- 1Institute-Clinic of Pediatrics, Department of Physiology, University of Debrecen, Debrecen, Hungary
- 2Department of Physiology, University of Debrecen, Debrecen, Hungary
Sirtuins compose a unique collection of histone deacetylase enzymes that have a wide variety of enzymatic activities and regulate diverse cell functions such as cellular metabolism, longevity and energy homeostasis, mitochondrial function, and biogenesis. Impaired sirtuin functions or alterations of their expression levels may result in several pathological conditions and contribute to the altered metabolic phenotype of malignantly transformed cells in a significant manner. In the twenty-first century, principles of personalized anticancer treatment need to involve not only the evaluation of changes of the genetic material, but also the mapping of epigenetic and metabolic alterations, to both of which the contribution of sirtuin enzymes is fundamental. Since sirtuins are central players in the maintenance of cellular energy and metabolic homeostasis, they are key elements in the development of metabolic transformation of cancer cells referred to as the Warburg effect. Although its most well-known features are enhanced glycolysis and excessive lactate production, Warburg effect has several aspects involving both carbohydrate, lipid, and amino acid metabolism, among which different tumor types have different preferences. Therefore, energy supply of cancer cells can be impaired by a growing number of antimetabolite agents, for which appropriate vectors are strongly needed. However, data are controversial about their tumor suppressor or oncogenic properties, the biological effects of sirtuin enzymes strongly depend on the tissue microenvironment (TME) in which they are expressed. Immune cells are regarded as key players of TME. Sirtuins regulate the survival, activation, metabolism, and mitochondrial function of these cells, therefore, they are not only single elements, but key regulators of the network that determines anticancer immunity. Altered metabolism of tumor cells induces changes in the gene expression pattern of cells in TME, due to altered concentrations of metabolite cofactors of epigenetic modifiers including sirtuins. In summary, epigenetic and metabolic alterations in malignant diseases are influenced by sirtuins in a significant manner, and should be treated in a personalized approach. Since they often develop in early stages of cancer, broad examination of these alterations is required at time of the diagnosis in order to provide a personalized combination of distinct therapeutic agents.
Introduction
First definition of epigenetics is derived from Conrad Hal Waddington (1942), who proposed it as “the causal interactions between genes and their products, which bring the phenotype into being” (1). Today, the term epigenetics involves all mechanisms that modify gene expression pattern without altering the sequence of the DNA (2). Key elements of epigenetic regulation are DNA methylation, histone modification, non-coding RNAs, and nucleosome remodeling. Epigenetic mechanisms are reversible and heritable (3). These features offer a great opportunity and at the same time, make high demands on personalized medicine.
First epigenetic alteration to be linked to cancer was global DNA hypomethylation described by Feinberg and Vogelstein in 1983 (3). To date, it has been confirmed that besides alterations in the DNA methylation pattern, changes in the histone code and in the expression levels of non-coding RNAs also contribute to the pathogenesis of malignant diseases in a significant manner.
Among the several hallmarks that cancer cells acquire during tumorigenesis (4) altered metabolism is also a unique feature (5). The best-known characteristics of this special metabolic phenotype are increased rates of glycolysis and lactate production, which will be further detailed. Due to numerous interactions, epigenetic and metabolic alterations cannot be considered as independent players in the big puzzle of pathogenesis. The vast majority of enzymes that are responsible for catalyzing epigenetic alterations require metabolite cofactors [Figure 1; (6)]. Enzymes of the intermedier metabolism are also regulated by epigenetic alterations, for which an elegant example is the impact of histone acetyltransferase enzymes (HAT) on the activity of enzymes involved in glycolysis and fatty acid metabolism (7).
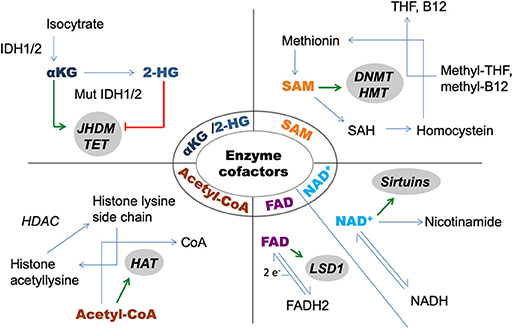
Figure 1. Metabolite cofactors of epigenetic modifier enzymes. 2-HG, 2-hydroxyglutarate; αKG, alpha-ketoglutarate; DNMT, DNA-methyltransferase; HAT, histone acetyltransferase; HDAC, histone deacetylase; HMT, histone methyltransferase; IDH, isocitrate-dehydrogenase; JHDM, Jumonji domain containing histone demethylase; LSD, lysinspecific demethylase; SAH, S-adenosyl homosysteine; SAM, S-adenosylmethionine; TET, DNA-hidroxymethylase enzyme; THF, tetrahydrofolate.
In recent years, numerous crosslinks have been established between epigenetics and metabolism in cancer, among which sirtuin enzymes have key significance.
Sirtuins and Cancer
The currently known 18 histone deacetylase enzymes (HDACs) are divided into four groups, among which sirtuin enzymes, that are homologs to the yeast SIR2 protein (Silent Information Regulator 2), comprise group III, requiring NAD+ as a cofactor. They are a seven member family of protein deacylases and ADP-ribosyl-transferases with different targets, enzymatic activities, subcellular localizations, and regulatory mechanisms [Table 1; (8, 9)]. Since they are major hubs in the regulatory network of energy homeostasis and metabolism, sirtuins are potential therapeutic targets both in oncology and in the field of inborn errors of metabolism as well.
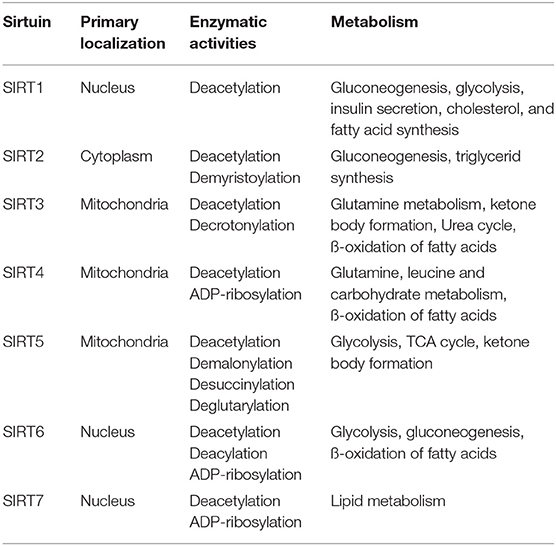
Table 1. Localization, enzymatic activity, and role of sirtuin enzymes in the regulation of metabolic pathways.
In cancer, sirtuins have both oncogenic and tumor suppressor properties, however, data are controversial at several points. As an example for emphasizing the importance of tissue microenvironment, SIRT1 has been proposed both as a tumor suppressor and as an oncogene in different types of malignancies (8). SIRT4 acts as a tumor suppressor by regulating cell metabolism and inflammation as well (10). Oncogenic and tumor suppressor effects of sirtuins are also determined by the targets that they regulate. For example, SIRT7 was identified as a suppressor of MYC function, however, SIRT7 is essential in maintaining low levels of H3K18ac in cancer cells that is associated with poor clinical outcome (11, 12).
Altered expression levels of sirtuins contribute to chemoresistance and metastasis formation, and in some cases, they are associated with clinical outcome. In endometria carcinoma cell lines, SIRT1 overexpression enhanced resistance to paclitaxel and cisplatin (13). SIRT1 activation by MYC promotes resistance of FLT3-ITD-mutated acute myeloid leukemia stem cells to tyrosine kinase inhibitors (14). SIRT4 enzyme enhances the sensitivity of breast cancer cells to tamoxifen (15). SIRT4 also inhibits the migration and metastasis formation of thyroid cancer cells (16). SIRT6 promotes papillary thyroid cancer progression by inducing epithelial-mesenchymal transition (EMT) (17). In non-small cell lung cancer (NSCLC), expression of SIRT1 and SIRT2 is associated with poor prognosis (18).
Sirtuins are also involved in the pathogenesis of hematological malignancies. SIRT1 is overexpressed in human leukemia stem cells (LSC), and its inhibition suppressed proliferation of primitive progenitor cells and increased apoptosis in LSC (19). Due to our previous results, the expression level of SIRT6 enzyme negatively correlates with the level of the tumor suppressor miR-124 in acute myeloid leukemia (AML) (20).
The central role of sirtuin enzymes in the metabolism of cancer cells is confirmed by a growing number of evidences about their role in both promoting and inhibiting the Warburg effect (see below) in several tumor types. This strong impact on metabolism is highly associated with the numerous interactions of sirtuins with oncogenic and tumor suppressor proteins, microRNAs that regulate metabolism, and proteins involved in signal transduction pathways as well.
The Warburg Effect
Nobel Laureate Otto Warburg observed in the 1920s that malignantly transformed cells prefer lactate production over oxidative phosphorylation regardless of the level of oxygen (21). The discovery of elevated glycolytic rate in tumor cells is still the basis of the worldwide used diagnostic method 18FDG PET (6).
Enhanced glycolysis is the most widely known feature of the altered metabolic phenotype of cancer cells. Glycolytic rate can be up to 200 times higher in malignantly transformed cells compared to healthy cells, to which up-regulation of GLUT transporters and overexpression of glycolytic enzymes also contribute (22, 23). MYC and HIF1α are both essential transcription factors in regulating the expression levels of enzymes involved in glycolysis, however, it is an important difference that while MYC enhances, HIF1α represses mitochondrial biogenesis (24, 25). Recently, many sirtuins have been proved to affect the activity of HIF1α: SIRT1 inhibits its transcriptional activity by deacetylation, SIRT2 increases its stability, while SIRT3 and SIRT7 destabilize it (26). The inhibition of HIF1α enzyme is a promising therapeutic target in several tumor types. Bortezomib, which was approved for the treatment of multiple myeloma in 2008, has also been proved to inhibit the transcriptional activity of HIF1α (27).
Though high rate of glycolysis results in excessive lactate production, systemic effects of lactate byproducts are rarely significant. However, type B lactate acidosis that develops under normoxic conditions is a rare, but life-threatening complication of hematological malignancies (28). Recent findings on a novel type of in vivo post-translational histone modifications, lysine lactylation, highlights the impact of elevated lactate production on gene expression (29). Histone lysine lactylation can directly promote the transcription of certain genes, however, its involvement in the metabolic switch of cancer cells has yet to be clarified (29).
Tumor suppressor proteins are also involved in the regulation of glycolysis. Excessive glycolysis and lactate production is counteracted by p53, which activates TIGAR enzyme and inhibits phosphoglycerate mutase 2 (PGM2). TIGAR is responsible for the cleavage of fructose-2,6-bisphosphate, the allosteric activator of phosphofructokinase I (PFK I) (23).
Opposite to oxidative phosphorylation that produces 36 molecules of ATP from one molecule glucose, glycolysis yields only 2. Despite this inefficient ATP generation, up-regulation of glycolysis provides cancer cells a number of advantages. Cancer cells accumulate several intermediary metabolites that may be shunted to interconnected pathways to support the biosynthesis of essential macromolecules and rapid proliferation (11, 30). One possible direction is the pentose phosphate pathway, while glyceraldehyde-3-phosphate, 3-phosphoglycerate, and fructose-6-phosphate are critical for the de novo synthesis of amino acids, phospholipids, and ribonucleotides, respectively (25, 31).
Increased glutamine demand and consumption are also important characteristics of the Warburg effect. Among transcription factors, MYC has been proven to induce glutamine transporters as well (25). Cancer cells utilize glutamine to fuel biosynthesis of nucleotides, to reload tricarboxylic acid cycle (TCA) intermediates, or to convert glutamine into lactate by the stepwise process of glutaminolysis (32). Besides biosynthesis, glutamine is also utilized for antioxidant defense function, since glutamine metabolism results in the concomitant production of NADPH, decreasing concentration of reactive oxygen species (ROS) and increasing glutathione levels, thereby protecting cells from oxidative stress (25, 33).
In malignantly transformed cells some tumor specific isoforms of metabolic enzymes also have been described. Opposite to normal cells that express pyruvate kinase M1 isoform (PKM1), tumor cells primarily express the M2 isoform that is responsible for the regulation of the final and rate-limiting reaction of the glycolytic pathway (34). PKM2 acts as a coactivator of HIF1α, inducing the expression of pyruvate dehydrogenase kinase (PDK) and lactate dehydrogenase (LDH) (34). Hexokinase II (HK II) is another example of tumor specific enzyme isoforms promoted by Akt protein (23).
Besides the tumor specific isoforms, the mutations of some metabolic enzymes also contribute to the metabolic switch featuring cancer cells. Gain of function mutations of isocitrate dehydrogenase (IDH) result in novel enzymatic activity and the production of the oncometabolite 2-hydroxyglutarate (2-HG) (35). Mutations of succinate dehydrogenase (SDH) and fumarate hydratase (FH) lead to the accumulation of succinate and fumarate, respectively, which inhibit α-ketoglutarate dependent enzymes including DNA-hydroxymethylating TET enzymes and Jumonji C domain containing histone demethylases (36). These mutations also contribute to the stabilization of HIF1α, due to the inhibition of α-ketoglutarate-dependent prolyl hydroxylase enzyme (37).
Cancer stem cells, the maintenance and self-renewal properties of which are tightly regulated by sirtuin enzymes, demonstrate unique metabolic flexibility. In general, they are characterized by even higher glycolytic rate (38), however, they can switch between glycolysis and oxidative phosphorylation in the presence of oxygen to maintain cellular homeostasis and promote tumor growth as well (39).
Epigenetic Background of Warburg Effect With Emphasis on Sirtuin Enzymes
Development of Warburg-like metabolic phenotype of cancer cells is strongly regulated by epigenetic mechanisms where sirtuin enzymes play a key role. Various enzymatic activities, subcellular localizations and an enormous interactome with metabolic enzymes, epigenetic modifiers, and proteins of signal transduction pathways enable sirtuins to be central players in this field. In this section, we summarize data about the involvement of distinct sirtuins in the regulation of metabolism of cancer cells.
SIRT1 is a key metabolic sensor and regulator of mitochondrial biogenesis as well. SIRT1 and AMP-activated protein kinase (AMPK) directly activate the nuclear receptor Peroxisome Proliferator Activated Receptor Gamma Coactivator 1 alpha (PGC1α) through deacetylation and phosphorylation, respectively, resulting in increased mitochondrial biogenesis (40). In glioblastoma multiforme, activation of PGC1α leads to the differentiation of cells into a mature phenotype by activating some transcription factors related to mitochondrial biogenesis, therefore counteracting the Warburg effect (41).
In acute myeloid leukemia (AML) cell models, SIRT2 promoted the Warburg effect by deacetylating and activating glucose-6-phosphate dehydrogenase (G6PD) enzyme, increasing the production of NADPH, and supporting the biosynthesis of macromolecules that are essential for rapid cell proliferation (42). However, SIRT2 also deacetylates and destabilizes the ATP-citrate lyase (ACLY) enzyme (43), the low expression level of which is associated with favorable overall survival in AML patients (44). In cholangiocarcinoma, SIRT2 induces Warburg-like metabolic reprogramming resulting in decreased oxidative phosphorylation and increased activity of the serine synthesis pathway, consequently protecting cholangiocarcinoma cells from oxidative stress and apoptosis (45).
SIRT3 is the best characterized mitochondrial sirtuin enzyme, its expression level is increased by caloric restriction, fasting, and exercise training (9). The first reported target of SIRT3 was acetyl-CoA synthetase 2 (AceCS2) (46), that promotes metastasis formation of renal cell carcinoma (47), however, in gastric cancer, loss of AceCS2 expression predicts poor prognosis (48), which further emphasizes the significance of TME. SIRT3 down-regulates HIF1α and pyruvate dehydrogenase kinase 1 (PDK1) in cholangiocarcinoma (49), and suppresses the Warburg effect also by the activation of pyruvate dehydrogenase complex (PDC) and the indirect inhibition of the tumor specific isoenzyme hexokinase II (HK II) (11). SIRT3 regulates fatty acid oxidation via the deacetylation and activation of long chain acyl-CoA dehydrogenase (LCAD) enzyme (50), opposite to HIF1α that suppresses fatty acid oxidation to facilitate cancer progression (51). In cancer cells, similarly to the reduction of ATP production, the decrease of mitochondrial ROS formation is also significant, in which SIRT3 has been proved to play a pivotal role by the deacetylation and activation of superoxide dismutase 2 (SOD2) enzyme (52). Though SIRT3 promotes mitochondrial biogenesis (53), it also protects mitochondrial DNA from oxidative damage because of the deacetylation of 8-oxoguanine-DNA glycosylase 1 (OGG1), an enzyme that is involved in the process of DNA repair (54). Despite the fact that SIRT3 counteracts the Warburg effect in several ways, Warburg-promoting effect of this enzyme has also been confirmed. SIRT3 deacetylates and activates mitochondrial glutamate dehydrogenase (GDH) (55), therefore contributing to enhanced glutaminolysis, that is characteristic of Warburg effect. The rate-limiting enzyme of ketone body formation, 3-hydroxy-3-methylglutaryl-CoA synthase 2 (HMGCS2) is also regulated by SIRT3 (56). HMGCS2 is a tumor suppressor in prostate cancer, the knockdown of which promotes cell proliferation, colony formation, migration, and invasion of prostate cancer cells (57). SIRT3 was also found to deacetylate the serine hydroxymethyltransferase 2 (SHMT2) enzyme. Deacetylated SHMT2 is less stable, and indirectly counteracts the proliferation of colon cancer cells (58).
SIRT4 also has an impact on metabolism of cancer cells by promoting the Warburg effect. Besides the inhibition of glutamate dehydrogenase (GDH) enzyme (59), SIRT4 was described to inhibit malonyl-CoA decarboxylase, resulting in the repression of fatty acid oxidation (54). SIRT4 is also involved in the regulation of carbohydrate metabolism: according to novel findings, it increases the activity of PDC by repressing the expression of PDK1 enzyme (60). SIRT4 has also been described to regulate the leucine oxidation pathway (61). In a rat model, leucine-rich diet resulted in less glycolytic phenotype and decreased tumor aggressiveness (62).
SIRT5 regulates metabolic enzymes by deacetlyation, desuccinylation, deglutarylation, and demalonylation as well. Carbamoyl phosphate synthetase 1 (CPS1) is the only known protein that is deacetylated by SIRT5 (54). Elevated expression level of CPS1 would be supported by acetylation, and was associated with poor overall survival in LKB1-inactivated lung adenocarcinoma (63). SIRT5 desuccinylates and activates superoxide dismutase 1 (SOD1) (9), the oncogene serine hydroxymethyltransferase (SHMT2) (64), and pyruvate kinase M2 (PKM2) at two residues, K311 and K498 (8). Desuccinylation of PKM2 at K498 was shown to promote tumor development (8), while the role of K311 desuccinylation in cancer has not been described yet (65). In hepatocellular carcinoma, SIRT5 was shown to keep oxidative damage below toxic levels by desuccinylating and inhibiting peroxisomal acyl-CoA oxidase 1 (ACOX1) enzyme (66). In colorectal carcinoma cell lines, SIRT5 deglutarylates and activates glutamate dehydrogenase (GDH) enzyme, contributing to the Warburg effect (67). SIRT5 demalonylates and inactivates succinate dehydrogenase (SDH) enzyme leading to succinate accumulation, that results in the inhibition of α-ketoglutarate dependent dioxygenases as mentioned before (68).
SIRT6, which is one of the major epigenetic regulator of the glucose homeostasis of cells, exerts anti-Warburg effect by the inhibition of increased glucose uptake and overexpression of glycolytic enzymes as well. The latter results from the deacetylase activity of the enzyme, because H3K9 and H3K56 deacetylation of glycolytic genes inhibits their transcription by HIF1α and MYC, respectively (11). SIRT6 also inhibits hepatic gluconeogenesis by promoting the deacetylation of PGC1α transcription factor (69). Among tumor specific enzyme isoforms, SIRT6 deacetylates PKM2 at K433, leading to its nuclear export and the inhibition of PKM2 oncogenic functions (70).
SIRT7 has recently been described as an enzyme with ADP-ribosyl transferase activity (71). SIRT7 controls mitochondrial biogenesis, increases hepatic lipid accumulation and enhances adipogenesis in white adipocytes (72), however, its role in Warburg effect has yet to be examined.
Besides sirtuins, microRNAs are also important epigenetic regulators of the metabolic switch characteristic to cancer cells. MicroRNAs can either promote or inhibit the Warburg effect, depending on the target metabolic enzymes. MiR-26a promotes the Warburg effect by targeting pyruvate dehydrogenase X component (PDHX) in colorectal cancer cells, which inhibits the conversion of pyruvate to acetyl-CoA in the tricarboxylic acid cycle (TCA) (73). Both tumor suppressor and oncogenic microRNAs are involved in the regulation of tumor specific isoforms of some metabolic enzymes. Oncogenic miR-155 promotes the expression of hexokinase II, while tumor suppressor miR-124 inhibits the expression of pyruvate kinase M2 isoform (74). In non-small cell lung cancer cells, down-regulation of miR-214 inhibits glycolysis and proliferation, resulting from the decreased expression levels of hexokinase II and PKM2 enzymes (75). MiR-378* contributes to the down-regulation of enzymes involved in TCA (22).
MicroRNAs and sirtuins cannot be regarded as independent regulators of the metabolism of cancer cells, since numerous interactions have been revealed between them. For example, miR-31 targets SIRT3 enzyme to increase oxidative stress in oral carcinoma (76).
Anticancer Immunity and Its Connection With Altered Metabolic Phenotype: Role of Sirtuin Enzymes
It has been clear for more than a half century that one of the most important functions of the immune system is to identify and eliminate transformed cell clones (Macfarlane Burnet−1950) (77). On the other hand, tumors dampen antitumor immunity by several mechanisms, which is also a hallmark of cancer (25). Besides widespread crosstalk between immune cells and transformed clones, this hallmark involves a strong connection with metabolism as well: “if T cells play the music during an adaptive immune response, the metabolic tumor microenvironment calls the tune” (25, 78).
Cancer cells have been proved to induce decreased levels of nutrients and a hypoxic, acidic condition in the tumor microenvironment (25). Therefore, metabolic reprogramming is required by both tumor cells and immune cells in order to adapt to this microenvironment (78). However, adaptation results in the preference of different metabolic pathways in case of distinct cell types, in which sirtuin enzymes play an important role (Table 2).
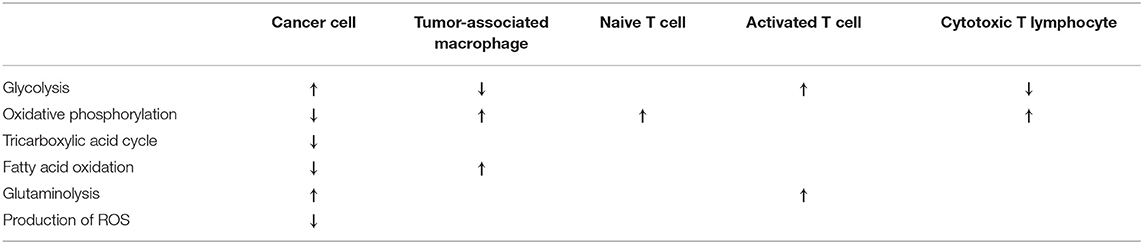
Table 2. Characteristic metabolic phenotypes of cancer cells and immune cells of tissue microenvironment.
T cells are the major components for antitumor immunity (78), however, a complex immunosuppressive network in cancer leads to the abrogation of immune and metabolic checkpoints, resulting in the limited activation and dysfunction of T cells (25). Similarly to cancer cells, T cells also undergo a metabolic switch upon their activation. Naive T cells rely mostly on oxidative phosphorylation and require relatively small amounts of glucose to maintain basic energetic demands (25). Though activated T cells engage in increased rates of glycolysis and glutaminolysis, it is important to note that opposite to transformed cells, this is the part of a physiological adaptation process in case of T cells (25). Metabolic intermediates generated by this metabolic switch are important for cytotoxicity and cytokine production as well (25). In recent years, sirtuins have been confirmed to regulate the differentiation and function of T cells in TME. SIRT1 negatively regulates the differentiation of IL-9-producing antitumor Th 9 cells in cancer (79) and suppresses the activity of regulatory T cells (80). On the contrary, SIRT3 has been proved to maintain immunosuppressive activity of regulatory T cells (80).
M2 polarized tumor-associated macrophages (TAMs) support tumor growth and provide a barrier against the natural killer (NK) cells and cytotoxic T lymphocytes (81). They exhibit up-regulated fatty acid synthesis and ß-oxidation (78), decreased glycolysis and the utilization of oxidative metabolism (82). TAMs are also characterized by high expression of IL-10, while they produce low levels of IL-12, tumor necrosis factor-α (TNFα) and inducible nitric oxide synthase (iNOS) (10). In hepatocellular carcinoma (HCC), SIRT1 was found to inhibit metastasis formation by promoting M1 macrophage polarization (83). Also in HCC, down-regulation of SIRT4 induces elevated monocyte chemoattractant protein-1 (MCP-1) expression, resulting in increased TAM infiltration of peritumor tissues (10).
Similarly to M2 polarized TAMs, cytotoxic T lymphocytes are also featured by a decreased rate of glycolysis and enhanced oxidative phosphorylation (78).
Central role of mitochondria in the maintenance of metabolic and redox homeostasis also has a strong impact on antitumor immunity. Mitochondrial oxidative metabolism was described as a critical suppressor of metastasis (84), while high levels of reactive oxygen species (ROS) in TME were confirmed to down-regulate the activity of antitumor effector T cells (85). Complexes of the electron transfer chain are regulated by the major mitochondrial deacetylase SIRT3, knockdown or deficiency of which correlates with decreased complex activity (86). In glioma stem cells, cooperative interplay between the mitochondrial chaperone TRAP1 and SIRT3 increases mitochondrial respiratory capacity and reduces the production of ROS (87).
Activation of the transcription factor aryl hydrocarbon receptor (AHR) induces the differentiation of CD4+ naive T cells into immunosuppressive regulatory T cells (88, 89). Besides playing critical roles in the initiation, promotion, progression, and metastasis of cancer (78), AHR is involved in the regulation of two NADases, contributing to decreased SIRT1 activity and the deregulation of glucose and fatty acid homeostasis as well (90). However, to our knowledge, interaction between AHR and sirtuin enzymes in cancer has not been established yet. There is also evidence that metabolites such as succinate and NAD+ are signals that regulate innate immunity, by acting via deacetylases such as SIRT1 and SIRT2 and regulating HIF1α, respectively (82).
Special Aspects of Novel Epigenetic and Metabolic Therapeutic Approaches
In the twenty-first century, principles of personalized anticancer treatment need to involve the mapping of epigenetic and metabolic alterations, to both of which the contribution of sirtuin enzymes is fundamental. In parallel with this aim, growing number of antimetabolites and sirtuin inhibitors are administered. As an example for the latter, inhibition of SIRT1 by the up-regulation of miR-211-5p was associated with the induction of apoptosis in breast cancer cells (91). Inhibition of SIRT2 has been confirmed to induce the susceptibility of melanoma cell to the multikinase inhibitor dasatinib (92). Inactivation of SIRT3 leads to metabolic alterations, loss of stemness, and suppression of tumor formation by glioma stem cells in vivo (87).
Manipulating metabolism is also a tool to enhance antitumor immunity, since metabolic pathways have been proved to shape both function and survival of antitumor T cells (25). However, different metabolic interventions may be required in transformed clones and in distinct cell types of the immune system. For example, while inhibition of mevalonate metabolism in tumor cells attenuates the proliferation and growth of innate immune cells, the mevalonate pathway contributes to trained immunity as well (78).
It should also be considered, that inhibitors of sirtuin enzymes can induce different changes in distinct cell types of anticancer immunity. In myeloid cells, SIRT1 inhibition leads to increased transcription of proinflammatory cytokines, but on the other hand, targeting SIRT1 results in net immunosuppressive effect in case of T cells (93).
Concluding Remarks and Future Perspectives
Sirtuins are key hubs in the regulatory network of etiological factors of tumors, having strong impact on the growth, survival, and metabolism of cancer cells, that also influences antitumor immunity in a significant manner. One of the major challenges of modern oncology is to establish novel elements of this regulatory-etiological network in order to provide personalized treatment for patients. Investigation of the exciting world of sirtuin enzymes is definitely one of the most effective tools for this. Further examinations are required to elucidate cell-specific metabolic and immunological effects of sirtuin inhibitors and activators. Appropriate vectors are also needed to deliver these small molecules and antimetabolites to their definitive target cells. Together with widespread genetic, epigenetic, and metabolic mapping at the time of the diagnosis, these efforts could improve therapeutic results, leading to longer disease-free and overall survival, with improved life quality as well.
Author Contributions
Text of the manuscript was written by ZG with the supervision and instructions of LC.
Funding
This work was supported by the GINOP-2.3.2-15-2016-00044 and NKFIH-1150-6/2019 grants of the National Research, Development and Innovation Office (Hungary) to the University of Debrecen. This project was cofinanced by the European Union and the European Regional Development Fund.
Conflict of Interest
The authors declare that the research was conducted in the absence of any commercial or financial relationships that could be construed as a potential conflict of interest.
Abbreviations
2-HG, 2-hydroxyglutarate; AceCS2, Acetyl-CoA synthetase 2; ACOX1, acyl-CoA oxidase 1; AHR, aryl hydrocarbon receptor; AML, acute myeloid leukemia; AMPK, AMP activated protein kinase; CPS1, carbamoyl phosphate synthetase 1; EMT, epithelial-mesenchymal transition; FDG, fluorodeoxyglucose; FH, fumarate hidratase; FLT3, fms-like tyrosine kinase 3; G6PD, glucose-6-phoshate dehydrogenase; GDH, glutamate dehydrogenase; HAT, histone acetyltransferase; HCC, hepatocellular carcinoma; HDAC, histone deacetylase; HK, hexokinase; HMGCS2, 3-hydroxy-3-methylglutaryl CoA synthase 2; iNOS, inducible nitric oxide synthase; ITD, internal tandem duplication; LCAD, long chain acyl CoA dehydrogenase; LDH, lactate dehydrogenase; LKB1, liver kinase B1; LSC, leukemia stem cell; MCAD, medium chain acyl CoA dehydrogenase; MCP-1, monocyte chemoattractant protein-1; NSCLC, non-small cell lung cancer; NK, natural killer; OGG1, 8-oxoguanine-DNA glycosylase 1; PDC, pyruvate dehydrogenase complex; PDHX, pyruvate dehydrogenase X component; PDK, pyruvate dehydrogenase kinase; PET, positron emission tomography; PGM, phosphoglycerate mutase; PFK, phosphofructokinase; PGC1α, Peroxisome proliferator-activated receptor gamma coactivator 1-alpha; PKM2, M2 isoform of pyruvate kinase; RORγ, RAR-related orphan receptor gamma; ROS, reactive oxygen species; SDH, succinate dehydrogenase; SHMT, serine hydroxymethyltransferase; SIR2, Silent Information Regulator 2; SOD, superoxide dismutase; STAT3, signal transducer and activator of transcription 3; TAM, tumor associated macrophage; TCA, tricarboxylic acid cycle; TIGAR, tumor protein 53-induced glycolysis and apoptosis regulator; TME, tissue microenvironment; TNFα, tumor necrosis factor-α; TRAP1, tumor necrosis factor receptor associated protein 1.
References
2. Bollati V, Baccarelli A. Environmental Epigenetics. Heredity. (2010) 105:105–12. doi: 10.1038/hdy.2010.2
3. Feinberg AP, Vogelstein B. Hypomethylation distinguishes genes of some human cancers from their normal counterparts. Nature. (1983) 301:89–92. doi: 10.1038/301089a0
4. Hanahan D, Weinberg RA. The hallmarks of cancer. Cell. (2000) 100:57–70. doi: 10.1016/S0092-8674(00)81683-9
5. Sebastián C, Zwaans BM, Silberman DM, Gymrek M, Goren A, Zhong L, et al. The histone deacetylase SIRT6 is a tumor suppressor that controls cancer metabolism. Cell. (2012) 151:1185–99. doi: 10.1016/j.cell.2012.10.047
6. Yun J, Johnson JL, Hanigan CL, Locasale JW. Interactions between epigenetics and metabolism in cancers. Front Oncol. (2012) 2:163. doi: 10.3389/fonc.2012.00163
7. Zhao S, Xu W, Jiang W, Yu W, Lin Y, Zhang T, et al. Regulation of cellular metabolism by protein lysine acetylation. Science. (2010) 327:1000–4. doi: 10.1126/science.1179689
8. Costa-Machado LF, Fernandez-Marcos PJ. The sirtuin family in cancer. Cell Cycle. (2019) 18:2164–96. doi: 10.1080/15384101.2019.1634953
9. Osborne B, Bentley NL, Montgomery MK, Turner N. The role of mitochondrial sirtuins in health and disease. Free Radic Biol Med. (2016) 100:164–74. doi: 10.1016/j.freeradbiomed.2016.04.197
10. Li Z, Li H, Zhao ZB, Zhu W, Feng PP, Zhu XW, et al. SIRT4 silencing in tumor-associated macrophages promotes HCC development via PPARδ signalling-mediated alternative activation of macrophages. J Exp Clin Cancer Res. (2019) 38:469. doi: 10.1186/s13046-019-1456-9
11. Zwaans BM, Lombard DB. Interplay between sirtuins, MYC and hypoxia-inducible factor in cancer-associated metabolic reprogramming. Dis Model Mech. (2014) 7:1023–32. doi: 10.1242/dmm.016287
12. Barber MF, Michishita-Kioi E, Xi Y, Tasselli L, Kioi M, Moqtaderi Z, et al. SIRT7 links H3K18 deacetylation to maintenance of oncogenic transformation. Nature. (2012) 487:114–8. doi: 10.1038/nature11043
13. Asaka R, Miyamoto T. Sirtuin 1 promotes the growth and cisplatin resistance of endometrial carcinoma cells: a novel therapeutic target. Lab. Invest. (2015) 95:1363–73. doi: 10.1038/labinvest.2015.119
14. Li L, Osdal T, Ho Y, Chun S, McDonald T, Agarwal P, et al. SIRT1 activation by a c-MYC oncogenic network promotes the maintenance and drug resistance of human FLT3-ITD acute myeloid leukemia stem cells. Cell Stem Cell. (2014) 15:431–46. doi: 10.1016/j.stem.2014.08.001
15. Xing J, Li J, Fu L, Gai J, Guan J, Li Q. SIRT4 enhances the sensitivity of ER-positive breast cancer to tamoxifen by inhibiting the IL-6/STAT3 signal pathway. Cancer Med. (2019) 8:7086–97. doi: 10.1002/cam4.2557
16. Chen Z, Lin J, Feng S, Chen X, Huang H, Wang C, et al. SIRT4 inhibits the proliferation, migration, and invasion abilities of thyroid cancer cells by inhibiting glutamine metabolism. Onco Targets Ther. (2019) 12:2397–408. doi: 10.2147/OTT.S189536
17. Yang Z, Yu W, Huang R, Ye M, Min Z. SIRT6/HIF-1α axis promotes papillary thyroid cancer progression by inducing epithelial-mesenchymal transition. Cancer Cell Int. (2019) 19:17. doi: 10.1186/s12935-019-0730-4
18. Grbesa I, Pajares MJ, Martínez-Terroba E, Agorreta J, Mikecin AM, Larráyoz M, et al. Expression of sirtuin 1 and 2 is associated with poor prognosis in non-small cell lung cancer patients. PLoS ONE. (2015) 10:e0124670. doi: 10.1371/journal.pone.0124670
19. Dong Z, Cui H. Function of sirtuins in cancer stem cells. Int J Stem Cell Res Ther. (2016) 3:024. doi: 10.23937/2469-570X/1410024
20. Gaál Z, Oláh É, Rejto L, Bálint BL, Csernoch L. Expression levels of warburg-effect related microRNAs correlate with each other and that of histone deacetylase enzymes in adult hematological malignancies with emphasis on acute myeloid leukemia. Pathol Oncol Res. (2017) 23:207–16. doi: 10.1007/s12253-016-0151-9
21. Warburg O, Minami S. Versuche an Überlebendem Carcinomgewebe. Klin Wochenschr. (1923) 2:776–7. doi: 10.1007/BF01712130
22. Gao P, Sun L, He X, Cao Y, Zhang H. MicroRNAs and the Warburg effect: new players in an old arena. Curr Gene Ther. (2012) 12:285–91. doi: 10.2174/156652312802083620
23. Koppenol WH, Bounds PL, Dang CV. Otto Warburg's contributions to current concepts of cancer metabolism. Nat Rev Cancer. (2011) 11:325–37. doi: 10.1038/nrc3038
24. Zhong L, Mostoslavsky R. SIRT6: a master epigenetic gatekeeper of glucose metabolism. Transcription. (2010) 1:17–21. doi: 10.4161/trns.1.1.12143
25. Kouidhi S, Elgaaied AB, Chouaib S. Impact of metabolism on T-cell differentiation and function and cross talk with tumor microenvironment. Front Immunol. (2017) 8:270. doi: 10.3389/fimmu.2017.00270
26. Zhu S, Dong Z, Ke X, Hou J, Zhao E, Zhang K, et al. The role of sirtuins family in cell metabolism during tumor development. Semin Cancer Biol. (2019) 57:59–71. doi: 10.1016/j.semcancer.2018.11.003
27. Kappler M, Pabst U, Weinholdt C, Taubert H, Rot S, Kaune T, et al. Causes and consequences of a glutamine induced normoxic HIF1 activity for the tumor metabolism. Int J Mol Sci. (2019) 20:E4742 doi: 10.3390/ijms20194742
28. Claudino WM, Dias A, Tse W, Sharma VR. Type B lactic acidosis: a rare but life threatening hematologic emergency. A case illustration and brief review. Am J Blood Res. (2015) 5:25–9.
29. Zhang D, Tang Z, Huang H, Zhou G, Cui C, Weng Y, et al. Metabolic regulation of gene expression by histone lactylation. Nature. (2019) 574:575–580. doi: 10.1038/s41586-019-1678-1
30. Vander Heiden MG, Cantley LC, Thompson CB. Understanding the Warburg effect: the metabolic requirements of cell proliferation. Science. (2009) 324:1029–33. doi: 10.1126/science.1160809
31. Chen X, Qian Y, Wu S. The Warburg effect: evolving interpretations of an established concept. Free Radic Biol Med. (2015) 79:253–63. doi: 10.1016/j.freeradbiomed.2014.08.027
32. Weinberg SE, Chandel NS. Targeting mitochondria metabolism for cancer therapy. Nat Chem Biol. (2015) 11:9–15. doi: 10.1038/nchembio.1712
33. Goto M, Miwa H, Shikami M, Tsunekawa-Imai N, Suganuma K, Mizuno S, et al. Importance of glutamine metabolism in leukemia cells by energy production through TCA cycle and by redox homeostasis. Cancer Invest. (2014) 32:241–7. doi: 10.3109/07357907.2014.907419
34. Chen L, Shi Y, Liu S, Cao Y, Wang X, Tao Y. PKM2: the thread linking energy metabolism reprogramming with epigenetics in cancer. Int J Mol Sci. (2014) 15:11435–45. doi: 10.3390/ijms150711435
35. Chan SM, Majeti R. Role of DNMT3A, TET2, and IDH1/2 mutations in pre-leukemic stem cells in acute myeloid leukemia. Int J Hematol. (2013) 98:648–57. doi: 10.1007/s12185-013-1407-8
36. Etchegaray JP, Mostoslavsky R. Interplay between metabolism and epigenetics: a nuclear adaptation to environmental changes. Mol Cell. (2016) 62:695–711. doi: 10.1016/j.molcel.2016.05.029
37. Selak MA, Armour SM, MacKenzie ED, Boulahbel H, Watson DG, Mansfield KD, et al. Succinate links TCA cycle dysfunction to oncogenesis by inhibiting HIF-alpha prolyl hydroxylase. Cancer Cell. (2005) 7:77–85. doi: 10.1016/j.ccr.2004.11.022
38. Liu PP, Liao J, Tang ZJ, Wu WJ, Yang J, Zeng ZL, et al. Metabolic regulation of cancer cell side population by glucose through activation of the Akt pathway. Cell Death Differ. (2014) 21:124–35. doi: 10.1038/cdd.2013.131
39. Snyder V, Reed-Newman TC, Arnold L, Thomas SM, Anant S. Cancer stem cell metabolism and potential therapeutic targets. Front Oncol. (2018) 8:203. doi: 10.3389/fonc.2018.00203
40. Witherspoon M, Sandu D, Lu C, Wang K, Edwards R, Yeung A, et al. ETHE1 overexpression promotes SIRT1 and PGC1α mediated aerobic glycolysis, oxidative phosphorylation, mitochondrial biogenesis and colorectal cancer. Oncotarget. (2019) 10:4004–4017. doi: 10.18632/oncotarget.26958
41. Xing F, Luan Y, Cai J, Wu S, Mai J, Gu J, et al. The anti-Warburg effect elicited by the cAMP-PGC1α pathway drives differentiation of glioblastoma cells into astrocytes. Cell Rep. (2017) 18:468–81. doi: 10.1016/j.celrep.2016.12.037
42. Xu S-N, Wang T-S, Li X, Wang Y-P. SIRT2 activates G6PD to enhance NADPH production and promote leukaemia cell proliferation. Sci Rep. (2016) 6:32734. doi: 10.1038/srep32734
43. Guo L, Guo YY, Li BY, Peng WQ, Chang XX, Gao X, et al. Enhanced acetylation of ATP-citrate lyase promotes the progression of nonalcoholic fatty liver disease. J Biol Chem. (2019) 294:11805–16. doi: 10.1074/jbc.RA119.008708
44. Wang J, Ye W, Yan X, Guo Q, Ma Q, Lin F, et al. Low expression of ACLY associates with favorable prognosis in acute myeloid leukemia. J Transl Med. (2019) 17:149. doi: 10.1186/s12967-019-1884-5
45. Xu L, Wang L, Zhou L, Dorfman RG, Pan Y, Tang D, et al. The SIRT2/cMYC pathway inhibits peroxidation-related apoptosis in cholangiocarcinoma through metabolic reprogramming. Neoplasia. (2019) 21:429–41. doi: 10.1016/j.neo.2019.03.002
46. Schwer B, Bunkenborg J, Verdin RO, Andersen JS, Verdin E. Reversible lysine acetylation controls the activity of the mitochondrial enzyme acetyl CoA synthetase 2. Proc Natl Acad Sci USA. (2006) 103:10224–9. doi: 10.1073/pnas.0603968103
47. Yao L, Guo X, Gui Y. Acetyl-CoA synthetase 2 promotes cell migration and invasion of renal cell carcinoma by upregulating lysosomal-associated membrane protein 1 Expression. Cell Physiol Biochem. (2018) 45:984–92. doi: 10.1159/000487293
48. Hur H, Kim YB, Ham IH, Lee D. Loss of ACSS2 expression predicts poor prognosis in patients with gastric cancer. J Surg Oncol. (2015) 112:585–91. doi: 10.1002/jso.24043
49. Xu L, Li Y, Zhou L, Dorfman RG, Liu L, Cai R, et al. SIRT3 elicited an anti-Warburg effect through HIF1α/PDK1/PDHA1 to inhibit cholangiocarcinoma tumorigenesis. Cancer Med. (2019) 8:2380–91. doi: 10.1002/cam4.2089
50. Hirschey MD, Shimazu T, Goetzman E, Jing E, Schwer B, Lombard DB, et al. SIRT3 regulates mitochondrial fatty-acid oxidation by reversible enzyme deacetylation. Nature. (2010) 464:121–5. doi: 10.1038/nature08778
51. Huang Li T, Li X, Zhang L, Sun L, He X, et al. HIF-1-mediated suppression of acyl-CoA dehydrogenases and fatty acid oxidation is critical for cancer progression. Cell Rep. (2014) 8:1930–42. doi: 10.1016/j.celrep.2014.08.028
52. Yu W, Denu RA, Krautkramer KA, Grindle KM, Yang DT, Asimakopoulos F, et al. Loss of SIRT3 provides growth advantage for B cell malignancies. J Biol Chem. (2016) 291:3268–79. doi: 10.1074/jbc.M115.702076
53. Kong X, Wang R, Xue Y, Liu X, Zhang H, Chen Y, et al. Sirtuin 3, a new target of PGC-1alpha, plays an important role in the suppression of ROS and mitochondrial biogenesis. PLoS ONE. (2010) 5:e11707. doi: 10.1371/journal.pone.0011707
54. Kleszcz R, Paluszczak J, Baer-Dubowska W. Targeting aberrant cancer metabolism – the role of sirtuins. Pharmacol Rep. (2015) 67:1068–80. doi: 10.1016/j.pharep.2015.03.021
55. Li M, Chiang Y-L, Lyssiotis C, Teater M, Shen H, Wang L, et al. SIRT3 is a novel metabolic driver of and therapeutic target for chemotherapy resistant Dlbcls. Blood. (2017) 130:643.
56. Shimazu T, Hirschey MD, Hua L, Dittenhafer-Reed KE, Schwer B, Lombard DB, et al. SIRT3 deacetylates mitochondrial 3-hydroxy-3-methylglutaryl CoA synthase 2 and regulates ketone body production. Cell Metabolism. (2010). 12:654–61. doi: 10.1016/j.cmet.2010.11.003
57. Wan S, Xi M, Zhao HB, Hua W, Liu YL, Zhou YL. HMGCS2 functions as a tumor suppressor and has a prognostic impact in prostate cancer. Pathol Res Pract. (2019) 215:152464. doi: 10.1016/j.prp.2019.152464
58. Wei Z, Song J, Wang G, Cui X, Zheng J, Tang Y, et al. Deacetylation of serine hydroxymethyl-transferase 2 by SIRT3 promotes colorectal carcinogenesis. Nat Commun. (2018) 9:4468. doi: 10.1038/s41467-018-06812-y
59. Jeong SM, Lee A, Lee J, Haigis MC. SIRT4 protein suppresses tumor formation in genetic models of Myc-induced B cell lymphoma. J Biol Chem. (2014) 289:4135–44. doi: 10.1074/jbc.M113.525949
60. Tao J, Zhang J, Ling Y, McCall CE, Liu TF. Mitochondrial sirtuin 4 resolves immune tolerance in monocytes by rebalancing glycolysis and glucose oxidation homeostasis. Front Immunol. (2018) 9:419. doi: 10.3389/fimmu.2018.00419
61. Anderson KA, Huynh FK, Fisher-Wellman K, Stuart JD, Peterson BS, et al. SIRT4 is a lysine deacylase that controls leucine metabolism and insulin secretion. Cell Metab. (2017) 25:838–55. doi: 10.1016/j.cmet.2017.03.003
62. Viana LR, Tobar N, Busanello ENB, Marques AC, de Oliveira AG, Lima TI, et al. Leucine-rich diet induces a shift in tumour metabolism from glycolytic towards oxidative phosphorylation, reducing glucose consumption and metastasis in Walker-256 tumour-bearing rats. Sci Rep. (2019) 9:15529. doi: 10.1038/s41598-019-52112-w
63. Çeliktas M, Tanaka I, Tripathi SC, Fahrmann JF, Aguilar-Bonavides C, Villalobos P, et al. Role of CPS1 in cell growth, metabolism and prognosis in LKB1-inactivated lung adenocarcinoma. J Natl Cancer Inst. (2017) 109:djw231. doi: 10.1093/jnci/djw231
64. Yang X, Wang Z, Li X, Liu B, Liu M, Liu L, et al. SHMT2 desuccinylation by SIRT5 drives cancer cell proliferation. Cancer Res. (2018) 78:372–86. doi: 10.1158/0008-5472.CAN-17-1912
65. Wang F, Wang K, Xu W, Zhao S, Ye D, Wang Y, et al. SIRT5 desuccinylates and activates pyruvate kinase M2 to block macrophage IL-1β production and to prevent DSS-induced colitis in mice. Cell Rep. (2017) 19:2331–44. doi: 10.1016/j.celrep.2017.05.065
66. Chen X-F, Tian M-X, Sun R-Q, Zhang M-L, Zhou L-S, Jin L, et al. SIRT5 inhibits peroxisomal ACOX1 to prevent oxidative damage and is downregulated in liver cancer. EMBO Rep. (2018) 19:e45124. doi: 10.15252/embr.201745124
67. Wang Y-Q, Wang H-L, Xu J, Tan J, Fu L-N, Wang J-L, et al. Sirtuin5 contributes to colorectal carcinogenesis by enhancing glutaminolysis in a deglutarylation dependent manner. Nat Commun. (2018) 9:545. doi: 10.1038/s41467-018-02951-4
68. Du Z, Liu X, Chen T, Gao W, Wu Z, Hu Z, et al. Targeting a Sirt5-positive subpopulation overcomes multidrug resistance in wild-type Kras colorectal carcinomas. Cell Rep. (2018) 22:2677–89. doi: 10.1016/j.celrep.2018.06.063
69. Dominy JE Jr, Lee Y, Jedrychowski MP, Chim H, Jurczak MJ, Camporez JP, et al. The deacetylase Sirt6 activates the acetyltransferase GCN5 and suppresses hepatic gluconeogenesis. Mol. Cell. (2012) 48:900–13. doi: 10.1016/j.molcel.2012.09.030
70. Bhardwaj A, Das S. SIRT6 deacetylates PKM2 to suppress its nuclear localization and oncogenic functions. Proc Natl Acad Sci USA. (2016) 113:E538–47. doi: 10.1073/pnas.1520045113
71. Mitra N, Dey S. Biochemical characterization of mono ADP ribosyl transferase activity of human sirtuin SIRT7 and its regulation. Arch Biochem Biophys. (2019) 13:108226. doi: 10.1016/j.abb.2019.108226
72. Yamagata K, Yoshizawa T. Transcriptional regulation of metabolism by SIRT1 and SIRT7. Int Rev Cell Mol Biol. (2018) 335:143–66. doi: 10.1016/bs.ircmb.2017.07.009
73. Chen B, Liu Y, Jin X, Lu W, Liu J, Xia Z, et al. MicroRNA-26a regulates glucose metabolism by direct targeting PDHX in colorectal cancer cells. BMC Cancer. (2014) 14:443. doi: 10.1186/1471-2407-14-443
74. Jin LH, Wei C. Role of microRNAs in the Warburg effect and mitochondrial metabolism in cancer. Asian Pac J Cancer Prev. (2014) 15:7015–9. doi: 10.7314/APJCP.2014.15.17.7015
75. Zhang K, Zhang M, Jiang H, Liu F, Liu H, Li Y. Down-regulation of miR-214 inhibits proliferation and glycolysis in non-small-cell lung cancer cells via down-regulating the expression of hexokinase 2 and pyruvate kinase isozyme M2. Biomed Pharmacother. (2018) 105:545–552. doi: 10.1016/j.biopha.2018.06.009
76. Kao YY, Chou CH, Yeh LY, Chen YF, Chang KW, Liu CJ, et al. MicroRNA miR-31 targets SIRT3 to disrupt mitochondrial activity and increase oxidative stress in oral carcinoma. Cancer Lett. (2019) 456:40–8. doi: 10.1016/j.canlet.2019.04.028
77. Berraondo P, Teijeira A, Melero I. Cancer immunosurveillance caught in the act. Immunity. (2016) 44:525–6. doi: 10.1016/j.immuni.2016.03.004
78. Li Y, Zhu B. Editorial: metabolism of cancer cells and immune cells in the tumor microenvironment. Front Immunol. (2018) 9:3080. doi: 10.3389/fimmu.2018.03080
79. Wang Y, Bi Y, Chen X, Li C, Li Y, Zhang Z, et al. Histone deacetylase SIRT1 negatively regulates the differentiation of interleukin-9-producing CD4(+) T cells. Immunity. (2016) 44:1337–49. doi: 10.1016/j.immuni.2016.05.009
80. Warren JL, MacIver NJ. Regulation of adaptive immune cells by sirtuins. Front Endocrinol. (2019) 10:466. doi: 10.3389/fendo.2019.00466
81. van Dalen FJ, van Stevendaal MHME, Fennemann FL, Verdoes M, Ilina O. Molecular repolarisation of tumour-associated macrophages. Molecules. (2018) 24:E9. doi: 10.3390/molecules24010009
82. McGettrick AF, O'Neill LA. How metabolism generates signals during innate immunity and inflammation. J Biol Chem. (2013) 288:22893–8. doi: 10.1074/jbc.R113.486464
83. Zhou B, Yang Y, Li C. SIRT1 inhibits hepatocellular carcinoma metastasis by promoting M1 macrophage polarization via NF-κB pathway. Onco Targets Ther. (2019) 12:2519–29. doi: 10.2147/OTT.S195234
84. Lu J, Tan M, Cai Q. The Warburg effect in tumor progression: mitochondrial oxidative metabolism as an anti-metastasis mechanism. Cancer Lett. (2015) 356(2 Pt A):156–64. doi: 10.1016/j.canlet.2014.04.001
85. Chen X, Song M, Zhang B, Zhang Y. Reactive oxygen species regulate T cell immune response in the tumor microenvironment. Oxid Med Cell Longev. (2016) 2016:1580967. doi: 10.1155/2016/1580967
86. Lombard DB, Tishkoff DX, Bao J. Mitochondrial sirtuins in the regulation of mitochondrial activity and metabolic adaptation. Handb Exp Pharmacol. (2011) 206:163–88. doi: 10.1007/978-3-642-21631-2_8
87. Park HK, Hong JH, Oh YT, Kim SS, Yin J, Lee AJ, et al. Interplay between TRAP1 and sirtuin-3 modulates mitochondrial respiration and oxidative stress to maintain stemness of glioma stem cells. Cancer Res. (2019) 79:1369–82. doi: 10.1158/0008-5472.CAN-18-2558
88. Bruhs A, Haarmann-Stemmann T, Frauenstein K, Krutmann J, Schwarz T, Schwarz A. Activation of the arylhydrocarbon receptor causes immunosuppression primarily by modulating dendritic cells. J Invest Dermatol. (2015) 135:435–44. doi: 10.1038/jid.2014.419
89. Liu X, Hu H, Fan H, Zuo D, Shou Z, Liao Y, et al. The role of STAT3 and AhR in the differentiation of CD4+ T cells into Th17 and Treg cells. Medicine. (2017) 96:e6615. doi: 10.1097/MD.0000000000006615
90. Bock KW. Aryl hydrocarbon receptor (AHR) functions in NAD+ metabolism, myelopoiesis and obesity. Biochem Pharmacol. (2019) 163:128–32. doi: 10.1016/j.bcp.2019.02.021
91. Yarahmadi S, Abdolvahabi Z, Hesari Z, Tavakoli-Yaraki M, Yousefi Z, Seiri P, et al. Inhibition of sirtuin 1 deacetylase by miR-211–5p provides a mechanism for the induction of cell death in breast cancer cells. Gene. (2019) 711:143939. doi: 10.1016/j.gene.2019.06.029
92. Karwaciak I, Sałkowska A, Karaś K, Sobalska-Kwapis M, Walczak-Drzewiecka A, Pułaski Ł, et al. SIRT2 contributes to the resistance of melanoma cells to the multikinase inhibitor dasatinib. Cancers. (2019) 11:E673. doi: 10.3390/cancers11050673
Keywords: cancer, epigenetics, metabolism, sirtuin enzymes, personalized treatment
Citation: Gaál Z and Csernoch L (2020) Impact of Sirtuin Enzymes on the Altered Metabolic Phenotype of Malignantly Transformed Cells. Front. Oncol. 10:45. doi: 10.3389/fonc.2020.00045
Received: 31 October 2019; Accepted: 10 January 2020;
Published: 14 February 2020.
Edited by:
Lucia Altucci, University of Campania Luigi Vanvitelli, ItalyReviewed by:
Weiping Zheng, Jiangsu University, ChinaDante Rotili, Sapienza University of Rome, Italy
Copyright © 2020 Gaál and Csernoch. This is an open-access article distributed under the terms of the Creative Commons Attribution License (CC BY). The use, distribution or reproduction in other forums is permitted, provided the original author(s) and the copyright owner(s) are credited and that the original publication in this journal is cited, in accordance with accepted academic practice. No use, distribution or reproduction is permitted which does not comply with these terms.
*Correspondence: László Csernoch, Y3NsQGVkdS51bmlkZWIuaHU=