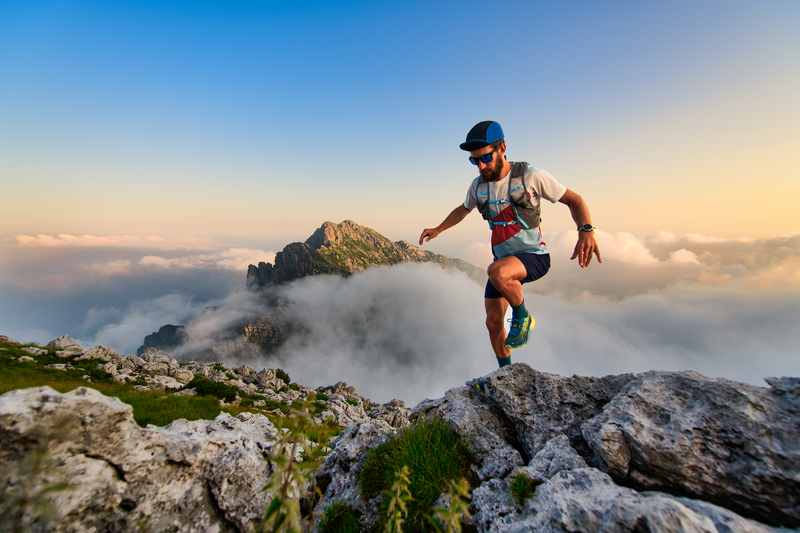
95% of researchers rate our articles as excellent or good
Learn more about the work of our research integrity team to safeguard the quality of each article we publish.
Find out more
MINI REVIEW article
Front. Oncol. , 26 February 2021
Sec. Molecular and Cellular Oncology
Volume 11 - 2021 | https://doi.org/10.3389/fonc.2021.616079
This article is part of the Research Topic New Roles of Autophagy Pathways in Cancer View all 24 articles
Pancreatic ductal adenocarcinoma (PDAC), one of the most aggressive solid malignancies, is characterized by the presence of oncogenic KRAS mutations, poor response to current therapies, prone to metastasis, and a low 5-year overall survival rate. Macroautophagy (herein referred to as autophagy) is a lysosome-dependent degradation system that forms a series of dynamic membrane structures to engulf, degrade, and recycle various cargoes, such as unused proteins, damaged organelles, and invading pathogens. Autophagy is usually upregulated in established cancers, but it plays a dual role in the regulation of the initiation and progression of PDAC. As a type of selective autophagy, mitophagy is a mitochondrial quality control mechanism that uses ubiquitin-dependent (e.g., the PINK1-PRKN pathway) and -independent (e.g., BNIP3L/NIX, FUNDC1, and BNIP3) pathways to regulate mitochondrial turnover and participate in the modulation of metabolism and cell death. Genetically engineered mouse models indicate that the loss of PINK1 or PRKN promotes, whereas the depletion of BNIP3L inhibits oncogenic KRAS-driven pancreatic tumorigenesis. Mitophagy also play a dual role in the regulation of the anticancer activity of certain cytotoxic agents (e.g., rocaglamide A, dichloroacetate, fisetin, and P. suffruticosa extracts) in PDAC cells or xenograft models. In this min-review, we summarize the latest advances in understanding the complex role of mitophagy in the occurrence and treatment of PDAC.
More than 90% of pancreatic cancers are ductal adenocarcinoma (PDAC), which is highly malignant, difficult to diagnose early, and has a very poor prognosis. It is estimated that by 2030, pancreatic cancer will become the second largest tumor-related death in humans (1). Although there have been a variety of “precision” targeted therapies for certain solid cancers (such as lung and breast cancer), the clinical treatment of PDAC is still in the “non-precision” era. The effective rate of the widely used gemcitabine regimen is only 30%, while FOLFIRINOX (a regimen consisting of 5-fluorouracil, leucovorin, irinotecan, and oxaliplatin) has serious adverse reactions, and the targeted drug erlotinib (an oral tyrosine kinase inhibitor of epidermal growth factor receptor [EGFR]) as well as cutting-edge immune checkpoint inhibitors have limited efficacy in patients with PDAC (2). How to achieve “precise” diagnosis and treatment of PDAC is a challenging issue in clinical practice. This clinical goal may require in-depth basic research to understand the complex pathological mechanisms of PDAC initiation and development.
Cells produce a large amount of waste every day, which needs to be removed by an integrated degradation system to maintain normal cell functions. In addition to the ubiquitin-proteasome system (UPS), autophagy is a lysosomal-dependent pathway that can remove various endogenous cellular materials (such as proteins and organelles) and exogenous invading pathogens. Autophagy dysfunction (including defects or over-activation) may cause abnormal cell components and functions, leading to various pathological conditions and diseases (3). Therefore, it is important to understand the types, functions, and regulation of autophagy under different conditions (4). The focus on autophagy provides a promising alternative to the development of new treatment options for human diseases. In this min-review, we describe the types of autophagy and the mechanism of mitophagy, and then analyze the effects of mitophagy on PDAC, including tumorigenesis and tumor treatment.
According to the different ways of transporting cellular components to lysosomes, autophagy is divided into the following categories (Figure 1A) (4). 1) Macroautophagy. The process of macroautophagy is a dynamic membrane reforming process involving the formation and maturation of three special structures: phagophore (also known as separated membrane produced by endoplasmic reticulum, mitochondria, or other subcellular membrane organelles), autophagosome (a double-membrane organelle phagocytosing degradable materials), and autolysosome (a hybrid organelle formed by the fusion of autophagosomes and lysosomes) where sequestered material is degraded by lysosomal hydrolases. 2) Microautophagy: lysosome membrane directly envelops longevity protein and then degrades in lysosome; 3) Chaperone-mediated autophagy (5): proteins containing KFERQ-like motifs bind to molecular chaperones (such as heat shock protein family A (Hsp70) member 8 [HSPA8/HSC70]), and then are transported to the lysosome cavity by lysosomal associated membrane protein 2 (LAMP2/LAMP2A) to be digested by lysosomal enzymes. It is worth noting that the multimerization of LAMP2 is required to transport the substrate into the lysosomal cavity (6, 7). Among them, macroautophagy (hereinafter referred to as autophagy) is the most common and well-studied form of autophagy in mammalian cells. The so-called autophagy-related (ATG) genes or proteins play a key role in the regulation of autophagy membrane dynamics through protein-protein interaction, and post-translational modifications (especially phosphorylation) further regulate autophagic process by affecting ATG function (8).
Figure 1 The role of mitophagy in pancreatic tumorigenesis. (A) In mammalian cells, there are three main types of autophagy: microautophagy, macroautophagy, and chaperone-mediated autophagy. Macroautophagy can be further divided into selective and non-selective forms. (B) Core mitophagy regulators mediate mitochondrial clearance. (C, D) PINK1/PRKN and BNIP3L-dependent mitophagy play different roles in inhibiting or promoting pancreatic tumorigenesis, respectively.
According to the selectivity of the substrate to be degraded, autophagy is further divided into selective autophagy and non-selective autophagy to control cell fate (9, 10) (Figure 1A). Non-selective autophagy refers to non-specific degradation processes, such as starvation-induced autophagic degradation. In addition to the core autophagy mechanism, selective autophagy also requires specific autophagy receptors to selectively degrade specific cargo (9, 11, 12). For example, xenophagy (13), clockophagy (14, 15), and mitophagy (16) can selectively degrade invading pathogens, aggregated circadian protein aryl hydrocarbon receptor nuclear translocator like (ARNTL), and damaged mitochondria, respectively (Figure 1A). This selective autophagy mainly depends on the molecular bridge-like autophagy receptor (also called adaptor protein), which not only specifically binds to the substrate, but also binds to members of the ATG8/LC3 family (MAP1LC3A, MAP1LC3B, MAP1LC3C, GABARAP, GABARAPL1/GEC1, GABARAPL2/GATE-16, and GABARAPL3) through different structure domains (12). The number of genes in the ATG8/LC3 family may be caused by gene duplication and loss events during evolution. LC3-II is a standard marker for autophagosomes, which is produced by conjugating cytoplasmic LC3-I with phosphatidylethanolamine on the surface of newborn autophagosomes (17). It is worth noting that certain autophagy receptors (such as sequestosome 1 [SQSTM1/p62]) act on both selective and non-selective autophagy during stress (18). In addition, the protein level of SQSTM1 is also regulated by the crosstalk between the UPS and autophagy pathways (19). Collectively, these kinetics indicate that a complex feedback network is involved in metabolism and signal transduction to control substrate degradation (20).
Mitochondria are organelles composed of two membranes (inner membrane and outer membrane) found in most cells. Normal mitochondria act as a “power factory” whose main function is to perform aerobic respiration to produce adenosine triphosphate (ATP). In addition, the interaction between mitochondrial and non-mitochondrial metabolic pathways is important for generating secondary signals (such as reactive oxygen species [ROS] and calcium) and biological macromolecules (such as proteins, carbohydrates, lipids, and nucleic acids). Therefore, maintaining healthy mitochondria, including quantity and quality, is essential for cell homeostasis. Conversely, damage to the mitophagy pathway can cause various pathological conditions (such as inflammation) and diseases (such as neurodegenerative diseases and cancer) (21–23). As an important component of the mitochondrial quality control mechanism, mitophagy can be activated through either ubiquitin (Ub)-dependent or independent pathway (Figure 1A), which is regulated by various proteins, including mitochondrial inner or outer membrane proteins (Figure 1B).
Mitophagy that rely on Ub can be further divided into classical and non-classical pathways (24). The classical pathway is mediated by the PTEN induced kinase 1 (PINK1, a serine–threonine protein kinase) and parkin RBR E3 ubiquitin protein ligase (PRKN/PARK2) (25). Mutations in PINK1 and PRKN are one of the important causes of Parkinson’s disease, a progressive neurodegenerative disease with motor and non-motor symptoms. The impaired PINK1-PRKN-dependent mitophagy pathway also promotes various types of tumor formation, including PDAC (discussed later). Mechanistically, oxidative damage to the mitochondria causes the accumulation of PINK1 on the mitochondrial outer membrane and the recruitment of PRKN from the cytoplasm to the mitochondria, leading to subsequent assembly of phosphorylated Ub chains on mitochondrial outer membrane proteins (25). In addition to the earliest reported SQSTM1 (25), other autophagy receptors, such as optineurin (OPTN) (26), neighbor of BRCA1 gene 1 (NBR1) (27), calcium binding and coiled-coil domain 2 (CALCOCO2/NDP52) (28), and tax1 binding protein 1 (TAX1BP1) (28), also help to recognize and degrade damaged mitochondria after activating the PINK1-PRKN pathway (Figure 1A). Moreover, PINK1-PRKN-mediated mitophagy can be reversed by deubiquitinating enzymes, such as ubiquitin-specific peptidase 8 (USP8), USP15, USP30, and USP35 (29). Non-classical Ub-dependent mitophagy is mediated by non-PRKN E3 ubiquitin ligases (such as mitochondrial E3 ubiquitin protein ligase 1 [MUL1] (30), siah E3 ubiquitin protein ligase 1 [SIAH1] (31), SMAD specific E3 ubiquitin protein ligase 1 [SMURF1] (32), and autocrine motility factor receptor [AMFR/GP78]) (33). The impact of crosstalk between Ub-dependent classical and non-classical mitophagy pathways on tumors is still poorly understood.
Ub-independent mitophagy is mediated by receptors, rather than E3 ligases. Recently, depending on the stimulus and cell type, the list of mitophagy receptors is increasing (34). In addition to the early reports of BCL2 interacting protein 3 like (BNIP3L/NIX) acting as a mitophagy receptor in red cells (35), other mitophagy receptors, including FUN14 domain containing 1 (FUNDC1) (36), BCL2 interacting protein 3 (BNIP3) (37), nipsnap homolog 1 (NIPSNAP1) (38), nipsnap homolog 2 (NIPSNAP2) (38), prohibitin 2 (PHB2) (39), BCL2 like 13 (BCL2L13) (40) and FKBP prolyl isomerase 8 (FKBP8) (41), have also been identified in cancer and non-cancer cells (Figure 1B). These unique receptors are responsible for binding to different mitochondrial membrane components in response to various stresses (such as hypoxia and oxidative damage). In addition to protein, non-protein mitochondrial components, such as cardiolipin and ceramide (42), also mediate mitophagy in some case, indicating that there are complex mitophagy sub-routes to regulate mitochondrial turnover and function.
Compared to normal cells, pancreatic cancer cells generally exhibit highly fragmented mitochondria, which is associated with increased mitochondrial fission and numbers as well as enhanced mitochondrial oxidative phosphorylation or glycolysis (43–45). Therefore, understanding the mechanism of mitochondrial biogenesis and turnover in different stages of pancreatic cancer, including initiation, progression, and metastasis, is essential for the next generation of cancer treatments. Indeed, increased autophagy or mitophagy levels are observed in various types of pancreatic cancer (46–48). However, autophagy plays a dual role in various cancer (including PDAC), depending on many factors, such as tumor stage, tumor microenvironment, gene mutation status involving oncogenes and tumor suppressor genes, and metabolic reprogramming (49–54). PDAC is a heterogeneous disease and can be morphologically classified into four types: conventional, tubulo-papillary, squamous, and “composite”, which exhibit different molecular and genetic characteristics (55). Generally, autophagy inhibits the growth of PDAC in the early stage by limiting DNA damage or inflammation, and upregulated autophagy in the later stage can promote PDAC survival by limiting cell death or anti-tumor immunity (56–60). Since covering all the effects of autophagy in PDAC is outside the scope of this min-review, we will only discuss the modulation and function of mitophagy in PDAC as described below.
Evidence is accumulating that both intrinsic genetic factor and extrinsic environmental factor are important for tumorigenesis. For pancreatic cancer, the oncogenic KRAS mutation is a key driving force for the formation of precursor lesions and subsequent development of PDAC with stromal response (61). KRAS activation is related to changes in mitochondrial morphology (e.g., increased mitochondrial fragmentation) and function (for example, reduction of mitochondrial respiratory complex I activity, enhancement of glycolytic activity, promotion of ROS production and induction of mitophagy) in various cancers (including PDAC) (62–66). Moreover, the conditional expression of endogenous KrasG12D in the pancreas of mice (Pdx1-Cre;KrasG12D; called KC mice) can mimic most of pathological development of human PDAC (67). This spontaneous transgenic PDAC mouse model is widely used to further consume or overexpress additional genes to evaluate the function of target genes in pancreatic tumorigenesis (Table 1). For example, based on KC mice, further depletion of the tumor suppressor high mobility group box 1 (HMGB1, Pdx1-Cre;KrasG12D;Hmgb1-/-) (69) or overexpression of tumor protein p53 (TP53) mutation (Pdx1-Cre;KrasG12D;Tp53R172H, termed KPC mice) (70) can significantly promote the development of KRAS-driven PDAC. HMGB1 is a positive regulator of autophagy and mitophagy, coupled with TP53 signaling in a variety of tumors (71–74). Cytoplasmic HMGB1 is a BECN1-binding protein that contributes to the formation of autophagosomes (72). Nuclear HMGB1 promotes the expression of heat shock protein β-1 (HSPB1) and subsequent HSPB1-mediated cytoskeletal integrity, which is required for the membrane dynamics of mitophagy (71). In addition, mitochondrial HMGB1 repairs mitochondrial genomic DNA damage, which also plays a potential role in suppressing tumorigenesis (75). However, depletion of mitophagy regulators, including PINK1 [Pdx1-Cre;KrasG12D;Pink1-/-] (22), PRKN [Pdx1-Cre;KrasG12D;Prkn-/-] (22), or BNIP3L/NIX [Pdx1-Cre;KrasG12D;Bnip3l-/- or Pdx1-Cre;KrasG12D;Tp53R172H; Bnip3l-/- (68), in KC mice exhibits different phenotype in pancreatic tumorigenesis. These transgenic animal studies show that Ub-dependent and independent mitophagy pathways play different roles in PDAC.
Dysregulated autophagy promotes or inhibits the growth of pancreatic cancer by interfering with different metabolic pathways or tumor signals, such as carbohydrate metabolism, fatty acid β-oxidation, and amino acid transport (48). For example, the reduced glycolysis gene PKM2 promotes survival by maintaining autophagy induced by low glucose in PDAC cells (76). Autophagy-mediated lipid degradation and subsequent fatty acid β-oxidation may provide additional resources for ATP production during PDAC growth (77, 78). Autophagy-mediated degradation of cellular material provides reusable amino acids for PDAC cell proliferation during glutamine deprivation (79). In addition, the PINK1-PRKN pathway can degrade mitochondrial iron importers (such as solute carrier family 25 member 37 [SLC25A37] and solute carrier family 25 member 28 [SLC25A28]) through SQSTM1-mediated mitophagy to inhibit carcinogenic KRAS-driven pancreatic tumorigenesis in mice, thereby inhibiting mitochondrial iron-mediated absent in melanoma 2 (AIM2)-dependent inflammasome activation and the subsequent activation of damage associated molecular pattern (DAMP, such as HMGB1)-dependent immune checkpoint expression (e.g., CD274/PD-L1) (Figure 1C) (22). These findings establish a role of PINK1/PRKN-mediated mitophagy to inhibit pancreatic tumorigenesis by limiting chronic inflammation-related immunosuppression in the hypoxic tumor microenvironment (80). Of note, high expression of PRKN mRNA was found to be associated with improved survival of pancreatic cancer patients, whereas mRNA expression of PINK1 did not influence patient survival (22), indicating that PINK1 is a contributor of PDAC, but it is not a potential biomarker. In addition, both PINK1 and PRKN may have mitophagy-independent functions in controlling the quality of mitochondria during pancreatic tumorigenesis (22).
In contrast, in a precursor lesion called pancreatic intraepithelial neoplasia (PanIN), oncogenic KRAS-mediated BNIP3L expression may activate mitophagy in a rapidly accelerated fibrosarcoma (RAF)-mitogen-activated protein kinase (MAPK)-dependent manner to limit the flux of glucose to mitochondria and enhance reduced nicotinamide adenine dinucleotide phosphate (NADPH)-dependent redox capacity, thereby promoting pancreatic tumorigenesis (Figure 1D) (68). In KC and KPC pancreatic cancer models, the depletion of additional BNIP3L will increase the content of mitochondria in PanIN, thereby increasing the production of mitochondrial ROS to limit the development of PanIN to PDAC (68). These observations indicate that BNIP3L-mediated mitophagy have different roles in promoting pancreatic tumorigenesis by enhancing the antioxidant capacity of cancer cells for cell proliferation and metastasis. However, oxidative stress and redox regulation are double-edged swords in tumorigenesis (81). Certain types of oxidative cell death, such as necroptosis (a caspase-independent regulated necrosis) and ferroptosis (an iron-dependent regulated necrosis), can promote KRAS-driven PDAC by activating inflammation-related immune suppression (82–84). Whether PINK1, PRKN2, and BNIP3L have non-mitochondrial functions in the modulation of the oncogene KRAS signal remains to be seen. In addition, various types of regulated cell death are closely related to autophagy (85–87), which may accelerate the complexity of the immune characteristics of the tumor microenvironment, thereby affecting anti-tumor immunity.
There is emerging evidence that impaired mitophagy is related to epithelial-mesenchymal transition and pancreatic cancer stem cells (pCSCs), which are pluripotent, self-renewable, and capable of forming tumors (88). In particular, the interferon signaling pathway-mediated the upregulation of Ub-like modifier interferon-stimulated gene 15 (ISG15) and its modification ISGylation maintain mitophagy and metabolic plasticity of pCSCs (89), suggesting a potential link between interferon, mitophagy, and metabolism in pCSCs. PDAC patients with high ISG15 levels showed increased expression of genes related to the CSC pathway, including epithelial-mesenchymal transition and oxidative phosphorylation (89). In contrast, the inhibition of ISG15/ISGylation impairs PRKN-dependent mitophagy, causing pCSCs to fail to eliminate dysfunctional and unhealthy mitochondria (89). Overall, these findings support a role of ISG15 in pCSCs by regulating mitochondrial dynamics and energy metabolism. The role of ISG15 in pancreatic tumorigenesis needs to be further studied using transgenic mice.
The purpose of tumor treatment is to induce death in tumor cells without damaging normal cells. Cell death can be divided into accidental or regulated cell death (90). Regulated cell death further includes apoptotic and non-apoptotic forms. In addition to the extensively studied apoptosis (91, 92), the induction of non-apoptotic regulated cell death [such as necroptosis (93, 94), alkaliptosis (95, 96), and ferroptosis (97–101)] in preclinical PDAC models has shown promising results in inhibiting tumor growth. As a metabolic center, mitochondria play a complex role in regulating apoptosis and non-apoptotic cell death by cooperating with other subcellular organelles (102). Accordingly, mitophagy-mediated mitochondrial degradation and turnover is reasonable to affect the anti-cancer activity of cytotoxic agents in PDAC cells. The best cell models for studying mitochondrial biology and mitophagy of pancreatic cancer are various human PDAC cell lines with KRAS mutations. For example, rocaglamide A, a natural product from the plant Aglaia elliptifolia, has the ability to induce PINK1/PRKN-mediated mitophagy as a negative feedback mechanism to limit rocaglamide A-induced apoptosis in various human PDAC cell lines with KRAS mutations (103). In contrast, the inhibition of mitophagy by Mdivi-1 enhances the anti-cancer activity of rocaglamide A in PDAC cells (103). Overexpression of serine/threonine kinase 25 (STK25, also known as MST1) in various PDAC cells induces apoptosis by inhibiting mitophagy mediated by mitofusin 2 (MFN2) (104). In contrast, leflunomide, an FDA-approved arthritis drug, can inhibit the growth of PDAC tumors by inducing MFN2 expression and subsequent mitophagy (44). In addition, in vitro and xenograft models, the combination of cyst(e)inase (an engineered human enzyme) and anuranofin (a thioredoxin reductase inhibitor) can inhibit mitophagy, thereby increase ROS production and apoptosis in the human PDAC cells (105). In other cases, dichloroacetate (an inhibitor of pyruvate dehydrogenase kinase) (106), fisetin (a bioactive flavonoid molecule found in fruits and vegetables) (107), and P. suffruticosa extracts (108) may play a context-related role in the induction of mitophagy and tumor suppression in PDAC cells. These findings further indicate that the complex relationship between mitophagy and mitochondrial dynamics can affect the effects of chemotherapy and targeted therapy.
In addition to PDAC cells, pCSCs is another cell model for studying mitochondrial dysfunction. pCSCs not only promote the growth and metastasis of pancreatic tumors, but also mediate chemoresistance. Metformin is a biguanide anti-diabetic drug that activates AMP-activated protein kinase (AMPK) to trigger autophagy (109). Retrospective studies have shown that compared with patients receiving insulin or sulfonylureas, many diabetic patients with solid tumors (including pancreatic cancer) treated with metformin have a survival benefit (109). The loss of ISG15 in pCSCs by CRISPR-Cas9 technology results in sensitivity to metformin therapy in xenograft models (89). These findings further indicate a potential role of ISG15 in regulating the anti-cancer activity of metformin in pCSCs. Further investigations are still needed to determine whether ISG15 directly regulates AMPK activation in pCSCs.
In the past decade, basic and clinical research on autophagy has involved various diseases, including pancreatic cancer (48, 59, 110–112). With the deepening of research, the functions of autophagy in tumor biology show diversity and complexity. One of the important reasons is that autophagy can have different degradation substrates, and these substrates can play a tumor-promoting and anti-tumor effect. In addition, the degree of substrate degradation (such as complete or partial degradation) also affects the function of autophagy in tumors. Similarly, mitochondrial coupling with mitochondrial biogenesis also plays a dual role in cancer. In this min-review, we discussed the context-dependent role of mitophagy in pancreatic cancer. Although this information enhances our understanding of the role of mitochondrial homeostasis in pancreatic cancer, there are still some key questions about the process and function of mitophagy in PDAC. How does the multi-step mitophagy actually proceed at different stages of PDAC? What are the key molecules or signals that distinguish the functions of mitophagy in promoting or inhibiting pancreatic tumorigenesis? Do tumor cells and non-tumor cells (such as immune cells or stromal cells) in the pancreatic tumor microenvironment have different mitophagy activities? In the pancreatic tumor microenvironment, what is the synergy or competition between mitophagy and other types of selective autophagy? How to develop specific mitophagy targeted drugs to kill pancreatic tumors? Are there specific markers to assess the level of mitophagy in PDAC patients?
YX and DT conceived of the topic for this review. All authors contributed to the article and approved the submitted version.
YX was supported by the National Natural Science Foundation of China (No. 81802476).
The authors declare that the research was conducted in the absence of any commercial or financial relationships that could be construed as a potential conflict of interest.
1. Rahib L, Smith BD, Aizenberg R, Rosenzweig AB, Fleshman JM, Matrisian LM. Projecting cancer incidence and deaths to 2030: the unexpected burden of thyroid, liver, and pancreas cancers in the United States. Cancer Res (2014) 74:2913–21. doi: 10.1158/0008-5472.CAN-14-0155
2. Amanam I, Chung V. Targeted Therapies for Pancreatic Cancer. Cancers (Basel) (2018) 2:36. doi: 10.3390/cancers10020036
3. Levine B, Kroemer G. Biological Functions of Autophagy Genes: A Disease Perspective. Cell (2019) 176:11–42. doi: 10.1016/j.cell.2018.09.048
4. Dikic I, Elazar Z. Mechanism and medical implications of mammalian autophagy. Nat Rev Mol Cell Biol (2018) 19:349–64. doi: 10.1038/s41580-018-0003-4
5. Bandyopadhyay U, Kaushik S, Varticovski L, Cuervo AM. The chaperone-mediated autophagy receptor organizes in dynamic protein complexes at the lysosomal membrane. Mol Cell Biol (2008) 28:5747–63. doi: 10.1128/MCB.02070-07
6. Chiang HL, Terlecky SR, Plant CP, Dice JF. A role for a 70-kilodalton heat shock protein in lysosomal degradation of intracellular proteins. Science (1989) 246:382–5. doi: 10.1126/science.2799391
7. Cuervo AM, Dice JF. A receptor for the selective uptake and degradation of proteins by lysosomes. Science (1996) 273:501–3. doi: 10.1126/science.273.5274.501
8. Xie Y, Kang R, Sun X, Zhong M, Huang J, Klionsky DJ, et al. Posttranslational modification of autophagy-related proteins in macroautophagy. Autophagy (2015) 11:28–45. doi: 10.4161/15548627.2014.984267
9. Liu J, Kuang F, Kroemer G, Klionsky DJ, Kang R, Tang D. Autophagy-Dependent Ferroptosis: Machinery and Regulation. Cell Chem Biol (2020) 27:420–35. doi: 10.1016/j.chembiol.2020.02.005
10. Green DR, Levine B. To be or not to be? How selective autophagy and cell death govern cell fate. Cell (2014) 157:65–75. doi: 10.1016/j.cell.2014.02.049
11. Vernon PJ, Tang D. Eat-me: autophagy, phagocytosis, and reactive oxygen species signaling. Antioxid Redox Signal (2013) 18:677–91. doi: 10.1089/ars.2012.4810
12. Gatica D, Lahiri V, Klionsky DJ. Cargo recognition and degradation by selective autophagy. Nat Cell Biol (2018) 20:233–42. doi: 10.1038/s41556-018-0037-z
13. Sharma V, Verma S, Seranova E, Sarkar S, Kumar D. Selective Autophagy and Xenophagy in Infection and Disease. Front Cell Dev Biol (2018) 6:147. doi: 10.3389/fcell.2018.00147
14. Yang M, Chen P, Liu J, Zhu S, Kroemer G, Klionsky DJ, et al. Clockophagy is a novel selective autophagy process favoring ferroptosis. Sci Adv (2019) 5:eaaw2238. doi: 10.1126/sciadv.aaw2238
15. Liu J, Yang M, Kang R, Klionsky DJ, Tang D. Autophagic degradation of the circadian clock regulator promotes ferroptosis. Autophagy (2019) 15:2033–5. doi: 10.1080/15548627.2019.1659623
16. Palikaras K, Lionaki E, Tavernarakis N. Mechanisms of mitophagy in cellular homeostasis, physiology and pathology. Nat Cell Biol (2018) 20:1013–22. doi: 10.1038/s41556-018-0176-2
17. Kabeya Y, Mizushima N, Ueno T, Yamamoto A, Kirisako T, Noda T, et al. LC3, a mammalian homologue of yeast Apg8p, is localized in autophagosome membranes after processing. EMBO J (2000) 19:5720–8. doi: 10.1093/emboj/19.21.5720
18. Katsuragi Y, Ichimura Y, Komatsu M. p62/SQSTM1 functions as a signaling hub and an autophagy adaptor. FEBS J (2015) 282:4672–8. doi: 10.1111/febs.13540
19. Liu WJ, Ye L, Huang WF, Guo LJ, Xu ZG, Wu HL, et al. p62 links the autophagy pathway and the ubiqutin-proteasome system upon ubiquitinated protein degradation. Cell Mol Biol Lett (2016) 21:29. doi: 10.1186/s11658-016-0031-z
20. Xie Y, Li J, Kang R, Tang D. Interplay Between Lipid Metabolism and Autophagy. Front Cell Dev Biol (2020) 8:431. doi: 10.3389/fcell.2020.00431
21. Kang R, Zeng L, Xie Y, Yan Z, Zhou B, Cao L, et al. A novel PINK1- and PARK2-dependent protective neuroimmune pathway in lethal sepsis. Autophagy (2016) 12:2374–85. doi: 10.1080/15548627.2016.1239678
22. Li C, Zhang Y, Cheng X, Yuan H, Zhu S, Liu J, et al. PINK1 and PARK2 Suppress Pancreatic Tumorigenesis through Control of Mitochondrial Iron-Mediated Immunometabolism. Dev Cell (2018) 46:441–455 e8. doi: 10.1016/j.devcel.2018.07.012
23. Sliter DA, Martinez J, Hao L, Chen X, Sun N, Fischer TD, et al. Parkin and PINK1 mitigate STING-induced inflammation. Nature (2018) 561:258–62. doi: 10.1038/s41586-018-0448-9
24. Harper JW, Ordureau A, Heo JM. Building and decoding ubiquitin chains for mitophagy. Nat Rev Mol Cell Biol (2018) 19:93–108. doi: 10.1038/nrm.2017.129
25. Geisler S, Holmstrom KM, Skujat D, Fiesel FC, Rothfuss OC, Kahle PJ, et al. PINK1/Parkin-mediated mitophagy is dependent on VDAC1 and p62/SQSTM1. Nat Cell Biol (2010) 12:119–31. doi: 10.1038/ncb2012
26. Wong YC, Holzbaur EL. Optineurin is an autophagy receptor for damaged mitochondria in parkin-mediated mitophagy that is disrupted by an ALS-linked mutation. Proc Natl Acad Sci U S A (2014) 111:E4439–48. doi: 10.1073/pnas.1405752111
27. Gao F, Chen D, Si J, Hu Q, Qin Z, Fang M, et al. The mitochondrial protein BNIP3L is the substrate of PARK2 and mediates mitophagy in PINK1/PARK2 pathway. Hum Mol Genet (2015) 24:2528–38. doi: 10.1093/hmg/ddv017
28. Lazarou M, Sliter DA, Kane LA, Sarraf SA, Wang C, Burman JL, et al. The ubiquitin kinase PINK1 recruits autophagy receptors to induce mitophagy. Nature (2015) 524:309–14. doi: 10.1038/nature14893
29. Wang Y, Serricchio M, Jauregui M, Shanbhag R, Stoltz T, Di Paolo CT, et al. Deubiquitinating enzymes regulate PARK2-mediated mitophagy. Autophagy (2015) 11:595–606. doi: 10.1080/15548627.2015.1034408
30. Yun J, Puri R, Yang H, Lizzio MA, Wu C, Sheng ZH, et al. MUL1 acts in parallel to the PINK1/parkin pathway in regulating mitofusin and compensates for loss of PINK1/parkin. Elife (2014) 3:e01958. doi: 10.7554/eLife.01958
31. Szargel R, Shani V, Abd Elghani F, Mekies LN, Liani E, Rott R, et al. The PINK1, synphilin-1 and SIAH-1 complex constitutes a novel mitophagy pathway. Hum Mol Genet (2016) 25:3476–90. doi: 10.1093/hmg/ddw189
32. Orvedahl A, Sumpter R Jr., Xiao G, Ng A, Zou Z, Tang Y, et al. Image-based genome-wide siRNA screen identifies selective autophagy factors. Nature (2011) 480:113–7. doi: 10.1038/nature10546
33. Fu M, St-Pierre P, Shankar J, Wang PT, Joshi B, Nabi IR. Regulation of mitophagy by the Gp78 E3 ubiquitin ligase. Mol Biol Cell (2013) 24:1153–62. doi: 10.1091/mbc.e12-08-0607
34. Xie Y, Liu J, Kang R, Tang D. Mitophagy Receptors in Tumor Biology. Front Cell Dev Biol (2020) 8:594203. doi: 10.3389/fcell.2020.594203
35. Sandoval H, Thiagarajan P, Dasgupta SK, Schumacher A, Prchal JT, Chen M, et al. Essential role for Nix in autophagic maturation of erythroid cells. Nature (2008) 454:232–5. doi: 10.1038/nature07006
36. Liu L, Feng D, Chen G, Chen M, Zheng Q, Song P, et al. Mitochondrial outer-membrane protein FUNDC1 mediates hypoxia-induced mitophagy in mammalian cells. Nat Cell Biol (2012) 14:177–85. doi: 10.1038/ncb2422
37. O’Sullivan TE, Johnson LR, Kang HH, Sun JC. BNIP3- and BNIP3L-Mediated Mitophagy Promotes the Generation of Natural Killer Cell Memory. Immunity (2015) 43:331–42. doi: 10.1016/j.immuni.2015.07.012
38. Princely Abudu Y, Pankiv S, Mathai BJ, Hakon Lystad A, Bindesboll C, Brenne HB, et al. NIPSNAP1 and NIPSNAP2 Act as “Eat Me” Signals for Mitophagy. Dev Cell (2019) 49:509–525 e12. doi: 10.1016/j.devcel.2019.03.013
39. Wei Y, Chiang WC, Sumpter R Jr., Mishra P, Levine B. Prohibitin 2 Is an Inner Mitochondrial Membrane Mitophagy Receptor. Cell (2017) 168:224–238 e10. doi: 10.1016/j.cell.2016.11.042
40. Murakawa T, Yamaguchi O, Hashimoto A, Hikoso S, Takeda T, Oka T, et al. Bcl-2-like protein 13 is a mammalian Atg32 homologue that mediates mitophagy and mitochondrial fragmentation. Nat Commun (2015) 6:7527. doi: 10.1038/ncomms8527
41. Bhujabal Z, Birgisdottir AB, Sjottem E, Brenne HB, Overvatn A, Habisov S, et al. FKBP8 recruits LC3A to mediate Parkin-independent mitophagy. EMBO Rep (2017) 18:947–61. doi: 10.15252/embr.201643147
42. Chu CT, Ji J, Dagda RK, Jiang JF, Tyurina YY, Kapralov AA, et al. Cardiolipin externalization to the outer mitochondrial membrane acts as an elimination signal for mitophagy in neuronal cells. Nat Cell Biol (2013) 15:1197–205. doi: 10.1038/ncb2837
43. Anderson GR, Wardell SE, Cakir M, Yip C, Ahn YR, Ali M, et al. Dysregulation of mitochondrial dynamics proteins are a targetable feature of human tumors. Nat Commun (2018) 9:1677. doi: 10.1038/s41467-018-04033-x
44. Yu M, Nguyen ND, Huang Y, Lin D, Fujimoto TN, Molkentine JM, et al. Mitochondrial fusion exploits a therapeutic vulnerability of pancreatic cancer. JCI Insight (2019) 4:e126915. doi: 10.1172/jci.insight.126915
45. Dai S, Peng Y, Zhu Y, Xu D, Zhu F, Xu W, et al. Glycolysis promotes the progression of pancreatic cancer and reduces cancer cell sensitivity to gemcitabine. BioMed Pharmacother (2020) 121:109521. doi: 10.1016/j.biopha.2019.109521
46. Daskalakis K, Alexandraki KI, Kloukina I, Kassi E, Felekouras E, Xingi E, et al. Increased autophagy/mitophagy levels in primary tumours of patients with pancreatic neuroendocrine neoplasms. Endocrine (2020) 68:438–47. doi: 10.1007/s12020-020-02228-1
47. Ko YH, Cho YS, Won HS, Jeon EK, An HJ, Hong SU, et al. Prognostic significance of autophagy-related protein expression in resected pancreatic ductal adenocarcinoma. Pancreas (2013) 42:829–35. doi: 10.1097/MPA.0b013e318279d0dc
48. Li J, Chen X, Kang R, Zeh H, Klionsky DJ, Tang D. Regulation and function of autophagy in pancreatic cancer. Autophagy (2020), 1–22. doi: 10.1080/15548627.2020.1847462
49. Levy JMM, Towers CG, Thorburn A. Targeting autophagy in cancer. Nat Rev Cancer (2017) 17:528–42. doi: 10.1038/nrc.2017.53
50. Monkkonen T, Debnath J. Inflammatory signaling cascades and autophagy in cancer. Autophagy (2018) 14:190–8. doi: 10.1080/15548627.2017.1345412
51. New M, Tooze S. The Role of Autophagy in Pancreatic Cancer-Recent Advances. Biol (Basel) (2019) 9:7. doi: 10.3390/biology9010007
52. Piffoux M, Eriau E, Cassier PA. Autophagy as a therapeutic target in pancreatic cancer. Br J Cancer (2021) 1244:333–44. doi: 10.1038/s41416-020-01039-5
53. Gorgulu K, Diakopoulos KN, Kaya-Aksoy E, Ciecielski KJ, Ai J, Lesina M, et al. The Role of Autophagy in Pancreatic Cancer: From Bench to the Dark Bedside. Cells (2020) 9:1063. doi: 10.3390/cells9041063
54. Kang R, Tang D. Autophagy in pancreatic cancer pathogenesis and treatment. Am J Cancer Res (2012) 2:383–96.
55. S NK, Wilson GW, Grant RC, Seto M, O’Kane G, Vajpeyi R, et al. Morphological classification of pancreatic ductal adenocarcinoma that predicts molecular subtypes and correlates with clinical outcome. Gut (2020) 69:317–28. doi: 10.1136/gutjnl-2019-318217
56. Yang S, Imamura Y, Jenkins RW, Canadas I, Kitajima S, Aref A, et al. Autophagy Inhibition Dysregulates TBK1 Signaling and Promotes Pancreatic Inflammation. Cancer Immunol Res (2016) 4:520–30. doi: 10.1158/2326-6066.CIR-15-0235
57. Loncle C, Molejon MI, Lac S, Tellechea JI, Lomberk G, Gramatica L, et al. The pancreatitis-associated protein VMP1, a key regulator of inducible autophagy, promotes Kras(G12D)-mediated pancreatic cancer initiation. Cell Death Dis (2016) 7:e2295. doi: 10.1038/cddis.2016.202
58. Rosenfeldt MT, O’Prey J, Morton JP, Nixon C, MacKay G, Mrowinska A, et al. p53 status determines the role of autophagy in pancreatic tumour development. Nature (2013) 504:296–300. doi: 10.1038/nature12865
59. Yamamoto K, Venida A, Yano J, Biancur DE, Kakiuchi M, Gupta S, et al. Autophagy promotes immune evasion of pancreatic cancer by degrading MHC-I. Nature (2020) 581:100–5. doi: 10.1038/s41586-020-2229-5
60. Kang R, Loux T, Tang D, Schapiro NE, Vernon P, Livesey KM, et al. 3rd, The expression of the receptor for advanced glycation endproducts (RAGE) is permissive for early pancreatic neoplasia. Proc Natl Acad Sci U.S.A. (2012) 109:7031–6. doi: 10.1073/pnas.1113865109
61. Waters AM, Der CJ. KRAS: The Critical Driver and Therapeutic Target for Pancreatic Cancer. Cold Spring Harb Perspect Med (2018) 8:a031435. doi: 10.1101/cshperspect.a031435
62. Lin HH, Chung Y, Cheng CT, Ouyang C, Fu Y, Kuo CY, et al. Autophagic reliance promotes metabolic reprogramming in oncogenic KRAS-driven tumorigenesis. Autophagy (2018) 14:1481–98. doi: 10.1080/15548627.2018.1450708
63. Nagdas S, Kashatus JA, Nascimento A, Hussain SS, Trainor RE, Pollock SR, et al. Drp1 Promotes KRas-Driven Metabolic Changes to Drive Pancreatic Tumor Growth. Cell Rep (2019) 28:1845–1859 e5. doi: 10.1016/j.celrep.2019.07.031
64. Palorini R, De Rasmo D, Gaviraghi M, Sala Danna L, Signorile A, Cirulli C, et al. Oncogenic K-ras expression is associated with derangement of the cAMP/PKA pathway and forskolin-reversible alterations of mitochondrial dynamics and respiration. Oncogene (2013) 32:352–62. doi: 10.1038/onc.2012.50
65. Meng N, Glorieux C, Zhang Y, Liang L, Zeng P, Lu W, et al. Oncogenic K-ras Induces Mitochondrial OPA3 Expression to Promote Energy Metabolism in Pancreatic Cancer Cells. Cancers (Basel) (2020) 12:65. doi: 10.3390/cancers12010065
66. Guo JY, Karsli-Uzunbas G, Mathew R, Aisner SC, Kamphorst JJ, Strohecker AM, et al. Autophagy suppresses progression of K-ras-induced lung tumors to oncocytomas and maintains lipid homeostasis. Genes Dev (2013) 27:1447–61. doi: 10.1101/gad.219642.113
67. Hingorani SR, Petricoin EF, Maitra A, Rajapakse V, King C, Jacobetz MA, et al. Preinvasive and invasive ductal pancreatic cancer and its early detection in the mouse. Cancer Cell (2003) 4:437–50. doi: 10.1016/S1535-6108(03)00309-X
68. Humpton TJ, Alagesan B, DeNicola GM, Lu D, Yordanov GN, Leonhardt CS, et al. Oncogenic KRAS Induces NIX-Mediated Mitophagy to Promote Pancreatic Cancer. Cancer Discovery (2019) 9:1268–87. doi: 10.1158/2159-8290.CD-18-1409
69. Kang R, Xie Y, Zhang Q, Hou W, Jiang Q, Zhu S, et al. Intracellular HMGB1 as a novel tumor suppressor of pancreatic cancer. Cell Res (2017) 27:916–32. doi: 10.1038/cr.2017.51
70. Hingorani SR, Wang L, Multani AS, Combs C, Deramaudt TB, Hruban RH, et al. Trp53R172H and KrasG12D cooperate to promote chromosomal instability and widely metastatic pancreatic ductal adenocarcinoma in mice. Cancer Cell (2005) 7:469–83. doi: 10.1016/j.ccr.2005.04.023
71. Tang D, Kang R, Livesey KM, Kroemer G, Billiar TR, Van Houten B, et al. High-mobility group box 1 is essential for mitochondrial quality control. Cell Metab (2011) 13:701–11. doi: 10.1016/j.cmet.2011.04.008
72. Tang D, Kang R, Livesey KM, Cheh CW, Farkas A, Loughran P, et al. Endogenous HMGB1 regulates autophagy. J Cell Biol (2010) 190:881–92. doi: 10.1083/jcb.200911078
73. Livesey KM, Kang R, Vernon P, Buchser W, Loughran P, Watkins SC, et al. p53/HMGB1 complexes regulate autophagy and apoptosis. Cancer Res (2012) 72:1996–2005. doi: 10.1158/0008-5472.CAN-11-2291
74. Chang HW, Kim MR, Lee HJ, Lee HM, Kim GC, Lee YS, et al. p53/BNIP3-dependent mitophagy limits glycolytic shift in radioresistant cancer. Oncogene (2019) 38:3729–42. doi: 10.1038/s41388-019-0697-6
75. Ito H, Fujita K, Tagawa K, Chen X, Homma H, Sasabe T, et al. HMGB1 facilitates repair of mitochondrial DNA damage and extends the lifespan of mutant ataxin-1 knock-in mice. EMBO Mol Med (2015) 7:78–101. doi: 10.15252/emmm.201404392
76. Li X, Deng S, Liu M, Jin Y, Zhu S, Deng S, et al. The responsively decreased PKM2 facilitates the survival of pancreatic cancer cells in hypoglucose. Cell Death Dis (2018) 9:133. doi: 10.1038/s41419-017-0158-5
77. Lee JS, Oh SJ, Choi HJ, Kang JH, Lee SH, Ha JS, et al. ATP Production Relies on Fatty Acid Oxidation Rather than Glycolysis in Pancreatic Ductal Adenocarcinoma. Cancers (Basel) (2020) 12:2477. doi: 10.3390/cancers12092477
78. Maan M, Peters JM, Dutta M, Patterson AD. Lipid metabolism and lipophagy in cancer. Biochem Biophys Res Commun (2018) 504:582–9. doi: 10.1016/j.bbrc.2018.02.097
79. Seo JW, Choi J, Lee SY, Sung S, Yoo HJ, Kang MJ, et al. Autophagy is required for PDAC glutamine metabolism. Sci Rep (2016) 6:37594. doi: 10.1038/srep37594
80. Kang R, Xie Y, Zeh HJ, Klionsky DJ, Tang D. Mitochondrial quality control mediated by PINK1 and PRKN: links to iron metabolism and tumor immunity. Autophagy (2019) 15:172–3. doi: 10.1080/15548627.2018.1526611
81. Reuter S, Gupta SC, Chaturvedi MM, Aggarwal BB. Oxidative stress, inflammation, and cancer: how are they linked? Free Radic Biol Med (2010) 49:1603–16. doi: 10.1016/j.freeradbiomed.2010.09.006
82. Seifert L, Werba G, Tiwari S, Giao Ly NN, Alothman S, Alqunaibit D, et al. The necrosome promotes pancreatic oncogenesis via CXCL1 and Mincle-induced immune suppression. Nature (2016) 532:245–9. doi: 10.1038/nature17403
83. Dai E, Han L, Liu J, Xie Y, Zeh HJ, Kang R, et al. Ferroptotic damage promotes pancreatic tumorigenesis through a TMEM173/STING-dependent DNA sensor pathway. Nat Commun (2020) 11:6339. doi: 10.1038/s41467-020-20154-8
84. Dai E, Han L, Liu J, Xie Y, Kroemer G, Klionsky DJ, et al. Autophagy-dependent ferroptosis drives tumor-associated macrophage polarization via release and uptake of oncogenic KRAS protein. Autophagy (2020) 16:2069–83. doi: 10.1080/15548627.2020.1714209
85. Tang D, Kang R, Berghe TV, Vandenabeele P, Kroemer G. The molecular machinery of regulated cell death. Cell Res (2019) 29:347–64. doi: 10.1038/s41422-019-0164-5
86. Bialik S, Dasari SK, Kimchi A. Autophagy-dependent cell death - where, how and why a cell eats itself to death. J Cell Sci (2018) 131:jcs215152. doi: 10.1242/jcs.215152
87. Zhou B, Liu J, Kang R, Klionsky DJ, Kroemer G, Tang D. Ferroptosis is a type of autophagy-dependent cell death. Semin Cancer Biol (2020) 66:89–100. doi: 10.1016/j.semcancer.2019.03.002
88. Guerra F, Guaragnella N, Arbini AA, Bucci C, Giannattasio S, Moro L. Mitochondrial Dysfunction: A Novel Potential Driver of Epithelial-to-Mesenchymal Transition in Cancer. Front Oncol (2017) 7:295. doi: 10.3389/fonc.2017.00295
89. Alcala S, Sancho P, Martinelli P, Navarro D, Pedrero C, Martin-Hijano L, et al. ISG15 and ISGylation is required for pancreatic cancer stem cell mitophagy and metabolic plasticity. Nat Commun (2020) 11:2682. doi: 10.1038/s41467-020-16395-2
90. Galluzzi L, Vitale I, Aaronson SA, Abrams JM, Adam D, Agostinis P, et al. Molecular mechanisms of cell death: recommendations of the Nomenclature Committee on Cell Death 2018. Cell Death Differ (2018) 25:486–541. doi: 10.1038/s41418-018-0102-y
91. Huang J, Chen P, Liu K, Liu J, Zhou B, Wu R, et al. CDK1/2/5 inhibition overcomes IFNG-mediated adaptive immune resistance in pancreatic cancer. Gut (2020). doi: 10.1136/gutjnl-2019-320441
92. Arlt A, Muerkoster SS, Schafer H. Targeting apoptosis pathways in pancreatic cancer. Cancer Lett (2013) 332:346–58. doi: 10.1016/j.canlet.2010.10.015
93. Xie Y, Zhu S, Zhong M, Yang M, Sun X, Liu J, et al. Inhibition of Aurora Kinase A Induces Necroptosis in Pancreatic Carcinoma. Gastroenterology (2017) 153:1429–1443 e5. doi: 10.1053/j.gastro.2017.07.036
94. Huang C, Lan W, Fraunhoffer N, Meilerman A, Iovanna J, Santofimia-Castano P. Dissecting the Anticancer Mechanism of Trifluoperazine on Pancreatic Ductal Adenocarcinoma. Cancers (Basel) (2019) 11:1869. doi: 10.3390/cancers11121869
95. Song X, Zhu S, Xie Y, Liu J, Sun L, Zeng D, et al. JTC801 Induces pH-dependent Death Specifically in Cancer Cells and Slows Growth of Tumors in Mice. Gastroenterology (2018) 154:1480–93. doi: 10.1053/j.gastro.2017.12.004
96. Liu J, Kuang F, Kang R, Tang D. Alkaliptosis: a new weapon for cancer therapy. Cancer Gene Ther (2020) 27:267–9. doi: 10.1038/s41417-019-0134-6
97. Li C, Zhang Y, Liu J, Kang R, Klionsky DJ, Tang D. Mitochondrial DNA stress triggers autophagy-dependent ferroptotic death. Autophagy (2020), 1–13. doi: 10.1080/15548627.2020.1739447
98. Zhu S, Zhang Q, Sun X, Zeh HJ 3rd3rd, Lotze MT, Kang R, et al. HSPA5 Regulates Ferroptotic Cell Death in Cancer Cells. Cancer Res (2017) 77:2064–77. doi: 10.1158/0008-5472.CAN-16-1979
99. Xie Y, Kuang F, Liu J, Tang D, Kang R. DUSP1 Blocks Autophagy-Dependent Ferroptosis in Pancreatic Cancer. J Pancreatol (2020) 3:154–60. doi: 10.1097/JP9.0000000000000054
100. Badgley MA, Kremer DM, Maurer HC, DelGiorno KE, Lee HJ, Purohit V, et al. Cysteine depletion induces pancreatic tumor ferroptosis in mice. Science (2020) 368:85–9. doi: 10.1126/science.aaw9872
101. Hou W, Xie Y, Song X, Sun X, Lotze MT, Zeh HJ 3rd3rd, et al. Autophagy promotes ferroptosis by degradation of ferritin. Autophagy (2016) 12:1425–8. doi: 10.1080/15548627.2016.1187366
102. Bock FJ, Tait SWG. Mitochondria as multifaceted regulators of cell death. Nat Rev Mol Cell Biol (2020) 21:85–100. doi: 10.1038/s41580-019-0173-8
103. Zhao C, He R, Shen M, Zhu F, Wang M, Liu Y, et al. PINK1/Parkin-Mediated Mitophagy Regulation by Reactive Oxygen Species Alleviates Rocaglamide A-Induced Apoptosis in Pancreatic Cancer Cells. Front Pharmacol (2019) 10:968. doi: 10.3389/fphar.2019.00968
104. Hu Y, Wang B, Wang L, Wang Z, Jian Z, Deng L. Mammalian STE20like kinase 1 regulates pancreatic cancer cell survival and migration through Mfn2mediated mitophagy. Mol Med Rep (2020) 22:398–404. doi: 10.3892/mmr.2020.11098
105. Kshattry S, Saha A, Gries P, Tiziani S, Stone E, Georgiou G, et al. Enzyme-mediated depletion of l-cyst(e)ine synergizes with thioredoxin reductase inhibition for suppression of pancreatic tumor growth. NPJ Precis Oncol (2019) 3:16. doi: 10.1038/s41698-019-0088-z
106. Tataranni T, Agriesti F, Pacelli C, Ruggieri V, Laurenzana I, Mazzoccoli C, et al. Dichloroacetate Affects Mitochondrial Function and Stemness-Associated Properties in Pancreatic Cancer Cell Lines. Cells (2019) 8:478. doi: 10.3390/cells8050478
107. Jia S, Xu X, Zhou S, Chen Y, Ding G, Cao L. Fisetin induces autophagy in pancreatic cancer cells via endoplasmic reticulum stress- and mitochondrial stress-dependent pathways. Cell Death Dis (2019) 10:142. doi: 10.1038/s41419-019-1366-y
108. Liu YH, Weng YP, Tsai HY, Chen CJ, Lee DY, Hsieh CL, et al. Aqueous extracts of Paeonia suffruticosa modulates mitochondrial proteostasis by reactive oxygen species-induced endoplasmic reticulum stress in pancreatic cancer cells. Phytomedicine (2018) 46:184–92. doi: 10.1016/j.phymed.2018.03.037
109. Saraei P, Asadi I, Kakar MA, Moradi-Kor N. The beneficial effects of metformin on cancer prevention and therapy: a comprehensive review of recent advances. Cancer Manag Res (2019) 11:3295–313. doi: 10.2147/CMAR.S200059
110. Liang C, Xu J, Meng Q, Zhang B, Liu J, Hua J, et al. TGFB1-induced autophagy affects the pattern of pancreatic cancer progression in distinct ways depending on SMAD4 status. Autophagy (2020) 16:486–500. doi: 10.1080/15548627.2019.1628540
111. Yang A, Herter-Sprie G, Zhang H, Lin EY, Biancur D, Wang X, et al. Autophagy Sustains Pancreatic Cancer Growth through Both Cell-Autonomous and Nonautonomous Mechanisms. Cancer Discovery (2018) 8:276–87. doi: 10.1158/2159-8290.CD-17-0952
Keywords: mitophagy, autophagy, PDAC - pancreatic ductal adenocarcinoma, tumorigenesis, therapy
Citation: Xie Y, Liu J, Kang R and Tang D (2021) Mitophagy in Pancreatic Cancer. Front. Oncol. 11:616079. doi: 10.3389/fonc.2021.616079
Received: 11 October 2020; Accepted: 22 January 2021;
Published: 26 February 2021.
Edited by:
Eugenia Morselli, Pontificia Universidad Católica de Chile, ChileReviewed by:
Maria Markaki, Foundation for Research and Technology Hellas (FORTH), GreeceCopyright © 2021 Xie, Liu, Kang and Tang. This is an open-access article distributed under the terms of the Creative Commons Attribution License (CC BY). The use, distribution or reproduction in other forums is permitted, provided the original author(s) and the copyright owner(s) are credited and that the original publication in this journal is cited, in accordance with accepted academic practice. No use, distribution or reproduction is permitted which does not comply with these terms.
*Correspondence: Yangchun Xie, eGlleWFuZ2NodW44OEBjc3UuZWR1LmNu; Daolin Tang, ZGFvbGluLnRhbmdAdXRzb3V0aHdlc3Rlcm4uZWR1
Disclaimer: All claims expressed in this article are solely those of the authors and do not necessarily represent those of their affiliated organizations, or those of the publisher, the editors and the reviewers. Any product that may be evaluated in this article or claim that may be made by its manufacturer is not guaranteed or endorsed by the publisher.
Research integrity at Frontiers
Learn more about the work of our research integrity team to safeguard the quality of each article we publish.