- 1Wuxi School of Medicine, Jiangnan University, Wuxi, China
- 2School of Life Sciences and Biotechnology, Shanghai Jiao Tong University, Shanghai, China
- 3Henan Key Laboratory of Tumor Epidemiology, Henan Institute of Medical and Pharmaceutical Sciences, Zhengzhou University, Zhengzhou, China
Alterations and personal variations of RNA interactions have been mechanistically coupled with disease etiology and phenotypical variations. RNA biomarkers, RNA mimics, and RNA antagonists have been developed for diagnostic, prognostic, and therapeutic uses. Long non-coding RNAs (lncRNAs) and microRNAs (miRNAs) are two major types of RNA molecules with regulatory roles, deregulation of which has been implicated in the initiation and progression of many human malignancies. Accumulating evidence indicated the clinical roles of regulatory RNAs in cancer control, stimulating a surge in exploring the functionalities of regulatory RNAs for improved understanding on disease pathogenesis and management. In this review, we highlight the critical roles of lncRNAs and miRNAs played in tumorigenesis, scrutinize their potential functionalities as diagnostic/prognostic biomarkers and/or therapeutic targets in clinics, outline opportunities that ncRNAs may bring to complement current clinical practice for improved cancer management and identify challenges faced by translating frontier knowledge on non-coding RNAs (ncRNAs) to bedside clinics as well as possible solutions.
Introduction
Non-coding RNAs (ncRNAs) are RNA molecules with no protein translation potential. The conception that ncRNAs are “junk RNAs” of the transcriptome has been subverted by the fact that an essential part of the genome is transcribed into RNAs without protein products, with the rapid development of high-throughput technologies (1, 2). NcRNAs can be classified as housekeeping ncRNAs for the maintenance of normal cell functionalities, and regulatory ncRNAs. House keeping ncRNAs include, e.g., snoRNAs (small nucleolar RNAs), snRNAs (small nuclear RNAs), gRNA (guide RNAs), RNaseP RNAs that take part in processing transcriptional products, and tRNAs (transfer RNAs), rRNAs (ribosome RNAs), and tmRNAs (transfer messenger RNAs) that are involved in protein translation, as well as telomere related RNAs and SRP RNAs (signal recognition particle RNAs). A diverse reservoir of ncRNAs with regulatory roles on gene expression or cellular events has been revealed including, e.g., long ncRNAs (lncRNAs), microRNAs (miRNAs), piwi interaction RNAs (piRNAs), and circular RNAs (circRNAs). This review focuses on regulatory ncRNAs, whose indispensible roles on maintaining the special-temporal architecture of transcriptional and translational programs under healthy and malignant states have been gaining incremental attentions.
NcRNAs modulate gene expression through various mechanistic programs. NcRNA-mediated gene silencing constitutes one important type of epigenetic alterations and has been implicated in several human carcinogenesis (3). An increasing number of studies have uncovered associations between ncRNAs and cancer predisposition or status during the past decade (4), opening a new paradigm for cancer control taking advantages of regulatory RNAs. Among the ever-increasing types of ncRNAs being deciphered, lncRNAs and miRNAs are the most intensively studied (5). In particular, lncRNAs, frequently found deregulated in various types of cancers, represent a novel goldmine for biomarker discovery as well as therapeutic applications (6–9); miRNAs have been subsequently identified dysregulated in almost all types of cancers and proposed for diagnosis and therapeutics ever since the discovery of the loss-of-function phenotypes conveyed by the miRNA let-7 (10). For instance, lncRNA LINC00261 was reported to suppress cell proliferation and invasion in human choriocarcinoma (11), and miR-21 was implicated as an oncogenic factor regulating cancer cell proliferation, migration and apoptosis in many diseases including cancers (12, 13).
This review will critically assess the features and functionalities of ncRNAs with a focus on lncRNAs and miRNAs, identify their current applications and potential in cancer management including diagnostics, prognosis and therapeutics, proposes opportunities that ncRNAs may bring to complement the current diagnostic/therapeutic modalities for improved cancer control, and discuss challenges faced by bringing ncRNAs from academic frontier to the bedside in clinics as well as the potential solutions (Figure 1).
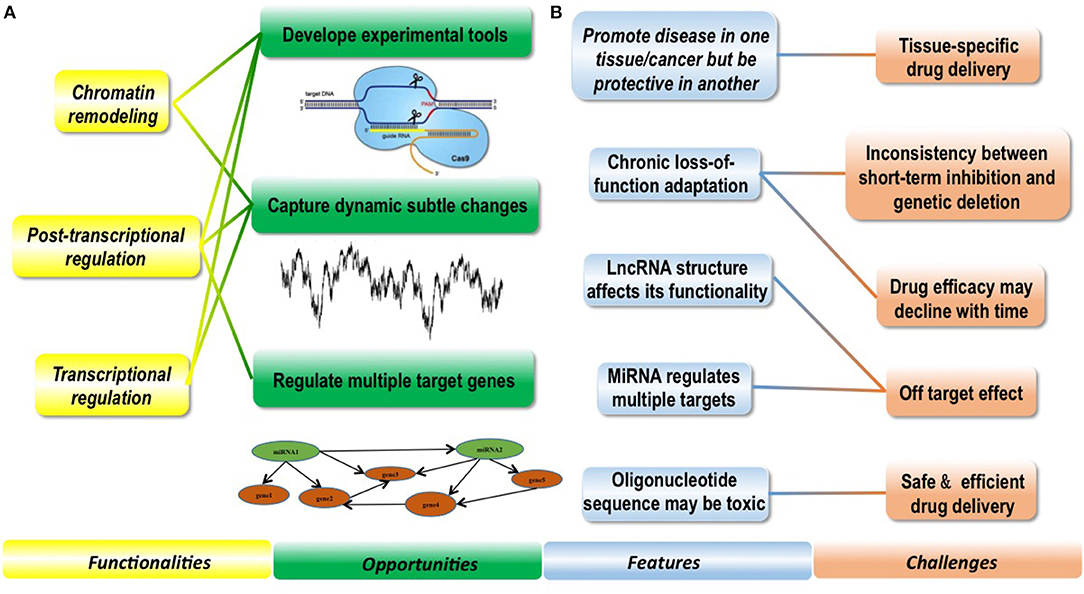
Figure 1. Conceptual scheme illustrating the features and functionalities of regulatory ncRNAs as well as opportunities and challenges they bring to clinics. (A) Functionalities of ncRNAs enable them with clinical potential and bring novel opportunities to clinics. Regulatory ncRNAs can function in chromatin remodeling, post-transcriptional regulation and transcriptional regulation. Based on these functionalities, experimental tools such as the CRISPR technique has been established to avail both research and clinics; besides, ncRNAs can capture dynamic subtle cellular changes pathological stimuli that are difficult to be precisely monitored using DNAs or proteins, and targeting miRNAs can effectively regulate many downstream target genes which is difficult to achieve using conventional strategies. (B) Several features of ncRNAs make clinical translation of ncRNAs challenging. NcRNAs are featured by promoting disease in one tissue but being protective in another, therefore, enabling tissue-specific drug delivery is of crucial importance when targeting ncRNAs in clinics. NcRNAs may be subjected to chronic loss-of-function adaptation, leading to inconsistencies observed between short-term inhibition and genetic deletion in some cases, and how to prevent drug efficacy decline with time imposes another big challenge. LncRNAs take advantages of both sequence matching and secondary and/or tertiary structures to take actions, and miRNAs can regulate multiple targets simultaneously, rendering it more important and complicated to prevent off-target effect. Oligonucleotide sequence may be toxic, which makes the safety issue more significant on drug delivery.
Long Non-coding RNAs
Features of lncRNAs
LncRNAs are, in general, >200 nts, and vary from 1,000 to 10,000 nts, some of which are upto 1,00,000 nts in length (14, 15). LncRNAs were firstly recognized in mice when people were attempting to sequence the full-length cDNAs (16). Ever since 2007 when HOTAIR, a 2.2 kilobase functional lncRNA, was identified to participate in several processes of epigenetic regulations (17), the mystery on the existence and prevalence of lncRNA that constitute genome redundancy was revealed, and a new regime of exploring the regulatory roles of lncRNAs was opened. With the development of transcriptomics, lots of lncRNAs were identified in the past decade as important functional products of the genome (15).
LncRNAs are highly diverse, with little shared feature regarding the localization, structure, mechanistics or mode of action across the entire mammalian lncRNA regime. However, lncRNAs are featured by low GC content, lack of introns and start codons (18). While some lncRNAs perfectly match Watson-Crick base-pairing, some employ imperfect pairing, and Watson-Crick and non-Watson-Crick base pairs are interspersed (14). These sequence traits enable them with some biological features such as nucleus positioning and low transcription activity (19). The secondary and tertiary structures of lncRNA play vital roles for them to take on any action modes such as protein recognition, catalysis and metabolite sensing (14, 20), where UV crosslinking and computational modeling have been used to characterize their functionalities in the 3D space (21–23).
Diverse approaches have been established to classify lncRNAs according to different features. LncRNAs can be categorized into 5 classes, i.e., sense lncRNA, antisense lncRNA, intronic lncRNA, intergenic lncRNA, and bidirectional lncRNA, based on their genomic locations and relative positions to protein-coding genes. Sense and antisense lncRNAs are, by their names, transcribed from the sense and antisense strand, respectively; while intronic lncRNAs are lncRNAs entirely transcribed from the introns of protein-coding genes, intergenic lncRNAs are transcribed within genomic intervals of neighboring protein-coding genes; lncRNAs are considered bidirectional if they were transcribed concomitantly with an adjacent protein-coding gene on the same strand (24). Based on the functionalities of lncRNAs exerted on DNA sequences, they can be categorized into cis- and trans-lncRNAs. While cis-lncRNAs regulate the expression of neighboring genes, trans-lncRNAs modulate those of the remote genes (25). Headway will be made toward more comprehensive understandings on the functionalities and mechanisms of lncRNAs with the advancement of high-throughput technologies that, ultimately, lead to more reasonable classifications of lncRNAs.
Functionalities of lncRNAs
Large number of lncRNAs have been recognized with the rapid advancement of experimental and computational technologies, with a small fraction of them being functionally annotated and even fewer been proved with in vivo functionalities. Through forming a RNA:DNA:DNA triplex, RNA:DNA hybrid (R-loop), RNA:RNA hybrid with a nascent transcript, or binding to a sequence-specific DNA binding protein, lncRNAs can function as decoys, scaffolds, and guides to interfere with and participate in various transcriptional and post-transcriptional programs (15, 26–28) (Figure 2). Functioning as decoys that block the access of other regulatory RNAs or proteins to the targeted DNA is the firstly identified mechanism of lncRNAs. For example, lncRNA AK015322 functions as a decoy for miR-19b-3p to promote the proliferation of spermatogonial stem cells C18-4 (29); and lncRNA Gas releases the glucocorticoid receptor from DNA as a decoy to prevent transcription of metabolic genes under starvation conditions (30) (Table 1). LncRNAs can also serve as scaffolds to bring regulatory elements including proteins and RNAs together for collaborative functionalities including gene expression enhancement. For instance, lncRNA GClnc1 rewires the histone modification pattern that ultimately contributes to gastric carcinogenesis through performing as a modular scaffold of the WDR5/KAT2A complex (58); the lncRNA KHPS1 re-activates a poised enhancer of the proto-oncogene SPHK1 via RNA-DNA-DNA triplex-dependent recruitment of epigenomic regulators E2F1 and p300 (59); and the lncRNA Evf2 spatially organizes distant genes and functions as an enhancer in the developing forebrain (60) (Table 1). Last but not least is the “guide” role played by lncRNAs, with a classical example being HOTAIR that guides PRC2 in regulating the expression of a plethora of developmental and cancer-related genes (36), and another example being lncRNA-21 that reroutes the nuclear factor hnRNP-K to specific promoters (61) (Table 1).
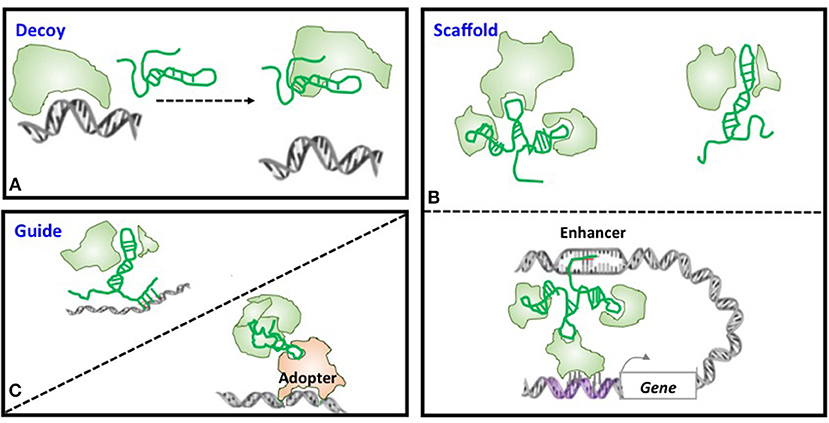
Figure 2. Models of lncRNA mechanisms of action. (A) Decoy. LncRNA can act as decoys that titrate away DNA-binding proteins such as transcription factors or regulatory RNAs such as miRNAs. (B) Scaffold. LncRNAs may act as scaffolds to bring two or more proteins into a complex or spatial proximity which, if through chromosome looping, can function as an enhancer. (C) Guide. LncRNAs may act as guides to recruit proteins such as chromatin modification enzymes to DNA.
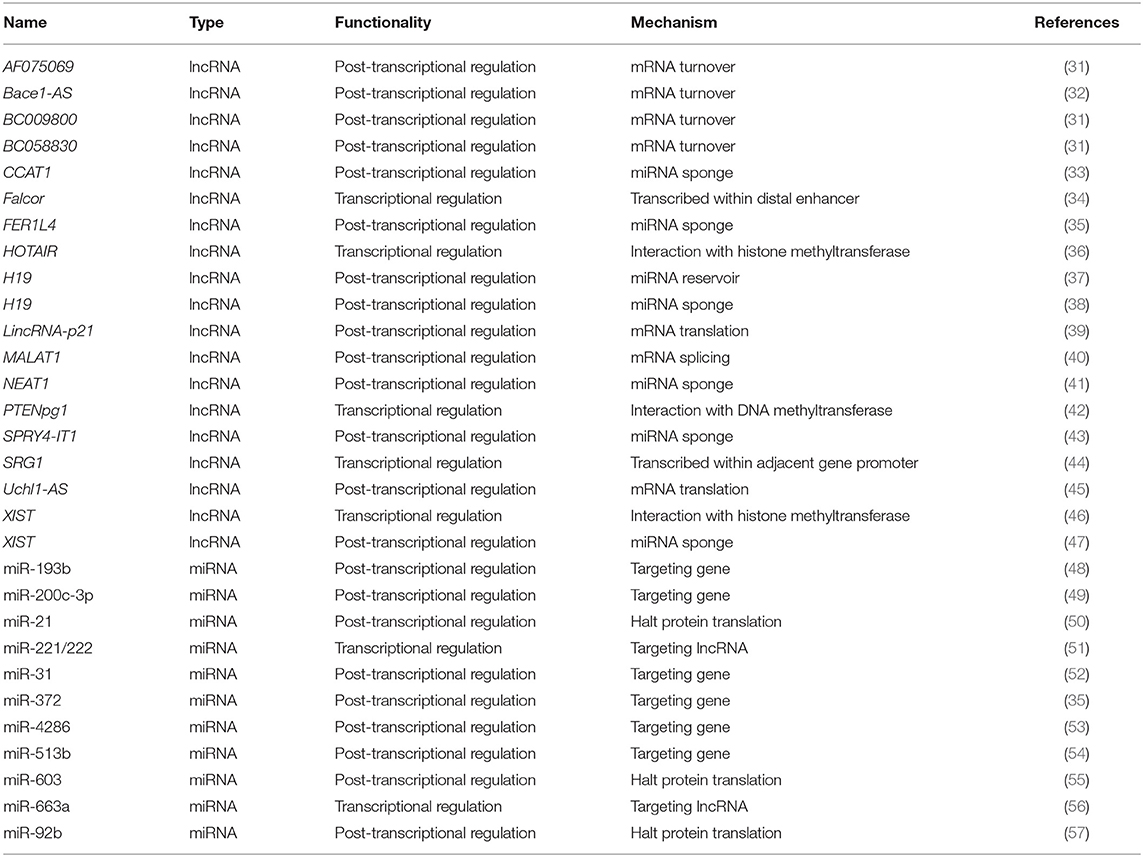
Table 1. Functionalities and mechanisms of example lncRNAs and miRNAs as biomarkers or therapeutic cancer targets.
Transcriptional Regulation
LncRNAs can regulate gene transcription via chromatin remodeling (Figure 3) through confining chromatin remodeling complexes to particular genomic regions via interactions between lncRNAs and histone methyltransferase PRC2 (polycomb repressive complex 2) (15, 26, 70). For example, the lncRNA HOTAIR induces H3K27me3 through direct interactions with EZH2 (the catalytic subunit of PRC2) that, ultimately, suppresses HOXD expression (71, 72). Another example is how lncRNA Xist regulates X chromosome dosage compensation in mammals, where Xist expressed from one X chromosome localizes PRC2 and H3K27me3 to the inactive X chromosome in female cells through physically interacting with PRC2 via RepA; and this results in altered chromatin structure of the entire inactive X chromosome and restored expression of genes located on this chromosome that were previously transcriptionally silenced (46). Besides, lncRNAs can utilize DNA methyltransferases to modify chromatin conformation (73–75). For instance, antisense lncRNAs such as PTENpg1 was shown to interact with a 5′UTR-containing promoter-spanning transcript followed by recruitment of DNA methylatransferase 3A (DNMT3a) to epigenetically control the transcription of the PTEN pseudogene (42) (Table 1).
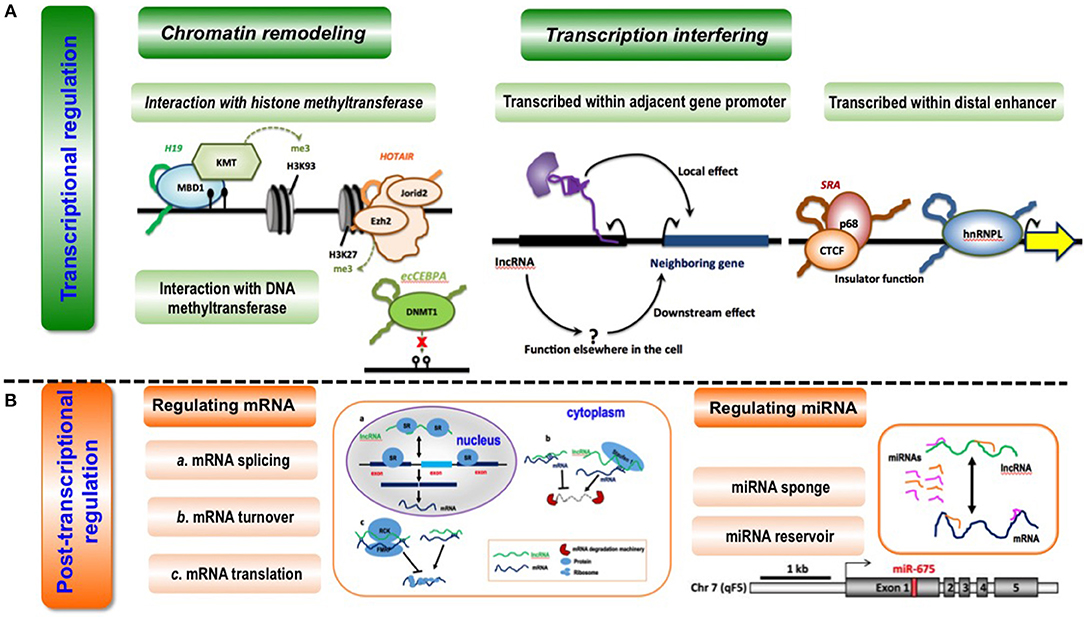
Figure 3. Conceptual scheme illustrating the primary functionalities of lncRNAs. (A) LncRNA-mediated transcriptional regulation can function through chromatin remodeling (via interaction with histone methyltransferase or DNA methyltransferase) and transcription interfering (via being transcribed within adjacent gene promoters or distal enhancers). Examples are given as below. During interactions with histone methyltransferases, lncRNA H19 binds to the methyl-CpG-binding protein MBD1 to control gene expression by recruiting a histone lysine methyltransferase (KMT) (62); lncRNA HOTAIR interacts with the histone methyltransferase Ezh2, a key component of the PRC2 complex, to mediate chromatin-dependent gene regulation, and also interacts with Jarid2, a PRC2-associated factor, to promote the targeting of PRC2 to chromatin (63). During interactions with DNA methyltransferase, lncRNA ecCEBPA interacts with the DNA methyltransferase DNMT1 to block DNA methylation and control gene expression (64). When being transcribed within adjacent gene promoters, lncRNAs affect the expression of neighboring genes directly via local effect or indirectly via downstream effect (65). When being transcribed within distal enhancers, lncRNA SRA interacts with transcription factor CTCF and its associated DEAD-box RNA helicase p68 to form a complex that is essential for insulator function (66); lncRNA THRIL binds to hnRNPL, a component of hnRNP complexes, and the THRIL-hnRNPL complex regulates transcription by binding to target gene promoters (67). (B) LncRNAs can regulate post-transcription via regulating miRNAs or mRNAs. LncRNAs can modulate mRNA splicing, mRNA turnover and mRNA translation at the post-transcriptional level. LncRNA MALAT1 competes for binding for splicing regulatory proteins SR to assist in pre-mRNA splicing (40); lncRNA Bace1-AS forms a hybrid with Bace1 mRNA to prevent its decay (68), and lncRNAs such as BC058830, AF075069, BC009800 promotes the decay of Alu-containing mRNAs (31); lincRNA-p21 interacts with partially complementary mRNAs of Junb and Ctnnb and suppress their translation via recruiting translation repressors Rck and Fmrp (39), and Uchl1-AS interacts with Uchl1 mRNA via a SINEB2 sequence and a segment fully complementary with the 5′ end of the mRNA to recruit ribosomes and activate Uchl1 mRNA translation (45). LncRNAs can function as a sponge or reservoir of mRNAs during post-transcriptional modulation. LncRNA CCAT1 could function as a molecular sponge of let-7 and to reduce its suppression on the endogenous targets Hmga2 and c-Myc in hepatocellular carcinoma (69); increased lncRNA H19 is associated with decreased Igf1R mRNA expression, as miR-675 that targets Igf1R is embedded in the first exon of H19 (37).
LncRNAs can regulate gene expression by interfering with the transcription of regulatory elements such as enhancers and promoters (76, 77) (Figure 3). Some lncRNAs are transcribed within adjacent gene promoters, and thus capable of modifying relevant gene expression through interfering the binding of transcription factors. For instance, the lncRNA SRG1 is transcribed across SER3 promoter, and SER3 expression is considerably reduced on SRG1 transcription (44) (Table 1). LncRNA can be transcribed within distal enhancers that can modulate the expression of neighboring genes through recruiting transcription factors to these loci (78, 79). Transcription factors are sensitive regulators of gene expression, deregulation of which at either transcriptional or translational levels may lead to life-threatening diseases including cancers. LncRNAs are spatially associated with TFs in the genome, and can regulate gene expression by interacting with TFs, with a recent example on the identified regulatory loop between FOXA2 (a transcription factor) and Falcor (a lncRNA) being reported. Specifically, the lncRNA Falcor represses the expression of TF FOXA2 through binding to its promoter that, in turn, regulates Falcor expression; and disruption of this Falcor-FOXA2 regulatory loop may lead to altered cell adhesion and migration that, ultimately, results in goblet cell metaplasia (34) (Table 1).
Post-transcriptional Regulation
LncRNAs can modulate multiple processes in the post-transcriptional modification of messenger RNAs (mRNAs), including pre-mRNA splicing, mRNA turnover and mRNA translation, through recognizing the complementary sequences (Figure 3). For instance, lncRNA MALAT1 competes for the binding with splicing regulatory proteins SR to assist in pre-mRNA splicing (40); lncRNA Bace1-AS forms a hybrid with Bace1 mRNA to prevent its decay (32), and lncRNAs such as BC058830, AF075069, BC009800 promote the decay of Alu-containing mRNAs (31); lincRNA-p21 interacts with partially complementary mRNAs of Junb and Ctnnb and suppresses their translation via recruiting translation repressors Rck and Fmrp (39), and Uchl1-AS recruits ribosomes and activates Uchl1 mRNA translation through short interspersed nuclear element B2 (SINEB2)-mediated interactions with Uchl1 mRNA (45) (Table 1).
Some lncRNAs act as competing endogenous RNAs (ceRNAs) capable of sponging miRNAs to reduce their suppressive effects on targeted genes (80), which is common in tumorigenesis (Figure 3). For instance, the lncRNA CCAT1 could elevate the expression of target genes Hmga2 and c-Myc in hepatocellular carcinoma via sponging their negative regulator let-7 (33); the lncRNA NEAT1 represses glioma progression and reduces its malignancy through sponging miR-107 and inhibiting CDK14 (41); the lncRNA SPRY4-IT1 promotes EMT of cervical cancer by sponging miR-101-3p (43); and the lncRNA FER1L4 functions as a sponge of miR-372 that targets E2F1 to regulate cell cycle progression in glioma cells (35) (Table 1). Also, lncRNAs can act as the reservoir of miRNAs that ultimately leads to gene repression via giving rise to miRNAs (Figure 3). For example, increased lncRNA H19 is associated with decreased Igf1R mRNA expression, as miR-675 that targets Igf1R is embedded in the first exon of H19 (37) (Table 1).
Micro RNAs
Features of miRNAs
MiRNAs are a group of petite ncRNA molecules ranging from 16 to 27 nt in length that are efficient to adjust gene expression transcriptionally and/or translationally. MiRNAs straightly interact with partial complementary target spots positioned in the 3′ untranslated region (UTR) of the targeted gene to repress its expression. Above 60% mRNAs have miRNA binding sites in their 3′UTR regions according to computational predictions, suggesting the critical roles of miRNAs in maintaining cellular homeostasis at both healthy and diseased states. Many miRNAs regulate up to hundreds of mRNAs, implicating complicated regulatory roles of miRNA on the topology of mRNA modulation network. The expression of miRNAs is tissue-specific (81), and tightly regulated temporally and spatially (82).
Generally, mammalian miRNAs are encoded in the genome and transcribed as initial miRNA transcripts (pri-miRNAs) through RNA Polymerase II, get processed to the precursor miRNAs (pre-miRNA) that harbor a stem-loop structure through the DROSHA-DGCR8 complex, and located to the cytosol through exportin5 (XPO-5); there, pre-miRNAs are processed further into dsRNAs that are nearly 21 nt in length by RNase III enzyme DICER1 coupled with PACT or TRBP in cytoplasm; short dsRNAs are merged into RNA-induced silencing complex (RISC) through binding with an Argonaute family member; while one strand of the dsRNA is preserved in RISC, the other stand undergoes fast degradation (83). The miRNA functions through binding to the 3′ UTR of the target messenger RNA (mRNA) via sequence complementarity (84). While perfect base pair match leads to mRNA degradation, imperfect pairing results in mRNA sequestration and translation inhibition (85).
MiRNAs undergo complicated post-transcriptional alterations such as miRNA accumulation, editing, processing and re-cycling inside P-bodies during maturation (86). This complex yet well-orchestrated miRNA maturation process renders it difficult to evaluate, in real time, the spatio-temporal pattern of miRNA expression and results in a gap between the expression and function of miRNAs. Therefore, understanding the functionalities of miRNAs under physiological and pathological conditions imposes a great challenge in the field of miRNA biology. Traditional miRNA profiling approaches such as microarray, real-time PCR, Northern blot, deep sequencing and in situ hybridization, though adding to our knowledge on miRNA biology, can rarely throw light on the spatiotemporal configurations and roles played by miRNAs in situ. Increasing attention has been paid to non-invasive molecular imaging methods which may potentially resolve these aforementioned issues (87).
Functionalities of miRNAs
MiRNAs can regulate gene regulation at the post-transcriptional level via degrading targeted mRNAs or repressing protein translation, and at the transcriptional level via targeting regulatory RNAs such as lncRNAs.
Transcriptional Regulation
MiRNAs can target regulatory molecules such as lncRNAs that may affect gene expression via functioning as decoys, scaffolds, or guides; and quite often a regulatory axis involving miRNA, lncRNA and mRNA is implicated in the regulatory mechanism that drives the observed pathological consequence. For instance, miR-221/222 can inhibit tumor apoptosis in breast cancers via targeting the lncRNA GAS5 (51) (Table 1). The miR-663a targets ZBTB7A that transcriptionally suppresses lncRNA GAS5 under ER stress (56), and lncRNA GAS5 suppresses tumor cell proliferation and EMT in oral squamous cell carcinoma via regulating the miR-21/Pten axis (88) (Table 1).
Post-transcriptional Regulation
Degrading target mRNAs at the post-transcriptional level is the common mechanism adopted by miRNA in gene regulation. The functional roles of miRNA in both physiological and pathological processes by adjusting their target gene expression have been demonstrated through vast number of evidences (89–91), with recent attentions attracted to the roles played by miRNAs in cancer initiation, progression, angiogenesis, metastasis and chemoresistance (92). For example, conditional knockout of miR-31 was shown to promote the development of colitis-associated cancers (52); miR-193b exhibited a tumor suppressive role in human esophageal squamous cell cancers via targeting KRAS (48); miR-200c-3p suppressed the proliferative and invasive abilities of nephroblastoma cells via targeting FRS2 (49); miR-4286 and miR-513b each promoted cell proliferation and migration via targeting Pten and HMGB3, respectively, in non-small cell lung cancer (53, 54) (Table 1).
MiRNA can also inhibit protein translation. For instance, miR-603 promoted tumor proliferation by inhibiting the translation of BRCC2 protein in osteosarcoma (55); miR-21 repressed the translation of the tumor suppressor PDCD4 (50); and miR-92b restored the sensitivity of hepatocellular carcinoma to ionizing radiation-based radiotherapy through inhibiting the protein expression of p57kip2 (57) (Table 1).
Clinical Implications of Non-coding RNAs
Variations in non-coding genomic regions have been associated with cancer susceptibility (93), and a plethora of cancer risk loci were documented to be transcribed into ncRNAs that play vital roles in tumorigenesis and progression.
Diagnosis and Prognosis
LncRNAs can serve as diagnostic and prognostic biomarkers if they were highly correlated with particular cancer states. For example, the expression of lncRNA PRNCR1 was elevated in the urine samples of prostate cancer patients, rendering it a fine non-invasive indicator of prostate cancers (94). Many other lncRNAs have been associated with various malignancies such as MALAT1 in non-small-cell lung cancer and hepatoblastoma, HOTAIR in pancreatic and colorectal malignancies (95), FOXD2-AS1 in colorectal cancer (96), LSINCT5 in breast and ovarian cancers (97), PTCSC3 in papillary thyroid cancer (98), TUG1 in bladder urothelial cancer (99), and UCA1 in squamous carcinoma (38, 100, 101), suggesting their diagnostic potential. However, we are still unclear on what alterations in the primary sequence of lncRNAs mean for their functionalities, and how to predict the activities of lncRNAs through their secondary structures and the properties of its interacting disease-associated proteins (102), answers to which may advance our understandings on the working mechanisms of lncRNAs that drive their prognostic roles.
Tumors ubiquitously exhibit dysregulated miRNA expression and such profiles convey useful information for tumor classification and prognosis. MiRNAs are present in numerous body fluids including saliva, plasma, serum, and amniotic fluid (103). Thus, extracellular miRNAs are potential biomarkers for disease diagnosis. Serum miRNAs have been associated with the incidence of both solid tumors and hematologic cancers, and proposed for early detection of various malignancies (104, 105). As miRNAs are featured by having multiple targets, they are typically used in panels for cancer diagnosis/therapeutics. A serum miRNA classifier consisting of 7 miRNAs (miR-29a, miR-29c, miR-133a, miR-143, miR-145, miR-192, miR-505) has been used for hepatocellular cancer early detection in a retrospective, longitudinal, multicenter biomarker identification study (106). The miRNA7™ panel was approved by CFDA for liver cancer diagnosis in August 2017, which becomes the first ncRNA panel translated into clinics and released on the market in the world. It outweighs the traditional alpha-fetoprotein (AFP) kit in being capable of identifying AFP-negative liver cancers from just 0.2 ml blood plasma with 84% sensitivity and 88% specificity. Another classifier consisting of 6 miRNAs, i.e., miR-20a-5p, miR-21-5p, miR-103a-3p, miR-106b-5p, miR-143-5p, and miR-215, was established to classify stage II colon carcinomas into patients having high and low risks regarding disease progression, and was demonstrated as a reliable prognostic tool for disease recurrence prediction (107). A microRNA-based signature (MiROvaR) comprised of 35 miRNAs was developed to predict disease progression or early relapse of epithelial ovarian cancers from a cohort study (108). Fourteen circulating miRNAs were identified associated with docetaxel chemotherapy response among prostate cancers from a phase I discovery study (that included 97 patients) (109), out of which six (miR-132, miR-200a, miR-200b, miR-200c, miR-375, miR-429) were verified with prognostic values from the following phase II validation study (that consisted of 89 patients) (110). Besides, circulating miRNAs have been correlated with diverse cardiovascular disorders (111). It is worth noting that the origin and functions of extracellular miRNAs, though being actively investigated, are not fully characterized. Whether circulating miRNAs in mammals exhibit hormone-like actions or achieve sufficient concentrations in certain tissues to repress distal targets remains unanswered. This, once addressed, can provide useful insights to avail clinical translation of miRNAs.
Therapeutics
LncRNAs in Therapeutics
LncRNAs can be targeted to combat against disease progression and enrich current treatment modalities in the control cancer patient who developed resistant to traditional therapies. For example, lncRNA aHIF is an antisense RNA (aRNA) overexpressed in human renal cancer with drugging potential; LINK-A overexpression was observed in breast and lung cancer patients that had developed resistance to AKT inhibitors, providing the rational for targeting LINK-A, either alone or in combination with AKT inhibitors, in the treatment of such cancers (112).
A well-characterized function of lncRNAs is its modulatory roles on chromatin states (113). Thus, we can target aRNAs to achieve locus-specific up-regulation of a protein and its natural variants, e.g., by targeting BDNF-AS, the natural antisense transcript of BDNF, 2- to 7-folds up-regulation on BDNF expression with no visible effect on the neighboring genes was observed both in vitro and in vivo (114). To achieve this, single-stranded oligonucleotides, namely antagoNATs, can be fabricated to degrade these antisense transcripts and/or block their interactions with sense mRNAs (114) which, however, needs in vivo adaptations such as chemical modifications toward improved metabolic stabilities and minimized length for enhanced cellular uptake. The locked nucleic acids (LNA)-modified antisense oligonucleotides strategy was established accordingly. Brown et al. combined the carbamate DNA backbone analogs with the LNA (LNA-CBM) to confer enzymatic resistance and thermodynamic stability, and oligonucleotides modified with LNA-CBM exhibited increased stability in the presence of fetal bovine serum and snake venom (115).
LncRNAs can also be targeted, at least in vitro, using siRNAs if the targeted region in the antisense transcript did not directly overlap with the sense transcript. For example, through a loss-of-function RNA interference screen targeting 797 aRNAs, the functionalities of aRNAs against CCPG1 and RAPGEF3 in disrupting signalings orchestrating cell viability were revealed (116). It is worth mentioning that some aRNAs such as BACE-AS has high basal expression level and, can mask miRNA binding sites through forming duplexes with mRNAs; and siRNAs targeting these antisense transcripts will down- rather than up-regulate the targeted transcripts (117).
MiRNAs in Therapeutics
Dysregulation of miRNAs can be well-tolerated in normal tissues yet can profoundly alter cell behavior in response to pathologic stress. Thus, miRNA offers a potential tool to perturb a disease progress without creating unexpected adverse effects in normal cells. The antagoNAT that is modified with LNA through a covalent bridge connecting the 2'-oxygen and 4' carbon of the ribose moiety of the nucleotide can form strong duplexes with its target miRNAs, sequester these targeted miRNAs but do not promote their degradation. Tiny LNAs were later created to inhibit miRNA families sharing homology by targeting their seed regions (118). AntagoNATs modified with LNA can be systemically delivered by injection with little or no toxicity, with sufficient uptake and desirable therapeutic efficacy being reported in, e.g., immune, vascular and heart systems in rodents. An LNA-modified antagoNAT against miR-122 (namely SPC3649) was developed to fight against hepatitis C virus infection, where a dose-dependent prolonged viral reduction was observed after 4 weeks of treatment without observable toxicity from a human Phase II study (119). TargomiRs are minicells carrying miR-16-based mimic miRNAs targeting EGFR, which counteract recessed expression of miR-15 and miR-16 family miRNAs in malignant pleural mesothelioma by design; and a phase I, open-label, dose-escalation study assessing the safety and activity of TargomiRs was conducted in patients carrying malignant pleural mesothelioma, with positive results being reported (120) (Table 2, Supplementary Table 1).
As many miRNAs appear to be beneficial rather than pathologic, the development of miRNA mimics represents another important therapeutic regime. Through encapsulating or conjugating synthetic miRNA duplexes inside/to nanoparticles such as liposome or using adeno-associated virus as the delivery vehicle, successes (recessed tumor growth without obvious toxicity to normal cells) in miRNA mimicry-based therapeutics have been achieved in many tumor animal models (121, 122). However, the use of injectable, naked miRNA mimics, unlike miRNA inhibitors, remains problematic, as the complementary strand needs to be modified to facilitate stability and cellular uptake but has the potential to act as an anti-miRNA.
Opportunities Brought by ncRNAs to Cancer Control
The regulatory roles of RNAs, in particular ncRNAs, on gene expression has led to the establishment of experimental tools such as RNA interference and CRISPR/Cas9 for gene modulation. The functions of ncRNAs in transducing signals of multiple core cancer pathways such as Hedgehog, JAK/STAT, WNT, HIF, NFkB, PI3K/PTEN, result in their translational applications in both diagnosis and therapeutics. As prognostic biomarkers, ncRNAs may capture dynamic subtle alterations due to pathological stimuli that are difficult to be precisely monitored using DNAs or proteins. Therapeutically, targeting ncRNAs offers us a direct tool to inhibit protein function or down-regulate gene expression and represents a promising strategy to up-regulate endogenous genes in a locus-specific manner. That is, inhibiting miRNAs using antagoNATs can effectively up-regulate many downstream target genes, which is difficult to achieve using conventional strategies. This is therapeutically advantageous as it is easier to inhibit rather than up-regulate a drug target where the latter typically requires the identification of appropriate agents; and conventional strategies that restore reduced gene expression or recessed protein functionalities possess various limitations including, e.g., a need of lifespan intake of therapeutic proteins that may be associated with adverse immune response by themselves, and difficulty in producing synthetic proteins that can fully mimic the plethora of endogenous counterparts of targeted proteins.
Challenges Faced by ncRNAs in Cancer Control
Many challenges must be overcome to fully convey the promise of ncRNA-based therapeutics. First, targeted and efficient delivery of oligonucleotide-based therapies, particularly anti-miRNA, miRNA mimics, siRNA, and shRNA, into tissues and cells remains a crucial hurdle. This is worthy of special attention as ncRNAs especially miRNAs may accelerate disease in one tissue and be tumor suppressive in another, e.g., miR-26a promotes gliomagenesis (123) but shows double-sided roles in the carcinogenesis of hepatocellular cancers (124, 125). Second, safety imposes an important challenge before drugging ncRNAs can be clinically applicable. Oligonucleotide sequence has been reported to have adverse effects due to, mostly, protein binding. For instance, phosphorothioate-containing oligonucleotides show pro-inflammatory properties. More preclinical and clinical studies are needed to decipher such correlations between sequence motifs and such liabilities. Off-target is another source of unanticipated events, which warrants our consideration from multiple aspects in the design of oligonucleotide-based drugs including, e.g., RNA structure, its association with various proteins, mismatch in the cleavage site etc. Third, the effects of ncRNAs may vary among cancer types, e.g., lncRNA Xist is tumor suppressive in osteosarcoma (126), cervical cancer (8), and breast tumor (127), but is oncogenic in pancreatic cancer (47), gastric cancer (128), thyroid cancer (129), colon cancer (130), hepatocellular carcinoma (131), non-small-cell lung cancer (132), bladder cancer (133), glioblastoma (134), and nasopharyngeal carcinoma (135). Fourth, there exists an inconsistency between short-term chemical inhibition and genetic deletion, complicating our understanding toward the functionalities of ncRNAs in relation to disease control. For instance, despite the pathological associations they demonstrate, germ-line depletion of miR-21 does not affect cardiovascular pathology, H19-knockout mice does not show a disease phenotype (136), HOTAIR-deletion does not abolish PRC2 targeting (137), and MALAT1 and NEAT1 removal yields normal and viable mice (138, 139). This indicates that chronic loss-of-function of ncRNA may lead to adaptive responses to compensate for its absence, which makes our last concern, i.e., whether therapeutic efficacy of drugs targeting ncRNAs will decline with time due to the compensation of the target network for diminished ncRNA activity, challenging.
It is also important to address the differences between targeting lncRNAs and miRNAs. First, aRNAs, a type of lncRNAs, are primarily chromatin modulators suppressing transcription in the nucleus, whereas miRNAs are post-transcriptional repressors affecting mRNA stability in the cytoplasm. Second, antagoNATs can be used to target aRNA through cleaving or blocking a RNA or protein target, whereas steric blockade is typically used to inhibit miRNA. Third, there are much more yet less-studied lncRNA species than miRNAs. Fourth, cis-acting aRNAs are gene-locus specific, whereas miRNAs can affect the stability of many mRNAs. As several problems faced by drugging miRNAs naturally vanish when targeting lncRNAs, lncRNAs deliver more clinical promises than miRNAs despite the fact that they are less-well studied for clinic translation. For example, it is difficult to establish a precise correlation between miRNA target engagement and therapeutic efficacy as miRNAs can moderately modulate myriad targets to evoke their effects and oligonucleotides may be sequestered in various cellular compartments to create further uncertainties; such a problem does not exist in the case of lncRNAs given their working mechanisms.
Perspectives
LncRNAs and miRNAs each represent a big class of ncRNAs with profound clinical implications, but differ greatly in terms of the nuclear acid biology, cellular behaviors and working mechanisms. Though both functioning through perfect or imperfect base-pairing with DNAs, RNAs and proteins, lncRNAs can take on more diversified action modes such as decoy, scaffold and guide which are all derived from their long length and the tertiary structure they can form. While lncRNAs can regulate gene expression in both directions, miRNAs, on the other hand, represent a class of sole negative gene expression regulators either through degrading target mRNAs or halting the translation of target genes; however, the regulatory flexibility of miRNAs can be largely extended by targeting lncRNAs, forming regulatory miRNA-lncRNA axes orchestrating the spatial and temporal cellular behavior under both normal and pathological conditions (140–145).
The identified mechanisms are not mutually exclusive and one ncRNA may have multiple working mechanisms under different circumstances. For example, the lncRNA H19 can function as a miRNA reservoir of miR-675 that suppresses cell growth (37) and as a miRNA sponge of let-7a that up-regulates cyclin D1 expression (146); the lncRNA XIST interacts with histone methyltransferase at the transcriptional level in regulating chromosome dosage compensation (46), and sponges miR-34a to promote colon cancer progression at the post-translational level (130).
Other types of ncRNAs may also function as disease indicators or therapeutic targets. For instance, deficiency in SNORD50A/B snoRNAs can augment the functionalities of K-Ras that ultimately leads to hyperactivated MAPK/ERK signaling and carcinogenesis; and SNORD50A/B deletion occurs at a frequency of >10% in each of 12 common cancers. Therefore, exploring the varieties of ncRNAs, and functionalities and clinical use of novel types of ncRNAs represent another promising research domain for improved cancer management.
NcRNAs have offered a plethora of opportunities toward delicate gene modulations and precision medicine. Challenges faced by bringing ncRNAs to the bed-side could be well-resolved only if multi-domain knowledges and techniques were intelligently brought together. Nanomaterials such as gold nanoparticles (AuNPs), carbon-based nano-composites, and liposome could help to achieve targeted and efficient delivery of ncRNAs given their flexibility in surface modification, size and hydrophobicity control, as well as other physiochemical features that can, e.g., protect cargoes from fast degradation. For instance, by encapsulating a miRNA antagoNAT against miRNA-122 harboring 2′-OMe and phosphorothioate modifications (namely, AMO122) inside YSK05-MEND that is a multifunctional nano-vehicle (MEND) containing a pH-sensitive lipid YSK05, more liver deposition and drug efficacy were observed for YSK05 carried by MEND than free YSK05 that ultimately led to enhanced liver cancer treatment efficacy in vivo (147). Computational approaches and high-throughput technologies could be utilized to resolve issues related to seemingly unpredictable effects of ncRNAs in different tissues by drawing a comprehensive picture on the spatio-temporal regulatory properties of a given ncRNA.
As being consistent with many biological networks, the experimentally derived RNA interactome is scale-free (148). A scale-free network is biologically plausible given its robustness to extra- or intra- cellular perturbations and evolutionary economy in pertaining functionalities. In such networks, the number of nodes is negatively correlated with that of interactions involved, formulating a hierarchical structure. A failure is more likely to hit a node with fewer degrees rather than a hub. In addition, a scale-free network is organized such that low-degree nodes are condensed in sub-networks that are connected by a few promiscuous nodes; thus, a given functionality can be easily reached by convolving small number of players through multiple paths. On the contrary, promiscuous nodes are prevalent in a flat network, and have been reported in many pathological events such as gene fusions (149, 150). Thus, a scale-free network may represent an ordered and homostatic state, and a flat network may be indicative of a chaotic and diseased state. Toward this end, alterations in a network's structure may suggest a switch between ergodic sets and the associated cell states, and we may consider selecting nodes or connections capable of toggling a network's structure as candidates for subsequent clinical translations.
Conclusions
With the rapid development of various bioinformatics technologies, a plethora of ncRNAs and their roles in tumorigenesis have been uncovered. Abnormally expressed ncRNAs might be adopted as diagnostic/prognostic biomarkers, and therapeutic targets for cancer management. More studies investigating novel mechanisms of ncRNAs in mediating cancer initiation and progression are needed for comprehensive understandings on their roles in carcinogenesis, and solutions to challenges faced by translating ncRNAs into clinics are imperatively needed to embrace the new paradigm shift from protein coding to non-coding RNA regime. Toward these goals, integrative efforts from multiple domains such as nanomaterials, computational system biology, molecular biology, and clinical medicine should be intelligently brought together.
Author Contributions
XD conceptualized the ideas and prepared the figures and tables. XD and AK conducted the literature search and drafted the manuscript. XD and JZ offered financial support. All authors have read and approved the manuscript.
Funding
This study was funded by the National Natural Science Foundation of China (Grant No. 81972789), the National Science and Technology Major Project (Grant No. 2018ZX10302205-004-002), the Natural Science Foundation of Jiangsu Province (Grant No. BK20161130), the Six Talent Peaks Project in Jiangsu Province (Grant No. SWYY-128), Technology Development Funding of Wuxi (Grant No. WX18IVJN017), Major Project of Science and Technology in Henan Province (Grant No. 161100311400), Research Funds for the Medical School of Jiangnan University ESI Special Cultivation Project (Grant No. 1286010241170320). These funding sources have no role in the writing of the manuscript or the decision to submit it for publication.
Conflict of Interest
The authors declare that the research was conducted in the absence of any commercial or financial relationships that could be construed as a potential conflict of interest.
The handling editor declared a past co-authorship with one of the authors XD.
Supplementary Material
The Supplementary Material for this article can be found online at: https://www.frontiersin.org/articles/10.3389/fonc.2019.00920/full#supplementary-material
References
1. Kapranov P, Cawley SE, Drenkow J, Bekiranov S, Strausberg RL, Fodor SP, et al. Large-scale transcriptional activity in chromosomes 21 and 22. Science. (2002) 296:916–9. doi: 10.1126/science.1068597
2. Kapranov P, Willingham AT, Gingeras TR. Genome-wide transcription and the implications for genomic organization. Nat Rev Genet. (2007) 8:413–23. doi: 10.1038/nrg2083
4. Majem B, Rigau M, Reventós J, Wong DT. Non-coding RNAs in saliva: emerging biomarkers for molecular diagnostics. Int J Mol Sci. (2015) 16:8676–98. doi: 10.3390/ijms16048676
5. Kapranov P, Cheng J, Dike S, Nix DA, Duttagupta R, Willingham AT, et al. RNA maps reveal new RNA classes and a possible function for pervasive transcription. Science. (2007) 316:1484–8. doi: 10.1126/science.1138341
6. Wahlestedt C. Targeting long non-coding RNA to therapeutically upregulate gene expression. Nat Rev Drug Discov. (2013) 12:433–46. doi: 10.1038/nrd4018
7. Takenaka K, Chen BJ, Modesitt SC, Byrne FL, Hoehn KL, Janitz M. The emerging role of long non-coding RNAs in endometrial cancer. Cancer Genet. (2016) 209:445–55. doi: 10.1016/j.cancergen.2016.09.005
8. Kobayashi R, Miyagawa R, Yamashita H, Morikawa T, Okuma K, Fukayama M, et al. Increased expression of long non-coding RNA XIST predicts favorable prognosis of cervical squamous cell carcinoma subsequent to definitive chemoradiation therapy. Oncol Lett. (2016) 12:3066–74. doi: 10.3892/ol.2016.5054
9. Amicone L, Citarella F, Cicchini C. Epigenetic regulation in hepatocellular carcinoma requires long noncoding RNAs. Biomed Res Int. (2015) 2015:473942. doi: 10.1155/2015/473942
10. Esteller M. Non-coding RNAs in human disease. Nat Rev Genet. (2011) 12:861–74. doi: 10.1038/nrg3074
11. Wang Y, Xue K, Guan Y, Jin Y, Liu S, Wang Y, et al. Long noncoding RNA LINC00261 suppresses cell proliferation and invasion and promotes cell apoptosis in human choriocarcinoma. Oncol Res. (2017) 25:733–42. doi: 10.3727/096504016X14772362173376
12. Pfeffer SR, Yang CH, Pfeffer LM. The role of miR-21 in cancer. Drug Dev Res. (2015) 76:270–7. doi: 10.1002/ddr.21257
13. Ge Y, Zhang L, Nikolova M, Reva B, Fuchs E. Strand-specific in vivo screen of cancer-associated miRNAs unveils a role for miR-21(*) in SCC progression. Nat Cell Biol. (2016) 18:111–21. doi: 10.1038/ncb3275
14. Novikova IV, Hennelly SP, Sanbonmatsu KY. Sizing up long non-coding RNAs: do lncRNAs have secondary and tertiary structure? Bioarchitecture. (2012) 2:189–99. doi: 10.4161/bioa.22592
15. Mercer TR, Dinger ME, Mattick JS. Long non-coding RNAs: insights into functions. Nat Rev Genet. (2009) 10:155–9. doi: 10.1038/nrg2521
16. Okazaki Y, Furuno M, Kasukawa T, Adachi J, Bono H, Kondo S, et al. Analysis of the mouse transcriptome based on functional annotation of 60,770 full-length cDNAs. Nature. (2002) 420:563–73. doi: 10.1038/nature01266
17. Rinn JL, Kertesz M, Wang JK, Squazzo SL, Xu X, Brugmann SA, et al. Functional demarcation of active and silent chromatin domains in human HOX loci by noncoding RNAs. Cell. (2007) 129:1311–23. doi: 10.1016/j.cell.2007.05.022
18. Haerty W, Ponting CP. Unexpected selection to retain high GC content and splicing enhancers within exons of multiexonic lncRNA loci. RNA. (2015) 21:320–32. doi: 10.1261/rna.047324.114
19. Niazi F, Valadkhan S. Computational analysis of functional long noncoding RNAs reveals lack of peptide-coding capacity and parallels with 3′ UTRs. RNA. (2012) 18:825–43. doi: 10.1261/rna.029520.111
20. Novikova IV, Hennelly SP, Tung CS, Sanbonmatsu KY. Rise of the RNA machines: exploring the structure of long non-coding RNAs. J Mol Biol. (2013) 425:3731–46. doi: 10.1016/j.jmb.2013.02.030
21. Liu F, Somarowthu S, Pyle AM. Visualizing the secondary and tertiary architectural domains of lncRNA RepA. Nat Chem Biol. (2017) 13:282–9. doi: 10.1038/nchembio.2272
22. Guo XL, Gao L, Wang Y, Chiu DK, Wang T, Deng Y. Advances in long noncoding RNAs: identification, structure prediction and function annotation. Brief Funct Genomics. (2016) 15:38–46. doi: 10.1093/bfgp/elv022
23. Zampetaki A, Albrecht A, Steinhofel K. Long-noncoding RNA structure and function: is there a link? Front Physiol. (2018) 9:1201. doi: 10.3389/fphys.2018.01201
24. Ponting CP, Oliver PL, Reik W. Evolution and functions of long noncoding RNAs. Cell. (2009) 136:629–41. doi: 10.1016/j.cell.2009.02.006
25. Ma L, Bajic VB, Zhang Z. On the classification of long non-coding RNAs. RNA Biol. (2013) 10:925–33. doi: 10.4161/rna.24604
26. Bartonicek N, Maag JL, Dinger ME. Long noncoding RNAs in cancer: mechanisms of action and technological advancements. Mol Cancer. (2016) 15:43. doi: 10.1186/s12943-016-0530-6
27. Iyer MK, Niknafs YS, Malik R, Singhal U, Sahu A, Hosono Y, et al. The landscape of long noncoding RNAs in the human transcriptome. Nat Genet. (2015) 47:199–208. doi: 10.1038/ng.3192
28. Cao J. The functional role of long non-coding RNAs and epigenetics. Biol Proced Online. (2014) 16:11. doi: 10.1186/1480-9222-16-11
29. Hu K, Zhang J, Liang M. LncRNA AK015322 promotes proliferation of spermatogonial stem cell C18-4 by acting as a decoy for microRNA-19b-3p. In Vitro Cell Dev Biol Anim. (2017) 53:277–84. doi: 10.1007/s11626-016-0102-5
30. Sawaya AP, Pastar I, Stojadinovic O, Lazovic S, Davis SC, Gil J, et al. Topical mevastatin promotes wound healing by inhibiting the transcription factor c-Myc via the glucocorticoid receptor and the long non-coding RNA Gas5. J Biol Chem. (2018) 293:1439–49. doi: 10.1074/jbc.M117.811240
31. Gong C, Maquat LE. lncRNAs transactivate STAU1-mediated mRNA decay by duplexing with 3′ UTRs via Alu elements. Nature. (2011) 470:284–8. doi: 10.1038/nature09701
32. Zeng T, Ni H, Yu Y, Zhang M, Wu M, Wang Q, et al. BACE1-AS prevents BACE1 mRNA degradation through the sequestration of BACE1-targeting miRNAs. J Chem Neuroanat. (2019) 98:87–96. doi: 10.1016/j.jchemneu.2019.04.001
33. Zhang J, Cai M, Jiang D, Xu L. Upregulated LncRNA-CCAT1 promotes hepatocellular carcinoma progression by functioning as miR-30c-2-3p sponge. Cell Biochem Funct. (2019) 37:84–92. doi: 10.1002/cbf.3375
34. Swarr DT, Herriges M, Li S, Morley M, Fernandes S, Sridharan A, et al. The long noncoding RNA Falcor regulates Foxa2 expression to maintain lung epithelial homeostasis and promote regeneration. Genes Dev. (2019) 33:656–68. doi: 10.1101/gad.320523.118
35. Xia L, Nie D, Wang G, Sun C, Chen G. FER1L4/miR-372/E2F1 works as a ceRNA system to regulate the proliferation and cell cycle of glioma cells. J Cell Mol Med. (2019) 23:3224–33. doi: 10.1111/jcmm.14198
36. Wu L, Murat P, Matak-Vinkovic D, Murrell A, Balasubramanian S. Binding interactions between long noncoding RNA HOTAIR and PRC2 proteins. Biochemistry. (2013) 52:9519–27. doi: 10.1021/bi401085h
37. Keniry A, Oxley D, Monnier P, Kyba M, Dandolo L, Smits G, et al. The H19 lincRNA is a developmental reservoir of miR-675 that suppresses growth and Igf1r. Nat Cell Biol. (2012) 14:659–65. doi: 10.1038/ncb2521
38. Sun S, Gong C, Yuan K. LncRNA UCA1 promotes cell proliferation, invasion and migration of laryngeal squamous cell carcinoma cells by activating Wnt/β catenin signaling pathway. Exp Ther Med. (2019) 17:1182–9. doi: 10.3892/etm.2018.7097
39. Yoon JH, Abdelmohsen K, Srikantan S, Yang X, Martindale JL, De S, et al. LincRNA-p21 suppresses target mRNA translation. Mol Cell. (2012) 47:648–55. doi: 10.1016/j.molcel.2012.06.027
40. Tripathi V, Ellis JD, Shen Z, Song DY, Pan Q, Watt AT, et al. The nuclearretained noncoding RNA MALAT1 regulates alternative splicing by modulating SR splicing factor phosphorylation. Mol Cell. (2010) 39:925–38. doi: 10.1016/j.molcel.2010.08.011
41. Zhen Y, Nan Y, Guo S, Zhang L, Li G, Yue S, et al. Knockdown of NEAT1 repressed the malignant progression of glioma through sponging miR-107 and inhibiting CDK14. J Cell Physiol. (2019) 234:10671–9. doi: 10.1002/jcp.27727
42. Lister N, Shevchenko G, Walshe JL, Groen J, Johnsson P, Vidarsdottir L, et al. The molecular dynamics of long noncoding RNA control of transcription in PTEN and its pseudogene. Proc Natl Acad Sci USA. (2017) 114:9942–7. doi: 10.1073/pnas.1621490114
43. Fan MJ, Zou YH, He PJ, Zhang S, Sun XM, Li CZ. Long non-coding RNA SPRY4-IT1 promotes epithelial-mesenchymal transition of cervical cancer by regulating the miR-101-3p/ZEB1 axis. Biosci Rep. (2019) 39:BSR20181339. doi: 10.1042/BSR20181339
44. Martens JA, Laprade L, Winston F. Intergenic transcription is required to repress the Saccharomyces cerevisiae SER3 gene. Nature. (2004) 429:571–4. doi: 10.1038/nature02538
45. Carrieri C, Cimatti L, Biagioli M, Beugnet A, Zucchelli S, Fedele S, et al. New long non-coding antisense RNA controls Uchl1 translation through an embedded SINEB2 repeat. Nature. (2012) 491:454–7. doi: 10.1038/nature11508
46. Chen YK, Yen Y. The ambivalent role of lncRNA Xist in carcinogenesis. Stem Cell Rev. (2019) 15:314–23. doi: 10.1007/s12015-019-9871-z
47. Sun Z, Zhang B, Cui T. Long non-coding RNA XIST exerts oncogenic functions in pancreatic cancer via miR-34a-5p. Oncol Rep. (2018) 39:1591–600. doi: 10.3892/or.2018.6245
48. Kang M, Li Y, Zhu S, Zhang S, Guo S, Li P. MicroRNA-193b acts as a tumor suppressor gene in human esophageal squamous cell carcinoma via target regulation of KRA. Oncol Lett. (2019) 17:3965–73. doi: 10.3892/ol.2019.10039
49. Li T, Zhao P, Li Z, Wang CC, Wang YL, Gu Q. miR-200c-3p suppresses the proliferative, migratory, and invasive capacities of nephroblastoma cells via targeting FRS2. Biopreserv Biobank. (2019). doi: 10.1089/bio.2019.0009. [Epub ahead of print].
50. Poria DK, Guha A, Nandi I, Ray PS. RNA-binding protein HuR sequesters microRNA-21 to prevent translation repression of proinflammatory tumor suppressor gene programmed cell death 4. Oncogene. (2016) 35:1703–15. doi: 10.1038/onc.2015.235
51. Zong Y, Zhang Y, Sun X, Xu T, Cheng X, Qin Y. miR-221/222 promote tumor growth and suppress apoptosis by targeting lncRNA GAS5 in breast cancer. Biosci Rep. (2019) 39:BSR20181859. doi: 10.1042/BSR20181859
52. Liu Z, Bai J, Zhang L, Lou F, Ke F, Cai W, et al. Conditional knockout of microRNA-31 promotes the development of colitis associated cancer. Biochem Biophys Res Commun. (2017) 490:62–8. doi: 10.1016/j.bbrc.2017.06.012
53. Ling C, Wang X, Zhu J, Tang H, Du W, Zeng Y, et al. MicroRNA-4286 promotes cell proliferation, migration, and invasion via PTEN regulation of the PI3K/Akt pathway in non-small cell lung cancer. Cancer Med. (2019) 8:3520–31. doi: 10.1002/cam4.2220
54. Wang J, Sheng Z, Cai Y. Effects of microRNA-513b on cell proliferation, apoptosis, invasion, and migration by targeting HMGB3 through regulation of mTOR signaling pathway in non-small-cell lung cancer. J Cell Physiol. (2019) 234:10934–41. doi: 10.1002/jcp.27921
55. Ma C, Zhan C, Yuan H, Cui Y, Zhang Z. MicroRNA-603 functions as an oncogene by suppressing BRCC2 protein translation in osteosarcoma. Oncol Rep. (2016) 35:3257–64. doi: 10.3892/or.2016.4718
56. Zhang L, Wang Y, Zhang L, Xia X, Chao Y, He R, et al. ZBTB7A, a miR-663a target gene, protects osteosarcoma from endoplasmic reticulum stress-induced apoptosis by suppressing LncRNA GAS5 expression. Cancer Lett. (2019) 448:105–16. doi: 10.1016/j.canlet.2019.01.046
57. Wang J, Zhao H, Yu J, Xu X, Liu W, Jing H, et al. MiR-92b targets p57kip2 to modulate the resistance of hepatocellular carcinoma (HCC) to ionizing radiation (IR) -based radiotherapy. Biomed Pharmacother. (2019) 110:646–55. doi: 10.1016/j.biopha.2018.11.080
58. Sun TT, He J, Liang Q, Ren LL, Yan TT, Yu TC, et al. LncRNA GClnc1 promotes gastric carcinogenesis and may act as a modular scaffold of WDR5 and KAT2A complexes to specify the histone modification pattern. Cancer Discov. (2016) 6:784–801. doi: 10.1158/2159-8290.CD-15-0921
59. Blank-Giwojna A, Postepska-Igielska A, Grummt I. lncRNA KHPS1 activates a poised enhancer by triplex-dependent recruitment of epigenomic regulators. Cell Rep. (2019) 26:2904–15 e4. doi: 10.1016/j.celrep.2019.02.059
60. Cajigas I, Chakraborty A, Swyter KR, Luo H, Bastidas M, Nigro M, et al. The Evf2 ultraconserved enhancer lncRNA functionally and spatially organizes megabase distant genes in the developing forebrain. Mol Cell. (2018) 71:956–72 e9. doi: 10.1016/j.molcel.2018.07.024
61. Dimitrova N, Zamudio JR, Jong RM, Soukup D, Resnick R, Sarma K, et al. LincRNA-p21 activates p21 in cis to promote Polycomb target gene expression and to enforce the G1/S checkpoint. Mol Cell. (2014) 54:777–90. doi: 10.1016/j.molcel.2014.04.025
62. Monnier P, Martinet C, Pontis J, Stancheva I, Ait-Si-Ali S, Dandolo L. H19 lncRNA controls gene expression of the Imprinted Gene Network by recruiting MBD1. Proc Natl Acad Sci USA. (2013) 110:20693–8. doi: 10.1073/pnas.1310201110
63. Xu B, Konze KD, Jin J, Wang GG. Targeting EZH2 and PRC2 dependence as novel anticancer therapy. Exp Hematol. (2015) 43:698–712. doi: 10.1016/j.exphem.2015.05.001
64. Nasrollahzadeh-Khakiani M, Emadi-Baygi M, Nikpour P. Augmented expression levels of lncRNAs ecCEBPA and UCA1 in gastric cancer tissues and their clinical significance. Iran J Basic Med Sci. (2017) 20:1149–58. doi: 10.22038/IJBMS.2017.9448
65. Engreitz JM, Haines JE, Perez EM, Munson G, Chen J, Kane M, et al. Local regulation of gene expression by lncRNA promoters, transcription and splicing. Nature. (2016) 539:452–55. doi: 10.1038/nature20149
66. Yao H, Brick K, Evrard Y, Xiao T, Camerini-Otero RD, Felsenfeld G. Mediation of CTCF transcriptional insulation by DEAD-box RNA-binding protein p68 and steroid receptor RNA activator SRA. Genes Dev. (2010) 24:2543–55. doi: 10.1101/gad.1967810
67. Li Z, Chao TC, Chang KY, Lin N, Patil VS, Shimizu C, et al. The long noncoding RNA THRIL regulates TNFalpha expression through its interaction with hnRNPL. Proc Natl Acad Sci USA. (2014) 111:1002–7. doi: 10.1073/pnas.1313768111
68. Faghihi MA, Modarresi F, Khalil AM, Wood DE, Sahagan BG, Morgan TE, et al. Expression of a noncoding RNA is elevated in Alzheimer's disease and drives rapid feed- forward regulation of beta-secretase. Nat Med. (2008) 14:723–30. doi: 10.1038/nm1784
69. Deng L, Yang SB, Xu FF, Zhang JH. Long noncoding RNA CCAT1 promotes hepatocellular carcinoma progression by functioning as let-7 sponge. J Exp Clin Cancer Res. (2015) 34:18. doi: 10.1186/s13046-015-0136-7
70. Zhang R, Xia LQ, Lu WW, Zhang J, Zhu JS. LncRNAs and cancer (Review). Oncol Lett. (2016) 12:1233–9. doi: 10.3892/ol.2016.4770
71. Cifuentes-Rojas C, Hernandez AJ, Sarma K, Lee JT. Regulatory interactions between RNA and polycomb repressive complex 2. Mol Cell. (2014) 55:171–85. doi: 10.1016/j.molcel.2014.05.009
72. Guil S, Soler M, Portela A, Carrere J, Fonalleras E, Gomez A, et al. Intronic RNAs mediate EZH2 regulation of epigenetic targets. Nat Struct Mol Biol. (2012) 19:664–70. doi: 10.1038/nsmb.2315
73. Mohammad F, Mondal T, Guseva N, Pandey GK, Kanduri C. Kcnq1ot1 noncoding RNA mediates transcriptional gene silencing by interacting with Dnmt1. Development. (2010) 137:2493–9. doi: 10.1242/dev.048181
74. Merry CR, Forrest ME, Sabers JN, Beard L, Gao XH, Hatzoglou M, et al. DNMT1-associated long non-coding RNAs regulate global gene expression and DNA methylation in colon cancer. Hum Mol Genet. (2015) 24:6240–53. doi: 10.1093/hmg/ddv343
75. Arab K, Park YJ, Lindroth AM, Schafer A, Oakes C, Weichenhan D, et al. Long noncoding RNA TARID directs demethylation and activation of the tumor suppressor TCF21 via GADD45. Mol Cell A. (2014) 55:604–14. doi: 10.1016/j.molcel.2014.06.031
76. Guenther MG, Levine SS, Boyer LA, Jaenisch R, Young RA. A chromatin landmark and transcription initiation at most promoters in human cells. Cell. (2007) 130:77–88. doi: 10.1016/j.cell.2007.05.042
77. Ashe HL, Monks J, Wijgerde M, Fraser P, Proudfoot NJ. Intergenic transcription and transinduction of the human beta-globin locus. Genes Dev. (1997) 11:2494–509. doi: 10.1101/gad.11.19.2494
78. Feng J, Bi C, Clark BS, Mady R, Shah P, Kohtz JD. The Evf-2 noncoding RNA is transcribed from the Dlx-5/6 ultraconserved region and functions as a Dlx-2 transcriptional coactivator. Genes Dev. (2006) 20:1470–84. doi: 10.1101/gad.1416106
79. Martianov I, Ramadass A, Serra Barros A, Chow N, Akoulitchev A. Repression of the human dihydrofolate reductase gene by a non-coding interfering transcript. Nature. (2007) 445:666–70. doi: 10.1038/nature05519
80. Tay Y, Rinn J, Pandolfi PP. The multilayered complexity of ceRNA crosstalk and competition. Nature. (2014) 505:344–52. doi: 10.1038/nature12986
81. Muhammad F, Trivett A, Wang D, Lee DJ. Tissue specific production of MicroRNA-155 inhibits melanocortin 5 receptor dependent suppressor macrophages to promote experimental autoimmune uveitis. Eur J Immunol. (2019). doi: 10.1002/eji.201848073. [Epub ahead of print].
82. Wai CM, VanBuren R, Zhang J, Huang L, Miao W, Edger PP, et al. Temporal and spatial transcriptomic and microRNA dynamics of CAM photosynthesis in pineapple. Plant J. (2017) 92:19–30. doi: 10.1111/tpj.13630
83. Kim VN, Han J, Siomi MC. Biogenesis of small RNAs in animals. Nat Rev Mol Cell Biol. (2009) 10:126–39. doi: 10.1038/nrm2632
84. Gagnon KT, Corey DR. Argonaute and the nuclear RNAs: new pathways for RNA-mediated control of gene expression. Nucleic Acid Ther. (2012) 22:3–16. doi: 10.1089/nat.2011.0330
85. Nelson P, Kiriakidou M, Sharma A, Maniataki E, Mourelatos Z. The microRNA world: small is mighty. Trends Biochem Sci. (2003) 28:534–40. doi: 10.1016/j.tibs.2003.08.005
86. Mounir M, Lucchetta M, Silva TC, Olsen C, Bontempi G, Chen X, et al. New functionalities in the TCGAbiolinks package for the study and integration of cancer data from GDC and GTEx. PLoS Comput Biol. (2019) 15:e1006701. doi: 10.1371/journal.pcbi.1006701
87. Baril P, Ezzine S, Pichon C. Monitoring the spatiotemporal activities of miRNAs in small animal models using molecular imaging modalities. Int J Mol Sci. (2015) 16:4947–72. doi: 10.3390/ijms16034947
88. Zeng B, Li Y, Jiang F, Wei C, Chen G, Zhang W, et al. LncRNA GAS5 suppresses proliferation, migration, invasion, and epithelial-mesenchymal transition in oral squamous cell carcinoma by regulating the miR-21/PTEN axis. Exp Cell Res. (2019) 374:365–73. doi: 10.1016/j.yexcr.2018.12.014
89. Liolios T, Kastora SL, Colombo G. MicroRNAs in female malignancies. Cancer Inform. (2019) 18:1176935119828746. doi: 10.1177/1176935119828746
90. Gustafson CE, Cavanagh MM, Jin J, Weyand CM, Goronzy JJ. Functional pathways regulated by microRNA networks in CD8 T-cell aging. Aging Cell. (2019) 18:e12879. doi: 10.1111/acel.12879
91. Deng L, Wang J, Zhang J. Predicting gene ontology function of human microRNAs by integrating multiple networks. Front Genet. (2019) 10:3. doi: 10.3389/fgene.2019.00003
92. Wang J, Du Y, Liu X, Cho WC, Yang Y. MicroRNAs as regulator of signaling networks in metastatic colon cancer. Biomed Res Int. (2015) 2015:823620. doi: 10.1155/2015/823620
93. Cheetham SW, Gruhl F, Mattick JS, Dinger ME. Long noncoding RNAs and the genetics of cancer. Br J Cancer. (2013) 108:2419–25. doi: 10.1038/bjc.2013.233
94. Chung S, Nakagawa H, Uemura M, Piao L, Ashikawa K, Hosono N, et al. Association of a novel long non-coding RNA in 8q24 with prostate cancer susceptibility. Cancer Sci. (2011) 102:245–52. doi: 10.1111/j.1349-7006.2010.01737.x
95. Jiang D, Xu L, Ni J, Zhang J, Cai M, Shen L. Functional polymorphisms in LncRNA HOTAIR contribute to susceptibility of pancreatic cancer. Cancer Cell Int. (2019) 19:47. doi: 10.1186/s12935-019-0761-x
96. Zhu Y, Qiao L, Zhou Y, Ma N, Wang C, Zhou J. Long non-coding RNA FOXD2-AS1 contributes to colorectal cancer proliferation through its interaction with microRNA-185-5p. Cancer Sci. (2018) 109:2235–42. doi: 10.1111/cas.13632
97. Silva JM, Boczek NJ, Berres MW, Ma X, Smith DI. LSINCT5 is over expressed in breast and ovarian cancer and affects cellular proliferation. RNA Biol. (2011) 8:496–505. doi: 10.4161/rna.8.3.14800
98. Jendrzejewski J, He H, Radomska HS, Li W, Tomsic J, Liyanarachchi S, et al. The polymorphism rs944289 predisposes to papillary thyroid carcinoma through a large intergenic noncoding RNA gene of tumor suppressor type. Proc Natl Acad Sci USA. (2012) 109:8646–51. doi: 10.1073/pnas.1205654109
99. Han Y, Liu Y, Gui Y, Cai Z. Long intergenic non-coding RNA TUG1 is overexpressed in urothelial carcinoma of the bladder. J Surg Oncol. (2013) 107:555–9. doi: 10.1002/jso.23264
100. Wang X, Liu H, Maimaitiaili A, Zhao G, Li S, Lv Z, et al. Prevalence of BRCA1 and BRCA2 gene mutations in Chinese patients with high-risk breast cancer. Mol Genet Genomic Med. (2019) 7:e677. doi: 10.1002/mgg3.677
101. Fang Z, Zhao J, Xie W, Sun Q, Wang H, Qiao B. LncRNA UCA1 promotes proliferation and cisplatin resistance of oral squamous cell carcinoma by sunppressing miR-184 expression. Cancer Med. (2017) 6:2897–908. doi: 10.1002/cam4.1253
102. Wapinski O, Chang HY. Long noncoding RNAs and human disease. Trends Cell Biol. (2011) 21:354–61. doi: 10.1016/j.tcb.2011.04.001
103. Boeri M, Verri C, Conte D, Roz L, Modena P, Facchinetti F, et al. MicroRNA signatures in tissues and plasma predict development and prognosis of computed tomography detected lung cancer. Proc Natl Acad Sci USA. (2011) 108:3713–8. doi: 10.1073/pnas.1100048108
104. Jurkovicova D, Magyerkova M, Kulcsar L, Krivjanska M, Krivjansky V, Gibadulinova A, et al. miR-155 as a diagnostic and prognostic marker in hematological and solid malignancies. Neoplasma. (2014) 61:241–51. doi: 10.4149/neo_2014_032
105. Michael JV, Wurtzel JG, Mao GF, Rao AK, Kolpakov MA, Sabri A, et al. Platelet microparticles infiltrating solid tumors transfer miRNAs that suppress tumor growth. Blood. (2017) 130:567–80. doi: 10.1182/blood-2016-11-751099
106. Lin XJ, Chong Y, Guo ZW, Xie C, Yang XJ, Zhang Q, et al. A serum microRNA classifier for early detection of hepatocellular carcinoma: a multicentre, retrospective, longitudinal biomarker identification study with a nested case-control study. Lancet Oncol. (2015) 16:804–15. doi: 10.1016/S1470-2045(15)00048-0
107. Zhang JX, Song W, Chen ZH, Wei JH, Liao YJ, Lei J, et al. Prognostic and predictive value of a microRNA signature in stage II colon cancer: a microRNA expression analysis. Lancet Oncol. (2013) 14:1295–306. doi: 10.1016/S1470-2045(13)70491-1
108. Bagnoli M, Canevari S, Califano D, Losito S, Maio MD, Raspagliesi F, et al. Multicentre Italian Trials in Ovarian cancer translational, Development and validation of a microRNA-based signature (MiROvaR) to predict early relapse or progression of epithelial ovarian cancer: a cohort study. Lancet Oncol. (2016) 17:1137–46. doi: 10.1016/S1470-2045(16)30108-5
109. Lin HM, Castillo L, Mahon KL, Chiam K, Lee BY, Nguyen Q, et al. Circulating microRNAs are associated with docetaxel chemotherapy outcome in castration-resistant prostate cancer. Br J Cancer. (2014) 110:2462–71. doi: 10.1038/bjc.2014.181
110. Lin HM, Mahon KL, Spielman C, Gurney H, Mallesara G, Stockler MR, et al. Phase 2 study of circulating microRNA biomarkers in castration-resistant prostate cancer. Br J Cancer. (2017) 116:1002–11. doi: 10.1038/bjc.2017.50
111. Orlicka-Plocka M, Gurda D, Fedoruk-Wyszomirska A, Smolarek I, Wyszko E. Circulating microRNAs in cardiovascular diseases. Acta Biochim Pol. (2016) 63:725–9. doi: 10.18388/abp.2016_1334
112. Lin A, Hu Q, Li C, Xing Z, Ma G, Wang C, et al. The LINK-A lncRNA interacts with PtdIns(3,4,5)P3 to hyperactivate AKT and confer resistance to AKT inhibitors. Nat Cell Biol. (2017) 19:238–51. doi: 10.1038/ncb3473
113. Magistri M, Faghihi MA, St. Laurent G III, Wahlestedt C. Regulation of chromatin structure by long noncoding RNAs: focus on natural antisense transcripts. Trends Genet. (2012) 28:389–96. doi: 10.1016/j.tig.2012.03.013
114. Modarresi F, Faghihi MA, Lopez-Toledano MA, Fatemi RP, Magistri M, Brothers SP, et al. Inhibition of natural antisense transcripts in vivo results in gene-specific transcriptional upregulation. Nat Biotechnol. (2012) 30:453–9. doi: 10.1038/nbt.2158
115. Thorpe C, Epple S, Woods B, El-Sagheer AH, Brown T. Synthesis and biophysical properties of carbamate-locked nucleic acid (LNA) oligonucleotides with potential antisense applications. Org Biomol Chem. (2019) 17:5341–8. doi: 10.1039/C9OB00691E
116. Faghihi MA, Kocerha J, Modarresi F, Engstrom PG, Chalk AM, Brothers SP, et al. RNAi screen indicates widespread biological function for human natural antisense transcripts. PLoS ONE. (2010) 5:e13177. doi: 10.1371/journal.pone.0013177
117. Zhang W, Zhao H, Wu Q, Xu W, Xia M. Knockdown of BACE1-AS by siRNA improves memory and learning behaviors in Alzheimer's disease animal model. Exp Ther Med. (2018) 16:2080–6. doi: 10.3892/etm.2018.6359
118. Obad S, dos Santos CO, Petri A, Heidenblad M, Broom O, Ruse C, et al. Silencing of microRNA families by seed-targeting tiny LNAs. Nat Genet. (2011) 43:371–8. doi: 10.1038/ng.786
119. Janssen HL, Reesink HW, Lawitz EJ, Zeuzem S, Rodriguez-Torres M, Patel K, et al. Treatment of HCV infection by targeting microRNA. N Engl J Med. (2013) 368:1685–94. doi: 10.1056/NEJMoa1209026
120. van Zandwijk N, Pavlakis N, Kao SC, Linton A, Boyer MJ, Clarke S, et al. Safety and activity of microRNA-loaded minicells in patients with recurrent malignant pleural mesothelioma: a first-in-man, phase 1, open-label, dose-escalation study. Lancet Oncol. (2017) 18:1386–96. doi: 10.1016/S1470-2045(17)30621-6
121. Pramanik D, Campbell NR, Karikari C, Chivukula R, Kent OA, Mendell JT, et al. Restitution of tumor suppressor microRNAs using a systemic nanovector inhibits pancreatic cancer growth in mice. Mol Cancer Ther. (2011) 10:1470–80. doi: 10.1158/1535-7163.MCT-11-0152
122. Trang P, Wiggins JF, Daige CL, Cho C, Omotola M, Brown D, et al. Systemic delivery of tumor suppressor microRNA mimics using a neutral lipid emulsion inhibits lung tumors in mice. Mol Ther. (2011) 19:1116–22. doi: 10.1038/mt.2011.48
123. Wang ZF, Liao F, Wu H, Dai J. Glioma stem cells-derived exosomal miR-26a promotes angiogenesis of microvessel endothelial cells in glioma. J Exp Clin Cancer Res. (2019) 38:201. doi: 10.1186/s13046-019-1181-4
124. Ma Y, Deng F, Li P, Chen G, Tao Y, Wang H. The tumor suppressive miR-26a regulation of FBXO11 inhibits proliferation, migration and invasion of hepatocellular carcinoma cells. Biomed Pharmacother. (2018) 101:648–55. doi: 10.1016/j.biopha.2018.02.118
125. Zhao WT, Lin XL, Liu Y, Han LX, Li J, Lin TY, et al. miR-26a promotes hepatocellular carcinoma invasion and metastasis by inhibiting PTEN and inhibits cell growth by repressing EZH2. Lab Invest. (2019). doi: 10.1038/s41374-019-0270-5. [Epub ahead of print].
126. Zhang R, Xia T. Long non-coding RNA XIST regulates PDCD4 expression by interacting with miR-21-5p and inhibits osteosarcoma cell growth and metastasis. Int J Oncol. (2017) 51:1460–70. doi: 10.3892/ijo.2017.4127
127. Zheng R, Lin S, Guan L, Yuan H, Liu K, Liu C, et al. Long non-coding RNA XIST inhibited breast cancer cell growth, migration, and invasion via miR-155/CDX1 axis. Biochem Biophys Res Commun. (2018) 498:1002–8. doi: 10.1016/j.bbrc.2018.03.104
128. Chen DL, Ju HQ, Lu YX, Chen LZ, Zeng ZL, Zhang DS, et al. Long non-coding RNA XIST regulates gastric cancer progression by acting as a molecular sponge of miR-101 to modulate EZH2 expression. J Exp Clin Cancer Res. (2016) 35:142. doi: 10.1186/s13046-016-0420-1
129. Xu Y, Wang J, Wang J. Long noncoding RNA XIST promotes proliferation and invasion by targeting miR-141 in papillary thyroid carcinoma. Onco Targets Ther. (2018) 11:5035–43. doi: 10.2147/OTT.S170439
130. Sun N, Zhang G, Liu Y. Long non-coding RNA XIST sponges miR-34a to promotes colon cancer progression via Wnt/beta-catenin signaling pathway. Gene. (2018) 665:141–8. doi: 10.1016/j.gene.2018.04.014
131. Chang S, Chen B, Wang X, Wu K, Sun Y. Long non-coding RNA XIST regulates PTEN expression by sponging miR-181a and promotes hepatocellular carcinoma progression. BMC Cancer. (2017) 17:248. doi: 10.1186/s12885-017-3216-6
132. Li W, Yu H, Ding D, Chen Z, Wang Y, Wang S, et al. Cold atmospheric plasma and iron oxide-based magnetic nanoparticles for synergetic lung cancer therapy. Free Radic Biol Med. (2018) 130:71–81. doi: 10.1016/j.freeradbiomed.2018.10.429
133. Yan D, Xu W, Yao X, Lin L, Sherman JH, Keidar M. The cell activation phenomena in the cold atmospheric plasma cancer treatment. Sci Rep. (2018) 8:15418. doi: 10.1038/s41598-018-33914-w
134. Cheng Z, Li Z, Ma K, Li X, Tian N, Duan J, et al. Long non-coding RNA XIST promotes glioma tumorigenicity and angiogenesis by acting as a molecular sponge of miR-429. J Cancer. (2017) 8:4106–16. doi: 10.7150/jca.21024
135. Cheng Q, Xu X, Jiang H, Xu L, Li Q. Knockdown of long non-coding RNA XIST suppresses nasopharyngeal carcinoma progression by activating miR-491-5p. J Cell Biochem. (2018) 119:3936–44. doi: 10.1002/jcb.26535
136. Bayley JP, van Minderhout I, Hogendoorn PC, Cornelisse CJ, van der Wal A, Prins FA, et al. Sdhd and SDHD/H19 knockout mice do not develop paraganglioma or pheochromocytoma. PLoS ONE. (2009) 4:e7987. doi: 10.1371/journal.pone.0007987
137. Schorderet P, Duboule D. Structural and functional differences in the long non-coding RNA hotair in mouse and human. PLoS Genet. (2011) 7:e1002071. doi: 10.1371/journal.pgen.1002071
138. Zhang B, Arun G, Mao YS, Lazar Z, Hung G, Bhattacharjee G, et al. The lncRNA Malat1 is dispensable for mouse development but its transcription plays a cis-regulatory role in the adult. Cell Rep. (2012) 2:111–23. doi: 10.1016/j.celrep.2012.06.003
139. Nakagawa S, Naganuma T, Shioi G, Hirose T. Paraspeckles are subpopulation-specific nuclear bodies that are not essential in mice. J Cell Biol. (2011) 193:31–9. doi: 10.1083/jcb.201011110
140. Zhao X, Tang DY, Zuo X, Zhang TD, Wang C. Identification of lncRNA-miRNA-mRNA regulatory network associated with epithelial ovarian cancer cisplatin-resistant. J Cell Physiol. (2019) 234:19886–94. doi: 10.1002/jcp.28587
141. Miao L, Yin RX, Zhang QH, Hu XJ, Huang F, Chen WX, et al. A novel lncRNA-miRNA-mRNA triple network identifies lncRNA TWF1 as an important regulator of miRNA and gene expression in coronary artery disease. Nutr Metab. (2019) 16:39. doi: 10.1186/s12986-019-0366-3
142. Fan CN, Ma L, Liu N. Systematic analysis of lncRNA-miRNA-mRNA competing endogenous RNA network identifies four-lncRNA signature as a prognostic biomarker for breast cancer. J Transl Med. (2018) 16:264. doi: 10.1186/s12967-018-1640-2
143. Liu J, Li H, Zheng B, Sun L, Yuan Y, Xing C. Competitive endogenous RNA (ceRNA) regulation network of lncRNA-miRNA-mRNA in colorectal carcinogenesis. Dig Dis Sci. (2019) 64:1868–77. doi: 10.1007/s10620-019-05506-9
144. Yue B, Li H, Liu M, Wu J, Li M, Lei C, et al. Characterization of lncRNA-miRNA-mRNA network to reveal potential functional ceRNAs in bovine skeletal muscle. Front Genet. (2019) 10:91. doi: 10.3389/fgene.2019.00091
145. Ye S, Yang L, Zhao X, Song W, Wang W, Zheng S. Bioinformatics method to predict two regulation mechanism: TF-miRNA-mRNA and lncRNA-miRNA-mRNA in pancreatic cancer. Cell Biochem Biophys. (2014) 70:1849–58. doi: 10.1007/s12013-014-0142-y
146. Sun W, Lv J, Duan L, Lin R, Li Y, Li S, et al. Long noncoding RNA H19 promotes vascular remodeling by sponging let-7a to upregulate the expression of cyclin D1. Biochem Biophys Res Commun. (2019) 508:1038–42. doi: 10.1016/j.bbrc.2018.11.185
147. Hatakeyama H, Murata M, Sato Y, Takahashi M, Minakawa N, Matsuda A, et al. The systemic administration of an anti-miRNA oligonucleotide encapsulated pH-sensitive liposome results in reduced level of hepatic microRNA-122 in mice. J Control Release. (2014) 173:43–50. doi: 10.1016/j.jconrel.2013.10.023
148. Nguyen TC, Cao X, Yu P, Xiao S, Lu J, Biase FH, et al. Mapping RNA-RNA interactome and RNA structure in vivo by MARI. Nat Commun. (2016) 7:12023. doi: 10.1038/ncomms12023
149. Latysheva NS, Babu MM. Discovering and understanding oncogenic gene fusions through data intensive computational approaches. Nucleic Acids Res. (2016) 44:4487–503. doi: 10.1093/nar/gkw282
Keywords: long non-coding RNA, microRNA, biomarker, therapeutics, cancer
Citation: Dai X, Kaushik AC and Zhang J (2019) The Emerging Role of Major Regulatory RNAs in Cancer Control. Front. Oncol. 9:920. doi: 10.3389/fonc.2019.00920
Received: 21 July 2019; Accepted: 03 September 2019;
Published: 24 September 2019.
Edited by:
Zhaohui Huang, Affiliated Hospital of Jiangnan University, ChinaReviewed by:
Shakti Sahi, Gautam Buddha University, IndiaBarbara Pasculli, Casa Sollievo della Sofferenza (IRCCS), Italy
Copyright © 2019 Dai, Kaushik and Zhang. This is an open-access article distributed under the terms of the Creative Commons Attribution License (CC BY). The use, distribution or reproduction in other forums is permitted, provided the original author(s) and the copyright owner(s) are credited and that the original publication in this journal is cited, in accordance with accepted academic practice. No use, distribution or reproduction is permitted which does not comply with these terms.
*Correspondence: Xiaofeng Dai, eGlhb2ZlbmcuZGFpJiN4MDAwNDA7amlhbmduYW4uZWR1LmNu