- 1Institute of Pharmacy and Pharmacology, University of South China, Hengyang, China
- 2Department of Biomedical Sciences, City University of Hong Kong, Hong Kong, China
- 3The Second Affiliated Hospital of Guilin Medical University, Guilin, China
- 4Department of Experimental Animals, University of South China, Hengyang, China
- 5Pharmacy School of Guilin Medical University, Guilin, China
- 6Shanghai Veterinary Research Institute, Chinese Academy of Agriculture Science, Shanghai, China
Tristetraprolin (TTP), a well-known RNA-binding protein, primarily affects the expression of inflammation-related proteins by binding to the targeted AU-rich element in the 3’ untranslated region after transcription and subsequently mediates messenger RNA decay. Recent studies have focused on the role of TTP in tumors and their related microenvironments, most of which have referred to TTP as a potential tumor suppressor involved in regulating cell proliferation, apoptosis, and metastasis of various cancers, as well as tumor immunity, inflammation, and metabolism of the microenvironment. Elevated TTP expression levels could aid the diagnosis and treatment of different cancers, improving the prognosis of patients. The aim of this review is to describe the role of TTP as a potential safeguard against carcinoma.
Introduction
Tristetraprolin (TTP), also known as ZFP36, NUP475, G0/G1 switch regulatory protein 24 (GOS24), and TPA-inducible sequence 11 (TIS11), is a well-known RNA-binding protein (1, 2). It is an early immediate response gene located on chromosome 19q13.2, contains one intron and two exons and encodes a 1.7 kb messenger RNA (mRNA) transcript and a 34 kD protein (3). The unique features of the structure of TTP include three proline-rich motifs, two conserved tandem zinc-finger (TZF) domains with the CX8CX5CX3H sequence, and several serine/threonine phosphorylation sites such as S66, S88, T92, S169, S186, S197, and S228 (4, 5).
TTP acts at the transcription, translation, and post-transcription cellular levels (6). Post-transcription regulation, during which TTP targets the AU-rich element (ARE)-containing mRNA and affects its stability, is essential for cells to rapidly respond to intracellular and extracellular stimuli (7). TTP optimally combines with 9-mer UUAUUUAUU and the relevant binding sequence at the 3′-UTR of the target mRNA through the TZF domain. Thus, TTP promotes the instability and degradation of mRNA (8). Additionally, TTP can shorten the poly (A) tail of the target mRNA of cytokines and mediate mRNA decay through the 3-5 exosome and 5-3 Xrn1 exonuclease pathways (9). In the 3-5 pathway, TTP identifies and recruits the exosome to ARE-mRNAs (10). After the 7-methyl guanosine cap is removed by TTP in the decapping complex, decay progresses in the 5-3 pathway. Subsequently, ARE transcripts are transferred to the processing body by TTP (11). Therefore, when factors related to inflammation and cancer are overexpressed, TTP interacts with specific mRNA to repress several crucial gene transcripts and rapidly degrade ARE-directed binding proteins (12).
In recent years, TTP has been found to be associated with some pro-inflammatory and cancer-promoting proteins, such as tumor necrosis factor (TNF) alpha, C-X-C motif chemokine receptor 4, mRNA-decapping enzyme 2, and enhancer of mRNA-decapping protein 3 (13, 14). By interacting with specific mRNA, TTP blocks post-transcriptional activity and protein synthesis and downregulates the expression of these mRNAs to counteract the pro-tumorigenic effects of these tumor growth factors (15, 16).
Roles of TTP in Carcinoma
Compared with that of normal tissues analyzed by GEPIA (Figure 1), mRNA expression of TTP is significantly lower in adrenocortical carcinoma (ACC), bladder urothelial carcinoma (BLCA), breast invasive carcinoma (BRCA), cervical squamous cell carcinoma (CESC), and colon adenocarcinoma (COAD) tissues. Furthermore, the expression level is always associated with clinicopathological features, overall survival rate, and patient prognosis (18–22). Some reports have illustrated that Area Under Curve (AUC) of TTP in prostate cancer is 0.84 to 0.85 (The closer to 1.0, the higher the authenticity of the detection is) using bioinformatics data combined with clinical model. Besides, lower level of TTP is related to more aggressive phenotype in HCC. Loss of TTP will show high correlation with late-stage, high-grade of HCC (23, 24). Therefore, TTP could be considered a tumor suppressor in most cancers, and upregulating TTP expression might help improve prognosis. Besides, TTP is involved in many cancer processes, including the proliferation, apoptosis, and metastasis. In the following, a detailed role of TTP will be stated respectively.
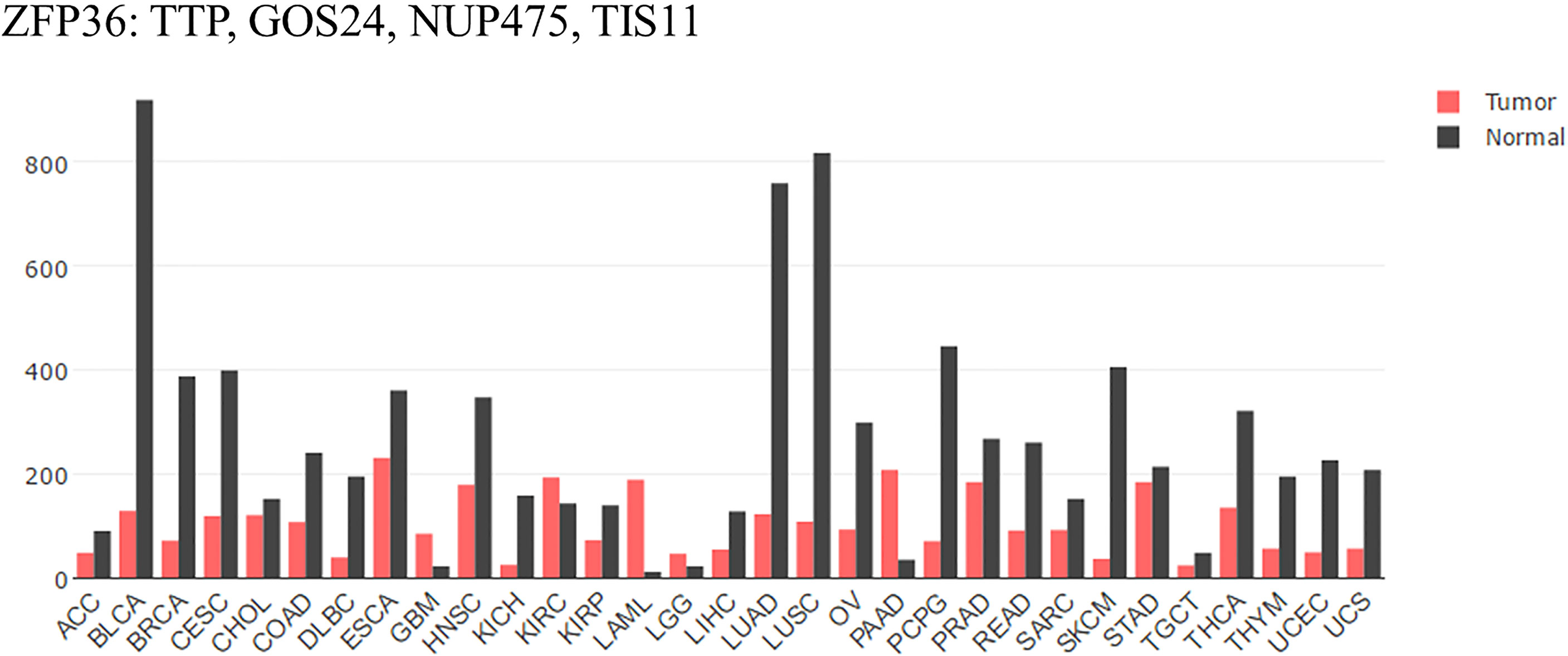
Figure 1 TTP/ZFP36 expression in cancer and normal tissues analyzed by GEPIA. Compared with normal tissues analyzed by GEPIA; TTP mRNA expression is significantly lower in the tissues of most forms of carcinoma. ACC, Adrenocortical carcinoma; BLCA, Bladder urothelial carcinoma; BRCA, Breast invasive carcinoma; CESC, Cervical squamous cell carcinoma; CHOL, Cholangiocarcinoma; COAD, Colon adenocarcinoma; DLBC, Lymphoid Neoplasm Diffuse Large B-cell Lymphoma; ESCA, Esophageal carcinoma; GBM, Glioblastoma multiforme; HNSC, Head and Neck squamous cell carcinoma. KICH, Kidney Chromophobe; KIRC, Kidney renal clear cell carcinoma; KIRP, Kidney renal papillary cell carcinoma; LAML, Acute Myeloid Leukemia; LGG, Brain Lower Grade Glioma; LIHC; liver hepatocellular carcinoma; LUAD; lung adenocarcinoma; LUSC; lung squamous cell carcinoma; OV, ovarian serous cystadenocarcinoma; PAAD, pancreatic adenocarcinoma; PCPG, Pheochromocytoma and Paraganglioma; PRAD, Prostate adenocarcinoma; READ, Rectum adenocarcinoma; SARC, Sarcoma; SKCM, Skin Cutaneous Melanoma; STAD, Stomach adenocarcinoma; TGCT, Testicular Germ Cell Tumors; THCA, Thyroid carcinoma; THYM, Thymoma; UCEC, Uterine Corpus Endometrial Carcinoma; UCS, Uterine Carcinosarcoma (17).
TTP Inhibits the Proliferation
The abnormality of protooncogene and tumor suppressors results in the tumorigenesis. Next, the uncontrolled cell replication and division stimulated by cancerous signal transduction promotes the continual proliferation. In this part, TTP has been reported from two sides, one is as a post-transcriptional regulator in plasma, and the other is as a transcriptional regulator in the nucleus (25).
TTP as a Post-Transcriptional Regulator in Plasma
TTP can inhibit the progression of several cancers (26–28). In 2009, TTP was first described to impair nuclear translocation of nuclear factor kappa-B (NF-κB) p65 (29). Thus, TTP and NF-κB target genes are also involved in mediating inflammatory responses in the cell cycle (30). In 2015, TTP was found to affect the c-Jun/Wee1 axis at the transcriptional level in an NF-κB-dependent manner in breast cancer. Being a transcriptional co-repressor that binds with the C-terminal Zn finger of NF-κB p65, TTP blocks p65 translocation. As a result, the decreased c-Jun level and increased Wee1 expression could delay the transition from S to G2 in the cell cycle, thereby reducing cell proliferation (26). Three years later, the same research group found that TTP inhibited the proliferation of lung adenocarcinoma cells by inducing cell cycle arrest in the S phase, which might be related to autophagy. Soon after, TTP overexpression was shown to downregulate autophagy-related mRNA and protein levels, such as those of Beclin1 and LC3II/I in lung cancer cells (27). In addition, TTP could also inhibit the proliferation of non-small cell lung cancer. Being an inhibitor of histone deacetylases (HDAC), sodium butyrate (NaBu) could downregulate Cyclin B1 expression by accelerating TTP binding with the 3′-UTR of Cyclin B1, which prevents the cell cycle transition from G1 to S and G2 to M (28).
Not merely TTP/ZFP36 itself, the other members of ZFP36 family including ZFP36L1 and L2 in mammals, L3 only in rodents (Figure 2) are all relevant with proliferation suppression of malignant neoplasms. In colorectal cancer (31), ZFP36L1 and L2 could inhibit proliferation by inducing cell cycle arrest in the G1 phase via Cyclin D- and p53-dependent pathways. Forced expression of ZFP36L1 or L2 downregulates cell cycle-related proteins such as Cyclin A, B, and D and p21, but upregulates p53 mRNA. Defects in ZFP36L1 and L2 in TZF did not lead to the inhibition of colorectal cancer proliferation, suggesting that TZF is a key factor in the regulation of ZFP36L1 and L2 (31).
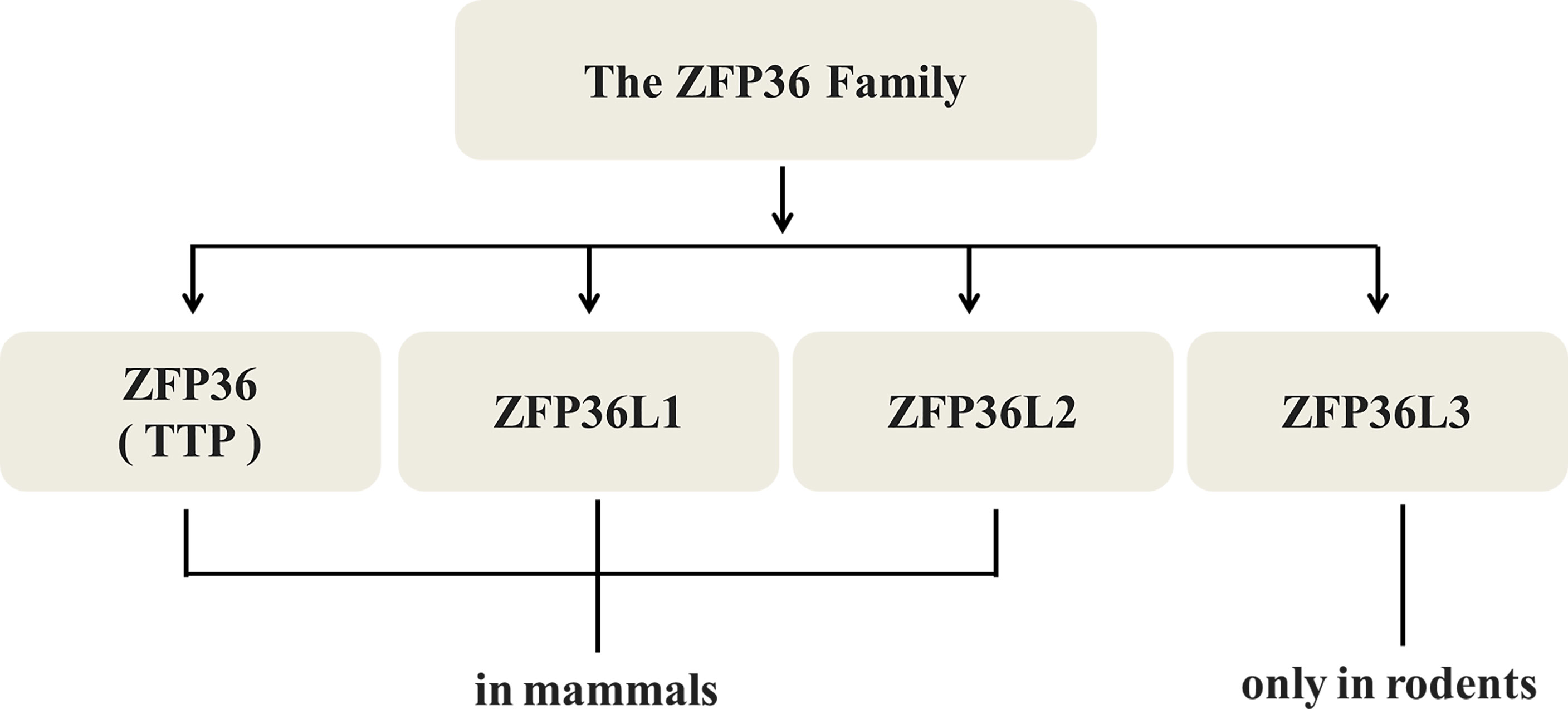
Figure 2 Types of TTP/ZFP36. TTP/ZFP36 along with ZFP36L1 and L2, all members of the ZFP36 family share similar structures and functions in mammals, while L3 is found only in rodents.
TTP as a Transcriptional Regulator in the Nucleus
As a vital transcription factor, TTP transcription also plays a crucial role in inhibiting cell proliferation through mRNA decay. In A549 lung cancer cells, Smad3, a regulatory protein involved in transforming growth factor beta (TGF-β) signaling, can bind to the promoter region of TTP (32). Subsequently, TTP transcription and increased expression leads to a decrease in the mRNA expression of cyclooxygenase-2 (COX-2), a pro-inflammatory factor that can inhibit the proliferation of cancer cells (33). In colorectal cancer cells, HDAC has a negative effect on transcription and silences tumor suppressor genes, such as TTP, in an epigenetic manner. Therefore, HDAC inhibitors such as trichostatin A, SAHA, and NaBu can induce TTP transcription by activating early growth reactive protein 1, another transcription factor (34). Increase in TTP expression was shown to promote COX-2 mRNA degradation and inhibit colorectal cancer cell proliferation (35). In breast cancer cells, TTP expression can be induced by p53 and inhibited by c-Myc (36). In contrast, metformin is believed to promote p53 and suppress c-Myc expression through the AMPK pathway. Moreover, metformin exerts its effects by inducing the promoter activity of TTP transcription (37).
TTP Induces the Apoptosis
Different from necrosis, the apoptosis refers to the natural programed cell death. For mammals, it is a critical adjustment to maintain homeostasis by eliminating senile or useless cells (25). B-cell lymphoma 2 (Bcl-2) is an anti-apoptotic protein overabundant in cancer cells that induces tumor resistance to anticancer drugs such as cisplatin (38). TTP enhances cisplatin sensitivity of squamous cell carcinoma of the head and neck (SCCHN) by inhibiting Bcl-2 expression at the post-transcriptional level, thereby enhancing cell apoptosis (39). Globular adiponectin (gAcrp), known for its anti-tumor effects, may accelerate AU-rich binding with the proteins TTP and AUF1. TTP subsequently promotes apoptosis of hepatocellular carcinoma (HCC) by promoting Bcl-2 mRNA instability associated with caspase-3 enhancement (40). Ripoptosome, composed of receptor-interacting protein kinase 1 (RIP1), caspase-8, and Fas-associating protein with a novel death domain (FADD), has been described in recent years as a cell death-inducing complex that aggregates in cancer cells (41–43). Its stability is regulated by the inhibitor of apoptosis proteins (IAPs) E3 ubiquitin ligase, XIAP, cIAP1, and baculoviral IAP repeat containing 3 (cIAP2) (44). TTP reduces the mRNA stability and expression of cIAP2 and XIAP (45, 46), which activates the death complex. RIP1 stimulation promotes the aggregation of RIP1, caspase 8, and FADD, as well as the apoptosis of glioma cancer stem cells (47). However, TTP is not expressed when the promoter is methylated or phosphorylated (48). MAPKAP kinase 2 (MK2) promotes tumor development through TTP phosphorylation (49). The combination of MK2 with a DNA methylation inhibitor (5-aza-dC) or with interferon α restores TTP expression and stimulates the apoptosis of HCC cells by upregulating apoptotic genes such as caspase-4 and -8 (50). The balance between the proliferation and apoptosis of cancer cells is critical for tumor development (51). The TTP signaling pathways in cancers are shown in Figure 3.
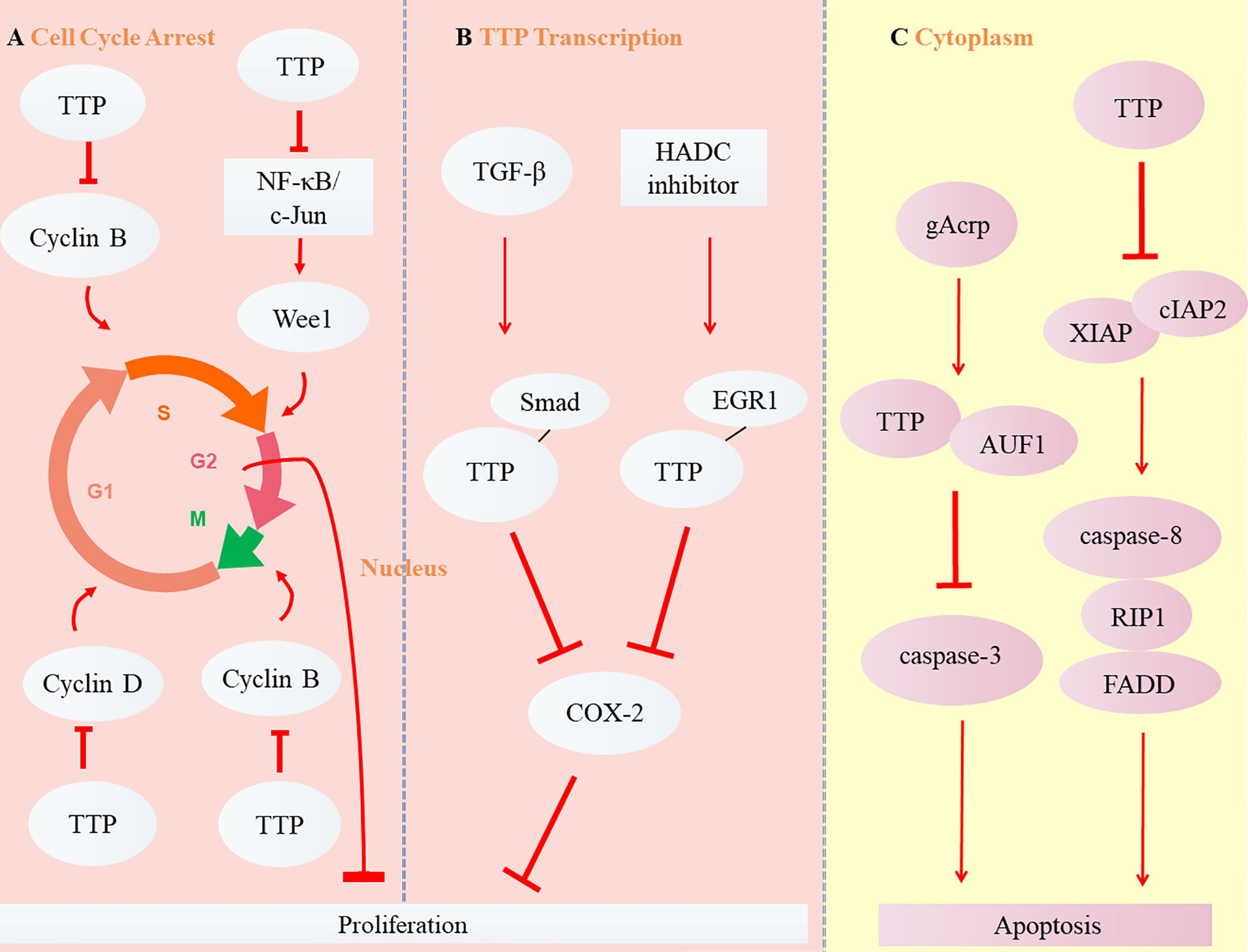
Figure 3 Role of TTP in carcinoma. (A) TTP inhibits Cyclin B expression by inducing the arrest of cell cycle from Phase G1 to S or G2 to M, Cyclin D expression in Phase G1, and Wee1 expression from Phase S to G2, thus suppressing the proliferation of cancer cells. (B) The transcription of TTP, induced by TGF-β and HADC inhibitor, binds with the other transcription factors, Smad and EGR1. Subsequently, TTP inhibits COX-2 expression and suppresses the proliferation of cancer cells. (C) Two cytoplasmic signaling pathways of TTP are involved in the apoptosis of tumor cells.
TTP Reins in the Metastasis
Metastasis is the main cause of death in cancer patients. When cancer cells exacerbate, they gradually invade in the epithelial cells through extracellular matrix (ECM). Along with the angiogenesis in the microenvironment, the cells spread to distant tissues of body through blood or lymph vessels. Plasminogen activator (uPA) and its receptor uPAR will be the key factors in the invasion. At the same time, TTP can delay the invasion and migration of cancer cells (25). TTP can counteract the invasion of glioma cells by directly binding the 3-UTR mRNA of urokinase uPA and uPAR, thus reducing mRNA abundance and subsequent protein expression (52–54). IL-13 is involved in regulating the proliferation, invasion, and metastasis of cancer cells by activating the PI3K/Akt/mTOR pathway (55, 56). TTP may inhibit the role of PI3K/Akt/mTOR by targeting IL-13 mRNA (20), thereby reducing the growth and invasion of glioma cells. Increased IL-33 expression is closely related to the growth and metastasis of cancer cells (57, 58). In gastric cancer cells, upregulation of TTP inhibits the proliferation, invasion, and migration of cancer cells by negatively correlating with IL-33 expression (22). PIM2, a kinase that is overabundant in cancer, phosphorylates a series of proteins critical for tumor progression (59). By binding to the TZF region of TTP, PIM2 not only promotes the degradation of TTP protein through the ubiquitin-proteasome pathway, but also inhibits the proliferation and migration of breast cancer cells (60).
The delay of metastasis depends on its regulation as well as that of the TTP-HuR axis. At present, thousands of overlapping binding sites between TTP and human antigen R (HuR), which are both antagonistic RNA-binding proteins, have been detected (61). TTP attenuates the targeted mRNAs, while HuR usually plays an essential role in stabilizing the targeted transcripts and promoting mRNA translation (62). Dysregulation of the TTP-HuR axis may increase the factors associated with cancer development (63). Elevation of high mobility group box 1 (HMGB1), a typical damage-related molecule, is often associated with gastric cancer (64). HuR, which has been found to be increased in gastric cancer, promotes HMGB1 expression at the translational level (65). TTP then attenuates the invasion and migration of gastric cancer cells by downregulating HuR expression (63, 65). In non-small cell lung cancer tissues, TTP expression is positively correlated with miR-133b, while HuR expression is negatively correlated with the expression of miR-133b and TTP (66). Consequently, by interacting with TTP-HuR axis (regaining TTP expression but reducing HuR level), miR-133b will rein in the development and progression of cancer (66).
TTP Acts as a Potential Safeguard Against Carcinoma Due to Its Role in the Tumor Microenvironment (TME)
Tumor development depends not only on genetic changes, but also on changes in the constituents of the TME, such as cytokines, growth factors, hormones, extracellular matrix (ECM), blood vessels, and invasive inflammation (67). In particular, the TME can release extracellular signals, promote tumor progression (especially for angiogenesis), and induce peripheral immune tolerance. Inflammation and immunity are the core components that drive the onset and development of cancer (68). Studies have recognized the role of TTP in the TME based on its participation in tumor-related inflammation and immunity, thus affecting the progress of cancer and predicting poor survival (69). The dynamic changes that occur when TTP is downregulated in the TME are shown in Figure 4.
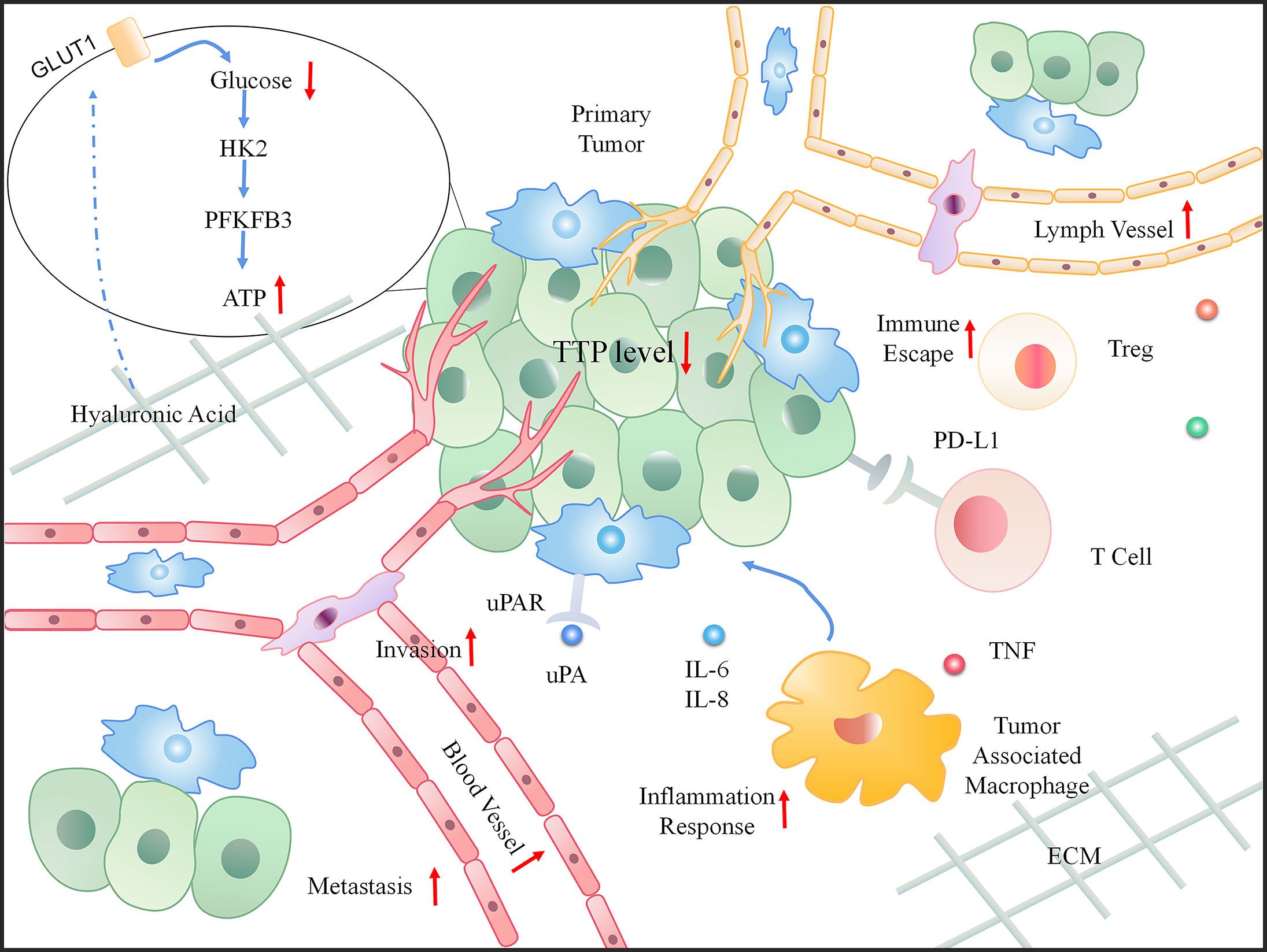
Figure 4 The dynamic change in the tumor microenvironment (TME) when TTP is downregulated. When TTP expression decreases in tumor cells, the primary tumor invades the basement membrane. Moreover, an increased number of blood and lymph vessels aids tumor metastasis. In the microenvironment, the increasing inflammatory response, immune escape, and energy also provide a favorable environment for tumor progression.
TTP Inhibits Angiogenesis and Lymphangiogenesis
Angiogenesis is the sprouting of new vessels from existing ones during embryogenesis. In the adult, angiogenesis is usually turned on, but only transiently. In contrast, during tumor progression, an ‘angiogenic switch’ is almost always activated and remains on, causing normally quiescent vasculature to continually sprout new vessels, which help sustain expanding neoplastic growths (25). TTP suppresses the metastasis through angiogenesis. TTP has been shown to inhibit the expression of vascular endothelial growth factor (VEGF) and COX-2, which are both key factors that influence angiogenesis in human colorectal cancer (34). Furthermore, galanin receptor type 2 (GALR2) and G-protein-coupled receptor (GPCR) induce the aggressive growth of SCCHN and stimulate angiogenesis through p38 MAPK-mediated phosphorylation of TTP. This allows for a decrease in TTP expression and an increase in the secretion of pro-angiogenic cytokines such as VEGF and IL-6 (70). In addition, resveratrol, a polyphenolic compound that naturally occurs in grapes, peanuts, and berries, induces the mRNA-decaying activity of TTP and suppresses the proliferation, invasion and migration of HCT116 and SNU81 colon cancer cells by activating TTP (45).
Similar to angiogenesis, lymphangiogenesis is the proliferation of a network of lymphatic vessels. As mentioned previously, GALR2 overexpression in SCCHN cells could suppress TTP phosphorylation and increase the secretion of IL-6 and VEGF, thereby promoting angiogenesis (34). However, antiangiogenic effects may be undermined by lymphatic vessel formation (71). The lymphatic system is one of the main routes of distant metastasis and growth of cancer cells (72), among which vascular endothelial growth factor C (VEGF-C) is currently regarded as the most representative lymphangiogenic factor (73). Simultaneously, sunitinib inactivates TTP, prolongs the half-life of VEGF-C, and hastens the progression of lymphatic vessels in clear cell renal cell carcinoma (74).
TTP Overexpression Prevents the Inflammation in Cancer
Inflammation, a key characteristic of the TME, can play a crucial role in the stages of tumor development, such as initiation, promotion, and metastasis (75). Elevated levels of pro-inflammatory genes, associated with continuous inflammation and tumorigenesis, advance the proliferation, angiogenesis, metastasis, survival, and drug resistance of cancer cells (76, 77). The secretion of IL-6 and -8 further enhances the inflammatory response and induces the production of additional cytokines, thus facilitating the inflammation-cancer cycle (78). TTP can negatively regulate many inflammatory and oncogenic cytokines (3). Therefore, inhibiting inflammation through TTP may help prevent the occurrence and progression of cancers.
NF-κB and STAT3, activators of transcription, collaboratively link inflammation to cancer (79). Compared with normal cells, a sustained inflammatory response has been attributed to negative regulation of the NF-κB-STAT3 pathway in tumors (79). TTP and SOCS3 can affect NF-κB and STAT3, respectively, and inhibit cancerous inflammation in prostate cancer (80). IL-6, which is overabundant in the inflammatory response, has been associated with lethal prostate cancer accompanied by low TTP levels (23). Calcineurin signals stabilize the level of TTP protein by degrading proteasomes, thereby downregulating skin inflammation and inhibiting keratinocyte tumors (81).
P38 MAPK is a key factor that drives TNF-α expression in tumor-associated macrophages at the post-transcriptional level, which is achieved by reducing TTP expression (82). The TNF-α-TTP pathway is controlled by two key factors, p38α MAPK and IL-10 (83). TTP expression is regulated by IL-10 through STAT3, but p38α has two different regulatory functions with TTP in the TME (84), the first being post-transcriptional mRNA decay through TTP phosphorylation, and the second is blocking mRNA translation. Following induction by Toll-like receptor (TLR), DUSP dephosphorylates p38α, which promotes TTP expression and then degrades TNF mRNA transcripts. IL-10 also inhibits TNF expression by restoring TTP expression after TLR induction (82). Collectively, these findings suggest that increasing TTP expression could be an approach for controlling inflammation in the TME.
TTP Plays an Important Role in Immune Surveillance in the TME
Changes in the status of the immune system in situ and activating the metastasis in the TME can both lead to the escape of tumor cells from local and systemic immune control (85). Various immunosuppressive cells and factors in the TME enable cancer cells to evade immune surveillance, except for the cells involved in tumor antigen presentation (86). TME status, including the presence of tumor-infiltrating immune cells, is a decisive factor for cell survival and tumor development (87). The maintenance of immune homoeostasis is mediated by various signaling molecules and involves complex mechanisms (88).
The immunosuppressive protein programmed death ligand 1 (PD-L1) and regulatory T cells (Tregs) play a key role in maintaining peripheral immune tolerance (89). PD-L1 is upregulated in many cancers and helps evade the host immune system (90). Downstream of RAS, MEK promotes kinase MK2-dependent TTP phosphorylation (91). Inactivation of oncogenic RAS-MEK signaling leads to reduction in TTP phosphorylation, stabilizes PD-L1 mRNA, and increases protein abundance. Thus, restoration of TTP expression enhances anti-tumor immunity through the degradation of PD-L1 mRNA (91). PD-L1 plays a crucial role in the infiltration and development of Tregs, a subset of T lymphocytes (92). TTP has a negative effect on Treg infiltration and enhances CD8+ T cell cytotoxicity. TTP inhibits Tregs infiltration in gastric cancer by reducing PD-L1 expression, which increases Treg-mediated effector cell cytotoxicity and promotes anti-tumor immunity through CD8+ T cells (92).
Doxorubicin can reduce PD-L1 expression in cancer cells (93). The underlying molecular mechanism involves anti-tumor immunity, which is achieved by increasing TTP expression and subsequently downregulating PD-L1 expression. Both PD-1 and PD-L1 levels are increased in patients with KRAS-mutant lung cancer (94). In this type of cancer, immune escape is mediated by phosphorylating ERK or by increasing the stability of PD-L1 mRNA (95). The phosphorylation of TTP downstream of ERK causes stabilization of PD-L1 mRNA (93). The relevance of TTP and PD-L1 in the anti-tumor immune response is supported by current evidence (91–94), which demonstrates that TTP could be a novel biomarker in cancer immunotherapy (Figure 5).
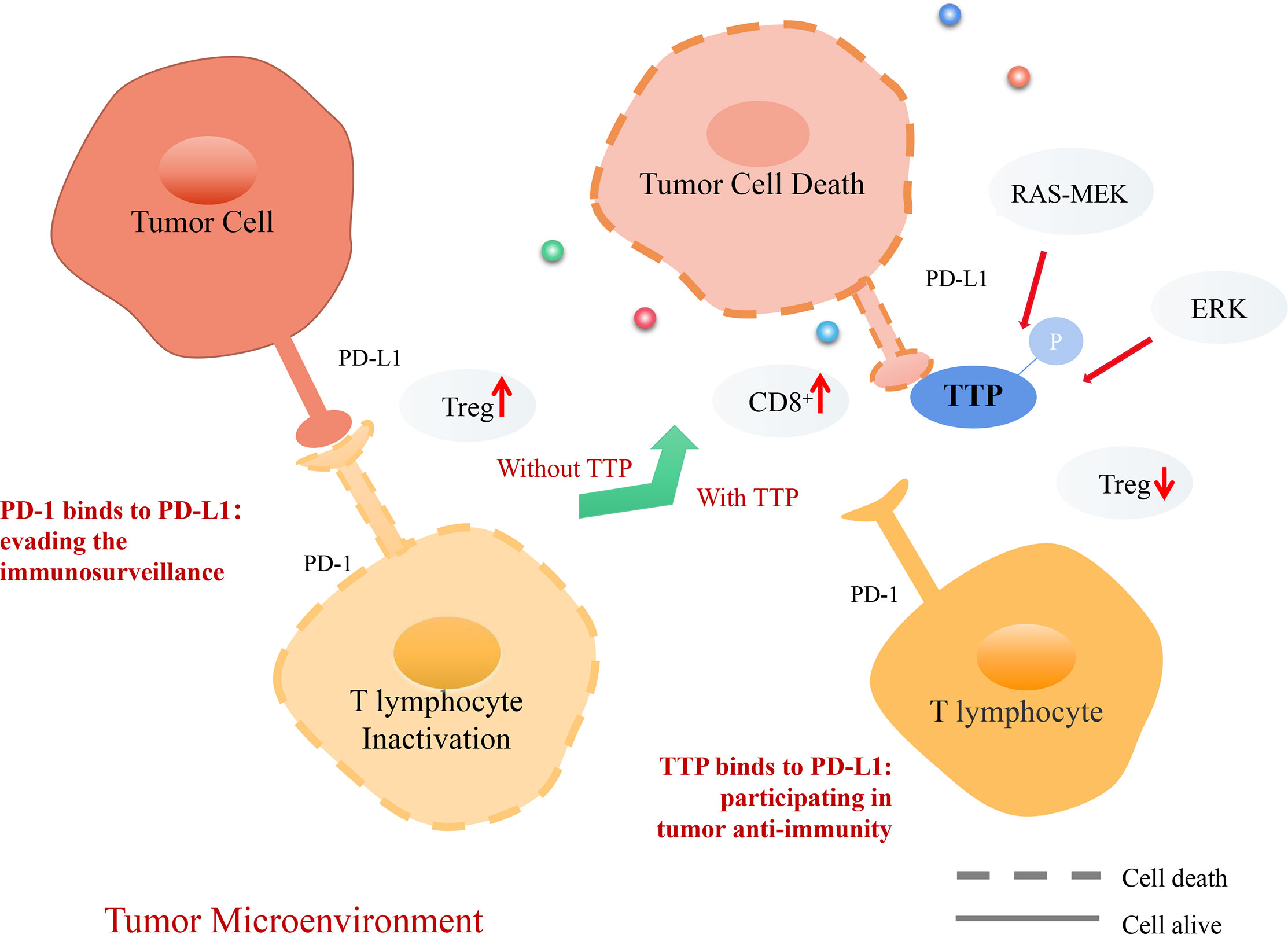
Figure 5 Mechanism of TTP-dependent anti-tumor immunity in the tumor microenvironment (TME). PD-1, a receptor expressed on T lymphocytes, interacts with its ligand PD-L1 on target cells, recognizing healthy cells and preventing induced cell death. However, tumor cells can express PD-L1 and interact with PD-1 on T lymphocytes. T lymphocytes infiltrate Treg cells, evading the tumor immunosurveillance to allow distant metastasis. Consequently, TTP recognizes tumor cells, destabilizes PD-L1 mRNA, and decreases its expression. Thus, T lymphocytes can recognize tumor cells and secrete CD8+ cells to promote tumor cell death.
TTP Suppresses the Cancer Metabolism
Mammalian cells produce ATP through mitochondrial (oxidative phosphorylation) and non-mitochondrial (glycolysis) metabolism, which provides energy for the cell (96). Cancer cells reprogram nutrient- and oxygen-poor microenvironments to meet their energy and anabolism needs (97). One of the hallmarks of cancer cells is that they enhance the uptake and utilization of glucose, known as the Warburg effect. Cancer cells tend to metabolize glucose through glycolysis, which involves glucose uptake and the subsequent production of glucose-6-phosphate, pyruvate, and lactic acid to support cell proliferation and anabolism (98).
Negative Report About TTP Suppressing the Cancer Metabolism
ECM remodeling, during which hyaluronan-mediated motility receptors are closely related to glycolysis, may be considered a basic step in the regulation of extracellular metabolism (99). Hyaluronidase can stimulate the expression of TTP downstream through a receptor tyrosine kinase (RTK)-dependent pathway (100). Then, TTP can mediate the transcription decay of thioredoxin interacting protein (TXNIP) and increase the intake of glucose transporter 1 (GLUT1). At the same time, RTK induces TTP and causes the rapid degradation of TXNIP. This sharp decline in TXNIP induces GLUT1 accumulation on the plasma membrane, thereby inducing the uptake and utilization of glucose (101). TTP-TXNIP-GLUT1 signaling promotes glycolysis by reducing hyaluronan, implying that it is necessary for cancer cells to accelerate migration through ECM decomposition (102).
Positive Report About TTP Suppressing the Cancer Metabolism
TTP upregulation decreases the expression of hexokinase 2 (HK2), the first catalytic enzyme of glycolysis. However, when HK2 expression is enhanced in cancer cells, glycolysis and mitochondrial energy production are both reduced. By disrupting the stability of HK2 mRNA, TTP alters the extracellular acidification rate, oxygen consumption rate, and ATP levels of cancer cells, suggesting that TTP is a negative regulator of HK2 expression and glucose metabolism (103). The first rate-limiting enzyme of glycolysis, 6-phosphofructo-2-kinase (PFKFB3), is overexpressed in cancer cells. TTP destabilizes PFKFB mRNA transcripts by binding to the ARE in the 3’ UTR. By downregulating PFKFB3 expression, TTP inhibits energy production and glycolytic flux in cancer cells, increases glutathione expression, and controls the balance between glycolysis and the pentose phosphate pathway at the post-transcriptional level (104).
Notably, TTP promotes glycolysis by increasing the uptake and utilization of glucose in the ECM, whereas in the tricarboxylic acid cycle, TTP inhibits the expression of major enzymes that impede glycolysis in cancer cells. In addition, TTP is also regarded as the promoter of cell metabolism, meaning that TTP provides energy to tumor cells. However, controversy exists regarding the role of TTP in tumor metabolism because some studies claim that TTP may destabilize the mRNA of key enzymes in glycolysis (99, 103). As a result, TTP might reduce the supply of energy to tumor cells. Collectively, these findings suggest that the role of TTP in cancer cell metabolism remains elusive and further research is needed to gain clarity.
Prospects
The core functions of TTP anti-tumor in the TME include the regulation of inflammation and immunity. As an inflammation-related protein, TTP plays a significant role in cancer onset and progression by modulating the TME, which suggests TTP’s potential mechanism in the transformation from inflammation to tumorigenesis. Moreover, TTP can be regarded as a safeguard against carcinoma due to its role in the TME. Surprisingly, the mechanism is involved in PD-1/PD-L1, the discovery of which won the Nobel Prize in 2018. PD-L1 mRNA degraded by TTP at the post-transcriptional level has the potential in reducing tumor immune evasion. However, a better understanding of the relationship between TTP and TME is required, such as which role TTP plays in regulating metabolism of the TME in the ECM.
In summary, TTP could be a clinical biomarker for cancer diagnosis. Furthermore, upregulating TTP expression may provide a novel approach to improve the prognosis of cancer patients. In 2015, President Obama in U.S.A. has announced the precision medicine project, aiming to provide personalized treatment for individual. With the development of bioinformatics and computer biology, high-throughput sequencing has offered the opportunity to deeply understand many biomarkers including TTP. For example, there have three subtypes of TTP/ZFP36 have been reported, ZFP36*2 (A>G), ZFP36*8 (C>T) and ZFP36*10 (2bp deletion). ZFP36*2 has been proved to be a potential biomarker in Caucasian breast cancer patients while ZFP36*8 has been found high associated with HER2-positive-breast cancer. The availability of accumulated TTP subtype data from patients offers vital and invaluable resources to identify precision treatment for multiple cancer. Further in the gender difference, high TTP level has appeared better overall survival in males than females (105, 106). All above have approved that in the future, a safe and effective way to improve overall survival of tumor patients may be achieved based on the TTP target treatment.
Author Contributions
JT, DZ and SZ designed and wrote the manuscript. DZ and RY drew the figures. BZ, YT, and LC revised and edited the manuscript. JT, ZZ and TL supervised and checked the review. All authors contributed to the article and approved the submitted version.
Funding
This work was supported by National Natural Science Foundation of China (82060662), National Innovative projects for University students (g201910555146, g201910555026, 202010601018), Natural Science Foundation of Hunan Province (2020JJ4081), the Foundation for Guangxi Key Laboratory of Diabetic Systems Medicine (20-065-77), Hunan Provincial Key Laboratory of Tumor Microenvironment Responsive Drug Research (2019-56), Hunan Provincial Cooperative Innovation Center for Molecular Target New Drug Study (2016-429), and Shanghai Talent Development Fund (2017116).
Conflict of Interest
The reviewer ZM declared a shared affiliation with several of the authors, ZZ, LC, JT and the reviewer CKT declared a shared affiliation with several of the authors, DZ, RY, SZ, BZ, YT to the handling editor at time of review.
Acknowledgments
Editage (www.editage.cn) has been acknowledged for English language editing.
Abbreviations
TTP, tristetraprolin; ARE, AU-rich element; TZF, tandem zinc-finger; TNF, tumor necrosis factor; ACC, Adrenocortical carcinoma; BLCA, Bladder urothelial carcinoma; BRCA, Breast invasive carcinoma; CESC, Cervical squamous cell carcinoma; COAD, Colon adenocarcinoma; NF-κB, nuclear factor kappa-B; HDAC, histone deacetylases; Bcl-2, B-cell lymphoma 2; SCCHN, squamous cell carcinoma of the head and neck; HMGB1, enhancement of high mobility group box 1; VEGF, vascular endothelial growth factor; gAcrp, globular adiponectin; HCC, hepatocellular carcinoma; RIP1, receptor-interacting protein kinase 1; FADD, Fas-associating protein with a novel death domain; IAP, inhibitor of apoptosis; GALR2, galanin receptor type 2; GPCR, G-protein-coupled receptor; NaBu, sodium butyrate; TME, tumor microenvironment; STAT3, signal transducer and activator of transcription 3; TLR, Toll-like receptor; PD-L1, programmed death ligand 1; Treg, regulatory T cell; ECM, extracellular matrix; TXNIP, thioredoxin interacting protein; GLUT-1, glucose transporter 1; HK2, of hexokinase 2; PFKFB3, 6-phosphofructo-2-kinase; COX-2, cyclooxygenase-2; RTK, receptor tyrosine kinase.
References
1. Stoecklin G, Gross B, Ming XF, Moroni C. A Novel Mechanism of Tumor Suppression by Destabilizing AU-rich Growth Factor Mrna. Oncogene (2003) 22:3554–61. doi: 10.1038/sj.onc.1206418
2. Shukla S, Elson G, Blackshear PJ, Lutz CS, Leibovich SJ. 3’utr AU-Rich Elements (Ares) and the RNA-Binding Protein Tristetraprolin (Ttp) Are Not Required for the LPS-Mediated Destabilization of Phospholipase-Cβ-2 mRNA in Murine Macrophages. Inflammation (2017) 40:645–56. doi: 10.1007/s10753-017-0511-y
3. Guo J, Wang H, Jiang S, Xia J, Jin S. The Cross-talk Between Tristetraprolin and Cytokines in Cancer. Anticer Agents Med Chem (2017) 17:1477–86. doi: 10.2174/1871520617666170327155124
4. Patial S, Blackshear PJ. Tristetraprolin as a Therapeutic Target in Inflammatory Disease. Trends Pharmacol Sci (2016) 37:811–21. doi: 10.1016/j.tips.2016.07.002
5. Lai WS, Wells ML, Perera L, Blackshear PJ. The Tandem Zinc Finger RNA Binding Domain of Members of the Tristetraprolin Protein Family. Wiley Interdiscip Rev RNA (2019) 10:e1531. doi: 10.1002/wrna.1531
6. Ostareck DH, Ostareck-Lederer A. Rna-Binding Proteins in the Control of LPS-Induced Macrophage Response. Front Genet (2019) 10:31. doi: 10.3389/fgene.2019.00031
7. Lee S, Micalizzi D, Truesdell SS, Bukhari SIA, Boukhali M, Lombardi-Story J, et al. A Post-Transcriptional Program of Chemoresistance by AU-rich Elements and TTP in Quiescent Cells Leukemic Cells. Genome Biol (2020) 21:33. doi: 10.1186/s13059-020-1936-4
8. Wells ML, Perera L, Blackshear PJ. An Ancient Family of RNA-Binding Proteins: Still Important! Trends Biochem Sci (2017) 42:285–96. doi: 10.1016/j.tibs.2016.12.003
9. Goldstrohm AC, Wickens M. Multifunctional Deadenylase Complexes Diversify mRNA Control. Nat Rev Mol Cell Biol (2008) 4:337–44. doi: 10.1038/nrm2370
10. Cougot N, Babajko S, Séraphin B. Cytoplasmic Foci are Sites of mRNA Decay in Human Cells. J Cell Biol (2004) 165:31–40. doi: 10.1083/jcb.200309008
11. Eulalio A, Behm-Ansmant I, Izaurralde E. P Bodies: At the Crossroads of Post-Transcriptional Pathways. Nat Rev Mol Cell Biol (2007) 8:9–22. doi: 10.1038/nrm2080
12. Gruber AR, Fallmann J, Kratochvill F, Kovarik P, Hofacker IL. Aresite: A Database for the Comprehensive Investigation of AU-rich Elements. Nucleic Acids Res (2011) 39:D66–9. doi: 10.1093/nar/gkq990
13. Lai WS, Stumpo DJ, Wells ML, Gruzdev A, Hicks SN, Nicholson CO, et al. Importance of the Conserved Carboxyl-Terminal CNOT1 Binding Domain to Tristetraprolin Activity In Vivo. Mol Cell Biol (2019) 39:e00029–19. doi: 10.1128/MCB.00029-19
14. Wang LJ, Lee YC, Huang CH, Shi YJ, Chen YJ, Pei SN, et al. Non-Mitotic Effect of Albendazole Triggers Apoptosis of Human Leukemia Cells Via the SIRT3/ROS/p38 MAPK/TTP Axis Mediated TNF-α Upregulation. Biochem Pharmacol (2019) 162:154–68. doi: 10.1016/j.bcp.2018.11.003
15. Silva-Cardoso SC, Bekker CPJ, Boes M, Radstake TRDJ, Angiolilli C. CXCL4 is a Driver of Cytokine mRNA Stability in Monocyte-Derived Dendritic Cells. Mol Immunol (2019) 114:524–34. doi: 10.1016/j.molimm.2019.09.004
16. Wang LJ, Chiou JT, Lee YC, Huang CH, Shi YJ, Chang LS. Sirt3, PP2A,and Ttpproteins Stability in the Presence of TNF-α on Vincristine-Induced Apoptosis Ofleukemiacells. J Cell Mol Med (2020) 24:2552–65. doi: 10.1111/jcmm.14949
17. Tang Z, Li C, Kang B, Gao G, Li C, Zhang Z. GEPIA: a web server for cancer and normal gene expression profiling and interactive analyses. Nucleic Acids Res (2017) 45:W98–102. doi: 10.1093/nar/gkx247
18. Guo J, Qu H, Chen Y, Xia J. The Role of RNA-binding Protein Tristetraprolin in Cancer and Immunity. Med Oncol (2017) 34:196. doi: 10.1007/s12032-017-1055-6
19. Park JM, Lee TH, Kang TH. Roles of Tristetraprolin in Tumorigenesis. Int J Mol Sci (2018) 19:3384. doi: 10.3390/ijms19113384
20. Zeng B, Zhu D, Su Z, Li Z, Yu Z. Tristetraprolin Exerts Tumor Suppressive Functions on the Tumorigenesis of Glioma by Targeting IL-13. Int Immunopharmacol (2016) 39:63–70. doi: 10.1016/j.intimp.2016.07.001
21. Lam SS, Ip CK, Mak AS, Wong AS. Novel P70 S6 kinase-microRNA Biogenesis Axis Mediates Multicellular Spheroid Formation in Ovarian Cancer Progression. Oncotarget (2016) 7:38064–77. doi: 10.18632/oncotarget.9345
22. Deng KY, Wang H, Shan T, Chen YG, Zhou H, Zhao Q, et al. Tristetraprolin Inhibits Gastric Cancer Progression Through Suppression of IL-33. Sci Rep (2016) 6:24505. doi: 10.1038/srep24505
23. Gerke T, Beltran H, Wang X, Lee GM, Sboner A, Karnes RJ, et al. Low Tristetraprolin Expression Is Associated With Lethal Prostate Cancer. Cancer Epidemiol Biomarkers Prev (2019) 28:584–90. doi: 10.1158/1055-9965.EPI-18-0667
24. Dolicka D, Sobolewski C, Gjorgjieva M, Correia de Sousa M, Berthou F, De Vito C, et al. Tristetraprolin Promotes Hepatic Inflammation and Tumor Initiation But Restrains Cancer Progression to Malignancy. Cell Mol Gastroenterol Hepatol (2021) 11:597–621. doi: 10.1016/j.jcmgh.2020.09.012
25. Hanahan D, Weinberg RA. Hallmarks of Cancer: The Next Generation. Cell (2011) 144:646–74. doi: 10.1016/j.cell.2011.02.013
26. Xu L, Ning H, Gu L, Wang Q, Lu W, Peng H, et al. Tristetraprolin Induces Cell Cycle Arrest in Breast Tumor Cells Through Targeting AP-1/c-Jun and NF-κb Pathway. Oncotarget (2015) 6:41679–91. doi: 10.18632/oncotarget.6149
27. Dong F, Li C, Wang P, Deng X, Luo Q, Tang X, et al. The RNA Binding Protein Tristetraprolin Down-Regulates Autophagy in Lung Adenocarcinoma Cells. Exp Cell Res (2018) 367:89–96. doi: 10.1016/j.yexcr.2018.03.028
28. Zheng XT, Xiao XQ. Sodium Butyrate Down-Regulates Tristetraprolin-Mediated Cyclin B1 Expression Independent of the Formation of Processing Bodies. Int J Biochem Cell Biol (2015) 69:241–8. doi: 10.1016/j.biocel.2015.11.002
29. Schichl YM, Resch U, Hofer-Warbinek R, de Martin R. Tristetraprolin Impairs NF-kappaB/p65 Nuclear Translocation. J Biol Chem (2009) 284:29571–81. doi: 10.1074/jbc.M109.031237
30. Lai WS, Stumpo DJ, Qiu L, Faccio R, Blackshear PJ. A Knock-In Tristetraprolin (Ttp) Zinc Finger Point Mutation in Mice: Comparison With Complete Ttp Deficiency. Mol Cell Biol (2018) 38:e00488–17. doi: 10.1128/MCB.00488-17
31. Suk FM, Chang CC, Lin RJ, Lin SY, Liu SC, Jau CF, et al. ZFP36L1 and ZFP36L2 Inhibit Cell Proliferation in a Cyclin D-dependent and p53-independent Manner. Sci Rep (2018) 8:2742. doi: 10.1038/s41598-018-21160-z
32. Kang S, Min A, Im SA, Song SH, Kim SG, Kim HA, et al. Tgf-β Suppresses COX-2 Expression by Tristetraprolin-Mediated RNA Destabilization in A549 Human Lung Cancer Cells. Cancer Res Treat (2015) 47:101–9. doi: 10.4143/crt.2013.192
33. Dong HW, Wang K, Chang XX, Jin FF, Wang Q, Jiang XF, et al. Beta-Ionone-Inhibited Proliferation of Breast Cancer Cells by Inhibited COX-2 Activity. Arch Toxicol (2019) 93:2993–3003. doi: 10.1007/s00204-019-02550-2
34. Sobolewski C, Sanduja S, Blanco FF, Hu L, Dixon DA. Histone Deacetylase Inhibitors Activate Tristetraprolin Expression Through Induction of Early Growth Response Protein 1 (EGR1) in Colorectal Cancer Cells. Biomolecules (2015) 5:2035–55. doi: 10.3390/biom5032035
35. Young LE, Sanduja S, Bemis-Standoli K, Pena EA, Price RL, Dixon DA. The mRNA Binding Proteins HuR and Tristetraprolin Regulate Cyclooxygenase 2 Expression During Colon Carcinogenesis. Gastroenterology (2009) 136:1669–79. doi: 10.1053/j.gastro.2009.01.010
36. Resetkova E, Gonzalez-Angulo AM, Sneige N, Mcdonnell TJ, Buzdar AU, Kau SW, et al. Prognostic Value of P53, Mdm-2, and MUC-1 in Patients With Inflammatory Breast Carcinoma Cancer. Cancer (2004) 101:913–7. doi: 10.1002/cncr.20465
37. Pandiri I, Chen Y, Joe Y, Kim HJ, Park J, Chung HT, et al. Tristetraprolin Mediates the Anti-Proliferative Effects of Metformin in Breast Cancer Cells. Breast Cancer Res Treat (2016) 156:57–64. doi: 10.1007/s10549-016-3742-y
38. Liu HN, Qie P, Yang G, Song YB. miR-181b Inhibits Chemoresistance in Cisplatin-Resistant H446 Small Cell Lung Cancer Cells by Targeting Bcl-2. Arch Med Sci (2018) 14:745–51. doi: 10.5114/aoms.2018.73131
39. Park SB, Lee JH, Jeong WW, Kim YH, Cha HJ, Joe Y, et al. TTP Mediates Cisplatin-Induced Apoptosis of Head and Neck Cancer Cells by Downregulating the Expression of Bcl-2. J Chemother (2015) 27:174–80. doi: 10.1179/1973947814Y.0000000234
40. Pun TN, Khakurel A, Shrestha A, Kim SH, Park PH. Critical Role of Tristetraprolin and AU-rich Element RNA-binding Protein 1 in the Suppression of Cancer Cell Growth by Globular Adiponectin. FEBS Open Bio (2018) 8:1964–76. doi: 10.1002/2211-5463.12541
41. Weng D, Marty-Roix R, Ganesan S, Proulx MK, Vladimer GI, Kaiser WJ, et al. Caspase-8 and RIP Kinases Regulate Bacteria-Induced Innate Immune Responses and Cell Death. Proc Natl Acad Sci USA (2014) 111:7391–6. doi: 10.1073/pnas.1403477111
42. Bellail AC, Olson JJ, Yang X, Chen ZJ, Hao C. A20 Ubiquitin Ligase-Mediated Polyubiquitination of RIP1 Inhibits Caspase-8 Cleavage and TRAIL-induced Apoptosis Glioblastoma. Cancer Discovery (2012) 2:140–55. doi: 10.1158/2159-8290.CD-11-0172
43. Chen G, Cheng X, Zhao M, Lin S, Lu J, Kang J, et al. RIP1-Dependent Bid Cleavage Mediates Tnfα-Induced But Caspase-3-independent Cell Death in L929 Fibroblastoma Cells. Apoptosis (2015) 20:92–109. doi: 10.1007/s10495-014-1058-0
44. Lalaoui N, Vaux DL. Recent Advances in Understanding Inhibitor of Apoptosis Proteins. F1000Res (2018) 7:F1000 Faculty Rev–1889. doi: 10.12688/f1000research.16439.1
45. Lee SR, Jin H, Kim WT, Kim WJ, Kim SZ, Leem SH, et al. Tristetraprolin Activation by Resveratrol Inhibits the Proliferation and Metastasis of Colorectal Cancer Cells. Int J Oncol (2018) 53:1269–78. doi: 10.3892/ijo.2018.4453
46. Selmi T, Martello A, Vignudelli T, Ferrari E, Grande A, Gemelli C, et al. ZFP36 Expression Impairs Glioblastoma Cell Lines Viability and Invasiveness by Targeting Multiple Signal Transduction Pathways. Cell Cycle (2012) 11:1977–87. doi: 10.4161/cc.20309
47. Selmi T, Alecci C, Aquila MD, Montorsi L, Martello A, Guizzetti F, et al. ZFP36 Stabilizes RIP1 Via Degradation of XIAP and cIAP2 Thereby Promoting Ripoptosome Assembly. BMC Cancer (2015) 15:357. doi: 10.1186/s12885-015-1388-5
48. Fudhaili A, Yoon NA, Kang S, Ryu J, Jeong JY, Lee DH, et al. Resveratrol Epigenetically Regulates the Expression of Zinc Finger Protein 36 in non-Small Cell Lung Cancer Cell Lines. Oncol Rep (2019) 41:1377–86. doi: 10.3892/or.2018.6898
49. Tran DDH, Koch A, Allister A, Saran S, Ewald F, Koch M, et al. Treatment With MAPKAP2 (MK2) Inhibitor and the DNA Methylation Inhibitor 5-Aza Dc Synergistically Triggers Apoptosis in Hepatocellular Carcinoma (HCC) Via Tristetraprolin (TTP). Cell Signal (2016) 28:1872–80. doi: 10.1016/j.cellsig.2016.09.002
50. Chawla-Sarkar M, Lindner DJ, Liu YF, Williams BR, Sen GC, Silverman RH, et al. Apoptosis and Interferons: Role of Interferon-Stimulated Genes as Mediators of Apoptosis. Apoptosis (2003) 8:237–49. doi: 10.1023/a:1023668705040
51. Peeters CF, Waal RM, Wobbes T, Westphal JR, Ruers TJ. Outgrowth of Human Liver Metastases After Resection of the Primary Colorectal Tumor: A Shift in the Balance Between Apoptosis and Proliferation. Int J Cancer (2006) 119:1249–53. doi: 10.1002/ijc.21928
52. Gondi CS, Lakka SS, Yanamandra N, Siddique K, Dinh DH, Olivero WC, et al. Expression of Antisense uPAR and Antisense uPA From a Bicistronic Adenoviral Construct Inhibits Glioma Cell Invasion, Tumor Growth, and Angiogenesis. Oncogene (2003) 22:5967–75. doi: 10.1038/sj.onc.1206535
53. Ryu J, Yoon NA, Lee YK, Jeong JY, Kang S, Seong H, et al. Tristetraprolin Inhibits the Growth of Human Glioma Cells Through Downregulation of Urokinase Plasminogen Activator/Urokinase Plasminogen Activator Receptor Mrnas. Mol Cells (2015) 38:156–62. doi: 10.14348/molcells.2015.2259
54. Al-Souhibani N, Al-Ahmadi W, Hesketh JE, Blackshear PJ, Khabar KS. The RNA-binding Zinc-Finger Protein Tristetraprolin Regulates AU-rich mRNAs Involved in Breast Cancer-Related Processes. Oncogene (2010) 29:4205–15. doi: 10.1038/onc.2010.168
55. Tu M, Wange W, Cai L, Zhu P, Gao Z, Zheng W. Il-13 Receptor α2 Stimulates Human Glioma Cell Growth and Metastasis Through the Src/PI3K/Akt/mTOR Signaling Pathway. Tumour Biol (2016) 37:14701–9. doi: 10.1007/s13277-016-5346-x
56. Bartolomé RA, García-Palmero I, Torres S, López-Lucendo M, Balyasnikova IV, Casal JI. Il13 Receptor α2 Signaling Requires a Scaffold Protein, FAM120A, to Activate the FAK and PI3K Pathways in Colon Cancer Metastasis. Cancer Res (2015) 75:2434–44. doi: 10.1158/0008-5472.CAN-14-3650
57. Andersson P, Yang Y, Hosaka K, Zhang Y, Fischer C, Braun H, et al. Molecular Mechanisms of IL-33-mediated Stromal Interactions in Cancer Metastasis. JCI Insight (2018) 3:e122375. doi: 10.1172/jci.insight.122375
58. Li Y, Shi J, Qi S, Zhang J, Peng D, Chen Z, et al. Il-33 Facilitates Proliferation of Colorectal Cancer Dependent on COX2/PGE (2). J Exp Clin Cancer Res (2018) 37:196. doi: 10.1186/s13046-018-0839-7
59. Yang T, Ren C, Lu C, Qiao P, Han X, Wang L, et al. Phosphorylation of HSF1 by PIM2 Induces PD-L1 Expression and Promotes Tumor Growth in Breast Cancer. Cancer Res (2019) 79:5233–44. doi: 10.1158/0008-5472.CAN-19-0063
60. Ren C, Yang TT, Qiao PY, Wang L, Han X, Lv S, et al. PIM2 Interacts With Tristetraprolin and Promotes Breast Cancer Tumorigenesis. Mol Oncol (2018) 12:690–704. doi: 10.1002/1878-0261.12192
61. Mukherjee N, Jacobs NC, Hafner M, Kennington EA, Nusbaum JD, Tuschl T, et al. Global Target mRNA Specification and Regulation by the RNA-binding Protein ZFP36. Genome Biol (2014) 15:R12. doi: 10.1186/gb-2014-15-1-r12
62. Wang H, Ding N, Guo J, Xia J, Ruan Y. Dysregulation of TTP and HuR Plays an Important Role in Cancers. Tumour Biol (2016) 37:14451–61. doi: 10.1007/s13277-016-5397-z
63. Al-Ahmadi W, Al-Ghamdi M, Al-Souhibani N, Khabar KS. miR-29a Inhibition Normalizes HuR Over-Expression and Aberrant AU-rich mRNA Stability in Invasive Cancer. J Pathol (2013) 230:28–38. doi: 10.1002/path.4178
64. Tao H, Tang T, Wang S, Wang Z, Ma Y, Cai T, et al. The Molecular Mechanisms of Aloin Induced Gastric Cancer Cell Apoptosis by Targeting the High Mobility Group Box 1. Drug Des Devel Ther (2019) 13:1221–31. doi: 10.2147/DDDT.S201818
65. Wang H, Chen Y, Guo J, Shan T, Deng K, Chen J, et al. Dysregulation of Tristetraprolin and Human Antigen R Promotes Gastric Cancer Progressions Partly by Upregulation of the High-Mobility Group Box 1. Sci Rep (2018) 8:7080. doi: 10.1038/s41598-018-25443-3
66. Qian L, Ji AH, Zhang WJ, Zhao N. Hur, TTP, and miR-133b Expression in NSCLC and Their Association With Prognosis. Eur Rev Med Pharmacol Sci (2018) 22:430–42. doi: 10.26355/eurrev_201801_14192
68. Wang M, Zhao J, Zhang L, Wei F, Lian Y, Wu Y, et al. Role of Tumor Microenvironment in Tumorigenesis. J Cancer (2017) 8:761–73. doi: 10.7150/jca.17648
69. Wu T, Dai Y. Tumor Microenvironment and Therapeutic Response. Cancer Lett (2017) 387:61–8. doi: 10.1016/j.let.2016.01.043
70. Banerjee R, Van Tubergen EA, Scanlon CS, Vander Broek R, Lints JP, Liu M, et al. The G the Protein-Coupled Receptor GALR2 Promotes Angiogenesis in Head and Neck Cancer. Mol Cancer Ther (2014) 13:1323–33. doi: 10.1158/1535-7163.MCT-13-0904
71. Goel S, Duda DG, Xu L, Munn LL, Boucher Y, Fukumura D, et al. Normalization of the Vasculature for Treatment of Cancer and Other Diseases. Physiol Rev (2011) 91:1071–121. doi: 10.1152/physrev.00038.2010
72. Tian Z, Wei B, Tang F, Wei W, Gilcrease MZ, Huo L, et al. Prognostic Significance of Tumor Grading and Staging in Mammary Carcinomas With Neuroendocrine Differentiation. Hum Pathol (2011) 42:1169–77. doi: 10.1016/j.humpath.2010.11.014
73. Nurmi H, Saharinen P, Zarkada G, Zheng W, Robciuc MR, Alitalo K. VEGF-C is Required for Intestinal Lymphatic Vessel Maintenance and Lipid Absorption. EMBO Mol Med (2015) 7:1418–25. doi: 10.15252/emmm.201505731
74. Dufies M, Giuliano S, Ambrosetti D, Claren A, Ndiaye PD, Mastri M, et al. Sunitinib Stimulates Expression of VEGFC by Tumor Cells and Promotes Lymphangiogenesis in Clear Cell Renal Cell Carcinomas. Cancer Res (2017) 77:1212–26. doi: 10.1158/0008-5472.CAN-16-3088
75. Comen EA, Bowman RL, Kleppe M. Underlying Causes and Therapeutic Targeting of the Inflammatory Tumor Microenvironment. Front Cell Dev Biol (2018) 6:56. doi: 10.3389/fcell.2018.00056
76. Diakos CI, Charles KA, McMillan DC, Clarke SJ. Cancer-Related Inflammation and Treatment Effectiveness. Lancet Oncol (2014) 15:e493–503. doi: 10.1016/S1470-2045(14)70263-3
77. Bottazzi B, Riboli E, Mantovani A. Aging, Inflammation and Cancer. Semin Immunol (2018) 40:74–82. doi: 10.1016/j.smim.2018.10.011
78. Wei ZR, Liang C, Feng D, Cheng YJ, Wang WM, Yang DJ, et al. Low Tristetraprolin Expression Promotes Cell Proliferation and Predicts Poor Patients Outcome in Pancreatic Cancer. Oncotarget (2016) 7:17737–50. doi: 10.18632/oncotarget.7397
79. Fan Y, Mao R, Yang J. Nf-κb and STAT3 Signaling Pathways Collaboratively Link Inflammation to Cancer. Protein Cell (2013) 4:176–85. doi: 10.1007/s13238-013-2084-3
80. Zhu JG, Yuan DB, Chen WH, Han ZD, Liang YX, Chen G, et al. Prognostic Value of ZFP36 and SOCS3 Expressions in Human Prostate Cancer. Clin Transl Oncol (2016) 18:782–91. doi: 10.1007/s12094-015-1432-6
81. Wu X, Tommasi di Vignano A, Zhou Q, Michel-Dziunycz PJ, Bai F, Mi J, et al. The ARE-binding Protein Tristetraprolin (TTP) is a Novel Target and Mediator of Calcineurin Tumor Suppressing Function in the Skin. PloS Genet (2018) 14:e1007366. doi: 10.1371/journal.pgen.1007366
82. Kratochvill F, Gratz N, Qualls JE, Van De Velde LA, Chi H, Kovarik P, et al. Tristetraprolin Limits Inflammatory Cytokine Production in Tumor-Associated Macrophages in an Mrna Decay-Independent Manner. Cancer Res (2015) 75:3054–64. doi: 10.1158/0008-5472.CAN-15-0205
83. Ehlting C, Trilling M, Tiedje C, Le-Trilling VTK, Albrecht U, Kluge S, et al. MAPKAP Kinase 2 Regulates IL-10 Expression and Prevents Formation of Intrahepatic Myeloid Cell Aggregates During Cytomegalovirus Infections. J Hepatol (2016) 64):380–9. doi: 10.1016/j.jhep.2015.08.012
84. Gaba A, Grivennikov SI, Do MV, Stumpo DJ, Blackshear PJ, Karin M. Cutting Edge: IL-10-mediated Tristetraprolin Induction is Part of a Feedback Loop That Controls Macrophage STAT3 Activation and Cytokine Production. J Immunol (2012) 189):2089–93. doi: 10.4049/jimmunol.1201126
85. Binnewies M, Roberts EW, Kersten K, Chan V, Fearon DF, Merad M, et al. Understanding the Tumor Immune Microenvironment (TIME) for Effective Therapy. Nat Med (2018) 24:541–50. doi: 10.1038/s41591-018-0014-x
86. Fu C, Jiang A. Dendritic Cells and CD8 T Cell Immunity in Tumor Microenvironment. Front Immunol (2018) 9:3059. doi: 10.3389/fimmu.2018.03059
87. Zhang Y, Zhang Z. The History and Advances in Cancer Immunotherapy: Understanding the Characteristics of Tumor-Infiltrating Immune Cells and Their Therapeutic Implications. Cell Mol Immunol (2020) 17:807–21. doi: 10.1038/s41423-020-0488-6
88. Mulder WJM, Ochando J, Joosten LAB, Fayad ZA, Netea MG. Therapeutic Targeting of Trained Immunity. Nat Rev Drug Discovery (2019) 18:553–66. doi: 10.1038/s41573-019-0025-4
89. Gianchecchi E, Fierabracci A. Inhibitory Receptors and Pathways of Lymphocytes: The Role of PD-1 in Treg Development and Their Involvement in Autoimmunity Onset and Cancer Progression. Front Immunol (2018) 9:2374. doi: 10.3389/fimmu.2018.02374
90. Juneja VR, McGuire KA, Manguso RT, LaFleur MW, Collins N, Haining WN, et al. Pd-L1 on Tumor Cells is Sufficient for Immune Evasion in Immunogenic Tumors and Inhibits CD8 T Cell Cytotoxicity. J Exp Med (2017) 214:895–904. doi: 10.1084/jem.20160801
91. Coelho MA, de Carné Trécesson S, Rana S, Zecchin D, Moore C, Molina-Arcas M, et al. Oncogenic RAS Signaling Promotes Tumor Immunoresistance by Stabilizing Pd-L1 Mrna. Immunity (2017) 47:1083–99.e6. doi: 10.1016/j.immuni.2017.11.016
92. Guo J, Qu H, Shan T, Chen Y, Chen Y, Xia J. Tristetraprolin Overexpression in Gastric Cancer Cells Suppresses PD-L1 Expression and Inhibits Tumor Progression by Enhancing Antitumor Immunity. Mol Cells (2018) 41:653–64. doi: 10.14348/molcells.2018.0040
93. Kim DJ, Jang JH, Ham SY, Choi SH, Park SS, Jeong SY, et al. Doxorubicin Inhibits PD-L1 Expression by Enhancing TTP-mediated Decay of PD-L1 mRNA in Cancer Cells. Biochem Biophys Res Commun (2020) 522:402–7. doi: 10.1016/j.bbrc.2019.11.106
94. Calles A, Liao X, Sholl LM, Rodig SJ, Freeman GJ, Butaney M, et al. Expression of PD-1 and Its Ligands, PD-L1 and PD-L2, in Smokers and Never Smokers With KRAS-Mutant Lung Cancer. J Thorac Oncol (2015) 10:1726–35. doi: 10.1097/JTO.0000000000000687
95. Altorki NK, Markowitz GJ, Gao D, Port JL, Saxena A, Stiles B, et al. The Lung Microenvironment: An Important Regulator of Tumour Growth and Metastasis. Nat Rev Cancer (2019) 19:9–31. doi: 10.1038/s41568-018-0081-9
96. DeBerardinis RJ, Chandel NS. Fundamentals of Cancer Metabolism. Sci Adv (2016) 2:e1600200. doi: 10.1126/sciadv.1600200
97. Lyssiotis CA, Kimmelman AC. Metabolic Interactions in the Tumor Microenvironment. Trends Cell Biol (2017) 27:863–75. doi: 10.1016/j.tcb.2017.06.003
98. Pavlova NN, Thompson CB. The Emerging Hallmarks of Cancer Metabolism. Cell Metab (2016) 23:27–47. doi: 10.1016/j.cmet.2015.12.006
99. Sullivan WJ, Mullen PJ, Schmid EW, Flores A, Momcilovic M, Sharpley MS, et al. Extracellular Matrix Remodeling Regulates Glucose Metabolism Through TXNIP Destabilization. Cell (2018) 175:117–32.e21. doi: 10.1016/j.cell.2018.08.017
100. Meng X, Zhao Y, Han B, Zha C, Zhang Y, Li Z, et al. Dual Functionalized Brain-Targeting Nanoinhibitors Restrain Temozolomide-Resistant Glioma Via Attenuating EGFR and MET Signaling Pathways. Nat Commun (2020) 11:594. doi: 10.1038/s41467-019-14036-x
101. Waldhart AN, Dykstra H, Peck AS, Boguslawski EA, Madaj ZB, Wen J, et al. Phosphorylation of TXNIP by AKT Mediates Acute Influx of Glucose in Response to Insulin. Cell Rep (2017) 19:2005–13. doi: 10.1016/j.celrep.2017.05.041
102. Cao H, Urban JF Jr, Anderson RA. Cinnamon Polyphenol Extract Affects Immune Responses by Regulating Anti- and Proinflammatory and Glucose Transporter Gene Expression in Mouse Macrophages. J Nutr (2008) 138:833–40. doi: 10.1093/jn/138.5.833
103. Kim DJ, Vo MT, Choi SH, Lee JH, Jeong SY, Hong CH, et al. Tristetraprolin-Mediated Hexokinase 2 Expression Regulation Contributes to Glycolysis in Cancer Cells. Mol Biol Cell (2019) 30:542–53. doi: 10.1091/mbc.E18-09-0606
104. Jang JH, Kim DJ, Ham SY, Vo MT, Jeong SY, Choi SH, et al. Tristetraprolin Posttranscriptionally Downregulates PFKFB3 in Cancer Cells. Biochem Biophys Res Commun (2020) 521:389–94. doi: 10.1016/j.bbrc.2019.10.128
105. Upadhyay R, Sanduja S, Kaza V, Dixon DA. Genetic Polymorphisms in RNA Binding Proteins Contribute to Breast Cancer Survival. Int J Cancer (2013) 132:E128–38. doi: 10.1002/ijc.27789
106. Griseri P, Bourcier C, Hieblot C, Essafi-Benkhadir K, Chamorey E, Touriol C, et al. A Synonymous Polymorphism of the Tristetraprolin (TTP) Gene, an AU-rich mRNA-binding Protein, Affects Translation Efficiency and Response to Herceptin Treatment in Breast Cancer Patients. Hum Mol Genet (2011) 20:4556–68. doi: 10.1093/hmg/ddr390
Keywords: tristetraprolin (TTP), RNA binding protein, potential safeguard, carcinoma, tumor microenvironment (TME)
Citation: Zhang D, Zhou Z, Yang R, Zhang S, Zhang B, Tan Y, Chen L, Li T and Tu J (2021) Tristetraprolin, a Potential Safeguard Against Carcinoma: Role in the Tumor Microenvironment. Front. Oncol. 11:632189. doi: 10.3389/fonc.2021.632189
Received: 22 November 2020; Accepted: 14 April 2021;
Published: 07 May 2021.
Edited by:
Hailin Tang, Sun Yat-sen University Cancer Center (SYSUCC), ChinaReviewed by:
Chao-Ke Tang, University of South China, ChinaPádraig D’Arcy, Linköping University, Sweden
Zhongcheng Mo, Guilin Medical University, China
Copyright © 2021 Zhang, Zhou, Yang, Zhang, Zhang, Tan, Chen, Li and Tu. This is an open-access article distributed under the terms of the Creative Commons Attribution License (CC BY). The use, distribution or reproduction in other forums is permitted, provided the original author(s) and the copyright owner(s) are credited and that the original publication in this journal is cited, in accordance with accepted academic practice. No use, distribution or reproduction is permitted which does not comply with these terms.
*Correspondence: Tao Li, bGl0YW9Ac2h2cmkuYWMuY24=; Jian Tu, dHVqaWFuMDczNEBhbGl5dW4uY29t
†These authors have contributed equally to this work