence of any commercial or financial relationships that could be construed as a potential conflict of interest.
All claims expressed in this article are solely those of the authors and do not necessarily represent those of their affiliated organizations, or those of the publisher, the editors and the reviewers. Any product that may be evaluated in this article, or claim that may be made by its manufacturer, is not guaranteed or endorsed by the publisher.
We thank all of the participants for their participation.
1. Baccelli I, Riethdorf S, Stenzinger A, Schillert A, Vogel V. Identification of a Population of Blood Circulating Tumor Cells From Breast Cancer Patients That Initiates Metastasis in a Xenograft Assay. Nat Biotechnol (2013) 31:539–44. doi: 10.1038/nbt.2576
2. Scheel C, Weinberg RA. Cancer Stem Cells and Epithelial-Mesenchymal Transition: Concepts and Molecular Links. Semin Cancer Biol (2012) 22:396–403. doi: 10.1016/j.semcancer.2012.04.001
3. Pavlopoulou A, Oktay Y, Vougas K, Louka M, Vorgias CE, Georgakilas AG. Determinants of Resistance to Chemotherapy and Ionizing Radiation in Breast Cancer Stem Cells. Cancer Lett (2016) 380:485–93. doi: 10.1016/j.canlet.2016.07.018
4. Wang Y, Li W, Patel SS, Cong J, Zhang N, Sabbatino F, et al. Blocking the Formation of Radiation-Induced Breast Cancer Stem Cells. Oncotarget (2014) 5:3743–55. doi: 10.18632/oncotarget.1992
5. Pandrangi SL, Chikati R, Chauhan PS, Kumar CS, Banarji A, Saxena S. Effects of Ellipticine on ALDH1A1-Expressing Breast Cancer Stem Cells–An In Vitro and In Silico Study. Tumour Biol (2014) 35:723–37. doi: 10.1007/s13277-013-1099-y
6. Sims-Mourtada J, Opdenaker LM, Davis J, Arnold KM, Flynn D. Taxane-Induced Hedgehog Signaling Is Linked to Expansion of Breast Cancer Stem-Like Populations After Chemotherapy. Mol Carcinog (2015) 54:1480–93. doi: 10.1002/mc.22225
7. Mukherjee P, Gupta A, Chattopadhyay D, Chatterji U. Modulation of SOX2 Expression Delineates an End-Point for Paclitaxel-Effectiveness in Breast Cancer Stem Cells. Sci Rep (2017) 7:9170. doi: 10.1038/s41598-017-08971-2
8. Kolev VN, Wright QG, Vidal CM, Ring JE, Shapiro IM, Ricono J, et al. Pi3k/mTOR Dual Inhibitor VS-5584 Preferentially Targets Cancer Stem Cells. Cancer Res (2015) 75:446–55. doi: 10.1158/0008-5472.CAN-14-1223
9. Burnett JP, Lim G, Li Y, Shah RB, Lim R, Paholak HJ, et al. Sulforaphane Enhances the Anticancer Activity of Taxanes Against Triple Negative Breast Cancer by Killing Cancer Stem Cells. Cancer Lett (2017) 394:52–64. doi: 10.1016/j.canlet.2017.02.023
10. Miyazaki Y, Shibuya M, Sugawara H, Kawaguchi O, Hirsoe C. Salinomycin, a New Polyether Antibiotic. J Antibiot (Tokyo) (1974) 27:814–21. doi: 10.7164/antibiotics.27.814
11. Dewangan J, Srivastava S, Rath SK. Salinomycin: A New Paradigm in Cancer Therapy. Tumour Biol (2017) 39:101042831769503. doi: 10.1177/1010428317695035
12. Mitani M, Yamanishi T, Miyazaki Y, Otake N. Salinomycin Effects on Mitochondrial Ion Translocation and Respiration. Antimicrob Agents Chemother (1976) 9:655–60. doi: 10.1128/aac.9.4.655
13. Gupta PB, Onder TT, Jiang G, Tao K, Kuperwasser C, Weinberg RA, et al. Identification of Selective Inhibitors of Cancer Stem Cells by High-Throughput Screening. Cell (2009) 138:645–59. doi: 10.1016/j.cell.2009.06.034
14. Zhou J, Li P, Xue X, He S, Kuang Y, Zhao H, et al. Salinomycin Induces Apoptosis in Cisplatin-Resistant Colorectal Cancer Cells by Accumulation of Reactive Oxygen Species. Toxicol Lett (2013) 222:139–45. doi: 10.1016/j.toxlet.2013.07.022
15. Wang F, He L, Dai WQ, Xu YP, Wu D, Lin CL, et al. Salinomycin Inhibits Proliferation and Induces Apoptosis of Human Hepatocellular Carcinoma Cells In Vitro and In Vivo. PloS One (2012) 7:e50638. doi: 10.1371/journal.pone.0050638
16. Jangamreddy JR, Ghavami S, Grabarek J, Kratz G, Wiechec E, Fredriksson BA, et al. Salinomycin Induces Activation of Autophagy, Mitophagy and Affects Mitochondrial Polarity: Differences Between Primary and Cancer Cells. Biochim Biophys Acta (2013) 1833:2057–69. doi: 10.1016/j.bbamcr.2013.04.011
17. Li T, Su L, Zhong N, Hao X, Zhong D, Singhal S, et al. Salinomycin Induces Cell Death With Autophagy Through Activation of Endoplasmic Reticulum Stress in Human Cancer Cells. Autophagy (2013) 9:1057–68. doi: 10.4161/auto.24632
18. Tang QL, Zhao ZQ, Li JC, Liang Y, Yin JQ, Zou CY, et al. Salinomycin Inhibits Osteosarcoma by Targeting its Tumor Stem Cells. Cancer Lett (2011) 311:113–21. doi: 10.1016/j.canlet.2011.07.016
19. Koo KH, Kim H, Bae YK, Kim K, Park BK, Lee CH, et al. Salinomycin Induces Cell Death Via Inactivation of Stat3 and Downregulation of Skp2. Cell Death Dis (2013) 4:e693. doi: 10.1038/cddis.2013.223
20. Antoszczak M. A Medicinal Chemistry Perspective on Salinomycin as a Potent Anticancer and Anti-CSCs Agent. Eur J Med Chem (2019) 164:366–77. doi: 10.1016/j.ejmech.2018.12.057
21. Markowska A, Sajdak S, Huczynski A, Rehlis S, Markowska J. Ovarian Cancer Stem Cells: A Target for Oncological Therapy. Adv Clin Exp Med (2018) 27:1017–20. doi: 10.17219/acem/73999
22. Antoszczak M, Huczynski A. Anticancer Activity of Polyether Ionophore-Salinomycin. Anticancer Agents Med Chem (2015) 15:575–91. doi: 10.2174/1871520615666150101130209
23. He M, Fu Y, Yan Y, Xiao Q, Wu H, Yao W, et al. The Hedgehog Signalling Pathway Mediates Drug Response of MCF-7 Mammosphere Cells in Breast Cancer Patients. Clin Sci (Lond) (2015) 129:809–22. doi: 10.1042/CS20140592
24. Naujokat C, Steinhart R. Salinomycin as a Drug for Targeting Human Cancer Stem Cells. J BioMed Biotechnol (2012) 2012:950658. doi: 10.1155/2012/950658
25. An H, Kim JY, Oh E, Lee N, Cho Y, Seo JH. Salinomycin Promotes Anoikis and Decreases the CD44+/CD24- Stem-Like Population Via Inhibition of STAT3 Activation in MDA-MB-231 Cells. PloS One (2015) 10:e0141919. doi: 10.1371/journal.pone.0141919
26. Kim JH, Chae M, Kim WK, Kim YJ, Kang HS, Kim HS, et al. Salinomycin Sensitizes Cancer Cells to the Effects of Doxorubicin and Etoposide Treatment by Increasing DNA Damage and Reducing p21 Protein. Br J Pharmacol (2011) 162:773–84. doi: 10.1111/j.1476-5381.2010.01089.x
27. Tyagi M, Patro BS. Salinomycin Reduces Growth, Proliferation and Metastasis of Cisplatin Resistant Breast Cancer Cells Via NF-kB Deregulation. Toxicol In Vitro (2019) 60:125–33. doi: 10.1016/j.tiv.2019.05.004
28. Hori A, Shimoda M, Naoi Y, Kagara N, Tanei T, Miyake T, et al. Vasculogenic Mimicry Is Associated With Trastuzumab Resistance of HER2-Positive Breast Cancer. Breast Cancer Res (2019) 21:88. doi: 10.1186/s13058-019-1167-3
29. Batlle E, Clevers H. Cancer Stem Cells Revisited. Nat Med (2017) 23:1124–34. doi: 10.1038/nm.4409
30. Pattabiraman DR, Weinberg RA. Tackling the Cancer Stem Cells - What Challenges Do They Pose? Nat Rev Drug Discov (2014) 13:497–512. doi: 10.1038/nrd4253
31. Brooks MD, Burness ML, Wicha MS. Therapeutic Implications of Cellular Heterogeneity and Plasticity in Breast Cancer. Cell Stem Cell (2015) 17:260–71. doi: 10.1016/j.stem.2015.08.014
32. Pellegrini P, Dyczynski M, Sbrana FV, Karlgren M, Buoncervello M, Hagg-Olofsson M, et al. Tumor Acidosis Enhances Cytotoxic Effects and Autophagy Inhibition by Salinomycin on Cancer Cell Lines and Cancer Stem Cells. Oncotarget (2016) 7:35703–23. doi: 10.18632/oncotarget.9601
33. Zhang JY, Luo Q, Xu JR, Bai J, Mu LM, Yan Y, et al. Regulating Stem Cell-Related Genes Induces the Plastic Differentiation of Cancer Stem Cells to Treat Breast Cancer. Mol Ther Oncolytics (2020) 18:396–408. doi: 10.1016/j.omto.2020.07.009
34. An H, Kim JY, Lee N, Cho Y, Oh E, Seo JH. Salinomycin Possesses Anti-Tumor Activity and Inhibits Breast Cancer Stem-Like Cells Via an Apoptosis-Independent Pathway. Biochem Biophys Res Commun (2015) 466:696–703. doi: 10.1016/j.bbrc.2015.09.108
35. Ketola K, Hilvo M, Hyotylainen T, Vuoristo A, Ruskeepaa AL, Oresic M, et al. Salinomycin Inhibits Prostate Cancer Growth and Migration Via Induction of Oxidative Stress. Br J Cancer (2012) 106:99–106. doi: 10.1038/bjc.2011.530
36. Lu W, Li Y. Salinomycin Suppresses LRP6 Expression and Inhibits Both Wnt/Beta-Catenin and mTORC1 Signaling in Breast and Prostate Cancer Cells. J Cell Biochem (2014) 115:1799–807. doi: 10.1002/jcb.24850
37. Huang X, Borgstrom B, Stegmayr J, Abassi Y, Kruszyk M, Leffler H, et al. The Molecular Basis for Inhibition of Stemlike Cancer Cells by Salinomycin. ACS Cent Sci (2018) 4:760–7. doi: 10.1021/acscentsci.8b00257
38. Lu Y, Zhang C, Li Q, Mao J, Ma W, Yu X, et al. Inhibitory Effect of Salinomycin on Human Breast Cancer Cells MDA-MB-231 Proliferation Through Hedgehog Signaling Pathway. Zhonghua Bing Li Xue Za Zhi (2015) 44:395–8.
39. Lu Y, Ma W, Mao J, Yu X, Hou Z, Fan S, et al. Salinomycin Exerts Anticancer Effects on Human Breast Carcinoma MCF-7 Cancer Stem Cells Via Modulation of Hedgehog Signaling. Chem Biol Interact (2015) 228:100–7. doi: 10.1016/j.cbi.2014.12.002
40. Mai TT, Emi M, Tanabe K. Salinomycin Kills Cancer Stem Cells by Sequestering Iron in Lysosomes. Nat Chem (2017) 9:1025–33. doi: 10.1038/nchem.2778
41. Yue W, Hamai A, Tonelli G, Bauvy C, Nicolas V, Tharinger H. Inhibition of the Autophagic Flux by Salinomycin in Breast Cancer Stem-Like/Progenitor Cells Interferes With Their Maintenance. Autophagy (2013) 9:714–29. doi: 10.4161/auto.23997
42. Arfaoui A, Rioualen C, Azzoni V, Pinna G, Finetti P, Wicinski J, et al. A Genome-Wide RNAi Screen Reveals Essential Therapeutic Targets of Breast Cancer Stem Cells. EMBO Mol Med (2019) 11:e9930. doi: 10.15252/emmm.201809930
43. Niwa AM, GF DE, Marques LA, Semprebon SC, Sartori D, Ribeiro LR, et al. Salinomycin Efficiency Assessment in Non-Tumor (HB4a) and Tumor (MCF-7) Human Breast Cells. Naunyn Schmiedebergs Arch Pharmacol (2016) 389:557–71. doi: 10.1007/s00210-016-1225-7
44. Al Dhaheri Y, Attoub S, Arafat K, Abuqamar S, Eid A, Al Faresi N, et al. Salinomycin Induces Apoptosis and Senescence in Breast Cancer: Upregulation of p21, Downregulation of Survivin and Histone H3 and H4 Hyperacetylation. Biochim Biophys Acta (2013) 1830:3121–35. doi: 10.1016/j.bbagen.2013.01.010
45. Verdoodt B, Vogt M, Schmitz I, Liffers ST, Tannapfel A, Mirmohammadsadegh A. Salinomycin Induces Autophagy in Colon and Breast Cancer Cells With Concomitant Generation of Reactive Oxygen Species. PloS One (2012) 7:e44132. doi: 10.1371/journal.pone.0044132
46. Kim KY, Park KI, Kim SH, Yu SN, Lee D, Kim YW, et al. Salinomycin Induces Reactive Oxygen Species and Apoptosis in Aggressive Breast Cancer Cells as Mediated With Regulation of Autophagy. Anticancer Res (2017) 37:1747–58. doi: 10.21873/anticanres.11507
47. Dewangan J, Srivastava S, Mishra S, Divakar A, Kumar S, Rath SK. Salinomycin Inhibits Breast Cancer Progression Via Targeting HIF-1alpha/VEGF Mediated Tumor Angiogenesis In Vitro and In Vivo. Biochem Pharmacol (2019) 164:326–35. doi: 10.1016/j.bcp.2019.04.026
48. Kim R, Emi M, Tanabe K. Role of Mitochondria as the Gardens of Cell Death. Cancer Chemother Pharmacol (2006) 57:545–53. doi: 10.1007/s00280-005-0111-7
49. Van Herreweghe F, Festjens N, Declercq W, Vandenabeele P. Tumor Necrosis Factor-Mediated Cell Death: to Break or To Burst, That’s the Question. Cell Mol Life Sci (2010) 67:1567–79. doi: 10.1007/s00018-010-0283-0
50. Li P, Nijhawan D, Budihardjo I, Srinivasula SM, Ahmad M, Alnemri ES, et al. Cytochrome C and dATP-Dependent Formation of Apaf-1/Caspase-9 Complex Initiates an Apoptotic Protease Cascade. Cell (1997) 91:479–89. doi: 10.1016/s0092-8674(00)80434-1
51. Fuchs D, Daniel V, Sadeghi M, Opelz G, Naujokat C. Salinomycin Overcomes ABC Transporter-Mediated Multidrug and Apoptosis Resistance in Human Leukemia Stem Cell-Like KG-1a Cells. Biochem Biophys Res Commun (2010) 394:1098–104. doi: 10.1016/j.bbrc.2010.03.138
52. Gong C, Yao H, Liu Q, Chen J, Shi J, Su F, et al. Markers of Tumor-Initiating Cells Predict Chemoresistance in Breast Cancer. PloS One (2010) 5:e15630. doi: 10.1371/journal.pone.0015630
53. Kim JH, Yoo HI, Kang HS, Ro J, Yoon S. Salinomycin Sensitizes Antimitotic Drugs-Treated Cancer Cells by Increasing Apoptosis Via the Prevention of G2 Arrest. Biochem Biophys Res Commun (2012) 418:98–103. doi: 10.1016/j.bbrc.2011.12.141
54. Fuchs D, Heinold A, Opelz G, Daniel V, Naujokat C. Salinomycin Induces Apoptosis and Overcomes Apoptosis Resistance in Human Cancer Cells. Biochem Biophys Res Commun (2009) 390:743–9. doi: 10.1016/j.bbrc.2009.10.042
55. Kim KY, Yu SN, Lee SY, Chun SS, Choi YL, Park YM, et al. Salinomycin-Induced Apoptosis of Human Prostate Cancer Cells Due to Accumulated Reactive Oxygen Species and Mitochondrial Membrane Depolarization. Biochem Biophys Res Commun (2011) 413:80–6. doi: 10.1016/j.bbrc.2011.08.054
56. Jiang J, Li H, Qaed E, Zhang J, Song Y, Wu R, et al. Salinomycin, as an Autophagy Modulator– A New Avenue to Anticancer: A Review. J Exp Clin Cancer Res (2018) 37:26. doi: 10.1186/s13046-018-0680-z
57. Xipell E, Gonzalez-Huarriz M, Martinez de Irujo JJ, Garcia-Garzon A, Lang FF, Jiang H, et al. Salinomycin Induced ROS Results in Abortive Autophagy and Leads to Regulated Necrosis in Glioblastoma. Oncotarget (2016) 7:30626–41. doi: 10.18632/oncotarget.8905
58. Kopp F, Hermawan A, Oak PS, Herrmann A, Wagner E, Roidl A. Salinomycin Treatment Reduces Metastatic Tumor Burden by Hampering Cancer Cell Migration. Mol Cancer (2014) 13:16. doi: 10.1186/1476-4598-13-16
59. Hero T, Buhler H, Kouam PN, Priesch-Grzeszowiak B, Lateit T, Adamietz IA. The Triple-negative Breast Cancer Cell Line Mda-MB 231 Is Specifically Inhibited by the Ionophore Salinomycin. Anticancer Res (2019) 39:2821–7. doi: 10.21873/anticanres.13410
60. Zhang C, Lu Y, Li Q, Mao J, Hou Z, Yu X, et al. Salinomycin Suppresses TGF-beta1-Induced Epithelial-to-Mesenchymal Transition in MCF-7 Human Breast Cancer Cells. Chem Biol Interact (2016) 248:74–81. doi: 10.1016/j.cbi.2016.02.004
61. Markowska A, Sajdak S, Markowska J, Huczynski A. Angiogenesis and Cancer Stem Cells: New Perspectives on Therapy of Ovarian Cancer. Eur J Med Chem (2017) 142:87–94. doi: 10.1016/j.ejmech.2017.06.030
62. Shen H, Sun CC, Kang L, Tan X, Shi P, Wang L, et al. Low-Dose Salinomycin Inhibits Breast Cancer Metastasis by Repolarizing Tumor Hijacked Macrophages Toward the M1 Phenotype. Eur J Pharm Sci (2021) 157:105629. doi: 10.1016/j.ejps.2020.105629
63. Ebokaiwe AP, Njoya EM, Sheng Y, Zhang Z, Li S, Zhou Z, et al. Salinomycin Promotes T-Cell Proliferation by Inhibiting the Expression and Enzymatic Activity of Immunosuppressive Indoleamine-2,3-dioxygenase in Human Breast Cancer Cells. Toxicol Appl Pharmacol (2020) 404:115203. doi: 10.1016/j.taap.2020.115203
64. Newman DJ, Cragg GM. Natural Products as Sources of New Drugs From 1981 to 2014. J Nat Prod (2016) 79:629–61. doi: 10.1021/acs.jnatprod.5b01055
65. Gerry CJ, Schreiber SL. Chemical Probes and Drug Leads From Advances in Synthetic Planning and Methodology. Nat Rev Drug Discovery (2018) 17:333–52. doi: 10.1038/nrd.2018.53
66. Dutton CJ, Banks BJ, Cooper CB. Polyether Ionophores. Nat Prod Rep (1995) 12:165–81. doi: 10.1039/np9951200165
67. Versini A, Colombeau L, Hienzsch A, Gaillet C, Retailleau P, Debieu S, et al. Salinomycin Derivatives Kill Breast Cancer Stem Cells by Lysosomal Iron Targeting. Chemistry (2020) 26:7416–24. doi: 10.1002/chem.202000335
68. Dixon SJ, Stockwell BR. The Role of Iron and Reactive Oxygen Species in Cell Death. Nat Chem Biol (2014) 10:9–17. doi: 10.1038/nchembio.1416
69. Wang F, Gomez-Sintes R, Boya P. Lysosomal Membrane Permeabilization and Cell Death. Traffic (2018) 19:918–31. doi: 10.1111/tra.12613
70. Fehrenbacher N, Bastholm L, Kirkegaard-Sorensen T, Rafn B, Bottzauw T, Nielsen C, et al. Sensitization to the Lysosomal Cell Death Pathway by Oncogene-Induced Down-Regulation of Lysosome-Associated Membrane Proteins 1 and 2. Cancer Res (2008) 68:6623–33. doi: 10.1158/0008-5472.CAN-08-0463
71. Truman JP, Garcia-Barros M, Obeid LM, Hannun YA. Evolving Concepts in Cancer Therapy Through Targeting Sphingolipid Metabolism. Biochim Biophys Acta (2014) 1841:1174–88. doi: 10.1016/j.bbalip.2013.12.013
72. Dielschneider RF, Eisenstat H, Mi S, Curtis JM, Xiao W, Johnston JB, et al. Lysosomotropic Agents Selectively Target Chronic Lymphocytic Leukemia Cells Due to Altered Sphingolipid Metabolism. Leukemia (2016) 30:1290–300. doi: 10.1038/leu.2016.4
73. Gyrd-Hansen M, Nylandsted J, Jaattela M. Heat Shock Protein 70 Promotes Cancer Cell Viability by Safeguarding Lysosomal Integrity. Cell Cycle (2004) 3:1484–5. doi: 10.4161/cc.3.12.1287
74. Xia P, Gamble JR, Wang L, Pitson SM, Moretti PA, Wattenberg BW, et al. An Oncogenic Role of Sphingosine Kinase. Curr Biol (2000) 10:1527–30. doi: 10.1016/s0960-9822(00)00834-4
75. Aits S, et al. Sensitive Detection of Lysosomal Membrane Permeabilization by Lysosomal Galectin Puncta Assay. Autophagy (2015) 11:1408–24. doi: 10.1080/15548627.2015.1063871
76. Papadopoulos C, Kirchner P, Bug M, Grum D, Koerver L, Schulze N, et al. Vcp/p97 Cooperates With YOD1, UBXD1 and PLAA to Drive Clearance of Ruptured Lysosomes by Autophagy. EMBO J (2017) 36:135–50. doi: 10.15252/embj.201695148
77. Aits S, Jaattela M. Lysosomal Cell Death at a Glance. J Cell Sci (2013) 126:1905–12. doi: 10.1242/jcs.091181
78. Galluzzi L, Vitale I, Aaronson SA, Abrams JM, Adam D, Agostinis P, et al. Molecular Mechanisms of Cell Death: Recommendations of the Nomenclature Committee on Cell Death 2018. Cell Death Differ (2018) 25:486–541. doi: 10.1038/s41418-017-0012-4
79. Serrano-Puebla A, Boya P. Lysosomal Membrane Permeabilization in Cell Death: New Evidence and Implications for Health and Disease. Ann NY Acad Sci (2016) 1371:30–44. doi: 10.1111/nyas.12966
80. de Castro MA, Bunt G, Wouters FS. Cathepsin B Launches an Apoptotic Exit Effort Upon Cell Death-Associated Disruption of Lysosomes. Cell Death Discovery (2016) 2:16012. doi: 10.1038/cddiscovery.2016.12
81. Gomez-Sintes R, Ledesma MD, Boya P. Lysosomal Cell Death Mechanisms in Aging. Ageing Res Rev (2016) 32:150–68. doi: 10.1016/j.arr.2016.02.009
82. Kai M, Kanaya N, Wu SV, Mendez C, Nguyen D, Luu T, et al. Targeting Breast Cancer Stem Cells in Triple-Negative Breast Cancer Using a Combination of LBH589 and Salinomycin. Breast Cancer Res Treat (2015) 151:281–94. doi: 10.1007/s10549-015-3376-5
83. Dubrovska A, Elliott J, Salamone RJ, Kim S, Aimone LJ, Walker JR, et al. Combination Therapy Targeting Both Tumor-Initiating and Differentiated Cell Populations in Prostate Carcinoma. Clin Cancer Res (2010) 16:5692–702. doi: 10.1158/1078-0432.CCR-10-1601
84. Zhao P, Dong S, Bhattacharyya J, Chen M. iTEP Nanoparticle-Delivered Salinomycin Displays an Enhanced Toxicity to Cancer Stem Cells in Orthotopic Breast Tumors. Mol Pharm (2014) 11:2703–12. doi: 10.1021/mp5002312
85. Al Faraj A, Shaik AS, Ratemi E, Halwani R. Combination of Drug-Conjugated SWCNT Nanocarriers for Efficient Therapy of Cancer Stem Cells in a Breast Cancer Animal Model. J Control Release (2016) 225:240–51. doi: 10.1016/j.jconrel.2016.01.053
86. Wang T, Narayanaswamy R, Ren H, Torchilin VP. Combination Therapy Targeting Both Cancer Stem-Like Cells and Bulk Tumor Cells for Improved Efficacy of Breast Cancer Treatment. Cancer Biol Ther (2016) 17:698–707. doi: 10.1080/15384047.2016.1190488
87. Gao J, Liu J, Xie F, Lu Y, Yin C, Shen X. Co-Delivery of Docetaxel and Salinomycin to Target Both Breast Cancer Cells and Stem Cells by PLGA/TPGS Nanoparticles. Int J Nanomed (2019) 14:9199–216. doi: 10.2147/IJN.S230376
88. Zhang Y, Zhang H, Wang X, Wang J, Zhang X, Zhang Q. The Eradication of Breast Cancer and Cancer Stem Cells Using Octreotide Modified Paclitaxel Active Targeting Micelles and Salinomycin Passive Targeting Micelles. Biomaterials (2012) 33:679–91. doi: 10.1016/j.biomaterials.2011.09.072
89. Muntimadugu E, Kumar R, Saladi S, Rafeeqi TA, Khan W. CD44 Targeted Chemotherapy for Co-Eradication of Breast Cancer Stem Cells and Cancer Cells Using Polymeric Nanoparticles of Salinomycin and Paclitaxel. Colloids Surf B Biointerfaces (2016) 143:532–46. doi: 10.1016/j.colsurfb.2016.03.075
90. Liang DS, Liu J, Peng TX, Peng H, Guo F, Zhong HJ, et al. Vitamin E-based Redox-Sensitive Salinomycin Prodrug-Nanosystem With Paclitaxel Loaded for Cancer Targeted and Combined Chemotherapy. Colloids Surf B Biointerfaces (2018) 172:506–16. doi: 10.1016/j.colsurfb.2018.08.063
91. Li J, Xu W, Yuan X, Chen H, Song H, Wang B, et al. Polymer-Lipid Hybrid Anti-HER2 Nanoparticles for Targeted Salinomycin Delivery to HER2-positive Breast Cancer Stem Cells and Cancer Cells. Int J Nanomed (2017) 12:6909–21. doi: 10.2147/IJN.S144184
92. Aydin RS. Herceptin-Decorated Salinomycin-Loaded Nanoparticles for Breast Tumor Targeting. J BioMed Mater Res A (2013) 101:1405–15. doi: 10.1002/jbm.a.34448
93. Oak PS, Kopp F, Thakur C, Ellwart JW, Rapp UR, Ullrich A, et al. Combinatorial Treatment of Mammospheres With Trastuzumab and Salinomycin Efficiently Targets HER2-Positive Cancer Cells and Cancer Stem Cells. Int J Cancer (2012) 131:2808–19. doi: 10.1002/ijc.27595
94. Sommer AK, Hermawan A, Mickler FM, Ljepoja B, Knyazev P, Brauchle C, et al. Salinomycin Co-Treatment Enhances Tamoxifen Cytotoxicity in Luminal A Breast Tumor Cells by Facilitating Lysosomal Degradation of Receptor Tyrosine Kinases. Oncotarget (2016) 7:50461–76. doi: 10.18632/oncotarget.10459
95. Manmuan S, Sakunrangsit N, Ketchart W. Salinomycin Overcomes Acquired Tamoxifen Resistance Through AIB1 and Inhibits Cancer Cell Invasion in Endocrine Resistant Breast Cancer. Clin Exp Pharmacol Physiol (2017) 44:1042–52. doi: 10.1111/1440-1681.12806
96. Bellat V, Verchere A, Ashe SA, Law B. Transcriptomic Insight Into Salinomycin Mechanisms in Breast Cancer Cell Lines: Synergistic Effects With Dasatinib and Induction of Estrogen Receptor Beta. BMC Cancer (2020) 20:661. doi: 10.1186/s12885-020-07134-3
97. Choi AR, Jung MJ, Kim JH, Yoon S. Co-Treatment of Salinomycin Sensitizes AZD5363-Treated Cancer Cells Through Increased Apoptosis. Anticancer Res (2015) 35:4741–7.
98. Dewangan J, Tandon D, Srivastava S, Verma AK, Yapuri A, Rath SK, et al. Novel Combination of Salinomycin and Resveratrol Synergistically Enhances the Anti-Proliferative and Pro-Apoptotic Effects on Human Breast Cancer Cells. Apoptosis (2017) 22:1246–59. doi: 10.1007/s10495-017-1394-y
99. Rai G, Suman S, Mishra S, Shukla Y. Evaluation of Growth Inhibitory Response of Resveratrol and Salinomycin Combinations Against Triple Negative Breast Cancer Cells. BioMed Pharmacother (2017) 89:1142–51. doi: 10.1016/j.biopha.2017.02.110
100. Shi J, Kantoff PW, Wooster R, Farokhzad OC. Cancer Nanomedicine: Progress, Challenges and Opportunities. Nat Rev Cancer (2017) 17:20–37. doi: 10.1038/nrc.2016.108
Keywords: salinomycin, breast cancer, mechanism, clinical application, cancer stem cells
Citation: Wang H, Zhang H, Zhu Y, Wu Z, Cui C and Cai F (2021) Anticancer Mechanisms of Salinomycin in Breast Cancer and Its Clinical Applications. Front. Oncol. 11:654428. doi: 10.3389/fonc.2021.654428
Received: 16 January 2021; Accepted: 28 June 2021;
Published: 26 July 2021.
Edited by:
Xiaosong Chen, Shanghai Jiao Tong University, ChinaReviewed by:
Adam Huczyński, Adam Mickiewicz University, PolandCopyright © 2021 Wang, Zhang, Zhu, Wu, Cui and Cai. This is an open-access article distributed under the terms of the Creative Commons Attribution License (CC BY). The use, distribution or reproduction in other forums is permitted, provided the original author(s) and the copyright owner(s) are credited and that the original publication in this journal is cited, in accordance with accepted academic practice. No use, distribution or reproduction is permitted which does not comply with these terms.
*Correspondence: Fengfeng Cai, Y2FpZmVuZ2ZlbmdAdG9uZ2ppLmVkdS5jbg==; Chunhong Cui, Y3VpY2hAc3VtaHMuZWR1LmNu
†These authors have contributed equally to this work and share first authorship
‡These authors have contributed equally to this work and share last authorship
Disclaimer: All claims expressed in this article are solely those of the authors and do not necessarily represent those of their affiliated organizations, or those of the publisher, the editors and the reviewers. Any product that may be evaluated in this article or claim that may be made by its manufacturer is not guaranteed or endorsed by the publisher.
Research integrity at Frontiers
Learn more about the work of our research integrity team to safeguard the quality of each article we publish.