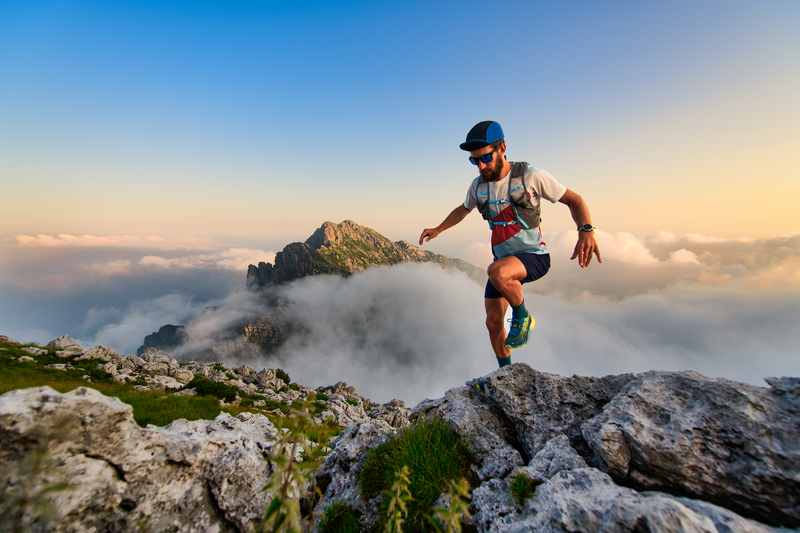
95% of researchers rate our articles as excellent or good
Learn more about the work of our research integrity team to safeguard the quality of each article we publish.
Find out more
REVIEW article
Front. Oncol. , 23 October 2020
Sec. Cancer Metabolism
Volume 10 - 2020 | https://doi.org/10.3389/fonc.2020.582884
This article is part of the Research Topic How do Metabolism, Angiogenesis, and Hypoxia Modulate Resistance? View all 10 articles
Advances in immunotherapy have achieved remarkable clinical outcomes in tumors with low curability, but their effects are limited, and increasing evidence has implicated tumoral and non-tumoral components of the tumor microenvironment as critical mediators of cancer progression. At the same time, the clinical successes achieved with minimally invasive and optically-guided surgery and image-guided and ablative radiation strategies have been successfully implemented in clinical care. More effective, localized and safer treatments have fueled strong research interest in radioimmunotherapy, which has shown the potential immunomodulatory effects of ionizing radiation. However, increasingly more observations suggest that immunosuppressive changes, metabolic remodeling, and angiogenic responses in the local tumor microenvironment play a central role in tumor recurrence. In this review, we address challenges to identify responders vs. non-responders to the immune checkpoint blockade, discuss recent developments in combinations of immunotherapy and radiotherapy for clinical evaluation, and consider the clinical impact of immunosuppressive changes in the tumor microenvironment in the context of surgery and radiation. Since the therapy-induced modulation of the tumor microenvironment presents a multiplicity of forms, we propose that overcoming microenvironment related resistance can become clinically relevant and represents a novel strategy to optimize treatment immunogenicity and improve patient outcome.
Cancer treatment modalities vary considerably depending on stage and location, however surgical excision and radiation therapy are an integral part of treatment for most solid tumors. In an era of exceptionally dynamic evolution of knowledge, some recently published clinical studies have reshaped the role of surgery such as neoadjuvant immunotherapy combinations leading to less invasive surgery for advanced melanoma, antiangiogenics as an alternative to immediate surgery in renal cell carcinoma or upfront treatments making surgery possible for more patients with pancreatic cancer (1). Most therapeutic combinations in clinical trials are based on knowledge of resistance mechanisms and recently immunotherapy, which has revolutionized the clinical management of multiple tumors, has been included in multiple clinical trials which are mainly based on T cell and pursue a maintained antitumor immune response. Accumulating evidence suggests that conditioning the tumor microenvironment (TME) toward an immunomodulatory state may have a major impact on cancer outcome (2, 3). However, the TME comprises all the non-malignant cellular and non-cellular components of the tumor, including the immune system, blood cells, endothelial cells, fat cells, and the stroma. The tumor stroma is a critical component of the TME with cancer-promoting capacity as part of the response to treatments and leads to cancer resistance. For example, immunosuppressive cytokine secretion and metabolic alterations strongly participate in the suppression of host immune responses against tumor cells facilitating tumor proliferation. Extensive work exploring the interactions between cancer cells and the TME has been done but the advancements still require a better understanding of the potential targets before implementation in conceptual antitumor strategies. In this regard, recent advances resulting in more effective and localized radiation treatments (stereotactic radiosurgery and stereotactic body radiotherapy, SRS/SBRT) can achieve an effective alteration and ablation of tumor stromal tissue, which can be a singular advantage against tumoral immune evasion [reviewed in (4)]. In addition, technological developments have led to minimally invasive surgery with evident clinical benefits in terms of less invasiveness, excellent outcomes, and a shorter hospital stay (5).
In this review, we address challenges to identify responders vs. non-responders to the immune checkpoint blockade (ICB), discuss recent developments in combinations of immunotherapy and radiotherapy for clinical evaluation, and consider the clinical impact of immunosuppressive changes in the TME in the context of surgery and radiation. Overcoming microenvironment related resistance may have a fundamental impact on treatment efficacy and patient outcome.
Immunotherapeutic approaches have transformed treatment and outcomes for some solid tumors, in particular, melanoma and non-small cell lung cancer (NSCLC), but do not benefit the majority of patients with cancer and have failed to induce broadly durable responses. Immunotherapy with ICB uses monoclonal antibodies that target the inhibitory proteins CTL antigen 4 (CTLA-4) or programmed death-1/programmed death-ligand 1 (PD-1/PD-L1) on T cells or cancer cells to unleash the immune response. However, response rates vary widely and predictive factors of response to ICB remain elusive. It has been suggested that PD-L1 expression, high tumor mutational burden (TMB) which is highly influenced by the epitopes displayed in the human leukocyte antigen (HLA) genes of a tumor, and the presence of CD8+ T cells are prognostic of clinical response to treatment with ICB (6).
The distinction between hot, altered (excluded and immunosuppressed) and cold tumors, based on the cytotoxic T cell landscape within a tumor, establishes the important role of the TME but only a thorough profiling of the TME can analyze the complexity of the tumors and provide dynamic information about the complex networks operating in the TME to guide clinical decisions (7, 8). Combining immune and genomic data has revealed six immune subtypes across 33 different cancer types including immune (macrophage or lymphocyte signatures, Th1:Th2 cell ratio, expression of immunomodulatory genes) and non-immune parameters (intratumoral heterogeneity, aneuploidy, neoantigen load, overall cell proliferation, and patients' prognosis) (9). It has been proposed that an integrative view of the multi-omics experimental platforms and computational power is required to identify signatures of immune response with improved predictive power (10).
It has been clearly established that CD8+ T cells are the ultimate effectors of tumor rejection and the strongest predictor of ICB response across tumor types. Significantly, the functional variability of tumor-infiltrating T cells can influence their cytotoxicity. Subsets with reactivation of dysfunctional CD8+, memory-like CD8+TCF7+, CD103+ tumor-resident CD8+, and Tcf1+PD-1+ CD8+ with stem-like properties T cells have shown durable responses. CD4+ T cell subpopulations that play a critical role in immunotherapy include CD4+ Th1 cells that generate functional CD8+ T cell responses, CD4+FoxP3+ regulatory T cells (Treg) generally associated with suppression of antitumor immune responses in several cancers although responses to CTLA-4 blockade have been shown, and CD4+FoxP3−PD-1Hi (4PD-1Hi) T cells can indicate a negative prognosis when there is persistence after PD-1 blockade (6). Emerging factors associated with ICB response include B cells and tertiary lymphoid structures (11, 12). As for innate immune populations, BDCA-3+CLEC9A+ dendritic cells (DC) and XCL1-producing NK cells are linked to ICB response (13).
ICB is most efficacious in tumors with a high degree of T cell infiltration (hot tumors), such as melanomas and NSCLC. Alternative combinations include other checkpoint molecules, such as T cell immunoglobulin and mucin domain-3 (TIM-3), lymphocyte-activation gene-3 (LAG3), and T cell immunoglobulin and ITIM domain (TIGIT) in the case of T cell exhaustion; or co-stimulatory checkpoint proteins, including OX-40, CD28, and 4-1BB ligand receptor to enhance T cell expansion or effector functions. Preliminary results also suggest a potential role of microbiome modulation. On the other hand, immune cold tumors, including pancreatic and prostate cancers, are not well-infiltrated by immune cells. Therefore, research efforts have focused on making cold tumors hot by increasing immune infiltration and activity, such as vascular normalization, increasing the neoantigen burden, oncolytic therapy, vaccines, adoptive T cell therapy, T cell immunomodulators, and radiotherapy. Clinical strategies in immune-altered tumors have an impact on T cell trafficking, inhibition of hypoxia-associated pathways, and the immune suppressive microenvironment (14).
As more combinations of immunotherapeutic strategies reach the clinical arena, two clinical challenges become more relevant. Checkpoint disruption leads to a wide range of inflammatory toxicities grouped as immune-related adverse events (irAEs). The majority occur in barrier tissues (gastrointestinal or pulmonary mucosa, skin) or in endocrine glands. Although many are mild, they can carry considerable morbidity, lead to reduced treatment dosage and/or duration, and on occasions may be fatal (e.g., in patients with pre-existing autoimmunity) (15, 16). On the other hand, it has been suggested that irAEs could help select responders to ICB in bladder cancer (17). Secondly, some patients experience an acceleration of tumor growth kinetics with poor survival called hyperprogression which, at present, remains difficult to characterize (18, 19).
The composition of the TME is dynamic and evolves during ICB treatment. It has been suggested that the TME evolves differently between responders and non-responders. Of interest, stronger differences were found early on-treatment than before the ICB based on the differences in the densities of CD4+ or CD8+ T cells and the expression of PD-1/PD-L1 after two or three anti-PD-1 doses than at baseline (20, 21). Another interesting feature is that PD-1 blockade can induce clonal replacement preferentially of exhausted CD8+ T cells, meaning that T cells present at baseline may show reduced proliferation and that the response to ICB could be due to T cell clones that enter the tumor during the course of treatment (22).
Clinical relevance of distinctions in the immune contexture mainly based on the cytotoxic landscape of T cells in tumors has been established although the potential of analyzing dynamics and plasticity of TME networks will offer more powerful stratification systems between responders and non-responders.
Interactions between malignant and non-malignant cells create the TME (Figure 1). Non-malignant cells are usually highly dynamic and display tumor-promoting capabilities. Major non-malignant cell types found in the TME are immune cells, vasculature and lymphatic vessels, and fibroblasts. Cell communication is accomplished by a network of cytokines, chemokines, and diverse metabolites that reacts to changes in the physical and chemical characteristics of the tissue (23). Cancer treatment effects induce a variety of mechanisms which lead to T cell exclusion and avoidance of their cytotoxic function (24) that ultimately shift the balance of stromal cell phenotypes in the TME toward an immunosuppressive state. These pro-tumorigenic responses to therapy can induce local and/or systemic changes that underlie tumor recurrence and treatment resistance.
Figure 1. A schematic representation of the immunosuppressive TME. In a tumor, cancer cells coexist with immune cells, fibroblasts, and blood vessels to form the TME. Cancer cells can alter the microenvironment and promote cancer growth and dissemination.
In a broad sense, the mechanisms leading to a pro-tumorigenic microenvironment can be grouped into three categories: immune cell regulation, metabolic reprogramming, and hypoxia (4). The biochemical and physical properties of the TME undergo substantial changes during tumor evolution and treatment determined by the increased demand for blood vessels to endure tumor growth, which requires an adequate supply of oxygen and nutrients delivered through the blood vasculature. The resulting abnormal vessels are leaky and compressed which can induce a dense stromal reaction and reduction of blood flow that promotes hypoperfusion. The TME then becomes hypoxic with enhanced potential for tumor progression in multiple ways. In this situation, hypoxia reduces immune cell activity and the TME acquires an immunosuppressive phenotype (25). Hence, better understanding and reprogramming of these components may greatly influence cancer outcome.
Clinically, this may significantly limit cancer treatment efficacy and represent a shift in our understanding of tumor progression and resistance. Major emphasis has been placed on advancing clinical applications that strengthen the effectiveness of immunotherapies, leading to rapid regulatory approval of ICB combined with targeted therapies and/or chemotherapy in large numbers of patients with cancer, facilitating their incorporation into clinical practice. However, in spite of the extensive use of surgery and radiation strategies in cancer, as a definitive strategy in early or moderately-advanced stages of cancer, as part of a multimodal strategy in advanced loco-regional disease and, more recently in selected cases of oligometastatic disease, there is very limited understanding of the biological changes in the TME induced by local treatments.
Radioimmunotherapy is an area of extensive research due to the potential immunomodulatory effects of ionizing radiation and has established a new paradigm in which radiation is as efficient as its capacity to elicit tumor-targeting immune responses (2).
Ionizing radiation is able to induce immunogenic cell death, a form of cell death that promotes a T cell-based immune response against antigens derived from dying cells, enhances antigen presentation, and activates cytotoxic T cells. Cytosolic DNA from dying cells function as neoantigens that are highly immunogenic. Radiation induces the release of danger signals, including calreticulin, high mobility group box 1 (HMGB1), and adenosine triphosphate (ATP), which are collectively known as damage-associated molecular patterns (DAMPs), and support the recruitment and maturation of antigen-presenting cells (APC), migrate to lymph nodes, and prime a cytotoxic T cell-dependent immune response.
Critical to the immunogenicity of radiotherapy is the fragmentation of nuclear DNA from the DNA damage response (DDR) of radiation, shuttled to the cytoplasm where it activates cyclic GMP-AMP synthase/stimulator of interferon genes (cGAS/STING) pathways and induces transcription of the IFN-stimulated genes. The cytoplasmic three-prime repair exonuclease 1 (Trex1), induced by radiation, is a negative regulator of this pathway. The release of IFN type I from APC supports antigen uptake by Batf3+ DC and cross-presentation of tumor antigens to CD8+ T cells. Activated CD8+ T cells are recruited to the irradiated tumor site by cytokines upregulated by radiation (CCL2, CXCL1, CXCL10, and CXCL16). In addition, radiation enhances the expression of major histocompatibility complex-I (MHC-I) antigens on cancer cells that favor antigen presentation (4).
Tumor cell-intrinsic events driven by DNA damage are central to the immunomodulatory effects of radiotherapy. Radiation-induced DNA damage alters gene transcription and modulates the expression of tumor neoantigens, resulting in activation of innate and/or adaptive antitumor immune response (6, 26, 27). The finding that a patient with metastatic, treatment refractory NSCLC who responded to ipilimumab plus radiotherapy was carrying a mutation in a KPNA2 gene, upregulated in expression by radiation; tumor-specific T cell clones were developed in peripheral blood shortly after completion of radiotherapy and the first dose of ipilimumab to a metastatic site and remained elevated while the patient achieved a complete response in all of the non-irradiated lesions supports the hypothesis of in situ tumor vaccination (28).
Identification of genetic determinants of radiotherapeutic efficacy has remained elusive but a recent report identifies genetic ATM inactivation to be strongly associated with clinical benefit from radiotherapy. The identification of a radiosensitive phenotype across multiple cancer types inaugurates the possibility of further testing in prospective clinical trials and progress in personalized radiation strategies. For example, patients with metastatic tumors harboring a somatic ATM mutation may receive a reduced dose of radiation with the goal of reducing toxicity and maintaining tumor control (Pitter et al., accepted).
Defects in DDR have been exploited for drug development as radiosensitizers including poly(ADP-ribose) polymerase (PARP), checkpoint kinase 1 (CHK1), DNA-dependent protein kinase (DNA-PK), or the chaperone HSP90 inhibitors. Radiation damage in the context of defective DDR pathways generates micronuclei in cancer cells that activate cGAS/STING pathways and propagate an inflammatory response that can enhance radiation effects. Adding ICB to the immunomodulation induced by DDR inhibitors plus radiotherapy is a new area of clinical research that can provide additional insights into the immunomodulatory effects of radiation given that DDR inhibitors can enhance the immunostimulatory effects of radiation while ICB can target the immunosuppressive radiation effects (27).
DC are a sparsely distributed immunological component of the TME with high biological heterogeneity that play a central role in linking innate and adaptive immune responses. Therefore, DC are a key element in the immunostimulatory effect of radiotherapy. It has been recently reported that poorly radioimmunogenic murine tumors fail to activate DC following treatment, and that it could be successfully reverted with an exogenous adjuvant, resulting in tumor cures (29). Therefore, it could be hypothesized that in patients with a poor TME, the combination of radiation with adjuvants that promote DC maturation or target the immunosuppressive TME can improve tumor control.
Toll-like receptors (TLR) signaling pathways activate innate immunity and regulate adaptive immune responses. Preclinical evidence suggests that TLR-agonists targeting TLR3, TLR 7/8 or TLR9 in combination with radiotherapy can enhance antitumor immunity with long-term tumor control. Mechanistically, TLR can enhance DC-mediated cross-presentation and activation of T cells. Novel formulations of TLR agonists with reduced toxicity and precise and image-guided radiation techniques are favorable aspects for this strategy (30, 31).
Studies on resistance to ICB reveal a complex and rapidly evolving network of mechanisms of immune resistance specific to each host and tumor (32). The absence of biomarkers that identify the different types of resistance obliges the use of empirical approaches to target them.
The immunogenicity of radiation has been approached with two different strategies, one that emphasizes the local interaction of radiotherapy and the immune system where the majority of clinical knowledge has been accumulated, and a second strategy where focal radiation elicits systemic disease control (abscopal effect) known as in situ tumor vaccination that has attracted a lot of attention. The basis for combining ICB with radiotherapy stems from the fact that radiation upregulates PD-L1, which leads to CD8+ T cell exhaustion. In addition, many tumors devoid of T cells at baseline (and secondary lack of PD-L1 expression on effector T cells) could benefit from the radiation-induced increase in PD-L1 and the combination (33). In the case of CTLA-4, upon radiation, it is recruited to the membrane of activated T cells and binds to the ligands CD80 and CD86, expressed on DC and other APC, thereby attenuating T cell activation (34).
Tumor burden has been regarded as a surrogate for ICB effectivity based on clinical observations that adjuvant ipilimumab in resected stage III melanomas obtains major benefits in recurrence-free survival and overall survival (48.3 and 65.4% at 5 years, respectively) (35), and locally advanced NSCLC treated with definitive chemoradiation followed by adjuvant durvalumab in the PACIFIC trial with an impressive prolongation of time to death or distant metastasis from 16.2 to 28.3 months and a favorable toxicity profile (36). Moreover, in patients that do respond to ICB, failure frequently occurs in sites of previous disease, with 60% of failures in anti-PD-1/PD-L1 treated NSCLC and 39% of failures in anti-PD-1 treated melanoma (37, 38). Although it is not specific criteria, the best outcome with ablative radiation in oligometastatic clinical trials has been obtained in patients with low tumor burden and as local consolidation (39, 40).
While the majority of clinical studies have targeted a single metastatic site, abscopal responses are relatively rare, and mainly in melanoma and NSCLC (41). Improved outcomes have been obtained in several phase 2 clinical trials using local consolidation with ablative doses of radiation in the oligometastatic setting (39, 40, 42) while ongoing phase 3 trials are investigating whether this approach may lead to improve overall survival in a subset of patients with limited metastatic disease (NCT02417662, NCT03137771, NCT02364557, NCT03862911, and NCT03721341).
It has been recently reported that tumor-resident CD8+ T cells play a significant role in mediating the immune effects of radiotherapy. Even if proliferation decreases after radiation, their functionality, measured as production of IFN-γ, augments, and mediates the early antitumoral effect of local SBRT doses. Nonetheless, as newly infiltrating CD8+ T cells play a key role in antitumor immunity, that may also be the case with radiation-induced immunogenicity (43). If radiation could increase the population of pro-immunogenic T cell subtypes within the local TME, it would enhance the response to ICB. This hypothesis raises the possibility that targeting multiple metastatic sites with SBRT to achieve complete cytoreduction in the metastatic setting may become clinically relevant (44). Moreover, the irradiation of each visible metastasis addresses the challenge of heterogeneity by attempting to convert each target into an in situ vaccine (45). Clinical support data comes from a phase 2 clinical trial in NSCLC with up to 4 metastatic sites (93% had <2 metastases), which underwent locally ablative treatment with metastasectomy or multi-site SBRT followed by pembrolizumab, with median survival of 19.1 months (vs. 6.6 months in historical controls) and favorable toxicity profile (46).
Research has been very controversial with variations in dose or fraction for radiation delivery in the metastatic setting, where the role of treatment parameters such as duration (more or <7 days), fraction size (1.8–3 to 8–30 Gy) and scheduling (single or multiple fractions) are largely unknown. While a short course (1–5 fractions) of high dose radiation can be safely administered and is able to elicit an immunogenic response that can benefit from the addition of ICB, the predominance of the immunosuppressive effects of radiation may limit the effectiveness of ablative doses of radiation, especially if single fractions are used (47, 48). Nonetheless, the immune context of the tumor type or even the metastatic organ may require a different dose and/or fractionation to elicit an immunogenic response. This possibility offers the potential to reduce the dose and volumes of radiation and still prove efficacious. In the PACIFIC trial (36), immunotherapy was administered sequentially (i.e., following chemoradiation) with a good toxicity profile but data on toxicity of concomitant radiation and immunotherapy in the clinical setting is scarce. Yet the biological context remains to be proven that would favor multiple rounds of high-end ablative dose schedules in oligometastatic patient as advocated by some groups (49). Another concept of potential clinical relevance that has been put forward is the possibility that the immunomulatory effect of low-dose radiation for stromal modulation could favor T cell infiltration and enhance the immune response (47, 50).
The next generation of clinical trials addressing radiotherapy-immunotherapy combinations will have to include immunological read-outs with proper endpoints for immune monitoring as well as the identification of immune biomarkers that optimize the selection of treatment strategies (31, 51).
Surgery and radiation remain strong curative modalities for treatment of established solid tumors but treatment failure continues to be a significant problem. The best established role of surgical oncology is the complete removal of the tumor, with an additional strong foundation to question the elective treatment of uninvolved regional lymph nodes in a large variety of tumor types and resection of metastatic disease which is increasingly offered to selected patients with indolent oligometastatic disease (52). Critical to all of them is securing negative surgical margins.
Less invasive technologies and advances in imaging leading to minimally invasive and robot-assisted surgeries are revolutionizing surgical care (5). Likewise, advanced image guidance and motion management strategies are shaping new therapeutic radiation strategies enabling the safe administration of ablative doses of radiation (2). Advanced imaging is fundamental and uniquely placed to serve both margin negativity rates and future radiation strategies.
Surgical margin positivity rate (cancer cells at the edge of tumor resection) has not significantly improved in recent decades and when it does occur prognosis is significantly affected in many tumor types. Margin positivity rates across all types of cancer range from 15 to 60% (53). A recent report on positive surgical margins in the ten most common solid cancers has identified oral cavity cancer with the highest rate with up to 25% of cases, no change over time, with significant effects on tumor relapse and overall survival. For advanced disease, the rates ranged between 20.9% (breast) and 65.5% (prostate) with related worse outcome in seven tumor types (54). Although not a true resistance type, we propose the term “margin-missing” effect to characterize this situation which leads to treatment failure and resistance.
Fluorescence-guided surgery, which allows intraoperative visualization of tumors, is an evolving image-guided surgical strategy to help differentiate tumor cells from normal surrounding tissues in real time. Near-infrared fluorescence imaging has a higher tumor to background ratio, high tissue penetration (5–10 mm), and little interference from intrinsic fluorescence. Indocyanine green is the most widely used probe in fluorescence-guided surgery although tumor detectability is not very good and optical technology is still evolving (55).
More than 50% of patients with cancer receive radiotherapy, which defines its leading role in cancer management, in particular for several locally advanced solid tumors. The latest developments in radiotherapy have swiftly enabled local dose escalation making it possible to deliver high doses of radiation with incredibly high anatomical precision and reduced risk of long-term adverse effects. As a consequence, relevant clinical benefit has been achieved in a variety of cancer types such as prostate, gynecologic, breast, head and neck cancers, and brain and lung metastases (2). However, no significant advance has occurred in the past 30 years in the development of strategies that enhance radiation effects. On the other hand, due to the recognition that the immune system can strongly contribute to therapeutic responses to radiation, radioimmunotherapy has become an intensive area of research.
The current challenge in near-infrared fluorescence-guided surgery is to design probes with high selectivity for tumors and clear visualization, referred to as smart probes, which are only activated at the tumor site (turn-on probes). There was a recent report about the design, synthesis, and characterization of three novel polymeric turn-on nanoprobes that are activated at the tumor site by cysteine cathepsins (highly expressed in multiple tumor types) showing a stable and well-defined signal from the tumor during the whole surgical procedure in orthotopic breast cancer and melanoma models resulting in less tumor recurrence and prolonged survival compared with standard commercial probes (56). This is a significant lead toward real-time image-guided tumor margin assessment during surgical oncology.
Emerging approaches seek to integrate analytical tools with optical technology to help improve the decision-making of fluorescence-guided surgery to reduce margin positivity rates. For example, combinations of fluorescence-guided surgery have been made with mass spectrometry (57), Raman spectroscopy (58), and hyper spectral imaging (59).
The most clinically advanced nanoprobe is LUM015 (a pegylated cathepsin-activatable probe) which is undergoing eight clinical trials, including a pivotal phase 3 study (60). The phase 3 trial is a multicenter study with the primary objective of assessing the ability of LUM015 and LUM fluorescence-guided surgery system to detect residual tumors in 250 breast cancer patients undergoing lumpectomy (NCT03686215).
It has been traditionally assumed that recurrent tumors arise from transformed neoplastic clones that are more resistant to oncological therapies, however, an early experience challenged this view and hypothesized that primary and recurrent tumors of equal size did have different microenvironments that explained their response to therapies. The study found that while small primary tumors had a healthy population of antitumor effector CD8+ T lymphocytes, recurrent tumors had an immunosuppressive condition consisting in expanded populations of tumor-associated macrophages (TAMs), Treg cells, and pro-tumoral cytokines that inhibited cytotoxic CD8+ T lymphocytes. These changes were also identified in regional draining lymph nodes. Disruption of these immunosuppressive pathways restored the efficacy of the tumor vaccine in recurrent tumors, as if they were primary tumors (61).
Research in preclinical models has shown that a syringeable immunomodulatory multidomain nanogel (iGel) containing gemcitabine, imiquimod, and clodronate locally applied as a postsurgical treatment is able to deplete immunosuppressive cells from the TME (myeloid-derived suppressor cells (MDSCs), M2 macrophages, and Treg cells), increase immunogenicity, and induce immunogenic cell death. Indeed, it generates systemic antitumor immunity and a memory T cell that significantly inhibits tumor recurrence and lung metastases. Reprogramming the immunosuppressive TME also converts tumors not responding to ICB to responding ones (62). This platform may serve to reshape immunosuppressive TME and synergize with other therapies.
Recent clinical data in melanoma and NSCLC have shown that response to ICB in individual patients with metastasis vary depending on the anatomical location of the metastasis, untangling the importance of the local TME in antitumor immunity. Of interest, tissue specific response to immune checkpoint inhibition depends on the cancer type, which implies that responsive and non-responsive sites are different among patients with NSCLC or melanoma (63, 64). These heterogeneous responses are an evident clinical problem, since patients with responses to ICB in all lesions survive longer than those with response in some of the lesions (65). Potential mechanisms include myeloid cell exclusion and alteration of T cell activation in response to tumor growth and local factors, but this will require unraveling a very complex network of interactions for differential responsiveness across different tissue sites of tumor deposits.
Treg cells are a small subset of circulating CD4+ T cells with potent suppressive functions with a central role in regulating immune responses and maintaining self-tolerance although they also impede antitumor immunity. In contrast with circulating Treg cells, intratumoral Treg cells maintain an active configuration, suggesting that antigen stimulation may play an important role in the activation and accumulation of Treg cells in the TME. The immunosuppression mediated by Treg cells is mainly mediated by the release of anti-inflammatory cytokines including IL-10 and transforming growth factor β (TGFβ), facilitating proliferation of CD4+ T cells to Treg cells, while suppressing proliferation to CD8+ T cells and NK cells. In addition, Treg cells can also reprogram macrophages to the M2 phenotype (via IL-4, IL-10, and IL-13) and favor MDSCs infiltration (via IL-10 and IL-35) (66).
Immunological cell death induced by radiation upregulates or releases DAMPs, including ATP, with further recruitment and activation of DC to initiate the antitumor immune response but ATP is rapidly catabolized in the TME into adenosine by the enzymes CD39 and CD73. Local accumulation of extracellular adenosine suppresses DC and CD8+ T cells and promotes proliferation of Treg cells, increases the expression of CTLA-4 and adenosine receptor A2 (A2AR) on Treg cells, and enhances the polarization of macrophages to the M2 phenotype. Radiation can also induce conversion of ATP to adenosine through the induction of reactive oxygen species (ROS) and TGFβ. Thus, targeting of A2AR, CD73, and TGFβ may reduce resistance to immunotherapy in the radiotherapy setting (33). Blockade of CD73 plus radiotherapy restored radiation-induced DC infiltration of tumors in a poor immunogenic setting, and the addition of CTLA-4 blockade improved control of non-irradiated lung metastases in murine models. These findings set the stage for clinical testing CD73 in patients who carry cGAS/STING tumors or show upregulation of soluble CD73 following radiotherapy to determine if CD73 blockade can enhance responses to ICB (67).
Treg cells also express PD-1 at a low level in the blood and at a high level in tumors, promoting the suppressive activity of PD-1-expressing Treg cells upon antibody-mediated PD-1 blockade (68). Recently reported, ~10% of cancer patients treated with anti-PD-1 antibody develop hyperprogressive disease, characterized by rapid cancer progression. Treg-specific depletion prior to, or combined with, an anti-PD-1 antibody may prevent hyperprogressive disease and enhance the effectiveness of anti-PD-1 therapy (69).
TAMs account for the largest fraction of the myeloid infiltrate in the majority of solid tumors. The tumor-associated macrophage compartment is highly dynamic in time (during tumor progression and response to treatment) and space (at different tumor sites) through an extensive remodeling of energy metabolism. In addition, the tumor-associated macrophage compartment is highly heterogeneous both within and across tumors in response to environmental changes ranging from a pro-inflammatory (M1) to an anti-inflammatory (M2) state. However, the M1/M2 phenotypes represent the extremes of a continuum and the plasticity of these cells makes therapeutic targeting challenging. Solid experimental evidence informs that the crosstalk between TAMs and the immune cells facilitates an immunosuppressive environment by supporting angiogenesis and extracellular matrix (ECM) remodeling, promoting active recruitment of Treg cells, and expression of PD-L1, paving the way for metastatic development (70). M2 polarization is mostly mediated by growth factors and cytokines secreted by cancer cells that reach M2 cells via exosomes (71). Intriguingly, ontogeny can influence the functional profile of TAMs, i.e., tissue-resident vs. circulating macrophages, such that they can have opposing functions depending on the tumor type (72). Based on these findings, it has been speculated that macrophage origins may be important in determining the permissiveness of an organ to metastatic growth.
Preliminary studies have evaluated the influence of radiation in macrophage polarization. Macrophages are highly radioresistant due to high production of anti-oxidative molecules such as manganese superoxide dismutase by a mechanism depending on tumor necrosis factor α (TNFα) signaling and nuclear factor-κβ (NFκβ) activation (73). Early studies established that radiation exposure recruited bone marrow-derived CD11b+ monocytes/macrophages to irradiated sites (74, 75) and related it to the transcription factor hypoxia-inducible factor-1α (HIF-1α) and effectors stromal cell-derived factor-1 (SDF-1) and C-X-C chemokine receptor type 4 (CXCR4) (76, 77). Therapy can polarize macrophages to the M2 phenotype with very high levels of proangiogenic molecules through the treatment-induced expression of colony stimulating factor 1 (CSF-1), the ligand for the colony stimulating factor 1 receptor (CSF-1R) on macrophages, which can be prevented by CSF-1R antagonists and enhance radiation effects (78, 79).
Ongoing research efforts are directed toward the alteration of the macrophage phenotype to attenuate immunosuppression and improve antitumor immunity (80). Current approaches aim to shift M2 cells to M1 by targeting secreted immunosuppressive factors released by cancer cells and cells in the TME (Figure 2).
Figure 2. Macrophage targeting in cancer. Macrophages are primarily recruited to tumors to acquire a pro-tumorigenic phenotype (M2 state). Several strategies target TAMs aiming to reprogram them into a pro-inflammatory phenotype (M1 state). Most macrophage-targeted therapies are focused on CSF-1R inhibitors. Another approach is via CXCR4 blockade, which acts on vasculogenesis and has been tested in the clinical setting after radiotherapy in glioblastoma.
Preclinical studies suggest that macrophage manipulation to avoid recruitment or prevent M2 polarization produce a significant enhancement of the radiation effect irregardless of the tumor model [reviewed in (81)]. The increase in radiosensitivity with this strategy has been attributed to blockade of vasculogenesis. If angiogenesis supports the formation of tumor blood vessels from the sprouting of local vessels, tumors can also develop or repair blood vessels from circulating proangiogenic cells mainly from the bone marrow, which is known as vasculogenesis (82). This effect could be exploited in radiation treatments, namely if the increase in hypoxia that occurs at the end of radiation through recruitment of circulating proangiogenic cells to rescue damaged tumor vasculature and promote tumor recurrence can be reversed. A first-in-human clinical trial of glioblastoma examined the effects of CXCR4 blockade through a 4-week continuous infusion of plerixafor, a small molecule CXCR4 inhibitor, at the end of irradiation in newly diagnosed glioblastoma and showed high efficacy and local control with an excellent median survival time of 21.3 months. Unexpectedly, a high proportion of patients had out-of-field recurrences with local tumor control which deserves further evaluation (83).
A relevant aspect that remains unanswered is whether the effect of blocking the CXCR4 pathway could be more pronounced with ablative doses of radiation which seems likely since greater vascular damage would be expected. Furthermore, it is of interest to know if CXCR4 blockade can enhance tumor immunity. Very limited information suggests that T cell exclusion from cancer cell deposits secondary to SDF-1 could be overcome by inhibiting the CXCR4 axis, improving the effect of checkpoint inhibitors or stroma normalizing strategies in pancreatic cancer (84, 85) and triple-negative breast cancer (86) models.
In addition to TAMs, radiation also recruits MDSCs in the irradiated tumors by tumor-secreted factors like SDF-1. MDSCs encompass a heterogeneous population of polymorphonuclear MDSCs and monocytic MDSCs which inhibit the activity of CD8+ T cells. Moreover, MDSCs play a prominent role in the establishment of the pre-metastatic niche, promote angiogenesis and facilitate the development of metastasis (87).
Tumors induce the formation of microenvironments in distant sites that support future metastatic tumor growth before their arrival at these sites, known as pre-metastatic niches. Tumor-secreted factors and tumor-shed extracellular vesicles promote a sequence of events that start with vascular leakiness, and are followed by alteration of local cells in the TME, recruitment of MDSCs, and finally attraction of circulating tumor cells (88). Following seeding in a secondary organ, cancer cells interact with their environment to create the metastatic niche. The microenvironment in pre-metastatic niches is immunosuppressive and MDSCs are the main cellular component, however, migration of MDSCs into pre-metastatic niches and subsequent activation is not well-characterized. More than 100 different immunosuppressive tumor-secreted proteins have been identified (89). Fibronectin accumulates and anchors to collagen in the ECM to facilitate the adherence of circulating tumor cells through high affinity of membrane integrins (90). The vascular changes allow for uptake of tumor-secreted exosomes by cancer-associated fibroblasts (CAFs) in the local stroma, which contributes to the formation of a tumor-associated desmoplastic stroma, characteristic of many carcinomas (91) (Figure 3). Exosomes are extracellular vesicles released by exocytosis and essential to intercellular communication. They can contain genetic material, proteins, and lipids; they can be found in all body fluids and are considered to be major drivers of pre-metastatic niche formation (92). Measurement of exosomal microRNA has been shown to accurately reflect tumor progression in several cancer types (93, 94) as well as dropping levels of exosomal microRNA after surgery indicate that the resected tumor was the main source of exosomal release (95). However, in animal models of abdominal cancer surgery can induce increased levels of ROS, that may downregulate tight junctions in the endothelium and peritoneum, form intercellular gaps and expose the underlying ECM; which can promote integrin-binding of circulating tumor cells during surgery, and result in an excess of liver metastases in a colorectal cancer model (96).
Figure 3. Role of the pre-metastatic niche in cancer metastasis. Primary tumor cells produce soluble factors and exosomes (A) to trigger the formation of an immature pre-metastatic niche in the target organ (B). Primary tumor conditions (hypoxia, acidity, and interstitial pressure) promote tumor cell migration into the blood vessels. Tumor-secreted factors and exosomes mobilize bone marrow-derived cells (such as CD11b+ myeloid cells) and suppressive immune cells (such as MDSCs, Treg, and TAMs) to target organs (C). Interactions with local stroma, hypoxia and active ECM remodeling may create a niche with suitable microenvironment conditions for tumor cell colonization (D). Surgery, inflammation, and immunosuppression may increase the number and survival of circulating tumor cells and favor the development of metastasis.
Research in animal models of breast cancer known to produce immunosuppressive MDSCs in the spleen and lungs, has shown that surgical resection of the primary tumor decreased levels of MDSCs in the spleen but persisted in the lungs for 2 weeks after resection, indicative of a pro-metastatic environment. Post-surgical treatment with gemcitabine depleted lung MDSCs and decreased posterior metastatic disease (97).
Accumulating evidence indicates that exposure to radiation induces the release of exosomes (98–100) that could contribute to radioresistance but additional mechanistic understanding to define potential interventions is lacking. The potential role of exosomes has also been explored as biomarkers of disease outcome in head and neck cancer patients treated with cetuximab, radiation, and ipilimumab; exosomes were isolated from plasma and the molecular cargo contents (derived from Treg cells) could separate patients who remained free 2 years after treatment from those who did not (101).
An important aspect required to characterize extracellular vesicles is the development of highly specific detection techniques. Since the distribution of extracellular vesicles in the TME depends on the cellular function, it is highly necessary to visualize them in freshly resected tissues. There was a recent report about an intraoperative optical imaging system that was able to provide rich details and molecular contrast thanks to a label-free multimodal nonlinear optical technology in human breast cancer showing good correlation with stained histological slides. The enriched areas with extracellular vesicles in the microenvironment correlated with macroscopic tissue deposits as well as increasing distance from tumor to margin (102).
A recent publication has shown that after surgical removal of resected primary lung, breast, and esophageal cancer, low-dose adjuvant therapy with epigenetic therapy can disable the pre-metastatic niche and inhibit the formation of lung metastases by avoiding the trafficking of MDSCs and promoting their differentiation into a macrophage-like phenotype (103). These preclinical findings represent a novel paradigm to be tested in clinical trials.
Fibroblasts, the major cell type in the TME, are critical determinants of cellular crosstalk (104). CAFs, a subpopulation of activated fibroblasts, are difficult to identify and in practice, are described as any mesenchymal cell that lacks lineage markers for epithelial cells, endothelial cells, and leukocytes. CAFs are proliferative, migratory, and highly secretory cells that promote extensive tissue remodeling which influences the physical and chemical properties of the tumor and increases the ECM stiffness, which promotes malignancy in experimental models. An extensive range of functions have also been attributed to CAFs, including secretion of growth factors, cytokines, and exosomes that promote tumor growth and alter treatment responses. The principal effect of CAFs is considered to be immunosuppressive with IL-6, SDF-1, and TGFβ as well-established mediators (105). These CAFs contribute to a rigid matrix that creates a physical barrier that leads to vessel compression and reduces diffusion of therapeutic agents to cancer cells which are particularly relevant for colorectal and pancreatic cancer (106, 107). CAFs are also effective in the remodeling of the tumor vasculature through the secretion of vascular endothelial growth factor (VEGF), fibroblast growth factor, and IL-6 to enhance angiogenesis (108, 109) (Figure 4).
Figure 4. Cancer-associated fibroblasts remodel the tumor stroma. The pro-tumorigenic functions of CAFs are generally associated with their highly secretory activity. Secretory functions and matrix remodeling contribute to tumor invasion and angiogenesis. In addition, secreted soluble factors also contribute to immune reprogramming and tumor growth. Metabolic remodeling by CAFs supports an immunosuppressive microenvironment and promotes tumor growth.
Emerging evidence implicates CAFs in immune escape and resistance to immunotherapy but not all subpopulations seem to have the same functions. A comprehensive identification of specific subsets of CAFs and their function is needed to become a viable targeting option (110). Currently, several preclinical strategies that target specific subsets of CAFs are under development (109).
Two promising strategies are normalization of activated CAFs, which intends to revert the activated state into a quiescent state or to induce them to acquire tumor-suppressor phenotypes (111), and targeting CAF-derived ECM proteins, either their production or degradation to alleviate the ECM stiffness (109). Reprogramming of CAFs to enhance immune responses, normalizing their ECM, is being investigated through the addition of vitamin D analogs (known to convert them into a quiescent state) to ICB in pancreatic cancer, and through TGFβ blockade combined with immune checkpoint inhibition in multiple tumor types (111).
Metabolic crosstalk across all cellular compartments is responsible for homeostasis and evolution of the TME. All cells of the TME, both malignant and non-malignant, compete for nutrients and oxygen, which are generally limited, especially in a stiffened and poorly vascularized TME, or secondary to the accumulation of the excessive production of metabolites by cancer cells. Additional aspects that influence how the TME reacts include immune-related substances released by cancer and/or immune cells, mechanical forces in the ECM, and reactions to treatment (112).
Although the metabolic pathways are shared between cellular compartments of the TME, the singularity of the reaction of stromal cells to energy demands is crucial. TAMs and CAFs are recruited to the tumor bed and activated in response to different stressful situations, such as limited nutrient disposal, hypoxia, and oxidative stress, attracted by cytokines such as TGFβ and CXCL2 or ROS from cancer cells [reviewed in (113)]. In such complex interactions, metabolites can serve different roles such as being a source of energy or communicate signals between different cellular compartments, and metabolism byproducts can favor an immunosuppressive phenotype. CAFs can rapidly adapt to these poor conditions through glycolysis and fatty acid oxidation in mitochondria. This increased consumption of glucose is coupled with extensive lactate secretion, which acidifies the TME and facilitates the activation of TAMs (114). The result of this swift metabolic adaptation of CAFs is the secretion of ECM-remodeling enzymes that promote fibrosis and further limit the availability of nutrients and oxygen, establishing a dynamic circuit in which lactate accumulation, glucose deprivation, and hypoxic conditions stimulate the recruitment and activation of additional stromal cells (113). Hypoxia supports the stabilization of the transcription factor HIF-1α to foster glycolysis. In this setting, HIF-1α also mediates CAF-secretion of proangiogenic factors such as VEGF, and hypoxia contributes to tumor progression by stimulating CAFs to secrete immunomodulatory molecules, growth factors, antioxidants, and ECM-remodeling enzymes. Taken together, the response of CAFs under poor nutritional conditions promotes tumor progression through engagement of endothelial cells. In addition, altered metabolism of cancer cells can create a gradient of metabolites around the tumor that can signal the distance to blood vessels and tailor the secretion of VEGF to match the tumor spatial organization and optimize the angiogenic response (115), and the metabolic switch in the TME may add to the disrupted immune cell metabolism (80).
Amino acids synthesize nucleotides and are also intermediate metabolites that contribute to other bioenergetic pathways. Glutamine is an abundant nutrient that provides carbon and nitrogen for pathways that contribute to energy formation, redox, homeostasis, macromolecular synthesis and signaling for cancer-cell growth of particular relevance in hypoxic conditions (116).
Endothelial cells form the lining of blood vessels and lymphatics and require glycolysis for proliferation and migration during angiogenesis. As the tumor grows, new vessels are required to supply the tumor with nutrients and oxygen and the endothelial cells increase their synthetic and energetic demands. Sprouting, the formation of new vessels, is a well-known mechanism in the angiogenic process and an area of intensive research. Although endothelial metabolism has been mainly described as glycolytic, recent findings suggest that mitochondrial oxidative phosphorylation is also required for endothelial cell proliferation during angiogenesis (117).
The interplay between metabolic remodeling and immune regulation in cancer is an active area of investigation. Preclinical models in glioblastoma have identified that tryptophan and adenosine metabolism result in accumulation of Treg cells and M2 macrophages, contributing to an immuno-suppressive phenotype. Future studies will need to define the role of the intermediary metabolites of these pathways to determine their therapeutic function (118).
Preclinical results with the prodrug JHU083, a glutamine antagonist that targets glutaminase and a broad range of glutamine-requiring enzymes, provide a strong and differentiated metabolic response in which cancer cells stop growth, through depletion of glutamine pathways and impairment of glucose uptake, and in addition stimulates T cell functionality, even with persistent antitumor memory (119). Disengaging the metabolism of cancer cells and that of T cells is an evolving therapeutic concept.
A link has been recently proposed between ECM stiffness and metabolic transformation that facilitates tumor progression. It was found that through metabolic crosstalk between CAFs and cancer cells, aspartate secreted by CAFs maintains cancer cell proliferation while glutamate secreted by cancer cells balances the redox state of CAFs to promote ECM remodeling. This amino acid exchange among glutamate and aspartate offers new targeting options for both stromal and cancer cells (120).
An abnormal vasculature is a paramount characteristic of solid tumors, with suboptimal function resulting from a leaky and immature vessel network (via overexpression of proangiogenic molecules such as VEGF), and compression of these anomalous vessels by physical forces (via TME cells and the ECM molecules they produce) (121). The resulting hypoxia enforces the stimulation of immune checkpoints and infiltration of immunosuppressive cells in the TME (122). Specifically, hypoxia up-regulates immune checkpoints, reprograms TAMs to an M2 state, may influence the efficacy of antigen presentation by DC, and affects the function of T cells, while hypoperfusion stiffens the TME that becomes a physical barrier to T cell infiltration into the tumor (123).
An emerging field of interest investigates the synergy of immune-vascular interactions to promote an antitumor effect (124). The objective of this strategy is to induce vascular normalization that needs to be coupled to vessel decompression (to avoid vessel collapse). Restoring vessel function by normalizing tumor stroma has been evaluated in preclinical models through targeting angiotensin signaling with anti-hypertensive agents (125) or inhibiting SDF-1/CXCR4 (86) which can target CAFs and collagen/hyaluronan to decompress tumor vessels and improve perfusion and effect of the ICB. Vascular normalization can be achieved with antiangiogenic agents to improve tumor perfusion and treatment delivery, but it is dose- and time-dependent, making outcome predictions for combinations of antiangiogenics, stroma normalization and immune therapies difficult to optimize (126) (Figure 5).
Figure 5. Strategies to improve tumor perfusion increase tumor immunogenicity. Angiogenesis, desmoplasia, and inflammation promote leaky and compressed tumor vessels. Vascular normalization strengthens the vessel wall reducing intercellular gaps and improving perfusion. Blood vessel decompression by depletion of CAFs or ECM reperfuses the vessel and augments perfusion. As a result, reprogramming of the TME to an immunomodulatory state enhances antitumor immunity.
Successful clinical evidence that the combination of ICB with antiangiogenic drugs has been recently reported in lung (127), renal (128, 129), and endometrial (130) cancer. However, the potential to improve the treatment outcome of this approach is under evaluation in an ongoing clinical trial, which tests the role of adding losartan (an antihypertensive angiotensin inhibitor) to chemo-radiation (delivered via SBRT) and nivolumab in pancreatic cancer patients (NCT03563248).
Apparently, any method that improves tumor perfusion is likely to enhance immunotherapy. It has been proposed that strategies that normalize the stroma would be more beneficial in tumors with abundant compressed vessels, while vascular normalization should improve perfusion in tumors with leaky vessels (131), and the combination when both co-exist. However, addressing the cause of hypoperfusion and identifying the normalization window for each tumor is challenging (126).
Since tumor perfusion is key for the efficacy of immunotherapy, perfusion markers could be used as markers for immunotherapy prediction (132).
While recent studies have improved our understanding of mechanisms supporting immune resistance, we still have an incomplete view of how the TME works as a whole. We propose that advancements in cancer metabolism and nanotechnology represent promising areas of research that have the potential to significantly improve our understanding of immune escape in nutrient- and oxygen-poor environments which may lead to opportunities for therapeutic intervention.
A comprehensive understanding of the metabolic needs of cancer cells has been achieved during this past decade. Significantly, metabolic signatures and hypoxia within the TME impact the immune function. The fact that these findings have been translated into actionable anticancer targets provides the basis for a metabolic characterization of the TME to identify novel targets and signatures in the future. Indeed, better technologies to investigate cancer metabolism at the single-cell level without disrupting the tissue will be required to achieve a deeper understanding of the role of metabolism in cancer.
Advancements in nanotechnology have been effectively developed in cancer therapy. Innovative nanomedicines can use the conditions and characteristics of the TME to deliver therapeutics with increased precision, while providing for signal outputs that allow to follow their effects in real time. Likewise, recent advances in nanotechnology have broadened opportunities for the development of radiosensitizers in synergy with other treatment modalities. We highlight recent progress of nanotechnology between radiotherapy and immunotherapy.
The complex interplay between cellular crosstalk, interactions in the ECM and the biochemical environment within a tumor has an impact on the metabolic phenotype and polarization of immune cells. Thus, the concerted actions of different immune subsets suppress or promote growth. Solid tumors have a dynamic oxygen supply with hypoxic regions where interactions among immune cells are not well understood. Untangling these interactions might offer new potential for response prediction. Tumor infiltrating lymphocytes are at a metabolic disadvantage within the TME since tumor cells impede their access to nutrients needed for activation and acidify the TME through lactate accumulation, favoring a Treg phenotype (133).
Targeting specific metabolic alterations shared by tumor cells and tumor promoting immune populations in the TME is a new strategy under evaluation. Preclinical research has focused on fatty acid metabolism as a source of metabolic plasticity in cancer cells (134), carbon metabolism to stimulate antitumor activity of macrophages (135), or targeting metabolism of ferroptosis (a form of death that relies on ROS) in tumors (136), among others. Strategies that reduce immunomodulatory metabolites are also under evaluation, which include altering the acidic microenvironment, blocking the thryptophan metabolism pathway, inhibition of adenosine within the TME (33), or avoiding lactate accumulation in the TME (137).
A coordinated approach, which takes into account tumor types and tumor biology with detailed molecular links between cancer genotypes and metabolic dependency in a longitudinal fashion, will be best suited to detect the patient populations that are most likely to benefit from metabolism-targeted therapies.
Nanoparticles (NP) have been increasingly studied for radiosensitization. The combination of hafnium oxide NP (NBTXR3, a high-Z nanomaterial with high-level electron density that increases energy dose deposit within cells) plus radiation vs. radiation alone has recently demonstrated meaningful clinical benefit in locally advanced soft tissue sarcoma by doubling pathologic response rates (16 vs. 8%) (138). Significantly, recent research has reported that radiation-activated hafnium oxide NP can augment tumor infiltrates of CD8+ T cells and generate an antitumor immune response, with systemic effect on untreated tumors on the same animals in a murine model of colon cancer (139).
Newly designed hafnium-based nanoscale metal-organic frameworks (nMOFs) have demonstrated effective radioenhancement for low-dose radiation in preclinical models. The combination of nMOF-mediated radiotherapy and PD-L1 blockade extended the local therapeutic effects of radiation to distant tumors via systemic antitumor immunity. This powerful platform can minimize toxic effects by lowering the administered dose of radiation; it can be redesigned for rational tuning and can significantly strengthen the effect of immunotherapy for treatment of non-immunogenic tumors (140, 141).
Evolution in the technological delivery of radiation and precision surgery parallels the rapid progress in immune biology that identifies novel strategies to enhance the antitumor immune response. In this setting, alterations in the TME could become especially relevant to optimize treatment immunogenicity and enhance patient outcome.
Defining the individual response of tumors to surgery and radiation offers the possibility to design innovative treatment strategies and re-adapt treatment to new emerging targets. This could have a major impact since it potentially represents a novel way to enhance local and systemic treatments.
The structure of the manuscript was compiled by all authors. SB and ML wrote the first draft. AS-G and SB assembled the figures. All authors reviewed and approved the final version of the manuscript.
This work was supported by grants from the Instituto de Salud Carlos III (ISCIII): PI15/01262 (ML) and CP03/00101 (ML), co-financed by the European Regional Fund (ERDF) and AECC (Spanish Association of Cancer Research) Founding Ref. GC16173720CARR (ML). AS-G was granted by VHIR fellowships.
The authors declare that the research was conducted in the absence of any commercial or financial relationships that could be construed as a potential conflict of interest.
4-1BB, tumor necrosis factor receptor superfamily member 9, CD137; A2AR, adenosine receptor A2; APC, antigen-presenting cells; ATM, ataxia telangiectasia mutated; ATP, adenosine triphosphate; CAFs, cancer-associated fibroblasts; CCL2, CC chemokine receptor 2; CD28, cluster of differentiation 28; CD39, cluster of differentiation 39; CD73, cluster of differentiation 73, ecto-5′-nucleotidase; CD80, cluster of differentiation 80; CD86, cluster of differentiation 86; cGAS, cyclic GMP-AMP synthase; CHK1, checkpoint kinase 1; CSF-1, colony stimulating factor 1; CSF-1R, colony stimulating factor 1 receptor; CTLA-4, CTL antigen 4; CXCL1, C-X-C motif chemokine ligand 1; CXCL2, C-X-C motif chemokine ligand 2; CXCL10, C-X-C motif chemokine ligand 10; CXCL16, C-X-C motif chemokine ligand 16; CXCR4, C-X-C chemokine receptor type 4; DAMPs, damage-associated molecular patterns; DC, dendritic cells; DDR, DNA damage response; DNA-PK, DNA-dependent protein kinase; ECM, extracellular matrix; HIF-1α, hypoxia-inducible factor-1α; HLA, human leukocyte antigen; HMGB1, high mobility group box 1; HSP90, heat shock protein 90; ICB, immune checkpoint blockade; IFN, interferon; IFN-γ, interferon gamma; IL-4, interleukin 4; IL-6, interleukin 6; IL-10, interleukin 10; IL-13, interleukin 13; IL-35, interleukin 35; irAEs, immune-related adverse events; KPNA2, karyopherin subunit alpha 2; LAG3, lymphocyte-activation gene-3; LUM, LUM imaging system (Lumicell Inc.); LUM015, cathepsin activatable fluorescent probe; MDSCs, myeloid-derived suppressor cells; MHC-I, major histocompatibility complex-I; NF-κβ, nuclear factor-kappa beta; NK, natural killer; nMOFs, nanoscale metal-organic frameworks; NP, nanoparticles; NSCLC, non-small cell lung cancer; OX-40, tumor necrosis factor receptor superfamily member 4, CD134; PARP, poly(ADP-ribose) polymerase; PD-1, programmed death-1; PD-L1, programmed death-ligand 1; ROS, reactive oxygen species; SBRT, stereotactic body radiotherapy; SDF-1, stromal cell-derived factor-1; SRS, stereotactic radiosurgery; STING, stimulator of interferon genes; TAMs, tumor-associated macrophages; TGFβ, transforming growth factor β; Th1, T helper type 1; Th2, T helper type 2; TIGIT, T cell immunoglobulin and ITIM domain; TIM-3, T cell immunoglobulin and mucin domain-3; TLR, toll-like receptors; TLR3, toll-like receptor 3; TLR7/8, toll-like receptors 7/8; TLR9, toll-like receptor 9; TMB, tumor mutational burden; TME, tumor microenvironment; TNFα, tumor necrosis factor α; Treg, regulatory T cells; Trex1, three-prime repair exonuclease 1; VEGF, vascular endothelial growth factor.
1. Markham MJ, Wachter K, Agarwal N, Bertagnolli MM, Chang SM, Dale W, et al. Clinical cancer advances 2020: annual report on progress against cancer from the American Society of Clinical Oncology. J Clin Oncol. (2020) 38:1081. doi: 10.1200/JCO.19.03141
2. Deutsch E, Chargari C, Galluzzi L, Kroemer G. Optimising efficacy and reducing toxicity of anticancer radioimmunotherapy. Lancet Oncol. (2019) 20:e452–e63. doi: 10.1016/S1470-2045(19)30171-8
3. Pitroda SP, Chmura SJ, Weichselbaum RR. Integration of radiotherapy and immunotherapy for treatment of oligometastases. Lancet Oncol. (2019) 20:e434–e42. doi: 10.1016/S1470-2045(19)30157-3
4. Menon H, Ramapriyan R, Cushman TR, Verma V, Kim HH, Schoenhals JE, et al. Role of radiation therapy in modulation of the tumor stroma and microenvironment. Front Immunol. (2019) 10:193. doi: 10.3389/fimmu.2019.00193
5. Kerr RS. Surgery in the 2020s: Implications of advancing technology for patients and the workforce. Future Healthc J. (2020) 7:46–9. doi: 10.7861/fhj.2020-0001
6. Havel JJ, Chowell D, Chan TA. The evolving landscape of biomarkers for checkpoint inhibitor immunotherapy. Nat Rev Cancer. (2019) 19:133–50. doi: 10.1038/s41568-019-0116-x
7. Galon J, Bruni D. Tumor immunology and tumor evolution: intertwined histories. Immunity. (2020) 52:55–81. doi: 10.1016/j.immuni.2019.12.018
8. Petitprez F, Meylan M, de Reyniès A, Sautès-Fridman C, Fridman WH. The tumor microenvironment in the response to immune checkpoint blockade therapies. Front Immunol. (2020) 11:784. doi: 10.3389/fimmu.2020.00784
9. Thorsson V, Gibbs DL, Brown SD, Wolf D, Bortone DS, Ou Yang T-H, et al. The immune landscape of cancer. Immunity. (2018) 48:812–830.e14. doi: 10.1016/j.immuni.2018.03.023
10. Lapuente-Santana Ó, Eduati F. Toward systems biomarkers of response to immune checkpoint blockers. Front Oncol. (2020) 10:1027. doi: 10.3389/fonc.2020.01027
11. Griss J, Bauer W, Wagner C, Simon M, Chen M, Grabmeier-Pfistershammer K, et al. B cells sustain inflammation and predict response to immune checkpoint blockade in human melanoma. Nat Commun. (2019) 10:4186. doi: 10.1038/s41467-019-12160-2
12. Helmink BA, Reddy SM, Gao J, Zhang S, Basar R, Thakur R, et al. B cells and tertiary lymphoid structures promote immunotherapy response. Nature. (2020) 577:549–555.
13. Demaria O, Cornen S, Daëron M, Morel Y, Medzhitov R, Vivier E. Harnessing innate immunity in cancer therapy. Nature. (2019) 574:45–56. doi: 10.1038/s41586-019-1593-5
14. Galon J, Bruni D. Approaches to treat immune hot, altered and cold tumours with combination immunotherapies. Nat Rev Drug Discov. (2019) 18:197–218. doi: 10.1038/s41573-018-0007-y
15. Dougan M, Pietropaolo M. Time to dissect the autoimmune etiology of cancer antibody immunotherapy. J Clin Invest. (2020) 130:51–61. doi: 10.1172/JCI131194
16. Akturk HK, Alkanani A, Zhao Z, Yu L, Michels AW. PD-1 inhibitor immune-related adverse events in patients with preexisting endocrine autoimmunity. J Clin Endocrinol Metab. (2018) 103:3589–92. doi: 10.1210/jc.2018-01430
17. Khan Z, Di Nucci F, Kwan A, Hammer C, Mariathasan S, Rouilly V, et al. Polygenic risk for skin autoimmunity impacts immune checkpoint blockade in bladder cancer. Proc Natl Acad Sci U S A. (2020) 117:12288–94. doi: 10.1073/pnas.1922867117
18. Champiat S, Ferrara R, Massard C, Besse B, Marabelle A, Soria J-C, et al. Hyperprogressive disease: recognizing a novel pattern to improve patient management. Nat Rev Clin Oncol. (2018) 15:748–62. doi: 10.1038/s41571-018-0111-2
19. Kas B, Talbot H, Ferrara R, Richard C, Lamarque J-P, Pitre-Champagnat S, et al. Clarification of definitions of hyperprogressive disease during immunotherapy for non-small cell lung cancer. JAMA Oncol. (2020) 6:1039. doi: 10.1001/jamaoncol.2020.1634
20. Chen P-L, Roh W, Reuben A, Cooper ZA, Spencer CN, Prieto PA, et al. Analysis of immune signatures in longitudinal tumor samples yields insight into biomarkers of response and mechanisms of resistance to immune checkpoint blockade. Cancer Discov. (2016) 6:827–37. doi: 10.1158/2159-8290.CD-15-1545
21. Amaria RN, Reddy SM, Tawbi HA, Davies MA, Ross MI, Glitza IC, et al. Neoadjuvant immune checkpoint blockade in high-risk resectable melanoma. Nat Med. (2018) 24:1649–54. doi: 10.1038/s41591-018-0197-1
22. Yost KE, Satpathy AT, Wells DK, Qi Y, Wang C, Kageyama R, et al. Clonal replacement of tumor-specific T cells following PD-1 blockade. Nat Med. (2019) 25:1251–9. doi: 10.1038/s41591-019-0522-3
23. Balkwill FR, Capasso M, Hagemann T. The tumor microenvironment at a glance. J Cell Sci. (2012) 125:5591–6. doi: 10.1242/jcs.116392
24. Joyce JA, Fearon DT. T cell exclusion, immune privilege, and the tumor microenvironment. Science. (2015) 348:74–80. doi: 10.1126/science.aaa6204
25. Stylianopoulos T, Munn LL, Jain RK. Reengineering the physical microenvironment of tumors to improve drug delivery and efficacy: from mathematical modeling to bench to bedside. Trends Cancer. (2018) 4:292–319. doi: 10.1016/j.trecan.2018.02.005
26. Lhuillier C, Rudqvist N-P, Elemento O, Formenti SC, Demaria S. Radiation therapy and anti-tumor immunity: exposing immunogenic mutations to the immune system. Genome Med. (2019) 11:40. doi: 10.1186/s13073-019-0653-7
27. McLaughlin M, Patin EC, Pedersen M, Wilkins A, Dillon MT, Melcher AA, et al. Inflammatory microenvironment remodelling by tumour cells after radiotherapy. Nat Rev Cancer. (2020) 20:203–217. doi: 10.1038/s41568-020-0246-1
28. Formenti SC, Rudqvist N-P, Golden E, Cooper B, Wennerberg E, Lhuillier C, et al. Radiotherapy induces responses of lung cancer to CTLA-4 blockade. Nat Med. (2018) 24:1845–51. doi: 10.1038/s41591-018-0232-2
29. Blair TC, Bambina S, Alice AF, Kramer GF, Medler TR, Baird JR, et al. Dendritic cell maturation defines immunological responsiveness of tumors to radiation therapy. J Immunol. (2020) 204:3416–24. doi: 10.4049/jimmunol.2000194
30. Walshaw RC, Honeychurch J, Choudhury A, Illidge TM. Toll-like receptor agonists and radiation therapy combinations: an untapped opportunity to induce anticancer immunity and improve tumor control. Int J Radiat Oncol. (2020) 108:27–37. doi: 10.1016/j.ijrobp.2020.04.020
31. Golden EB, Marciscano AE, Formenti SC. Radiation and in-situ tumor vaccination. Int J Radiat Oncol. (inpress) S0360301620341122. doi: 10.1016/j.ijrobp.2020.08.023
32. Sharma P, Hu-Lieskovan S, Wargo JA, Ribas A. Primary, adaptive, and acquired resistance to cancer immunotherapy. Cell. (2017) 168:707–23. doi: 10.1016/j.cell.2017.01.017
33. Darragh LB, Oweida AJ, Karam SD. overcoming resistance to combination radiation-immunotherapy: a focus on contributing pathways within the tumor microenvironment. Front Immunol. (2019) 9:3154. doi: 10.3389/fimmu.2018.03154
34. Demaria S, Kawashima N, Yang AM, Devitt ML, Babb JS, Allison JP, et al. Immune-mediated inhibition of metastases after treatment with local radiation and CTLA-4 blockade in a mouse model of breast cancer. Clin Cancer Res Off J Am Assoc Cancer Res. (2005) 11:728–734.
35. Eggermont AMM, Chiarion-Sileni V, Grob J-J, Dummer R, Wolchok JD, Schmidt H, et al. Prolonged survival in stage III melanoma with ipilimumab adjuvant therapy. N Engl J Med. (2016) 375:1845–55. doi: 10.1056/NEJMoa1611299
36. Antonia SJ, Villegas A, Daniel D, Vicente D, Murakami S, Hui R, et al. Overall survival with durvalumab after chemoradiotherapy in stage III NSCLC. N Engl J Med. (2018) 379:2342–50. doi: 10.1056/NEJMoa1809697
37. Shah S, Wood K, Labadie B, Won B, Brisson R, Karrison T, et al. Clinical and molecular features of innate and acquired resistance to anti-PD-1/PD-L1 therapy in lung cancer. Oncotarget. (2018) 9:4375–84. doi: 10.18632/oncotarget.23315
38. Wang DY, Eroglu Z, Ozgun A, Leger PD, Zhao S, Ye F, et al. Clinical features of acquired resistance to anti-PD-1 therapy in advanced melanoma. Cancer Immunol Res. (2017) 5:357–62. doi: 10.1158/2326-6066.CIR-16-0287
39. Palma DA, Olson R, Harrow S, Gaede S, Louie AV, Haasbeek C, et al. Stereotactic ablative radiotherapy for the comprehensive treatment of oligometastatic cancers: long-term results of the SABR-COMET phase II randomized trial. J Clin Oncol. (2020) 38:2830–8. doi: 10.1101/2020.03.26.20044305
40. Gomez DR, Tang C, Zhang J, Blumenschein GR, Hernandez M, Lee JJ, et al. Local consolidative therapy vs. maintenance therapy or observation for patients with oligometastatic non-small-cell lung cancer: long-term results of a multi-institutional, phase II, randomized study. J Clin Oncol. (2019) 37:1558–65. doi: 10.1200/JCO.19.00201
41. Brooks ED, Chang JY. Time to abandon single-site irradiation for inducing abscopal effects. Nat Rev Clin Oncol. (2019) 16:123–35. doi: 10.1038/s41571-018-0119-7
42. Phillips R, Shi WY, Deek M, Radwan N, Lim SJ, Antonarakis ES, et al. Outcomes of observation vs stereotactic ablative radiation for oligometastatic prostate cancer: the ORIOLE phase 2 randomized clinical trial. JAMA Oncol. (2020) 6:650. doi: 10.1001/jamaoncol.2020.0147
43. Arina A, Beckett M, Fernandez C, Zheng W, Pitroda S, Chmura SJ, et al. Tumor-reprogrammed resident T cells resist radiation to control tumors. Nat Commun. (2019) 10:3959. doi: 10.1038/s41467-019-11906-2
44. Gutiontov SI, Pitroda SP, Chmura SJ, Arina A, Weichselbaum RR. Cytoreduction and the optimization of immune checkpoint inhibition with radiation therapy. Int J Radiat Oncol. (2020) 108:17–26. doi: 10.1016/j.ijrobp.2019.12.033
45. Formenti SC, Demaria S. Future of radiation and immunotherapy. Int J Radiat Oncol. (2020) 108:3–5. doi: 10.1016/j.ijrobp.2020.04.034
46. Bauml JM, Mick R, Ciunci C, Aggarwal C, Davis C, Evans T, et al. Pembrolizumab after completion of locally ablative therapy for oligometastatic non-small cell lung cancer: a phase 2 trial. JAMA Oncol. (2019) 5:1283. doi: 10.1001/jamaoncol.2019.1449
47. Savage T, Pandey S, Guha C. Postablation modulation after single high-dose radiation therapy improves tumor control via enhanced immunomodulation. Clin Cancer Res. (2020) 26:910–21. doi: 10.1158/1078-0432.CCR-18-3518
48. Patel RR, Verma V, Barsoumian H, Ning MS, Chun SG, Tang C, et al. Use of multi-site radiation therapy as systemic therapy: a new treatment approach personalized by patient immune status. Int J Radiat Oncol. (2020) S0360301620341146. doi: 10.1016/j.ijrobp.2020.08.025. [Epub ahead of print].
49. Greco C, Fuks Z. Forging new strategies in the cure of human oligometastatic cancer. JAMA Oncol. (2020) 6:659. doi: 10.1001/jamaoncol.2020.0195
50. Menon H, Chen D, Ramapriyan R, Verma V, Barsoumian HB, Cushman TR, et al. Influence of low-dose radiation on abscopal responses in patients receiving high-dose radiation and immunotherapy. J Immunother Cancer. (2019) 7:237. doi: 10.1186/s40425-019-0718-6
51. Chargari C, Levy A, Paoletti X, Soria J-C, Massard C, Weichselbaum RR, et al. Methodological development of combination drug and radiotherapy in basic and clinical research. Clin Cancer Res. (2020) 26:4723–36. doi: 10.1158/1078-0432.CCR-19-4155
52. Coit DG. Society of Surgical Oncology Presidential Address: Seattle, WA, USA, March 17, 2017: the future ain't what it used to be. Ann Surg Oncol. (2017) 24:2055–64. doi: 10.1245/s10434-017-5892-5
53. Nagaya T, Nakamura YA, Choyke PL, Kobayashi H. Fluorescence-guided surgery. Front Oncol. (2017) 7:314. doi: 10.3389/fonc.2017.00314
54. Orosco RK, Tapia VJ, Califano JA, Clary B, Cohen EEW, Kane C, et al. Positive surgical margins in the 10 most common solid cancers. Sci Rep. (2018) 8:5686. doi: 10.1038/s41598-018-23403-5
55. Zheng Y, Yang H, Wang H, Kang K, Zhang W, Ma G, et al. Fluorescence-guided surgery in cancer treatment: current status and future perspectives. Ann Transl Med. (2019) 7:S6. doi: 10.21037/atm.2019.01.26
56. Blau R, Epshtein Y, Pisarevsky E, Tiram G, Dangoor SI, Yeini E, et al. Image-guided surgery using near-infrared Turn-ON fluorescent nanoprobes for precise detection of tumor margins. Theranostics. (2018) 8:3437–60. doi: 10.7150/thno.23853
57. Kröger S, Niehoff A-C, Jeibmann A, Sperling M, Paulus W, Stummer W, et al. Complementary molecular and elemental mass-spectrometric imaging of human brain tumors resected by fluorescence-guided surgery. Anal Chem. (2018) 90:12253–60. doi: 10.1021/acs.analchem.8b03516
58. Santos IP, Barroso EM, Bakker Schut TC, Caspers PJ, van Lanschot CGF, Choi D-H, et al. Raman spectroscopy for cancer detection and cancer surgery guidance: translation to the clinics. Analyst. (2017) 142:3025–47. doi: 10.1039/C7AN00957G
59. Kho E, de Boer LL, Van de Vijver KK, van Duijnhoven F, Vrancken Peeters M-JTFD, Sterenborg HJCM, et al. Hyperspectral imaging for resection margin assessment during cancer surgery. Clin Cancer Res. (2019) 25:3572–80. doi: 10.1158/1078-0432.CCR-18-2089
60. Wojtynek NE, Mohs AM. Image-guided tumor surgery: the emerging role of nanotechnology. WIREs Nanomed Nanobiotechnol. (2020) 12:e1624. doi: 10.1002/wnan.1624
61. Predina J, Eruslanov E, Judy B, Kapoor V, Cheng G, Wang L-C, et al. Changes in the local tumor microenvironment in recurrent cancers may explain the failure of vaccines after surgery. Proc Natl Acad Sci U S A. (2013) 110:E415–24. doi: 10.1073/pnas.1211850110
62. Song C, Phuengkham H, Kim YS, Dinh VV, Lee I, Shin IW, et al. Syringeable immunotherapeutic nanogel reshapes tumor microenvironment and prevents tumor metastasis and recurrence. Nat Commun. (2019) 10:3745. doi: 10.1038/s41467-019-11730-8
63. Schmid S, Diem S, Li Q, Krapf M, Flatz L, Leschka S, et al. Organ-specific response to nivolumab in patients with non-small cell lung cancer (NSCLC). Cancer Immunol Immunother. (2018) 67:1825–32. doi: 10.1007/s00262-018-2239-4
64. Lee JHJ, Lyle M, Menzies AM, Chan MMK, Lo S, Clements A, et al. Metastasis-specific patterns of response and progression with anti-PD-1 treatment in metastatic melanoma. Pigment Cell Melanoma Res. (2018) 31:404–10. doi: 10.1111/pcmr.12675
65. Gauci M-L, Lanoy E, Champiat S, Caramella C, Ammari S, Aspeslagh S, et al. Long-term survival in patients responding to anti-PD-1/PD-L1 therapy and disease outcome upon treatment discontinuation. Clin Cancer Res. (2019) 25:946–56. doi: 10.1158/1078-0432.CCR-18-0793
66. Ashrafizadeh M, Farhood B, Eleojo Musa A, Taeb S, Najafi M. The interactions and communications in tumor resistance to radiotherapy: therapy perspectives. Int Immunopharmacol. (2020) 87:106807. doi: 10.1016/j.intimp.2020.106807
67. Wennerberg E, Spada S, Rudqvist N-P, Lhuillier C, Gruber S, Chen Q, et al. CD73 blockade promotes dendritic cell infiltration of irradiated tumors and tumor rejection. Cancer Immunol Res. (2020) 8:465–78. doi: 10.1158/2326-6066.CIR-19-0449
68. Wei SC, Levine JH, Cogdill AP, Zhao Y, Anang N-AAS, Andrews MC, et al. Distinct cellular mechanisms underlie anti-CTLA-4 and anti-PD-1 checkpoint blockade. Cell. (2017) 170:1120–33.e17. doi: 10.1016/j.cell.2017.07.024
69. Kamada T, Togashi Y, Tay C, Ha D, Sasaki A, Nakamura Y, et al. PD-1 + regulatory T cells amplified by PD-1 blockade promote hyperprogression of cancer. Proc Natl Acad Sci U S A. (2019) 116:9999–10008. doi: 10.1073/pnas.1822001116
70. Engblom C, Pfirschke C, Pittet MJ. The role of myeloid cells in cancer therapies. Nat Rev Cancer. (2016) 16:447–62. doi: 10.1038/nrc.2016.54
71. Wang X, Luo G, Zhang K, Cao J, Huang C, Jiang T, et al. Hypoxic tumor-derived exosomal miR-301a mediates M2 macrophage polarization via PTEN/PI3Kγ to promote pancreatic cancer metastasis. Cancer Res. (2018) 78:4586–98. doi: 10.1158/0008-5472.CAN-17-3841
72. Zhu Y, Herndon JM, Sojka DK, Kim K-W, Knolhoff BL, Zuo C, et al. Tissue-resident macrophages in pancreatic ductal adenocarcinoma originate from embryonic hematopoiesis and promote tumor progression. Immunity. (2017) 47:323–38.e6. doi: 10.1016/j.immuni.2017.07.014
73. Tsai C-S, Chen F-H, Wang C-C, Huang H-L, Jung S-M, Wu C-J, et al. Macrophages from irradiated tumors express higher levels of iNOS, arginase-I and COX-2, and promote tumor growth. Int J Radiat Oncol. (2007) 68:499–507. doi: 10.1016/j.ijrobp.2007.01.041
74. Kozin SV, Kamoun WS, Huang Y, Dawson MR, Jain RK, Duda DG. Recruitment of myeloid but not endothelial precursor cells facilitates tumor regrowth after local irradiation. Cancer Res. (2010) 70:5679–85. doi: 10.1158/0008-5472.CAN-09-4446
75. Lecavalier-Barsoum M, Chaudary N, Han K, Pintilie M, Hill RP, Milosevic M. Targeting CXCL12/CXCR4 and myeloid cells to improve the therapeutic ratio in patient-derived cervical cancer models treated with radio-chemotherapy. Br J Cancer. (2019) 121:249–56. doi: 10.1038/s41416-019-0497-3
76. Ceradini DJ, Kulkarni AR, Callaghan MJ, Tepper OM, Bastidas N, Kleinman ME, et al. Progenitor cell trafficking is regulated by hypoxic gradients through HIF-1 induction of SDF-1. Nat Med. (2004) 10:858–64. doi: 10.1038/nm1075
77. Jin DK, Shido K, Kopp H-G, Petit I, Shmelkov SV, Young LM, et al. Cytokine-mediated deployment of SDF-1 induces revascularization through recruitment of CXCR4+ hemangiocytes. Nat Med. (2006) 12:557–67. doi: 10.1038/nm1400
78. Escamilla J, Schokrpur S, Liu C, Priceman SJ, Moughon D, Jiang Z, et al. CSF1 Receptor targeting in prostate cancer reverses macrophage-mediated resistance to androgen blockade therapy. Cancer Res. (2015) 75:950–62. doi: 10.1158/0008-5472.CAN-14-0992
79. Stafford JH, Hirai T, Deng L, Chernikova SB, Urata K, West BL, et al. Colony stimulating factor 1 receptor inhibition delays recurrence of glioblastoma after radiation by altering myeloid cell recruitment and polarization. Neuro-Oncol. (2016) 18:797–806. doi: 10.1093/neuonc/nov272
80. Binnewies M, Roberts EW, Kersten K, Chan V, Fearon DF, Merad M, et al. Understanding the tumor immune microenvironment (TIME) for effective therapy. Nat Med. (2018) 24:541–50. doi: 10.1038/s41591-018-0014-x
81. Brown JM. Radiation damage to tumor vasculature initiates a program that promotes tumor recurrences. Int J Radiat Oncol. (2020) 108:734–44. doi: 10.1016/j.ijrobp.2020.05.028
82. Okubo M, Kioi M, Nakashima H, Sugiura K, Mitsudo K, Aoki I, et al. M2-polarized macrophages contribute to neovasculogenesis, leading to relapse of oral cancer following radiation. Sci Rep. (2016) 6:27548. doi: 10.1038/srep27548
83. Thomas RP, Nagpal S, Iv M, Soltys SG, Bertrand S, Pelpola JS, et al. Macrophage exclusion after radiation therapy (MERT): a first in human phase I/II trial using a CXCR4 inhibitor in glioblastoma. Clin Cancer Res. (2019) 25:6948–57. doi: 10.1158/1078-0432.CCR-19-1421
84. Feig C, Jones JO, Kraman M, Wells RJB, Deonarine A, Chan DS, et al. Targeting CXCL12 from FAP-expressing carcinoma-associated fibroblasts synergizes with anti-PD-L1 immunotherapy in pancreatic cancer. Proc Natl Acad Sci U S A. (2013) 110:20212–7. doi: 10.1073/pnas.1320318110
85. Blair AB, Kim VM, Muth ST, Saung MT, Lokker N, Blouw B, et al. Dissecting the stromal signaling and regulation of myeloid cells and memory effector T cells in pancreatic cancer. Clin Cancer Res. (2019) 25:5351–63. doi: 10.1158/1078-0432.CCR-18-4192
86. Chen IX, Chauhan VP, Posada J, Ng MR, Wu MW, Adstamongkonkul P, et al. Blocking CXCR4 alleviates desmoplasia, increases T-lymphocyte infiltration, and improves immunotherapy in metastatic breast cancer. Proc Natl Acad Sci U S A. (2019) 116:4558–66. doi: 10.1073/pnas.1815515116
87. Safarzadeh E, Orangi M, Mohammadi H, Babaie F, Baradaran B. Myeloid-derived suppressor cells: important contributors to tumor progression and metastasis. J Cell Physiol. (2018) 233:3024–36. doi: 10.1002/jcp.26075
88. Peinado H, Zhang H, Matei IR, Costa-Silva B, Hoshino A, Rodrigues G, et al. Pre-metastatic niches: organ-specific homes for metastases. Nat Rev Cancer. (2017) 17:302–17. doi: 10.1038/nrc.2017.6
89. Kano A. Tumor cell secretion of soluble factor(s) for specific immunosuppression. Sci Rep. (2015) 5:8913. doi: 10.1038/srep08913
90. Barbazán J, Alonso-Alconada L, Elkhatib N, Geraldo S, Gurchenkov V, Glentis A, et al. Liver metastasis is facilitated by the adherence of circulating tumor cells to vascular fibronectin deposits. Cancer Res. (2017) 77:3431–41. doi: 10.1158/0008-5472.CAN-16-1917
91. Hoshino A, Costa-Silva B, Shen T-L, Rodrigues G, Hashimoto A, Tesic Mark M, et al. Tumour exosome integrins determine organotropic metastasis. Nature. (2015) 527:329–35. doi: 10.1038/nature15756
92. Rajagopal C, Harikumar KB. The origin and functions of exosomes in cancer. Front Oncol. (2018) 8:66. doi: 10.3389/fonc.2018.00066
93. Liu Q, Yu Z, Yuan S, Xie W, Li C, Hu Z, et al. Circulating exosomal microRNAs as prognostic biomarkers for non-small-cell lung cancer. Oncotarget. (2017) 8:13048–58. doi: 10.18632/oncotarget.14369
94. Tokuhisa M, Ichikawa Y, Kosaka N, Ochiya T, Yashiro M, Hirakawa K, et al. Exosomal miRNAs from peritoneum lavage fluid as potential prognostic biomarkers of peritoneal metastasis in gastric cancer. PLOS ONE. (2015) 10:e0130472. doi: 10.1371/journal.pone.0130472
95. Shao N, Xue L, Wang R, Luo K, Zhi F, Lan Q. miR-454-3p is an exosomal biomarker and functions as a tumor suppressor in glioma. Mol Cancer Ther. (2019) 18:459–69. doi: 10.1158/1535-7163.MCT-18-0725
96. van der Bij GJ, Oosterling SJ, Bögels M, Bhoelan F, Fluitsma DM, Beelen RHJ, et al. Blocking α2 integrins on rat CC531s colon carcinoma cells prevents operation-induced augmentation of liver metastases outgrowth. Hepatology. (2007) 47:532–43. doi: 10.1002/hep.22013
97. Bosiljcic M, Cederberg RA, Hamilton MJ, LePard NE, Harbourne BT, Collier JL, et al. Targeting myeloid-derived suppressor cells in combination with primary mammary tumor resection reduces metastatic growth in the lungs. Breast Cancer Res. (2019) 21:103. doi: 10.1186/s13058-019-1189-x
98. Al-Mayah A, Bright S, Chapman K, Irons S, Luo P, Carter D, et al. The non-targeted effects of radiation are perpetuated by exosomes. Mutat Res Mol Mech Mutagen. (2015) 772:38–45. doi: 10.1016/j.mrfmmm.2014.12.007
99. Jelonek K, Wojakowska A, Marczak L, Muer A, Tinhofer-Keilholz I, Lysek-Gladysinska M, et al. Ionizing radiation affects protein composition of exosomes secreted in vitro from head and neck squamous cell carcinoma. Acta Biochim Pol. (2015) 62:265–72. doi: 10.18388/abp.2015_970
100. Mrowczynski OD, Madhankumar AB, Sundstrom JM, Zhao Y, Kawasawa YI, Slagle-Webb B, et al. Exosomes impact survival to radiation exposure in cell line models of nervous system cancer. Oncotarget. (2018) 9:36083–101. doi: 10.18632/oncotarget.26300
101. Theodoraki M-N, Yerneni S, Gooding WE, Ohr J, Clump DA, Bauman JE, et al. Circulating exosomes measure responses to therapy in head and neck cancer patients treated with cetuximab, ipilimumab, and IMRT. OncoImmunology. (2019) 8:e1593805. doi: 10.1080/2162402X.2019.1593805
102. Sun Y, You S, Tu H, Spillman DR, Chaney EJ, Marjanovic M, et al. Intraoperative visualization of the tumor microenvironment and quantification of extracellular vesicles by label-free nonlinear imaging. Sci Adv. (2018) 4:eaau5603. doi: 10.1126/sciadv.aau5603
103. Lu Z, Zou J, Li S, Topper MJ, Tao Y, Zhang H, et al. Epigenetic therapy inhibits metastases by disrupting premetastatic niches. Nature. (2020) 579:284–290. doi: 10.1038/s41586-020-2054-x
104. Yamaguchi H, Sakai R. Direct interaction between carcinoma cells and cancer associated fibroblasts for the regulation of cancer invasion. Cancers. (2015) 7:2054–62. doi: 10.3390/cancers7040876
105. Sahai E, Astsaturov I, Cukierman E, DeNardo DG, Egeblad M, Evans RM, et al. A framework for advancing our understanding of cancer-associated fibroblasts. Nat Rev Cancer. (2020) 20:174–86. doi: 10.1038/s41568-019-0238-1
106. Bauer J, Emon MAB, Staudacher JJ, Thomas AL, Zessner-Spitzenberg J, Mancinelli G, et al. Increased stiffness of the tumor microenvironment in colon cancer stimulates cancer associated fibroblast-mediated prometastatic activin A signaling. Sci Rep. (2020) 10:50. doi: 10.1038/s41598-020-64239-2
107. Porter RL, Magnus NKC, Thapar V, Morris R, Szabolcs A, Neyaz A, et al. Epithelial to mesenchymal plasticity and differential response to therapies in pancreatic ductal adenocarcinoma. Proc Natl Acad Sci U S A. (2019) 116:26835–45. doi: 10.1073/pnas.1914915116
108. Kalluri R. The biology and function of fibroblasts in cancer. Nat Rev Cancer. (2016) 16:582–98. doi: 10.1038/nrc.2016.73
109. Chen X, Song E. Turning foes to friends: targeting cancer-associated fibroblasts. Nat Rev Drug Discov. (2019) 18:99–115. doi: 10.1038/s41573-018-0004-1
110. Wong PF, Wei W, Gupta S, Smithy JW, Zelterman D, Kluger HM, et al. Multiplex quantitative analysis of cancer-associated fibroblasts and immunotherapy outcome in metastatic melanoma. J Immunother Cancer. (2019) 7:194. doi: 10.1186/s40425-019-0675-0
111. De Jaeghere EA, Denys HG, De Wever O. Fibroblasts fuel immune escape in the tumor microenvironment. Trends Cancer. (2019) 5:704–23. doi: 10.1016/j.trecan.2019.09.009
112. Casey SC, Amedei A, Aquilano K, Azmi AS, Benencia F, Bhakta D, et al. Cancer prevention and therapy through the modulation of the tumor microenvironment. Semin Cancer Biol. (2015) 35(Suppl):S199–S223. doi: 10.1016/j.semcancer.2015.02.007
113. Vitale I, Manic G, Galassi C, Galluzzi L. Stress responses in stromal cells and tumor homeostasis. Pharmacol Ther. (2019) 200:55–68. doi: 10.1016/j.pharmthera.2019.04.004
114. Avnet S, Di Pompo G, Chano T, Errani C, Ibrahim-Hashim A, Gillies RJ, et al. Cancer-associated mesenchymal stroma fosters the stemness of osteosarcoma cells in response to intratumoral acidosis via NF-κB activation: tumor acidic microenvironment fosters osteosarcoma stemness via mesenchymal stroma. Int J Cancer. (2017) 140:1331–45. doi: 10.1002/ijc.30540
115. Carmona-Fontaine C, Deforet M, Akkari L, Thompson CB, Joyce JA, Xavier JB. Metabolic origins of spatial organization in the tumor microenvironment. Proc Natl Acad Sci U S A. (2017) 114:2934–9. doi: 10.1073/pnas.1700600114
116. Hensley CT, Wasti AT, DeBerardinis RJ. Glutamine and cancer: cell biology, physiology, and clinical opportunities. J Clin Invest. (2013) 123:3678–84. doi: 10.1172/JCI69600
117. Diebold LP, Gil HJ, Gao P, Martinez CA, Weinberg SE, Chandel NS. Mitochondrial complex III is necessary for endothelial cell proliferation during angiogenesis. Nat Metab. (2019) 1:158–71. doi: 10.1038/s42255-018-0011-x
118. Kesarwani P, Prabhu A, Kant S, Chinnaiyan P. Metabolic remodeling contributes towards an immune-suppressive phenotype in glioblastoma. Cancer Immunol Immunother. (2019) 68:1107–20. doi: 10.1007/s00262-019-02347-3
119. Leone RD, Zhao L, Englert JM, Sun I-M, Oh M-H, Sun I-H, et al. Glutamine blockade induces divergent metabolic programs to overcome tumor immune evasion. Science. (2019) 366:1013–21. doi: 10.1126/science.aav2588
120. Bertero T, Oldham WM, Grasset EM, Bourget I, Boulter E, Pisano S, et al. Tumor-stroma mechanics coordinate amino acid availability to sustain tumor growth and malignancy. Cell Metab. (2019) 29:124–40.e10. doi: 10.1016/j.cmet.2018.09.012
121. Noman MZ, Hasmim M, Messai Y, Terry S, Kieda C, Janji B, et al. Hypoxia: a key player in antitumor immune response. A review in the theme: cellular responses to hypoxia. Am J Physiol Cell Physiol. (2015) 309:C569–C79. doi: 10.1152/ajpcell.00207.2015
122. Jain RK. Antiangiogenesis strategies revisited: from starving tumors to alleviating hypoxia. Cancer Cell. (2014) 26:605–22. doi: 10.1016/j.ccell.2014.10.006
123. Mariathasan S, Turley SJ, Nickles D, Castiglioni A, Yuen K, Wang Y, et al. TGFβ attenuates tumour response to PD-L1 blockade by contributing to exclusion of T cells. Nature. (2018) 554:544–8. doi: 10.1038/nature25501
124. Fukumura D, Kloepper J, Amoozgar Z, Duda DG, Jain RK. Enhancing cancer immunotherapy using antiangiogenics: opportunities and challenges. Nat Rev Clin Oncol. (2018) 15:325–40. doi: 10.1038/nrclinonc.2018.29
125. Pinter M, Jain RK. Targeting the renin-angiotensin system to improve cancer treatment: Implications for immunotherapy. Sci Transl Med. (2017) 9:eaan5616. doi: 10.1126/scitranslmed.aan5616
126. Mpekris F, Voutouri C, Baish JW, Duda DG, Munn LL, Stylianopoulos T, et al. Combining microenvironment normalization strategies to improve cancer immunotherapy. Proc Natl Acad Sci U S A. (2020) 117:3728–37. doi: 10.1073/pnas.1919764117
127. Socinski MA, Jotte RM, Cappuzzo F, Orlandi F, Stroyakovskiy D, Nogami N, et al. Atezolizumab for first-line treatment of metastatic nonsquamous NSCLC. N Engl J Med. (2018) 378:2288–301. doi: 10.1056/NEJMoa1716948
128. Rini BI, Plimack ER, Stus V, Gafanov R, Hawkins R, Nosov D, et al. Pembrolizumab plus axitinib versus sunitinib for advanced renal-cell carcinoma. N Engl J Med. (2019) 380:1116–27. doi: 10.1056/NEJMoa1816714
129. Motzer RJ, Penkov K, Haanen J, Rini B, Albiges L, Campbell MT, et al. Avelumab plus axitinib versus sunitinib for advanced renal-cell carcinoma. N Engl J Med. (2019) 380:1103–15. doi: 10.1056/NEJMoa1816047
130. Makker V, Rasco D, Vogelzang NJ, Brose MS, Cohn AL, Mier J, et al. Lenvatinib plus pembrolizumab in patients with advanced endometrial cancer: an interim analysis of a multicentre, open-label, single-arm, phase 2 trial. Lancet Oncol. (2019) 20:711–8. doi: 10.1016/S1470-2045(19)30020-8
131. Stylianopoulos T, Jain RK. Combining two strategies to improve perfusion and drug delivery in solid tumors. Proc Natl Acad Sci U S A. (2013) 110:18632–7. doi: 10.1073/pnas.1318415110
132. Martin JD, Seano G, Jain RK. Normalizing function of tumor vessels: progress, opportunities, and challenges. Annu Rev Physiol. (2019) 81:505–34. doi: 10.1146/annurev-physiol-020518-114700
133. Kouidhi S, Ben Ayed F, Benammar Elgaaied A. Targeting tumor metabolism: a new challenge to improve immunotherapy. Front Immunol. (2018) 9:353. doi: 10.3389/fimmu.2018.00353
134. Vriens K, Christen S, Parik S, Broekaert D, Yoshinaga K, Talebi A, et al. Evidence for an alternative fatty acid desaturation pathway increasing cancer plasticity. Nature. (2019) 566:403–6. doi: 10.1038/s41586-019-0904-1
135. Liu M, O'Connor RS, Trefely S, Graham K, Snyder NW, Beatty GL. Metabolic rewiring of macrophages by CpG potentiates clearance of cancer cells and overcomes tumor-expressed CD47–mediated ‘don't-eat-me’ signal. Nat Immunol. (2019) 20:265–75. doi: 10.1038/s41590-018-0292-y
136. Wang W, Green M, Choi JE, Gijón M, Kennedy PD, Johnson JK, et al. CD8+ T cells regulate tumour ferroptosis during cancer immunotherapy. Nature. (2019) 569:270–4. doi: 10.1038/s41586-019-1170-y
137. Goliwas KF, Deshane JS, Elmets CA, Athar M. Moving immune therapy forward targeting TME. Physiol Rev. (2020). doi: 10.1152/physrev.00008.2020. [Epub ahead of print].
138. Bonvalot S, Rutkowski PL, Thariat J, Carrère S, Ducassou A, Sunyach M-P, et al. NBTXR3, a first-in-class radioenhancer hafnium oxide nanoparticle, plus radiotherapy versus radiotherapy alone in patients with locally advanced soft-tissue sarcoma (Act.In.Sarc): a multicentre, phase 2-3, randomised, controlled trial. Lancet Oncol. (2019) 20:1148–59. doi: 10.1016/S1470-2045(19)30326-2
139. Zhang P, Darmon A, Marill J, Mohamed Anesary N, Paris S. Radiotherapy-activated hafnium oxide nanoparticles produce abscopal effect in a mouse colorectal cancer model. Int J Nanomed. (2020) 15:3843–50. doi: 10.2147/IJN.S250490
140. Ni K, Lan G, Chan C, Quigley B, Lu K, Aung T, et al. Nanoscale metal-organic frameworks enhance radiotherapy to potentiate checkpoint blockade immunotherapy. Nat Commun. (2018) 9:2351. doi: 10.1038/s41467-018-04703-w
Keywords: radiotherapy, immunotherapy, tumor microenvironment, surgery, cancer
Citation: Benavente S, Sánchez-García A, Naches S, LLeonart ME and Lorente J (2020) Therapy-Induced Modulation of the Tumor Microenvironment: New Opportunities for Cancer Therapies. Front. Oncol. 10:582884. doi: 10.3389/fonc.2020.582884
Received: 13 July 2020; Accepted: 16 September 2020;
Published: 23 October 2020.
Edited by:
Chris Albanese, Georgetown University, United StatesReviewed by:
Walter J. Storkus, University of Pittsburgh, United StatesCopyright © 2020 Benavente, Sánchez-García, Naches, LLeonart and Lorente. This is an open-access article distributed under the terms of the Creative Commons Attribution License (CC BY). The use, distribution or reproduction in other forums is permitted, provided the original author(s) and the copyright owner(s) are credited and that the original publication in this journal is cited, in accordance with accepted academic practice. No use, distribution or reproduction is permitted which does not comply with these terms.
*Correspondence: Sergi Benavente, c2JlbmF2ZW50ZUB2aGVicm9uLm5ldA==
Disclaimer: All claims expressed in this article are solely those of the authors and do not necessarily represent those of their affiliated organizations, or those of the publisher, the editors and the reviewers. Any product that may be evaluated in this article or claim that may be made by its manufacturer is not guaranteed or endorsed by the publisher.
Research integrity at Frontiers
Learn more about the work of our research integrity team to safeguard the quality of each article we publish.