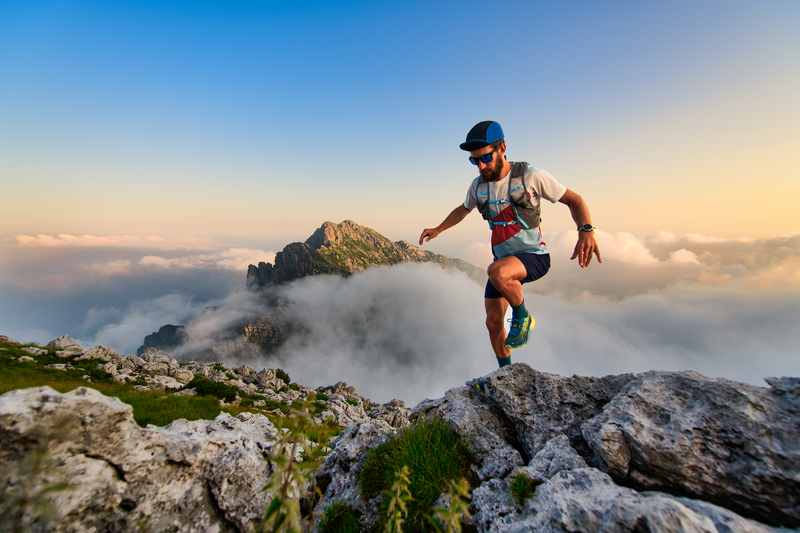
94% of researchers rate our articles as excellent or good
Learn more about the work of our research integrity team to safeguard the quality of each article we publish.
Find out more
REVIEW article
Front. Oncol. , 05 August 2020
Sec. Pharmacology of Anti-Cancer Drugs
Volume 10 - 2020 | https://doi.org/10.3389/fonc.2020.01389
The oncogene MDMX, also known as MDM4 is a critical negative regulator of the tumor suppressor p53 and has been implicated in the initiation and progression of human cancers. Increasing evidence indicates that MDMX is often amplified and highly expressed in human cancers, promotes cancer cell growth, and inhibits apoptosis by dampening p53-mediated transcription of its target genes. Inhibiting MDMX-p53 interaction has been found to be effective for restoring the tumor suppressor activity of p53. Therefore, MDMX is becoming one of the most promising molecular targets for developing anticancer therapeutics. In the present review, we mainly focus on the current MDMX-targeting strategies and known MDMX inhibitors, as well as their mechanisms of action and in vitro and in vivo anticancer activities. We also propose other potential targeting strategies for developing more specific and effective MDMX inhibitors for cancer therapy.
In 1996, Shvarts et al. discovered a new binding protein of the tumor suppressor p53, which is highly homologous to a critical negative regulator of p53, i.e., the oncoprotein MDM2 (murine double minute 2) (1). Therefore, this new p53-associated protein was named MDMX (murine double minute X, also called MDM4) (1, 2). Unsurprisingly, MDMX shows functional similarity to MDM2, which binds to p53 and inhibits its transcriptional activity (3, 4). MDM2 has been identified as an E3 ubiquitin ligase to degrade p53 (5–7). Different from MDM2, MDMX does not have the E3 ligase activity and cannot directly promote the ubiquitination and degradation of p53 (8). Although it has been established that MDMX inhibits p53-mediated transactivation, regardless of MDM2 status, MDMX had been considered as a “backup” regulator of p53 for a while (9). Due to the findings that MDMX is essential for controlling p53 activity during embryonic development in vivo (10–12), as well as that MDMX directly interacts with MDM2 and plays an important role in enhancing MDM2's activity and stabilizing MDM2 protein (13, 14), MDMX started to be recognized as a key player in the p53-MDM2/MDMX circuitry (15–18).
The p53-MDM2 pathway has been extensively investigated during the past three decades and has been found to play critical roles in cancer initiation and progression (19–21). Because the dual functions of MDMX in the regulation of MDM2 and p53 are not redundant but essential in cancer cells, much more attention has been paid to investigate the roles of MDMX in human cancer. Recent studies have indicated that MDMX is frequently amplified and overexpressed in various types of human cancer and contributes to the development and progression of this disease. Therefore, MDMX has been demonstrated as a promising but underutilized molecular target for cancer therapy. Over the past few years, there have been several review articles on the roles of MDMX in cancer and development (15–18, 22–24), MDMX isoforms (25), inhibitors of p53-MDM2/MDMX interactions (26–29), as well as mouse modeling of MDMX (12). In the present review, we will discuss the MDMX protein structure and the role of MDMX in the p53-MDM2/MDMX loop but mainly focus on the clinical relevance of MDMX in cancer, current MDMX-targeting strategies and related MDMX inhibitors, and other potential strategies for developing new MDMX inhibitors.
The MDMX protein consists of 490 amino acids with four main conserved domains, including the N-terminal p53-binding domain, the central acidic domain, the zinc-finger domain, and the C-terminal RING domain (Figure 1) (15, 22). The inhibitory effect of MDMX on p53-activated transcription depends on the p53-binding domain, which is strictly conserved on both MDM2 and MDMX proteins (30, 31). MDMX directly binds to the N-terminal transactivation domains (TAD) of p53 through the p53-binding domain, thereby inhibiting the transcriptional activity of p53. It has been found that MDM2 works collaboratively with MDMX to repress the transcriptional activity of p53 (32). Okamoto et al. have demonstrated that serine 367 on MDMX is critical for its binding to 14-3-3 as well as for the cooperative repression on p53 activation by MDM2 and MDMX (33). Alanine substitution at serine 367 not only blocks the binding of MDMX to 14-3-3 but also enhances cooperative inhibition of p53's transcriptional activity by MDM2 and MDMX (33). Importantly, the precise amino acids that are essential for the interactions of the p53 TAD with both MDM2 and MDMX are identical (30), leading to the development of dual inhibitors of p53-MDM2/MDMX interactions (26–29). However, the MDM2- and MDMX-binding pockets for p53 are not the same, despite the high resemblance between them (31), which results in the differences in the binding selectivity of small-molecule inhibitors to MDM2 and MDMX (34).
Figure 1. The structure of MDMX protein. MDMX has four conserved domains, including an N-terminal p53-binding domain, the central acidic domain, the zinc-finger domain, and the C-terminal RING domain. MDMX also has an autoinhibitory sequence element, i.e., WWW motif, which inhibits the MDMX-p53 interaction. The C-terminal tail (residues 485–490) of MDMX is also important for the formation of MDM2-MDMX heterodimer.
MDMX protein also contains a central acidic domain as MDM2. It has previously been reported that MDM2 inhibits the interaction between p53 and DNA via the acidic domain whereas MDMX only slightly mitigates this interaction (35). However, a recent study by Huang et al. has revealed that the MDMX acidic domain inhibits the p53-DNA binding and complements the role of MDM2 in controlling p53 level in vivo (36). Besides, the MDMX acidic domain also plays a role in the intramolecular interactions with the p53-binding domain and disrupts the p53-MDMX interaction (37, 38). The zinc-finger domain is also conserved in MDMX protein and has been found to interact with retinoblastoma protein (Rb); however, its function is yet to be determined (39). Besides, there is an MDMX autoinhibitory sequence motif, i.e., the WWW element located in a tryptophan-rich segment and centered around residues Trp200 and Trp201 in MDMX (40). This conserved WWW motif binds to MDMX's N-terminal domain and prevents its interaction with p53, while MDMX without this autoinhibitory element binds 32-fold more tightly to p53 than the full-length MDMX (40).
The C-terminal RING domain of MDMX has a 53.2% sequence identity with that of MDM2 and this RING domain is critical for the dimerization of MDM2 and MDMX proteins (41, 42). Importantly, seven amino acid residues (485–491) at the extreme C terminus plays a crucial role in the interactions among the RING domains (43, 44). The MDM2-MDMX heterodimerization is much more stable than the homodimerization of either protein (41). The RING domain of MDM2 has sufficient E3 ubiquitin ligase activity, whereas MDMX does not have the intrinsic E3 ligase function (42, 45). Although MDMX does not directly promote the ubiquitination and degradation of p53, this protein also influences the levels of both p53 and MDM2 by interacting with MDM2 (13). The C-terminal tail of MDMX can substitute for that of MDM2 and reactivate the E3 ligase function of MDM2 protein with mutated C-terminal domain, suggesting that MDMX can actively modulate MDM2's E3 activity and the C-terminal tail of MDMX plays an essential role in the cooperativity of MDMX in MDM2 ubiquitin ligase activity (43, 44). It has been found that the RING domain-mediated interaction between MDM2 and MDMX not only increases the steady-state level of MDM2 but also enhances its E3 ligase activity (13, 46). Indeed, the MDM2-MDMX hetero-RING complexes have a greater E3 ligase activity than the MDM2 homo-RING complexes, which emphasizes the importance of the MDM2-MDMX heterodimer in p53 control (46).
The p53-MDM2 feedback loop has been comprehensively discussed in previous review articles (9, 22, 47, 48). In brief, MDM2 is a transcriptional target and a negative regulator of p53 (Figure 2). MDM2 directly binds to p53 TAD and inhibits p53-mediated transcription (49, 50); it also promotes p53 polyubiquitination and proteasomal degradation (5–7). Conversely, p53 specifically binds to the MDM2 P2 promoter and activates its transcription, thus forming an autoregulatory feedback loop (51).
Figure 2. The role of MDMX in p53-MDM2/MDMX Loop. MDMX directly binds to p53 transactivation domains through its N-terminal p53-binding domain and inhibits p53-mediated transcription. MDMX also interacts with MDM2 via their RING domains and forms MDM2-MDMX heterodimer, increasing MDM2 protein stability and enhancing MDM2-mediated MDMX protein ubiquitination and degradation. Besides, the MDM2-MDMX complex shows better inhibitory effects on p53 than MDM2 alone, repressing p53-mediated transcription and promoting p53 ubiquitination and proteasomal degradation.
Accumulating evidence suggests that MDMX plays a pivotal role in the regulation of MDM2 and p53, thereby forming the p53-MDM2/MDMX loop (Figure 2). As mentioned above, MDMX inactivates p53 through interacting with p53 and repressing its transcriptional activity, but it does not directly affect p53 protein stability (1, 2, 52). The MDMX phosphorylation by casein kinase 1α (CK1α) is required for the MDMX-p53 interaction as well as MDMX-mediated p53 inactivation (53). Besides, MDMX is a p53 target gene with a weaker p53-inducible P2 promoter (54). Furthermore, MDMX interacts with MDM2 through their respective RING domains and forms heterodimers, thereby enhancing E3 ubiquitin ligase activity of MDM2 and promoting MDM2-mediated p53 ubiquitination (46). However, upon DNA damage, MDMX interacts with p53 and induces a conformational change of p53 protein toward an active form, protecting p53 from proteasomal degradation in an MDM2- and ubiquitin-independent manner, whereas MDM2 lacks this activity (55). Interestingly, the formation of the MDM2-MDMX complex not only increases the stability of MDM2 but also causes the degradation of MDMX (13). The MDM2-MDMX heterodimer also functions as a Nedd8 ligase and promotes the neddylation of p53 (56). Therefore, MDMX not only can directly interact with p53 and inhibit its activity but also can bind to MDM2 and redirect its ubiquitin ligase activity toward p53 and MDMX itself.
It has been frequently observed that MDMX is amplified and/or overexpressed in various types of human cancer (as summarized in Table 1). Riemenschneider et al. have firstly reported the aberrant expression of MDMX in malignant gliomas, including glioblastoma (4%) and anaplastic oligodendroglioma (4%) (57). They have found that the malignant gliomas harboring MDMX amplification and overexpression have neither p53 mutation nor MDM2 amplification, supporting the role of MDMX in helping gliomas escape from p53-dependent growth control (57, 58). Similar results have been obtained in other studies (59, 60). However, no association has been found between the aberrant expression of MDMX and the survival of patients with glioblastoma (60). Danovi et al. have further shown that MDMX is overexpressed in breast (19%), lung (18%), and colon (19%) cancer and MDMX amplification (5%) is responsible for MDMX overexpression in breast cancer (61). The amplification and overexpression of MDMX in breast cancer have also been confirmed in Nottingham/Tenovus cohort with a well-characterized series of 990 cases and other studies and have been associated with p53 mutation, breast carcinogenesis, invasive disease, small tumor size, low grade, longer breast carcinoma specific survival (BCSS), and disease-free survival (DFS) (62–65). However, MDMX amplification is rare (1.4%) in colon cancer and its functional significance in this disease still needs further investigations (66). Mancini et al. have found that the expression of full-length MDMX (MDMX-FL), but not its oncogenic MDMX-S variant is significantly associated with the responses of ovarian cancer patients to chemotherapy (67).
The overexpression of MDMX has been found in 36.6% gastric cancer and contributes to lymph node metastasis (68, 69). Although MDMX overexpression can influence the prognosis of gastric cancer, it cannot be used as an independent prognostic factor for this disease (69). The amplification and overexpression of MDMX have also been observed in bladder cancer and has been implicated in high-grade and invasive disease (70). Interestingly, MDMX amplification is significantly associated with low recurrence risk in bladder cancer and predicts better recurrence-free survival of patients with this disease (71). Besides, the amplification and high-level expression of MDMX has been reported in subtypes of liver cancer, i.e., hepatoblastoma and fibrolamellar hepatocellular carcinoma, but the clinical relevance of these findings in liver cancer is not yet clear (72, 73).
MDMX amplification has been reported in both soft tissue sarcomas (17%) and osteosarcomas (35%) (74–77). Bartel et al. have found that MDMX amplification is significantly associated with the poor prognosis of soft tissue sarcomas (74). It has further been found that the MDMX splice variant MDMX-S is overexpressed and the ratio of the transcript levels of MDMX-S over MDMX-FL (the MDMX-S/MDMX-FL ratio) is increased and correlated with high tumor grade and poor overall survival (74). Lenos et al. have shown an increased copy number of MDMX in 71% osteosarcomas, which is correlated to poor response to chemotherapy (78). Further studies have indicated that the high MDMX-S/MDMX-FL ratio in osteosarcomas is positively associated with the low MDMX protein expression, rapid metastatic progression, and poor overall survival of this disease (78). The overexpression of both MDMX-FL and MDMX-S has been found in chronic lymphocytic leukemia (CLL) (79), acute myeloid leukemia (80), mantle cell lymphoma (81), and pediatric Burkitt lymphoma (82). Liu et al. have further found a significant increase in the expression of MDMX-FL and MDMX-S in CLL patients with p53 deletion and/or mutation (79). Importantly, the high expression level of MDMX-S mRNA has been associated with the short treatment-free survival (TFS) of CLL patients (79).
Genomic aberration of MDMX has also been observed in salivary gland cancer (SGC) and has been correlated with the poor outcomes of patients with this disease (83). Ach et al. have found that MDMX is amplified in 8.1% salivary gland carcinomas and significantly associated with high-grade malignancy, lymph node metastasis, advanced tumor size and tumor stage, and poor overall survival of SGC patients (83). Interestingly, Prodosmo et al. have reported the MDMX-S overexpression and a highly significant downregulation of MDMX mRNA in papillary thyroid carcinomas, which has been correlated with the multifocality of tumors and advanced tumor stage (84). In addition, MDMX overexpression has been observed in melanomas (65%) (85), head and neck squamous carcinomas (50%) (86), and retinoblastoma (10%) (87). Several studies on The Cancer Genome Atlas (TCGA) project have reported that SNP rs4245739 in the 3' UTR of the MDMX gene is associated with the risk of hormonally mediated cancers (88–91). However, the precise mechanisms and clinical significance should be further investigated.
There is a lot of evidence indicating that cancer often upregulates the expression of MDMX to dampen the tumor-suppressor function of p53. Inhibiting MDMX helps restore the tumor suppressor activity of p53 as well as repress the oncogenic function of MDM2. Therefore, MDMX has recently been demonstrated as a promising therapeutic target for cancer therapy (15). Several MDMX-targeting strategies (Figure 3) have been proposed to develop MDMX inhibitors, including (1) directly blocking p53-MDMX interaction, (2) inhibiting MDMX expression, and (3) inducing MDMX protein degradation. Here we summarize the MDMX inhibitors (Table 2) in recent years and describe their in vitro and in vivo activities and mechanisms of action.
Figure 3. MDMX-targeting strategies and representative MDMX inhibitors. Currently, three MDMX-targeting strategies have been used to develop MDMX inhibitors, including (1) blocking p53-MDMX interaction or p53-MDM2/MDMX interactions to activate p53, (2) inhibiting MDMX expression at multiple levels, and (3) inducing MDMX protein degradation by increasing MDM2-MDMX binding and/or enhancing MDMX ubiquitination.
Due to advances in understanding the structure and binding mechanisms of p53-MDM2 and p53-MDMX complexes, many inhibitors have been developed to dually inhibit the interactions of p53-MDM2/MDMX, which have been comprehensively discussed in the recent review articles (26–29). Several specific p53-MDMX binding inhibitors, including, but not limited to, SAH-p53-8 (92), SJ-172550 (94), compound B1 (96), CTX1 (97), and K-178 (98) have recently been discovered and shown promising anticancer efficacy and safety profiles in preclinical models in vitro and in vivo (Table 2). Bernal et al. have designed and synthesized a stapled p53 helix, named SAH-p53-8 that specifically binds to MDMX with strong affinity (KD = 2.3 nM) (92). SAH-p53-8 has further been shown to block the p53-MDMX binding and activate the p53 signaling pathway, thus inhibiting cancer cell viability, inducing apoptosis, and overcoming MDMX-mediated cancer chemoresistance in vitro and in vivo. Hoppmann and Wang have designed and synthesized covalent peptide inhibitors of p53-MDMX binding based on the structure of SAH-p53-8, leading to the discovery of mSF-SAH (93). Although mSF-SAH has shown more efficient inhibition on the p53-MDMX interaction than SAH-p53-8, the anticancer efficacy of this new peptide is yet to be determined.
Reed et al. have performed a high-throughput screening of the St. Jude chemical library (containing 295,848 unique compounds) by using a fluorescence polarization (FP)-based MDMX-p53 binding assay as well as a cell-based assay and identified 11 representative compounds (94). Among these 11 compounds, SJ-134433 and SJ-044557 have been found to covalently modify MDMX and thus be abandoned for further investigations, whereas SJ-172550 has been shown to reversibly bind to the p53-binding pocket of MDMX, block the p53-MDMX binding, activate wild-type p53, and induce apoptosis in retinoblastoma cells with MDMX overexpression (94). However, the same group has also found that SJ-172550 covalently but reversibly interacts with MDMX and causes a conformational change of this protein, which consequently loses the ability to bind p53 (95). This complicated binding mechanism of SJ-172550 hinders its further evaluation as an MDMX inhibitor (95). Boltjes et al. have recently generated a peptidomimetic MDMX inhibitor, named compound B1 and examined its inhibitory effects on MDMX-p53 binding (Ki = 5.5 μM) using the FP-based assay. However, the anticancer efficacy of compound B1 has yet to be determined (96).
Karan et al. have also performed a cell-based screening of a compound library (over 20,000 small molecules) and identified a lead compound CTX1, which induces strong p53-dependent activation in MCF-7 cells expressing a high level of MDMX (97). Further studies have shown that CTX1 directly binds to MDMX (KD = 450 nM), prevents the formation of p53-MDMX complex, and activates p53 without causing DNA damage (97). CTX1 alone or in combination with an MDM2 inhibitor nutlin-3 has been found to induce cancer cell growth arrest and apoptosis in vitro and extend the survival of mice bearing acute myeloid leukemia (AML) xenograft tumors in vivo (97). In addition, Uesato et al. have designed and synthesized a class of o-aminothiophenol derivatives as selective p53-MDMX binding inhibitors. The lead compound K-178 has been shown to activate the p53 signaling and inhibit the viability of cancer cell lines with wild-type p53 and overexpressing MDMX, including human breast (MCF-7), lung (A427), and colon (HCT116) cancer cell lines. It has also been demonstrated that K-178 suppresses tumor growth in an HCT116 xenograft mouse model without affecting the average mouse body weight during the treatment period (98). Further studies on both CTX1 and K-178 in more clinically relevant cancer models are needed for the further development of these MDMX inhibitors as anticancer drugs.
Several dual inhibitors of the p53-MDM2/MDMX interactions have been identified and shown promising efficacy in vitro and in vivo. Optimization of p53 stapled peptides has been considered as a reasonable strategy to discover effective p53 activators, which leads to the identification of ATSP-7041 and ALRN-6924 (99, 100). Chang et al. have reported that ATSP-7041 directly binds to both MDM2 and MDMX with high affinities (Ki = 0.9 and 7 nM, respectively) and effectively disrupts the p53-MDM2/MDMX bindings, thereby activating p53 signaling in p53 wild-type cancer cells. ATSP-7041 has been found to induce cell cycle arrest in G1 and G2/M phase and apoptosis in vitro and suppress xenograft tumor growth in vivo in a p53-dependent manner (99). Importantly, ATSP-7041 has shown favorable DMPK and tissue distribution properties, which are critical for the further development of this peptide as an anticancer drug. Moreover, Carvajal et al. have identified a stapled α-helical peptide ALRN-6924 as a dual p53-MDM2/MDMX inhibitor, which has recently entered phase I clinical trial (100). ALRN-6924 has also shown nanomolar binding affinities to both MDM2 and MDMX (Ki = 1.3 and 7 nM, respectively). It has further been found that ALRN-6924 inhibits the p53-MDM2/MDMX interactions and activates p53, thus inhibiting cell proliferation and clonogenic capacity and causing cell cycle arrest and apoptosis in AML cells harboring wild-type p53 (100). ALRN-6924 has also shown robust anti-leukemic efficacy in primary patient-derived AML cells and AML xenograft mouse models. Besides, protoporphyrin IX (PpIX), a metabolite of the clinically approved drug aminolevulinic acid has recently been characterized as a dual inhibitor of the p53-MDM2/MDMX interactions (101). Jiang et al. have found that PpIX inhibits cell proliferation and promotes apoptosis in B-cell chronic lymphocytic leukemia cells without significant effects on normal cells. The PpIX-induced apoptosis in leukemia cells has been attributed to the stabilization of p53 and TAp73 proteins by the compound (101).
Direct inhibition of MDMX expression has been demonstrated as an effective approach for p53 activation. Several small-molecule inhibitors have recently been discovered to down-regulate MDMX expression and up-regulate the expression of p53 and its target genes, e.g., p21. Wang et al. have carried out a promoter-based screening of NCI Diversity-Set chemical library and identified a lead compound, XI-006 (NSC207895), which decreases both the MDMX mRNA and protein levels through repressing MDMX promoter activity (102). Further studies have found that XI-006 activates p53 and increases the expression of p53 target genes related to cell cycle arrest (e.g., p21) and apoptosis (e.g., PUMA, BAX, and PIG3), causing the accumulation of MCF-7 cells at subG0/G1 phase and apoptosis (102). It has also been shown that XI-006 additively enhances nutlin-3-induced p53 activation and its inhibitory effects on cancer cell viability in vitro (102). Pishas et al. have further demonstrated the anticancer activity of XI-006 in Ewing and osteosarcoma cell lines in vitro (103). However, neither the protein/mRNA levels of MDMX nor the p53 status has played an essential role in XI-006-mediated apoptosis in these cell lines, suggesting a complicated mechanism of action (103). In addition, Wang and Yan have reported another MDMX inhibitor, termed XI-011 (NSC146109), which induces apoptosis in breast cancer MCF7 cells by activating p53 (104). Further studies have shown that XI-011 decreases MDMX mRNA level by repressing MDMX promoter activity and MDMX expression plays an important role in the anticancer activity of XI-011 (104). Further investigations on the precise mechanisms of action for both XI-006 and XI-011 are warranted.
Jiang et al. have reported that luteolin exerts its anticancer activity by enhancing microRNA-34a-5p-mediated MDMX repression (105). It has been found that luteolin inhibits cell proliferation and induces apoptosis in non-small cell lung cancer (NSCLC) A549 and H460 cell lines in vitro and suppresses tumor growth in an H460 xenograft mouse model in vivo. The molecular mechanism studies have shown that luteolin up-regulates the expression of miR-34a-5p in NSCLC cells, which consequently leads to the down-regulation of its downstream target MDMX as well as the upregulation of p53 and p21 (105). The importance of the miR-34a-5p-MDMX axis in the anticancer activity of luteolin has also been validated using the transient transfection experiments of a miR-34a-5p inhibitor (105). Zu et al. have recently characterized tanshinone IIA as a new MDMX inhibitor (106). Tanshinone IIA has been found to inhibit MDMX expression by repressing MDMX mRNA synthesis, which leads to the downregulation of IAP3 (Inhibitor of apoptosis 3) (106). Further studies have shown that tanshinone IIA not only induces the apoptosis of H1299 cells but also enhances the doxorubicin-induced apoptosis (106).
Ginsenosides are a class of natural products with diverse biological activities (114). Wang et al. have identified a novel ginsenoside, 25-OCH3-PPD that possesses potent anticancer activities in preclinical cancer models in vitro and in vivo, with minimal host toxicity (115, 116). A recent study by the same group has reported that 25-OCH3-PPD inhibits MDMX expression and MDMX-MDM2 binding, thereby inducing the degradation of MDM2 (107). They have found that 25-OCH3-PPD inhibits the viability, proliferation, migration, and invasion of prostate cancer LNCaP, PC3, and DU145 cells in vitro, regardless of the p53 and androgen receptor statuses. Further in vivo studies have shown that 25-OCH3-PPD prevents prostate tumorigenesis and metastasis in the TRAMP mouse model without causing any significant host toxicity (107). However, the detailed mechanisms for the inhibitory effects of 25-OCH3-PPD on MDMX expression should be further investigated.
Antisense oligonucleotides (ASOs) have been used to efficiently modulate MDMX mRNA splicing (108). Dewaele et al. have demonstrated that enhanced exon 6 inclusion increases MDMX-FL expression level in human melanoma while exon 6 skipping causes MDMX-S production and decreases the expression level of MDMX-FL. It has further been shown that ASO-mediated exon 6 skipping decreases MDMX protein level and inhibits the growth of melanoma and diffuse large B cell lymphoma in vitro and in vivo. Because MDMX plays an important role in cancer cell chemoresistance, it is not surprising that the MDMX inhibition by ASO-mediated exon 6 skipping enhances the sensitivity of melanoma cells to BRAFV600E inhibitors in vitro and in vivo (108).
Protein arginine methyltransferase 5 (PRMT5) has recently been identified as a master regulator of splicing, which regulates MDMX abundance by controlling its alternative splicing (117). Herein, Gerhart et al. have examined the effects of PRMT5 inhibitors on the p53-MDMX axis and their anticancer efficacy (109). It has been found that PRMT5 inhibition by a specific inhibitor GSK3203591 induces the alternative splicing of MDMX and activates the p53 pathway. Further studies have shown that GSK3203591 inhibits cancer cell growth and survival in vitro and suppresses tumor growth in lymphoma xenograft mouse models in vivo (109).
Given that the formation of MDM2-MDMX complex stabilizes MDM2 protein, enhances its E3 ubiquitin ligase activity, and promotes the ubiquitination and degradation of MDMX, the MDM2-MDMX binding has been considered as a promising target for cancer therapy. Ling et al. have reported that FL118, a previously discovered inhibitor of survivin expression inhibits p53 ubiquitination but promotes MDMX protein degradation (110). They have further found that FL118 moderately increases the MDM2-MDMX binding and enhances MDM2-mediated MDMX ubiquitination and degradation. Importantly, MDMX overexpression has also increased FL118-induced death of HCT116 cancer cells, confirming the importance of MDMX expression for its anticancer activity. Moreover, FL118 has been shown to induce p53/p21-dependent senescence and p53-independent apoptosis in HCT116 cells (110).
Sesquiterpenoids are a class of structurally diverse and pharmacologically active natural products (118, 119). We have previously identified several dimeric sesquiterpenoids with potent anticancer activity in preclinical breast cancer models in vitro and in vivo (120, 121). Among these dimeric sesquiterpenoids, Inulanolide A has recently been shown to directly bind to the RING domains of MDM2 and MDMX, disrupt the MDM2-MDMX interaction, and promote the ubiquitination and proteasomal degradation of both proteins (112). We have shown that Inulanolide A inhibits cell viability and proliferation, induces G2/M-phase cell cycle arrest and apoptosis, and prevents migration and invasion in breast and prostate cancer cell lines in vitro (111, 112). Its in vivo inhibitory effects on tumor growth and metastasis have also been demonstrated in an MDA-MB-231 orthotopic mouse model (111). However, further evaluation of Inulanolide A in more clinically relevant models is warranted for the development of this compound as an anticancer agent.
Vaseva et al. have recently found that the Hsp90 inhibitor 17-allylamino-17-demethoxygeldanamycin (17AAG) induces cancer cell apoptosis and synergistically enhances nutlin-3-induced apoptosis in a p53-dependent manner (113). 17AAG has also been shown to suppress tumor growth in an H460 xenograft mouse model alone or in combination with nutlin-3. Mechanistically, 17AAG has been found to induce MDMX protein degradation, thereby disrupting the p53-MDMX interaction and activating p53 (113). However, the molecular mechanisms for 17AAG-induced MDMX degradation remain to be determined in the future.
Over the last two-and-a-half decades, MDMX has been developed from the status of the little brother of its well-studied and important “older” relative MDM2 to another key negative regulator of p53 in embryonic and, perhaps most importantly, cancer cells. Given that MDMX is frequently amplified and/or overexpressed in various types of human cancer (as summarized in Table 1) and plays an essential role in controlling the p53-MDM2/MDMX loop, MDMX has been demonstrated as a promising molecular target for cancer therapy. More and more researchers invest in the discovery of natural-product and rationally designed MDMX inhibitors and demonstrate their anticancer efficacy and mechanism(s) of action in preclinical cancer models in vitro and in vivo. Their main focus has been on the design and development of p53-MDMX binding inhibitors by targeting the p53-binding pocket of MDMX, such as SAH-p53-8 (92), mSF-SAH (93), SJ-172550 (94), compound B1 (96), CTX1 (97), and K-178 (98). All these inhibitors exert their anticancer activity through activating wild-type p53 and the transcription of its downstream target genes related to cell cycle arrest (e.g., p21) and apoptosis (e.g., Puma and Bax). However, the mutations and deletions of p53 are common genetic events in human cancer and lead to the limited efficacy of the p53-MDMX binding inhibitors in such types of cancer (20, 122).
Several small-molecule inhibitors of MDMX expression have been developed to inhibit both the p53-dependent and the p53-independent functions of MDMX, such as XI-006 (NSC207895) (102), XI-011 (104), luteolin (105), tanshinone IIA (106), 25-OCH3-PPD (107), ASO (108), and GSK3203591 (109). However, inhibiting the expression of a molecular target often causes unexpected adverse effects (123, 124). Of note, the regulatory mechanisms driving MDMX expression have not yet been fully elucidated, which is critical for developing such type of MDMX inhibitors. Recent studies have been performed to identify small-molecule inducers of MDMX protein degradation, such as FL118 (110), Inulanolide A (111), and 17AAG (113). Although targeting MDM2-MDMX interaction to enhance MDM2-mediated MDMX ubiquitination is an effective approach for promoting MDMX degradation, other potential strategies are also valuable and need to be examined. For example, inhibiting USP2a (ubiquitin-specific protease 2a)-mediated MDMX deubiquitination and stabilization and developing MDMX PROTACs (proteolysis targeting chimeras) should be considered for developing selective MDMX inhibitors for cancer therapy (125, 126).
The recently identified alternative splicing-dependent mechanism for MDMX overexpression in cancer has offered an alternative option for inhibiting MDMX (15, 127, 128). The highly expressed MDMX further inhibits p53 activation and compromises the p53-dependent cellular response to ionizing radiation and ribosomal stress as well as to the treatment of MDMX inhibitors (129, 130). The ectopically expressed MDMX-S protein, an MDMX spliceosome has shown a higher binding affinity to p53 and thus causes more potent inhibition on p53 than full-length MDMX. Although the expression of MDMX-S mRNA is often observed, the endogenous MDMX-S protein is undetectable in any normal or cancer cell lines, which implies that the endogenous MDMX-S may be a very unstable protein and the switch from MDMX-FL to MDMX-S may play a critical role in controlling the protein level of MDMX-FL and activating p53 in normal and/or cancer cells (128, 131). Besides, MDMX-211, another aberrantly spliced form of MDMX spliceosome has been found to bind to and stabilize MDM2 protein (132). Although there is no direct binding of MDMX-211 to p53, MDMX-211 also stabilizes p53 by counteracting MDM2-mediated p53 degradation (132). These MDMX spliceosomes may also be targeted for developing anticancer drugs due to their pivotal roles in regulating the protein stabilities and activities of MDMX, MDM2, and p53.
Paradoxically, increasing evidence has suggested a tumor suppressor role for MDMX under different stress conditions. In response to DNA damage, MDMX has been found to stably localize at the mitochondria, in which it enhances p53 phosphorylation at serine 46 (Ser46) and its mitochondrial localization, facilitates the p53Ser46p-Bcl2 (B-cell lymphoma 2) binding, and p53-HIPK2 (Homeodomain-interacting protein kinase 2) functional interaction, and promotes p53-mediated apoptosis (67, 125, 133). Besides, DNA damage and other stress conditions activate c-Abl tyrosine kinase that phosphorylates MDMX at tyrosine 99 (Tyr99), thereby reducing the MDMX-p53 binding and activating p53-dependent apoptosis pathway (134). It has also been shown that MDMX enhances the sensitivity of cancer cells to cisplatin (67, 135, 136). Although the pro-apoptotic roles of MDMX in cancer remain to be determined, it is worthy to be investigated whether MDMX inhibitors attenuate the anticancer efficacy of platinum-based chemotherapy, especially cisplatin. Considering the critical role of MDMX expression in cisplatin-induced cancer cell death, the MDMX inhibitor and cisplatin combination may exert an antagonistic effect and even cause chemoresistance. More detailed investigations are warranted to answer these questions.
In conclusion, the accumulating knowledge about the roles of MDMX in the p53-MDM2/MDMX loop, its p53-independent oncogenic functions, and the regulatory mechanisms for MDMX expression and protein stability may provide new opportunities to develop novel MDMX inhibitors. A better understanding of these MDMX inhibitors, especially their selectivity, efficacy, pharmacological, and toxicological properties is critical for moving them forward from bench to clinical trials.
J-JQ conceptualized the manuscript. D-HY, Z-YX, SM, LY, X-DC, and J-JQ collected the literature, wrote the manuscript, and made the figures. J-JQ edited and made significant revisions to the manuscript. All authors read and approved the final manuscript.
This work was supported by National Natural Science Foundation of China (81903842, 81573953), Program of Zhejiang Provincial TCM Sci-tech Plan (2020ZZ005, 2016ZZ012), Zhejiang Chinese Medical University Startup Funding (111100E014), Medical Science and Technology Project of Zhejiang Province (WKJ-ZJ-1728), Traditional Chinese Medical Science and Technology Major Project of Zhejiang Province (2018ZY006), and Science and Technology Projects of Zhejiang Province (2019C03049).
The authors declare that the research was conducted in the absence of any commercial or financial relationships that could be construed as a potential conflict of interest.
We thank the current and former members of our laboratories and collaborators for their contributions to the publications cited in this review article. The research field in MDMX is rapidly growing, and we apologize for not being able to cite all the recent publications, due to space limitation.
1. Shvarts A, Steegenga WT, Riteco N, Van Laar T, Dekker P, Bazuine M, et al. MDMX: a novel p53-binding protein with some functional properties of MDM2. EMBO J. (1996) 15:5349–57. doi: 10.1002/j.1460-2075.1996.tb00919.x
2. Shvarts A, Bazuine M, Dekker P, Ramos YF, Steegenga WT, Merckx G, et al. Isolation and identification of the human homolog of a new p53-binding protein, Mdmx. Genomics. (1997) 43:34–42. doi: 10.1006/geno.1997.4775
3. Momand J, Zambetti GP, Olson DC, George D, Levine AJ. The mdm-2 oncogene product forms a complex with the p53 protein and inhibits p53-mediated transactivation. Cell. (1992) 69:1237–45. doi: 10.1016/0092-8674(92)90644-R
4. Wang W, Qin JJ, Rajaei M, Li X, Yu X, Hunt C, et al. Targeting MDM2 for novel molecular therapy: beyond oncology. Med Res Rev. (2019) 40:856–80. doi: 10.1002/med.21637
5. Haupt Y, Maya R, Kazaz A, Oren M. Mdm2 promotes the rapid degradation of p53. Nature. (1997) 387:296–9. doi: 10.1038/387296a0
6. Honda R, Tanaka H, Yasuda H. Oncoprotein MDM2 is a ubiquitin ligase E3 for tumor suppressor p53. FEBS Lett. (1997) 420:25–7. doi: 10.1016/S0014-5793(97)01480-4
7. Kubbutat MH, Jones SN, Vousden KH. Regulation of p53 stability by Mdm2. Nature. (1997) 387:299–303. doi: 10.1038/387299a0
8. Linares LK, Hengstermann A, Ciechanover A, Muller S, Scheffner M. HdmX stimulates Hdm2-mediated ubiquitination and degradation of p53. Proc Natl Acad Sci USA. (2003) 100:12009–14. doi: 10.1073/pnas.2030930100
9. Nag S, Qin J, Srivenugopal KS, Wang M, Zhang R. The MDM2-p53 pathway revisited. J Biomed Res. (2013) 27:254–71. doi: 10.7555/JBR.27.20130030
10. Parant J, Chavez-Reyes A, Little NA, Yan W, Reinke V, Jochemsen AG, et al. Rescue of embryonic lethality in Mdm4-null mice by loss of Trp53 suggests a nonoverlapping pathway with MDM2 to regulate p53. Nat Genet. (2001) 29:92–5. doi: 10.1038/ng714
11. Finch RA, Donoviel DB, Potter D, Shi M, Fan A, Freed DD, et al. mdmx is a negative regulator of p53 activity in vivo. Cancer Res. (2002) 62:3221–5.
12. Tackmann NR, Zhang Y. Mouse modelling of the MDM2/MDMX-p53 signalling axis. J Mol Cell Biol. (2017) 9:34–44. doi: 10.1093/jmcb/mjx006
13. Sharp DA, Kratowicz SA, Sank MJ, George DL. Stabilization of the MDM2 oncoprotein by interaction with the structurally related MDMX protein. J Biol Chem. (1999) 274:38189–96. doi: 10.1074/jbc.274.53.38189
14. Wang X, Wang J, Jiang X. MdmX protein is essential for Mdm2 protein-mediated p53 polyubiquitination. J Biol Chem. (2011) 286:23725–34. doi: 10.1074/jbc.M110.213868
15. Marine JC, Jochemsen AG. MDMX (MDM4), a promising target for p53 reactivation therapy and beyond. Cold Spring Harb Perspect Med. (2016) 6:a026237. doi: 10.1101/cshperspect.a026237
16. Tan BX, Liew HP, Chua JS, Ghadessy FJ, Tan YS, Lane DP, et al. Anatomy of Mdm2 and Mdm4 in evolution. J Mol Cell Biol. (2017) 9:3–15. doi: 10.1093/jmcb/mjx002
17. Haupt S, Mejia-Hernandez JO, Vijayakumaran R, Keam SP, Haupt Y. The long and the short of it: the MDM4 tail so far. J Mol Cell Biol. (2019) 11:231–44. doi: 10.1093/jmcb/mjz007
18. De Polo A, Vivekanandan V, Little JB, Yuan ZM. MDMX under stress: the MDMX-MDM2 complex as stress signals hub. Transl Cancer Res. (2016) 5:725–32. doi: 10.21037/tcr.2016.12.18
19. Wang S, Zhao Y, Aguilar A, Bernard D, Yang CY. Targeting the MDM2-p53 protein-protein interaction for new cancer therapy: progress and challenges. Cold Spring Harb Perspect Med. (2017) 7:a026245. doi: 10.1101/cshperspect.a026245
20. Qin JJ, Li X, Hunt C, Wang W, Wang H, Zhang R. Natural products targeting the p53-MDM2 pathway and mutant p53: recent advances and implications in cancer medicine. Genes Dis. (2018) 5:204–19. doi: 10.1016/j.gendis.2018.07.002
21. Gupta A, Shah K, Oza MJ, Behl T. Reactivation of p53 gene by MDM2 inhibitors: a novel therapy for cancer treatment. Biomed Pharmacother. (2019) 109:484–92. doi: 10.1016/j.biopha.2018.10.155
22. Karni-Schmidt O, Lokshin M, Prives C. The roles of MDM2 and MDMX in cancer. Annu Rev Pathol. (2016) 11:617–44. doi: 10.1146/annurev-pathol-012414-040349
23. El-Dahr S, Hilliard S, Saifudeen Z. Regulation of kidney development by the Mdm2/Mdm4-p53 axis. J Mol Cell Biol. (2017) 9:26–33. doi: 10.1093/jmcb/mjx005
24. Haupt S, Vijayakumaran R, Miranda PJ, Burgess A, Lim E, Haupt Y. The role of MDM2 and MDM4 in breast cancer development and prevention. J Mol Cell Biol. (2017) 9:53–61. doi: 10.1093/jmcb/mjx007
25. Bardot B, Toledo F. Targeting MDM4 splicing in cancers. Genes. (2017) 8:82. doi: 10.3390/genes8020082
26. Tisato V, Voltan R, Gonelli A, Secchiero P, Zauli G. MDM2/X inhibitors under clinical evaluation: perspectives for the management of hematological malignancies and pediatric cancer. J Hematol Oncol. (2017) 10:133. doi: 10.1186/s13045-017-0500-5
27. Espadinha M, Barcherini V, Lopes EA, Santos MMM. An update on MDMX and dual MDM2/X inhibitors. Curr Top Med Chem. (2018) 18:647–60. doi: 10.2174/1568026618666180604080119
28. Liu Y, Wang X, Wang G, Yang Y, Yuan Y, Ouyang L. The past, present and future of potential small-molecule drugs targeting p53-MDM2/MDMX for cancer therapy. Eur J Med Chem. (2019) 176:92–104. doi: 10.1016/j.ejmech.2019.05.018
29. Skalniak L, Surmiak E, Holak TA. A therapeutic patent overview of MDM2/X-targeted therapies (2014-2018). Expert Opin Ther Pat. (2019) 29:151–70. doi: 10.1080/13543776.2019.1582645
30. Bottger V, Bottger A, Garcia-Echeverria C, Ramos YF, Van Der Eb AJ, Jochemsen AG, et al. Comparative study of the p53-mdm2 and p53-MDMX interfaces. Oncogene. (1999) 18:189–99. doi: 10.1038/sj.onc.1202281
31. Popowicz GM, Czarna A, Holak TA. Structure of the human Mdmx protein bound to the p53 tumor suppressor transactivation domain. Cell Cycle. (2008) 7:2441–3. doi: 10.4161/cc.6365
32. Gu J, Kawai H, Nie L, Kitao H, Wiederschain D, Jochemsen AG, et al. Mutual dependence of MDM2 and MDMX in their functional inactivation of p53. J Biol Chem. (2002) 277:19251–4. doi: 10.1074/jbc.C200150200
33. Okamoto K, Kashima K, Pereg Y, Ishida M, Yamazaki S, Nota A, et al. DNA damage-induced phosphorylation of MdmX at serine 367 activates p53 by targeting MdmX for Mdm2-dependent degradation. Mol Cell Biol. (2005) 25:9608–20. doi: 10.1128/MCB.25.21.9608-9620.2005
34. Shangary S, Qin D, Mceachern D, Liu M, Miller RS, Qiu S, et al. Temporal activation of p53 by a specific MDM2 inhibitor is selectively toxic to tumors and leads to complete tumor growth inhibition. Proc Natl Acad Sci USA. (2008) 105:3933–8. doi: 10.1073/pnas.0708917105
35. Cross B, Chen L, Cheng Q, Li B, Yuan ZM, Chen J. Inhibition of p53 DNA binding function by the MDM2 protein acidic domain. J Biol Chem. (2011) 286:16018–29. doi: 10.1074/jbc.M111.228981
36. Huang Q, Chen L, Yang L, Xie X, Gan L, Cleveland JL, et al. MDMX acidic domain inhibits p53 DNA binding in vivo and regulates tumorigenesis. Proc Natl Acad Sci USA. (2018) 115:E3368–77. doi: 10.1073/pnas.1719090115
37. Wu S, Chen L, Becker A, Schonbrunn E, Chen J. Casein kinase 1α regulates an MDMX intramolecular interaction to stimulate p53 binding. Mol Cell Biol. (2012) 32:4821–32. doi: 10.1128/MCB.00851-12
38. Chen L, Borcherds W, Wu S, Becker A, Schonbrunn E, Daughdrill GW, et al. Autoinhibition of MDMX by intramolecular p53 mimicry. Proc Natl Acad Sci USA. (2015) 112:4624–9. doi: 10.1073/pnas.1420833112
39. Uchida C, Miwa S, Isobe T, Kitagawa K, Hattori T, Oda T, et al. Effects of MdmX on Mdm2-mediated downregulation of pRB. FEBS Lett. (2006) 580:1753–8. doi: 10.1016/j.febslet.2006.02.029
40. Bista M, Petrovich M, Fersht AR. MDMX contains an autoinhibitory sequence element. Proc Natl Acad Sci USA. (2013) 110:17814–9. doi: 10.1073/pnas.1317398110
41. Tanimura S, Ohtsuka S, Mitsui K, Shirouzu K, Yoshimura A, Ohtsubo M. MDM2 interacts with MDMX through their RING finger domains. FEBS Lett. (1999) 447:5–9. doi: 10.1016/S0014-5793(99)00254-9
42. Leslie PL, Ke H, Zhang Y. The MDM2 RING domain and central acidic domain play distinct roles in MDM2 protein homodimerization and MDM2-MDMX protein heterodimerization. J Biol Chem. (2015) 290:12941–50. doi: 10.1074/jbc.M115.644435
43. Poyurovsky MV, Priest C, Kentsis A, Borden KL, Pan ZQ, Pavletich N, et al. The Mdm2 RING domain C-terminus is required for supramolecular assembly and ubiquitin ligase activity. EMBO J. (2007) 26:90–101. doi: 10.1038/sj.emboj.7601465
44. Uldrijan S, Pannekoek WJ, Vousden KH. An essential function of the extreme C-terminus of MDM2 can be provided by MDMX. EMBO J. (2007) 26:102–12. doi: 10.1038/sj.emboj.7601469
45. Ghosh M, Huang K, Berberich SJ. Overexpression of Mdm2 and MdmX fusion proteins alters p53 mediated transactivation, ubiquitination, and degradation. Biochemistry. (2003) 42:2291–9. doi: 10.1021/bi0271291
46. Kawai H, Lopez-Pajares V, Kim MM, Wiederschain D, Yuan ZM. RING domain-mediated interaction is a requirement for MDM2's E3 ligase activity. Cancer Res. (2007) 67:6026–30. doi: 10.1158/0008-5472.CAN-07-1313
47. Wade M, Li YC, Wahl GM. MDM2, MDMX and p53 in oncogenesis and cancer therapy. Nat Rev Cancer. (2013) 13:83–96. doi: 10.1038/nrc3430
48. Levav-Cohen Y, Goldberg Z, Tan KH, Alsheich-Bartok O, Zuckerman V, Haupt S, et al. The p53-Mdm2 loop: a critical juncture of stress response. Subcell Biochem. (2014) 85:161–86. doi: 10.1007/978-94-017-9211-0_9
49. Oliner JD, Pietenpol JA, Thiagalingam S, Gyuris J, Kinzler KW, Vogelstein B. Oncoprotein MDM2 conceals the activation domain of tumour suppressor p53. Nature. (1993) 362:857–60. doi: 10.1038/362857a0
50. Wu X, Bayle JH, Olson D, Levine AJ. The p53-mdm-2 autoregulatory feedback loop. Genes Dev. (1993) 7:1126–32. doi: 10.1101/gad.7.7a.1126
51. Zauberman A, Flusberg D, Haupt Y, Barak Y, Oren M. A functional p53-responsive intronic promoter is contained within the human mdm2 gene. Nucleic Acids Res. (1995) 23:2584–92. doi: 10.1093/nar/23.14.2584
52. Huang L, Yan Z, Liao X, Li Y, Yang J, Wang ZG, et al. The p53 inhibitors MDM2/MDMX complex is required for control of p53 activity in vivo. Proc Natl Acad Sci USA. (2011) 108:12001–6. doi: 10.1073/pnas.1102309108
53. Chen L, Li C, Pan Y, Chen J. Regulation of p53-MDMX interaction by casein kinase 1 alpha. Mol Cell Biol. (2005) 25:6509–20. doi: 10.1128/MCB.25.15.6509-6520.2005
54. Phillips A, Teunisse A, Lam S, Lodder K, Darley M, Emaduddin M, et al. HDMX-L is expressed from a functional p53-responsive promoter in the first intron of the HDMX gene and participates in an autoregulatory feedback loop to control p53 activity. J Biol Chem. (2010) 285:29111–27. doi: 10.1074/jbc.M110.129726
55. Di Conza G, Mancini F, Buttarelli M, Pontecorvi A, Trimarchi F, Moretti F. MDM4 enhances p53 stability by promoting an active conformation of the protein upon DNA damage. Cell Cycle. (2012) 11:749–60. doi: 10.4161/cc.11.4.19208
56. Singh RK, Iyappan S, Scheffner M. Hetero-oligomerization with MdmX rescues the ubiquitin/Nedd8 ligase activity of RING finger mutants of Mdm2. J Biol Chem. (2007) 282:10901–7. doi: 10.1074/jbc.M610879200
57. Riemenschneider MJ, Buschges R, Wolter M, Reifenberger J, Bostrom J, Kraus JA, et al. Amplification and overexpression of the MDM4 (MDMX) gene from 1q32 in a subset of malignant gliomas without TP53 mutation or MDM2 amplification. Cancer Res. (1999) 59:6091–6.
58. Riemenschneider MJ, Knobbe CB, Reifenberger G. Refined mapping of 1q32 amplicons in malignant gliomas confirms MDM4 as the main amplification target. Int J Cancer. (2003) 104:752–7. doi: 10.1002/ijc.11023
59. Furgason JM, Koncar RF, Michelhaugh SK, Sarkar FH, Mittal S, Sloan AE, et al. Whole genome sequence analysis links chromothripsis to EGFR, MDM2, MDM4, and CDK4 amplification in glioblastoma. Oncoscience. (2015) 2:618–28. doi: 10.18632/oncoscience.178
60. Gonzalez-Tablas M, Arandia D, Jara-Acevedo M, Otero A, Vital AL, Prieto C, et al. Heterogeneous EGFR, CDK4, MDM4, and PDGFRA gene expression profiles in primary GBM: no association with patient survival. Cancers. (2020) 12:231. doi: 10.3390/cancers12010231
61. Danovi D, Meulmeester E, Pasini D, Migliorini D, Capra M, Frenk R, et al. Amplification of Mdmx (or Mdm4) directly contributes to tumor formation by inhibiting p53 tumor suppressor activity. Mol Cell Biol. (2004) 24:5835–43. doi: 10.1128/MCB.24.13.5835-5843.2004
62. Abdel-Fatah TM, Powe DG, Agboola J, Adamowicz-Brice M, Blamey RW, Lopez-Garcia MA, et al. The biological, clinical and prognostic implications of p53 transcriptional pathways in breast cancers. J Pathol. (2010) 220:419–34. doi: 10.1002/path.2663
63. Tan WJ, Lai JC, Thike AA, Lim JC, Tan SY, Koh VC, et al. Novel genetic aberrations in breast phyllodes tumours: comparison between prognostically distinct groups. Breast Cancer Res Treat. (2014) 145:635–45. doi: 10.1007/s10549-014-2982-y
64. Yu Q, Li Y, Mu K, Li Z, Meng Q, Wu X, et al. Amplification of Mdmx and overexpression of MDM2 contribute to mammary carcinogenesis by substituting for p53 mutations. Diagn Pathol. (2014) 9:71. doi: 10.1186/1746-1596-9-71
65. Cao L, Basudan A, Sikora MJ, Bahreini A, Tasdemir N, Levine KM, et al. Frequent amplifications of ESR1, ERBB2 and MDM4 in primary invasive lobular breast carcinoma. Cancer Lett. (2019) 461:21–30. doi: 10.1016/j.canlet.2019.06.011
66. Suda T, Yoshihara M, Nakamura Y, Sekiguchi H, Godai TI, Sugano N, et al. Rare MDM4 gene amplification in colorectal cancer: the principle of a mutually exclusive relationship between MDM alteration and TP53 inactivation is not applicable. Oncol Rep. (2011) 26:49–54. doi: 10.3892/or.2011.1270
67. Mancini F, Di Conza G, Pellegrino M, Rinaldo C, Prodosmo A, Giglio S, et al. MDM4 (MDMX) localizes at the mitochondria and facilitates the p53-mediated intrinsic-apoptotic pathway. EMBO J. (2009) 28:1926–39. doi: 10.1038/emboj.2009.154
68. Busuttil RA, Zapparoli GV, Haupt S, Fennell C, Wong SQ, Pang JM, et al. Role of p53 in the progression of gastric cancer. Oncotarget. (2014) 5:12016–26. doi: 10.18632/oncotarget.2434
69. Bao J, Nanding A, Song H, Xu R, Qu G, Xue Y. The overexpression of MDM4: an effective and novel predictor of gastric adenocarcinoma lymph node metastasis. Oncotarget. (2016) 7:67212–22. doi: 10.18632/oncotarget.11971
70. Veerakumarasivam A, Scott HE, Chin SF, Warren A, Wallard MJ, Grimmer D, et al. High-resolution array-based comparative genomic hybridization of bladder cancers identifies mouse double minute 4 (MDM4) as an amplification target exclusive of MDM2 and TP53. Clin Cancer Res. (2008) 14:2527–34. doi: 10.1158/1078-0432.CCR-07-4129
71. Salvi S, Calistri D, Gurioli G, Carretta E, Serra L, Gunelli R, et al. Copy number analysis of 24 oncogenes: MDM4 identified as a putative marker for low recurrence risk in non-muscle invasive bladder cancer. Int J Mol Sci. (2014) 15:12458–68. doi: 10.3390/ijms150712458
72. Arai Y, Honda S, Haruta M, Kasai F, Fujiwara Y, Ohshima J, et al. Genome-wide analysis of allelic imbalances reveals 4q deletions as a poor prognostic factor and MDM4 amplification at 1q32.1 in hepatoblastoma. Genes Chromosomes Cancer. (2010) 49:596–609. doi: 10.1002/gcc.20770
73. Karki A, Putra J, Kim SS, Laquaglia MJ, Perez-Atayde AR, Sadri-Vakili G, et al. MDM4 expression in fibrolamellar hepatocellular carcinoma. Oncol Rep. (2019) 42:1487–96. doi: 10.3892/or.2019.7241
74. Bartel F, Schulz J, Bohnke A, Blumke K, Kappler M, Bache M, et al. Significance of HDMX-S (or MDM4) mRNA splice variant overexpression and HDMX gene amplification on primary soft tissue sarcoma prognosis. Int J Cancer. (2005) 117:469–75. doi: 10.1002/ijc.21206
75. Pissaloux D, Loarer FL, Decouvelaere AV, Paindavoine S, Houlier A, Vernay L, et al. MDM4 amplification in a case of de-differentiated liposarcoma and in-silico data supporting an oncogenic event alternative to MDM2 amplification in a subset of cases. Histopathology. (2017) 71:1019–23. doi: 10.1111/his.13331
76. Ito M, Barys L, O'reilly T, Young S, Gorbatcheva B, Monahan J, et al. Comprehensive mapping of p53 pathway alterations reveals an apparent role for both SNP309 and MDM2 amplification in sarcomagenesis. Clin Cancer Res. (2011) 17:416–26. doi: 10.1158/1078-0432.CCR-10-2050
77. Duhamel LA, Ye H, Halai D, Idowu BD, Presneau N, Tirabosco R, et al. Frequency of Mouse Double Minute 2 (MDM2) and Mouse Double Minute 4 (MDM4) amplification in parosteal and conventional osteosarcoma subtypes. Histopathology. (2012) 60:357–9. doi: 10.1111/j.1365-2559.2011.04023.x
78. Lenos K, Grawenda AM, Lodder K, Kuijjer ML, Teunisse AF, Repapi E, et al. Alternate splicing of the p53 inhibitor HDMX offers a superior prognostic biomarker than p53 mutation in human cancer. Cancer Res. (2012) 72:4074–84. doi: 10.1158/0008-5472.CAN-12-0215
79. Liu L, Fan L, Fang C, Zou ZJ, Yang S, Zhang LN, et al. S-MDM4 mRNA overexpression indicates a poor prognosis and marks a potential therapeutic target in chronic lymphocytic leukemia. Cancer Sci. (2012) 103:2056–63. doi: 10.1111/cas.12008
80. Li L, Tan Y, Chen X, Xu Z, Yang S, Ren F, et al. MDM4 overexpressed in acute myeloid leukemia patients with complex karyotype and wild-type TP53. PLoS ONE. (2014) 9:e113088. doi: 10.1371/journal.pone.0113088
81. Liang M, Han X, Vadhan-Raj S, Nguyen M, Zhang YH, Fernandez M, et al. HDM4 is overexpressed in mantle cell lymphoma and its inhibition induces p21 expression and apoptosis. Mod Pathol. (2010) 23:381–91. doi: 10.1038/modpathol.2009.170
82. Leventaki V, Rodic V, Tripp SR, Bayerl MG, Perkins SL, Barnette P, et al. TP53 pathway analysis in paediatric Burkitt lymphoma reveals increased MDM4 expression as the only TP53 pathway abnormality detected in a subset of cases. Br J Haematol. (2012) 158:763–71. doi: 10.1111/j.1365-2141.2012.09243.x
83. Ach T, Schwarz-Furlan S, Ach S, Agaimy A, Gerken M, Rohrmeier C, et al. Genomic aberrations of MDM2, MDM4, FGFR1, and FGFR3 are associated with poor outcome in patients with salivary gland cancer. J Oral Pathol Med. (2016) 45:500–9. doi: 10.1111/jop.12394
84. Prodosmo A, Giglio S, Moretti S, Mancini F, Barbi F, Avenia N, et al. Analysis of human MDM4 variants in papillary thyroid carcinomas reveals new potential markers of cancer properties. J Mol Med. (2008) 86:585–96. doi: 10.1007/s00109-008-0322-6
85. Gembarska A, Luciani F, Fedele C, Russell EA, Dewaele M, Villar S, et al. MDM4 is a key therapeutic target in cutaneous melanoma. Nat Med. (2012) 18:1239–47. doi: 10.1038/nm.2863
86. Valentin-Vega YA, Barboza JA, Chau GP, El-Naggar AK, Lozano G. High levels of the p53 inhibitor MDM4 in head and neck squamous carcinomas. Hum Pathol. (2007) 38:1553–62. doi: 10.1016/j.humpath.2007.03.005
87. Guo Y, Pajovic S, Gallie BL. Expression of p14ARF, MDM2, and MDM4 in human retinoblastoma. Biochem Biophys Res Commun. (2008) 375:1–5. doi: 10.1016/j.bbrc.2008.07.055
88. Eeles RA, Olama AA, Benlloch S, Saunders EJ, Leongamornlert DA, Tymrakiewicz M, et al. Identification of 23 new prostate cancer susceptibility loci using the iCOGS custom genotyping array. Nat Genet. (2013) 45:385–91. doi: 10.1038/ng.2560
89. Garcia-Closas M, Couch FJ, Lindstrom S, Michailidou K, Schmidt MK, Brook MN, et al. Genome-wide association studies identify four ER negative-specific breast cancer risk loci. Nat Genet. (2013) 45:392–8. doi: 10.1038/ng.2561
90. Sakoda LC, Jorgenson E, Witte JS. Turning of COGS moves forward findings for hormonally mediated cancers. Nat Genet. (2013) 45:345–8. doi: 10.1038/ng.2587
91. Milne RL, Kuchenbaecker KB, Michailidou K, Beesley J, Kar S, Lindstrom S, et al. Identification of 10 variants associated with risk of estrogen-receptor-negative breast cancer. Nat Genet. (2017) 49:1767–78. doi: 10.1038/ng.3785
92. Bernal F, Wade M, Godes M, Davis TN, Whitehead DG, Kung AL, et al. A stapled p53 helix overcomes HDMX-mediated suppression of p53. Cancer Cell. (2010) 18:411–22. doi: 10.1016/j.ccr.2010.10.024
93. Hoppmann C, Wang L. Proximity-enabled bioreactivity to generate covalent peptide inhibitors of p53-Mdm4. Chem Commun. (2016) 52:5140–3. doi: 10.1039/C6CC01226D
94. Reed D, Shen Y, Shelat AA, Arnold LA, Ferreira AM, Zhu F, et al. Identification and characterization of the first small molecule inhibitor of MDMX. J Biol Chem. (2010) 285:10786–96. doi: 10.1074/jbc.M109.056747
95. Bista M, Smithson D, Pecak A, Salinas G, Pustelny K, Min J, et al. On the mechanism of action of SJ-172550 in inhibiting the interaction of MDM4 and p53. PLoS ONE. (2012) 7:e37518. doi: 10.1371/journal.pone.0037518
96. Boltjes A, Huang Y, Van De Velde R, Rijkee L, Wolf S, Gaugler J, et al. Fragment-based library generation for the discovery of a peptidomimetic p53-Mdm4 inhibitor. ACS Comb Sci. (2014) 16:393–6. doi: 10.1021/co500026b
97. Karan G, Wang H, Chakrabarti A, Karan S, Liu Z, Xia Z, et al. Identification of a small molecule that overcomes HdmX-mediated suppression of p53. Mol Cancer Ther. (2016) 15:574–82. doi: 10.1158/1535-7163.MCT-15-0467
98. Uesato S, Matsuura Y, Matsue S, Sumiyoshi T, Hirata Y, Takemoto S, et al. Discovery of new low-molecular-weight p53-Mdmx disruptors and their anti-cancer activities. Bioorg Med Chem. (2016) 24:1919–26. doi: 10.1016/j.bmc.2016.03.021
99. Chang YS, Graves B, Guerlavais V, Tovar C, Packman K, To KH, et al. Stapled alpha-helical peptide drug development: a potent dual inhibitor of MDM2 and MDMX for p53-dependent cancer therapy. Proc Natl Acad Sci USA. (2013) 110:E3445–54. doi: 10.1073/pnas.1303002110
100. Carvajal LA, Neriah DB, Senecal A, Benard L, Thiruthuvanathan V, Yatsenko T, et al. Dual inhibition of MDMX and MDM2 as a therapeutic strategy in leukemia. Sci Transl Med. (2018) 10:aao3003. doi: 10.1126/scitranslmed.aao3003
101. Jiang L, Malik N, Acedo P, Zawacka-Pankau J. Protoporphyrin IX is a dual inhibitor of p53/MDM2 and p53/MDM4 interactions and induces apoptosis in B-cell chronic lymphocytic leukemia cells. Cell Death Discov. (2019) 5:77. doi: 10.1038/s41420-019-0157-7
102. Wang H, Ma X, Ren S, Buolamwini JK, Yan C. A small-molecule inhibitor of MDMX activates p53 and induces apoptosis. Mol Cancer Ther. (2011) 10:69–79. doi: 10.1158/1535-7163.MCT-10-0581
103. Pishas KI, Adwal A, Neuhaus SJ, Clayer MT, Farshid G, Staudacher AH, et al. XI-006 induces potent p53-independent apoptosis in Ewing sarcoma. Sci Rep. (2015) 5:11465. doi: 10.1038/srep13328
104. Wang H, Yan C. A small-molecule p53 activator induces apoptosis through inhibiting MDMX expression in breast cancer cells. Neoplasia. (2011) 13:611–9. doi: 10.1593/neo.11438
105. Jiang ZQ, Li MH, Qin YM, Jiang HY, Zhang X, Wu MH. Luteolin inhibits tumorigenesis and induces apoptosis of non-small cell lung cancer cells via regulation of microRNA-34a-5p. Int J Mol Sci. (2018) 19:447. doi: 10.3390/ijms19020447
106. Zu Y, Wang J, Ping W, Sun W. Tan IIA inhibits H1299 cell viability through the MDM4IAP3 signaling pathway. Mol Med Rep. (2018) 17:2384–92. doi: 10.3892/mmr.2017.8152
107. Wang W, Qin JJ, Li X, Tao G, Wang Q, Wu X, et al. Prevention of prostate cancer by natural product MDM2 inhibitor GS25: in vitro and in vivo activities and molecular mechanisms. Carcinogenesis. (2018) 39:1026–36. doi: 10.1093/carcin/bgy063
108. Dewaele M, Tabaglio T, Willekens K, Bezzi M, Teo SX, Low DH, et al. Antisense oligonucleotide-mediated MDM4 exon 6 skipping impairs tumor growth. J Clin Invest. (2016) 126:68–84. doi: 10.1172/JCI82534
109. Gerhart SV, Kellner WA, Thompson C, Pappalardi MB, Zhang XP, Montes De Oca R, et al. Activation of the p53-MDM4 regulatory axis defines the anti-tumour response to PRMT5 inhibition through its role in regulating cellular splicing. Sci Rep. (2018) 8:9711. doi: 10.1038/s41598-018-28002-y
110. Ling X, Xu C, Fan C, Zhong K, Li F, Wang X. FL118 induces p53-dependent senescence in colorectal cancer cells by promoting degradation of MdmX. Cancer Res. (2014) 74:7487–97. doi: 10.1158/0008-5472.CAN-14-0683
111. Qin JJ, Wang W, Sarkar S, Voruganti S, Agarwal R, Zhang R. Inulanolide A as a new dual inhibitor of NFAT1-MDM2 pathway for breast cancer therapy. Oncotarget. (2016) 7:32566–78. doi: 10.18632/oncotarget.8873
112. Qin JJ, Li X, Wang W, Zi X, Zhang R. Targeting the NFAT1-MDM2-MDMX network inhibits the proliferation and invasion of prostate cancer cells, independent of p53 and androgen. Front Pharmacol. (2017) 8:917. doi: 10.3389/fphar.2017.00917
113. Vaseva AV, Yallowitz AR, Marchenko ND, Xu S, Moll UM. Blockade of Hsp90 by 17AAG antagonizes MDMX and synergizes with Nutlin to induce p53-mediated apoptosis in solid tumors. Cell Death Dis. (2011) 2:e156. doi: 10.1038/cddis.2011.39
114. Nag SA, Qin JJ, Wang W, Wang MH, Wang H, Zhang R. Ginsenosides as anticancer agents: in vitro and in vivo activities, structure-activity relationships, and molecular mechanisms of action. Front Pharmacol. (2012) 3:25. doi: 10.3389/fphar.2012.00025
115. Wang W, Zhang X, Qin JJ, Voruganti S, Nag SA, Wang MH, et al. Natural product ginsenoside 25-OCH3-PPD inhibits breast cancer growth and metastasis through down-regulating MDM2. PLoS ONE. (2012) 7:e41586. doi: 10.1371/journal.pone.0041586
116. Voruganti S, Qin JJ, Sarkar S, Nag S, Walbi IA, Wang S, et al. Oral nano-delivery of anticancer ginsenoside 25-OCH3-PPD, a natural inhibitor of the MDM2 oncogene: nanoparticle preparation, characterization, in vitro and in vivo anti-prostate cancer activity, and mechanisms of action. Oncotarget. (2015) 6:21379–94. doi: 10.18632/oncotarget.4091
117. Bezzi M, Teo SX, Muller J, Mok WC, Sahu SK, Vardy LA, et al. Regulation of constitutive and alternative splicing by PRMT5 reveals a role for Mdm4 pre-mRNA in sensing defects in the spliceosomal machinery. Genes Dev. (2013) 27:1903–16. doi: 10.1101/gad.219899.113
118. Li X, Yang X, Liu Y, Gong N, Yao W, Chen P, et al. Japonicone A suppresses growth of Burkitt lymphoma cells through its effect on NF-kappaB. Clin Cancer Res. (2013) 19:2917–28. doi: 10.1158/1078-0432.CCR-12-3258
119. Wang GW, Qin JJ, Cheng XR, Shen YH, Shan L, Jin HZ, et al. Inula sesquiterpenoids: structural diversity, cytotoxicity and anti-tumor activity. Expert Opin Investig Drugs. (2014) 23:317–45. doi: 10.1517/13543784.2014.868882
120. Qin JJ, Jin HZ, Huang Y, Zhang SD, Shan L, Voruganti S, et al. Selective cytotoxicity, inhibition of cell cycle progression, and induction of apoptosis in human breast cancer cells by sesquiterpenoids from Inula lineariifolia Turcz. Eur J Med Chem. (2013) 68:473–81. doi: 10.1016/j.ejmech.2013.07.018
121. Qin JJ, Wang W, Voruganti S, Wang H, Zhang WD, Zhang R. Identification of a new class of natural product MDM2 inhibitor: in vitro and in vivo anti-breast cancer activities and target validation. Oncotarget. (2015) 6:2623–40. doi: 10.18632/oncotarget.3098
122. Bykov VJN, Eriksson SE, Bianchi J, Wiman KG. Targeting mutant p53 for efficient cancer therapy. Nat Rev Cancer. (2018) 18:89–102. doi: 10.1038/nrc.2017.109
123. Qin JJ, Yan L, Zhang J, Zhang WD. STAT3 as a potential therapeutic target in triple negative breast cancer: a systematic review. J Exp Clin Cancer Res. (2019) 38:195. doi: 10.1186/s13046-019-1206-z
124. Dong J, Qin Z, Zhang WD, Cheng G, Yehuda AG, Ashby CRJr, et al. Medicinal chemistry strategies to discover P-glycoprotein inhibitors: an update. Drug Resist Updat. (2020) 49:100681. doi: 10.1016/j.drup.2020.100681
125. Wang CL, Wang JY, Liu ZY, Ma XM, Wang XW, Jin H, et al. Ubiquitin-specific protease 2a stabilizes MDM4 and facilitates the p53-mediated intrinsic apoptotic pathway in glioblastoma. Carcinogenesis. (2014) 35:1500–9. doi: 10.1093/carcin/bgu015
126. Liu J, Ma J, Liu Y, Xia J, Li Y, Wang ZP, et al. PROTACs: a novel strategy for cancer therapy. Semin Cancer Biol. (2020). doi: 10.1016/j.semcancer.2020.02.006. [Epub ahead of print].
127. Rallapalli R, Strachan G, Cho B, Mercer WE, Hall DJ. A novel MDMX transcript expressed in a variety of transformed cell lines encodes a truncated protein with potent p53 repressive activity. J Biol Chem. (1999) 274:8299–308. doi: 10.1074/jbc.274.12.8299
128. Rallapalli R, Strachan G, Tuan RS, Hall DJ. Identification of a domain within MDMX-S that is responsible for its high affinity interaction with p53 and high-level expression in mammalian cells. J Cell Biochem. (2003) 89:563–75. doi: 10.1002/jcb.10535
129. Wade M, Wong ET, Tang M, Stommel JM, Wahl GM. Hdmx modulates the outcome of p53 activation in human tumor cells. J Biol Chem. (2006) 281:33036–44. doi: 10.1074/jbc.M605405200
130. Toledo F, Wahl GM. MDM2 and MDM4: p53 regulators as targets in anticancer therapy. Int J Biochem Cell Biol. (2007) 39:1476–82. doi: 10.1016/j.biocel.2007.03.022
131. Ramos YF, Stad R, Attema J, Peltenburg LT, Van Der Eb AJ, Jochemsen AG. Aberrant expression of HDMX proteins in tumor cells correlates with wild-type p53. Cancer Res. (2001) 61:1839–42.
132. Giglio S, Mancini F, Gentiletti F, Sparaco G, Felicioni L, Barassi F, et al. Identification of an aberrantly spliced form of HDMX in human tumors: a new mechanism for HDM2 stabilization. Cancer Res. (2005) 65:9687–94. doi: 10.1158/0008-5472.CAN-05-0450
133. Mancini F, Pieroni L, Monteleone V, Luca R, Fici L, Luca E, et al. MDM4/HIPK2/p53 cytoplasmic assembly uncovers coordinated repression of molecules with anti-apoptotic activity during early DNA damage response. Oncogene. (2016) 35:228–40. doi: 10.1038/onc.2015.76
134. Chen X, Gohain N, Zhan C, Lu WY, Pazgier M, Lu W. Structural basis of how stress-induced MDMX phosphorylation activates p53. Oncogene. (2016) 35:1919–25. doi: 10.1038/onc.2015.255
135. Zhu Y, Regunath K, Jacq X, Prives C. Cisplatin causes cell death via TAB1 regulation of p53/MDM2/MDMX circuitry. Genes Dev. (2013) 27:1739–51. doi: 10.1101/gad.212258.112
Keywords: MDMX, MDM2, p53, oncogene, tumor suppressor, inhibitors
Citation: Yu D-H, Xu Z-Y, Mo S, Yuan L, Cheng X-D and Qin J-J (2020) Targeting MDMX for Cancer Therapy: Rationale, Strategies, and Challenges. Front. Oncol. 10:1389. doi: 10.3389/fonc.2020.01389
Received: 07 April 2020; Accepted: 01 July 2020;
Published: 05 August 2020.
Edited by:
David A. Gewirtz, Virginia Commonwealth University, United StatesReviewed by:
Aart Jochemsen, Leiden University Medical Center, NetherlandsCopyright © 2020 Yu, Xu, Mo, Yuan, Cheng and Qin. This is an open-access article distributed under the terms of the Creative Commons Attribution License (CC BY). The use, distribution or reproduction in other forums is permitted, provided the original author(s) and the copyright owner(s) are credited and that the original publication in this journal is cited, in accordance with accepted academic practice. No use, distribution or reproduction is permitted which does not comply with these terms.
*Correspondence: Jiang-Jiang Qin, anFpbkB6Y211LmVkdS5jbg==; enlseXNqdHVAaG90bWFpbC5jb20=
Disclaimer: All claims expressed in this article are solely those of the authors and do not necessarily represent those of their affiliated organizations, or those of the publisher, the editors and the reviewers. Any product that may be evaluated in this article or claim that may be made by its manufacturer is not guaranteed or endorsed by the publisher.
Research integrity at Frontiers
Learn more about the work of our research integrity team to safeguard the quality of each article we publish.