- 1School of Medicine, Life and Health Sciences Research Institute (ICVS), University of Minho, Braga, Portugal
- 2ICVS/3B's—PT Government Associate Laboratory, Guimarães, Portugal
To sustain their high proliferation rates, most cancer cells rely on glycolytic metabolism, with production of lactic acid. For many years, lactate was seen as a metabolic waste of glycolytic metabolism; however, recent evidence has revealed new roles of lactate in the tumor microenvironment, either as metabolic fuel or as a signaling molecule. Lactate plays a key role in the different models of metabolic crosstalk proposed in malignant tumors: among cancer cells displaying complementary metabolic phenotypes and between cancer cells and other tumor microenvironment associated cells, including endothelial cells, fibroblasts, and diverse immune cells. This cell metabolic symbiosis/slavery supports several cancer aggressiveness features, including increased angiogenesis, immunological escape, invasion, metastasis, and resistance to therapy. Lactate transport is mediated by the monocarboxylate transporter (MCT) family, while another large family of G protein-coupled receptors (GPCRs), not yet fully characterized in the cancer context, is involved in lactate/acidosis signaling. In this mini-review, we will focus on the role of lactate in the tumor microenvironment, from metabolic affairs to signaling, including the function of lactate in the cancer–cancer and cancer–stromal shuttles, as well as a signaling oncometabolite. We will also review the prognostic value of lactate metabolism and therapeutic approaches designed to target lactate production and transport.
Introduction
The first discoveries involving lactate were reported in 1808, when it was described in the muscle of animals; only many years later was lactate associated with energy metabolism in muscle contraction (1, 2). The glycolysis pathway, transformation of glucose into pyruvate and ATP, was described in the 1940s by the joined efforts of numerous scientists, in a cascade-like chronology. It started with the discovery of fermentation in microorganisms by Louis Pasteur; then, Meyerhof et al. described the lactate cycle, providing essential knowledge on the transformation of energy in cells (3–5).
Lactate formation and functions were incorrectly described for a long time, since lactate was considered as a waste product of cellular metabolism (6). Although history did not give lactate its due importance, it is believed at presentthat lactate has a crucial role, especially as a shuttle molecule. The concept was introduced by Brooks more than 30 years ago (7–9), and despite some initial disbelief (10, 11), several reports have finally acknowledged the role of lactate in shuttles between glycolytic and oxidative cells, being the product of one and used by another (12). It is well-established that lactate is formed from the reduction of pyruvate via lactate dehydrogenase (LDHA), under aerobic or anaerobic conditions, produced, and transformed continuously by resting/exercising muscle, brain, heart, and gut tissues (13). Lactate is a major source of energy, the major gluconeogenic precursor and, as a signaling molecule, is capable of inducing autocrine, paracrine, and endocrine-like effects. This molecule is responsible for several homeostatic functions: For instance, in hepatocytes, it feeds gluconeogenesis; in the brain, it is used by astrocytes and neurons for oxidative metabolism (12, 14).
Lactate homeostasis in a healthy environment requires adequate transporters. The lactate transporters, monocarboxylate transporters (MCTs), are members of the SLC16 gene family and several have been identified by gene homology, as it will be further explained (15). Physiological levels of lactate are considered to be in the range of 1.5–3 mM in blood and tissue from healthy individuals (14, 16); higher values are usually an indication of a health problem. Lactate shuttles are key players in many conditions involving pregnancy and reproduction (17, 18), the human heart (19), brain (12), and cancer (13). The use of lactate levels as a marker of clinical outcome was first suggested in 1964 by Broder and Weil, when studying patients with undifferentiated shock (20). Since then, high lactate levels have been associated with several diseases such as shock, cardiac arrest, trauma, ischemia, diabetic ketoacidosis, liver dysfunction, and sepsis (21). Lactate also modulates the immune system and promotes immune-inflammatory responses (22). The levels of lactate are increased in several inflammatory and autoimmune disorders, and lactate transporters were overexpressed at the surface of immune cells (14, 23). Lactate accumulation and transport has become particularly relevant in rheumatoid arthritis, where MCT4 inhibition was pointed as a possible therapeutic strategy (24). In the cancer setting, Otto Warburg was the first to observe that tumor cells share a common metabolic feature: high glucose consumption and increased glycolysis leading to lactate production, regardless of oxygen availability (25, 26).
Role of Lactate in Cancer Metabolic Rewiring
Cancer metabolism emerged as an area of research that has increasingly gained attention in the last decades. In order to sustain the proliferative phenotype, cancer cells enroll metabolic changes, such as the “Warburg effect” disclosed by Otto Warburg in 1926 (27). These changes consist on upregulation of glucose metabolism (glycolysis) even in the presence of oxygen, thereby producing high levels of lactate and reducing the use of the tricarboxylic acid (TCA) cycle. This addictive glycolytic phenotype arises as a distinctive metabolic characteristic of many types of cancer, being introduced as a new hallmark of cancer in 2011 (28).
Oncogenic Triggers of Glycolytic Metabolism
Tumorigenesis is characterized by genetic alterations, and several findings demonstrate that high expression of specific transcription factors or oncogenic tumor pathways, principally MYC, hypoxia-inducible factor-1 alpha (HIF-1α), nuclear factor kappa-light-chain-enhancer of activated B cells (NF-κB), and phosphatidylinositol-3-OH kinase (PI3K), can sustain the Warburg effect. As the tumor starts to grow, oxygen diffusion becomes limited and cancer cells respond to these environmental changes by upregulating HIF-1α (29). HIF-1α, in turn, induces the overexpression of key players in the conversion of glucose into lactate, such as glucose transporters (GLUTs) and hexokinase (HK) 1 and 2, which are responsible for the initial steps of glycolysis; lactate dehydrogenase A (LDHA), which transforms pyruvate into lactate (30, 31); and the lactate-extruding monocarboxylate transporter 4 (MCT4) (32). Conversely, HIF-1α blocks the entry of pyruvate into the TCA cycle by upregulating pyruvate dehydrogenase kinase 1 (PDK1), driving tumor cell energy to glycolysis (33). The major oncogene Ras, when mutated, can also induce glycolysis through the activation of the mammalian target of rapamycin complex I (mTORC1). Akt kinase activation by PI3K results in increased glucose uptake, HK2 targeting to the mitochondria, and increase in glycolytic flux (34), while the transcription factor MYC increases glutaminolysis and upregulates MCT1 expression (35). Cancer cell metabolism is also influenced by the activity of tumor suppressor genes. Loss of the p53 protein prevents expression of the synthesis of cytochrome c oxidase (SCO2) gene, decreasing mitochondrial respiration (36). Lactate can also function as a paracrine tumor molecule (37). Acidosis often precedes angiogenesis and lactate can stimulate HIF expression independently of hypoxia (38). Thus, instead of one event promoting the Warburg effect, numerous factors play a role in determining the fate of glucose in cancer cells. Also true is that somatic mutations in genes involved in metabolism either cause/predispose cells to become malignant. For instance, mutations in succinate dehydrogenase are related to paraganglioma, and mutations in fumarase can induce leiomyoma and leiomyosarcoma formation (39); isocitrate dehydrogenase mutations are related to glioma development (39).
Lactate Transport in Cancer
The major oncometabolite resulting from tumor metabolic rewiring is lactate, which is abundant in the tumor microenvironment (TME). Because lactic acid is hydrophilic and a weak acid, its transport across membranes requires transporters that belong to the monocarboxylate transporter family. MCTs 1–4 facilitate the transmembrane H+-linked transport of monocarboxylates, including lactate, pyruvate, acetoacetate, and β-hydroxybutyrate (15), having the cell surface glycoprotein CD147 as an obligatory chaperone (40). MCT1 and MCT4 isoforms are strongly associated with the hyperglycolytic phenotype of cancer cells. These transporters display distinct affinities for monocarboxylic acids that are associated with their expression patterns within tissues (41). MCT1 expression was identified in most tissues, being associated with the uptake/extrusion of lactate, while MCT4 has an important role in the export of lactate in highly glycolytic tissues. MCTs have been widely studied by our group and others, and found to be robustly expressed in a variety of solid human tumors such as colon, glioblastoma, breast, prostate, stomach and others, as depicted in Table 1 [for a comprehensive review see (41, 72)]. MCT expression/cell localization can differ from cancer to cancer. Importantly, the prognostic potential of MCTs was found in various tumor types (Table 1). Given the key role of MCTs in cancer, these transporters are promising therapeutic targets in cancer. A less studied family of lactate transporters, also known to facilitate the transport of monocarboxylates, are the sodium-coupled monocarboxylate transporters (SMCTs), containing two members, SLC5A8 and SLC5A12 (73).
Lactate Roles in the Tumor Microenvironment: From Metabolic Affairs to Signaling
Tumor growth occurs under a nutrient/oxygen-restrictive microenvironment where cancer cells are enrolled in a reprogrammed metabolism that allows them to surpass those limitations while facilitating malignant dissemination. Not only cancer cells, but also cancer-associated stromal cells, take part in such scavenging program, being cytosolic lactate the main driver of those metabolic alterations. Lactate is formed exclusively from pyruvate regardless of oxygen availability, and robustly exported to the TME, reaching concentrations that can be 20-fold higher (about 40 mM) (74) than in non-tumoral tissues (about 1.5–3 mM) (13, 15). Lactate is a major fuel source, providing energetic and anabolic support to cancer cells, and an important oncometabolite with both extracellular and intracellular signaling functions that equally contribute to cancer progression (Figure 1) (75, 76).
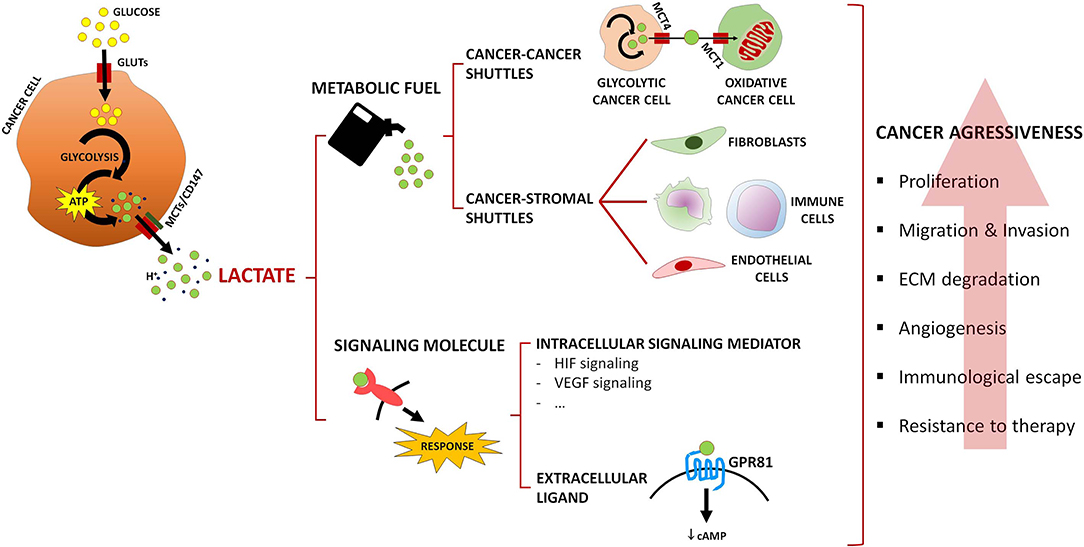
Figure 1. Summary of lactate roles in the tumor microenvironment. Lactate acts as a metabolic fuel, driving metabolic crosstalks involving MCT-mediated lactate shuttles among cancer cells, or between cancer cells and cancer-associated stromal cells. In addition, lactate acts as a signaling oncometabolite, intracellularly activating signaling pathways or acting as an extracellular ligand of the lactate receptor GPR81. Ultimately, cancer aggressiveness features are promoted, such as proliferation, migration, and invasion of cancer cells, extracellular matrix (ECM) degradation, angiogenesis, immunological escape, and resistance to therapy.
Lactate as a Metabolic Substrate
Lactate acts as a powerful regulator of multiple hallmarks of cancer, supporting cell proliferation and promoting immune suppression, angiogenesis, migration, metastasis, and resistance to therapy (20), namely chemotherapy (77), radiotherapy (78), and targeted therapy (79, 80). Cancer cells exploiting aerobic glycolysis upregulate GLUTs and MCTs, secreting large amounts of lactate (Figure 1), while deviating glycolytic intermediates to fuel alternative anabolic pathways (e.g., pentose phosphate pathway), thus sustaining rapid cell proliferation (81). Due to the metabolic heterogeneity of the TME, cancer cells are also able to engage into context-dependent metabolic affairs, similarly to what occurs in muscle (82) and brain (83). One such example is the symbiosis between well and poorly-oxygenated cancer cell populations (Figure 1): at the hypoxic, nutrient-poor/normoxic, nutrient-rich interface, lactate is released by glycolytic cancer cells through MCT4, and taken up by oxidative cancer cells through MCT1, where it fuels oxidative phosphorylation, thus sparing glucose for glycolytic cancer cells (84). Occurrence of this “two compartment model” was additionally described between lactate-avid breast cancer cells and “corruptible” glycolytic cancer-associated fibroblasts (CAF) by Lisantis's group (85), and further amplified to a “three compartment model” in the study by Curry et al. (86); in head and neck cancer samples, the authors showed that catabolic compartments composed of Warburg-adapted MCT4-expressing cancer cells and CAFs provided anabolic MCT1-expressing cancer cells with glycolysis-originating lactate (86). Since those original observations, numerous studies reported similar associations in several cancer models, such as non-Hodgkin lymphoma (87), pancreas (88), lung (89), prostate (90), and bladder (91); clinically, this metabolic phenotype has been associated with cancer aggressiveness, resistance to therapy and poor survival (89–91). Several mechanisms have been pointed out as mediators of those metabolic affairs, such as secretion of growth factors [e.g., cancer cell-secreted basic fibroblast growth factor (bFGF) in response to CAF-secreted hepatocyte growth factor (HGF) (92)], interleukins [e.g., IL-1β secretion by cancer cells (93)], and exosomal microRNAs (94). Interestingly, microRNA-containing exosomes secreted by CAFs were able to inhibit oxidative metabolism in cancer cells, while providing them with intact metabolites, namely glucose, to sustain their growth (95). In such an inverted scenario, CAFs oxidize cancer cell-derived lactate to support tumor proliferation (96); this has been correlated with resistance to targeted therapy (80).
In addition to CAFs, the metabolic promiscuity described above involves other cells of the TME, namely immune and endothelial cells (Figure 1). Cytotoxic T cells' transition from an anergic to a fully activated state relies on an accelerated glucose metabolism (97) and, in a glucose-restricted TME, cancer cells easily succeed in such metabolic competition (98). Dampening of lymphocyte proliferation and motility, cytokine production and cytotoxic activity ultimately leads to immunosuppression, as a result of the excess cancer cell-derived lactate that blocks lactate export by immune cells (99) and might be inclusively taken up by those cells, thus impairing their glycolysis-dependent activation (100). Lactate also mediates polarization of macrophages from an M1- (anti-tumoral type) to an M2-like phenotype (pro-tumoral type) (101, 102); induction of VEGF (vascular endothelial growth factor) expression has been linked to this pro-tumoral state (103). Moreover, it was proposed that endothelial cells rely on extracellular lactate uptake, via MCT1, as a fuel source for their oxidative metabolism, promoting VEGF/VEGFR-2 production through HIF-1α stabilization, endothelial cell migration and tube formation (104–106).
Lactate stimulates motility, migration and invasion of cancer cells (38, 107), as a probable result of CD44 expression and hyaluronan production (108), as well as activation of matrix metalloproteinases (MMPs) (109), both promoted by extracellular acidosis.
Lactate as a Signaling Metabolite
As stated above, lactate can serve additional purposes beyond acting as a metabolic substrate, functioning as an intracellular signaling mediator and as an extracellular ligand. At the intracellular level (Figure 1), hypoxia adaptation is assured by lactate in HIF-1α-dependent [direct HIF-1α stabilization by prolyl hydroxylase (PHD) 2 inhibition (110)] and independent [binding to N-Myc downstream-regulated (NDRG3) protein, preventing association with PHD2 (111)] fashions. HIF-2α stabilization is also induced by lactate, which ultimately potentiates glutaminolysis in cancer cells (112). Lactate promotes HIF-1α-mediated VEGF expression in the cancer cell, and expression of bFGF and VEGFR-2 by neighboring endothelial cells (105). Apart from this HIF-1α-dependent angiogenic signals, endothelial cells are also activated by NF-κB stabilization (104) in a lactate-dependent manner. NF-κB activation in cancer cells' instructed CAFs additionally drives resistance to targeted therapies, being lactate secreted by cancer cells the instructor in such phenotype (80). Pyruvate kinase M2/HIF-1α-driven gene expression in prostate cancer cells promoted epithelial-to-mesenchymal in response to CAF-secreted lactate (113). Immune suppression is also mediated by lactate signaling, as different studies reported that this oncometabolite interferes with key tumor pathways that lead to IFN-γ production by cytotoxic T cells (114), activates the IL-23/IL-17 proinflammatory pathway (115) and promotes polarization of macrophages toward an M2-like phenotype (103). Recently, functions in histone post-translational modification, termed histone lysine lactylation, were attributed to lactate and shown to regulate gene expression in macrophages; increased lactate production led to this epigenetic modification, inducing an M2-like phenotype during wound healing (116).
The signaling functions of lactate at the extracellular space (Figure 1) are mediated by the lactate-activated G-protein-coupled receptor GPR81 (5, 117). Its expression is not limited to the plasma membrane but also to other intracellular organelles (118). GPR81 activation occurs at a lactate concentration of 0.2–1.0 mM (119), followed by cyclic AMP downregulation and inhibition of protein kinase A (PKA)-mediated signaling (120). In the physiological context, lactate binds to GPR81, which inhibits lipolysis in fat cells (121). In the cancer context, modulation of lactate-sensing proteins, such as MCTs, ultimately leads to tumor proliferation and dissemination (122), escape from the immune system (123) and therapy resistance (124). GPR81 expression is upregulated in cervical (125), breast (126) and liver cancer (122), and associated with the progression of cervical squamous carcinoma (125). GPR81 is highly expressed in different cancer cell lines including colon, breast, lung, hepatocellular, cervical, and pancreatic (122, 126). In vitro, GPR81 expression associated with cancer cell survival, proliferation, migration, invasion and resistance to chemotherapy, and is involved in the suppression of antitumor immunity by promoting the overexpression of PD-L1 in lung cancer cells lines (123, 124, 126). Knockdown of GPR81 in a xenograft cancer model resulted in reduction of tumor growth and metastasis (122, 126).
The putative lactate sensors GPR4, GPR65, GPR68, and GPR132 have been described as proton-sensitive, and are activated at the acidic TME due to the low pH levels obtained from lactic and carbonic acids (5). GPR132 and GPR65 were additionally described in macrophages and linked to their polarization toward a pro-tumoral phenotype (127, 128). It remains to be clarified whether the modulation of lactate-sensing signaling pathways occurs through a direct GPR-lactate interaction ou through a conformational modification in the receptor induced by lactic acidosis.
Lactate Metabolism as A Prognostic and Therapeutic Tool
As mentioned above, lactate levels in tissues can mirror their metabolic status. Lactate concentrations vary either within healthy or diseased tissues, reflecting the distribution of the metabolic activity in the tissue, phenomenon known as “metabolic zonation” (129). This term was first described in the liver, in which there is hepatocyte metabolic heterogeneity along the porto-central axis, resulting from the physiological occurring oxygen gradient (130). In solid malignant tumors, which are characterized by high heterogeneity, “metabolic zonation” results from different intrinsic properties of cancer cells, co-existence of different cell populations within the tumor and different distribution of the vascular supply (129). The clinical significance of the variable levels of lactate in human solid tumors was first described in 2000 by the group of Walenta et al. (131), where significantly higher lactate levels in cervical metastatic tumors were found, compared with non-metastatic malignancies, suggesting that tumor lactate content could be used as a prognostic biomarker. Interestingly, the levels of lactate were inversely correlated with the levels of glucose, and directly correlated with the expression of MCT4. Later studies have also linked intratumoral lactate levels with higher incidence of distant metastasis and poor patient survival (76).
Prognostic value has also been attributed to important players in lactate metabolism, namely involved in lactate production (LDHA) and lactate transport (MCTs). There are several studies on the prognostic value of LDH levels, supported by systematic reviews/meta-analyses (132). As examples, higher pretreatment LDH concentration is associated with increased risk of overall mortality in lung cancer patients (133), high LDH serum levels are associated with lower event-free survival (EFS) in osteosarcoma patients (134) and with overall survival (OS)/progression-free survival (PFS) in urinary system cancer patients (135). Besides serum LDH, LDHA levels in cancer tissues have been reported as a biomarker of malignancy and prognosis (136). As examples, upregulation of LDHA levels in pancreatic and esophageal cancer have been associated with metastasis, tumor stage, tumor recurrence, and patient survival (137). However, LDHA expression in malignant tumor tissues does not correlate consistently with serum LDH levels, which may indicate that these are independent prognostic factors in cancer (138, 139). Studies on lactate transporters (MCTs/CD147) are not as solid as for LDH, but also reveal prognostic value (Table 1) (41). In a recent meta-analysis Bovenzi et al. (140) identified association between increased MCT4/CD147 expression with decreased OS and disease-free survival (DFS) across many cancer types, while there was no clear association for MCT1 expression with these parameters.
Besides prognostic biomarkers, both LDHA and MCTs have been recognized as attractive targets for cancer therapy. LDHA overexpression has been associated with increased cancer aggressiveness and targeting has been tackled both genetically and pharmacologically. There are different types of LDHA inhibitors, including the pyruvate-competitive (e.g., oxamate), the NADH-competitive (e.g., gossypol), the pyruvate and NADH-competitive (N-hydroxyindoles), and the free enzyme-binding inhibitors (galloflavin) (138). LDH pharmacological inhibition reduces lactate production, impairs cell proliferation in vitro, and reduces tumor size in vivo, either with LDH inhibitors alone or in combination with other agents (138, 141). Additionally, gossypol has also demonstrated promising results in different clinical trials, being relatively safe and effective in reducing tumor markers (138, 142). These results are supported by LDHA silencing studies in tumor models, where cell proliferation, migration and tumor growth were prevented (143, 144). However, genetic studies deleting LDHA/LDHB or glucose phospho-isomerase (GPI) have demonstrated that Warburg effect is dispensable as agressive tumors, relying on OXPHOS, are able to survive and to develop tumors in nude mice (145).
As stated above, upregulation of MCT1 and MCT4 has been described in a variety of human cancers, and inhibition of MCT activity has been showing promising results in pre-clinical models (41). In vitro, MCT inhibition impairs lactate transport, cell proliferation, invasion and migration, and induces cell death, while it delays tumor growth, induces necrosis and decreases invasion in vivo. MCT activity has been inhibited either genetically (gene downregulation or knockout) or using pharmacological inhibitors. MCT classical inhibitors include the α-cyano-4-hydroxycinnamate CHC, 4,4′-di-isothiocyanostilbene-2,2′-disulfonates (e.g., DIDS) and flavonoids (e.g., quercetin) (15). However, these inhibitors are not MCT/MCT isoform specific, which prompted the search for new inhibitors. AstraZeneca developed MCT1 specific inhibitors, and one of them (AZD3965) already reached clinical trials in the cancer setting. After a first evaluation of compound tolerability, the trial is now set to evaluate the effect of AZD3965 in MCT1 positive tumors with pre-clinical positive results (146).
Information on the use of lactate by tumors could also be of value in cancer therapy. Van Hée et al. developed a PET tracer of lactate [(±)- [18F]-3-fluoro-2-hydroxypropionate, [18F]-FLac] to monitor MCT1-dependent lactate uptake in tumors (147). The authors propose that this tracer can be used to predict response to treatments that disrupt lactate consumption, with potential to allow personalized patient treatment.
Discussion
Along the previous decades, the role of lactate has been overlooked, as it was seen as a mere metabolic waste of cell glycolytic metabolism. However, recent evidence has been revealing new and important oncogenic roles of lactate in malignant tumors. Lactate can function either as metabolic fuel for oxidative cells or as signaling molecule in the TME, being responsible for several aggressiveness cancer cell features, namely proliferation, migration and invasion, angiogenesis, escape to the immune system and resistance to therapy (Figure 1). Besides, upregulation of key proteins involved in lactate metabolism, namely LDHA and MCTs, have demonstrated clinical prognostic value and are seen as rational targets for cancer therapy. Thus, given the important role of lactate metabolism in cancer aggressiveness and response to therapy, lactate metabolism inhibitors should to be further explored in the clinical setting, especially in combination with classical therapy, molecular targeted drugs and immunotherapy.
Author Contributions
All authors listed have made a substantial, direct and intellectual contribution to the work, and approved it for publication.
Funding
This article has been developed under the scope of the project NORTE-01-0145-FEDER- 000013, supported by the Northern Portugal Regional Operational Programme (NORTE 2020) under the Portugal Partnership Agreement, through the European Regional Development Fund (FEDER), and through the Competitiveness Factors Operational Programme (COMPETE) and by National funds, through the Foundation for Science and Technology (FCT), under the scope of the project POCI-01-0145-FEDER-007038. JA and SG received fellowships from FCT, ref. SFRH/BPD/116784/2016 and SFRH/BPD/117858/2016, respectively.
Conflict of Interest
The authors declare that the research was conducted in the absence of any commercial or financial relationships that could be construed as a potential conflict of interest.
The handling editor declared a shared affiliation, though no other collaboration, with one of the authors FB.
References
1. Vonmuralt A. The development of muscle-chemistry, a lesson in neurophysiology. Biochim Biophys Acta. (1950) 4:126–9. doi: 10.1016/0006-3002(50)90015-1
2. McComas AJ. The neuromuscular system. In: Tipton C, editor. Exercise Physiology: People and Ideas. New York, NY: Springer-Verlag (2003). p. 72–7.
3. Kresge N, Simoni RD, Hill RL. JBC Centennial - 1905-2005 - 100 years of biochemistry and molecular biology - Otto Fritz Meyerhof and the elucidation of the glycolytic pathway. J Biol Chem. (2005) e3:280. Available online at: http://www.jbc.org/content/280/4/e3
4. Brooks GA. Metabolic systems: the formation and utilization of lactate. In: Tipton CM, editor. History of Exercise Physiology. Champaign, IL: Human Kinetics (2014). p. 447–75.
5. Parks SK, Mueller-Klieser W, Pouysségur J. Lactate and acidity in the cancer microenvironment. Ann Rev Cancer Biol. (2020) 4:141–58. doi: 10.1146/annurev-cancerbio-030419-033556
6. Heiden MGV, Cantley LC, Thompson CB. Understanding the warburg effect: the metabolic requirements of cell proliferation. Science. (2009) 324:1029–33. doi: 10.1126/science.1160809
7. Brooks GA. Lactate: glycolytic end product and oxidative substrate during sustained exercise in mammals - the “lactate shuttle.” In: Gilles R, editor. Circulation, Respiration and Metabolism: Current Comparative Approaches. Champaign, IL: Human Kinetics (2014). p. 3-65.
8. Brooks GA. The lactate shuttle during exercise and recovery. Med Sci Sports Exerc. (1986) 18:360–8. doi: 10.1249/00005768-198606000-00019
9. Brooks GA. Lactate production under fully aerobic conditions - the lactate shuttle during rest and exercise. Fed Proc. (1986) 45:2924–9.
10. Sahlin K, Fernstrom M, Svensson M, Tonkonogi M. No evidence of an intracellular lactate shuttle in rat skeletal muscle. J Physiol. (2002) 541:569–74. doi: 10.1113/jphysiol.2002.016683
11. Laureys G, Valentino M, Demol F, Zammit C, Muscat R, Cambron M, et al. β2-adrenergic receptors protect axons during energetic stress but do not influence basal glio-axonal lactate shuttling in mouse white matter. Neuroscience. (2014) 277:367–74. doi: 10.1016/j.neuroscience.2014.07.022
12. Mason S. Lactate shuttles in neuroenergetics-homeostasis, allostasis and beyond. Front Neurosci. (2017) 11:43. doi: 10.3389/fnins.2017.00043
13. de Bari L, Atlante A. Including the mitochondrial metabolism of L-lactate in cancer metabolic reprogramming. Cell Mol Life Sci. (2018) 75:2763–76. doi: 10.1007/s00018-018-2831-y
14. Pucino V, Bombardieri M, Pitzalis C, Mauro C. Lactate at the crossroads of metabolism, inflammation, and autoimmunity. Eur J Immunol. (2017) 47:14–21. doi: 10.1002/eji.201646477
15. Halestrap AP. The SLC16 gene family - structure, role and regulation in health and disease. Mol Aspects Med. (2013) 34:337–49. doi: 10.1016/j.mam.2012.05.003
16. Phypers B, Pierce JT. Lactate physiology in health and disease. Cont Educ Anaesth Crit Care Pain. (2006) 6:128–32. doi: 10.1093/bjaceaccp/mkl018
17. Zuo RJ, Gu XW, Qi QR, Wang TS, Zhao XY, Liu JL Warburg-like glycolysis and lactate shuttle in mouse decidua during early pregnancy. J Biol Chem. (2015) 290:21280–91. doi: 10.1074/jbc.M115.656629
18. Kuchiiwa T, Nio-Kobayashi J, Takahashi-Iwanaga H, Yajima T, Iwanaga T. Cellular expression of monocarboxylate transporters in the female reproductive organ of mice: implications for the genital lactate shuttle. Histochem Cell Biol. (2011) 135:351–60. doi: 10.1007/s00418-011-0794-2
19. Rakus D, Gizak A, Wisniewski JR. Proteomics unveils fibroblast-cardiomyocyte lactate shuttle and hexokinase paradox in mouse muscles. J Proteome Res. (2016) 15:2479–90. doi: 10.1021/acs.jproteome.5b01149
20. San-Millan I, Brooks GA. Reexamining cancer metabolism: lactate production for carcinogenesis could be the purpose and explanation of the Warburg Effect. Carcinogenesis. (2017) 38:119–33. doi: 10.1093/carcin/bgw127
21. Andersen LW, Mackenhauer J, Roberts JC, Berg KM, Cocchi MN, Donnino MW. Etiology and therapeutic approach to elevated lactate levels. Mayo Clin Proc. (2013) 88:1127–40. doi: 10.1016/j.mayocp.2013.06.012
22. Cassim S, Pouyssegur J. Tumor microenvironment: a metabolic player that shapes the immune response. Int J Mol Sci. (2019) 21:E157. doi: 10.3390/ijms21010157
23. Haas R, Smith J, Rocher-Ros V, Nadkarni S, Montero-Melendez T, D'Acquisto F, et al. Lactate regulates metabolic and pro-inflammatory circuits in control of T cell migration and effector functions. PLoS Biol. (2015) 13:e1002202. doi: 10.1371/journal.pbio.1002202
24. Yang Z, Matteson EL, Goronzy JJ, Weyand CM. T-cell metabolism in autoimmune disease. Arthritis Res Ther. (2015) 17:29. doi: 10.1186/s13075-015-0542-4
25. Warburg O. On the origin of cancer cells. Science. (1956) 123:309–14. doi: 10.1126/science.123.3191.309
26. Kroemer G, Pouyssegur J. Tumor cell metabolism: cancer's Achilles' heel. Cancer Cell. (2008) 13:472–82. doi: 10.1016/j.ccr.2008.05.005
27. Warburg O, Wind F, Negelein E. The metabolism of tumors in the body. J Gen Physiol. (1927) 8:519–30. doi: 10.1085/jgp.8.6.519
28. Hanahan D, Weinberg RA. Hallmarks of cancer: the next generation. Cell. (2011) 144:646–74. doi: 10.1016/j.cell.2011.02.013
30. Yu L, Chen X, Sun X, Wang L, Chen S. The glycolytic switch in tumors: how many players are involved? J Cancer. (2017) 8:3430–40. doi: 10.7150/jca.21125
31. Semenza GL. HIF-1: upstream and downstream of cancer metabolism. Curr Opin Genet Dev. (2010) 20:51–6. doi: 10.1016/j.gde.2009.10.009
32. Ullah MS, Davies AJ, Halestrap AP. The plasma membrane lactate transporter MCT4, but not MCT1, is up-regulated by hypoxia through a HIF-1α-dependent mechanism. J Biol Chem. (2006) 281:9030–7. doi: 10.1074/jbc.M511397200
33. Kim JW, Tchernyshyov I, Semenza GL, Dang CV. HIF-1-mediated expression of pyruvate dehydrogenase kinase: a metabolic switch required for cellular adaptation to hypoxia. Cell Metab. (2006) 3:177–85. doi: 10.1016/j.cmet.2006.02.002
34. Koundouros N, Poulogiannis G. Phosphoinositide 3-Kinase/Akt signaling and redox metabolism in cancer. Front Oncol. (2018) 8:160. doi: 10.3389/fonc.2018.00160
35. Burns JS, Manda G. Metabolic pathways of the warburg effect in health and disease: perspectives of choice, chain or chance. Int J Mol Sci. (2017) 18:E2755. doi: 10.3390/ijms18122755
36. Wanka C, Brucker DP, Bahr O, Ronellenfitsch M, Weller M, Steinbach JP, et al. Synthesis of cytochrome C oxidase 2: a p53-dependent metabolic regulator that promotes respiratory function and protects glioma and colon cancer cells from hypoxia-induced cell death. Oncogene. (2012) 31:3764–76. doi: 10.1038/onc.2011.530
37. Santos N, Pereira-Nunes A, Baltazar F, Granja S. Lactate as a regulator of cancer inflammation and immunity. Immunometabolism. (2019) 1:e190015. doi: 10.20900/immunometab20190015
38. Estrella V, Chen T, Lloyd M, Wojtkowiak J, Cornnell HH, Ibrahim-Hashim A, et al. Acidity generated by the tumor microenvironment drives local invasion. Cancer Res. (2013) 73:1524–35. doi: 10.1158/0008-5472.CAN-12-2796
39. Thompson CB. Metabolic enzymes as oncogenes or tumor suppressors. N Engl J Med. (2009) 360:813–5. doi: 10.1056/NEJMe0810213
40. Muramatsu T. Basigin (CD147), a multifunctional transmembrane glycoprotein with various binding partners. J Biochem. (2016) 159:481–90. doi: 10.1093/jb/mvv127
41. Granja S, Tavares-Valente D, Queiros O, Baltazar F. Value of pH regulators in the diagnosis, prognosis and treatment of cancer. Semin Cancer Biol. (2017) 43:17–34. doi: 10.1016/j.semcancer.2016.12.003
42. Miranda-Goncalves V, Honavar M, Pinheiro C, Martinho O, Pires MM, Pinheiro C, et al. Monocarboxylate transporters (MCTs) in gliomas: expression and exploitation as therapeutic targets. Neurooncology. (2013) 15:172–88. doi: 10.1093/neuonc/nos298
43. Simoes-Sousa S, Granja S, Pinheiro C, Fernandes D, Longatto-Filho A, Laus AC, et al. Prognostic significance of monocarboxylate transporter expression in oral cavity tumors. Cell Cycle. (2016) 15:1865–73. doi: 10.1080/15384101.2016.1188239
44. Pinheiro C, Albergaria A, Paredes J, Sousa B, Dufloth R, Vieira D, et al. Monocarboxylate transporter 1 is up-regulated in basal-like breast carcinoma. Histopathology. (2010) 56:860–7. doi: 10.1111/j.1365-2559.2010.03560.x
45. Doyen J, Trastour C, Ettore F, Peyrottes I, Toussant N, Gal J, et al. Expression of the hypoxia-inducible monocarboxylate transporter MCT4 is increased in triple negative breast cancer and correlates independently with clinical outcome. Biochem Biophys Res Commun. (2014) 451:54–61. doi: 10.1016/j.bbrc.2014.07.050
46. Granja S, Marchiq I, Le Floch R, Moura CS, Baltazar F, Pouyssegur J. Disruption of BASIGIN decreases lactic acid export and sensitizes non-small cell lung cancer to biguanides independently of the LKB1 status. Oncotarget. (2015) 6:6708–21. doi: 10.18632/oncotarget.2862
47. Eilertsen M, Andersen S, Al-Saad S, Kiselev Y, Donnem T, Stenvold H, et al. Monocarboxylate transporters 1-4 in NSCLC: MCT1 is an independent prognostic marker for survival. PLoS ONE. (2014) 9:e105038. doi: 10.1371/journal.pone.0105038
48. Stewart PA, Parapatics K, Welsh EA, Muller AC, Cao H, Fang B, et al. A Pilot Proteogenomic Study with Data Integration Identifies MCT1 and GLUT1 as prognostic markers in lung adenocarcinoma. PLoS ONE. (2015) 10:e0142162. doi: 10.1371/journal.pone.0142162
49. Alves VA, Pinheiro C, Morais-Santos F, Felipe-Silva A, Longatto-Filho A, Baltazar F. Characterization of monocarboxylate transporter activity in hepatocellular carcinoma. World J Gastroenterol. (2014) 20:11780–7. doi: 10.3748/wjg.v20.i33.11780
50. Gao HJ, Zhao MC, Zhang YJ, Zhou DS, Xu L, Li GB, et al. Monocarboxylate transporter 4 predicts poor prognosis in hepatocellular carcinoma and is associated with cell proliferation and migration. J Cancer Res Clin Oncol. (2014) 141:1151–62. doi: 10.1007/s00432-014-1888-8
51. Schneiderhan W, Scheler M, Holzmann KH, Marx M, Gschwend JE, Bucholz M, et al. CD147 silencing inhibits lactate transport and reduces malignant potential of pancreatic cancer cells in in vivo and in vitro models. Gut. (2009) 58:1391–8. doi: 10.1136/gut.2009.181412
52. Sukeda A, Nakamura Y, Nishida Y, Kojima M, Gotohda N, Akimoto T, et al. Expression of monocarboxylate transporter 1 is associated with better prognosis and reduced nodal metastasis in pancreatic ductal adenocarcinoma. Pancreas. (2019) 48:1102–10. doi: 10.1097/MPA.0000000000001369
53. Pinheiro C, Longatto-Filho A, Simoes K, Jacob CE, Bresciani CJ, Zilberstein B, et al. The prognostic value of CD147/EMMPRIN is associated with monocarboxylate transporter 1 co-expression in gastric cancer. Eur J Cancer. (2009) 45:2418–24. doi: 10.1016/j.ejca.2009.06.018
54. Pinheiro C, Longatto-Filho A, Scapulatempo C, Ferreira L, Martins S, Pellerin L, et al. Increased expression of monocarboxylate transporters 1, 2, and 4 in colorectal carcinomas. Virchows Arch. (2008) 452:139–46. doi: 10.1007/s00428-007-0558-5
55. Abe Y, Nakayama Y. The prognostic significance of the expression of monocarboxylate transporter 4 in patients with right- or left-sided colorectal cancer. Asia Pac J Clin Onco. (2019) 15:e49–55. doi: 10.1111/ajco.13077
56. Javaeed A, Ghauri SK. MCT4 has a potential to be used as a prognostic biomarker - a systematic review and meta-analysis. Oncol Rev. (2019) 13:403. doi: 10.4081/oncol.2019.403
57. Afonso J, Santos LL, Miranda-Goncalves V, Morais A, Amaro T, Longatto-Filho A, et al. CD147 and MCT1-potential partners in bladder cancer aggressiveness and cisplatin resistance. Mol Carcinogen. (2015) 54:1451–66. doi: 10.1002/mc.22222
58. Choi JW, Kim Y, Lee JH, Kim YS. Prognostic significance of lactate/proton symporters MCT1, MCT4, and their chaperone CD147 expressions in urothelial carcinoma of the bladder. Urology. (2014) 84:245.e249–15. doi: 10.1016/j.urology.2014.03.031
59. Pertega-Gomes N, Vizcaino JR, Miranda-Goncalves V, Pinheiro C, Silva J, Pereira H, et al. Monocarboxylate transporter 4 (MCT4) and CD147 overexpression is associated with poor prognosis in prostate cancer. BMC Cancer. (2011) 11:312. doi: 10.1186/1471-2407-11-312
60. Kim Y, Choi JW, Lee JH, Kim YS. Expression of lactate/H+ symporters MCT1 and MCT4 and their chaperone CD147 predicts tumor progression in clear cell renal cell carcinoma: immunohistochemical and The Cancer Genome Atlas data analyses. Hum Pathol. (2015) 46:104–12. doi: 10.1016/j.humpath.2014.09.013
61. Cao YW, Liu Y, Dong Z, Guo L, Kang EH, Wang YH, et al. Monocarboxylate transporters MCT1 and MCT4 are independent prognostic biomarkers for the survival of patients with clear cell renal cell carcinoma and those receiving therapy targeting angiogenesis. Urol Oncol. (2018) 36:311.e315–25. doi: 10.1016/j.urolonc.2018.03.014
62. Ambrosetti D, Dufies M, Dadone B, Durand M, Borchiellini D, Amiel J, et al. The two glycolytic markers GLUT1 and MCT1 correlate with tumor grade and survival in clear-cell renal cell carcinoma. PLoS ONE. (2018) 13:e0193477. doi: 10.1371/journal.pone.0193477
63. Almeida L, Silva R, Cavadas B, Lima J, Pereira L, Soares P, et al. GLUT1, MCT1/4 and CD147 overexpression supports the metabolic reprogramming in papillary renal cell carcinoma. Histol Histopathol. (2017) 32:1029–40. doi: 10.14670/HH-11-863
64. Chen H, Wang L, Beretov J, Hao J, Xiao W, Li Y. Co-expression of CD147/EMMPRIN with monocarboxylate transporters and multiple drug resistance proteins is associated with epithelial ovarian cancer progression. Clin Exp Metast. (2010) 27:557–69. doi: 10.1007/s10585-010-9345-9
65. Pinheiro C, Longatto-Filho A, Ferreira L, Pereira SM, Etlinger D, Moreira MA, et al. Increasing expression of monocarboxylate transporters 1 and 4 along progression to invasive cervical carcinoma. Int J Gynecol Pathol. (2008) 27:568–74. doi: 10.1097/PGP.0b013e31817b5b40
66. Pinheiro C, Longatto-Filho A, Pereira SM, Etlinger D, Moreira MA, Jube LF, et al. Monocarboxylate transporters 1 and 4 are associated with CD147 in cervical carcinoma. Disease Mark. (2009) 26:97–103. doi: 10.1155/2009/169678
67. Ho J, de Moura MB, Lin Y, Vincent G, Thorne S, Duncan LM, et al. Importance of glycolysis and oxidative phosphorylation in advanced melanoma. Mol Cancer. (2012) 11:76. doi: 10.1186/1476-4598-11-76
68. Pinheiro C, Miranda-Goncalves V, Longatto-Filho A, Vicente AL, Berardinelli GN, Scapulatempo-Neto C, et al. The metabolic microenvironment of melanomas: Prognostic value of MCT1 and MCT4. Cell Cycle. (2016) 15:1462–70. doi: 10.1080/15384101.2016.1175258
69. Pinheiro C, Granja S, Longatto-Filho A, Faria AM, Fragoso MC, Lovisolo SM, et al. Metabolic reprogramming: a new relevant pathway in adult adrenocortical tumors. Oncotarget. (2015) 6:44403–21. doi: 10.18632/oncotarget.5623
70. Martinez-Outschoorn UE, Whitaker-Menezes D, Valsecchi M, Martinez-Cantarin MP, Dulau-Florea A, et al. Reverse Warburg effect in a patient with aggressive B-cell lymphoma: is lactic acidosis a paraneoplastic syndrome? Semin Oncol. (2013) 40:403–18. doi: 10.1053/j.seminoncol.2013.04.016
71. Afonso J, Pinto T, Simoes-Sousa S, Schmitt F, Longatto-Filho A, Pinheiro C, et al. Clinical significance of metabolism-related biomarkers in non-Hodgkin lymphoma - MCT1 as potential target in diffuse large B cell lymphoma. Cell Oncol. (2019) 42:303–18. doi: 10.1007/s13402-019-00426-2
72. Céline Pinheiro FM, Granja S, Gonçalves VM, Afonso J, Amorim R, Baltazar F. Targeting metabolic reprogramming as an anti-cancer strategy: aiming at monocarboxylate transporters. In: Atta-ur-Rahman FRS, ChoudharyMI, editors. Frontiers in Anti-Cancer Drug Discovery. Vol. 6. Bentham: e books (2016). p. 3–65.
73. Morris ME, Felmlee MA. Overview of the proton-coupled MCT (SLC16A) family of transporters: characterization, function and role in the transport of the drug of abuse γ-hydroxybutyric acid. AAPS J. (2008) 10:311–21. doi: 10.1208/s12248-008-9035-6
74. Walenta S, Mueller-Klieser WF. Lactate: mirror and motor of tumor malignancy. Semin Radiat Oncol. (2004) 14:267–74. doi: 10.1016/j.semradonc.2004.04.004
75. Ippolito L, Morandi A, Giannoni E, Chiarugi P. Lactate: a metabolic driver in the tumour landscape. Trends Biochem Sci. (2019) 44:153–66. doi: 10.1016/j.tibs.2018.10.011
76. de la Cruz-Lopez KG, Castro-Munoz LJ, Reyes-Hernandez DO, Garcia-Carranca A, Manzo-Merino J. Lactate in the regulation of tumor microenvironment and therapeutic approaches. Front Oncol. (2019) 9:1143. doi: 10.3389/fonc.2019.01143
77. Wagner W, Ciszewski WM, Kania KD. L- and D-lactate enhance DNA repair and modulate the resistance of cervical carcinoma cells to anticancer drugs via histone deacetylase inhibition and hydroxycarboxylic acid receptor 1 activation. Cell Commun Signal. (2015) 13:36. doi: 10.1186/s12964-015-0114-x
78. Hao J, Graham P, Chang L, Ni J, Wasinger V, Beretov J, et al. Proteomic identification of the lactate dehydrogenase A in a radioresistant prostate cancer xenograft mouse model for improving radiotherapy. Oncotarget. (2016) 7:74269–85. doi: 10.18632/oncotarget.12368
79. Allen E, Mieville P, Warren CM, Saghafinia S, Li L, Peng MW, et al. Metabolic symbiosis enables adaptive resistance to anti-angiogenic therapy that is dependent on mTOR signaling. Cell Rep. (2016) 15:1144–60. doi: 10.1016/j.celrep.2016.04.029
80. Apicella M, Giannoni E, Fiore S, Ferrari KJ, Fernandez-Perez D, Isella C, et al. Increased lactate secretion by cancer cells sustains non-cell-autonomous adaptive resistance to MET and EGFR targeted therapies. Cell Metab. (2018) 28:848–65.e846. doi: 10.1016/j.cmet.2018.08.006
81. Lunt SY, Vander Heiden MG. Aerobic glycolysis: meeting the metabolic requirements of cell proliferation. Annu Rev Cell Dev Biol. (2011) 27:441–64. doi: 10.1146/annurev-cellbio-092910-154237
82. Park JM, Josan S, Mayer D, Hurd RE, Chung Y, Bendahan D, et al. Hyperpolarized 13C NMR observation of lactate kinetics in skeletal muscle. J Exp Biol. (2015) 218:3308–18. doi: 10.1242/jeb.123141
83. Machler P, Wyss MT, Elsayed M, Stobart J, Gutierrez R, von Faber-Castell A, et al. In vivo evidence for a lactate gradient from astrocytes to neurons. Cell Metab. (2016) 23:94–102. doi: 10.1016/j.cmet.2015.10.010
84. Sonveaux P, Vegran F, Schroeder T, Wergin MC, Verrax J, Rabbani ZN, et al. Targeting lactate-fueled respiration selectively kills hypoxic tumor cells in mice. J Clin Invest. (2008) 118:3930–42. doi: 10.1172/JCI36843
85. Pavlides S, Whitaker-Menezes D, Castello-Cros R, Flomenberg N, Witkiewicz AK, Frank PG, et al. The reverse Warburg effect: aerobic glycolysis in cancer associated fibroblasts and the tumor stroma. Cell Cycle. (2009) 8:3984–4001. doi: 10.4161/cc.8.23.10238
86. Curry JM, Tuluc M, Whitaker-Menezes D, Ames JA, Anantharaman A, Butera A, et al. Cancer metabolism, stemness and tumor recurrence: MCT1 and MCT4 are functional biomarkers of metabolic symbiosis in head and neck cancer. Cell Cycle. (2013) 12:1371–84. doi: 10.4161/cc.24092
87. Gooptu M, Whitaker-Menezes D, Sprandio J, Domingo-Vidal M, Lin Z, Uppal G, et al. Mitochondrial and glycolytic metabolic compartmentalization in diffuse large B-cell lymphoma. Semin Oncol. (2017) 44:204–17. doi: 10.1053/j.seminoncol.2017.10.002
88. Shan T, Chen S, Chen X, Lin WR, Li W, Ma J, et al. Cancer-associated fibroblasts enhance pancreatic cancer cell invasion by remodeling the metabolic conversion mechanism. Oncol Rep. (2017) 37:1971–9. doi: 10.3892/or.2017.5479
89. Cruz-Bermudez A, Laza-Briviesca R, Vicente-Blanco RJ, Garcia-Grande A, Coronado MJ, Laine-Menendez S, et al. Cancer-associated fibroblasts modify lung cancer metabolism involving ROS and TGF-β signaling. Free Rad Biol Med. (2019) 130:163–73. doi: 10.1016/j.freeradbiomed.2018.10.450
90. Pertega-Gomes N, Vizcaino JR, Attig J, Jurmeister S, Lopes C, et al. A lactate shuttle system between tumour and stromal cells is associated with poor prognosis in prostate cancer. BMC Cancer. (2014) 14:352. doi: 10.1186/1471-2407-14-352
91. Afonso J, Santos LL, Morais A, Amaro T, Longatto-Filho A, Baltazar F. Metabolic coupling in urothelial bladder cancer compartments and its correlation to tumor aggressiveness. Cell Cycle. (2016) 15:368–80. doi: 10.1080/15384101.2015.1121329
92. Kumar D, New J, Vishwakarma V, Joshi R, Enders J, Lin F, et al. Cancer-associated fibroblasts drive glycolysis in a targetable signaling loop implicated in head and neck squamous cell carcinoma progression. Cancer Res. (2018) 78:3769–82. doi: 10.1158/0008-5472.CAN-17-1076
93. Wu J, Hong Y, Wu T, Wang J, Chen X, Wang Z, et al. Stromal-epithelial lactate shuttle induced by tumorderived interleukin-1β promotes cell proliferation in oral squamous cell carcinoma. Int J Mol Med. (2018) 41:687–96. doi: 10.3892/ijmm.2017.3267
94. Dai G, Yao X, Zhang Y, Gu J, Geng Y, Xue F, et al. Colorectal cancer cell-derived exosomes containing miR-10b regulate fibroblast cells via the PI3K/Akt pathway. Bull Du Cancer. (2018) 105:336–49. doi: 10.1016/j.bulcan.2017.12.009
95. Zhao H, Yang L, Baddour J, Achreja A, Bernard V, Moss T, et al. Tumor microenvironment derived exosomes pleiotropically modulate cancer cell metabolism. eLife. (2016) 5:e10250. doi: 10.7554/eLife.10250
96. Koukourakis MI, Kalamida D, Mitrakas AG, Liousia M, Pouliliou S, Sivridis E, et al. Metabolic cooperation between co-cultured lung cancer cells and lung fibroblasts. Lab Invest. (2017) 97:1321–31. doi: 10.1038/labinvest.2017.79
97. Palmer CS, Ostrowski M, Balderson B, Christian N, Crowe SM. Glucose metabolism regulates T cell activation, differentiation, and functions. Front Immunol. (2015) 6:1. doi: 10.3389/fimmu.2015.00001
98. Renner K, Singer K, Koehl GE, Geissler EK, Peter K, Siska PJ, et al. Metabolic hallmarks of tumor and immune cells in the tumor microenvironment. Front Immunol. (2017) 8:248. doi: 10.3389/fimmu.2017.00248
99. Romero-Garcia S, Moreno-Altamirano MM, Prado-Garcia H, Sanchez-Garcia FJ. Lactate contribution to the tumor microenvironment: mechanisms, effects on immune cells and therapeutic relevance. Front Immunol. (2016) 7:52. doi: 10.3389/fimmu.2016.00052
100. Hargadon KM. Strategies to improve the efficacy of dendritic cell-based immunotherapy for melanoma. Front Immunol. (2017) 8:1594. doi: 10.3389/fimmu.2017.01594
101. Lin S, Sun L, Lyu X, Ai X, Du D, Su N, et al. Lactate-activated macrophages induced aerobic glycolysis and epithelial-mesenchymal transition in breast cancer by regulation of CCL5-CCR5 axis: a positive metabolic feedback loop. Oncotarget. (2017) 8:110426–43. doi: 10.18632/oncotarget.22786
102. Zhao Y, Wang D, Xu T, Liu P, Cao Y, Wang Y, et al. Bladder cancer cells re-educate TAMs through lactate shuttling in the microfluidic cancer microenvironment. Oncotarget. (2015) 6:39196–3210. doi: 10.18632/oncotarget.5538
103. Colegio OR, Chu NQ, Szabo AL, Chu T, Rhebergen AM, Jairam V, et al. Functional polarization of tumour-associated macrophages by tumour-derived lactic acid. Nature. (2014) 513:559–63. doi: 10.1038/nature13490
104. Vegran F, Boidot R, Michiels C, Sonveaux P, Feron O. Lactate influx through the endothelial cell monocarboxylate transporter MCT1 supports an NF-κB/IL-8 pathway that drives tumor angiogenesis. Cancer Res. (2011) 71:2550–60. doi: 10.1158/0008-5472.CAN-10-2828
105. Sonveaux P, Copetti T, De Saedeleer CJ, Vegran F, Verrax J, Kennedy KM, et al. Targeting the lactate transporter MCT1 in endothelial cells inhibits lactate-induced HIF-1 activation and tumor angiogenesis. PLoS ONE. (2012) 7:e33418. doi: 10.1371/journal.pone.0033418
106. Miranda-Goncalves V, Bezerra F, Costa-Almeida R, Freitas-Cunha M, Soares R, Martinho O, et al. Monocarboxylate transporter 1 is a key player in glioma-endothelial cell crosstalk. Mol Carcinog. (2017) 56:2630–42. doi: 10.1002/mc.22707
107. Goetze K, Walenta S, Ksiazkiewicz M, Kunz-Schughart LA, Mueller-Klieser W. Lactate enhances motility of tumor cells and inhibits monocyte migration and cytokine release. Int J Oncol. (2011) 39:453–63. doi: 10.3892/ijo.2011.1055
108. Stern R, Shuster S, Neudecker BA, Formby B. Lactate stimulates fibroblast expression of hyaluronan and CD44: the Warburg effect revisited. Exp Cell Res. (2002) 276:24–31. doi: 10.1006/excr.2002.5508
109. Kato Y, Ozawa S, Tsukuda M, Kubota E, Miyazaki K, St-Pierre Y, et al. Acidic extracellular pH increases calcium influx-triggered phospholipase D activity along with acidic sphingomyelinase activation to induce matrix metalloproteinase-9 expression in mouse metastatic melanoma. FEBS J. (2007) 274:3171–83. doi: 10.1111/j.1742-4658.2007.05848.x
110. Lu H, Dalgard CL, Mohyeldin A, McFate T, Tait AS, Verma A. Reversible inactivation of HIF-1 prolyl hydroxylases allows cell metabolism to control basal HIF-1. J Biol Chem. (2005) 280:41928–39. doi: 10.1074/jbc.M508718200
111. Lee DC, Sohn HA, Park ZY, Oh S, Kang YK, Lee KM, et al. A lactate-induced response to hypoxia. Cell. (2015) 161:595–609. doi: 10.1016/j.cell.2015.03.011
112. Perez-Escuredo J, Dadhich RK, Dhup S, Cacace A, Van Hee VF, De Saedeleer CJ, et al. Lactate promotes glutamine uptake and metabolism in oxidative cancer cells. Cell Cycle. (2016) 15:72–83. doi: 10.1080/15384101.2015.1120930
113. Giannoni E, Taddei ML, Morandi A, Comito G, Calvani M, Bianchini F, et al. Targeting stromal-induced pyruvate kinase M2 nuclear translocation impairs oxphos and prostate cancer metastatic spread. Oncotarget. (2015) 6:24061–74. doi: 10.18632/oncotarget.4448
114. Mendler AN, Hu B, Prinz PU, Kreutz M, Gottfried E, Noessner E. Tumor lactic acidosis suppresses CTL function by inhibition of p38 and JNK/c-Jun activation. Int J Cancer. (2012) 131:633–40. doi: 10.1002/ijc.26410
115. Shime H, Yabu M, Akazawa T, Kodama K, Matsumoto M, Seya T, et al. Tumor-secreted lactic acid promotes IL-23/IL-17 proinflammatory pathway. J Immunol. (2008) 180:7175–7183. doi: 10.4049/jimmunol.180.11.7175
116. Zhang D, Tang Z, Huang H, Zhou G, Cui C, Weng Y, et al. Metabolic regulation of gene expression by histone lactylation. Nature. (2019) 574:575–80. doi: 10.1038/s41586-019-1678-1
117. Sun S, Li H, Chen J, Qian Q. Lactic acid: no longer an inert and end-product of glycolysis. Physiology. (2017) 32:453–63. doi: 10.1152/physiol.00016.2017
118. Liu C, Wu J, Zhu J, Kuei C, Yu J, Shelton J, et al. Lactate inhibits lipolysis in fat cells through activation of an orphan G-protein-coupled receptor, GPR81. J Biol Chem. (2009) 284:2811–22. doi: 10.1074/jbc.M806409200
119. Dienel GA. Brain lactate metabolism: the discoveries and the controversies. J Cereb Blood Flow Metab. (2012) 32:1107–38. doi: 10.1038/jcbfm.2011.175
120. Langin D. Adipose tissue lipolysis revisited (again!): lactate involvement in insulin antilipolytic action. Cell Metab. (2010) 11:242–3. doi: 10.1016/j.cmet.2010.03.003
121. Ristic B, Bhutia YD, Ganapathy V. Cell-surface G-protein-coupled receptors for tumor-associated metabolites: a direct link to mitochondrial dysfunction in cancer. Biochim Biophys Acta. (2017) 1868:246–57. doi: 10.1016/j.bbcan.2017.05.003
122. Roland CL, Arumugam T, Deng D, Liu SH, Philip B, Gomez S, et al. Cell surface lactate receptor GPR81 is crucial for cancer cell survival. Cancer Res. (2014) 74:5301–10. doi: 10.1158/0008-5472.CAN-14-0319
123. Feng J, Yang H, Zhang Y, Wei H, Zhu Z, Zhu B, et al. Tumor cell-derived lactate induces TAZ-dependent upregulation of PD-L1 through GPR81 in human lung cancer cells. Oncogene. (2017) 36:5829–39. doi: 10.1038/onc.2017.188
124. Wagner W, Kania KD, Blauz A, Ciszewski WM. The lactate receptor (HCAR1/GPR81) contributes to doxorubicin chemoresistance via ABCB1 transporter up-regulation in human cervical cancer HeLa cells. J Physiol Pharmacol. (2017) 68:555–64.
125. Hu Y, Zeng F. Expressions of GPR81, MCT1 and MCT4 in squamous carcinoma and their clinical significance. J Central South Univ Med Sci. (2018) 43:950–6. doi: 10.11817/j.issn.1672-7347.2018.09.004
126. Lee YJ, Shin KJ, Park SA, Park KS, Park S, Heo K, et al. G-protein-coupled receptor 81 promotes a malignant phenotype in breast cancer through angiogenic factor secretion. Oncotarget. (2016) 7:70898–911. doi: 10.18632/oncotarget.12286
127. Chen P, Zuo H, Xiong H, Kolar MJ, Chu Q, Saghatelian A, et al. Gpr132 sensing of lactate mediates tumor-macrophage interplay to promote breast cancer metastasis. Proc Natl Acad Sci USA. (2017) 114:580–5. doi: 10.1073/pnas.1614035114
128. Bohn T, Rapp S, Luther N, Klein M, Bruehl TJ, Kojima N, et al. Tumor immunoevasion via acidosis-dependent induction of regulatory tumor-associated macrophages. Nat Immunol. (2018) 19:1319–29. doi: 10.1038/s41590-018-0226-8
129. Walenta S, Voelxen NF, Mueller-Klieser W. Lactate-an integrative mirror of cancer metabolism. Recent Results Cancer Res. (2016) 207:23–37. doi: 10.1007/978-3-319-42118-6_2
130. Kietzmann T. Metabolic zonation of the liver: The oxygen gradient revisited. Redox Biol. (2017) 11:622–30. doi: 10.1016/j.redox.2017.01.012
131. Walenta S, Chau TV, Schroeder T, Lehr HA, Kunz-Schughart LA, Fuerst A, et al. Metabolic classification of human rectal adenocarcinomas: a novel guideline for clinical oncologists? J Cancer Res Clin Oncol. (2003) 129:321–6. doi: 10.1007/s00432-003-0450-x
132. Petrelli F, Cabiddu M, Coinu A, Borgonovo K, Ghilardi M, Lonati V, et al. Prognostic role of lactate dehydrogenase in solid tumors: a systematic review and meta-analysis of 76 studies. Acta Oncol. (2015) 54:961–70. doi: 10.3109/0284186X.2015.1043026
133. Deng T, Zhang J, Meng Y, Zhou Y, Li W. Higher pretreatment lactate dehydrogenase concentration predicts worse overall survival in patients with lung cancer. Medicine. (2018) 97:e12524. doi: 10.1097/MD.0000000000012524
134. Fu Y, Lan T, Cai H, Lu A, Yu W. Meta-analysis of serum lactate dehydrogenase and prognosis for osteosarcoma. Medicine. (2018) 97:e0741. doi: 10.1097/MD.0000000000010741
135. Zhang Y, Xu T, Wang Y, Zhang H, Zhao Y, Yang X, et al. Prognostic role of lactate dehydrogenase expression in urologic cancers: a systematic review and meta-analysis. Oncol Res Treat. (2016) 39:592–604. doi: 10.1159/000449138
136. Kayser G, Kassem A, Sienel W, Schulte-Uentrop L, Mattern D, Aumann K, et al. Lactate-dehydrogenase 5 is overexpressed in non-small cell lung cancer and correlates with the expression of the transketolase-like protein 1. Diagn Pathol. (2010) 5:22. doi: 10.1186/1746-1596-5-22
137. Cui J, Shi M, Xie D, Wei D, Jia Z, Zheng S, et al. FOXM1 promotes the warburg effect and pancreatic cancer progression via transactivation of LDHA expression. Clin Cancer Res. (2014) 20:2595–606. doi: 10.1158/1078-0432.CCR-13-2407
138. Feng Y, Xiong Y, Qiao T, Li X, Jia L, Han Y. Lactate dehydrogenase A: A key player in carcinogenesis and potential target in cancer therapy. Cancer Med. (2018) 7:6124–36. doi: 10.1002/cam4.1820
139. Dong T, Liu Z, Xuan Q, Wang Z, Ma W, Zhang Q. Tumor LDH-A expression and serum LDH status are two metabolic predictors for triple negative breast cancer brain metastasis. Sci Rep. (2017) 7:6069. doi: 10.1038/s41598-017-06378-7
140. Bovenzi CD, Hamilton J, Tassone P, Johnson J, Cognetti DM, Luginbuhl A, et al. Prognostic Indications of Elevated MCT4 and CD147 across cancer types: a meta-analysis. Biomed Res Int. (2015) 2015:242437. doi: 10.1155/2015/242437
141. Miskimins WK, Ahn HJ, Kim JY, Ryu S, Jung YS, Choi JY. Synergistic anti-cancer effect of phenformin and oxamate. PLoS ONE. (2014) 9:e85576. doi: 10.1371/journal.pone.0085576
142. Bushunow P, Reidenberg MM, Wasenko J, Winfield J, Lorenzo B, Lemke S, et al. Gossypol treatment of recurrent adult malignant gliomas. J Neuro-oncol. (1999) 43:79–86. doi: 10.1023/A:1006267902186
143. Yao F, Zhao T, Zhong C, Zhu J, Zhao H. LDHA is necessary for the tumorigenicity of esophageal squamous cell carcinoma. Tumour Biol. (2013) 34:25–31. doi: 10.1007/s13277-012-0506-0
144. Rong Y, Wu W, Ni X, Kuang T, Jin D, Wang D, et al. Lactate dehydrogenase A is overexpressed in pancreatic cancer and promotes the growth of pancreatic cancer cells. Tumour Biol. (2013) 34:1523–30. doi: 10.1007/s13277-013-0679-1
145. Zdralevic M, Vucetic M, Daher B, Marchiq I, Parks SK, Pouyssegur J. Disrupting the “Warburg effect” re-routes cancer cells to OXPHOS offering a vulnerability point via “ferroptosis”-induced cell death. Adv Biol Regul. (2018) 68:55–63. doi: 10.1016/j.jbior.2017.12.002
146. Halford SER, Jones P, Wedge S, Hirschberg S, Katugampola S, Veal G, et al. A first-in-human first-in-class (FIC) trial of the monocarboxylate transporter 1 (MCT1) inhibitor AZD3965 in patients with advanced solid tumours. J Clin Oncol. (2017) 35:2516. doi: 10.1200/JCO.2017.35.15_suppl.2516
147. Van Hee VF, Labar D, Dehon G, Grasso D, Gregoire V, Muccioli GG, et al. Radiosynthesis and validation of (+/-)-[18F]-3-fluoro-2-hydroxypropionate ([18F]-FLac) as a PET tracer of lactate to monitor MCT1-dependent lactate uptake in tumors. Oncotarget. (2017) 8:24415–28. doi: 10.18632/oncotarget.14705
Keywords: lactate, warburg effect, monocarboxylate transporters, GPR81, metabolic fuel, lactate shuttles, signaling molecule
Citation: Baltazar F, Afonso J, Costa M and Granja S (2020) Lactate Beyond a Waste Metabolite: Metabolic Affairs and Signaling in Malignancy. Front. Oncol. 10:231. doi: 10.3389/fonc.2020.00231
Received: 15 December 2019; Accepted: 11 February 2020;
Published: 18 March 2020.
Edited by:
Paolo E. Porporato, University of Turin, ItalyReviewed by:
Jacques Pouyssegur, Université Côte d'Azur, FranceFranklin David Rumjanek, Federal University of Rio de Janeiro, Brazil
Copyright © 2020 Baltazar, Afonso, Costa and Granja. This is an open-access article distributed under the terms of the Creative Commons Attribution License (CC BY). The use, distribution or reproduction in other forums is permitted, provided the original author(s) and the copyright owner(s) are credited and that the original publication in this journal is cited, in accordance with accepted academic practice. No use, distribution or reproduction is permitted which does not comply with these terms.
*Correspondence: Fátima Baltazar, ZmJhbHRhemFyJiN4MDAwNDA7bWVkLnVtaW5oby5wdA==
†These authors have contributed equally to this work