- 1Translational Oncology Research Group, Institute of Genetics and Molecular Medicine, Western General Hospital, University of Edinburgh, Edinburgh, United Kingdom
- 2The Royal (Dick) School of Veterinary Studies and Roslin Institute, University of Edinburgh, Edinburgh, United Kingdom
- 3Breast Cancer Now Edinburgh Research Team, Institute of Genetics and Molecular Medicine, Western General Hospital, University of Edinburgh, Edinburgh, United Kingdom
- 4School of Applied Science, Sighthill Campus, Edinburgh Napier University, Edinburgh, United Kingdom
- 5Cancer Research UK Edinburgh Centre and Division of Pathology Laboratories, Institute of Genetics and Molecular Medicine, University of Edinburgh, Edinburgh, United Kingdom
Radiotherapy remains an important treatment modality in nearly two thirds of all cancers, including the primary curative or palliative treatment of breast cancer. Unfortunately, largely due to tumor heterogeneity, tumor radiotherapy response rates can vary significantly, even between patients diagnosed with the same tumor type. Although in recent years significant technological advances have been made in the way radiation can be precisely delivered to tumors, it is proving more difficult to personalize radiotherapy regimens based on cancer biology. Biomarkers that provide prognostic or predictive information regarding a tumor's intrinsic radiosensitivity or its response to treatment could prove valuable in helping to personalize radiation dosing, enabling clinicians to make decisions between different treatment options whilst avoiding radiation-induced toxicity in patients unlikely to gain therapeutic benefit. Studies have investigated numerous ways in which both patient and tumor radiosensitivities can be assessed. Tumor molecular profiling has been used to develop radiosensitivity gene signatures, while the assessment of specific intracellular or secreted proteins, including circulating tumor cells, exosomes and DNA, has been performed to identify prognostic or predictive biomarkers of radiation response. Finally, the investigation of biomarkers related to radiation-induced toxicity could provide another means by which radiotherapy could become personalized. In this review, we discuss studies that have used these methods to identify or develop prognostic/predictive signatures of radiosensitivity, and how such assays could be used in the future as a means of providing personalized radiotherapy.
Radiotherapy in Breast Cancer Treatment
In 2018 it was estimated that ~18 million new cancer cases and 10 million cancer-related deaths occurred worldwide (1). If current trends in global population growth continue, combined with the effects of an aging population, these figures are predicted to increase to 20 million new cases and 13 million deaths per year by 2030 (2). Breast cancer (BC) is the most common female cancer, with ~2 million new cases and 0.7 million cancer-related deaths occurring per year (1). Despite the multitude of advances made in both the surgical and systemic treatment of cancer patients, radiotherapy (RT) has a key role in the management of nearly two thirds of all cancers (3).
RT is commonly given to BC patients after surgery. These adjuvant RT treatment plans typically involve the delivery of radiation to the tumor in multiple fractions over a period of several weeks. The most common adjuvant RT fractionation regimen following breast-conserving surgery is 25 fractions of 2 Gy over a 5 week period, or hypofractionated regimens consisting of a total of 40 Gy delivered in 15 fractions over 3 weeks (4). An external beam boost to the tumor bed can also be employed following whole-breast RT or integrated simultaneously with whole-breast irradiation (5) for invasive BCs which have a high risk of local recurrence. This boost procedure typically involves either 10 Gy in 5 fractions or 16 Gy in 8 fractions (6, 7), both delivered over the course of 1 week, or a radiobiologically equivalent dose such as 12 Gy in 4 fractions (8). Local recurrences occur most commonly at the original site of the primary tumor due to remaining microscopic tumor cells left following surgery; the objective of adjuvant and boost RT is to eradicate these tumor cells (7, 9). Accelerated partial breast irradiation (APBI) can be considered as an alternative treatment approach to conventional external beam RT or exclusive hormonal therapy which may be particularly useful for patients with a low-risk of local tumor recurrence or for elderly patients. APBI allows the delivery of higher radiation doses in the area of the tumor bed whilst reducing the dose received by normal breast tissue and adjacent organs at risk. Shorter treatment times and acceptable acute toxicity rates can improve patient quality of life whilst also reducing the total cost of treatment (10–14). Linear accelerators are the most commonly used devices for delivering external beam radiation to patients. In recent years, these machines have undergone significant technology-driven improvements that have culminated in the generation of modern radiation-delivery techniques such as intensity-modulated RT and image-guided RT approaches, which are designed to allow highly conformal and precise distribution of radiation to the tumor. Radiation delivery methods such as “dose painting by numbers” or sculpturing are becoming achievable through the use of these machines, meaning that identified subvolumes can be targeted specifically, limiting the radiation dose received by the nearby normal tissues (15). Although these current radiation planning techniques are largely based on advanced imaging to identify the gross tumor volume, the identification of a biological target volume based on the underlying tumor biology could lead to the development of a more personalized radiation treatment plan.
Of patients diagnosed with BC in England between 2013 and 2014, 63% received curative or palliative RT as part of their primary treatment (16). The Oxford overview shows that adjuvant RT halves the 10-year first risk of recurrence (most of which are local breast recurrences) after breast-conserving surgery and systemic therapy in all risk categories (9) and improves survival in lymph node positive patients receiving adjuvant systemic therapy (17). Studies have suggested that RT can benefit up to 83% of BC patients (18) and that breast-conserving surgery followed by whole-breast RT can provide local control and survival rates equivalent to mastectomy (19–21). These results, combined with the advantages of improved cosmetic outcomes and reduced side effects, have made the incorporation of RT into BC treatment standard practice for a large proportion of patients. Unfortunately, not all BC patients gain therapeutic benefit from RT. Although overall 5-year survival rates after RT are ~80%, it is estimated that 30% of these patients will develop local recurrences or metastatic disease, the majority of whom will die within 5 years (22). Additionally, others in the neoadjuvant and palliative settings may only experience an initial partial response or may not respond at all. Side effects, which can affect a patient's quality of life, can also occur with RT.
A variety of imaging approaches for measuring tumor response to RT during or after treatment have been developed; these typically analyse how often and by how much a tumor shrinks anatomically. The RECIST (Response Evaluation Criteria in Solid Tumors) criteria have been widely adopted and are commonly used in oncology clinical trials. This categorizes the tumor response into complete (disappearance of tumor lesions for at least 4 weeks), partial (tumor diameter reduction of at least 30% for at least 4 weeks), stable (neither progressive disease nor partial response) or progressive (tumor diameter increase of at least 20%). Imaging techniques used to measure these changes in tumor size include X-ray, X-ray computed tomography, magnetic resonance imaging and positron emission tomography (23). However, as many of these techniques measure changes in tumor size alone to assess response to treatment, they ignore the underlying biology that drives the response to radiation. Additionally, analyzing changes in tumor size is a measurable clinical outcome that is seen only towards the end or after the treatment has finished, with patients who fail to respond to treatment initially going undetected. This delay may contribute to tumor progression, impact long-term survival and ultimately delay the initiation of an alternative treatment strategy. Non-responding patients will also be at risk of developing RT-induced side effects for no therapeutic gain. Despite the significant evidence that RT can benefit BC patients as a whole, there are still no clinically validated biomarkers that can be used to predict whether neoadjuvant/adjuvant RT will improve outcomes for individual patients.
Precision Medicine, Prognostic and Predictive Biomarkers
Precision medicine is defined as the incorporation of disease biomarkers, molecular signatures and phenotypes in combination with patient lifestyle and environment into the prevention, investigation and treatment of diseases (24). Using these criteria, patients can be classified into cohorts according to differences in disease susceptibility, prognosis and likely treatment response rates. To improve clinical outcomes, this type of information can be used to select patients that may require more aggressive treatments and those that are most likely to benefit from specific treatments (25). Genomic instability is a key feature of cancer that is characterized by genetic and epigenetic heterogeneity (26–28); it is therefore not surprising that patients diagnosed with the same cancer type vary in prognosis and in their responses to treatment. As we improve our understanding of the fundamental processes that control carcinogenesis and pathogenesis, precision medicine will become more and more important in the management of cancer patients. An era of personalized cancer medicine, in which biomarkers can be used to tailor treatment to each specific patient, is a major goal in oncology.
Biomarkers can be defined as characteristics which can be evaluated and measured as indicators of normal biological processes, pathogenesis or response to therapy (29). Cancer biomarkers may be diagnostic, prognostic, predictive, or used to monitor treatment responses. Prognostic biomarkers provide information about a patient's overall cancer outcome, irrespective of therapy. They can identify high-risk patients who may benefit from more aggressive treatments but provide no information on which patients will most likely derive a clinical benefit from a specific therapy. Conversely, predictive biomarkers can indicate the probability of a patient gaining a therapeutic benefit from a specific treatment (30–32). These fundamental prognostic and predictive biomarker concepts have been integrated into the precision medicine initiative.
BC radiomics, an emerging field in precision medicine, is the process of extracting quantitative information from medical images to influence patient treatment. This concept assumes that extracted imaging data are the result of biological processes occurring at a genetic and molecular level, and are therefore associated with the genotypic and phenotypic characteristics of the tumor (33). Radiomic features have been correlated with BC clinical data including stage, lymph node involvement and hormone receptor status (34), and also have the ability to discriminate between malignant and benign lesions (35). While radiomics has the potential to contribute to BC precision medicine in the future, BC molecular classification systems based on microarray gene expression analysis are currently being used clinically. These classification systems have identified several intrinsic BC molecular subtypes which have been shown to differ in treatment responses and predict disease-free survival and overall survival (36–39). Unfortunately, microarray analysis or genome sequencing for individual patients is cost-prohibitive for routine clinical use. To overcome this issue, studies have investigated the potential utility of using smaller gene sets to stratify BC patients, providing prognostic and/or predictive information which can be employed by clinicians to guide the use of adjuvant chemotherapy or endocrine therapy (40). These clinically-available tests include the breast cancer index (41), Endopredict (42), the Oncotype DX 21-gene recurrence score (43), the BreastOncPx 14-gene distant metastasis signature (44), and the MammaPrint 70-gene prognosis signature (45). Finally, a 50-gene signature called PAM50 (now commercialized under the name Prosigna) has improved the ability to predict recurrence of estrogen receptor+/lymph node− BC patients compared to models using only clinical variables (46, 47). Although these clinically validated genetic tests have made significant changes in the way patients are selected for receiving chemotherapy or endocrine therapy, RT treatment plans still rely upon historically standardized, one-size-fits-all therapeutic approaches.
A move toward more personalized RT treatment, tailored to individual risk and tumor biology, would help improve patient outcomes (48). Many factors are known to influence a tumor's response to irradiation, including total dose, fractionation, tumor doubling time, hypoxia and intrinsic radiosensitivity. If RT is to become part of the precision medicine initiative, we need to identify clinically-validated biomarkers that can predict response to RT before starting treatment or biomarkers that can help clinicians assess a tumor's response to RT during treatment (49). Currently there are no sufficiently validated biomarkers of radiosensitivity for routine application in the clinic (49). These assays/biomarkers might allow the tailoring of radiation dose regimens to individual patients based on tumor biology or the prediction of the risk of localized tissue toxicity. Patients unlikely to benefit from RT could be spared radiation-induced toxicities and associated co-morbidities, whilst allowing more effective therapies to be instigated at an earlier stage of the treatment process (Figure 1). As discussed above, molecular signatures have been developed that enable clinicians to more individually tailor systemic therapies for patients with BC. Slower progress is being made to develop tools to predict RT response; in part this is due to radiation oncology vendors not having the financial capacity to support genomic-orientated collaborations with radiation oncology investigators (50). Nonetheless, much work has been performed to try and develop such biomarkers. The aim of this review is to highlight recent developments in this exciting yet under-researched field.
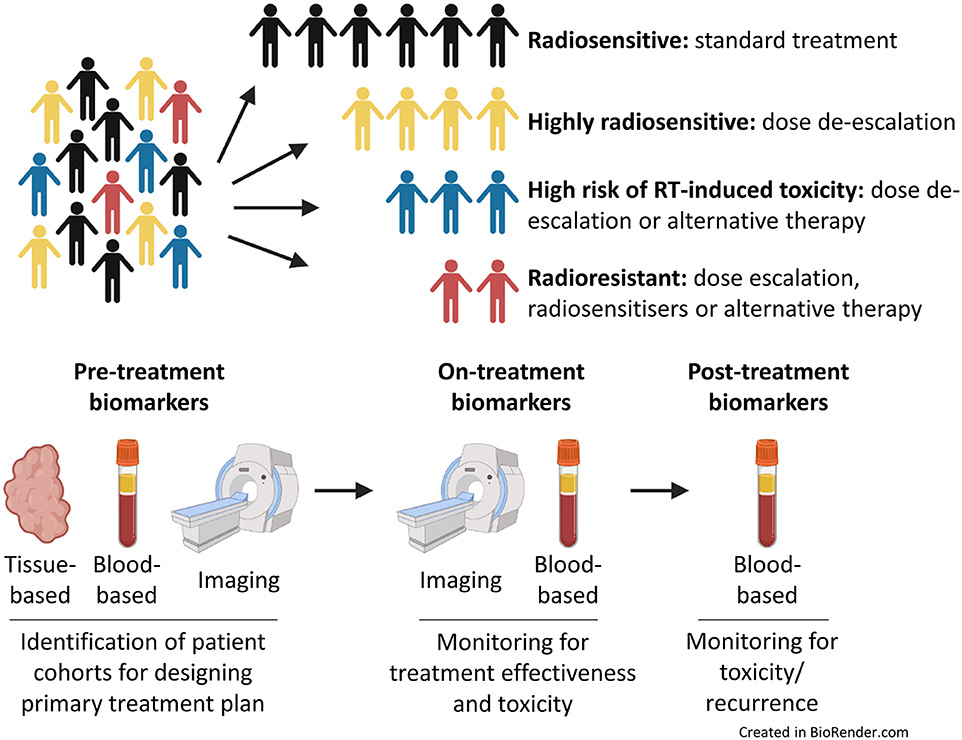
Figure 1. Precision medicine and radiotherapy. Patients could be stratified into different cohorts based on predicted intrinsic radiosensitivity and risk of toxicity. On-treatment monitoring may provide information on response to treatment, enabling adaptive changes to a patient's treatment to be made if necessary. Post-treatment biomarkers could be used to assess for evidence of toxicity, tumor recurrence or the development of metastatic disease.
Molecular Signatures of Radiosensitivity
Intrinsic Subtypes
Gene expression profiling and histopathological classification using the expression of estrogen receptor (ER), progesterone receptor (PgR) and human epidermal growth factor receptor 2 (HER2) can be used to classify BC into different subtypes. These subtypes can be broadly categorized into luminal, normal-like, HER2-overexpressing and triple negative breast cancer (TNBC) (36–39, 51). Gene expression profiling can stratify BC subtypes to a greater degree than histological assessment; however, as previously mentioned, this is often not feasible for use with large scale patient populations due to financial constraints (52). Both classification methods have confirmed the heterogeneous nature of BC which can lead to substantial differences in biology, pathogenesis, treatment response and patient outcome (39, 53, 54). Luminal subtypes (which correspond to ER+ BC) are associated with a more favorable prognosis, whereas HER2-overexpressing and TNBC subtypes are associated with significantly worse recurrence-free survival and overall survival.
BC subtyping has also been investigated for its use in predicting response to RT. The major advantage of using these subtyping markers in the selection of patients for RT is that testing for these markers using biopsy samples is now mandatory in the clinics, as they are used for selecting patients for hormonal or targeted therapies. In vitro studies using BC cell lines have shown that individual subtypes exhibit differential inherent sensitivities to radiation (55). Multiple clinical analyses have also shown that subtype is related to radiosensitivity; one large study reported that local recurrence for invasive BC treated with breast-conserving surgery followed by RT was 0.8% for luminal A, 1.5% for luminal B, 8.4% for HER2-overexpressing and 7.1% for TNBC (56). HER2-overexpressing and TNBC have also been associated with an increased risk of local recurrence and distant metastasis in combination with reduced overall survival in patients treated with post-mastectomy RT or RT alone (56–58). Improved overall survival after post-mastectomy RT has also been identified in ER+/ PgR+/HER2− patients, whereas no significant overall survival improvement was seen following the same treatment in ER−/ PgR−/HER2+ patients (58). Similar results were observed when using the Oncotype DX recurrence score to predict overall survival following post-mastectomy RT; this study suggested that low-risk patients, as determined by low OncotypeDX recurrence scores, had significantly improved overall survival following post-mastectomy RT compared to those low-risk patients that did not receive this treatment. In comparison, post-mastectomy RT was not of significant benefit to intermediate and high-risk patients. The authors suggested that OncotypeDX recurrence score may be a predictor of survival benefit from post-mastectomy RT (59). Furthermore, improved overall survival has been documented in patients with ER+/ PgR+/HER2− tumor who received post-mastectomy RT when compared with those who received no RT (58). This suggests that RT is particularly effective for breast cancers of the luminal phenotype.
Pan-Cancer Genomic Signatures
The first pan-cancer genomic radiosensitivity signature was developed using 35 cancer cell lines from the National Cancer Institute-60 (NCI-60) panel (60). Torres-Roca et al. (60) used gene expression data combined with the survival fraction of cells that received a dose of 2 Gy (SF2), an accepted experimental measure of cellular radiosensitivity, to develop a radiation classifier that could predict inherent radiosensitivity. Their results showed that the classifier successfully predicted SF2 values in 22 of 35 NCI-60 cell lines. The authors then went on to identify three novel genes (RbAp48, RGS19, and R5PIA) whose expression was correlated with radiation sensitivity. These results were the first to show that gene expression profiles had the potential to predict radiation sensitivity and that genomics could be used to identify novel radiosensitivity molecular markers. Unfortunately, subsequent studies have failed to reproduce these in vitro results using modern gene expression techniques; therefore, the validity of this signatures use in cell lines remains open to debate (61).
This pan-cancer genomic radiosensitivity signature has since been developed by the same group to include biological variables such as tissue of origin, p53 and ras status, known influencers of radiosensitivity. Using SF2 values from 48 cancer cell lines from the NCI-60 panel, gene expression analysis was performed to identify a 10 gene signature associated with intrinsic radiosensitivity (AR, cJun, STAT1, PKC, RelA, cABL, SUMO1, CDK1, HDAC1, and IRF1). These 10 genes are associated with specific pathways that included cell cycle, DNA damage response, histone deacetylation, proliferation and apoptosis. These results were used to produce a radiosensitivity index (RSI), whereby lower RSI correlates with greater radiosensitivity (62). The RSI has been used in clinical studies; these have shown the signature to be disease-site independent, predicting clinical outcomes in esophageal, rectal, head and neck, prostate, pancreas, colon, glioblastoma and non-small cell lung cancer patients following RT (62–66). However, some of these results were obtained from pilot studies which had low patient numbers, whereas others did not compare the results with non-RT treated controls. The RSI has also been evaluated in 2 independent BC patient cohorts. Results indicated that RT-treated patients classified as radiosensitive by RSI had improved recurrence-free survival or distant metastasis-free survival at 5 years, but there was no difference in recurrence-free survival or distant metastasis-free survival between radiosensitive and radioresistant patients in those not treated with RT. The authors suggested that RSI is RT-specific, because it was not prognostic for patients treated with surgery alone, but importantly can be used as a predictive signature of RT benefit. Furthermore, they demonstrated that the impact of RSI was affected by ER status, with RSI-classified radiosensitive patients having greater distant metastasis-free survival in ER+ patients (67). RSI has also been integrated with BC molecular subtyping to predict local recurrence; Torres-Roca et al. (68) showed that although RSI did not uniformly predict for local recurrence, it was able to identify a subpopulation of TNBC that had high RSI scores which were classified as radioresistant with the highest risk of local recurrence. They also illustrated that RSI could identify a luminal radioresistant subpopulation that would benefit from radiation dose escalation. They concluded that the combined use of RSI and molecular subtyping could help guide the selection of patients for RT treatment in BC (68).
In an attempt to tailor radiation doses across differing BC subtypes, researchers have integrated the RSI with the linear quadratic model to derive a genomic-adjusted radiation dose (GARD); this aimed to predict which tumors would gain an enhanced therapeutic effect from RT. GARD values were calculated for over 800 tumor samples using data from the prospective, observational Total Cancer Care cohort. The results demonstrated that there was a wide range of GARD values within tumor types and that GARD values could independently predict clinical outcome in lung, pancreatic, glioblastoma and BC patients (69). The limitations of this study included the failure to evaluate the prognostic potential of GARD (no data were evaluated for patients that did not receive RT) and a lack of external validation. However, this GARD-based clinical model could allow for RT personalisation based on radiation dose tailored to tumor radiosensitivity and may provide a means to develop genomically-guided RT-based clinical trials.
A similar approach which used the same NCI-60 cell line panel was undertaken to identify not only genes whose expression profiles were related to intrinsic radiosensitivity, but also genes whose expression changed following radiation treatment and were associated with post-radiation survival. Changes in gene expression induced by radiation were found to be similar between different tumor types and were also associated with p53 status. The authors suggested that there was possibly a conserved set of genes responsible for a specific radiation response (70). However, this work contradicts a more comprehensive study that profiled the radiation response of over 500 cell lines which showed sensitivity to radiation was characterized by significant genetic variation within and between cell line lineages. As well as identifying genes whose expression was associated with response, they also identified somatic copy number alterations and gene mutations that correlated with post-radiation survival (71).
In addition to radiation-induced gene response signatures, other groups have looked at signatures that may predict response to radiation treatment for a range of different cancer types. These gene expression profiles include hypoxia-related signatures (72–74), cell cycle and DNA damage gene-related signatures (75, 76), along with signatures predicting response to radiosensitising drugs (77, 78). As with many of the other in vitro derived signatures, none of these has thus far withstood stringent external validation and therefore have yet to be translated into clinical practice.
Breast Cancer Specific Genomic Signatures
With a technique similar to that employed to produce the RSI, a different study used only BC cell lines to develop a BC specific radiation sensitivity signature (RSS) (79). Their aim was to produce a gene signature that could predict the radiation response of BC patients and allow the identification of patients with tumors refractive to conventional RT regimens. To derive their gene signature, intrinsic radiosensitivity was correlated with gene expression using SF2 values from a panel of 16 BC cell lines. Interestingly, Speers et al. (79) found no association between intrinsic radiosensitivity of the BC cell lines and subtype classification, contradicting findings from previous publications. A 51 gene signature, enriched for pathways involved in DNA damage response and cell cycle, was developed from their results. Validation of this gene signature, the most promising to date, was performed in two independent clinical BC datasets in which patients had been treated with breast-conserving surgery and RT. The results showed that the RSS could provide information on which patients were likely to respond poorly to standard RT regimens (79). However, as all patients in the studied cohorts received standard RT, the 51 gene signature could not be validated for its predictive potential. This RSS has subsequently been marketed as RadiotypeDX and is regarded as having potentially similar applications to that of OncotypeDX, which is used for selecting the most appropriate systemic therapies for BC patients. RadiotypeDX is currently undergoing external validation, using tissue and clinical data from a randomized controlled trial evaluating post-operative RT after breast-conserving surgery in patients with early BC, who received appropriate adjuvant systemic therapy according to ER status (80). Although the results are yet to be published, these types of trials, as well as prospective randomized trials or “prospective retrospective” analyses from phase III trials, are essential in order to show that these kinds of signatures have clinical applications and that they could be integrated into clinical practice (81).
More recently a refined version of RadiotypeDX (Adjuvant Radiotherapy Intensification Classifier [ARTIC]) has been validated in a Swedish Conservation trial in which patients were randomized to post-operative whole-breast RT or no RT (82). Of note, a limited number of patients in this trial received systemic therapy. ARTIC was found to be highly prognostic for loco-regional recurrence and predictive of benefit from RT. Patients who had a low ARTIC score derived a substantial benefit from RT; in contrast, patients with high ARTIC scores had less benefit from RT. The authors recognized the requirement to validate ARTIC within a trial of patients treated by breast-conserving therapy, systemic therapy +/– post-operative whole-breast irradiation.
A gene profile called DBCG-RT, predictive for therapeutic benefit from post-mastectomy RT and prognostic for locoregional control, was developed by the Danish Breast Cancer Cooperative Group and has been independently validated (83). The DBCG-RT gene profile can divide patients into those with a high- and low-risk of local recurrence and can identify a subcategory of low-risk patients who obtain no additional benefit from RT. A correlation between patients that were at an increased risk of developing local recurrence and non-luminal, ER− tumors (basal and HER2-overexpressing) was observed, while the low local recurrence risk group correlated with the luminal A subtype. These findings indicate that intrinsic subtyping and the DBCG-RT profile can identify the same tumor types and their responses to RT treatment.
Others have attempted to develop specific radiation signatures for BC that distinguish patients who require treatment intensification, for whom traditional therapies (surgery, chemotherapy and RT) are inadequate. Gene analysis has been conducted on early stage BC patients, all treated with RT after surgery, to try and distinguish gene signatures prognostic of local recurrence in patients treated with radiation. A radiation signature consisting of 81 genes outperformed pathologic and clinical characteristics for predicting local recurrence (84). A similar study performed gene expression microarray profiling to identify differentially expressed genes between tumors from patients who developed local recurrence after breast-conserving surgery and radiation, and patients who did not; Kreike et al. (85) derived a 111 gene signature, enriched for cell proliferation, which was independently associated with local recurrence. Unfortunately, both of these signatures failed external validation.
The Early Breast Cancer Trialists' Collaborative Group meta-analysis suggested that most early stage BC patients treated with breast-conserving surgery are cured of their disease with both surgery and endocrine therapy alone, without the need for RT (9). Studies have also suggested that RT may be omitted in selected elderly patients with low-risk disease (86). RT omission after breast-conserving surgery has also been explored in several randomized phase III trials; unfortunately, heterogeneous eligibility criteria across the trials has resulted in differing results with confounding interpretations (87). These types of studies have led to an increased interest in the development of radiation omission signatures specific for BC, whereby low-risk patients can be spared RT. An omission signature has yet to be developed; however, different groups are attempting to validate a variety of previously described molecular classifiers for this purpose. Trials are ongoing to assess the potential of OncotypeDx (IDEA trial), Prosigna (PRECISION trial), IHC (LUMINA trial), and IHC4 (PRIMETIME trial) scores as stratification methods for radiation omission (81).
Barriers to Clinical Adoption of Molecular Signatures
Although many molecular signatures have been developed to predict a tumor's response to radiation, most have failed external validation and, as a result, none have gained approval for clinical use. In part, as indicated earlier, the barriers are the high costs of academic-industry collaborations and in part scientific (50). Unfortunately, the gene profiles derived from the studies outlined in this review show little similarity to each other, which suggests that the methods used to produce the signatures may influence which genes and pathways are selected. Like many other cancers, BC is a complex heterogenous disease; differences in pathway activation can lead to different drivers of oncogenesis, even within the same subtype. These significant differences will influence how each tumor reacts to RT; it is possible that gene signatures are unable to account for the complexity of a tumor's radiation response. These issues are likely to be more pronounced in profiles derived from cell lines, as these are clonal populations that cannot account for tumor heterogeneity and the effects of the tumor microenvironment. Validation of the signatures using clinical trial data is also complicated; variations between trials in terms of the radiation treatment regimens used, inclusion of different patient subtypes and inconsistencies between the numbers of treated and control patients included in the studies makes the generation of meaningful data and rigid signature validation challenging. The methods used for RNA extraction and subsequent gene expression analysis can also differ between the original studies which developed the profiles and the clinical trials in which they are being validated. These issues are specific for gene signature validation; there are also more general hurdles that must be overcome if lab-based research is to be successfully translated into a clinically applicable test. These include the development of standard operating procedures and making tests cost effective and easy to use, while also providing evidence that they can improve upon standard practices already in place in the clinics. The combination of these gene signature specific and general issues are factors that are currently contributing to their lack of clinical translation and use in the clinic.
Gene Mutations, mRNA and Intracellular Protein Markers
Several studies have looked at individual biomarkers, rather than cohorts of genes, for their potential to correlate with a tumor's response to RT. In BC, expression levels of Holliday junction recognition protein mRNA can be prognostic for disease-free survival and overall survival, thereby predicting patient sensitivity to RT (88). High cytoplasmic expression of peroxiredoxin-I has also been shown to correlate with increased local recurrence after RT (89). Proteasome (prosome, macropain) 26S subunit, non-ATPase, 9 (PSMD9) is another protein whose elevated expression was associated with increased incidence of local recurrence in a cohort of patients that received adjuvant RT, but not in those that did not receive RT; the authors concluded that this protein might therefore be a predictive biomarker for RT response (52).
The BRCA1/2 genes are part of the granin gene family and function as tumor suppressors; they play critical roles in maintaining genome stability through controlling pathways involved in DNA damage response/repair, cell cycle and transcription (90). Previous studies have shown that BRCA1/2 gene deregulation is associated with BC carcinogenesis (91), with germline BRCA1/2 mutations accounting for up to 10% of all cases (92). Inherited BRCA1/2 mutations are estimated to increase the risk of BC by to 84% (93–95). These tumors exhibit ineffective homologous recombination DNA repair, causing an accumulation of genetic mutations, which can drive carcinogenesis. Although tumors deficient in a DNA repair mechanism may be expected to exhibit radiation sensitivity, they are instead thought to rely upon alternative DNA repair mechanisms that are more effective at repairing radiation-induced DNA damage. PARP enzymes play a key role in the repair of DNA single-strand breaks through the repair of base excisions, a process normally performed by error-free homologous recombination (96). The inhibition of PARPs can result in the accumulation of DNA single-strand breaks, leading to the generation of double-strand breaks at replication forks. Double-strand breaks are usually repaired by error-free homologous recombination, a process that is inhibited in BRCA1/2 mutated tumors; these double-strand breaks can lead to apoptosis of cancer cells within the tumor. The PARP enzyme inhibitor olaparib is currently undergoing trials to assess its clinical usefulness and cost effectiveness in the treatment of BRCA1/2 mutated HER2− metastatic BC patients following chemotherapy (97). This drug has previously gained clinical approval for use in patients with recurrent, platinum-sensitive, BRCA1/2 mutated ovarian cancers (98). Furthermore, the small molecular PARP inhibitor niraparib has been shown to radiosensitise a variety of human xenograft tumors, including the triple-negative MDA-MB-231 human BC cell line (99). It has therefore been suggested that BRCA status could be a useful biomarker for stratifying patients for the use of PARP inhibitors in combination with RT.
Blood-Associated Biomarkers of Tumor Radiosensitivity
The identification of circulating prognostic or predictive biomarkers in the blood has advantages over tissue-based approaches as it is non-invasive and does not require a biopsy. These liquid biopsies can be taken pre-, post- or on-treatment, allowing for continual patient monitoring with the potential to assess the tumors response to treatment. These biomarkers could hold particular promise in patients with metastatic disease, where monitoring for progression is critical but where repeated biopsy sampling is often unfeasible due to the location of the lesions. Studies have investigated the use of blood based biomarkers, such as carcinoembryonic antigen and carbohydrate antigen 15–3 for primary diagnosis and the detection of metastatic disease (100–105), with others examining the correlation between serum HER2 concentration and tumor HER2 status (106–109). Although the use of blood-based biomarkers to assess tumor pre-treatment radiosensitivity or on-treatment response to RT has been less intensively studied, there is a growing interest in the use of these types of biomarkers for precision RT. Areas of research currently under investigation include exosomes and circulating tumor cells (CTCs).
Exosomes are formed from the inward budding of the membrane of multi-vesicular bodies and are ~40–100 nm in diameter. The contents of exosomes are released from cells via endocytosis through fusion of their membrane with that of the cell's plasma membrane. Exosomes have been shown to contain nucleic acids, proteins, lipids and enzymes (110). There is increasing evidence that exosomes play roles in tumorigenesis and cancer progression, including immune suppression, angiogenesis, cell migration and invasion (111–115); as a result, their use as liquid biopsy biomarkers through exosomal profiling is being investigated for disease diagnosis and therapy efficacy monitoring. The transfer of exosome contents to local tumor or stromal cells in the tumor microenvironment, or to a distant site within the body, has been shown to be a mechanism through which cancer cells can transmit the malignant phenotype to normal cells and establish a suitable environment for metastatic colonization (113). Exosomes transferred from stromal to BC cells can also contribute to chemotherapy and radiotherapy resistance. Resistance mechanisms mediated by exosomal transfer are thought to involve anti-viral and NOTCH3 pathways (116). Tumor oxygen concentrations at the time of radiation have been shown to influence cell radiosensitivity (117). When exposed to a given dose of radiation, cancer cells in low oxygen states can withstand 2–3 times higher doses than aerobic cells. Known as the oxygen enhancement effect, the role that oxygen plays in RT is described through the oxygen fixation hypothesis (118, 119). Oxygen present at the time of RT can react with radiation-induced DNA radicals producing permanent DNA damage; however, in the absence of oxygen this damage can be repaired by free radical scavengers such as endogenous thiols (120), giving these hypoxic cells a significant survival advantage. Hypoxia leads to the increased production of exosomes (121, 122), with the transfer of exosomes from stromal to BC cells stimulating signaling pathways related to radiation resistance (116). The tumor-associated exosome profile may also give an indication of the oxygenation status of breast tumors, and therefore could be used to identify radioresistant tumors (122).
CTCs and circulating tumor DNA are cells or DNA that have been shed by the tumor into the systemic circulation; these can be indicators of residual disease following treatment and are likely to represent an important mechanism through which a tumor can metastasise (123, 124). CTCs have been detected in up to 30% of non-metastatic BC patients and their presence (even just one CTC) has been shown to be prognostic for recurrence-free survival and overall survival (125–135). CTC detection is also related to metastasis and poor survival in low-risk patients with lymph node negative disease who did not receive adjuvant therapy (133). Data from the National Cancer Database and SUCCESS (Simultaneous Study of Gemcitabine-Docetaxel Combination Adjuvant Treatment as well as Extended Bisphosphonate and Surveillance) clinical trials have been used to investigate the use of CTC status to predict the benefit of RT in early stage BC (136). The results suggested that CTC status could predict the effectiveness of RT and showed that CTC+ patients had improved local recurrence-free survival and disease-free survival following RT, whereas CTC− patients obtained no benefit. Furthermore, CTC+ patients that received RT had improved overall survival compared with CTC+ patients who did not receive RT. Pooled analysis using both cohorts who underwent breast-conserving surgery indicated that RT was associated with longer overall survival in CTC+ patients, but not in CTC− patients. However, CTC status was not associated with an overall survival benefit in patients who underwent mastectomy and RT. Overall, these results suggested that CTC status may be used as a predictive marker for assessing the potential benefit from incorporating RT into the treatment of early stage BC for patients undergoing breast-conserving surgery (136, 137).
Although circulating tumor biomarkers have the potential to be used for cancer diagnosis, measuring response to treatment and monitoring for recurrence, studies evaluating their clinical usefulness are still limited in number. While advances have been made in this field, circulating tumor biomarkers in BC patients are not yet employed for primary diagnosis, largely due to their low sensitivity and specificity, and also because of a lack of validation through large prospective trials. Such trials are essential to fully evaluate their prognostic or predictive potential in determining tumor radiosensitivity and to enable their use in the future (138).
Biomarkers of Radiation-Induced Toxicity
In contrast to the relatively limited research involving blood-based biomarkers for tumor radiosensitivity, the use of circulating biomarkers to assess radiation-induced toxicities has attracted much greater interest. Like any cancer treatment, RT has the potential to cause toxic side effects in normal tissues; these ultimately dictate the total dose that can be delivered to a patient, which can influence outcome. In BC, radiation-associated side effects include cardiac and skin toxicities, fibrosis, lymphedema, secondary cancers, rib fractures and brachial plexopathy (139). In some studies the combination of tamoxifen and RT has resulted in an increased risk of fibrosis, hypothesized to be due to a tamoxifen-induced increase in TGF-β (140–144). Even with highly conformal intensity-modulated RT and image-guided RT, which aim to spare regional organs from radiation exposure, these side effects can occur in up to 15% of patients and can seriously affect patients' physiological and physical quality of life (145, 146). Taking into account the high survival rate of BC patients, there is a need to develop tests that can be used either pre-treatment, or at an early stage of the treatment process, to predict which patients are at a higher risk of developing radiation-associated side effects (147). Predicting radiation-induced toxicity could enable better treatment regimens to be devised for individual patients in a range of cancer types. Radiation toxicity is related to treatment schedule (dose and duration), patient specific factors and genetic factors. With these in mind, several tests have been proposed to classify individual patient radiosensitivity based on the induction of DNA damage and radiation-induced apoptosis, in addition to gene expression profiles (148).
One area of research that holds promise in predicting the risk of late radiation-associated toxicity is through assessment of the radiation-induced initial DNA damage response of peripheral blood lymphocytes through DNA double strand break quantification, comet or micronucleus assays (148). A relationship between high rates of double strand breaks in peripheral blood lymphocytes and late grade 3 skin and subcutaneous tissue toxicities in BC patients has suggested that assessing the initial DNA damage could be useful for predicting radiation toxicity (149). Unfortunately, various studies assessing the radiation-induced DNA damage through other methods have shown both negative (150, 151) and positive (152–154) associations with radiation toxicity. Additionally, the clinical usefulness of radiation-induced DNA damage response assays is yet to be fully determined. Determining peripheral blood lymphocyte radiation-induced apoptosis appears to be the most promising assay for determining radiation toxicity. Studies have shown that radiation-induced apoptosis increases with higher doses of radiation; however, elevated levels of radiation-induced apoptosis were associated with reduced risk of late radiation toxicities (155). In BC patients, studies have used a combination of DNA damage assessment and levels of radiation-induced apoptosis to assess radiation-induced toxicity. Henríquez-Hernández et al. (156) demonstrated that patients showing lower levels of initial DNA damage and higher levels of radiation-induced apoptosis were considered resistant to RT. These patients were at a lower risk of suffering severe subcutaneous late toxicities after treatment with high radiation doses. Although these results were based on a small number of patients, it provided evidence that dose escalation can be achieved in patients that are resistant and tolerant to higher radiation doses.
There is an association between the dose of radiation received by the heart and increased risk of heart disease (157–159); as the dose increases so does the risk of developing heart complications. Cardiovascular side effects are a major concern for clinicians treating BC patients with RT (160, 161), especially for left sided BC or patients with pre-existing cardiovascular disease. Cardiotoxic effects of RT can be seen for several years after RT, and increased risk may remain for at least 2 years post-RT (161). Pericarditis, valvular dysfunction, cardiomyopathy and coronary artery disease are some of the severe late cardiotoxic effects seen in up to 30% of BC cases within 5–10 years following RT (162). Blood biomarkers have been investigated for their roles in detecting and monitoring cardiotoxicity and assessing early signs of cardiovascular dysfunction following RT. Brain natriuretic peptide and its amino terminal fragment are biomarkers produced by ventricular myocytes, with elevated blood levels commonly seen in unstable angina, myocardial infarction and cardiac failure (163). Several studies have shown that levels of these biomarkers increase after RT, suggesting that these may be also be biomarkers of early radiation-induced cardiotoxicity (164–166). Circulating cardiac troponin I and cardiac troponin T are highly sensitive and specific biomarkers for cardiac disease and have been shown to be useful for detecting cardiotoxicity following chemotherapy (167, 168) and, although some studies indicate they do not increase following RT (165, 169), others show that they do (164, 170, 171). While these traditional cardiac biomarkers are the most extensively studied for cardiotoxicity following RT, other blood biomarkers that have been investigated include C-reactive protein and lipopolysaccharide. C-reactive protein, an acute phase protein whose expression is related to inflammatory cytokines, has been proposed as a biomarker of radiation-associated cytotoxicity as studies have highlighted an association between elevated C-reactive protein levels and adverse prognosis in patients with heart failure (172–174). Although Lipshultz et al. (166) found increased levels of C-reactive protein in children following treatment for a variety of cancers with chemotherapy and RT, the majority of studies have failed to find an association between circulating C-reactive protein levels and myocardial damage post-chemotherapy (175–177) or RT (178). Lipopolysaccharide-binding protein has been investigated as a potential biomarker for lung toxicity after chest RT (179). In BC, lipopolysaccharide-binding protein concentrations were observed to increase within 24 h post-RT and remained elevated 1 month after RT. Raised lipopolysaccharide-binding protein levels were also correlated with cardiac dysfunction, which was evaluated up to 3 years following the completion of RT, suggesting that lipopolysaccharide-binding protein could be a potentially useful prognostic biomarker of RT-induced cardiotoxicity (178). Characteristics such as early detection in the blood and persistent kinetics, in combination with concentrations being related to toxicity, have made lipopolysaccharide-binding protein an attractive biomarker for clinical use. Other proposed biomarkers include heart-type fatty acid-binding protein, glycogen phosphorylase isoenzyme BB, myeloperoxidase and nitric oxide (180). Although these biomarkers have yet to be used clinically, they could in the future play key roles in determining which RT patients may require dose de-escalation, or even the provision of alternative treatments if BC patients can be classified as high-risk for developing radiation-induced side effects post-treatment.
A summary of the techniques used to identify biomarkers of radiosensitivity that have been described in this paper is provided in Figure 2 and Table 1. Table 1 also outlines the specific advantages and disadvantages associated with each method.
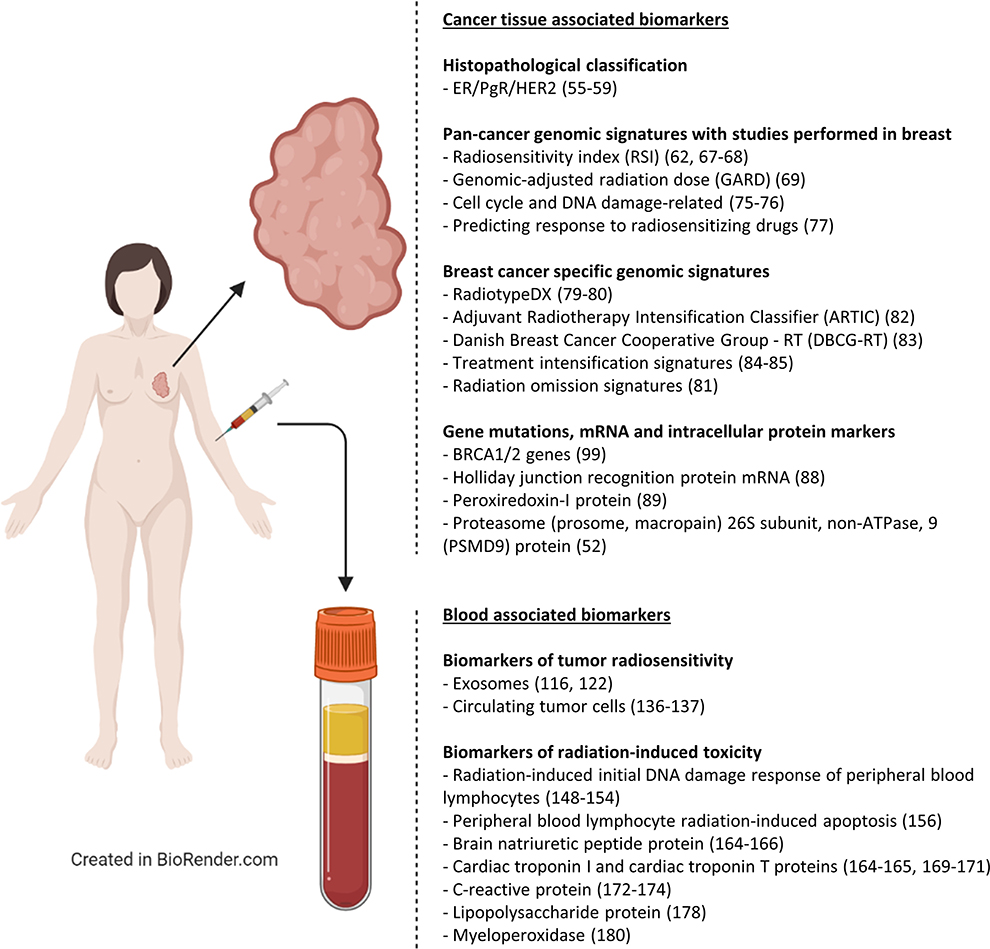
Figure 2. Biomarkers of radiotherapy response for breast cancer. An overview of cancer tissue-associated and blood-associated biomarkers of radiotherapy response that have been developed for breast cancer patients (ER, Estrogen receptor; PgR, Progesterone receptor; HER2, human epidermal growth factor receptor 2).
Conclusion
Significant advances have been made in the development of molecular signatures to stratify BC patients for more personalized targeted and endocrine therapies; however, similar improvements in the field of personalized RT have yet to be adopted in the clinic. Technological developments in the methods used for radiation delivery have allowed radiation oncologists to accurately conform radiation to the tumor; however, only limited methods of analyzing response to treatment are available. To truly achieve personalized RT, we also need to be able to stratify patient's pre-treatment based on individual patient radiosensitivity and through analyzing the tumor's response to RT during treatment. Unfortunately, there are currently no clinically-validated prognostic or predictive signatures/biomarkers that can reliably classify patients into those that would benefit the most from RT, those that could be safely treated with dose escalation or de-escalation, or those that should be treated without RT. While preliminary efforts to develop these signatures/biomarkers have been encouraging, there is still much work to do in order to refine and validate them. Ultimately, for any of these tests to be translated into the clinic, studies will need to demonstrate their accuracy and reproducibility, and perhaps more importantly, exhibit their utility in improving outcomes or refining the selection of patients for RT through clinical trials. While not yet realized, the ongoing development of these signatures and biomarkers holds much promise; the linking of these signatures and biomarkers with other techniques, such as imaging, would help deliver an overall precision medicine package that could greatly enhance the effectiveness of RT. There is confidence within the scientific community that personalized medicine will finally be realized for BC patients undergoing radiation treatment in the decades to come.
Author Contributions
JM, MG, DA, and AT conceptualized the article. JM wrote the majority of the manuscript. Figures and tables were composed by JM and MG. Critical revisions were made by JM, MG, CM-P, CK, LP, JAF, AVP, IK, SPL, DA, and AT. All authors read and approved the final manuscript.
Funding
This work was supported by the Breast Cancer Institute Fund (S03181) from the Edinburgh Lothian Health Foundation.
Conflict of Interest
The authors declare that the research was conducted in the absence of any commercial or financial relationships that could be construed as a potential conflict of interest.
References
1. Bray F, Ferlay J, Soerjomataram I, Siegel RL, Torre LA, Jemal A. Global cancer statistics 2018: GLOBOCAN estimates of incidence and mortality worldwide for 36 cancers in 185 countries. Cancer J Clin. (2018) 68:394–424. doi: 10.3322/caac.21492
2. Bray F, Jemal A, Grey N, Ferlay J, Forman D. Global cancer transitions according to the Human Development Index (2008–2030): a population-based study. Lancet Oncol. (2012) 13:790–801. doi: 10.1016/S1470-2045(12)70211-5
3. Siegel R, DeSantis C, Virgo K, Stein K, Mariotto A, Smith T, et al. Cancer treatment and survivorship statistics, 2012. Cancer J Clin. (2012) 62:220–41. doi: 10.3322/caac.21149
4. Haviland JS, Owen JR, Dewar JA, Agrawal RK, Barrett J, Barrett-Lee PJ, et al. The UK standardisation of breast radiotherapy (START) trials of radiotherapy hypofractionation for treatment of early breast cancer: 10-year follow-up results of two randomised controlled trials. Lancet Oncol. (2013) 14:1086–94. doi: 10.1016/S1470-2045(13)70386-3
5. Nitsche M, Dunst J, Carl UM, Hermann RM. Emerging role of hypofractionated radiotherapy with simultaneous integrated boost in modern radiotherapy of breast cancer. Breast Care. (2015) 10:320–4. doi: 10.1159/000436951
6. Bartelink H, Maingon P, Poortmans P, Weltens C, Fourquet A, Jager J, et al. Whole-breast irradiation with or without a boost for patients treated with breast-conserving surgery for early breast cancer: 20-year follow-up of a randomised phase 3 trial. Lancet Oncol. (2015) 16:47–56. doi: 10.1016/S1470-2045(14)71156-8
7. Kindts I, Laenen A, Depuydt T, Weltens C. Tumour bed boost radiotherapy for women after breast-conserving surgery. Cochrane Database Syst Rev. (2017) 11:CD011987. doi: 10.1002/14651858.CD011987.pub2
8. Janssen S, Glanzmann C, Lang S, Verlaan S, Streller T, Wisler D, et al. Hypofractionated radiotherapy for breast cancer acceleration of the START A treatment regime: intermediate tolerance and efficacy. Radiat Oncol. (2014) 9:165. doi: 10.1186/1748-717X-9-165
9. E.B.C. Group TC. Effect of radiotherapy after breast-conserving surgery on 10-year recurrence and 15-year breast cancer death: meta-analysis of individual patient data for 10 801 women in 17 randomised trials. Lancet. (2011) 378:1707–16. doi: 10.1016/S0140-6736(11)61629-2
10. Strnad V, Ott OJ, Hildebrandt G, Kauer-Dorner D, Knauerhase H, Major T, et al. 5-year results of accelerated partial breast irradiation using sole interstitial multicatheter brachytherapy versus whole-breast irradiation with boost after breast-conserving surgery for low-risk invasive and in-situ carcinoma of the female breast: a randomised, phase 3, non-inferiority trial. Lancet. (2016) 387:229–38. doi: 10.1016/S0140-6736(15)00471-7
11. Livi L, Buonamici FB, Simontacchi G, Scotti V, Fambrini M, Compagnucci A, et al. Accelerated partial breast irradiation with IMRT: new technical approach and interim analysis of acute toxicity in a phase iii randomized clinical trial. Int J Radiat Oncol Biol Phys. (2010) 77:509–15. doi: 10.1016/j.ijrobp.2009.04.070
12. Aristei C, Maranzano E, Lancellotta V, Chirico L, Zucchetti C, Italiani M, et al. Partial breast irradiation with interstitial multi-catheter high-dose-rate brachytherapy. Long-term results of a phase II prospective study. Radiother Oncol. (2017) 124:208–13. doi: 10.1016/j.radonc.2017.07.015
13. Tagliaferri L, Lancellotta V, Colloca G, Marazzi F, Masiello V, Garganese G, et al. Could a personalized strategy using accelerated partial breast irradiation be an advantage for elderly patients? A systematic review of the literature and multidisciplinary opinion. J Oncol. (2020) 2020:3928976. doi: 10.1155/2020/3928976
14. Lancellotta V, Kovács G, Tagliaferri L, Perrucci E, Colloca G, Valentini V, et al. Age is not a limiting factor in interventional radiotherapy (brachytherapy) for patients with localized cancer. BioMed Res Int. (2018) 2018:2178469. doi: 10.1155/2018/2178469
15. Baumann M, Krause M, Overgaard J, Debus J, Bentzen SM, Daartz J, et al. Radiation oncology in the era of precision medicine. Nat Rev Cancer. (2016) 16:234–49. doi: 10.1038/nrc.2016.18
16. National Cancer Registration & Analysis Service and Cancer Research UK. Chemotherapy, Radiotherapy and Tumour Resections in England: 2013–2014. London: NCRAS London (2017).
17. McGale P, Taylor C, Correa C, Cutter D, Duane F, Ewertz M, et al. Effect of radiotherapy after mastectomy and axillary surgery on 10-year recurrence and 20-year breast cancer mortality: meta-analysis of individual patient data for 8135 women in 22 randomised trials. Lancet. (2014) 383:2127–2135. doi: 10.1016/S0140-6736(14)60488-8
18. Delaney G, Jacob S, Featherstone C, Barton M. The role of radiotherapy in cancer treatment: estimating optimal utilization from a review of evidence-based clinical guidelines. Cancer. (2005) 104:1129–37. doi: 10.1002/cncr.21324
19. Onitilo AA, Engel JM, Stankowski RV, Doi SA. Survival comparisons for breast conserving surgery and mastectomy revisited: community experience and the role of radiation therapy. Clin Med Res. (2015) 13:65–73. doi: 10.3121/cmr.2014.1245
20. Cao J, Olson R, Tyldesley S, Comparison of recurrence and survival rates after breast-conserving therapy and mastectomy in young women with breast cancer. Curr Oncol. (2013) 20:593–601. doi: 10.3747/co.20.1543
21. Poortmans P. Evidence based radiation oncology: breast cancer. Radiother Oncol. (2007) 84:84–101. doi: 10.1016/j.radonc.2007.06.002
22. Allemani C, Sant M, Weir HK, Richardson LC, Baili P, Storm H, et al. Breast cancer survival in the US and Europe: A CONCORD high-resolution study. Int J Cancer. (2013) 132:1170–81. doi: 10.1002/ijc.27725
23. Eisenhauer EA, Therasse P, Bogaerts J, Schwartz LH, Sargent D, Ford R, et al. New response evaluation criteria in solid tumours: revised RECIST guideline (version 1.1). Eur J Cancer. (2009) 45:228–47. doi: 10.1016/j.ejca.2008.10.026
24. Ghasemi M, Nabipour I, Omrani A, Alipour Z, Assadi M. Precision medicine and molecular imaging: new targeted approaches toward cancer therapeutic and diagnosis. Am J Nuclear Med Mol Imaging. (2016) 6:310–27.
25. Penet MF, Krishnamachary B, Chen Z, Jin J, Bhujwalla ZM. Molecular imaging of the tumor microenvironment for precision medicine and theranostics. Adv Cancer Res. (2014) 124:235–56. doi: 10.1016/B978-0-12-411638-2.00007-0
26. Burrell RA, McGranahan N, Bartek J, Swanton C. The causes and consequences of genetic heterogeneity in cancer evolution. Nature. (2013) 501:338–45. doi: 10.1038/nature12625
27. Hanahan D, Weinberg RA. The Hallmarks of Cancer. Cell. (2000) 100:57–70. doi: 10.1016/S0092-8674(00)81683-9
28. Hanahan D, Weinberg RA. Hallmarks of cancer: the next generation. Cell. (2011) 144:646–74. doi: 10.1016/j.cell.2011.02.013
29. Atkinson AJ Jr, Colburn WA, DeGruttola VG, DeMets DL, Downing GJ, Hoth DF, et al. Biomarkers and surrogate endpoints: preferred definitions and conceptual framework. Clin Pharmacol Ther. (2001) 69:89–95. doi: 10.1067/mcp.2001.113989
30. Mandrekar SJ, Sargent DJ. Clinical trial designs for predictive biomarker validation: theoretical considerations and practical challenges. J Clin Oncol. (2009) 27:4027–34. doi: 10.1200/JCO.2009.22.3701
31. Polley MYC, Freidlin B, Korn EL, Conley BA, Abrams JS, McShane LM. Statistical and practical considerations for clinical evaluation of predictive biomarkers. J Natl Cancer Inst. (2013) 105:1677–83. doi: 10.1093/jnci/djt282
32. Ludwig JA, Weinstein JN. Biomarkers in cancer staging, prognosis and treatment selection. Nat Rev Cancer. (2005) 5:845–56. doi: 10.1038/nrc1739
33. Valdora F, Houssami N, Rossi F, Calabrese M, Tagliafico AS. Rapid review: radiomics and breast cancer. Breast Cancer Res Treat. (2018) 169:217–29. doi: 10.1007/s10549-018-4675-4
34. Guo W, Li H, Zhu Y, Lan L, Yang S, Drukker K, et al. Prediction of clinical phenotypes in invasive breast carcinomas from the integration of radiomics and genomics data. J Med Imaging. (2015) 2:041007. doi: 10.1117/1.JMI.2.4.041007
35. Bickelhaupt S, Paech D, Kickingereder P, Steudle F, Lederer W, Daniel H, et al. Prediction of malignancy by a radiomic signature from contrast agent-free diffusion MRI in suspicious breast lesions found on screening mammography. J Magn ResonImaging. (2017) 46:604–16. doi: 10.1002/jmri.25606
36. Perou CM, Sorlie T, Eisen MB, van de Rijn M, Jeffrey SS, Rees CA, et al. Molecular portraits of human breast tumours. Nature. (2000) 406:747–52. doi: 10.1038/35021093
37. Sorlie T, Perou CM, Tibshirani R, Aas T, Geisler S, Johnsen H, et al. Gene expression patterns of breast carcinomas distinguish tumor subclasses with clinical implications. Proc Natl Acad Sci USA. (2001) 98:10869–74. doi: 10.1073/pnas.191367098
38. Sorlie T, Tibshirani R, Parker J, Hastie T, Marron JS, Nobel A, et al. Repeated observation of breast tumor subtypes in independent gene expression data sets. Proc Natl Acad Sci USA. (2003) 100:8418–23. doi: 10.1073/pnas.0932692100
39. Sotiriou C, Neo SY, McShane LM, Korn EL, Long PM, Jazaeri A, et al. Breast cancer classification and prognosis based on gene expression profiles from a population-based study. Proc Natl Acad Sci USA. (2003) 100:10393–8. doi: 10.1073/pnas.1732912100
40. Markopoulos C, van de Velde C, Zarca D, Ozmen V, Masetti R. Clinical evidence supporting genomic tests in early breast cancer: do all genomic tests provide the same information? Eur J Surg Oncol. (2017) 43:909–20. doi: 10.1016/j.ejso.2016.08.012
41. Sgroi DC, Sestak I, Cuzick J, Zhang Y, Schnabel CA, Schroeder B, et al. Prediction of late distant recurrence in patients with oestrogen-receptor-positive breast cancer: a prospective comparison of the breast-cancer index (BCI) assay, 21-gene recurrence score, and IHC4 in the TransATAC study population. Lancet Oncol. (2013) 14:1067–76. doi: 10.1016/S1470-2045(13)70387-5
42. Dubsky P, Brase J, Jakesz R, Rudas M, Singer C, Greil R, et al. The EndoPredict score provides prognostic information on late distant metastases in ER+/HER2– breast cancer patients. Br J Cancer. (2013) 109:2959–64. doi: 10.1038/bjc.2013.671
43. Paik S, Shak S, Tang G, Kim C, Baker J, Cronin M, et al. A multigene assay to predict recurrence of tamoxifen-treated, node-negative breast cancer. N Engl J Med. (2004) 351:2817–26. doi: 10.1056/NEJMoa041588
44. Tutt A, Wang A, Rowland C, Gillett C, Lau K, Chew K, et al. Risk estimation of distant metastasis in node-negative, estrogen receptor-positive breast cancer patients using an RT-PCR based prognostic expression signature. BMC Cancer. (2008) 8:339. doi: 10.1186/1471-2407-8-339
45. Van De Vijver MJ, He YD, Van't Veer LJ, Dai H, Hart AA, Voskuil DW, et al. A gene-expression signature as a predictor of survival in breast cancer. N Engl J Med. (2002) 347:1999–2009. doi: 10.1056/NEJMoa021967
46. Parker JS, Mullins M, Cheang MC, Leung S, Voduc D, Vickery T, et al. Supervised risk predictor of breast cancer based on intrinsic subtypes. J Clin Oncol. (2009) 27:1160–7. doi: 10.1200/JCO.2008.18.1370
47. Ellis MJ, Suman VJ, Hoog J, Lin L, Snider J, Prat A, et al. Randomized phase II neoadjuvant comparison between letrozole, anastrozole, and exemestane for postmenopausal women with estrogen receptor–rich stage 2 to 3 breast cancer: clinical and biomarker outcomes and predictive value of the baseline PAM50-based intrinsic subtype—ACOSOG Z1031. J Clin Oncol. (2011) 29:2342–9. doi: 10.1200/JCO.2010.31.6950
48. Bernier J. Precision medicine for early breast cancer radiotherapy: opening up new horizons? Crit Rev Oncol Hematol. (2017) 113:79–82. doi: 10.1016/j.critrevonc.2017.03.015
49. Forker LJ, Choudhury A, Kiltie A. Biomarkers of tumour radiosensitivity and predicting benefit from radiotherapy. Clin Oncol. (2015) 27:561–9. doi: 10.1016/j.clon.2015.06.002
50. Hall WA, Bergom C, Thompson RF, Baschnagel AM, Vijayakumar S, Willers H, et al. Precision oncology and genomically guided radiation therapy: a report from the American Society for radiation oncology/American association of physicists in medicine/national cancer institute precision medicine conference. Int J Radiat Oncol Biol Phys. (2018) 101:274–84. doi: 10.1016/j.ijrobp.2017.05.044
51. Brenton JD, Carey LA, Ahmed AA, Caldas C. Molecular classification and molecular forecasting of breast cancer: ready for clinical application? J Clin Oncol. (2005) 23:7350–60. doi: 10.1200/JCO.2005.03.3845
52. Langlands F, Horgan K, Dodwell D, Smith L. Breast cancer subtypes: response to radiotherapy and potential radiosensitisation. Br J Radiol. (2013) 86:20120601. doi: 10.1259/bjr.20120601
53. Carey LA, Perou CM, Livasy CA, Dressler LG, Cowan D, Conway K, et al. Race, breast cancer subtypes, and survival in the Carolina breast cancer study. JAMA. (2006) 295:2492–502. doi: 10.1001/jama.295.21.2492
54. Wang Y, Yin Q, Yu Q, Zhang J, Liu Z, Wang S, et al. A retrospective study of breast cancer subtypes: the risk of relapse and the relations with treatments. Breast Cancer Res Treat. (2011) 130:489–98. doi: 10.1007/s10549-011-1709-6
55. Smith L, Qutob O, Watson MB, Beavis AW, Potts D, Welham KJ, et al. Proteomic identification of putative biomarkers of radiotherapy resistance: a possible role for the 26S proteasome? Neoplasia. (2009) 11:1194–207. doi: 10.1593/neo.09902
56. Nguyen PL, Taghian AG, Katz MS, Niemierko A, Abi Raad RF, Boon WL, et al. Breast cancer subtype approximated by estrogen receptor, progesterone receptor, and HER-2 is associated with local and distant recurrence after breast-conserving therapy. J Clin Oncol. (2008) 26:2373–8. doi: 10.1200/JCO.2007.14.4287
57. Stål O, Sullivan S, Wingren S, Skoog L, Rutqvist L, Carstensen J, et al. c-erbB-2 expression and benefit from adjuvant chemotherapy and radiotherapy of breast cancer. Eur J Cancer. (1995) 31:2185–90. doi: 10.1016/0959-8049(95)00344-4
58. Kyndi M, Sørensen FB, Knudsen H, Overgaard M, Nielsen HM, Overgaard J. Estrogen receptor, progesterone receptor, HER-2, and response to postmastectomy radiotherapy in high-risk breast cancer: the Danish Breast Cancer Cooperative Group. J Clin Oncol. (2008) 26:1419–26. doi: 10.1200/JCO.2007.14.5565
59. Goodman CR, Seagle BLL, Shahabi S, Strauss JB. Oncotype score and benefit of post-mastectomy radiotherapy in T1–2 N1 breast cancer. Int J Radiat Oncol Biol Phys. (2017) 99:S53. doi: 10.1016/j.ijrobp.2017.06.134
60. Torres-Roca JF, Eschrich S, Zhao H, Bloom G, Sung J, McCarthy S, et al. Prediction of radiation sensitivity using a gene expression classifier. Cancer Res. (2005) 65:7169–76. doi: 10.1158/0008-5472.CAN-05-0656
61. Hall JS, Iype R, Senra J, Taylor J, Armenoult L, Oguejiofor K, et al. Investigation of radiosensitivity gene signatures in cancer cell lines. PLoS ONE. (2014) 9:e86329. doi: 10.1371/journal.pone.0086329
62. Eschrich SA, Pramana J, Zhang H, Zhao H, Boulware D J., Lee H, et al. A gene expression model of intrinsic tumor radiosensitivity: prediction of response and prognosis after chemoradiation. Int J Radiat Oncol Biol Phys. (2009) 75:489–96. doi: 10.1016/j.ijrobp.2009.06.014
63. Ahmed KA, Chinnaiyan P, Fulp WJ, Eschrich S, Torres-Roca JF, Caudell JJ. The radiosensitivity index predicts for overall survival in glioblastoma. Oncotarget. (2015) 6:34414–22. doi: 10.18632/oncotarget.5437
64. Torres-Roca J, Erho N, Vergara I, Davicioni E, Jenkins R, Den R, et al. A molecular signature of radiosensitivity (RSI) is an RT-specific biomarker in prostate cancer. Int J Radiat Oncol Biol Phys. (2014) 90:S157. doi: 10.1016/j.ijrobp.2014.05.642
65. Strom T, Hoffe SE, Fulp W, Frakes J, Coppola D, Springett GM, et al. Radiosensitivity index predicts for survival with adjuvant radiation in resectable pancreatic cancer. Radiother Oncol. (2015) 117:159–64. doi: 10.1016/j.radonc.2015.07.018
66. Ahmed KA, Fulp WJ, Berglund AE, Hoffe SE, Dilling TJ, Eschrich SA, et al. Differences between colon cancer primaries and metastases using a molecular assay for tumor radiation sensitivity suggest implications for potential oligometastatic SBRT patient selection. Int J Radiat Oncol Biol Phys. (2015) 92:837–42. doi: 10.1016/j.ijrobp.2015.01.036
67. Eschrich SA, Fulp WJ, Pawitan Y, Foekens JA, Smid M J., Martens WM, et al. Validation of a radiosensitivity molecular signature in breast cancer. Clin Cancer Res. (2012) 18:5134–43. doi: 10.1158/1078-0432.CCR-12-0891
68. Torres-Roca JF, Fulp WJ, Caudell JJ, Servant N, Bollet MA, van de Vijver M, et al. Integration of a radiosensitivity molecular signature into the assessment of local recurrence risk in breast cancer. Int J Radiat Oncol Biol Phys. (2015) 93:631–8. doi: 10.1016/j.ijrobp.2015.06.021
69. Scott JG, Berglund A, Schell MJ, Mihaylov I, Fulp WJ, Yue B, et al. A genome-based model for adjusting radiotherapy dose (GARD): a retrospective, cohort-based study. Lancet Oncol. (2017) 18:202–11. doi: 10.1016/S1470-2045(16)30648-9
70. Amundson SA, Do KT, Vinikoor LC, Lee RA, Koch-Paiz CA, Ahn J, et al. Integrating global gene expression and radiation survival parameters across the 60 cell lines of the National Cancer Institute Anticancer Drug Screen. Cancer Res. (2008) 68:415–24. doi: 10.1158/0008-5472.CAN-07-2120
71. Yard BD, Adams DJ, Chie EK, Tamayo P, Battaglia JS, Gopal P, et al. A genetic basis for the variation in the vulnerability of cancer to DNA damage. Nat Commun. (2016) 7:11428. doi: 10.1038/ncomms11428
72. Eustace A, Mani N, Span PN, Irlam JJ, Taylor J, Betts GN, et al. A 26-gene hypoxia signature predicts benefit from hypoxia-modifying therapy in laryngeal cancer but not bladder cancer. Clin Cancer Res. (2013) 19:4879–88. doi: 10.1158/1078-0432.CCR-13-0542
73. Toustrup K, Sørensen BS M., Metwally AH, Tramm T, Mortensen LS, Overgaard J, et al. Validation of a 15-gene hypoxia classifier in head and neck cancer for prospective use in clinical trials. Acta Oncologica. (2016) 55:1091–8. doi: 10.3109/0284186X.2016.1167959
74. Yang L, Taylor J, Eustace A, Irlam JJ, Denley H, Hoskin PJ, et al. A gene signature for selecting benefit from hypoxia modification of radiotherapy for high-risk bladder cancer patients. Clin Cancer Res. (2017) 23:4761–8. doi: 10.1158/1078-0432.CCR-17-0038
75. Oh DS, Cheang MC, Fan C, Perou CM. Radiation-induced gene signature predicts pathologic complete response to neoadjuvant chemotherapy in breast cancer patients. Radiat Res. (2014) 181:193–207. doi: 10.1667/RR13485.1
76. Kim HS, Kim SC, Kim SJ, Park CH, Jeung HC, Kim YB, et al. Identification of a radiosensitivity signature using integrative metaanalysis of published microarray data for NCI-60 cancer cells. BMC Genom. (2012) 13:348. doi: 10.1186/1471-2164-13-348
77. Feng FY, Speers C, Liu M, Jackson WC, Moon D, Rinkinen J, et al. Targeted radiosensitization with PARP1 inhibition: optimization of therapy and identification of biomarkers of response in breast cancer. Breast Cancer Res Treat. (2014) 147:81–94. doi: 10.1007/s10549-014-3085-5
78. Jonsson M, Ragnum HB, Julin CH, Yeramian A, Clancy T, Frikstad KAM, et al. Hypoxia-independent gene expression signature associated with radiosensitisation of prostate cancer cell lines by histone deacetylase inhibition. Br J Cancer. (2016) 115:929. doi: 10.1038/bjc.2016.278
79. Speers C, Zhao S, Liu M, Bartelink H, Pierce LJ, Feng FY. Development and validation of a novel radiosensitivity signature in human breast cancer. Clin Cancer Res. (2015) 21:3667–77. doi: 10.1158/1078-0432.CCR-14-2898
80. Forrest AP, Stewart HJ, Everington D, Prescott RJ, McArdle CS, Harnett AN, et al. Randomised controlled trial of conservation therapy for breast cancer: 6-year analysis of the Scottish trial. Lancet. (1996) 348:708–13. doi: 10.1016/S0140-6736(96)02133-2
81. Speers C, Pierce LJ. Molecular signatures of radiation response in breast cancer: towards personalized decision-making in radiation treatment. Int J Breast Cancer. (2017) 2017:4279724. doi: 10.1155/2017/4279724
82. Sjöström M, Chang SL, Fishbane N, Davicioni E, Zhao SG, Hartman L, et al. Clinicogenomic radiotherapy classifier predicting the need for intensified locoregional treatment after breast-conserving surgery for early-stage breast cancer. J Clin Oncol. (2019) 37:3340–9. doi: 10.1200/JCO.19.00761
83. Tramm T, Kyndi M, Myhre S, Nord S, Alsner J, Sørensen FB, et al. Relationship between the prognostic and predictive value of the intrinsic subtypes and a validated gene profile predictive of loco-regional control and benefit from post-mastectomy radiotherapy in patients with high-risk breast cancer. Acta Oncologica. (2014) 53:1337–46. doi: 10.3109/0284186X.2014.925580
84. Niméus-Malmström E, Krogh M, Malmström P, Strand C, Fredriksson I, Karlsson P, et al. Gene expression profiling in primary breast cancer distinguishes patients developing local recurrence after breast-conservation surgery, with or without postoperative radiotherapy. Breast Cancer Res. (2008) 10:R34. doi: 10.1186/bcr1997
85. Kreike B, Halfwerk H, Armstrong N, Bult P, Foekens JA, Veltkamp SC, et al. Local recurrence after breast-conserving therapy in relation to gene expression patterns in a large series of patients. Clin Cancer Res. (2009) 15:4181–90. doi: 10.1158/1078-0432.CCR-08-2644
86. Albert JM, Liu DD, Shen Y, Pan IWY., Shih CT, Hoffman KE, et al. Nomogram to predict the benefit of radiation for older patients with breast cancer treated with conservative surgery. J Clin Oncol. (2012) 30:2837–43. doi: 10.1200/JCO.2011.41.0076
87. Franco P, De Rose F, De Santis MC, Pasinetti N, Lancellotta V, Meduri B, et al. Omission of postoperative radiation after breast conserving surgery: a progressive paradigm shift towards precision medicine. Clin Transl Radiat Oncol. (2020) 21:112–9. doi: 10.1016/j.ctro.2020.02.003
88. Hu Z, Huang G, Sadanandam A, Gu S, Lenburg ME, Pai M, et al. The expression level of HJURP has an independent prognostic impact and predicts the sensitivity to radiotherapy in breast cancer. Breast Cancer Res. (2010) 12:R18. doi: 10.1186/bcr2487
89. Woolston CM, Storr SJ, Ellis IO D., Morgan AL, Martin SG. Expression of thioredoxin system and related peroxiredoxin proteins is associated with clinical outcome in radiotherapy treated early stage breast cancer. Radiother Oncol. (2011) 100:308–13. doi: 10.1016/j.radonc.2011.05.029
90. Venkitaraman AR. Cancer susceptibility and the functions of BRCA1 and BRCA2. Cell. (2002) 108:171–82. doi: 10.1016/S0092-8674(02)00615-3
91. Söderlund K, Skoog L, Fornander T, Askmalm MS. The BRCA1/BRCA2/Rad51 complex is a prognostic and predictive factor in early breast cancer. Radiother Oncol. (2007) 84:242–51. doi: 10.1016/j.radonc.2007.06.012
92. Martin A, Greshock J, Rebbeck T, Weber B. Allele Frequencies of Cytokine Gene Polymorphisms in Caucasians and African Americans. Chicago, IL: American Journal Of Human Genetics; Univ Chicago Press 5720 South Woodlawn AVE (2000). p. 318.
93. Ford D, Easton D, Stratton M, Narod S, Goldgar D, Devilee P, et al. Genetic heterogeneity and penetrance analysis of the BRCA1 and BRCA2 genes in breast cancer families. Am J Hum Genet. (1998) 62:676–89. doi: 10.1086/301749
94. King MC, Marks JH, Mandell JB. Breast and ovarian cancer risks due to inherited mutations in BRCA1 and BRCA2. Science. (2003) 302:643–46. doi: 10.1126/science.1088759
95. Struewing JP, Hartge P, Wacholder S, Baker SM, Berlin M, McAdams M, et al. The risk of cancer associated with specific mutations of BRCA1 and BRCA2 among Ashkenazi Jews. N Engl J Med. (1997) 336:1401–8. doi: 10.1056/NEJM199705153362001
96. Dantzer F, de la Rubia G, Ménissier-de Murcia J, Hostomsky Z, de Murcia G, Schreiber V. Base excision repair is impaired in mammalian cells lacking Poly (ADP-ribose) polymerase-1. Biochemistry. (2000) 39:7559–69. doi: 10.1021/bi0003442
97. Robson M, Im SA, Senkus E, Xu B, Domchek SM, Masuda N, et al. Olaparib for metastatic breast cancer in patients with a germline BRCA mutation. N Engl J Med. (2017) 377:523–33. doi: 10.1056/NEJMoa1706450
98. Tucker H, Charles Z, Robertson J, Adam J. NICE guidance on olaparib for maintenance treatment of patients with relapsed, platinum-sensitive, BRCA mutation-positive ovarian cancer. Lancet Oncol. (2016) 17:277–8. doi: 10.1016/S1470-2045(16)00062-0
99. Wang L, Mason KA, Ang KK, Buchholz T, Valdecanas D, Mathur A, et al. MK-4827, a PARP-1/-2 inhibitor, strongly enhances response of human lung and breast cancer xenografts to radiation. Invest New Drugs. (2012) 30:2113–20. doi: 10.1007/s10637-011-9770-x
100. Lamerz R, Stieber P, Fateh-Moghadam A. Serum marker combinations in human breast cancer. In Vivo. (1993) 7:607–13.
101. Dnistrian AM, Schwartz MK, Greenberg EJ, Smith CA, Schwartz DC. Evaluation of CA M26, CA M29, CA 15–3 and CEA as circulating tumor markers in breast cancer patients. Tumor Biol. (1991) 12:82–90. doi: 10.1159/000217692
102. Ebeling FG, Stieber P, Untch M, Nagel D, Konecny GE, Schmitt UM, et al. Serum CEA and CA 15–3 as prognostic factors in primary breast cancer. Br J Cancer. (2002) 86:1217–22. doi: 10.1038/sj.bjc.6600248
103. Stieber P, Nagel D, Ritzke C, Rössler N, Kirsch C, Eiermann W, et al. Significance of bone alkaline phosphatase, CA 15–3 and CEA in the detection of bone metastases during the follow-up of patients suffering from breast carcinoma. Clin Chem Lab Med. (1992) 30:809–14. doi: 10.1515/cclm.1992.30.12.809
104. Vizcarra E, Lluch A, Cibrian R, Jarque F, Garcia-Conde J. CA15. 3, CEA and TPA tumor markers in the early diagnosis of breast cancer relapse. Oncology. (1994) 51:491–6. doi: 10.1159/000227391
105. Duffy MJ, Evoy D, McDermott EW. CA 15–3: uses and limitation as a biomarker for breast cancer. Clin Chim Acta. (2010) 411:1869–74. doi: 10.1016/j.cca.2010.08.039
106. Ludovini V, Gori S, Colozza M, Pistola L, Rulli E, Floriani I, et al. Evaluation of serum HER2 extracellular domain in early breast cancer patients: correlation with clinicopathological parameters and survival. Ann Oncol. (2008) 19:883–90. doi: 10.1093/annonc/mdm585
107. Molina R, Augé JM, Escudero JM, Filella X, Zanon G, Pahisa J, et al. Evaluation of tumor markers (HER-2/neu oncoprotein, CEA, and CA 15.3) in patients with locoregional breast cancer: prognostic value. Tumor Biol. (2010) 31:171–80. doi: 10.1007/s13277-010-0025-9
108. Asgeirsson KS, Agrawal A, Allen C, Hitch A, Ellis IO, Chapman C, et al. Serum epidermal growth factor receptor and HER2 expression in primary and metastatic breast cancer patients. Breast Cancer Res. (2007) 9:R75. doi: 10.1186/bcr1788
109. Leyland-Jones B, Smith BR. Serum HER2 testing in patients with HER2-positive breast cancer: the death knell tolls. Lancet Oncol. (2011) 12:286–95. doi: 10.1016/S1470-2045(10)70297-7
110. Vader P, Breakefield XO, Wood MJ. Extracellular vesicles: emerging targets for cancer therapy. Trends Mol Med. (2014) 20:385–93. doi: 10.1016/j.molmed.2014.03.002
111. Valadi H, Ekström K, Bossios A, Sjöstrand M, Lee JJ, Lötvall JO. Exosome-mediated transfer of mRNAs and microRNAs is a novel mechanism of genetic exchange between cells. Nat Cell Biol. (2007) 9:654–59. doi: 10.1038/ncb1596
112. Iero M, Valenti R, Huber V, Filipazzi P, Parmiani G, Fais S, et al. Tumour-released exosomes and their implications in cancer immunity. Cell Death Differ. (2008) 15:80–8. doi: 10.1038/sj.cdd.4402237
113. Greening DW, Gopal SK, Mathias RA, Liu L, Sheng J, Zhu HJ, et al. Emerging roles of exosomes during epithelial–mesenchymal transition and cancer progression. Semin Cell Dev Biol. (2015) 40:60–71. doi: 10.1016/j.semcdb.2015.02.008
114. Kahlert C, Kalluri R. Exosomes in tumor microenvironment influence cancer progression and metastasis. J Mol Med. (2013) 91:431–7. doi: 10.1007/s00109-013-1020-6
115. Wang J, Hendrix A, Hernot S, Lemaire M, De Bruyne E, Van Valckenborgh E, et al. Bone marrow stromal cell–derived exosomes as communicators in drug resistance in multiple myeloma cells. Blood. (2014) 124:555–66. doi: 10.1182/blood-2014-03-562439
116. Boelens MC, Wu TJ, Nabet BY, Xu B, Qiu Y, Yoon T, et al. Exosome transfer from stromal to breast cancer cells regulates therapy resistance pathways. Cell. (2014) 159:499–513. doi: 10.1016/j.cell.2014.09.051
117. Gray LH, Conger AD, Ebert M, Hornsey S, Scott OCA. The concentration of oxygen dissolved in tissues at the time of irradiation as a factor in radiotherapy. Br J Radiol. (1953) 26:638–48. doi: 10.1259/0007-1285-26-312-638
118. Bertout JA, Patel SA, Simon MC. The impact of O2 availability on human cancer. Nat Rev Cancer. (2008) 8:967–75. doi: 10.1038/nrc2540
119. Okunieff P, Fenton B, Chen Y. Past, present, and future of oxygen in cancer research. Adv Exp Med Biol. (2005) 566:213–22. doi: 10.1007/0-387-26206-7_29
120. Vos O. Role of endogenous thiols in protection. Adv Space Res. (1992) 12:201–7. doi: 10.1016/0273-1177(92)90109-B
121. Eldh M, Ekström K, Valadi H, Sjöstrand M, Olsson B, Jernås M, et al. Exosomes communicate protective messages during oxidative stress; possible role of exosomal shuttle RNA. PLoS ONE. (2010) 5:e15353. doi: 10.1371/journal.pone.0015353
122. Thomas SN, Liao Z, Clark D, Chen Y, Samadani R, Mao L, et al. Exosomal proteome profiling: a potential multi-marker cellular phenotyping tool to characterize hypoxia-induced radiation resistance in breast cancer. Proteomes. (2013) 1:87–108. doi: 10.3390/proteomes1020087
123. Kallergi G, Papadaki MA, Politaki E, Mavroudis D, Georgoulias V, Agelaki S. Epithelial to mesenchymal transition markers expressed in circulating tumour cells of early and metastatic breast cancer patients. Breast Cancer Res. (2011) 13:R59. doi: 10.1186/bcr2896
124. Massagué J, Obenauf AC. Metastatic colonization by circulating tumour cells. Nature. (2016) 529:298–306. doi: 10.1038/nature17038
125. Krishnamurthy S, Cristofanilli M, Singh B, Reuben J, Gao H, Cohen EN, et al. Detection of minimal residual disease in blood and bone marrow in early stage breast cancer. Cancer. (2010) 116:3330–7. doi: 10.1002/cncr.25145
126. Bidard FC, Mathiot C, Delaloge S, Brain E, Giachetti S, De Cremoux P, et al. Single circulating tumor cell detection and overall survival in nonmetastatic breast cancer. Ann Oncol. (2009) 21:729–33. doi: 10.1093/annonc/mdp391
127. Janni WJ, Rack B, Terstappen LW J., Pierga Y, Taran FA, Fehm T, et al. Pooled analysis of the prognostic relevance of circulating tumor cells in primary breast cancer. Clin Cancer Res. (2016) 22:2583–93. doi: 10.1158/1078-0432.CCR-15-1603
128. Rack B, Schindlbeck C, Jückstock J, Andergassen U, Hepp P, Zwingers T, et al. Circulating tumor cells predict survival in early average-to-high risk breast cancer patients. J Natl Cancer Inst. (2014) 106:dju066. doi: 10.1093/jnci/dju066
129. Franken B, De Groot MR, Mastboom WJ, Vermes I, van der Palen J, Tibbe AG, et al. Circulating tumor cells, disease recurrence and survival in newly diagnosed breast cancer. Breast Cancer Res. (2012) 14:R133. doi: 10.1186/bcr3333
130. Lucci A, Hall CS, Lodhi AK, Bhattacharyya A, Anderson AE, Xiao L, et al. Circulating tumour cells in non-metastatic breast cancer: a prospective study. Lancet Oncol. (2012) 13:688–95. doi: 10.1016/S1470-2045(12)70209-7
131. Bidard FC, Belin L, Delaloge S, Lerebours F, Ngo C, Reyal F, et al. Time-dependent prognostic impact of circulating tumor cells detection in non-metastatic breast cancer: 70-month analysis of the REMAGUS02 study. Int J Breast Cancer. (2013) 2013:130470. doi: 10.1155/2013/130470
132. Bidard FC, Hajage D, Bachelot T, Delaloge S, Brain E, Campone M, et al. Assessment of circulating tumor cells and serum markers for progression-free survival prediction in metastatic breast cancer: a prospective observational study. Breast Cancer Res. (2012) 14:R29. doi: 10.1186/bcr3114
133. Molloy TJ, Bosma AJ, Baumbusch LO, Synnestvedt M, Borgen E, Russnes HG, et al. The prognostic significance of tumour cell detection in the peripheral blood versus the bone marrow in 733 early-stage breast cancer patients. Breast Cancer Res. (2011) 13:R61. doi: 10.1186/bcr2898
134. Hall C, Karhade M, Laubacher B, Anderson A, Kuerer H, DeSynder S, et al. Circulating tumor cells after neoadjuvant chemotherapy in stage I–III triple-negative breast cancer. Ann Surg Oncol. (2015) 22:552–8. doi: 10.1245/s10434-015-4600-6
135. Hall CS, Karhade MG J., Bauldry BB, Valad LM, Kuerer HM, DeSnyder SM, et al. Prognostic value of circulating tumor cells identified before surgical resection in nonmetastatic breast cancer patients. J Am Coll Surg. (2016) 223:20–9. doi: 10.1016/j.jamcollsurg.2016.02.021
136. Goodman CR, Seagle BLLT, Friedl WP, Rack B, Lato K, Fink V, et al. Association of circulating tumor cell status with benefit of radiotherapy and survival in early-stage breast cancer. JAMA Oncol. (2018) 4:e180163. doi: 10.1001/jamaoncol.2018.0163
137. Speers C, Rugo HS. Circulating tumors cells as a biomarker of radiation benefit. JAMA Oncol. (2018) 4:e180194. doi: 10.1001/jamaoncol.2018.0194
138. Tsé C, Gauchez AS, Jacot W, Lamy PJ. HER2 shedding and serum HER2 extracellular domain: biology and clinical utility in breast cancer. Cancer Treat Rev. (2012) 38:133–42. doi: 10.1016/j.ctrv.2011.03.008
139. Shapiro CL, Recht A. Side effects of adjuvant treatment of breast cancer. N Engl J Med. (2001) 344:1997–2008. doi: 10.1056/NEJM200106283442607
140. Wazer DE, DiPetrillo T, Schmidt-Ullrich R, Weld L, Smith T, Marchant D, et al. Factors influencing cosmetic outcome and complication risk after conservative surgery and radiotherapy for early-stage breast carcinoma. J Clin Oncol. (1992) 10:356–63. doi: 10.1200/JCO.1992.10.3.356
141. Azria D, Gourgou S, Sozzi W, Zouhair A, Mirimanoff R, Kramar A, et al. Concomitant use of tamoxifen with radiotherapy enhances subcutaneous breast fibrosis in hypersensitive patients. Br J Cancer. (2004) 91:1251–60. doi: 10.1038/sj.bjc.6602146
142. Collette S, Collette L, Budiharto T, Horiot JC, Poortmans PM, Struikmans H, et al. Predictors of the risk of fibrosis at 10 years after breast conserving therapy for early breast cancer–A study based on the EORTC trial 22881–10882 ‘boost versus no boost’. Eur J Cancer. (2008) 44:2587–99. doi: 10.1016/j.ejca.2008.07.032
143. Colletta A, Wakefield L, Howell F, Van Roozendaal K, Danielpour D, Ebbs S, et al. Anti-oestrogens induce the secretion of active transforming growth factor beta from human fetal fibroblasts. Br J Cancer. (1990) 62:405–9. doi: 10.1038/bjc.1990.307
144. Aristei C, Palumbo I, Capezzali G, Farneti A, Bini V, Falcinelli L, et al. Outcome of a phase II prospective study on partial breast irradiation with interstitial multi-catheter high-dose-rate brachytherapy. Radiother Oncol. (2013) 108:236–41. doi: 10.1016/j.radonc.2013.08.005
145. Hau E, Browne L, Capp A, Delaney GP, Fox C, Kearsley JH, et al. The impact of breast cosmetic and functional outcomes on quality of life: long-term results from the St. George and Wollongong randomized breast boost trial. Breast Cancer Res Treat. (2013) 139:115–23. doi: 10.1007/s10549-013-2508-z
146. Taghian NR, Miller CL, Jammallo LS, O'Toole J, Skolny MN. Lymphedema following breast cancer treatment and impact on quality of life: a review. Crit Rev Oncol Hematol. (2014) 92:227–34. doi: 10.1016/j.critrevonc.2014.06.004
147. Back M, Guerrieri M, Wratten C, Steigler A. Impact of radiation therapy on acute toxicity in breast conservation therapy for early breast cancer. Clin Oncol. (2004) 16:12–6. doi: 10.1016/j.clon.2003.08.005
148. Henríquez-Hernández LA, Bordón E, Pinar B, Lloret M, Rodríguez-Gallego C, Lara PC. Prediction of normal tissue toxicity as part of the individualized treatment with radiotherapy in oncology patients. Surg Oncol. (2012) 21:201–6. doi: 10.1016/j.suronc.2011.12.002
149. Pinar B, Lara PC, Lloret M, Bordón E, Núñez MI, Villalobos M, et al. Radiation-induced DNA damage as a predictor of long-term toxicity in locally advanced breast cancer patients treated with high-dose hyperfractionated radical radiotherapy. Radiat Res. (2007) 168:415–22. doi: 10.1667/RR0746.1
150. Rached E, Schindler R, Beer K, Vetterli D, Greiner R. No predictive value of the micronucleus assay for patients with severe acute reaction of normal tissue after radiotherapy. Eur J Cancer. (1998) 34:378–83. doi: 10.1016/S0959-8049(97)00373-0
151. Słonina D, Klimek M, Szpytma T, Gasinska A. Comparison of the radiosensitivity of normal-tissue cells with normal-tissue reactions after radiotherapy. Int J Radiat Biol. (2000) 76:1255–64. doi: 10.1080/09553000050134483
152. Alapetite C, Thirion P, de la Rochefordière A, Cosset JM, Moustacchi E. Analysis by alkaline comet assay of cancer patients with severe reactions to radiotherapy: defective rejoining of radioinduced DNA strand breaks in lymphocytes of breast cancer patients. Int J Cancer. (1999) 83:83–90. doi: 10.1002/(SICI)1097-0215(19990924)83:1<83::AID-IJC16>3.0.CO;2-8
153. Müller WU, Bauch T, Stüben G, Sack H, Streffer C. Radiation sensitivity of lymphocytes from healthy individuals and cancer patients as measured by the comet assay. Radiat Environ Biophys. (2001) 40:83–9. doi: 10.1007/s004110000087
154. Widel M, Jedrus S, Lukaszczyk B, Raczek-Zwierzycka K, Swierniak A. Radiation-induced micronucleus frequency in peripheral blood lymphocytes is correlated with normal tissue damage in patients with cervical carcinoma undergoing radiotherapy. Radiat Res. (2003) 159:713–21. doi: 10.1667/0033-7587
155. Ozsahin M, Crompton NE, Gourgou S, Kramar A, Li L, Shi Y, et al. CD4 and CD8 T-lymphocyte apoptosis can predict radiation-induced late toxicity: a prospective study in 399 patients. Clin Cancer Res. (2005) 11:7426–33. doi: 10.1158/1078-0432.CCR-04-2634
156. Henríquez-Hernández LA, Carmona-Vigo R, Pinar B, Bordón E, Lloret M, Núñez MI, et al. Combined low initial DNA damage and high radiation-induced apoptosis confers clinical resistance to long-term toxicity in breast cancer patients treated with high-dose radiotherapy. Radiat Oncol. (2011) 6:60. doi: 10.1186/1748-717X-6-60
157. Carr ZA, Land CE, Kleinerman RA, Weinstock RW, Stovall M, Griem ML, et al. Coronary heart disease after radiotherapy for peptic ulcer disease. Int J Radiat Oncol Biol Phys. (2005) 61:842–50. doi: 10.1016/j.ijrobp.2004.07.708
158. Aznar MC, Korreman S, Pedersen AN, Persson GF, Josipovic M, Specht L. Evaluation of dose to cardiac structures during breast irradiation. Br J Radiol. (2011) 84:743–46. doi: 10.1259/bjr/12497075
159. Lohr F, El-Haddad M, Dobler B, Grau R, Wertz HJ, Kraus-Tiefenbacher U, et al. Potential effect of robust and simple IMRT approach for left-sided breast cancer on cardiac mortality. Int J Radiat Oncol Biol Phys. (2009) 74:73–80. doi: 10.1016/j.ijrobp.2008.07.018
160. van den Bogaard VA, Ta BD, van der Schaaf A, Bouma AB, Middag AM, Bantema-Joppe EJ, et al. Validation and modification of a prediction model for acute cardiac events in patients with breast cancer treated with radiotherapy based on three-dimensional dose distributions to cardiac substructures. J Clin Oncol. (2017) 35:1171–8. doi: 10.1200/JCO.2016.69.8480
161. Darby SC, Ewertz M, McGale P, Bennet AM, Blom-Goldman U, Brønnum D, et al. Risk of ischemic heart disease in women after radiotherapy for breast cancer. N Engl J Med. (2013) 368:987–98. doi: 10.1056/NEJMoa1209825
162. Carver JR, Shapiro CL, Ng A, Jacobs L, Schwartz C, Virgo KS, et al. American Society of clinical oncology clinical evidence review on the ongoing care of adult cancer survivors: cardiac and pulmonary late effects. J Clin Oncol. (2007) 25:3991–4008. doi: 10.1200/JCO.2007.10.9777
163. Mant J, Doust J, Roalfe A, Barton P, Cowie M, Glasziou P, et al. Systematic review and individual patient data meta-analysis of diagnosis of heart failure, with modelling of implications of different diagnostic strategies in primary care. Health Technol Assess. (2009) 13:1–207. doi: 10.3310/hta13320
164. Nellessen U, Zingel M, Hecker H, Bahnsen J, Borschke D. Effects of radiation therapy on myocardial cell integrity and pump function: which role for cardiac biomarkers? Chemotherapy. (2010) 56:147–52. doi: 10.1159/000313528
165. D'Errico MP, Grimaldi L, Petruzzelli MF, Gianicolo EA, Tramacere F, Monetti A, et al. N-Terminal Pro-B–type natriuretic peptide plasma levels as a potential biomarker for cardiac damage after radiotherapy in patients with left-sided breast cancer. Int J Radiat Oncol Biol Phys. (2012) 82:e239–46. doi: 10.1016/j.ijrobp.2011.03.058
166. Lipshultz SE, Landy DC, Lopez-Mitnik G, Lipsitz SR, Hinkle AS, Constine LS, et al. Cardiovascular status of childhood cancer survivors exposed and unexposed to cardiotoxic therapy. J Clin Oncol. (2012) 30:1050–7. doi: 10.1200/JCO.2010.33.7907
167. Cardinale D, Sandri MT, Colombo A, Colombo N, Boeri M, Lamantia G, et al. Prognostic value of troponin I in cardiac risk stratification of cancer patients undergoing high-dose chemotherapy. Circulation. (2004) 109:2749–54. doi: 10.1161/01.CIR.0000130926.51766.CC
168. Garrone O, Crosetto N, Nigro CL, Catzeddu T, Vivenza D, Monteverde M, et al. Prediction of anthracycline cardiotoxicity after chemotherapy by biomarkers kinetic analysis. Cardiovasc Toxicol. (2012) 12:135–42. doi: 10.1007/s12012-011-9149-4
169. Hughes-Davies L, Sacks D, Rescigno J, Howard S, Harris J. Serum cardiac troponin T levels during treatment of early-stage breast cancer. J Clin Oncol. (1995) 13:2582–4. doi: 10.1200/JCO.1995.13.10.2582
170. Erven K, Florian A, Slagmolen P, Sweldens C, Jurcut R, Wildiers H, et al. Subclinical cardiotoxicity detected by strain rate imaging up to 14 months after breast radiation therapy. Int J Radiat Oncol Biol Phys. (2013) 85:1172–8. doi: 10.1016/j.ijrobp.2012.09.022
171. Skyttä T, Tuohinen S, Boman E, Virtanen V, Raatikainen P, Kellokumpu-Lehtinen PL. Troponin T-release associates with cardiac radiation doses during adjuvant left-sided breast cancer radiotherapy. Radiat Oncol. (2015) 10:141. doi: 10.1186/s13014-015-0436-2
172. Mueller C, Laule-Kilian K, Christ A, Brunner–La Rocca HP, Perruchoud AP. Inflammation and long-term mortality in acute congestive heart failure. Am Heart J. (2006) 151:845–50. doi: 10.1016/j.ahj.2005.06.046
173. Windram JD, Loh PH, Rigby AS, Hanning I, Clark AL, Cleland JG. Relationship of high-sensitivity C-reactive protein to prognosis and other prognostic markers in outpatients with heart failure. Am Heart J. (2007) 153:1048–55. doi: 10.1016/j.ahj.2007.03.044
174. Arruda-Olson AM, Enriquez-Sarano M, Bursi F, Weston SA, Jaffe AS, Killian JM, et al. Left ventricular function and C-reactive protein levels in acute myocardial infarction. Am J Cardiol. (2010) 105:917–21. doi: 10.1016/j.amjcard.2009.11.025
175. Morris PG, Chen C, Steingart R, Fleisher M, Lin N, Moy B, et al. Troponin I and C-reactive protein are commonly detected in patients with breast cancer treated with dose-dense chemotherapy incorporating trastuzumab and lapatinib. Clin Cancer Res. (2011) 17:3490–9. doi: 10.1158/1078-0432.CCR-10-1359
176. Lipshultz SE, Miller TL, Scully RE, Lipsitz SR, Rifai N, Silverman LB, et al. Changes in cardiac biomarkers during doxorubicin treatment of pediatric patients with high-risk acute lymphoblastic leukemia: associations with long-term echocardiographic outcomes. J Clin Oncol. (2012) 30:1042–9. doi: 10.1200/JCO.2010.30.3404
177. Ky B, Putt M, Sawaya H, French B, Januzzi JL, Sebag IA, et al. Early increases in multiple biomarkers predict subsequent cardiotoxicity in patients with breast cancer treated with doxorubicin, taxanes, and trastuzumab. J Am Coll Cardiol. (2014) 63:809–16. doi: 10.1016/j.jacc.2013.10.061
178. Chalubinska-Fendler J, Graczyk L, Piotrowski G, Wyka K, Nowicka Z, Tomasik B, et al. Lipopolysaccharide-binding protein is an early biomarker of cardiac function after radiation therapy for breast cancer. Int J Radiat Oncol Biol Phys. (2019) 104:1074–83. doi: 10.1016/j.ijrobp.2019.04.002
179. Chalubinska-Fendler J, Fendler W, Spych M, Wyka K, Luniewska-Bury J, Fijuth J. Lipopolysaccharide-binding protein is efficient in biodosimetry during radiotherapy of lung cancer. Biomed Rep. (2016) 5:450–4. doi: 10.3892/br.2016.739
Keywords: biomarkers of radiosensitivity, breast cancer, precision medicine, molecular signatures of radiosensitivity, biomarkers of radiation-induced toxicity
Citation: Meehan J, Gray M, Martínez-Pérez C, Kay C, Pang LY, Fraser JA, Poole AV, Kunkler IH, Langdon SP, Argyle D and Turnbull AK (2020) Precision Medicine and the Role of Biomarkers of Radiotherapy Response in Breast Cancer. Front. Oncol. 10:628. doi: 10.3389/fonc.2020.00628
Received: 05 March 2020; Accepted: 06 April 2020;
Published: 24 April 2020.
Edited by:
Francesco Cellini, Agostino Gemelli University Polyclinic, ItalyReviewed by:
Valentina Lancellotta, Agostino Gemelli University Polyclinic, ItalyMichele Fiore, Policlinico Universitario Campus Bio-Medico, Italy
Copyright © 2020 Meehan, Gray, Martínez-Pérez, Kay, Pang, Fraser, Poole, Kunkler, Langdon, Argyle and Turnbull. This is an open-access article distributed under the terms of the Creative Commons Attribution License (CC BY). The use, distribution or reproduction in other forums is permitted, provided the original author(s) and the copyright owner(s) are credited and that the original publication in this journal is cited, in accordance with accepted academic practice. No use, distribution or reproduction is permitted which does not comply with these terms.
*Correspondence: James Meehan, am1lZWhhbkBlZC5hYy51aw==