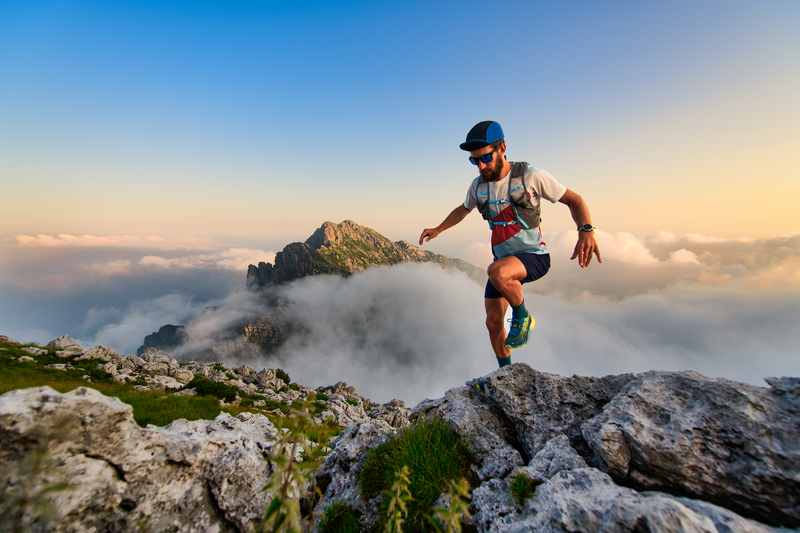
95% of researchers rate our articles as excellent or good
Learn more about the work of our research integrity team to safeguard the quality of each article we publish.
Find out more
REVIEW article
Front. Oncol. , 17 January 2020
Sec. Radiation Oncology
Volume 9 - 2019 | https://doi.org/10.3389/fonc.2019.01563
This article is part of the Research Topic Advances in Biological Understanding of Tumor Radiation Resistance View all 20 articles
A correction has been applied to this article in:
Corrigendum: Ultra-High Dose Rate (FLASH) Radiotherapy: Silver Bullet or Fool's Gold?
Radiotherapy is a cornerstone of both curative and palliative cancer care. However, radiotherapy is severely limited by radiation-induced toxicities. If these toxicities could be reduced, a greater dose of radiation could be given therefore facilitating a better tumor response. Initial pre-clinical studies have shown that irradiation at dose rates far exceeding those currently used in clinical contexts reduce radiation-induced toxicities whilst maintaining an equivalent tumor response. This is known as the FLASH effect. To date, a single patient has been subjected to FLASH radiotherapy for the treatment of subcutaneous T-cell lymphoma resulting in complete response and minimal toxicities. The mechanism responsible for reduced tissue toxicity following FLASH radiotherapy is yet to be elucidated, but the most prominent hypothesis so far proposed is that acute oxygen depletion occurs within the irradiated tissue. This review examines the tissue response to FLASH radiotherapy, critically evaluates the evidence supporting hypotheses surrounding the biological basis of the FLASH effect, and considers the potential for FLASH radiotherapy to be translated into clinical contexts.
In the UK, almost 30% of diagnosed tumors are treated with radiotherapy (RT) (1). External beam RT is a non-invasive procedure whereby tumors are targeted with ionizing radiation causing lethal damage to cancer cells resulting in cell death. However, RT also inflicts acute and chronic toxicities to the normal tissue surrounding the tumor (2–6). These radiation-induced toxicities limit the dose of radiation that can be delivered and subsequently limits the extent to which RT can be curative. Furthermore, as the number of long-term cancer survivors increases, late onset toxicities resulting from RT are emerging that significantly impact the quality of life of those patients. Consequently, there is a need for novel RT strategies that maintain the anti-tumor effect whilst limiting the extent of toxicities induced in the surrounding healthy tissue. Limiting the induction of toxicities to normal tissue would subsequently increase the therapeutic index of RT regimes (7). A number of recent studies have demonstrated that irradiation at ultra-high dose rates (FLASH) diminishes the severity of toxicities in normal tissues compared to irradiation at the conventional dose rates (CONV) currently used in clinical practice (8–18). Notably, limited data also shows that FLASH-RT reduces normal tissue toxicities whilst maintaining the anti-tumor response of CONV-RT (8–10, 15, 17, 19). FLASH-RT delivery uses irradiators with a high radiation output that allows for the entire RT treatment, or large fraction doses, to be delivered in parts of a second, compared to several minutes for CONV-RT. The short treatment times used in FLASH-RT, often shorter than 0.1 s, have the added value of minimizing treatment delivery uncertainties caused by intra-fraction motion. Carefully implemented, this would allow for smaller treatment margins and therefore smaller volumes of normal tissue being unnecessarily irradiated. Given both the radiobiological advantageous FLASH effect and its potential to “freeze” physiological motion (15, 20), FLASH-RT has the potential to be an important evolutionary step in cancer treatment. The biology underpinning the FLASH effect, however, remains unknown.
Investigation of the dose rate at which RT is delivered harks back to the 1960s, when it was demonstrated that non-cancerous mammalian cells irradiated at ultra-high dose rates had greater viability than those irradiated at conventional dose rates (21). More recently, this toxicity-limiting property of ultra-high dose rate was rediscovered and named FLASH by Favaudon et al. (10). In their study, they demonstrated that thoracic irradiation of mice with a single fraction of 17 Gy at conventional dose rates (0.03 Gy/s) induced “moderate” and “severe” regions of pulmonary fibrosis at 36 weeks post-irradiation. In contrast, when mice received the same dose at ultra-high dose rates (40–60 Gy/s) the induction of pulmonary fibrosis was starkly reduced. A greater dose of 30 Gy delivered by FLASH-RT was required to induce comparable levels of pulmonary fibrosis as seen following CONV-RT (10). Whilst exploring this reduction in pulmonary fibrosis following FLASH-RT, the same group investigated any changes in the induction of the transforming growth factor beta (TGFβ) signaling cascade—a well-documented molecular marker of radiation-induced pulmonary fibrosis (22). In accordance with their prior findings, CONV-RT of 17 Gy significantly induced TGFβ signaling; this signaling was reduced in mice that had been subjected to FLASH-RT. Once again, a greater dose of 30 Gy delivered by FLASH-RT was required to induce TGFβ signaling to the equivalent extent as seen following irradiation with CONV-RT (10). Limited TGFβ signaling following FLASH-RT has also been shown in vitro (23): this study demonstrated that even 24 h post-irradiation, CONV-RT induced 3-fold greater TGFβ signaling compared to FLASH-RT.
In addition to thoracic irradiation, it has been shown in several studies that whole brain irradiation using FLASH-RT confers neuroprotection compared to CONV-RT (13, 14, 24, 25). In one such study, mice were exposed to varying dose rates, ranging from 0.1 Gy/s to 10 Gy delivered in a single 1.8 μs pulse; at all dose rates mice were exposed to 10 Gy in a single fraction (14). Any radiation-induced neurotoxicity was measured by a novel object recognition test 2 months post-irradiation. Analysis of these data showed that mice irradiated at 0.1 Gy/s performed significantly worse on the novel object recognition test compared to the non-irradiated control. Notably, as dose rate increased, mice performed significantly better in the recognition test when irradiated at dose rates ≥ 30 Gy/s. Furthermore, there was no statistical difference in novel object recognition between mice irradiated at dose rates exceeding 100 Gy/s and non-irradiated mice (14).
In earlier studies, it was observed in rodent models that radiation-induced skin reactions could be significantly reduced at ultra-high dose rates (26, 27). Specifically, it was shown in a rat model that irradiation at 67 Gy/s induced less severe skin reactions, e.g., reddening, moist desquamation, and skin breakdown, in the short and long term compared to rats irradiated at either 1 or 0.03 Gy/s. This study also measured the deformity of the irradiated feet 6 months post-irradiation; consistent with the induction of skin reactions, the extent of deformation was less in the rats irradiated at 67 Gy/s compared to the two lower dose rates (26). Pre-clinical FLASH-RT studies have also been extended from rodent models to higher mammals such as mini-pigs and cats (16). As recently and succinctly reviewed (28), this study irradiated ten 26 mm in diameter circular patches of skin on the back of a single mini-pig to five different dose levels from 22 to 34 Gy (in 3 Gy increments), with either FLASH-RT at a dose rate of 300 Gy/s, or CONV-RT at 0.083 Gy/s. Examination 48 weeks post-irradiation showed that FLASH-RT had been well-tolerated, with only mild cutaneous depigmentation at the site of irradiation (16). In contrast, sites subjected to CONV-RT presented with clear fibronecrotic lesions. By way of extension, this study used FLASH-RT to treat six cats, all presenting with squamous cell carcinoma of the nasal planum, to a total dose ranging from 25 to 41 Gy. All six cats responded extremely well to treatment with complete remission of tumors with minimal toxicity; cats treated with the largest doses of radiation exhibited moist desquamation around the site of irradiation (16). An obvious limitation of this study is the lack of a parallel arm of cat subjects treated with CONV-RT.
Many pre-clinical studies have reported a successful FLASH normal tissue sparing effect, but it cannot be overlooked that there have also been several studies reporting no significant sparing of normal tissues following irradiation at ultra-high dose rates (29–33). For example, Smyth et al. delivered whole and partial body (abdominal or head) synchrotron irradiation to mice, at ultra-high dose rates of 37–41 Gy/s in the hope of characterizing the equivalent CONV-RT dose (32). However, comparing TD50 values (dose predicted to cause toxicity, i.e., >15–20% weight loss, severe diarrhea, moribund behavior, in 50% of the animals), this study did not observe any differential sparing between broad beam irradiation of ultra-high and conventional dose rates. A similar study by Montay-Gruel et al. delivering whole brain synchrotron irradiation at a dose rate of 37 Gy/s to mice, did however show significant neurocognitive sparing compared to conventional X-ray irradiation (24). Synchrotron irradiation beams are very flat, several cm in width but with a height on the μm-mm scale, requiring the irradiated sample to be scanned through this beam slice. For studies investigating the FLASH effect with synchrotron irradiation, the dose rate within the beam slice is likely the most important parameter. So even though the average dose rate was similar in these two studies, and probably just high enough for a FLASH sparing effect (14), the height of the beam slice through which the mice were scanned was different by a factor 20 (50 μm compared to 1 mm), corresponding to the same difference in dose rate in the slice (12 000 Gy/s compared to 600 Gy/s) (14, 32). This difference in beam slice dose rate, and of course the difference in the investigated end-points, could explain why one study found a FLASH sparing effect whilst the other study did not. A summary of in vivo studies investigating the tissue response to FLASH-RT compared to CONV-RT, across a range of tissue types, are shown in Tables 1, 2, many of which have demonstrated a reduction in radiation-induced toxicities for FLASH-RT (10–16, 24–27, 34).
Table 1. Summary of irradiation parameters and outcomes for in vivo studies investigating the FLASH effect in normal tissues (organized in order of model species and targeted tissue, as well as color coded by radiation modality).
Table 2. Summary of irradiation parameters and outcomes for in vivo studies investigating the FLASH effect in tumor tissues (organized in order of model species and targeted tissue, as well as color coded by radiation modality).
In addition to limiting toxicities, there have also been reports of FLASH-RT maintaining the same tumor response as seen following CONV-RT (8, 10, 17, 19, 35). In one such study, breast cancer, and head and neck carcinoma xenografts were established in mice (10). Both tumor models were then exposed to either FLASH-RT or CONV-RT; tumor volume was controlled independent of dose rate in breast, and head and neck xenografts. In the same study, mouse lung carcinoma luciferase-positive (luc+) TC-1 cells were transpleurally injected to generate an orthotopic lung tumor model. Thoracic irradiation of the mice with either CONV-RT or FLASH-RT, and subsequent evaluation of tumor growth using bioluminescence, showed no difference in treatment efficacy (10). Similarly in another study, human glioblastoma (GBM) were engrafted to nude mice and locally irradiated with either FLASH-RT or CONV-RT, resulting in similar tumor growth retardation (19). In the study by Bourhis et al. H454-luc+ murine GBM cells were implanted orthotopically in the striatum of nude mice. This was subsequently followed by whole brain irradiation 3 days post-implantation with either single pulse (1.8 μs) FLASH-RT or CONV-RT (0.1 Gy/s) (8). The mice were irradiated with a 10 Gy single fraction, 3 times 8 Gy, or 5 times 5 Gy, with 24 h in-between fractions. Using bioluminescence to assess the tumor burden, no significant difference could be seen between FLASH-RT and CONV-RT for any of the fractionation schemes (8). In a study by Rama et al. Lewis Lung Carcinoma (LLC) cells were inoculated into the left lung of C57Bl/6J mice (36). Two weeks post-inoculation, the whole lungs of tumor-bearing mice were irradiated with a single fraction dose of 18 Gy, using a clinical pencil beam scanning proton system. One week post treatment, CT-scans were performed to measure tumor size. Tumor size was also measured with a caliper after the mice had been sacrificed 10 days post-treatment. Surprisingly, the tumors of the mice treated with proton FLASH-RT were smaller than the tumors of the mice treated with proton CONV-RT. Moreover, immuno?uorescent staining on harvested tumor sections showed an improved recruitment of T lymphocytes into the tumor microenvironment for tumors treated with FLASH-RT compared to CONV-RT (36). Evidentially in some cases, the anti-tumor response to FLASH-RT might even be better than that of CONV-RT.
An important caveat of the pre-clinical studies investigating FLASH-RT is the lack of consistency between variables that could potentially influence the induction of the FLASH effect such as: dose rate, total dose, pulse rate, fractionation, and modality of radiation (Tables 1, 2). The study by Montay-Gruel et al. using a wide range of dose rates has helped to elucidate the extent to which dose rate modulates the FLASH effect (14). As previously described, a neuroprotective FLASH effect was apparent at dose rates ≥ 30 Gy/s with a maximal FLASH effect induced at dose rates ≥ 100 Gy/s. This relationship is important to consider when examining studies such as those by Favaudon et al. (10), and Vozenin et al. (15, 16), which used 40–60 and 300 Gy/s, respectively when administering FLASH-RT. In contrast to previously mentioned studies, a recent interesting study by Venkatesulu et al. showed a higher toxicity for FLASH-RT delivered at 35 Gy/s than for CONV-RT delivered at 0.1 Gy/s (33). This dose rate is probably on the low side for a sparing effect to occur but that does not explain the highly unexpected increased toxicity they found for FLASH-RT in all of their experiments, especially the increased toxicity of a factor 1.3–1.4 for their in vitro data. There could be many reasons for these results, e.g., the dose-rate needed for a FLASH sparing effect might not be universal but rather tissue-specific, model and/or assay specific, or there could be dosimetric differences between the two delivery modes/setups, all of which highlights the challenge in performing studies at these dose rates, finding, and exploring a beneficial FLASH effect (33). Furthermore, there is a large degree of variation in the total dose of radiation used in pre-clinical FLASH-RT studies. Compounding this, the majority of studies administer FLASH-RT in single fractions of 10 Gy or more; in many clinical situations, these are currently considered to be extremely large and unattainable fraction doses.
The source of radiation must also be considered when evaluating the FLASH effect. The FLASH effect has been predominantly observed following FLASH-RT using dedicated electron linear accelerators as the source of radiation (10, 14, 15, 18, 37). However, recent studies have expanded the FLASH field and include observations of a FLASH effect following proton (11, 23, 36) and X-ray (24) irradiation. Again, it must be noted that there have been a couple of studies that have been unable to induce a FLASH effect using proton and X-ray sources (Table 1). The reason for one X-ray study showing a FLASH effect and one study not showing an effect was discussed above. The proton study compared quasi-continuous proton beam delivery at a CONV-RT dose rate of 5 Gy/min to FLASH-RT of 100 Gy/s, without seeing any toxicity difference for zebrafish embryos (29). A reason for the absent FLASH effect might be the quasi-continuous proton beam delivery with several orders of magnitude lower dose rates within each micro-pulse (≈ 103 Gy/s) than the FLASH electron studies macro-pulses (≈ 106 Gy/s) (29). So, further to mean dose rate, total dose, and the source of radiation, the pulsatile nature of irradiation may also influence the FLASH effect. In order to induce a FLASH effect, it seems that the irradiation beam should ideally be pulsed at a frequency in the order of 100 Hz (Figure 1). Furthermore, within each pulse; irradiation should be delivered at sufficiently high dose-per-pulse, and dose rate within the pulse (≥ 1 Gy and ≥ 106 Gy/s, respectively). Together, resulting in a total treatment delivery time of maximum a few tenths of a second (Table 1). The range of variables and outcomes seen to date warrants further investigation to confirm that these are the key parameters for inducing the FLASH effect (Figure 1).
Figure 1. (Ideal) Pulsed FLASH-RT delivery. A schematic view of a pulsed beam delivery, specifying some parameters which seems to be important for inducing the FLASH effect.
The biological mechanism responsible for the reduction in normal tissue toxicities following irradiation at FLASH dose rates is not currently understood, yet several non-mutually exclusive hypotheses have been proposed. Some researchers have suggested that the differential response between FLASH-RT and CONV-RT may be due to the radiochemical depletion of oxygen at ultra-high dose rates and subsequent radioresistance conferred to the irradiated tissue (32, 38, 39). It is widely accepted that hypoxic tissues are more radioresistant than well-oxygenated tissues. This is because in the presence of molecular oxygen there is fixation of indirect radiation-induced DNA damage. Indirect damage, the predominant mechanism by which low linear energy transfer (LET) radiation induces DNA damage, occurs when radiation results in the radiolysis of water molecules and the subsequent generation of free radicals. Free radicals are then incorporated into DNA, causing damage—yet this can be easily resolved. However, if a free radical reacts with molecular oxygen, this yields a peroxyl radical. Peroxyl radicals have the potential to induce permanent damage, and are therefore a more efficacious DNA damaging agent. Hence, a lack of oxygen in the immediate environment of a cell limits the extent of radiation-induced DNA damage (40).
When considering the oxygen depletion theory, it is important to note the nature of physiologically relevant oxygen concentrations, or “physoxia” (41). Normal tissues in vivo are perfused at much lower oxygen concentrations than in vitro cell lines cultured in atmospheric oxygen concentrations. Depending on tissue type, physoxia generally lies between 3.4 and 6.8% oxygen (42). Especially relevant for current treatment with FLASH-RT limited to superficial tissues, physoxia in skin increases with depth from the surface of the skin to the dermis, from around 1.1–4.6% (43). Considering physoxia, and given the critical relationship between oxygen concentration and radiosensitivity radiochemical oxygen depletion has the potential to significantly dampen the radiobiological response.
A relationship between dose rate and oxygen consumption was proposed by Dewey and Boag in 1959 (44). They demonstrated that bacteria irradiated at ultra-high dose rates had greater survival compared to bacteria irradiated at what we now consider to be conventional dose rates. The survival curve generated following ultra-high dose rate irradiation was indicative of bacteria irradiated in a hypoxic environment. The authors hypothesized at the time that this response was a consequence of oxygen depletion following a large dose of radiation in such a short timeframe; the time for which the bacteria were irradiated for was shorter than the time required for oxygen to diffuse and restore the oxygen that had been depleted. Given that molecular oxygen is depleted as it reacts with free radicals generated from the radiolysis of water, irradiation at ultra-high dose rates is able to significantly deplete oxygen before it can replenish. This gives rise to a small window of radiobiological hypoxia.
The oxygen-depletion hypothesis has been strengthened by work demonstrating that as dose rate is increased, cellular survival mimics that of cells irradiated in an increasingly hypoxic environment (45, 46). Furthermore, it was subsequently shown in mammalian cells that the oxygen-dependent fixation of indirect DNA damage could be dampened at ultra-high dose rates (47). Importantly, the total dose at which these cells exhibited a hypoxic-like response was linear with respect to increasing the oxygen concentration in which the cells were cultured. The range of oxygen concentrations used in this study was relatively narrow (0.44–0.7% O2) and therefore the phenomenon could have been limited to cells already in hypoxic environments. However, the recent in vitro study by Adrian et al. used physiologically relevant oxygen concentrations (1.6–8.3% O2) and showed that the sparing effect of FLASH irradiation is dependent on oxygen concentration (48). An in vivo mouse model has also shown that irradiation of mouse tails at ultra-high dose rates induced radioresistance indicative of oxygen depletion (49).
Together, these data suggest that the irradiation of tissues with FLASH-RT results in radiochemical oxygen depletion, giving rise to an extremely acute period of hypoxia within the irradiated tissue and consequently a transient radioresistance (Figure 2). This phenomenon is not seen following irradiation with CONV-RT as radiation is delivered with much smaller pulses and over a longer timeframe. Hence during CONV-RT, oxygen depletion is limited, and there is sufficient time for oxygen to diffuse into the irradiated region to replace oxygen that has been lost. Therefore, oxygen concentration within the irradiated tissue is maintained.
Figure 2. The oxygen depletion hypothesis. The relationship between oxygen tension (horizontal axis) and radiation sensitivity (vertical axis) is shown schematically and has been widely reported (40, 41). In response to FLASH-RT, the physiological level of oxygen (physoxic) found in normal tissues decreases rapidly (pink arrow) and has an important impact on radiation sensitivity. This temporary or transient hypoxia protects the normal tissues as radiation resistance increases. In contrast, oxygen levels are low (hypoxic) in tumor tissues and consequently FLASH-RT has less of an impact on radiation sensitivity.
There is growing interest surrounding other oxygen-based radicals as a potential mechanism bridging the local oxygen depletion observed following irradiation at ultra-high dose rates, and reduced toxicities to normal tissue. A recent study proposes that oxygen depletion at ultra-high dose rates promotes the protection of normal tissue by limiting the production of reactive oxygen species (ROS) (13). This study repeated previous work, demonstrating that whole brain irradiation of C57Bl6/J mice with FLASH-RT did not induce cognitive impairments at dose rates exceeding 100 Gy/s compared to non-irradiated controls. Moreover, in support of a critical role for oxygen in the FLASH effect, increasing the local oxygen concentration in mice brains through carbogen breathing reversed the cognitive protection conferred by FLASH-RT. Furthermore, zebrafish embryos were subjected to either FLASH-RT or CONV-RT in the presence or absence of two well-documented ROS scavengers: N-acetyl-cysteine (NAC), and amifostine (13). Giving weight to the involvement of ROS in the FLASH effect, zebrafish embryos exposed to FLASH-RT in combination with a ROS scavenger had no effect on zebrafish length 5 days post-irradiation. However, zebrafish embryos exposed to CONV-RT alone were significantly shorter than those exposed to CONV-RT in combination with a ROS scavenger (13). This provides crude but encouraging evidence suggesting that toxicities arising from CONV-RT are in part due to the generation of ROS, and that the generation of these species is reduced following FLASH-RT. The largest limitation of this study is that there are no direct measurements of ROS in a physiological context. Instead, water containing 4% aqueous oxygen was irradiated at either ultra-high or conventional dose rates; conventional dose rates generated significantly greater ROS than ultra-high dose rates (13). Despite this short fall, the interesting findings detailed upon irradiation in combination with antioxidants merits further exploration into the role of ROS for the FLASH effect.
The oxygen depletion hypothesis seems to explain the reduced toxicity of FLASH-RT to normal tissue. However, it does not easily explain how FLASH-RT can maintain tumor response relative to CONV-RT. Although tumors are more hypoxic compared to their normal tissue counterparts, most are not completely anoxic (42). Therefore, following FLASH-RT, there will also be radiochemical depletion of oxygen within the tumor, hence it would be expected that this would confer radioresistance to the tumor. In contrast to experimental data (8, 10, 19), one would subsequently expect to observe reduced tumor control following FLASH-RT relative to CONV-RT. Though, for highly hypoxic tumor models the reduced tumor control would be expected to be minimal (Figure 2). A possible explanation for the maintained tumor control is proposed in a recent paper by Spitz et al. They hypothesized that higher levels of redox-active iron (labile iron) in tumor compared to normal tissue and differences in oxidative metabolism between normal and tumor tissues, with the more rapid removal and decay of the organic hydroperoxides and free radicals derived from peroxidation chain reactions in normal tissue, defines the beneficial therapeutic index of the FLASH effect (50). Interestingly, a recent computational model of oxygen depletion induced by FLASH-RT concluded that radiochemical oxygen depletion at an expected rate of 0.42 mmHg/Gy would be sufficient to confer radioresistance (51). However, this conclusion was predicated on the basis that radioresistance would only be conferred to already hypoxic tissues. To explore this, it would be interesting to compare the DNA repair proficiency of normal tissue relative to tumor tissue; perhaps radioresistance induced in tumor tissue by oxygen depletion is compensated for by a lower ability of DNA repair compared to normal tissue. Regions of hypoxia occur in the majority of solid tumors as opposed to the physoxia found in the surrounding normal tissue. This may well be relevant to the relative repair of DNA damage induced by FLASH-RT as exposure to hypoxia has also been described to lead to the repression of the DNA repair pathways including homologous recombination (HR), non-homologous end joining (NHEJ), and base excision repair (BER) (52, 53). To test this hypothesis, the rate of DNA repair, assayed for example by determining the appearance and resolution of 53BP1 foci, should be measured in both normal and tumor cells after exposure to FLASH-RT.
The vast majority of data pertaining to the oxygen depletion theory has been extrapolated from cell survival responses following irradiation at different dose rates (44–47, 49, 54). Therefore, there must be more direct measurements of any potential oxygen flux in tissues following irradiation at ultra-high dose rates. However, given the supposed brevity of any hypoxia induced by FLASH-RT, this is extremely difficult; it has been inferred that reoxygenation by diffusion of a tissue following FLASH-RT occurs after just 10−3 s (54). Hypoxia for such a brief moment can certainly not be detected by measuring markers of a hypoxia-mediated transcriptional response, which would be observed following a longer period of hypoxia (41). However, it is unknown whether a chemical marker of hypoxia, such as pimonidazole (55) is sufficiently sensitive to detect such an acute period of hypoxia.
A modified immune response following FLASH-RT relative to CONV-RT has also been proposed as a potential mechanism for the FLASH effect (9, 38). The fractionated RT regimes commonly used in CONV-RT, result in the irradiation of a greater proportion of circulating lymphocytes compared to total dose delivered in a single fraction (56). Following a standard regime of thirty fractions of 2 Gy, 98.8% of the blood pool has been exposed to more than 0.5 Gy. Additionally, it has been reported that the induction of chromosomal aberrations in the circulating blood pool is dependent on the total volume of the blood pool irradiated (57). Therefore, in accordance with the short irradiation time, characteristic of FLASH-RT, it would follow that fewer lymphocytes would be irradiated and subsequently reduced induction of chromosomal aberrations (9, 38, 56). However, FLASH-RT would expose lymphocytes to a greater dose of radiation, albeit much fewer of them, in comparison to CONV-RT. If a modified immune response contributes to the FLASH effect, one would expect a fractionated FLASH-RT regime to, at least in part, reduce any protection conferred by the FLASH effect.
This hypothesis has been strengthened recently by a study that carried out genome-wide microarray analysis on mice following FLASH-RT and CONV-RT (11). This study reported that immune system wide activation and maturation was dampened in mice following FLASH-RT relative to CONV-RT. Also as mentioned above, the study by Rama et al. showed an improved recruitment of T lymphocytes into the tumor microenvironment for tumors treated with FLASH-RT compared to CONV-RT, which gives merit to this hypothesis (36). In several studies, immunocompromised animals were used to compare treatment efficacy of FLASH-RT and CONV-RT with no observed difference in tumor response (Table 2), which could be interpreted to further strengthen the hypothesis (7, 8, 10, 35). It is worth noting however, that any evidence linking an immune role to the FLASH effect is correlative rather than causative; it is unclear whether any differential immune response following irradiation at ultra-high dose rates contributes to the FLASH effect, or is a consequence of it. Additionally, since the FLASH effect has been observed in vitro in bacterial and cell culture models, which are devoid of a functioning immune system, any immunological component is likely to be responsible for only part of the underlying mechanism. More studies are needed to clarify if the immune response or other biological responses like DNA damage response or inflammation is different following FLASH-RT compared to CONV-RT, and if they are part of the underlying mechanism resulting in the FLASH effect.
The obvious endpoint of investigation into the FLASH effect is the translation of FLASH-RT to the clinic. FLASH-RT could be translated to the clinic to serve two general purposes. Firstly, the FLASH effect could be exploited to allow for escalation of total dose in the treatment of radioresistant tumors that are currently associated with poorer patient outcomes (8). In this case, it is hypothesized that a greater dose of radiation could be delivered to the tumor without inducing as severe toxicities to the normal surrounding tissue as would be expected following CONV-RT. Secondly, FLASH-RT could be used in situations in which RT confers good levels of tumor control but is associated with severe normal tissue toxicity—the same total dose would be administered, but hypothetically FLASH-RT would induce less severe toxicities compared to CONV-RT.
Despite these exciting potential applications of FLASH-RT, the extent to which it is clinically viable in practice is questionable. As reviewed above, there are some inconsistencies in the results from the pre-clinical studies. Furthermore, a proportion of these studies are designed with significant limitations, such as using a single subject and a lack of controls irradiated at conventional dose rates (15). Moreover, the results emerging from pre-clinical studies put into question the suitability of FLASH-RT in many clinical situations. Independent studies that have successfully observed a FLASH effect report a dose-modifying factor of about 20–40% in favor of FLASH-RT relative to CONV-RT (Table 1). However, these same studies only report a FLASH effect at total doses of 10 Gy or more. This point is particularly well-illustrated in the recent study by Vozenin et al. (16). In a zebrafish model, whereby zebrafish embryos were irradiated with FLASH-RT or CONV-RT at doses ranging from 5 to 12 Gy, increasing in 1 Gy increments, zebrafish length was recorded 5 days post-irradiation as a measure of radiation-induced toxicity. A significant difference in morphology between those irradiated with FLASH-RT or CONV-RT was only apparent at doses ≥ 10 Gy. Even when accounting for the dose modifying factor of FLASH-RT, an equivalent dose per fraction of 6–8 Gy given by CONV-RT may still be considered as too large a dose in various clinical scenarios (58–60), such as in the treatment of larger, locally advanced tumors. A previous phase I dose escalation study in locally advanced non-small cell lung cancer (NSCLC) utilized hypofractionated treatment with doses per fraction well-below those required for a FLASH effect (58). Six patients developed late onset, grade 4–5 toxicities that were attributed to damage to the proximal bronchial tree, ergo highlighting the need for caution when employing hypofractionated regimes. Hypofractionation is nevertheless getting more widely used in the clinic for a variety of treatments sites (59, 61–64), and could be proven even more useful together with FLASH-RT and its (potentially) lower level of normal tissue toxicity.
One of the most interesting advancements in the FLASH field is the first human patient treated with FLASH-RT (9). A 75-year-old male presenting with multiresistant CD30+ T cell cutaneous lymphoma was offered the opportunity to be first human subject of FLASH-RT. A 35 mm lesion was exposed to a dose rate exceeding 106 Gy/s in each of ten discreet 1 μs pulses to a total dose of 15 Gy. This equates to a mean dose rate of 167 Gy/s, and 1.5 Gy per pulse. Following treatment, shrinkage of the lesion was observed 10 days post-irradiation culminating in a complete tumor response 36 days post-irradiation which was maintained for the following 5 months. From the point at which the lesion initially began to shrink, the patient presented with redness and mild (grade 1) oedema and epithelitis around the site of irradiation. This was starkly different to the patient's other lesions treated with CONV-RT that resulted in high-grade acute reactions to the surrounding skin that took ~3–4 months to heal (9). Despite the promising outcome for this patient, this should not be considered evidence confirming that FLASH-RT can be successfully translated to the clinic. This study was performed in a single patient that only allowed for limited comparison of the differential response between FLASH-RT and CONV-RT. An appropriately powered, randomized controlled trial with FLASH-RT and CONV-RT arms would be required to definitively show whether FLASH-RT is associated with superior clinical outcomes. At the very least, a positive phase II, single-arm study of FLASH-RT in a sample of participants truly representative of real-world patients is required before the routine adoption of FLASH-RT can be seriously entertained. If 4.5–20 MeV electron beams are to be used for the clinical trials, they would be limited to treating surficial tumors or treating tumors with intra-operative radiation therapy (IORT). Currently, FLASH-RT clinical trials on deep-seated tumors can only be performed with proton beams (Table 3). However, to treat tumors with a proton beam in a clinical trial, the beam needs to be scattered or scanned to cover the target volume which reduces the average dose rate (65). So before performing clinical trial, pre-clinical studies are needed to ensure that the FLASH effect is not lost due to either the increased LET in the Bragg peak or to the required scattering/scanning of the beam.
Table 3. Some relevant advantages and disadvantages of current and prospective FLASH radiotherapy sources (color coded by radiation modality).
As previously mentioned, most studies showing a FLASH effect has dedicated electron linear accelerators as the source of radiation (9, 10, 14, 15, 18, 37). Recent studies have shown that clinical linear accelerators can be modified to deliver FLASH-RT with electrons, largely increasing the potential availability of FLASH-RT devices and facilitating the translation to clinical trials (66, 67). However, an obvious limitation is the depth penetration with 4.5–20 MeV electron beams, only reaching to a few cm depths in tissue (Table 3). Consequently, other treatment devices/techniques are needed for FLASH-RT to be clinically useful for more than superficial treatments with external beam RT or IORT. A solution to the limited depth penetration would be to use electron beams of higher energy, so called Very High Energy Electron (VHEE) beams, with beam energies of 100–250 MeV. Such beams have good depth penetration, sharp beam penumbra, and are less sensitive to tissue heterogeneity than conventional X-ray beams (68, 69). Also, using electromagnets, the beam can in theory be focused to the tumor volume, resulting in dose-to-target conformity with a single beam comparable to that of modern X-ray treatment techniques, e.g., intensity-modulated radiation therapy (IMRT) and volumetric modulated arc therapy (VMAT). A single beam delivery might prove essential for retaining the FLASH effect in clinical trials. Unfortunately, these beams are currently limited to research accelerators which are either rather large (linear accelerator) or suffers from a low pulse rate, a small beam size, and stability issues (laser-based accelerators) (68–71). A recent paper showed (using a 160 kV X-ray beam) that conventional X-ray tubes could potentially be used for FLASH-RT studies (72). This is interesting as such systems are small, relatively inexpensive and clinically available (Table 3). Similar however to the electron linear accelerators, the depth penetration is a limiting factor making it useful only down to a few mm depth in tissue, an additional limitation is the beam size of only a few cm. Synchrotron sources has similar beam energies as X-ray tubes but has the added advantage of the possibility of using spatially fractionated ultra-high dose rate microbeam radiation therapy (MRT). MRT is characterized by arrays of quasi-parallel micro-planar beams with a width of 25–100 μm, typically separated by 100–400 μm (32). Since its invention in 1992, numerous pre-clinical studies have shown extraordinary tolerance of normal organs and blood vessels exposed to fractionated radiation doses in excess of 100 Gy in-beam (peak) doses, with dose rates exceeding several hundred Gy/s. The combined effect of spatially fractionated microbeams and FLASH dose rates have been shown in small animal models to achieve therapeutic ratios that clearly exceed those obtained by conventional X-ray with a homogeneous dose distribution and CONV-RT dose rates, in a range of malignancies, including gliomas, gliosarcomas, human squamous cell carcinomas, and glioblastomas (73). The disadvantage of this technique is the requirement of synchrotrons, which are very large, expensive, and therefore of limited availability. A platform that might solve both the size and stability issue of VHEE beams and also allow for the production of 6–10 MV FLASH X-ray beams, is PHASER (Pluridirectional High-energy Agile Scanning Electronic Radiotherapy). The PHASER concept has been presented by Maxim et al. and might be an ideal way for introducing FLASH into the clinic (20). Included in the concept is a novel and quick image-guided technique. New or highly adapted image-guidance techniques are needed for the clinical treatment of deep-seated tumors with FLASH-RT, regardless of radiation modality. The PHASER is reliant on technical advances and novel innovations in linear accelerator technology, radiofrequency science and medical physics, which in turn requires time and funding for research and development. Therefore, it is still under development (Table 3). Alternative concepts of producing 6–10 MV FLASH X-ray beams would be to use multiple synchronized linear accelerators or a powerful recirculating accelerator (74). Albeit large and expensive, a clinically available system for treating deep-seated tumors with FLASH-RT is with proton beams (75, 76). Clinical proton beams have good depth penetration, are often electromagnetically steered, and can produce conformal dose distributions with a single to a few beams (65). There have been studies (published and unpublished) with mixed reports on a FLASH effect with protons but significant resources have now been put into research on proton FLASH-RT by the principal vendors for proton RT devices, which should expedite the translation of proton FLASH-RT into clinical trials (77–79).
The FLASH effect is an extremely interesting radiobiological phenomenon that confers some degree of protection compared to CONV-RT. The FLASH effect has now been observed across a range of animal models, and more recently has been suggested in a human patient for the first time. Of equal importance, limited data would suggest that FLASH-RT maintains a similar tumor response to CONV-RT. Together, this raises the prospect that FLASH-RT will allow patients to receive a greater total dose of radiation prior to the induction of unacceptable toxicities that currently limit RT regimes.
There has been much speculation regarding the biological mechanism(s) underpinning the FLASH effect. It is well-established that irradiation results in the radiochemical depletion of oxygen; this is particularly prevalent at ultra-high dose rates. From the data currently available, we can safely conclude that oxygen depletion contributes, at least in part, to the FLASH effect. However, the extent of its contribution remains unknown and therefore warrants further investigation. Aside from oxygen depletion, an immune modulatory role has been broadly implicated in the FLASH effect, yet evidence to support this is currently sparse and preliminary. Likewise, any potential immune-mediated contribution to the FLASH effect requires much greater exploration.
Aside from mechanistic insights, the overarching question remains of the translational potential of FLASH-RT to clinical environments. Despite independent studies concluding that FLASH-RT confers a dose modifying factor of 20–40%, the repeated finding that the FLASH effect is only evident at total doses of 10 Gy or more means that FLASH-RT would not be suitable in many clinical cases. As a result of further investigation into the biological basis of the FLASH effect, it may eventually be possible to generate a FLASH effect at smaller doses, therefore further increasing the clinical potential of FLASH-RT. Another limiting factor in translating FLASH-RT to the clinic is the availability of radiation sources, capable of producing beams suitable for treatment of deep-seated as well as superficial tumors with ultra-high dose rates. In summary, with shorter treatment times and lower levels of toxicity, FLASH-RT may 1 day have the potential to be a paradigm shift in the field of RT. For this to be the case, however, there is a real need to identify the mechanism(s) behind the FLASH effect. The currently available data more than justifies this further investigation.
JW and KP wrote the article. EH and GH contributed ideas to, read, and edited the article.
JW was grateful for support from the Royal College of Radiologists, and Oriel College, Oxford.
The authors declare that the research was conducted in the absence of any commercial or financial relationships that could be construed as a potential conflict of interest.
The reviewer M-CV declared a past supervisory role with one of the authors KP to the handling editor.
1. NCRAS, CRUK. Chemotherapy, Radiotherapy and Tumour Resection By Tumour & Patient Characteristics in England, 2013–2015. National Cancer Registration & Analysis Service and Cancer Research UK (2018).
2. Siddiqui F, Movsas B. Management of radiation toxicity in head and neck cancers. Semin Radiat Oncol. (2017) 27:340–9. doi: 10.1016/j.semradonc.2017.04.008
3. Nicholas S, Chen L, Choflet A, Fader A, Guss Z, Hazell S, et al. Pelvic radiation and normal tissue toxicity. Semin Radiat Oncol. (2017) 27:358–69. doi: 10.1016/j.semradonc.2017.04.010
4. Smart D. Radiation toxicity in the central nervous system: mechanisms and strategies for injury reduction. Semin Radiat Oncol. (2017) 27:332–9. doi: 10.1016/j.semradonc.2017.04.006
5. Jelonek K, Pietrowska M, Widlak P. Systemic effects of ionizing radiation at the proteome and metabolome levels in the blood of cancer patients treated with radiotherapy: the influence of inflammation and radiation toxicity. Int J Radiat Biol. (2017) 93:683–96. doi: 10.1080/09553002.2017.1304590
6. Simone CB. Thoracic radiation normal tissue injury. Semin Radiat Oncol. (2017) 27:370–7. doi: 10.1016/j.semradonc.2017.04.009
7. Montay-Gruel P, Meziani L, Yakkala C, Vozenin MC. Expanding the therapeutic index of radiation therapy by normal tissue protection. Br J Radiol. (2018). doi: 10.1259/bjr.20180008. [Epub ahead of print].
8. Bourhis J, Montay-Gruel P, Goncalves Jorge P, Bailat C, Petit B, Ollivier J, et al. Clinical translation of FLASH radiotherapy: why and how? Radiother Oncol. (2019) 139:11–7. doi: 10.1016/j.radonc.2019.04.008
9. Bourhis J, Sozzi WJ, Jorge PG, Gaide O, Bailat C, Duclos F, et al. Treatment of a first patient with FLASH-radiotherapy. Radiother Oncol. (2019) 139:18–22. doi: 10.1016/j.radonc.2019.06.019
10. Favaudon V, Caplier L, Monceau V, Pouzoulet F, Sayarath M, Fouillade C, et al. Ultrahigh dose-rate FLASH irradiation increases the differential response between normal and tumor tissue in mice. Sci Transl Med. (2014) 6:245ra293. doi: 10.1126/scitranslmed.3008973
11. Girdhani S, Abel E, Katsis A, Rodriquez A, Senapati S, KuVillanueva A, et al. Abstract LB-280: FLASH: A novel paradigm changing tumor irradiation platform that enhances therapeutic ratio by reducing normal tissue toxicity and activating immune pathways. Cancer Res. (2019) 79(13 Suppl):LB–280. doi: 10.1158/1538-7445.AM2019-LB-280
12. Loo BW, Schuler E, Lartey FM, Rafat M, King GJ, Trovati S, et al. (P003) Delivery of ultra-rapid flash radiation therapy and demonstration of normal tissue sparing after abdominal irradiation of mice. Int J Radiat Oncol Biol Phys. (2017) 98:E16. doi: 10.1016/j.ijrobp.2017.02.101
13. Montay-Gruel P, Acharya MM, Petersson K, Alikhani L, Yakkala C, Allen BD, et al. Long-term neurocognitive benefits of FLASH radiotherapy driven by reduced reactive oxygen species. Proc Natl Acad Sci USA. (2019) 116:10943–51. doi: 10.1073/pnas.1901777116
14. Montay-Gruel P, Petersson K, Jaccard M, Boivin G, Germond JF, Petit B, et al. Irradiation in a flash: unique sparing of memory in mice after whole brain irradiation with dose rates above 100Gy/s. Radiother Oncol. (2017) 124:365–9. doi: 10.1016/j.radonc.2017.05.003
15. Vozenin MC, De Fornel P, Petersson K, Favaudon V, Jaccard M, Germond JF, et al. The advantage of FLASH radiotherapy confirmed in mini-pig and cat-cancer patients. Clin Cancer Res. (2019) 25:35–42. doi: 10.1158/1078-0432.CCR-17-3375
16. Vozenin MC, Hendry JH, Limoli CL. Biological benefits of ultra-high dose rate FLASH radiotherapy: sleeping beauty awoken. Clin Oncol. (2019) 31:407–15. doi: 10.1016/j.clon.2019.04.001
17. Levy K, Natarajan S, Wang J, Chow S, Eggold J, Loo P, et al. FLASH irradiation enhances the therapeutic index of abdominal radiotherapy in mice. bioRxiv [Prepint]. (2019). doi: 10.1101/2019.12.12.873414
18. Fouillade C, Curras-Alonso S, Giuranno L, Quelennec E, Heinrich S, Bonnet-Boissinot S, et al. FLASH irradiation spares lung progenitor cells and limits the incidence of radio-induced senescence. Clin Cancer Res. (2019). doi: 10.1158/1078-0432.CCR-19-1440
19. Montay - Gruel P, Petit B, Bochud F, Favaudon V, Bourhis J, Vozenin MC. PO-0799: normal brain, neural stem cells and glioblastoma responses to FLASH radiotherapy. Radiother Oncol. (2015) 115:S400–1. doi: 10.1016/S0167-8140(15)40791-1
20. Maxim PG, Tantawi SG, Loo BW. PHASER: a platform for clinical translation of FLASH cancer radiotherapy. Radiother Oncol. (2019) 139:28–33. doi: 10.1016/j.radonc.2019.05.005
21. Berry RJ, Hall EJ, Forster DW, Storr TH, Goodman MJ. Survival of mammalian cells exposed to x rays at ultra-high dose-rates. Br J Radiol. (1969) 42:102–7. doi: 10.1259/0007-1285-42-494-102
22. Rube CE, Uthe D, Schmid KW, Richter KD, Wessel J, Schuck A, et al. Dose-dependent induction of transforming growth factor beta (TGF-beta) in the lung tissue of fibrosis-prone mice after thoracic irradiation. Int J Radiat Oncol. (2000) 47:1033–42. doi: 10.1016/S0360-3016(00)00482-X
23. Buonanno M, Grilj V, Brenner DJ. Biological effects in normal cells exposed to FLASH dose rate protons. Radiother Oncol. (2019) 139:51–5. doi: 10.1016/j.radonc.2019.02.009
24. Montay-Gruel P, Bouchet A, Jaccard M, Patin D, Serduc R, Aim W, et al. X-rays can trigger the FLASH effect: Ultra-high dose-rate synchrotron light source prevents normal brain injury after whole brain irradiation in mice. Radiother Oncol. (2018) 129:582–8. doi: 10.1016/j.radonc.2018.08.016
25. Simmons DA, Lartey FM, Schüler E, Rafat M, King G, Kim A, et al. Reduced cognitive deficits after FLASH irradiation of whole mouse brain are associated with less hippocampal dendritic spine loss and neuroinflammation. Radiother Oncol. (2019) 139:4–10. doi: 10.1016/j.radonc.2019.06.006
26. Field SB, Bewley DK. Effects of dose-rate on the radiation response of rat skin. Int J Radiat Biol Relat Stud Phys Chem Med. (1974) 26:259–67. doi: 10.1080/09553007414551221
27. Inada T, Nishio H, Amino S, Abe K, Saito K. High dose-rate dependence of early skin reaction in mouse. Int J Radiat Biol Relat Stud Phys Chem Med. (1980) 38:139–45. doi: 10.1080/09553008014551031
28. Harrington KJ. Ultrahigh dose-rate radiotherapy: next steps for FLASH-RT. Clin Cancer Res. (2019) 25:3–5. doi: 10.1158/1078-0432.CCR-18-1796
29. Beyreuther E, Brand M, Hans S, Hideghéty K, Karsch L, Leßmann E, et al. Feasibility of proton FLASH effect tested by zebrafish embryo irradiation. Radiother Oncol. (2019) 139:46–50. doi: 10.1016/j.radonc.2019.06.024
30. Beyreuther E, Karsch L, Laschinsky L, Leßmann E, Naumburger D, Oppelt M, et al. Radiobiological response to ultra-short pulsed megavoltage electron beams of ultra-high pulse dose rate. Int J Radiat Biol. (2015) 91:643–52. doi: 10.3109/09553002.2015.1043755
31. Oppelt M, Baumann M, Bergmann R, Beyreuther E, Brüchner K, Hartmann J, et al. Comparison study of in vivo dose response to laser-driven versus conventional electron beam. Radiat Environ Biophys. (2015) 54:155–66. doi: 10.1007/s00411-014-0582-1
32. Smyth LML, Donoghue JF, Ventura JA, Livingstone J, Bailey T, Day LRJ, et al. Comparative toxicity of synchrotron and conventional radiation therapy based on total and partial body irradiation in a murine model. Sci Rep. (2018) 8:12044. doi: 10.1038/s41598-018-30543-1
33. Venkatesulu BP, Sharma A, Pollard-Larkin JM, Sadagopan R, Symons J, Neri S, et al. Ultra high dose rate (35 Gy/sec) radiation does not spare the normal tissue in cardiac and splenic models of lymphopenia and gastrointestinal syndrome [published online ahead of print 2019/11/22]. Sci Rep. (2019) 9:17180. doi: 10.1038/s41598-019-53562-y
34. Hornsey S, Bewley DK. Hypoxia in mouse intestine induced by electron irradiation at high dose-rates. Int J Radiat Biol Relat Stud Phys Chem Med. (1971) 19:479–83. doi: 10.1080/09553007114550611
35. Zlobinskaya O, Siebenwirth C, Greubel C, Hable V, Hertenberger R, Humble N, et al. The effects of ultra-high dose rate proton irradiation on growth delay in the treatment of human tumor xenografts in nude mice. Radiat Res. (2014) 181:177–83. doi: 10.1667/RR13464.1
36. Rama N, Saha T, Shukla S, Goda C, Milewski D, Mascia AE, et al. Improved tumor control through t-cell infiltration modulated by ultra-high dose rate proton FLASH using a clinical pencil beam scanning proton system. Int J Radiat Oncol Biol Phys. (2019) 105:S164–5. doi: 10.1016/j.ijrobp.2019.06.187
37. Jaccard M, Durán MT, Petersson K, Germond JF, Liger P, Vozenin MC, et al. High dose-per-pulse electron beam dosimetry: commissioning of the Oriatron eRT6 prototype linear accelerator for preclinical use. Med Phys. (2018) 45:863–74. doi: 10.1002/mp.12713
38. Durante M, Bräuer-Krisch E, Hill M. Faster and safer? FLASH ultra-high dose rate in radiotherapy. Br J Radiol. (2018) 91:20170628. doi: 10.1259/bjr.20170628
39. Wilson P, Jones B, Yokoi T, Hill M, Vojnovic B. Revisiting the ultra-high dose rate effect: implications for charged particle radiotherapy using protons and light ions. Br J Radiol. (2012) 85:e933–9. doi: 10.1259/bjr/17827549
40. Hall EJ, Giaccia AJ. Radiobiology for the Radiologist, 8th Ed. Philadelphia, PA: Lippincott Williams & Wilkins (2018).
41. Hammond EM, Asselin MC, Forster D, O'Connor JP, Senra JM, Williams KJ. The meaning, measurement and modification of hypoxia in the laboratory and the clinic. Clin Oncol. (2014) 26:277–88. doi: 10.1016/j.clon.2014.02.002
42. McKeown SR. Defining normoxia, physoxia and hypoxia in tumours-implications for treatment response. Br J Radiol. (2014) 87:20130676. doi: 10.1259/bjr.20130676
43. Carreau A, El Hafny-Rahbi B, Matejuk A, Grillon C, Kieda C. Why is the partial oxygen pressure of human tissues a crucial parameter? Small molecules and hypoxia. J Cell Mol Med. (2011) 15:1239–53. doi: 10.1111/j.1582-4934.2011.01258.x
44. Dewey DL, Boag JW. Modification of the oxygen effect when bacteria are given large pulses of radiation. Nature. (1959) 183:1450–1. doi: 10.1038/1831450a0
45. Ewing D. Breaking survival curves and oxygen removal times in irradiated bacterial spores. Int J Radiat Biol Relat Stud Phys Chem Med. (1980) 37:321–9. doi: 10.1080/09553008014550371
46. Weiss H, Epp ER, Heslin JM, Ling CC, Santomasso A. Oxygen depletion in cells irradiated at ultra-high dose-rates and at conventional dose-rates. Int J Radiat Biol Relat Stud Phys Chem Med. (1974) 26:17–29. doi: 10.1080/09553007414550901
47. Michaels HB, Epp ER, Ling CC, Peterson EC. Oxygen sensitization of CHO cells at ultrahigh dose rates: prelude to oxygen diffusion studies. Radiat Res. (1978) 76:510–21. doi: 10.2307/3574800
48. Adrian G, Konradsson E, Lempart M, Bäck S, Ceberg C, Petersson K. The FLASH effect depends on oxygen concentration. Br J Radiol. (2019). doi: 10.1259/bjr.20190702. [Epub ahead of print].
49. Hendry JH, Moore JV, Hodgson BW, Keene JP. The constant low oxygen concentration in all the target cells for mouse tail radionecrosis. Radiat Res. (1982) 92:172–81. doi: 10.2307/3575852
50. Spitz DR, Buettner GR, Petronek MS, St-Aubin JJ, Flynn RT, Waldron TJ, et al. An integrated physico-chemical approach for explaining the differential impact of FLASH versus conventional dose rate irradiation on cancer and normal tissue responses. Radiother Oncol. (2019) 139:23–7. doi: 10.1016/j.radonc.2019.03.028
51. Pratx G, Kapp DS. A computational model of radiolytic oxygen depletion during FLASH irradiation and its effect on the oxygen enhancement ratio. Phys Med Biol. (2019) 64:185005. doi: 10.1088/1361-6560/ab3769
52. Chan N, Bristow RG. “Contextual” synthetic lethality and/or loss of heterozygosity: tumor hypoxia and modification of DNA repair. Clin Cancer Res. (2010) 16:4553–60. doi: 10.1158/1078-0432.CCR-10-0527
53. Leszczynska KB, Göttgens EL, Biasoli D, Olcina MM, Ient J, Anbalagan S, et al. Mechanisms and consequences of ATMIN repression in hypoxic conditions: roles for p53 and HIF-1. Sci Rep. (2016) 6:21698. doi: 10.1038/srep21698
54. Ling CC, Michaels HB, Epp ER, Peterson EC. Oxygen diffusion into mammalian cells following ultrahigh dose rate irradiation and lifetime estimates of oxygen-sensitive species. Radiat Res. (1978) 76:522–32. doi: 10.2307/3574801
55. Varia MA, Calkins-Adams DP, Rinker LH, Kennedy AS, Novotny DB, Fowler WC, et al. Pimonidazole: a novel hypoxia marker for complementary study of tumor hypoxia and cell proliferation in cervical carcinoma. Gynecol Oncol. (1998) 71:270–7. doi: 10.1006/gyno.1998.5163
56. Yovino S, Kleinberg L, Grossman SA, Narayanan M, Ford E. The etiology of treatment-related lymphopenia in patients with malignant gliomas: modeling radiation dose to circulating lymphocytes explains clinical observations and suggests methods of modifying the impact of radiation on immune cells. Cancer Invest. (2013) 31:140–4. doi: 10.3109/07357907.2012.762780
57. Durante M, Yamada S, Ando K, Furusawa Y, Kawata T, Majima H, et al. Measurements of the equivalent whole-body dose during radiation therapy by cytogenetic methods. Phys Med Biol. (1999) 44:1289–98. doi: 10.1088/0031-9155/44/5/314
58. Cannon DM, Mehta MP, Adkison JB, Khuntia D, Traynor AM, Tomé WA, et al. Dose-limiting toxicity after hypofractionated dose-escalated radiotherapy in non-small-cell lung cancer. J Clin Oncol. (2013) 31:4343–8. doi: 10.1200/JCO.2013.51.5353
59. Masucci GL. Hypofractionated radiation therapy for large brain metastases. Front Oncol. (2018) 8:379. doi: 10.3389/fonc.2018.00379
60. Shaw E, Scott C, Souhami L, Dinapoli R, Kline R, Loeffler J, et al. Single dose radiosurgical treatment of recurrent previously irradiated primary brain tumors and brain metastases: final report of RTOG protocol 90–05. Int J Radiat Oncol Biol Phys. (2000) 47:291–8. doi: 10.1016/S0360-3016(99)00507-6
61. Gupta A, Khan AJ, Yegya-Raman N, Sayan M, Ahlawat S, Ohri N, et al. 5-year results of a prospective phase 2 trial evaluating 3-week hypofractionated whole breast radiation therapy inclusive of a sequential boost. Int J Radiat Oncol Biol Phys. (2019) 105:267–74. doi: 10.1016/j.ijrobp.2019.05.063
62. Widmark A, Gunnlaugsson A, Beckman L, Thellenberg-Karlsson C, Hoyer M, Lagerlund M, et al. Ultra-hypofractionated versus conventionally fractionated radiotherapy for prostate cancer: 5-year outcomes of the HYPO-RT-PC randomised, non-inferiority, phase 3 trial. Lancet. (2019) 394:385–95. doi: 10.1016/S0140-6736(19)31131-6
63. Milano MT, Mihai A, Kang J, Singh DP, Verma V, Qiu H, et al. Stereotactic body radiotherapy in patients with multiple lung tumors: a focus on lung dosimetric constraints. Exp Rev Anticancer Ther. (2019) 17:959–69. doi: 10.1080/14737140.2019.1686980
64. Nabavizadeh N, Waller JG, Fain R, Chen Y, Degnin CR, Elliott DA, et al. Safety and efficacy of accelerated hypofractionation and stereotactic body radiation therapy for hepatocellular carcinoma patients with varying degrees of hepatic impairment. Int J Radiat Oncol Biol Phys. (2018) 100:577–85. doi: 10.1016/j.ijrobp.2017.11.030
65. van Marlen P, Dahele M, Folkerts M, Abel E, Slotman BJ, Verbakel WFAR. Bringing FLASH to the clinic: treatment planning considerations for ultrahigh dose-rate proton beams. Int J Radiat Oncol Biol Phys. (2019). doi: 10.1016/j.ijrobp.2019.11.011. [Epub ahead of print].
66. Lempart M, Blad B, Adrian G, Bäck S, Knöös T, Ceberg C, et al. Modifying a clinical linear accelerator for delivery of ultra-high dose rate irradiation. Radiother Oncol. (2019) 139:40–5. doi: 10.1016/j.radonc.2019.01.031
67. Schüler E, Trovati S, King G, Lartey F, Rafat M, Villegas M, et al. Experimental platform for ultra-high dose rate FLASH irradiation of small animals using a clinical linear accelerator. Int J Radiat Oncol Biol Phys. (2017) 97:195–203. doi: 10.1016/j.ijrobp.2016.09.018
68. Bazalova-Carter M, Liu M, Palma B, Dunning M, McCormick D, Hemsing E, et al. Comparison of film measurements and Monte Carlo simulations of dose delivered with very high-energy electron beams in a polystyrene phantom. Med Phys. (2015) 42:1606–13. doi: 10.1118/1.4914371
69. Schüler E, Eriksson K, Hynning E, Hancock SL, Hiniker SM, Bazalova-Carter M, et al. Very high-energy electron (VHEE) beams in radiation therapy; Treatment plan comparison between VHEE, VMAT, and PPBS. Med Phys. (2017) 44:2544–55. doi: 10.1002/mp.12233
70. Kokurewicz K, Brunetti E, Welsh GH, Wiggins SM, Boyd M, Sorensen A, et al. Focused very high-energy electron beams as a novel radiotherapy modality for producing high-dose volumetric elements. Sci Rep. (2019) 9:10837. doi: 10.1038/s41598-019-46630-w
71. Lundh O, Rechatin C, Faure J, Ben-Ismaïl A, Lim J, De Wagter C, et al. Comparison of measured with calculated dose distribution from a 120-MeV electron beam from a laser-plasma accelerator. Med Phys. (2012) 39:3501–8. doi: 10.1118/1.4719962
72. Bazalova-Carter M, Esplen N. On the capabilities of conventional x-ray tubes to deliver ultra-high (FLASH) dose rates. Med Phys. (2019) 46:5690–5. doi: 10.1002/mp.13858
73. Grotzer MA, Schültke E, Bräuer-Krisch E, Laissue JA. Microbeam radiation therapy: clinical perspectives. Phys Med. (2015) 31:564–7. doi: 10.1016/j.ejmp.2015.02.011
74. Dubinov AE, Ochkina EI. Recirculating electron accelerators with noncircular electron orbits as radiation sources for applications (a Review). Phys Particles Nuclei. (2018) 49:431–56. doi: 10.1134/S1063779618030048
75. Karsch L, Beyreuther E, Enghardt W, Gotz M, Masood U, Schramm U, et al. Towards ion beam therapy based on laser plasma accelerators. Acta Oncol. (2017) 56:1359–66. doi: 10.1080/0284186X.2017.1355111
76. Patriarca A, Fouillade C, Auger M, Martin F, Pouzoulet F, Nauraye C, et al. Experimental set-up for FLASH proton irradiation of small animals using a clinical system. Int J Radiat Oncol Biol Phys. (2018) 102:619–26. doi: 10.1016/j.ijrobp.2018.06.403
78. Fiddian-Green RG, Silen W. First Flash Irradiation Delivered in an IBA Gantry Treatment Room [Press Release] (2019).
Keywords: FLASH, radiotherapy, hypoxia, normal tissue, immune
Citation: Wilson JD, Hammond EM, Higgins GS and Petersson K (2020) Ultra-High Dose Rate (FLASH) Radiotherapy: Silver Bullet or Fool's Gold? Front. Oncol. 9:1563. doi: 10.3389/fonc.2019.01563
Received: 06 September 2019; Accepted: 24 December 2019;
Published: 17 January 2020.
Edited by:
Ira Ida Skvortsova, Innsbruck Medical University, AustriaReviewed by:
Virginie Monceau, Collège de France, FranceCopyright © 2020 Wilson, Hammond, Higgins and Petersson. This is an open-access article distributed under the terms of the Creative Commons Attribution License (CC BY). The use, distribution or reproduction in other forums is permitted, provided the original author(s) and the copyright owner(s) are credited and that the original publication in this journal is cited, in accordance with accepted academic practice. No use, distribution or reproduction is permitted which does not comply with these terms.
*Correspondence: Kristoffer Petersson, a3Jpc3RvZmZlci5wZXRlcnNzb25Ab25jb2xvZ3kub3guYWMudWs=
†ORCID: Joseph D. Wilson orcid.org/0000-0001-8878-5882
Ester M. Hammond orcid.org/0000-0002-2335-3146
Geoff S. Higgins orcid.org/0000-0003-3072-909X
Kristoffer Petersson orcid.org/0000-0003-0300-5790
Disclaimer: All claims expressed in this article are solely those of the authors and do not necessarily represent those of their affiliated organizations, or those of the publisher, the editors and the reviewers. Any product that may be evaluated in this article or claim that may be made by its manufacturer is not guaranteed or endorsed by the publisher.
Research integrity at Frontiers
Learn more about the work of our research integrity team to safeguard the quality of each article we publish.