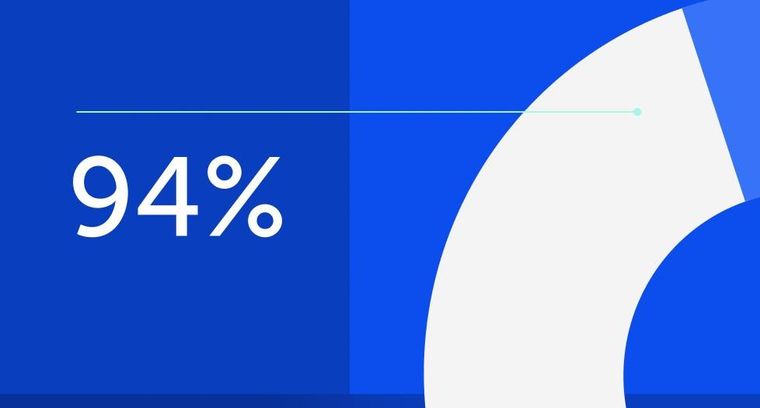
94% of researchers rate our articles as excellent or good
Learn more about the work of our research integrity team to safeguard the quality of each article we publish.
Find out more
REVIEW article
Front. Oncol., 31 January 2020
Sec. Cancer Immunity and Immunotherapy
Volume 9 - 2019 | https://doi.org/10.3389/fonc.2019.01529
This article is part of the Research TopicCurrent Perspectives, Challenges and Advances in Cell Based TherapiesView all 14 articles
Chimeric Antigen Receptor (CAR)-based therapies offer a promising, targeted approach to effectively treat relapsed or refractory B cell malignancies. However, the treatment-related toxicity defined as cytokine-release syndrome (CRS) often develops in patients, and if uncontrolled, can be fatal. Grading systems have now been developed to further characterize and objectify clinical findings in order to provide algorithm-based guidance on CRS-related treatment decisions. The pharmacological treatments associated with these algorithms suppress inflammation through a variety of mechanisms and are paramount to improving the safety profile of CAR-based therapies. However, fatalities are still occurring, and there are downsides to these treatments, including the possibility of disrupting CAR-T cell persistence. This review article will describe the clinical presentation and current management of CRS, and through our now deeper understanding of downstream signaling pathways, will provide a molecular framework to formulate new hypotheses regarding clinical applications to contain proinflammatory cytokines responsible for CRS.
Although great progress has been made in treating hematologic malignancies, patients having relapsed or refractory disease often have poor outcomes. In adults, one of the most common hematologic malignancies is diffuse large B-cell lymphoma (DLBCL), which affects an estimated 28,000 patients in the United States each year (1). Up to 50% of patients with DLBCL have refractory disease or experience relapse after initial treatment. For these patients, outcomes are very poor with long term survival rates of 20–53% (2, 3). In the pediatric population, precursor B-cell acute lymphoblastic leukemia (B-ALL) is the most common malignancy. Although >90% of children enter remission and have excellent rates of long-term survival, relapse can occur in approximately 20% of all cases. Those who relapse often succumb to their disease (4–7).
Chimeric antigen receptor (CAR) T-cell based therapies are an exciting, targeted approach to effectively treat relapsed or refractory hematologic malignancies and have helped bring immunotherapy to the forefront of cancer treatment. CAR-T cells are a biological drug that targets cancer-associated antigens using genetically-modified autologous T cells transduced with the CAR (8). Successful outcomes from early clinical trials using CD19-targeted CAR T cells have led to the FDA approval of tisagenlecleucel in 2017 and 2018 for relapsed or refractory pediatric B-ALL and large B cell lymphomas, respectively, and axicabtagene ciloleucel in 2017 for relapsed or refractory large B cell lymphomas. Building off these recent successes, new clinical trials are exploring a variety of ways to expand this therapy, including the development of CAR-based natural killer (NK) cell immunotherapy to work in diseases and circumstances where CAR-T cells may falter (9). Despite high rates of complete and partial remissions, allowing for long-term durable survivals and/or providing bridging therapy until hematopoietic cell transplantation, the trials leading to approval of CAR-T products also demonstrated significant therapy-related toxicities, specifically cytokine release syndrome (CRS) and its accompanying neurological effects (10–13).
Cytokine storm was initially used to describe the systemic inflammatory response that occurred following any antibody-based immune therapy; however, since the incidence of what is now coined CRS following CAR T-cell based therapies is high, the use of this term is now fairly synonymous with the major adverse effect of this therapy (13). CRS can begin with mild symptoms such as fever, tachycardia, tachypnea, nausea, and diarrhea. Concurrently, a spectrum of neurological toxicities known as CRS-related encephalopathy syndrome (CRES) [or immune effector cell-associated neurotoxicity syndrome (ICANS)] can develop, consisting of headaches, confusion, aphasia, and seizures. In its more severe forms, the inflammation related from CRS and/or CRES/ICANS can result in hypotension, coagulopathies, cerebral edema, and multi-organ failure (14–16). If uncontrolled, death can result. Across nine separate studies of CD19 CAR T cells including 387 patients, 83% developed CRS and 51% developed severe (>grade II) CRS (13, 17–24). The ELIANA trial (a phase III trial of tisagenlecleucel for pediatric and young adult patients) illustrates the potential morbidity of CRS. After receiving tisagenlecleucel, 77% of patients developed CRS, 25% required high-dose vasopressors for cardiac support, 13% were intubated, and 9% required dialysis (17).
Numerous CAR-based clinical trials are underway to treat hematological malignancies and solid tumors and have been life-saving for many patients (13, 25–35). However, CRS caused by CAR T cells is a major limiting factor in the successful utilization of cellular immunotherapy due to the competing risk of morbidity and mortality from the treatment itself (14). In order to improve CAR-based therapies, it is important to understand the limitations of the current CRS treatment approach and explore new strategies of CRS treatment and prevention.
Worldwide, well-over 500 active clinical trials are registered for CAR-based therapies, and the list continues to grow1. In general, autologous (or more rarely, allogeneic) T cells are transduced with retroviral, lentiviral, or transposon-based systems with the CAR construct. NK cells are also being investigated as viable alternatives to T cells (9, 36). While CD19 is the most utilized antigen in CAR trials to date, a variety of other antigens are now being investigated (37–46). Lymphodepletion with agents such as fludarabine and/or cyclophosphamide chemotherapy prior to CAR-T infusions provides reduction of T regulatory cells which can inhibit CAR-T cell activity and promotes expansion of these CAR-T cells. The use of lymphodepleting chemotherapy is now standard in clinical trials. This chemotherapy-enhanced expansion of engineered CAR-T cells can provoke initial cytokine secretion. The secondary effects of these activated T cells can later produce another wave of cytokine production by activating other surrounding immune and antigen-presenting cells, which will be discussed later (47, 48).
CARs are molecules synthetically designed to comprise an extracellular single-chain variable fragment, scFv (variable light chain, VL, and variable heavy chain, VH), from an antigen-specific antibody. This antigen-specific antibody allows CAR T cells to recognize tumor cells in a T-cell receptor (TCR) and human leukocyte antigen (HLA)-independent manner. In regard to CD19 as an extracellular receptor on CARs, since it is expressed throughout B cell maturation starting from the pro-B cell stage, targeting CD19 facilitates its use against different B cell tumors that have originated at distinct developmental stages. The cytoplasmic tail of CD3ζ is added as the primary signaling module, and in some cases, two out of four tyrosines within the immunoreceptor tyrosine-based inhibition motifs are mutated to optimize the signaling threshold. The extracellular domains are joined to intracellular signaling modules via hinge and transmembrane regions. The intracellular domains contain both the obligatory signaling module (CD3ζ) and the cytoplasmic tail of co-stimulatory molecules such as CD28 or CD137 (4-1BB). At a cellular level, combining the cytoplasmic tails of CD3ζ with CD28 or CD137 provides strong activating signals along with robust survival and proliferation signals. Early phase studies with CD19 CARs have demonstrated the utility of including these co-stimulatory signaling molecules in the CAR product (49–51).
CD3ζ uses ZAP-70/LAT/PLC-γ1 to activate NFAT or NF-κB pathways via augmenting Ca2+ and diacylglycerol (DAG) (52). Also, CD3ζ activates AP-1 through the Ras/MAPK pathway. CD28 and CD137 are also known to regulate overlapping and distinct signaling pathways (53, 54). Both CD28 and CD137 can regulate proliferation, survival, differentiation, and effector functions in CAR T cells by activating the PI(3)K/AKT/Bcl-XL cascade (55). CD28 can also affect T cell proliferation and function through Grb2, FLNa, and Lck pathways (56, 57). Similarly, 4-1BB signaling is dependent on TNF-receptor Associated Factor, or TRAF pathway. Thus, the interactions of these multiple downstream signaling pathways from upstream inducible costimulatory molecules could be important keys to understanding how CAR-T cells produce cytokines at supraphysiologic levels.
Ultimately, the transduced CAR helps these engineered T cells to mediate a redirected response toward antigen positive tumor cells, which involves both granzyme-B and perforin for tumor lysis as well as generation of inflammatory cytokines and chemokines. The outcome of these signaling pathways in CAR T cells can provide effective anti-tumor cytotoxicity and enhance the production of inflammatory cytokines. Unique signaling pathways that exclusively regulate each of these functions are being defined.
T cells play a pivotal role in tumor immunosurveillance (58). Effector functions of T cells correlate with beneficial graft-vs.-tumor responses (59). However, they are also the primary producers of pro-inflammatory cytokines and chemokines (60). Inflammation is an essential component of effector T cell mediated immune responses. However, acute inflammation at enhanced levels is highly destructive.
Antibody-based CAR receptors possess 10 to 60-fold higher affinity than the average affinity of TCR. An intrinsic dissociation constant of an affinity matured antibody can exhibit a Kd 5 × 10−14 compared to a CD8+ T cell-derived TCR that has a Kd 10−7. While TCR-engineered T cells can also potently engage cancer targets and have been shown to cause significant toxicity, there appear to be interactive antigen differences between a CAR and a TCR (61–63). Accordingly, the potency of CAR T cells is increased significantly compared to native T cells. While the extraordinary affinity of the CAR to its cognate antigen is the basis for augmented tumor killing, it also causes significant toxicity through supraphysiologic stimulation and cytokine production (49, 64, 65).
While cytokine production can occur physiologically during severe infections and graft-vs.-host disease, CRS is a complex clinical phenomenon characterized by the high activation of immune cells and immense production of proinflammatory cytokines (11, 14–16, 65–68). Acute CRS generally begins hours to days after CAR T cell infusion. In some cases, late CRS has appeared in patients 1–4 weeks after infusion, at a time when there is a significant CAR T cell expansion (47). Patients with CRS experience symptoms associated with this elevated amount of proinflammatory cytokines, including IFN-γ, IL-2, TNF-α, MIP-1, and GM-CSF (10, 50, 69). In addition to cytokines that originate from the CAR T cells themselves, additional cytokines (including IL-6, IL-8, and IL-10) primarily generated by bystander cells and professional antigen presenting cells, are also significantly elevated during CRS (32, 50, 70). This accounts for a secondary wave of cytokine production, which can often be higher than what is directly produced from CAR-T cells (32, 47, 48). Notably, this secondary burst of cytokines is associated with other hyperactive immune disorders such as hemophagocytic lymphohistiocytosis (HLH) and macrophage activation syndrome (MAS), with inflammatory markers such as C Reactive protein and ferritin becoming elevated (32).
The role of macrophages and other myeloid cells in the development of CRS has been confirmed through not only clinical assays and patient samples, but also in animal models. Giavridis et al. established that activated CAR T cells can recruit and activate macrophages and other myeloid cells and are the main source of IL-6. Authors also confirmed the important role of IL-1 blockade in improving symptoms and suggested that its ability to cross the blood brain barrier made it an ideal pharmacological candidate compared to tocilizumab (71). Similarly, Norelli et al. also confirmed though their preclinical animal studies that IL-1 was critically important in the pathogenesis of CRS, and also suggested that Anakira be considered as part of CRS therapy (72).
CRS begins with mild symptoms, but it can quickly progress to life-threatening complications (13, 14, 26, 31, 35, 73–76). Multiple organ systems are involved in all phases. Fevers are an obligatory sign of CRS and typically precedes any other manifestation. Constitutionally, in addition to fevers, patients can develop rigors, nausea, and arthralgias. Hematologically, a picture consistent with disseminated intravascular coagulation can be seen, consisting of coagulopathy and hemorrhage. B cell aplasia is very common and a result of on-target-off-tumor effects. Gastrointestinal toxicities include colitis with diarrhea and abdominal pain and hepatitis. Cardiovascular side effects often prompt transfer to critical care settings, where tachycardia and hypotension can be supported with fluids and pressors. Other organ systems, including renal and pulmonary, can be affected by edema and third spacing, causing hypoxia and respiratory decompensation requiring intubation and ventilatory support. The neurological toxicities (CRES/ICANS) are some of the most puzzling and disturbing complications seen (77). While some patients can develop mild symptoms of headache and confusion, others have progressed (and in some cases rapidly) to seizures, cerebral edema, and death. Aphasia has also been identified as part of the neurotoxicity profile identified with CRES/ICANS. While some symptoms can occur during the CRS period, this has not been consistently seen, and CRES/ICANS can even occur days or weeks later (78). Table 1 highlights a summary of CAR-T trials with focus on CRS grade results (14, 17, 19, 20, 22, 42, 46, 79–82). The degree of CRS and CRES/ICANS have appeared to correlate in most cases with the use of preceding lymphodepletion, leukemia burden, and CAR-T cell dose, although it is still not completely clear why some patients do not develop any CRS and/or CRES/ICANS. Identifying biomarkers for use in CRS prediction algorithms is an emerging area of investigation to help guide patient risk, and if combined with patient characteristics, may become a helpful tool to determine CRS and CRES/ICANS risk (32).
Currently, immunosuppression is the primary therapeutic approach to treat life-threatening complications of CRS. With the concurrent development of distinct CAR-T constructs and clinical trials across different research groups, numerous CRS grading scales have developed over the years [Table 2; (31, 78, 83–86)]. While these scales are similar in the fact that they begin with mild CRS symptoms (grade I) and end in death (grade V), they differ in how they progress between the different grades. This makes it challenging to compare CRS results across multiple trials. New consensus workshops have identified a uniform method that will likely be adopted when considering future CRS reporting, although in the interim, it is important to look closely at the grading scale used when comparing the safety results across trials (85).
When identified early, CRS can be managed with supportive care ± anti-IL-6R mAb (tocilizumab) (13, 14, 87). Patients who respond to tocilizumab resolve CRS within a few hours to days; however, there are others who do not respond to this monoclonal antibody and require the administration of corticosteroids (31). With experience gained in identifying, classifying, and treating CRS early, outcomes have improved greatly with proactive supportive care and pre-emptive pharmacological support. Furthermore, tocilizumab was approved for treatment of CRS in August 2017, easing its ability to be accepted more uniformly as a standard of care treatment for CRS (88).
However, there are several concerns with using pharmacological agents to treat CRS. First, there is some concern that systemic immunosuppression caused by these drugs may diminish the efficacy of CAR-T cells. Furthermore, dampening the immune system may make patients who are already sick and compromised from lymphodepleting chemotherapy more prone to infections. Furthermore, with more studies focused on decreased persistence of CAR-T, it is unclear if dampening their response with immune suppressive therapies will ultimately affect their ability to persist in order to be a true “living” biological drug in patients (89). Finally, understanding the biochemistry of CRS brings to light that targeting the IL-6 receptor with tocilizumab does not always work for CRES, and by the time a direct Il-6 antagonist such as siltuximab is used, it may be too late in the process to have a substantial impact. Many have speculated that use of many of these agents, including anti IL-1, may be targeting cytokines that are further downstream from the instigating events that begin this cascade of inflammation. Thus, waiting for CRS/CRES to occur and using agents to neutralize downstream cytokines may not be the best strategy to consider. While some have advocated for pre-emptive treatment with agents such as tocilizumab to prevent severe CRS, this is still under investigation (90).
Perhaps most importantly, the outcome of CRS can be life-threatening, and there have been a number of patient deaths following treatment with CAR-T cells (16). Because current algorithms are waiting until CRS symptoms occur before treating, patients are being put at risk. Therefore, novel approaches are needed to manage and prevent CRS. Because CAR's are inherently engineered cellular products, designing a safer CAR could be one approach to consider.
With the advent of clustered regularly interspaced short palindromic repeats (CRISPR) technology, it is now possible to engineer a CAR-T that may have different and more specifically-controlled properties than virally-transduced cells. Specifically, it has been shown that targeting a CAR-coding sequence to the T cell receptor (TCR) locus may prevent accelerated T-cell exhaustion by decreasing tonic activation and TCR-induced autoimmunity. This decreased tonic activation may also decrease CRS (91). Another method that directs the engineered CAR to operate through the native TCR is a T-cell antigen coupler, or TAC (92). The TAC has three components, including an antigen-binding domain, a TCR-recruitment domain, and a co-receptor domain, for a more controlled design that can also decrease off-target toxicity. Another method that has been explored is the use of synthetic Notch receptors to induce T cell response in a customizable manner. Specifically, these receptors, when engaged to its cognate antigen, induce a transmembrane cleavage that releases the intracellular transcriptional domain to penetrate the nucleus and activate a synthetic response (93). While these constructs are using the newest technologies for cellular engineering, they are still in the early phases of development. Finally, another method to consider is to decipher the downstream signaling pathways that become activated once a CAR is engaged with its cognate ligand. By targeting the activation of these pathways that produce excessive cytokines but maintain cytotoxic potential, there is strong potential to regulate CRS (54, 66).
Our lab is focused on NK cell immunobiology, and NK cell intracellular signaling pathways are directly applicable to understanding T cell functionality (66). Our work has shown that adhesion and degranulation-promoting adapter protein (ADAP) serves as a positive regulator of proinflammatory cytokine production (54). The FynADAP complex exclusively regulates the production of inflammatory cytokines (94). Most importantly, lack of ADAP does not affect the NK cell-mediated anti-tumor cytotoxicity. These findings establish ADAP as a potential molecular target to reduce the production of inflammatory cytokine and chemokine production. This 130 kDa protein is expressed in multiple cell types including NK and T cells. It functions as a connecting link between the upstream Fyn and downstream signaling proteins Carma1 and TAK1. ADAP also interacts with SLP76 and SKAP55. The potential interaction sites of these signaling proteins in ADAP have been largely defined. Recently, as shown in Figure 1, we defined a Lck → Fyn → ADAP → CARMA1 → Bcl10 pathway that is obligatory for the production of inflammatory cytokines (54). Lack of ADAP in T or NK cells significantly reduces the production of IFN-γ, GM-CSF, TNF-α, MIP-1α, MIP-1β, and RANTES; however, the anti-tumor cytotoxicity was intact. In both T and NK cells, ADAP plays an essential role in immunoreceptor tyrosine-based activation motif-dependent receptor activation and is involved in activation integrins including LFA1. Loss of ADAP in T cells decreases their proliferation and cytokine production efficiency in response to limiting antigen doses (95).
Figure 1. Fyn activates divergent signaling cascades in effector lymphocytes. Signaling via the Lck→Fyn→ADAP→CARMA1→Bcl10 pathway that is obligatory for the production of inflammatory cytokines. Divergent signaling via the Lck→Fyn→PI(3)K pathway primarily facilitates cell-mediated cytotoxicity. Lack of ADAP significantly reduces pro-inflammatory cytokine production without affecting cell-mediated cytotoxicity in pre-clinical models.
These findings provide a feasible clinical approach to reduce the production of inflammatory cytokines and chemokines, and thereby the severity of CRS. Stimulation through TCR and CD28 utilizes ADAP to facilitate signaling downstream of the Carma1-Bcl10-Malt1 (CBM) complex, which leads to phosphorylation and degradation of IκBα and nuclear translocation of NF-κB (96). While the molecular mechanism whereby ADAP regulates the formation of the CBM has not been fully elucidated, the essential function of ADAP in linking CBM via Carma1 to PKC-θ is well-documented (97). NF-κB, which is sequestered in the cytosol through binding to IκBα, translocates into the nucleus (97). Carma1 plays an obligatory role in the nuclear translocation of NF-κB following activation of T or NK cells (98, 99). In addition to interactions with Carma1, ADAP also recruits TAK1, which facilitates the phosphorylation of IKKα and IKKβ, components of NF-κB signaling pathway. In T cells, ADAP contributes to CBM complex formation in response to ITAM-containing receptors (96, 97, 99–101). Thus, targeting ADAP in T cells could help to selectively attenuate cytokine production, without reducing cytotoxicity. The feasible approaches to target ADAP include CRISPR-CAS9-based deletion of ADAP in CAR-transduced T and NK cells, small molecule-based interference of interactions in the Fyn-ADAP-CBM pathway, and utilization of small hairpin interfering RNAs.
There are three major concerns with this approach that need to be addressed prior to blocking the interaction of Fyn and ADAP in clinical trials. (1) Cytokines such as IFN-γ are obligatory to clear certain types of malignancies. In general, cytokines and chemokines play a central role in orchestrating a productive anti-cancer response. Therefore, strategies to reduce the production of inflammatory cytokines should not completely curtail overall cytokine production, which would likely negatively impact anti-tumor cytotoxicity. (2) Cytokines are required for CAR-T homeostasis and likely survival and persistence. While curtailing cytokine production will not eliminate their production entirely, it is unclear to what degree of innate cytokine needs are necessary to maintain CAR-T perseverance. (3) As discussed earlier, cytokines responsible for CRS likely come from two sources. The primary source is the CAR T cells themselves that initiate the first wave of proinflammatory cytokine production, which this approach should help control. However, the secondary wave of cytokines, such as increased IL-6 production, originate from myeloid cells in response to augmented cytokine signaling from CAR T cells and native effector lymphocytes. In theory, reducing the levels of proinflammatory cytokines generated by CAR T cells should help to contain both the primary as well as indirectly, the secondary waves of cytokine productions.
CAR-T immunotherapy has changed the landscape of cancer treatment. However, CRS occurs in two-thirds of patients, and in its worst form can lead to death. While tocilizumab can be effective in treating CRS, once it occurs, CRS development may lead to increased morbidity, hospitalization, and cost, and may limit the dose of CAR T cells that can be used clinically. And while current algorithms have enhanced identification and treatment for CRS to decrease mortality, using these pharmacological interventions pre-emptively has not yet been established as a standard of care. Therefore, knowledge of the signaling pathways that uniquely regulate anti-tumor cytotoxicity and inflammation is critical in identifying potential novel targets for containing CRS. Our recent work evaluating the FynADAP pathway provides an archetypical model to validate blocking these unique signaling pathways to contain cytokine production as one method to engineer a safer CAR-T cells. Successful translation of this and other engineered strategies to reduce CRS in this intrinsic manner is a compelling approach to this important problem.
MT, TK, and SM wrote and edited this manuscript.
This work was supported in part by NIH R01 AI102893 (SM) and NCI R01 CA179363 (SM and MT); HRHM Program of MACC Fund/Children's Hospital of Wisconsin (SM), Nicholas Family Foundation (SM); Gardetto Family (SM); MCW-Cancer Center-Large Seed Grant (SM and MT); MACC Fund/Children's Hospital of Wisconsin (MT and SM); Ann's Hope Melanoma Foundation (SM and MT); and Advancing a Healthier Wisconsin (SM).
The authors declare that the research was conducted in the absence of any commercial or financial relationships that could be construed as a potential conflict of interest.
We thank Lucia Sammarco and her Lulu's Lemonade Stand for inspiration, motivation, and support. We thank Cassandra Longsine for help with manuscript preparation.
1. ^https://www.clinicaltrials.gov (cited July 10, 2019).
1. Teras LR, DeSantis CE, Cerhan JR, Morton LM, Jemal A, Flowers CR. 2016 US lymphoid malignancy statistics by World Health Organization subtypes. CA Cancer J Clin. (2016) 66:443–59. doi: 10.3322/caac.21357
2. Crump M, Neelapu SS, Farooq U, Van Den Neste E, Kuruvilla J, Westin J, et al. Outcomes in refractory diffuse large B-cell lymphoma: results from the international SCHOLAR-1 study. Blood. (2017) 130:1800–8. doi: 10.1182/blood-2017-03-769620
3. Gisselbrecht C, Schmitz N, Mounier N, Singh Gill D, Linch DC, Trneny M, et al. Rituximab maintenance therapy after autologous stem-cell transplantation in patients with relapsed CD20(+) diffuse large B-cell lymphoma: final analysis of the collaborative trial in relapsed aggressive lymphoma. J Clin Oncol. (2012) 30:4462–9. doi: 10.1200/JCO.2012.41.9416
4. Siegel DA, Henley SJ, Li J, Pollack LA, Van Dyne EA, White A. Rates and trends of pediatric acute lymphoblastic leukemia - United States, 2001-2014. MMWR Morb Mortal Wkly Rep. (2017) 66:950–4. doi: 10.15585/mmwr.mm6636a3
5. Oskarsson T, Soderhall S, Arvidson J, Forestier E, Montgomery S, Bottai M, et al. Relapsed childhood acute lymphoblastic leukemia in the Nordic countries: prognostic factors, treatment and outcome. Haematologica. (2016) 101:68–76. doi: 10.3324/haematol.2015.131680
6. Eckert C, Henze G, Seeger K, Hagedorn N, Mann G, Panzer-Grumayer R, et al. Use of allogeneic hematopoietic stem-cell transplantation based on minimal residual disease response improves outcomes for children with relapsed acute lymphoblastic leukemia in the intermediate-risk group. J Clin Oncol. (2013) 31:2736–42. doi: 10.1200/JCO.2012.48.5680
7. Nguyen K, Devidas M, Cheng SC, La M, Raetz EA, Carroll WL, et al. Factors influencing survival after relapse from acute lymphoblastic leukemia: a Children's Oncology Group study. Leukemia. (2008) 22:2142–50. doi: 10.1038/leu.2008.251
8. June CH, Sadelain M. Chimeric antigen receptor therapy. N Engl J Med. (2018) 379:64–73. doi: 10.1056/NEJMra1706169
9. Liu E, Tong Y, Dotti G, Shaim H, Savoldo B, Mukherjee M, et al. Cord blood NK cells engineered to express IL-15 and a CD19-targeted CAR show long-term persistence and potent antitumor activity. Leukemia. (2018) 32:520–31. doi: 10.1038/leu.2017.226
10. Brentjens RJ, Davila ML, Riviere I, Park J, Wang X, Cowell LG, et al. CD19-targeted T cells rapidly induce molecular remissions in adults with chemotherapy-refractory acute lymphoblastic leukemia. Sci Transl Med. (2013) 5:177ra38. doi: 10.1126/scitranslmed.3005930
11. da Silva AJ, Janssen O, Rudd CE. T cell receptor zeta/CD3-p59fyn(T)-associated p120/130 binds to the SH2 domain of p59fyn(T). J Exp Med. (1993) 178:2107–13. doi: 10.1084/jem.178.6.2107
12. Brudno JN, Kochenderfer JN. Toxicities of chimeric antigen receptor T cells: recognition and management. Blood. (2016) 127:3321–30. doi: 10.1182/blood-2016-04-703751
13. Maude SL, Barrett D, Teachey DT, Grupp SA. Managing cytokine release syndrome associated with novel T cell-engaging therapies. Cancer J. (2014) 20:119–22. doi: 10.1097/PPO.0000000000000035
14. Lee DW, Kochenderfer JN, Stetler-Stevenson M, Cui YK, Delbrook C, Feldman SA, et al. T cells expressing CD19 chimeric antigen receptors for acute lymphoblastic leukaemia in children and young adults: a phase 1 dose-escalation trial. Lancet. (2015) 385:517–28. doi: 10.1016/S0140-6736(14)61403-3
15. Gauthier J, Turtle CJ. Insights into cytokine release syndrome and neurotoxicity after CD19-specific CAR-T cell therapy. Curr Res Transl Med. (2018) 66:50–2. doi: 10.1016/j.retram.2018.03.003
16. Xu XJ, Tang YM. Cytokine release syndrome in cancer immunotherapy with chimeric antigen receptor engineered T cells. Cancer Lett. (2014) 343:172–8. doi: 10.1016/j.canlet.2013.10.004
17. Maude SL, Laetsch TW, Buechner J, Rives S, Boyer M, Bittencourt H, et al. Tisagenlecleucel in children and young adults with B-cell lymphoblastic leukemia. N Engl J Med. (2018) 378:439–48. doi: 10.1056/NEJMoa1709866
18. Park JH, Riviere I, Gonen M, Wang X, Senechal B, Curran KJ, et al. Long-term follow-up of CD19 CAR therapy in acute lymphoblastic leukemia. N Engl J Med. (2018) 378:449–59. doi: 10.1056/NEJMoa1709919
19. Schuster SJ, Svoboda J, Chong EA, Nasta SD, Mato AR, Anak O, et al. Chimeric antigen receptor T cells in refractory B-cell lymphomas. N Engl J Med. (2017) 377:2545–54. doi: 10.1056/NEJMoa1708566
20. Neelapu SS, Locke FL, Bartlett NL, Lekakis LJ, Miklos DB, Jacobson CA, et al. Axicabtagene ciloleucel CAR T-cell therapy in refractory large B-cell lymphoma. N Engl J Med. (2017) 377:2531–44. doi: 10.1056/NEJMoa1707447
21. Turtle CJ, Hay KA, Hanafi LA, Li D, Cherian S, Chen X, et al. Durable molecular remissions in chronic lymphocytic leukemia treated with CD19-specific chimeric antigen receptor-modified T cells after failure of ibrutinib. J Clin Oncol. (2017) 35:3010–20. doi: 10.1200/JCO.2017.72.8519
22. Gardner RA, Finney O, Annesley C, Brakke H, Summers C, Leger K, et al. Intent-to-treat leukemia remission by CD19 CAR T cells of defined formulation and dose in children and young adults. Blood. (2017) 129:3322–31. doi: 10.1182/blood-2017-02-769208
23. Garfall AL, Maus MV, Hwang WT, Lacey SF, Mahnke YD, Melenhorst JJ, et al. Chimeric antigen receptor T cells against CD19 for multiple myeloma. N Engl J Med. (2015) 373:1040–7. doi: 10.1056/NEJMoa1504542
24. Maude SL, Teachey DT, Porter DL, Grupp SA. CD19-targeted chimeric antigen receptor T-cell therapy for acute lymphoblastic leukemia. Blood. (2015) 125:4017–23. doi: 10.1182/blood-2014-12-580068
25. Cooley S, Trachtenberg E, Bergemann TL, Saeteurn K, Klein J, Le CT, et al. Donors with group B KIR haplotypes improve relapse-free survival after unrelated hematopoietic cell transplantation for acute myelogenous leukemia. Blood. (2009) 113:726–32. doi: 10.1182/blood-2008-07-171926
26. Davila ML, Riviere I, Wang X, Bartido S, Park J, Curran K, et al. Efficacy and toxicity management of 19-28z CAR T cell therapy in B cell acute lymphoblastic leukemia. Sci Transl Med. (2014) 6:224ra25. doi: 10.1126/scitranslmed.3008226
27. Sadelain M, Brentjens R, Riviere I, Park J. CD19 CAR Therapy for acute lymphoblastic leukemia. Am Soc Clin Oncol Educ Book. (2015) 35:e360–3. doi: 10.14694/EdBook_AM.2015.35.e360
28. Suntharalingam G, Perry MR, Ward S, Brett SJ, Castello-Cortes A, Brunner MD, et al. Cytokine storm in a phase 1 trial of the anti-CD28 monoclonal antibody TGN1412. N Engl J Med. (2006) 355:1018–28. doi: 10.1056/NEJMoa063842
29. Topp MS, Gokbuget N, Stein AS, Zugmaier G, O'Brien S, Bargou RC, et al. Safety and activity of blinatumomab for adult patients with relapsed or refractory B-precursor acute lymphoblastic leukaemia: a multicentre, single-arm, phase 2 study. Lancet Oncol. (2015) 16:57–66. doi: 10.1016/S1470-2045(14)71170-2
30. Shimabukuro-Vornhagen A, Godel P, Subklewe M, Stemmler HJ, Schlosser HA, Schlaak M, et al. Cytokine release syndrome. J Immunother Cancer. (2018) 6:56. doi: 10.1186/s40425-018-0343-9
31. Lee DW, Gardner R, Porter DL, Louis CU, Ahmed N, Jensen M, et al. Current concepts in the diagnosis and management of cytokine release syndrome. Blood. (2014) 124:188–95. doi: 10.1182/blood-2014-05-552729
32. Teachey DT, Lacey SF, Shaw PA, Melenhorst JJ, Maude SL, Frey N, et al. Identification of predictive biomarkers for cytokine release syndrome after chimeric antigen receptor T-cell therapy for acute lymphoblastic leukemia. Cancer Discov. (2016) 6:664–79. doi: 10.1158/2159-8290.CD-16-0040
33. Fitzgerald JC, Weiss SL, Maude SL, Barrett DM, Lacey SF, Melenhorst JJ, et al. Cytokine release syndrome after chimeric antigen receptor T cell therapy for acute lymphoblastic leukemia. Crit Care Med. (2017) 45:e124–31. doi: 10.1097/CCM.0000000000002053
34. Porter DL, Levine BL, Kalos M, Bagg A, June CH. Chimeric antigen receptor-modified T cells in chronic lymphoid leukemia. N Engl J Med. (2011) 365:725–33. doi: 10.1056/NEJMoa1103849
35. Grupp SA, Kalos M, Barrett D, Aplenc R, Porter DL, Rheingol‘d SR, et al. Chimeric antigen receptor-modified T cells for acute lymphoid leukemia. N Engl J Med. (2013) 368:1509–18. doi: 10.1056/NEJMoa1215134
36. Romanski A, Uherek C, Bug G, Seifried E, Klingemann H, Wels WS, et al. CD19-CAR engineered NK-92 cells are sufficient to overcome NK cell resistance in B-cell malignancies. J Cell Mol Med. (2016) 20:1287–94. doi: 10.1111/jcmm.12810
37. June CH, Maus MV, Plesa G, Johnson LA, Zhao Y, Levine BL, et al. Engineered T cells for cancer therapy. Cancer Immunol Immunother. (2014) 63:969–75. doi: 10.1007/s00262-014-1568-1
38. Maus MV, Grupp SA, Porter DL, June CH. Antibody-modified T cells: CARs take the front seat for hematologic malignancies. Blood. (2014) 123:2625–35. doi: 10.1182/blood-2013-11-492231
39. Dotti G, Gottschalk S, Savoldo B, Brenner MK. Design and development of therapies using chimeric antigen receptor-expressing T cells. Immunol Rev. (2014) 257:107–26. doi: 10.1111/imr.12131
40. Carpenter RO, Evbuomwan MO, Pittaluga S, Rose JJ, Raffeld M, Yang S, et al. B-cell maturation antigen is a promising target for adoptive T-cell therapy of multiple myeloma. Clin Cancer Res. (2013) 19:2048–60. doi: 10.1158/1078-0432.CCR-12-2422
41. Berger C, Sommermeyer D, Hudecek M, Berger M, Balakrishnan A, Paszkiewicz PJ, et al. Safety of targeting ROR1 in primates with chimeric antigen receptor-modified T cells. Cancer Immunol Res. (2015) 3:206–16. doi: 10.1158/2326-6066.CIR-14-0163
42. Fry TJ, Shah NN, Orentas RJ, Stetler-Stevenson M, Yuan CM, Ramakrishna S, et al. CD22-targeted CAR T cells induce remission in B-ALL that is naive or resistant to CD19-targeted CAR immunotherapy. Nat Med. (2018) 24:20–8. doi: 10.1038/nm.4441
43. Heczey A, Louis CU, Savoldo B, Dakhova O, Durett A, Grilley B, et al. CAR T Cells administered in combination with lymphodepletion and PD-1 inhibition to patients with neuroblastoma. Mol Ther. (2017) 25:2214–24. doi: 10.1016/j.ymthe.2017.05.012
44. Migliorini D, Dietrich PY, Stupp R, Linette GP, Posey AD Jr, June CH. CAR T-Cell therapies in glioblastoma: a first look. Clin Cancer Res. (2018) 24:535–40. doi: 10.1158/1078-0432.CCR-17-2871
45. Ahmed N, Brawley VS, Hegde M, Robertson C, Ghazi A, Gerken C, et al. Human Epidermal Growth Factor Receptor 2 (HER2) -specific chimeric antigen receptor-modified T cells for the immunotherapy of HER2-positive sarcoma. J Clin Oncol. (2015) 33:1688–96. doi: 10.1200/JCO.2014.58.0225
46. Ramos CA, Ballard B, Zhang H, Dakhova O, Gee AP, Mei Z, et al. Clinical and immunological responses after CD30-specific chimeric antigen receptor-redirected lymphocytes. J Clin Invest. (2017) 127:3462–71. doi: 10.1172/JCI94306
47. Hay KA, Hanafi LA, Li D, Gust J, Liles WC, Wurfel MM, et al. Kinetics and biomarkers of severe cytokine release syndrome after CD19 chimeric antigen receptor-modified T-cell therapy. Blood. (2017) 130:2295–306. doi: 10.1182/blood-2017-06-793141
48. Matthys P, Dillen C, Proost P, Heremans H, Van Damme J, Billiau A. Modification of the anti-CD3-induced cytokine release syndrome by anti-interferon-gamma or anti-interleukin-6 antibody treatment: protective effects and biphasic changes in blood cytokine levels. Eur J Immunol. (1993) 23:2209–16. doi: 10.1002/eji.1830230924
49. Kochenderfer JN, Dudley ME, Feldman SA, Wilson WH, Spaner DE, Maric I, et al. B-cell depletion and remissions of malignancy along with cytokine-associated toxicity in a clinical trial of anti-CD19 chimeric-antigen-receptor-transduced T cells. Blood. (2012) 119:2709–20. doi: 10.1182/blood-2011-10-384388
50. Kalos M, Levine BL, Porter DL, Katz S, Grupp SA, Bagg A, et al. T cells with chimeric antigen receptors have potent antitumor effects and can establish memory in patients with advanced leukemia. Sci Transl Med. (2011) 3:95ra73. doi: 10.1126/scitranslmed.3002842
51. Porter DL, Kalos M, Zheng Z, Levine B, June C. Chimeric antigen receptor therapy for B-cell malignancies. J Cancer. (2011) 2:331–2. doi: 10.7150/jca.2.331
52. Chuck MI, Zhu M, Shen S, Zhang W. The role of the LAT-PLC-gamma1 interaction in T regulatory cell function. J Immunol. (2010) 184:2476–86. doi: 10.4049/jimmunol.0902876
53. Savoldo B, Ramos CA, Liu E, Mims MP, Keating MJ, Carrum G, et al. CD28 costimulation improves expansion and persistence of chimeric antigen receptor-modified T cells in lymphoma patients. J Clin Invest. (2011) 121:1822–6. doi: 10.1172/JCI46110
54. Rajasekaran K, Kumar P, Schuldt KM, Peterson EJ, Vanhaesebroeck B, Dixit V, et al. Signaling by Fyn-ADAP via the Carma1-Bcl-10-MAP3K7 signalosome exclusively regulates inflammatory cytokine production in NK cells. Nat Immunol. (2013) 14:1127–36. doi: 10.1038/ni.2708
55. Zhong XS, Matsushita M, Plotkin J, Riviere I, Sadelain M. Chimeric antigen receptors combining 4-1BB and CD28 signaling domains augment PI3kinase/AKT/Bcl-XL activation and CD8+ T cell-mediated tumor eradication. Mol Ther. (2010) 18:413–20. doi: 10.1038/mt.2009.210
56. Riha P, Rudd CE. CD28 co-signaling in the adaptive immune response. Self Nonself. (2010) 1:231–40. doi: 10.4161/self.1.3.12968
57. Dobbins J, Gagnon E, Godec J, Pyrdol J, Vignali DA, Sharpe AH, et al. Binding of the cytoplasmic domain of CD28 to the plasma membrane inhibits Lck recruitment and signaling. Sci Signal. (2016) 9:ra75. doi: 10.1126/scisignal.aaf0626
58. Rao S, Gharib K, Han A. Cancer immunosurveillance by T cells. Int Rev Cell Mol Biol. (2019) 342:149–73. doi: 10.1016/bs.ircmb.2018.08.001
59. Kolb HJ. Graft-versus-leukemia effects of transplantation and donor lymphocytes. Blood. (2008) 112:4371–83. doi: 10.1182/blood-2008-03-077974
60. Raphael I, Nalawade S, Eagar TN, Forsthuber TG. T cell subsets and their signature cytokines in autoimmune and inflammatory diseases. Cytokine. (2015) 74:5–17. doi: 10.1016/j.cyto.2014.09.011
61. Foote J, Eisen HN. Kinetic and affinity limits on antibodies produced during immune responses. Proc Natl Acad Sci USA. (1995) 92:1254–6. doi: 10.1073/pnas.92.5.1254
62. Foote J, Eisen HN. Breaking the affinity ceiling for antibodies and T cell receptors. Proc Natl Acad Sci USA. (2000) 97:10679–81. doi: 10.1073/pnas.97.20.10679
63. Morgan RA, Chinnasamy N, Abate-Daga D, Gros A, Robbins PF, Zheng Z, et al. Cancer regression and neurological toxicity following anti-MAGE-A3 TCR gene therapy. J Immunother. (2013) 36:133–51. doi: 10.1097/CJI.0b013e3182829903
64. Brentjens R, Yeh R, Bernal Y, Riviere I, Sadelain M. Treatment of chronic lymphocytic leukemia with genetically targeted autologous T cells: case report of an unforeseen adverse event in a phase I clinical trial. Mol Ther. (2010) 18:666–8. doi: 10.1038/mt.2010.31
65. Morgan RA, Yang JC, Kitano M, Dudley ME, Laurencot CM, Rosenberg SA. Case report of a serious adverse event following the administration of T cells transduced with a chimeric antigen receptor recognizing ERBB2. Mol Ther. (2010) 18:843–51. doi: 10.1038/mt.2010.24
66. Gerbec ZJ, Thakar MS, Malarkannan S. The Fyn-ADAP axis: cytotoxicity versus cytokine production in killer cells. Front Immunol. (2015) 6:472. doi: 10.3389/fimmu.2015.00472
67. da Silva AJ, Yamamoto M, Zalvan CH, Rudd CE. Engagement of the TcR/CD3 complex stimulates p59fyn(T) activity: detection of associated proteins at 72 and 120-130 kD. Mol Immunol. (1992) 29:1417–25. doi: 10.1016/0161-5890(92)90215-J
68. Peterson EJ, Woods ML, Dmowski SA, Derimanov G, Jordan MS, Wu JN, et al. Coupling of the TCR to integrin activation by Slap-130/Fyb. Science. (2001) 293:2263–5. doi: 10.1126/science.1063486
69. Geng L, Raab M, Rudd CE. Cutting edge: SLP-76 cooperativity with FYB/FYN-T in the Up-regulation of TCR-driven IL-2 transcription requires SLP-76 binding to FYB at Tyr595 and Tyr651. J Immunol. (1999) 163:5753–7.
70. da Silva AJ, Raab M, Li Z, Rudd CE. TcR zeta/CD3 signal transduction in T-cells: downstream signalling via ZAP-70, SLP-76 and FYB. Biochem Soc Trans. (1997) 25:361–6. doi: 10.1042/bst0250361
71. Giavridis T, van der Stegen SJC, Eyquem J, Hamieh M, Piersigilli A, Sadelain M. CAR T cell-induced cytokine release syndrome is mediated by macrophages and abated by IL-1 blockade. Nat Med. (2018) 24:731–8. doi: 10.1038/s41591-018-0041-7
72. Norelli M, Camisa B, Barbiera G, Falcone L, Purevdorj A, Genua M, et al. Monocyte-derived IL-1 and IL-6 are differentially required for cytokine-release syndrome and neurotoxicity due to CAR T cells. Nat Med. (2018) 24:739–48. doi: 10.1038/s41591-018-0036-4
73. Maude SL, Frey N, Shaw PA, Aplenc R, Barrett DM, Bunin NJ, et al. Chimeric antigen receptor T cells for sustained remissions in leukemia. N Engl J Med. (2014) 371:1507–17. doi: 10.1056/NEJMoa1407222
74. Kochenderfer JN, Dudley ME, Carpenter RO, Kassim SH, Rose JJ, Telford WG, et al. Donor-derived CD19-targeted T cells cause regression of malignancy persisting after allogeneic hematopoietic stem cell transplantation. Blood. (2013) 122:4129–39. doi: 10.1182/blood-2013-08-519413
75. Brudno JN, Somerville RP, Shi V, Rose JJ, Halverson DC, Fowler DH, et al. Allogeneic T cells that express an anti-CD19 chimeric antigen receptor induce remissions of B-cell malignancies that progress after allogeneic hematopoietic stem-cell transplantation without causing graft-versus-host disease. J Clin Oncol. (2016) 34:1112–21. doi: 10.1200/JCO.2015.64.5929
76. Kochenderfer JN, Dudley ME, Kassim SH, Somerville RP, Carpenter RO, Stetler-Stevenson M, et al. Chemotherapy-refractory diffuse large B-cell lymphoma and indolent B-cell malignancies can be effectively treated with autologous T cells expressing an anti-CD19 chimeric antigen receptor. J Clin Oncol. (2015) 33:540–9. doi: 10.1200/JCO.2014.56.2025
77. Gust J, Hay KA, Hanafi L-A, Li D, Myerson D, Gonzalez-Cuyar LF, et al. Endothelial activation and blood–brain barrier disruption in neurotoxicity after adoptive immunotherapy with CD19 CAR-T Cells. J Cancer Discovery. (2017) 7:1404–19. doi: 10.1158/2159-8290.CD-17-0698
78. Neelapu SS, Tummala S, Kebriaei P, Wierda W, Gutierrez C, Locke FL, et al. Chimeric antigen receptor T-cell therapy — assessment and management of toxicities. Nat Rev Clin Oncol. (2018) 15:47. doi: 10.1038/nrclinonc.2017.148
79. O'Rourke DM, Nasrallah MP, Desai A, Melenhorst JJ, Mansfield K, Morrissette JJD, et al. A single dose of peripherally infused EGFRvIII-directed CAR T cells mediates antigen loss and induces adaptive resistance in patients with recurrent glioblastoma. Sci Transl Med. (2017) 9:eaaa0984. doi: 10.1126/scitranslmed.aaa0984
80. Bishop MR, Maziarz RT, Waller EK, Jager U, Westin JR, McGuirk JP, et al. Tisagenlecleucel in relapsed/refractory diffuse large B-cell lymphoma patients without measurable disease at infusion. Blood Adv. (2019) 3:2230–6. doi: 10.1182/bloodadvances.2019000151
81. Cao Y, Lu W, Sun R, Jin X, Cheng L, He X, et al. Anti-CD19 chimeric antigen receptor T cells in combination with nivolumab are safe and effective against relapsed/refractory B-cell non-hodgkin lymphoma. Front Oncol. (2019) 9:767. doi: 10.3389/fonc.2019.00767
82. Zhao WH, Liu J, Wang BY, Chen YX, Cao XM, Yang Y, et al. A phase 1, open-label study of LCAR-B38M, a chimeric antigen receptor T cell therapy directed against B cell maturation antigen, in patients with relapsed or refractory multiple myeloma. J Hematol Oncol. (2018) 11:141. doi: 10.1186/s13045-018-0681-6
83. Park JH, Romero FA, Taur Y, Sadelain M, Brentjens RJ, Hohl TM, et al. Cytokine release syndrome grade as a predictive marker for infections in patients with relapsed or refractory B-cell acute lymphoblastic leukemia treated with chimeric antigen receptor T cells. Clin Infect Dis. (2018) 67:533–40. doi: 10.1093/cid/ciy152
84. Porter D, Frey N, Wood PA, Weng Y, Grupp SA. Grading of cytokine release syndrome associated with the CAR T cell therapy tisagenlecleucel. J Hematol Oncol. (2018) 11:35. doi: 10.1186/s13045-018-0571-y
85. Lee DW, Santomasso BD, Locke FL, Ghobadi A, Turtle CJ, Brudno JN, et al. ASTCT consensus grading for cytokine release syndrome and neurologic toxicity associated with immune effector cells. Biol Blood Marrow Transplant. (2019) 25:625–38. doi: 10.1016/j.bbmt.2018.12.758
86. Common Terminology Criteria for Adverse Events (CTCAE). Services DoHaH, editor. v5.0 ed (2017), p. 1–155. Available online at: https://ctep.cancer.gov/protocoldevelopment/electronic_applications/docs/CTCAE_v5_Quick_Reference_8.5x11.pdf (accessed October 07, 2019).
87. Wu JN, Gheith S, Bezman NA, Liu QH, Fostel LV, Swanson AM, et al. Adhesion- and degranulation-promoting adapter protein is required for efficient thymocyte development and selection. J Immunol. (2006) 176:6681–9. doi: 10.4049/jimmunol.176.11.6681
88. Le RQ, Li L, Yuan W, Shord SS, Nie L, Habtemariam BA, et al. FDA approval summary: tocilizumab for treatment of chimeric antigen receptor T cell-induced severe or life-threatening cytokine release syndrome. Oncologist. (2018) 23:943–7. doi: 10.1634/theoncologist.2018-0028
89. Yang Y, Kohler ME, Fry TJ. Effect of chronic endogenous antigen stimulation on CAR T cell persistence and memory formation. Blood. (2017) 130(Suppl. 1):166. doi: 10.1182/blood.V130.Suppl_1.166.166
90. Kadauke S, Maude S, Gladney W, Motley L, Shenoy V, Callahan C, et al. Early administration of tocilizumab (Toci) for the prevention of grade 4 cytokine release syndrome (CRS) after CD19-directed CAR T-cell therapy (CTL019). Cytotherapy. (2019) 21(Suppl. 5):e2–3. doi: 10.1016/j.jcyt.2019.04.009
91. Eyquem J, Mansilla-Soto J, Giavridis T, van der Stegen SJ, Hamieh M, Cunanan KM, et al. Targeting a CAR to the TRAC locus with CRISPR/Cas9 enhances tumour rejection. Nature. (2017) 543:113–7. doi: 10.1038/nature21405
92. Helsen CW, Hammill JA, Lau VWC, Mwawasi KA, Afsahi A, Bezverbnaya K, et al. The chimeric TAC receptor co-opts the T cell receptor yielding robust anti-tumor activity without toxicity. Nat Commun. (2018) 9:3049. doi: 10.1038/s41467-018-05395-y
93. Roybal KT, Williams JZ, Morsut L, Rupp LJ, Kolinko I, Choe JH, et al. Engineering T cells with customized therapeutic response programs using synthetic notch receptors. Cell. (2016) 167:419–32.e16. doi: 10.1016/j.cell.2016.09.011
94. Mueller KL, Thomas MS, Burbach BJ, Peterson EJ, Shimizu Y. Adhesion and degranulation-promoting adapter protein (ADAP) positively regulates T cell sensitivity to antigen and T cell survival. J Immunol. (2007) 179:3559–69. doi: 10.4049/jimmunol.179.6.3559
95. May RM, Okumura M, Hsu CJ, Bassiri H, Yang E, Rak G, et al. Murine natural killer immunoreceptors use distinct proximal signaling complexes to direct cell function. Blood. (2013) 121:3135–46. doi: 10.1182/blood-2012-12-474361
96. Srivastava R, Burbach BJ, Shimizu Y. NF-κB activation in T cells requires discrete control of IκB kinase alpha/beta (IKKα/β) phosphorylation and IKKγ ubiquitination by the ADAP adapter protein. J Biol Chem. (2010) 285:11100–5. doi: 10.1074/jbc.M109.068999
97. Medeiros RB, Burbach BJ, Mueller KL, Srivastava R, Moon JJ, Highfill S, et al. Regulation of NF-κB activation in T cells via association of the adapter proteins ADAP and CARMA1. Science. (2007) 316:754–8. doi: 10.1126/science.1137895
98. Gross O, Grupp C, Steinberg C, Zimmermann S, Strasser D, Hannesschlager N, et al. Multiple ITAM-coupled NK-cell receptors engage the Bcl10/Malt1 complex via Carma1 for NF-κB and MAPK activation to selectively control cytokine production. Blood. (2008) 112:2421–8. doi: 10.1182/blood-2007-11-123513
99. Rajasekaran K, Chu H, Kumar P, Xiao Y, Tinguely M, Samarakoon A, et al. Transforming growth factor-β-activated kinase 1 regulates natural killer cell-mediated cytotoxicity and cytokine production. J Biol Chem. (2011) 286:31213–24. doi: 10.1074/jbc.M111.261917
100. Burbach BJ, Srivastava R, Medeiros RB, O'Gorman WE, Peterson EJ, Shimizu Y. Distinct regulation of integrin-dependent T cell conjugate formation and NF-κ B activation by the adapter protein ADAP. J Immunol. (2008) 181:4840–51. doi: 10.4049/jimmunol.181.7.4840
Keywords: cytokine release syndrome, chimeric antigen receptor, T cells, NK cells, Fyn-ADAP
Citation: Thakar MS, Kearl TJ and Malarkannan S (2020) Controlling Cytokine Release Syndrome to Harness the Full Potential of CAR-Based Cellular Therapy. Front. Oncol. 9:1529. doi: 10.3389/fonc.2019.01529
Received: 02 May 2019; Accepted: 18 December 2019;
Published: 31 January 2020.
Edited by:
Jason Roszik, University of Texas MD Anderson Cancer Center, United StatesReviewed by:
Haidong Dong, Mayo Clinic College of Medicine and Science, United StatesCopyright © 2020 Thakar, Kearl and Malarkannan. This is an open-access article distributed under the terms of the Creative Commons Attribution License (CC BY). The use, distribution or reproduction in other forums is permitted, provided the original author(s) and the copyright owner(s) are credited and that the original publication in this journal is cited, in accordance with accepted academic practice. No use, distribution or reproduction is permitted which does not comply with these terms.
*Correspondence: Monica S. Thakar, bXN0aGFrYXJAZnJlZGh1dGNoLm9yZw==; Subramaniam Malarkannan, c21hbGFya2FubmFuQHZlcnNpdGkub3Jn
Disclaimer: All claims expressed in this article are solely those of the authors and do not necessarily represent those of their affiliated organizations, or those of the publisher, the editors and the reviewers. Any product that may be evaluated in this article or claim that may be made by its manufacturer is not guaranteed or endorsed by the publisher.
Research integrity at Frontiers
Learn more about the work of our research integrity team to safeguard the quality of each article we publish.