- 1Department of Biomedical Engineering, Department of Biomedicine and Health Sciences, College of Medicine, The Catholic University of Korea, Seoul, South Korea
- 2College of Medicine, Research Institute of Biomedical Engineering, The Catholic University of Korea, Seoul, South Korea
- 3Department of Radiation Oncology, Seoul National University Bundang Hospital, Seongnam, South Korea
- 4Department of Radiation Oncology, Seoul National University Hospital, Seoul, South Korea
- 5Department of Radiation Oncology, Seoul National University Boramae Medical Center, Seoul, South Korea
- 6Department of Radiation Oncology, Ajou University School of Medicine, Suwon, South Korea
- 7Departments of Radiation Oncology, Dongnam Institute of Radiological & Medical Sciences, Busan, South Korea
- 8Department of Radiation Oncology, University of Texas Southwestern Medical Center, Dallas, TX, United States
Purpose: The aim of this study was to develop a dosimetric verification system (DVS) using a solid phantom for patient-specific quality assurance (QA) of high-dose-rate brachytherapy (HDR-BT).
Methods: The proposed DVS consists of three parts: dose measurement, dose calculation, and analysis. All the dose measurements were performed using EBT3 film and a solid phantom. The solid phantom made of acrylonitrile butadiene styrene (ABS, density = 1.04 g/cm3) was used to measure the dose distribution. To improve the accuracy of dose calculation by using the solid phantom, a conversion factor [CF(r)] according to the radial distance between the water and the solid phantom material was determined by Monte Carlo simulations. In addition, an independent dose calculation program (IDCP) was developed by applying the obtained CF(r). To validate the DVS, dosimetric verification was performed using gamma analysis with 3% dose difference and 3 mm distance-to-agreement criterion for three simulated cases: single dwell position, elliptical dose distribution, and concave elliptical dose distribution. In addition, the possibility of applying the DVS in the high-dose range (up to 15 Gy) was evaluated.
Results: The CF(r) between the ABS and water phantom was 0.88 at 0.5 cm. The factor gradually increased with increasing radial distance and converged to 1.08 at 6.0 cm. The point doses 1 cm below the source were 400 cGy in the treatment planning system (TPS), 373.73 cGy in IDCP, and 370.48 cGy in film measurement. The gamma passing rates of dose distributions obtained from TPS and IDCP compared with the dose distribution measured by the film for the simulated cases were 99.41 and 100% for the single dwell position, 96.80 and 100% for the elliptical dose distribution, 88.91 and 99.70% for the concave elliptical dose distribution, respectively. For the high-dose range, the gamma passing rates in the dose distributions between the DVS and measurements were above 98% and higher than those between TPS and measurements.
Conclusion: The proposed DVS is applicable for dosimetric verification of HDR-BT, as confirmed through simulated cases for various doses.
Introduction
High dose-rate brachytherapy (HDR-BT) can be used to effectively treat cancer by delivering high doses of radiation locally and improving both target coverage and organ sparing. Its effectiveness is remarkably high for large clinical targets with complex topologies (1). However, the risks during HDR-BT are higher than those during external beam radiotherapy when a treatment accident occurs and the causing error is not immediately identified. Errors in HDR-BT have been reported in the previous studies (2–5), and they are mainly caused by inappropriate radiation source selection, source strength units, entry into a treatment planning system (TPS), and source dwell position.
To prevent such errors, recommendations and guidelines for quality assurance (QA) of HDR-BT have been proposed (6–10). Most existing QA procedures are performed as basic tests of specific dosimetric parameters and include measuring the source activity, verifying the source position, and checking the timer accuracy and linearity using well-type chambers, special rulers, and established techniques for teletherapy sources. Although existing QA procedures are suitable for conventional HDR-BT, they may fail for the latest HDR-BT interventions. This is because the accuracy assessment paradigm for HDR-BT has shifted from determining the conventional source dwell pattern using 2D imaging to patient-specific 3D image-based optimization and inverse planning. Therefore, QA of HDR-BT based on dosimetric verification has been addressed recently (11–14).
Qi et al. (11) performed treatment plan verification for HDR-BT using a specific water phantom and metal–oxide–semiconductor field-effect transistor. Palmer et al. (12) proposed dose distribution verification using radiochromic film dosimetry in clinical brachytherapy and found that the EBT3 GAFCHROMICTM film can perform accurate dose verification in HDR-BT owing to its excellent spatial resolution, tissue equivalence, and self-development properties. In addition, they compared planned with measured dose distributions using an in-house water phantom. However, these methods can only be used to measure dose in a specific water phantom. To overcome this limitation, various methods have been proposed to replace water phantoms with solid phantoms by adopting water equivalent materials (13, 14). Meigooni et al. (13) obtained the conversion factor (CF) for media between water and various materials, such as solid water, polystyrene, and acrylic, using Monte Carlo (MC) simulations. Aldelaijan et al. (14) used the CF to perform dosimetric QA of HDR-BT with the Solid WaterTM phantom replacing a water phantom. However, the CF has not been applied to compare the planned and measured dose distributions but only to evaluate point doses due to limitations of commercial TPSs. In fact, dosimetric verification of HDR-BT using a commercially available TPS generally provides dose distributions by using embedded algorithms based on the AAPM (American Association of Physicists in Medicine) Task Group 43U1 report (15). These algorithms assume that the overall dose calculation is done in water, and access to the algorithm is restricted. Therefore, several previous studies have been either limited to specific water phantoms to measure and verify the dose distribution in the treatment plan or focused on evaluation of point doses by applying the CF (11–14).
In this study, a dosimetric verification system (DVS) intended for the solid phantom was developed for HDR-BT. First, we fabricated a solid phantom that can be used for film dosimetry and calculate CF(r), a CF value that is a function of the radial distance between water and the phantom material. Second, we developed an independent dose calculation program (IDCP) to apply the obtained CF(r). Third, we compared the gamma evaluation for the dose distribution calculated by the IDCP with the measured dose distribution obtained by using EBT3 film in the solid phantom. Thus, this study aimed to demonstrate the feasibility of the proposed DVS as a patient-specific QA tool for HDR-BT through various simulated cases.
Materials and Methods
Simple Solid Phantom
Figure 1 shows the solid phantom made of acrylonitrile butadiene styrene (ABS, density: 1.04 g/cm3) used to measure the dose distribution. The solid phantom comprises a normal slab (dimensions 30 × 30 × 1 cm3) and a catheter-inserted slab (dimensions 30 × 30 × 2 cm3). The catheter-inserted slab contains a parallel hole in the center of one side and the depth of the hole is 133 mm. The hole accommodated the catheter of 3 mm, and the end of the hole was attached to the catheter tip.
Conversion Factor Between Water and ABS
The CF(r) between water and ABS was determined as a function of radial distance r by MC simulations using the Geant4 Application for Tomographic Emission (GATE4, Version 8.1). For the MC simulations, the 192Ir mHDR-v2 source was modeled as described by Granero et al. (16), and the calculated grid size was 1 × 1 × 1 mm3. The electromagnetic standard model, option3 (Emstandard_opt3), was selected from the physics engine list. The virtual phantoms with water and ABS had the same dimensions, 30 × 30 × 20 cm3. The ABS was composed of 45.5% carbon, 51.5% hydrogen, and 3% nitrogen (17). The CF(r) was obtained by the ratio of the dose profiles for ABS and water, ABS/water, along the vertical axis with respect to the source.
Independent Dose Calculation Program (IDCP)
The proposed IDCP combines the obtained CF(r) to calculate the dose in the solid phantom. The IDCP is based on the AAPM Task Group 43U1 report and the dose calculation algorithm proposed in our previous study (18). The line source model is implemented in the IDCP, and the equation of the model is expressed as
where r is the distance from the center of the source, θ is the polar angle between the source longitudinal axes, r0 and θ0 are the reference distance (1 cm) and angle (90°), respectively, and the air-kerma strength (unit U: cGy·cm2·h−1), dose-rate constant (unit: cGy·h−1·U−1), geometry factor, radial dose function, and anisotropy function are denoted as SK, Λ, G(r, θ), g(r), and F(r, θ), respectively. The values of SK and Λ are obtained from the TPS, and those of g(r) and F(r, θ) are provided by the source manufacturer. Then, CF(r) can be obtained through the MC simulations.
Validation of the Developed Dosimetric Verification System Using Film Dosimetry
All the measurements were performed using EBT3 film (Ashland ISP Advanced Materials, NJ, USA) from a single batch. Before film dosimetry, doses of 0–19 Gy were irradiated using a 6 MV external photon beam generated by VitalBeam (Varian Medical Systems, Palo Alto, CA, USA) to calibrate the film. The net optical density (netOD) curve was obtained from two channels (i.e., red and green), as shown in Figure 2, because each channel has a different sensitivity depending on the dose range. Specifically, the red channel provides the optimal performance at doses below 10 Gy, whereas the green channel is adaptable to high doses above 10 Gy. The netOD curve was applied with an appropriate selection for the dose range in the simulated cases.
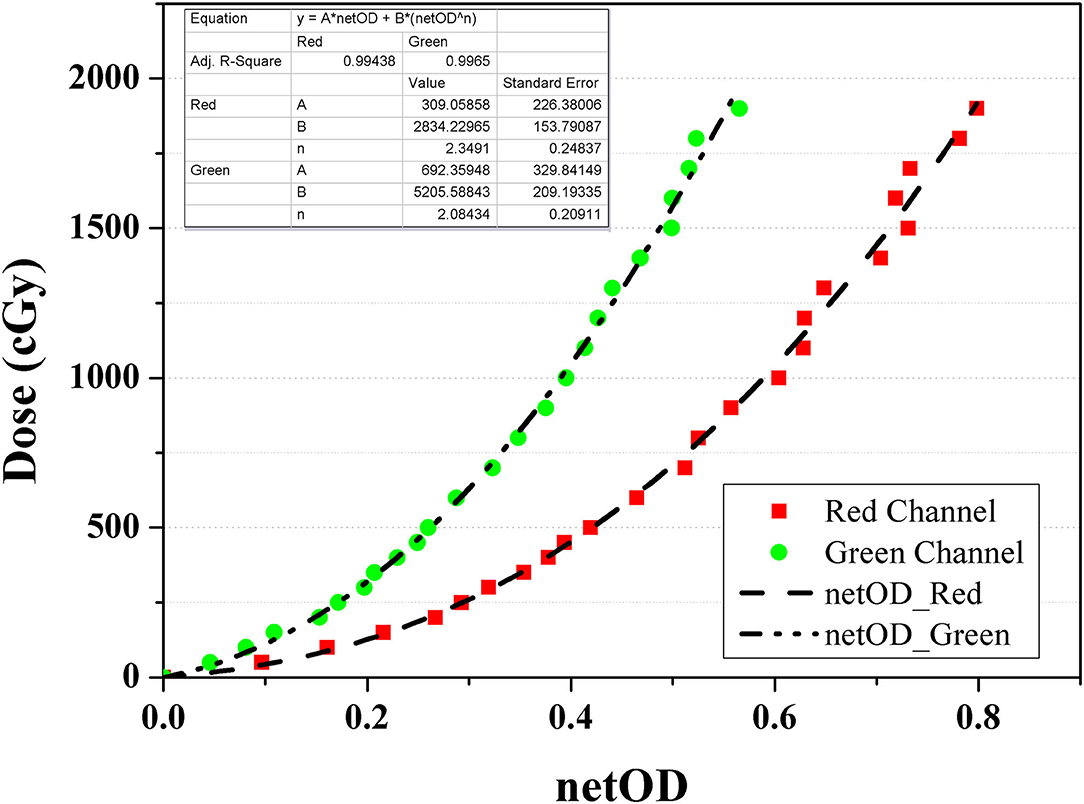
Figure 2. Net optical density curve for GAFCHROMIC EBT3 film calibration using red (square) and green (circle) channel.
The measurements for DVS validation were performed 1 cm below the source. The radiation exposure was implemented by NUCLETRON microSelectron Afterloader (Elekta, Stockholm, Sweden) with an 192Ir mHDR-v2 source. The measurements adhered to the procedure for handling EBT3 film recommended by the AAPM Task Group 53 report. The DVS validation was executed by dosimetric verifications for three cases: First, the single dwell position was measured to evaluate the feasibility of the CF(r) and DVS operation. The source was in the catheter tip in the phantom, and doses of 4 Gy were irradiated 1 cm below the source. The corresponding dose distributions and profiles according to the angle were then measured. Second, the elliptical dose distributions at the same dwell-time at a linear dwell position of 5 mm was measured. Third, the concave elliptical dose distribution was established using different dwell-times, and the corresponding dose distribution was measured. We also evaluated the applicability of the DVS for high-dose by performing dosimetric verification for various high-dose ranges including 9.5, 10.75, 13.5, and 15 Gy.
Each dose distribution on the same measurement plane was calculated using the Oncentra Brachy software (Elekta) and the proposed IDCP. The gamma analysis developed by Low et al. (19) was used to evaluate the measured and calculated dose distributions using global normalization with a 3% dose difference and 3 mm distance to agreement (3%/3 mm criterion).
Results
Percentage Dose and CF(r) for Water and ABS Phantom Using MC Simulation
Figure 3 shows the calculated percentage dose profiles according to the radial distances obtained from the virtual water and ABS phantoms in the MC simulations. The CF(r) obtained from the ratio between both profiles is also depicted. All profiles were normalized with the calculated dose at a radial distance of 1 cm from the virtual source for water. The percentage dose for the radial distance of 1 cm was 93.20% in ABS, being lower than that in water. In addition, the acquired profiles for water were higher than those for ABS up to a radial distance of 2.1 cm and smaller for radial distances above 2.1 cm. The percentage difference between both profiles was 47% at a radial distance of 0.5 cm and gradually decreased until the difference between profiles can be negligible. At a radial distance above 1.5 cm, the difference was below 1%.
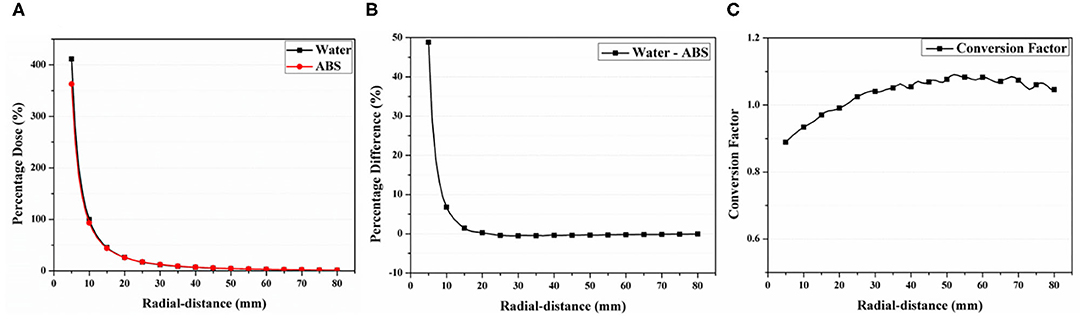
Figure 3. (A) Percentage dose profiles calculated by Monte Carlo simulation for water and acrylonitrile butadiene styrene (ABS) material. (B) Percentage difference between calculated dose profiles and (C) conversion factor obtained using ABS/water ratio.
The CF(r) was 0.88 at a radial distance of 0.5 cm and gradually increased until a radial distance of 6 cm, reaching 1.08 [Figure 3 (bottom)]. At a radial distance above 6 cm, the value slowly decreased, reaching 1.04 at 8 cm. For both materials, the CF(r) was 1 at a radial distance of 2.1 cm. In this study, the values of CF(r) were only obtained within 8 cm because the percentage dose difference between both profiles at radial distances above 8 cm was negligible (< 1.5%).
Validation of the Developed Dosimetric Verification System
Figure 4 shows the isodose map obtained from the film measurements, IDCP, and TPS at a single dwell position. The central point dose for each dose distribution was 3.70 Gy in film measurements, 3.73 Gy for the IDCP, and 4 Gy for the TPS. The IDCP and film measurement profiles were similar. Although the TPS profile was higher than the other profiles within 0.8 cm from the center of the isodose map, there was no considerable difference in each profile according to the angle.
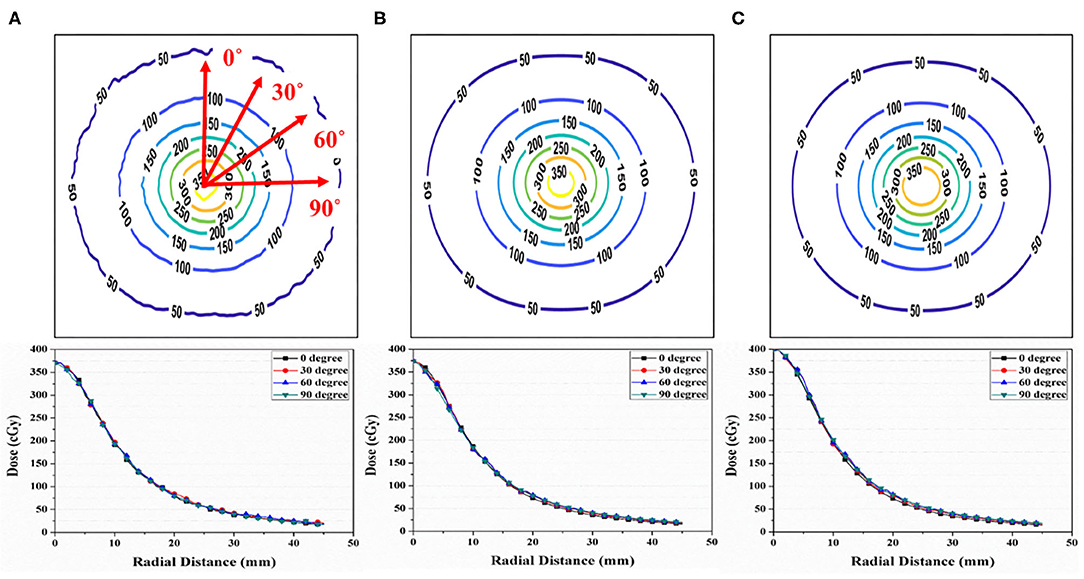
Figure 4. (A) Isodose map from EBT3 film measurement. (B) Independent dose calculation program (IDCP) and (C) treatment planning system (TPS) results at a single dwell position and 400 cGy. Each dose profile was obtained at angles of 0, 30, 60, and 90°.
Figure 5 shows the gamma analysis between the measured and calculated dose distributions for the three simulated cases. Based on the measured dose distribution at a single dwell position, the gamma passing rates using the 3%/3 mm criterion were 99.41 and 100% for the TPS and DVS, respectively. In the dose distribution near the source, there was a lower passing rate in the TPS than in the DVS. For the elliptical dose distribution, the passing rates analyzed with 3%/3 mm criterion were 96.80 and 100% for the TPS and DVS, respectively. The gamma failure of the TPS was higher than that of the DVS near the source. For the concave elliptical dose distribution, the gamma passing rates were 88.91 and 99.70% for the TPS and DVS, respectively.
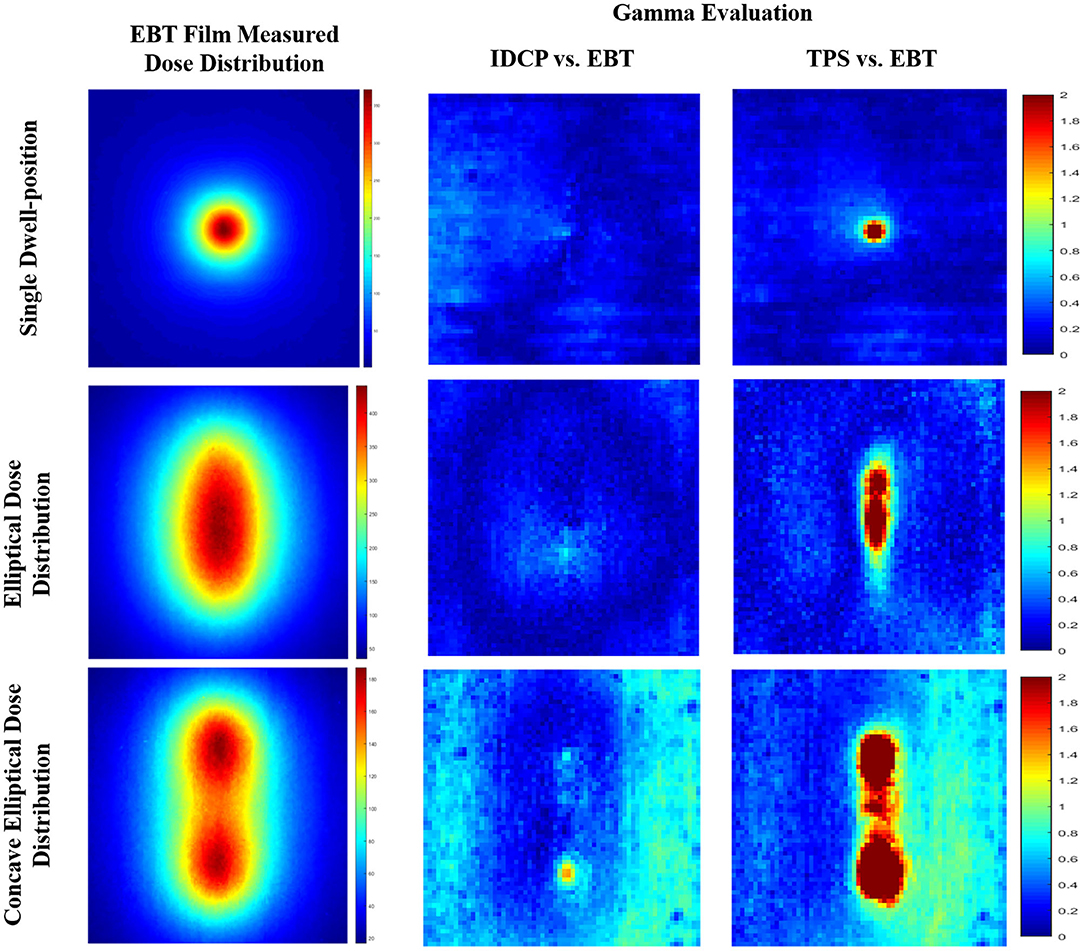
Figure 5. Gamma analysis between measured and calculated dose distributions using 3%/3 mm criterion for three simulated cases: single dwell position, elliptical dose distribution, and concave elliptical dose distribution.
Figure 6 shows the gamma analysis for the concave elliptical dose distributions at high doses. The gamma passing rates using the 3%/3 mm criterion in the dose distributions between the DVS results and measurements were 98.12, 98.32, 99.68, and 98.36% for 9.50, 10.75, 13.50, and 15 Gy, respectively. For these doses, the gamma passing rates in the dose distributions between the TPS results and measurements were 84.76, 89.71, 91.92, and 89.75%, respectively. Compared with the measured distributions, the dose distributions calculated by the DVS have higher gamma passing rates than those calculated by the TPS at all the high doses.
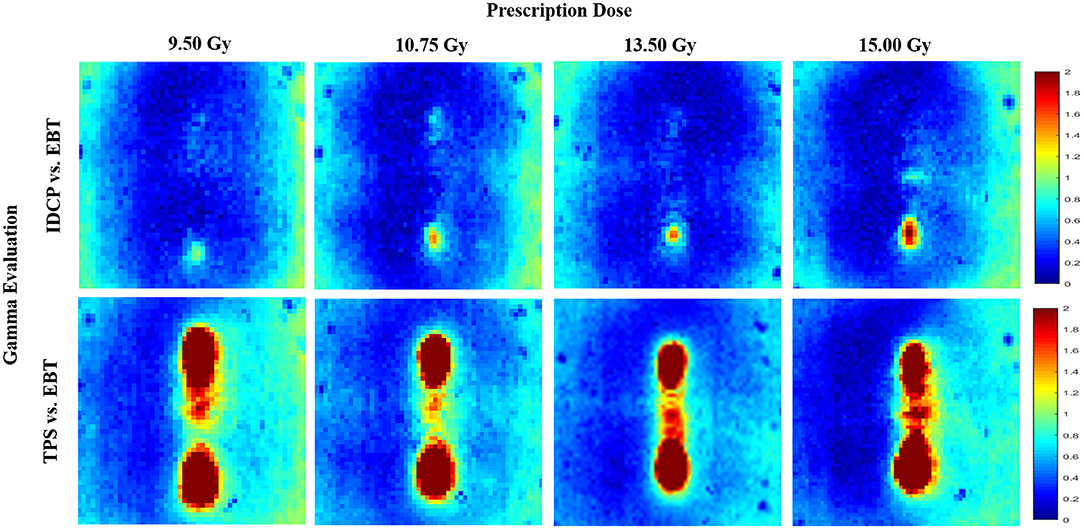
Figure 6. Gamma analysis between measured and calculated dose distributions using 3%/3 mm criterion for concave elliptical dose distribution at high doses.
Discussion
Accurate QA procedures for HDR-BT are important to increase the likelihood of desired treatment outcomes, minimize the risk of errors in clinical practice, and ensure the efficacy of clinical trials. In previous studies (11–14), these dosimetric verifications were able to perform point-dose measurements using the specific water phantom or Solid WaterTM phantom. However, these QA procedures had limitations in its practical application because there were inconvenient to use a specific water phantom and were insufficient to verify the treatment plan with only point-dose measurement. Therefore, the DVS, which can easily apply QA and verify dose distribution as well as point-dose, was developed in this study.
The CF(r) is the most important factor for the DVS to perform the dosimetric verification with the solid-phantom. The concept of the CF has already been reported in previous studies (13, 14, 20). However, since the CF was obtained only at a certain-depth and was limitedly used to verify the point-dose, it was not applied to convert the dose distribution in the solid phantom. In this study, we determined the modified CF as a function of the radial distance, the CF(r), and applied it to the IDCP to calculate the accurate dose distribution in the solid phantom. Through the DVS validation, it has been proven that the QA procedure using IDCP and ABS solid phantom can be applied to the dosimetric verification of HDR-BT if the CF(r) is appropriately considered.
To investigate the feasibility of the DVS for QA of HDR-BT, we compared the gamma evaluations of the dose distributions calculated by the DVS/TPS with those measured using film for the three cases under various dose ranges. All gamma passing rates were higher in the dose distributions between the DVS results and measurements than in those between the TPS results and measurements. Thus, the dose distribution calculated by the DVS is more consistent with that measured by the film. In addition, the results suggest that the DVS establishes an effective verification method for complex dose distributions using high dose ranges. Thus, we believe that the DVS can be used as a QA tool for pretreatment verification in HDR-BT.
We consider that the DVS can support the verification of the dosimetric parameters of the source and the QA of HDR-BT. If dosimetric parameters such as radial dose and anisotropic functions are not accurate, the dose distribution cannot be calculated correctly. This can be simply verified by comparison with the measured dose distribution at a single dwell position. In addition, the evaluation of the elliptical dose distribution can support various QA procedures for HDR-BT, such as source position verification, timer accuracy, and linearity testing.
For pretreatment verification of HDR-BT, the DVS was derived from patient-specific QA used in external beam radiotherapy delivery techniques, such as intensity modulated radiation therapy and volumetric modulated arc therapy. The HDR-BT treatment plan was established by determining the dwell position of the source with respect to the shape of the applicator and using multiple catheters depending on the number of channels. To perform pretreatment verification in the DVS, the complex dwell position of each channel was modified to a linear dwell position that fitted the catheter hole in the solid phantom. Thus, the verification of the treatment plan for HDR-BT can be performed using the DVS and the solid phantom. However, the treatment plan converter was not applied in this study because only a simulated plan instead of a clinical plan was used. In a future study, we will perform dosimetric verification in clinical cases using the DVS to apply the treatment plan converter.
To evaluate the DVS performance, film dosimetry was used in this study. Although films are generally energy dependent, some studies have demonstrated that the EBT3 film can be used in dose measurements for HDR-BT. Parmer et al. (11) reported the successful application of the EBT3 film to dose measurements in HDR-BT. In addition, Devic et al. (21) noted that the energy range response of the film does not change by more than 0.3%. Consistent with previous studies, the DVS relies on the EBT3 film to verify treatment plans generated at high doses as well as doses for actual clinical conditions.
Conclusion
The proposed DVS is applicable for dosimetric verification, as demonstrated through simulated cases at various doses. From this study, we believe that the DVS can be used for QA of HDR-BT and to deliver more accurate and safer treatments. In addition, this study showed the possibility of performing patient-specific QA of HDR-BT using a solid phantom instead of a water phantom if CF(r) is correctly determined.
Data Availability Statement
The original contributions presented in the study are included in the article/supplementary material, further inquiries can be directed to the corresponding author/s.
Author Contributions
J-BC and TS supervised the project. S-WK, SK, and JP conceived and designed the experiments. CC, H-JP, K-YE, J-YK, CS, IK, and J-SK. contributed the experiments. S-WK, K-HK, SK, D-SS, and WC built the in-house software. S-WK, J-BC, and JP wrote the manuscript. All authors contributed to the article and approved the submitted version.
Funding
This work was supported by the National Research Foundation of Korea (NRF) grant funded by the Korea Government (Ministry of Science and ICT, MSIT) (No. 2018R1D1A1B07049159 and 2020R1C1C100936611) and grant no. 13-2016-012 from the SNUBH Research Fund.
Conflict of Interest
The authors declare that the research was conducted in the absence of any commercial or financial relationships that could be construed as a potential conflict of interest.
References
1. Guthier CV, Devilin PM, Harris TC, O'Farrell DA, Cormack RA, Buzurovic I, et al. Development and clinical implementation of semi-automated treatment planning including 3D printable applicator holders in complex skin brachytherapy. Med Phys. (2020) 47:869–79. doi: 10.1002/mp.13975
2. Palmer A. Impact of software changes: transit dose and source position accuracy of the Eckert and Ziegler BEBIG GmbH MultiSource high dose rate (HDR) brachytherapy treatment unit. J Radiother Pract. (2012) 12:80–7. doi: 10.1017/S1460396912000192
3. Daigh JD. Department of Veterans Affairs Office of Inspector General, Health Inspection: Review of Brachytherapy Treatment of Prostate Cancer, Philadelphia, Pennsylvania and Other VA Medical Centres. Washington, DC (2010).
4. Stern RL, Liu T. Dwell position inaccuracy in the Varian GammaMed HDR ring applicator. J Appl Clin Med Phys. (2010) 11:1–6. doi: 10.1120/jacmp.v11i4.3158
5. NRC. NRC information notice 2009-17: reportable medical eventsinvolving treatment delivery errors caused by confusion of unitsfor the specification of brachytherapy sources. (2009). Available online at: https://www.nrc.gov/docs/ML0807/ML080710054.pdf
6. Nath R, Anderson LL, Meli JA, Olch AJ, Stitt JA, Williamson JF. Code of practice for brachytherapy physics: report of the AAPM radiation therapy committee task group No. 56. Med Phys. (1997) 24:1557–98. doi: 10.1118/1.597966
7. Mayles WPM, Lake R, McKenzie A. Physics Aspects of Quality Control in Radiotherapy Report Number 81. New York, NY: The Institute of Physics and Engineering in Medicine (IPEM) (1999).
8. Vanselaar J, Perez-Calatayud J. A Practical Guide to Quality Control of Brachytherapy Equipment. Brussels: European Guidelines for Quality Assurance in Radiotherapy (2004).
9. Wilkinson DA. High dose rate (HDR) brachytherapy quality assurance: a practical guide. Biomed Imaging Interv J. (2006) 2:e34. doi: 10.2349/biij.2.2.e34
10. American College of Radiology. ACR-AAPM Technical Standards for the Performance of High-Dose-Rate Brachytherapy Physics. (2020). Available online at: https://www.acr.org/-/media/ACR/Files/Practice-Parameters/hdr-brachyts.pdf?la=en
11. Qi ZY, Deng XW, Huang SM, Lu J, Lerch M, Cutajar D, et al. Verification of the plan dosimetry for high dose rate brachytherapy using metal–oxide–semiconductor field effect transistor detectors. Med Phys. (2007) 34:2007–13. doi: 10.1118/1.2736288
12. Palmer AL, Nisbet Bradley AD. Verification of high dose rate brachytherapy dose distributions with EBT3 Gafchromic film quality control techniques. Phys Med Biol. (2013) 58:497–511. doi: 10.1088/0031-9155/58/3/497
13. Meigooni AS, Awan SB, Thompson NS, Dini SA. Updated Solid Water™ to water conversion factors for 125I and 103Pd brachytherapy sources. Med Phys. (2006) 33:3988–92. doi: 10.1118/1.2357018
14. Alderlaijan S, Mohammed H, Tomic N, Liang LH, DeBlois F, Sarfehnia A, et al. Radiochromic film dosimetry of HDR 192Ir source radiation fields. Med Phys. (2011) 38:6074–83. doi: 10.1118/1.3651482
15. Rivard MJ, Coursey BN, DeWerd LA, Hanson WF, Huq MS, Ibbott GS, et al. Update of AAPM task group no. 43 report: a revised AAPM protocol for brachytherapy dose calculations. Med Phys. (2004) 31:633–74. doi: 10.1118/1.1646040
16. Granero D, Vijande J, Ballester F, Rivard MJ. Dosimetry revisited for the HDR 192Ir brachytherapy source model mHDR-v2. Med Phys. (2011) 38:487–94. doi: 10.1118/1.3531973
17. Adams DW. A Comparison of MCNP Modeling Against Empirical Data for the Measurement of Gamma Fields Due to Actinide Oxide in a Glove box, Colorado State University. (2012). Available online at: http://hdl.handle.net/10217/72373
18. Kang SW, Chung JB, Kim KH, Park JY, Park HJ, Cho W, et al. Development of volumetric independent dose calculation system for verification of the treatment plan in image-guided adaptive brachytherapy. Front Oncol. (2020) 10:1–9. doi: 10.3389/fonc.2020.00609
19. Daniel AL, James FD. Evaluation of the gamma dose distribution comparison method. Med Phys. (2003) 30:2455–64. doi: 10.1118/1.1598711
20. Williamson JF. Comparison of measured and calculated dose rates in water near I-125 and Ir-192 seeds. Med Phys. (1991) 18:776–86. doi: 10.1118/1.596631
Keywords: patient-specific quality assurance, dosimetric verification, high-dose-rate brachytherapy, film measurement, independent dose calculation
Citation: Kang S-W, Chung J-B, Kim K-H, Choi CH, Kang S, Shin D-S, Cho W, Eom K-Y, Park H-J, Kim J-Y, Song C, Kim IA, Kim J-S, Suh TS and Park JC (2021) Development of Dosimetric Verification System for Patient-Specific Quality Assurance of High-Dose-Rate Brachytherapy. Front. Oncol. 11:647222. doi: 10.3389/fonc.2021.647222
Received: 29 December 2020; Accepted: 15 February 2021;
Published: 09 March 2021.
Edited by:
Amar U. Kishan, University of California, Los Angeles, United StatesReviewed by:
Steven N. Seyedin, University of California, Irvine, United StatesHilary Bagshaw, Stanford University, United States
Copyright © 2021 Kang, Chung, Kim, Choi, Kang, Shin, Cho, Eom, Park, Kim, Song, Kim, Kim, Suh and Park. This is an open-access article distributed under the terms of the Creative Commons Attribution License (CC BY). The use, distribution or reproduction in other forums is permitted, provided the original author(s) and the copyright owner(s) are credited and that the original publication in this journal is cited, in accordance with accepted academic practice. No use, distribution or reproduction is permitted which does not comply with these terms.
*Correspondence: Jin-Beom Chung, jbchung1213@gmail.com; Tae Suk Suh, suhsanta@catholic.ac.kr