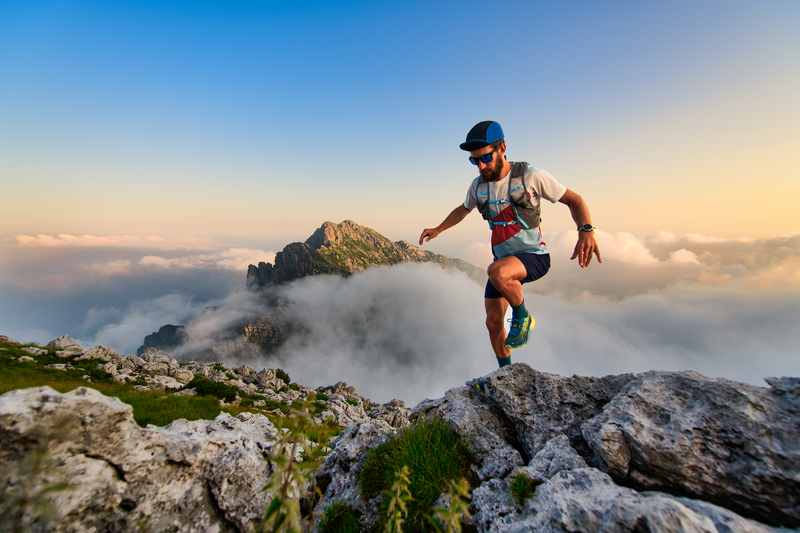
94% of researchers rate our articles as excellent or good
Learn more about the work of our research integrity team to safeguard the quality of each article we publish.
Find out more
ORIGINAL RESEARCH article
Front. Oncol. , 02 August 2019
Sec. Genitourinary Oncology
Volume 9 - 2019 | https://doi.org/10.3389/fonc.2019.00714
This article is part of the Research Topic Prostate Cancer: Molecular Entities Targeting This Challenging Disease View all 9 articles
Prostate cancer is the leading cause of cancer death among men globally, with castration development resistant contributing significantly to treatment failure and death. By analyzing the differentially expressed genes between castration-induced regression nadir and castration-resistant regrowth of the prostate, we identified soluble guanylate cyclase 1 subunit alpha as biologically significant to driving castration-resistant prostate cancer. A virtual screening of the modeled protein against 242 experimentally-validated anti-prostate cancer phytochemicals revealed potential drug inhibitors. Although, the identified four non-synonymous somatic point mutations of the human soluble guanylate cyclase 1 gene could alter its form and ligand binding ability, our analysis identified compounds that could effectively inhibit the mutants together with wild-type. Of the identified phytochemicals, (8′R)-neochrome and (8′S)-neochrome derived from the Spinach (Spinacia oleracea) showed the highest binding energies against the wild and mutant proteins. Our results identified the neochromes and other phytochemicals as leads in pharmacotherapy and as nutraceuticals in management and prevention of castration-resistance prostate cancers.
Malignancy of the prostate is the most commonly diagnosed cancer in men worldwide and ranked second as the cause of death, in cancer-related diseases (1). The burden of this disease is on the black population, who have one in four chance of getting the disease in their lifetime (2, 3). Hence, being black is a major risk factor for this disease and accounts for the disparity in the risk and outcome of the disease. Recent data suggest that the developing countries experience, and may continue to experience, disproportionate morbidity and mortality of this disease (4).
The development of both normal and malignant prostatic cells, as well as the proliferation of advanced carcinoma of the prostate, is highly dependent on androgens (5). Therefore, it has been hypothesized that androgens play a causal role in prostate tumorigenesis. Consequently, the primary therapeutic goal for prostate cancer is to reduce the levels of androgen (5). This is achieved through a group of treatment called androgen deprivation therapy. This treatment is universally accepted as the first line of treatment for prostate cancer, and it can be achieved either pharmacologically (chemical castration) or surgically (orchiectomy) (6). Despite these treatment options, a complication associated with a resurgence of androgen and elevation of prostate-specific antigen (PSA), arises toward the late stage of the disease. This state is referred to as castration-resistant prostate cancer (CRPC), and it is characterized by loss of ability to respond to androgen deprivation therapy and the recurrence of prostate cancer and subsequent metastasis (7). This recurrence of disease may occur in up to 40% of the patients (8, 9). For example, Bello (9) reported that 48 of 161 prostate cancer patients in Sub-Saharan Africa treated with androgen deprivation therapy developed CRPC, over a period of 4 years. Hence, CRPC continues to make prostate cancer the leading source of cancer mortality for men, particularly among the black race (4).
The transformation of hormone-dependent prostate cancer cells to castration-resistant ones is largely driven by upregulation of the activity of androgen receptor (10). This upregulation is often a consequence of: (1) the overexpression of the androgen receptor, which is observed in 22–30% of CRPC (11), (2) gain-of-function mutation of androgen receptor gene which occurs more often in 10–30% CRPC patients (12, 13), or (3) metabolic changes to the source of intratumoral dihydrotestosterone, as reviewed by Sharifi (14). Despite the cocktail of drugs targeting these well-established mechanisms, most of the pharmacological agents are ineffective against CRPC.
The limited treatment options for CRPC include secondary hormonal manipulations (with agents such as diethylstilbestrol, cyproterone and megestrol acetate), radiotherapy (with radium 223) (7, 15), drugs (such as docetaxel-which was approved by US Food and Drug Administration due to survival benefit only) (16) and novel drugs (like abiraterone, which has shown more promise in men of African ancestry in clinical trial) (17). Hence, CRPC continues to be a major factor contributing to the high mortality rate of prostate cancer, particularly in low resource countries, where the incidence of the disease is on the rise (3). In order to understand the factors that underlie the development of CRPC, previous studies have employed differential gene expression analysis to characterize the genetic and molecular factors that drive a prostate cell into being resistant to castration (18). It is therefore expedient to explore the available data in identifying novel targets, and subsequently, putative therapeutics for this disease in order to improve clinical outcomes and increase survival.
One significant and continuous source of novel drug-leads is the medicinal plants (19, 20). Phytochemicals derived from medicinal plants are structurally complex and diverse. Furthermore, many phytochemicals have been reported to possess cytotoxic properties and potentially useful as anti-cancer agents (21, 22). For example, Taxol, derived from Pacific yew tree (Taxus brevifolia) has been a success story and remains the best-selling anticancer drug for the treatment of ovarian cancer, breast cancer, and non-small cell lung cancer for up to four decades (20), as well as gastroesophageal, endometrial, cervical, prostate, and head and neck cancers (23). Also, Omacetaxine mepesuccinate originally derived from bark extracts of Cephalotaxus harringtonii and Cephalotaxus fortune with the trade name Synribo®, is used for the chronic myeloid leukemia (22). The success story has led to the clinical trials of over 100 natural products or natural product-derived compounds, the majority of which are on cancer treatment (24). Although a derivative of Toxol, Cabazitaxel®, is now in phase III clinical trial for the CRPC, the extension of life expectancy has only been by 3 months (25). Yet more phytochemicals have been suggested to be useful as preventive nutraceuticals and/or neo-adjuvant for prostate cancer in diverse populations (26, 27).
There is, therefore, need to use reverse pharmacology approach in developing the treatment for CRPC (28). To achieve this, this study analyzed the differentially expressed genes that drive CRPC and identified novel drug targets, as well as putative phytochemicals that can serve as inhibitors for the identified targets and its somatic variants.
The gene expression profile of GSE21887 (https://www.ncbi.nlm.nih.gov/geo/query/acc.cgi?acc=GSE21887) (18) was obtained from Gene expression omnibus (GEO) of the National Center for Biotechnology Information (NCBI). GSE21887 was based on GPL570 [HG-U133_Plus_2] Affymetrix Human Genome U133 Plus 2.0 Array. These data were derived from a xenograft model of prostate cancer, KUCaP-2, expressing wild-type androgen receptor and producing PSA. In order to identify the genes that drive the proliferation of prostate cancer cell following castration, we extracted data from eight chips for further analysis. These chips represented four samples of castration-induced regression nadir (GSM544233, GSM544234, GSM544235, and GSM544236) and compared with four samples of castration-resistant regrowth (GSM544237, GSM544238, GSM544239, and GSM544240).
The derived raw Affymetrix expression data were initially pre-processed and normalized and then analyzed to identify the differentially expressed genes using Limma package in R language (29). First, the raw data from the probe set were summarized by calculating the expression values for the probe set using Microarray Suite 5.0 (MAS5, the standard Affymetrix algorithm) in R (30, 31). Furthermore, we used the linear regression model in Limma package to compare the castration-induced regression nadir samples and castration-resistant regrowth samples. Only the genes with |logFC| > 2.0 and the p < 0.01 were chosen as differentially expressed genes. Out of the list of the differentially expressed genes, we considered the gene with the highest fold change and lowest p-value for further analysis. Hence, we carried out further analysis on the human soluble guanylate cyclase 1 subunit alpha 2 (GUCY1A2).
Human genes are often highly polymorphic, and protein mutants determine the outcome of therapy.
In order to identify the somatic genetic variants of human GUCY1A2 in prostate cancer, we downloaded its missense mutation data from the Genomic Data Commons (GDC) Portal of National Cancer Institute (https://portal.gdc.cancer.gov/) (32). The missense mutation data from GDC was downloaded with Variant Effect Predictor (VEP) (33), SIFT (34), and PolyPhen (35) results.
The amino acid sequence of wild-type human GUCY1A2 (Uniprot ID: P33402) was retrieved from the UniProt database (http://www.uniprot.org). This protein sequence was used for predicting the 3D structure of the wild and mutant human GUCY1A2 using SWISS-MODEL (https://swissmodel.expasy.org/) (36). SWISS-MODEL is a fully automated server for predicting the 3D structure of proteins using the crystal structure of the similar protein as the template. For this purpose, we used human guanylate cyclase soluble subunit alpha-3 (pdb ID: 3uvj.1.A) as a template.
The effect of the mutations on the stability of human GUCY1A2 protein was assessed using I-Mutant adaptation 2.0. I-Mutant is an internet support vector that evaluates mutation prompted adjustments in protein dependability (37). It estimates the free energy changes value (DDG) as the difference between the unfolding Gibbs free energy value (DG) for the wild-type protein and that of the mutant protein (DDG or DDG = DG mutant – DG wild-type). Potential (surge or reduction) in the DDG is also predicted, along with a reliability index (RI) for the results, where the lowest and highest reliability are 0 and 10, respectively (38). Meanwhile, project HOPE (www.cmbi.ru.nl/hope/) (39) and MutPred (http://mutpred.mutdb.org/) (40) were used to identify the structural and functional consequences of the mutations on the human GUCY1A2 protein.
Structural data of 242 experimentally-validated naturally occurring anti-prostate cancer compounds were obtained from Naturally Occurring Plant-based Anti-cancer Compound-Activity-Target (NPACT) dataset (http://crdd.osdd.net/raghava/npact/index.html) (41). The compounds and those of the protein variants were imported into Molegro Virtual Docker (MDV). MDV was used for structural optimization and virtual screening as earlier described (42).
Characteristics of theoretical ADME and toxicological effects of the phytochemicals were determined by in silico analysis, using the SwissADME software (43). SwissADME is an online computational tool that also allows the prediction of the following pharmacokinetic characteristics: gastrointestinal absorption (GI), P-glycoprotein (P-gp) substrate, the inhibitor of some cytochromes P450 (CYP) known to be regularly involved in the interactions with xenobiotics (CYP1A2, CYP2C19, CYP2C9, CYP2D6, and CYP3A423) and blood-brain barrier permeant (BBBP).
The normalized Affymetrix data were used to determine the biological significance of each gene in driving castration-induced regression of prostate cancer into castration-resistant regrowth. The results for genes with |logFC| > 2.0 and the p < 0.01 are presented in Table S1. Meanwhile, Figure 1 represents the volcano plot of the distribution of the level of expression of genes not just according to statistical significance but also biological significance, as demonstrated by fold change. The genes represented by points at the upper far right of the graph are those considered to be significantly important in driving the castration responsive prostate cancer cells into castration resistance. The analysis showed that GUCY1A2, GRIN3A, and SYT4 are the most biologically important genes involved in the pathogenesis of CRPC in this patient-derived xenograft model. This differential expression analysis identified GUCY1A2, as the most significantly upregulated gene and biologically important in driving prostate cancer from castration-induced regression to castration-resistant growth. Hence, it was selected as the putative drug target for virtual screening. This gene codes for one of the peptides that make up soluble guanylyl cyclase (sGC) (44). sGC is a heterodimeric hemoprotein that is made up of two alpha and two beta subunits and serves as the intracellular receptor for nitric oxide. It mediates the biological function of nitric oxide, resulting in the formation of 3′, 5′-cyclic guanosine monophosphate and activation of protein kinase G (45). However, the alpha subunit of this protein complex has now been recognized to be regulated by the androgen receptor, in a non-nitric oxide-dependent mechanism, to mediate the growth of prostate cancer, both in the presence or absence of physiological concentration of androgen (46). Cai et al. (46) further reported an elevated level of expression of the alpha subunit of sGC in hormone-refractory prostate cancer at both mRNA level and protein (47). This is consistent with the immunohistological data in the Human Protein Atlas (48), that show the localization and elevated expression of this protein at the cytoplasmic/membranous nuclear in high-grade prostate adenocarcinoma. A major mechanism by which sGCα promotes prostate cancer is by associating with and sequestering p53 in the cytoplasm, leading to suppression of apoptosis (46). This observation strongly suggests that sGCα is a drug-able target for CRPC.
Figure 1. Volcano plot of –log10 (p values) vs. log2 fold change. The –log10 (p values) represents the level of significance of each gene while log2 fold change represents the difference between the levels of expression for each gene between the castration-induced regression nadir and castration-resistant regrowth groups.
Interestingly, valproic acid, an anticonvulsant derived from Valeriana officinalis and shown to repress the expression of sGCα mRNA, has been reported by previous studies and a clinical trial to be useful in treating CRPC (49). However, this effect has solely been attributed to its histone deacetylases-inhibitory property (50, 51), without considering its anti-GUCY1A2 property. It is, therefore, possible that the suppression of GUCY1A2 is a complementary mechanism that was not explored by these previous investigators.
Although previous studies (44, 46, 52) have identified sGCα as a drug-able target for prostate cancer, our investigation represents the first to single it out as a major drug target, particularly, for CRPC. Again, these previous efforts have been directed at developing novel peptide that targets and inhibits sGCα activity (44, 52). However, therapeutic peptides have some significant drawbacks which include low membrane permeability, poor stability, and short half-life (53).
Since plant-derived chemicals have served, and continue to serve, as major sources for cancer chemotherapeutic and chemopreventive agents from time immemorial, we, therefore, exploited this vast resource of experimentally determined anti-prostate cancer phytochemicals to identify compounds or leads that could inhibit this protein.
The 242 plant-derived natural compounds with experimentally determined anti-prostate cancer activity were downloaded from NPACT database (41). The molecular docking results of the top 20 of these compounds are presented in Table 1. Also presented in the table is the chemical class of the compounds, the sources, and chemical structures. The highest number of the compounds are terpenoids with (8′R)-neochrome being the putatively most active compound. The binding energy and parameters of these compounds compared with methylene blue showed that all 20 phytochemicals have stronger binding energy than methylene blue (Table 2). However, the structural alterations induced by the somatic mutations alter the binding of these ligands. The compounds investigated have been experimentally validated for anti-prostate cancer activity, and because some are from edible plants, they may serve as neo-adjuvants or nutraceuticals in the prevention of CRPC (26). It is worthy of note that the phytochemicals we selected have higher binding energy to the modeled GUCY1A2 protein than methylene blue-which has been approved a pharmaceutical antagonist of guanylate cyclase (54). (8′R)-Neochrome being the compound with the higher binding energy −160.75 Kcal/mol, followed by (8′S)-neochrome (−152.102 Kcal/mol), 22-epicalamistrin (−151.131 Kcal/mol), 3-beta-O-(E)-feruloylbetulin (−145.363 Kcal/mol), 3-hydroxy-6′-desmethyl-9-O-methylthalifaboramine (−140.511 Kcal/mol) and Aculeatin A (−142.851 Kcal/mol).
The somatic mutations of GUCY1A2 that have been experimentally recorded in the prostate cancer were retrieved from TCGA and presented in Table 3. The mutations are R381Q, G723S, Q217H, and P676L. Polyphen recognizes all mutations as probably damaging while only R381Q is considered tolerated following SIFT analysis. However, as illustrated in Table 4, all the mutations could alter the stability of the protein, with P676L increasing the stability but with a low reliability index. Furthermore, functional analysis of the structural impact of these mutations predicted substantial alterations, not just in the function but also, in the ability of the protein to bind ligand (Table 5). This has clinical and pharmacological implications because such mutations now constitute a major problem resulting in the reduction of the efficacy of cancer chemotherapy. In order to account for this, we studied the effects of reported non-synonymous somatic mutation in GUCY1A2 on its protein structure and function. We further investigated the alterations in the binding energy between the phytochemicals and the mutant GUCY1A2 proteins. The phytochemicals have varying degrees of preference for different forms of this protein. We observed that none of the phytochemical could effectively bind all the mutant protein. (8′R)-neochrome, (8′S)-neochrome, 3-beta-O-(E)-feruloylbetulin, Foveoglin A, Foveoglin B, Longimicins A, Melianin C, Meliavolkinin, Silvestrol, and Vinblastine were able to effectively bind three of the four mutant protein, with the neochromes having the highest binding energy (Table 2). This phenomenon has been reported in different cancer drug-receptor interaction and often results in the reduction of drug efficiency or drug resistance and an important factor in drug pharmacogenomics (55). For example, somatic mutations in the aromatase gene CYP19 alter the efficacy of aromatase inhibitors when used as neoadjuvant therapy for breast cancer (56), while T790M mutation (rs121434569) reduces the effectiveness of Epidermal growth factor receptor inhibitors in treating lung adenocarcinomas (57). It is therefore of clinical importance to consider the somatic mutations in the drug receptors and target in the drug development process. Our results showed that (8′R/S)-Neochromes have a high binding affinity for all the variants of GUCY1A2 protein. (8′R/S)-Neochromes are neoxanthins that are naturally derived from vegetables such as spinach (Spinacia oleracea) during gastrointestinal digestion (58). Previous studies have reported the cytotoxic effects of Neochromes and their contribution to the anticancer effect of spinach (59, 60). Precisely, Kotake-Nara et al. (61) reported it to be cytotoxic against PC-3 human prostate cancer cells with IC50 of 1.2 μMol/L, and it has been suggested that p53 plays a minimal role in its mechanism of cytotoxicity (62). Although p53 protein is critical to cancer therapy due to its universal inactivation in human malignancies, the observation of Kotake-Nara et al. (61) and that of Cai et al. (46) imply that (8′R/S)-Neochrome may be potent in inducing apoptosis in cancer cells with or without p53 inactivation.
Table 5. Schematic structures of the original (left) and mutant (right) amino acid for each mutation with the functional consequence.
It is worthy of note that most of these phytochemicals failed the in silico drug-likeness test and showed poor gastrointestinal absorption (Table 6). However, this is not unusual of anticancer phytochemicals including polyphenols such as curcumin and green tea polyphenols; hence, it has been suggested that bioavailability of a compound cannot be accurately predicted (63). For many phytochemicals in this class, the uptake and efflux transporters at the epithelial cell surface also play a critical role in their bioavailability. One of such transporters that is relevant to cancer prevention and treatment is P-glycoprotein (64). Although some of these compounds are also P-glycoprotein substrates and this could eventually reduce their bioavailability, the coadministration of the P-glycoprotein inhibitors, such as erythromycin or clarithromycin, to patients receiving such P-glycoprotein substrate drugs have been noted to increase in their plasma and tissue concentrations (65). P-glycoprotein inhibitors have been employed as adjuncts in cancer chemotherapy, but their use in routine clinical practice is not approved due, in part, to inhibition of the CYP P-450 drug metabolizing enzyme (64). Our data also identified some of these compounds that do not inhibit CYP P-450 (Table 7). It is worthy of note that the Neochromes, although, are the substrate for P-glycoprotein, does not inhibit any of the CYP P-450 reported.
In conclusion, while these compounds may also have other targets in inducing cytotoxicity in prostate cancer, their ability to inhibit sGCα makes them more useful in addressing a complex disease like CRPC, rather than the usual “one gene, one target, one disease” approach which has limited the success of most anticancer drugs (66). The results of this investigation, therefore, suggest Neochrome a putative lead and possible nutraceutical in the treatment of CRPC.
Data generated from this study are contained in the manuscript and Supplementary File.
SR, OR, and AS conceived and designed the approach and methodology. Data Analysis and interpretation was carried out by SR, OR, and PJ. JO and EI contributed to the writing of the manuscript. All authors reviewed the manuscript.
The authors declare that the research was conducted in the absence of any commercial or financial relationships that could be construed as a potential conflict of interest.
The authors acknowledge the publication support of Covenant University Centre for Research, Innovation and Discovery (CUCRID).
The Supplementary Material for this article can be found online at: https://www.frontiersin.org/articles/10.3389/fonc.2019.00714/full#supplementary-material
Table S1. List of most significant differently expressed genes.
1. Zhou CK, Check DP, Lortet-Tieulent J, Laversanne M, Jemal A, Ferlay J, et al. Prostate cancer incidence in 43 populations worldwide: an analysis of time trends overall and by age group. Int J Cancer. (2016) 138:1388–400. doi: 10.1002/ijc.29894
2. Odedina FT, Akinremi TO, Chinegwundoh F, Roberts R, Yu D, Reams RR, et al. Prostate cancer disparities in Black men of African descent: a comparative literature review of prostate cancer burden among Black men in the United States, Caribbean, United Kingdom, and West Africa. Infect Agent Cancer. (2009) 4(Suppl. 1):S2. doi: 10.1186/1750-9378-4-S1-S2
3. Adeloye D, David RA, Aderemi AV, Iseolorunkanmi A, Oyedokun A, Iweala EE, et al. An estimate of the incidence of prostate cancer in Africa: a systematic review and meta-analysis. PLoS ONE. (2016) 11:e0153496 doi: 10.1371/journal.pone.0153496
4. Bray F, Ferlay J, Soerjomataram I, Siegel RL, Torre LA, Jemal A. Global cancer statistics 2018: GLOBOCAN estimates of incidence and mortality worldwide for 36 cancers in 185 countries. CA Cancer J Clin. (2018) 68:394–424. doi: 10.3322/caac.21492
5. Zhou Y, Bolton EC, Jones JO. Androgens and androgen receptor signaling in prostate tumorigenesis. J Mol Endocrinol. (2015) 54:R15–29. doi: 10.1530/JME-14-0203
6. Perlmutter MA, Lepor H. Androgen deprivation therapy in the treatment of advanced prostate cancer. Rev Urol. (2007) 9(Suppl. 1):S3–8.
7. Hotte SJ, Saad F. Current management of castrate-resistant prostate cancer. Curr Oncol. (2010) 17(Suppl. 2):S72–9. doi: 10.3747/co.v17i0.718
8. Petrylak DP. Current state of castration-resistant prostate cancer. Am J Manag Care. (2013) 19(18 Suppl):s358–65.
9. Bello JO. Natural history of castration-resistant prostate cancer in sub-Saharan African black men: a single-centre study of Nigerian men. Ecancermedicalscience. (2018) 12:797. doi: 10.3332/ecancer.2018.797
10. Coutinho I, Day TK, Tilley WD, Selth LA. Androgen receptor signaling in castration-resistant prostate cancer: a lesson in persistence. Endocr Relat Cancer. (2016) 23:T179–97. doi: 10.1530/ERC-16-0422
11. Urbanucci A, Sahu B, Seppala J, Larjo A, Latonen LM, Waltering KK, et al. Overexpression of androgen receptor enhances the binding of the receptor to the chromatin in prostate cancer. Oncogene. (2012) 31:2153–63. doi: 10.1038/onc.2011.401
12. Hay CW, McEwan IJ. The impact of point mutations in the human androgen receptor: classification of mutations on the basis of transcriptional activity. PLoS ONE. (2012) 7:e32514. doi: 10.1371/journal.pone.0032514
13. Eisermann K, Wang D, Jing Y, Pascal LE, Wang Z. Androgen receptor gene mutation, rearrangement, polymorphism. Transl Androl Urol. (2013) 2:137–47. doi: 10.3978/j.issn.2223-4683.2013.09.15
14. Sharifi N. Mechanisms of androgen receptor activation in castration-resistant prostate cancer. Endocrinology. (2013) 154:4010–7. doi: 10.1210/en.2013-1466
15. Al-Asaaed S, Winquist E. Secondary hormonal manipulation in castration resistant prostate cancer. Can J Urol. (2014) 21(2 Suppl. 1):37–41.
16. Chen Y, Clegg NJ, Scher HI. Anti-androgens and androgen-depleting therapies in prostate cancer: new agents for an established target. Lancet Oncol. (2009) 10:981–91. doi: 10.1016/S1470-2045(09)70229-3
17. George DJ, Heath EI, Sartor AO, Sonpavde G, Berry WR, Healy P, et al. Abi race: a prospective, multicenter study of black (B) and white (W) patients (pts) with metastatic castrate resistant prostate cancer (mCRPC) treated with abiraterone acetate and prednisone (AAP). Am Soc Clin Oncol. (2018) 36(18_suppl):LBA5009.
18. Terada N, Shimizu Y, Kamba T, Inoue T, Maeno A, Kobayashi T, et al. Identification of EP4 as a potential target for the treatment of castration-resistant prostate cancer using a novel xenograft model. Cancer Res. (2010) 70:1606–15. doi: 10.1158/0008-5472.CAN-09-2984
19. Cragg GM, Newman DJ. Natural products: a continuing source of novel drug leads. Biochim Biophys Acta. (2013) 1830:3670–95. doi: 10.1016/j.bbagen.2013.02.008
20. Zulkipli IN, David SR, Rajabalaya R, Idris A. Medicinal plants: a potential source of compounds for targeting cell division. Drug Target Insights. (2015) 9:9–19. doi: 10.4137/DTI.S24946
21. Orang-Ojong BB, Munyangaju JE, Wei MS, Lin M, Wei FG, Foukunang C, et al. Impact of natural resources and research on cancer treatment and prevention: a perspective from Cameroon. Mol Clin Oncol. (2013) 1:610–20. doi: 10.3892/mco.2013.132
22. Seca AML, Pinto D. Plant secondary metabolites as anticancer agents: successes in clinical trials and therapeutic application. Int J Mol Sci. (2018) 19:E263. doi: 10.3390/ijms19010263
23. Weaver BA. How taxol/paclitaxel kills cancer cells. Mol Biol Cell. (2014) 25:2677–81. doi: 10.1091/mbc.e14-04-0916
24. Butler MS, Robertson AA, Cooper MA. Natural product and natural product derived drugs in clinical trials. Nat Prod Rep. (2014) 31:1612–61. doi: 10.1039/C4NP00064A
25. Paller CJ, Antonarakis ES. Cabazitaxel: a novel second-line treatment for metastatic castration-resistant prostate cancer. Drug Des Devel Ther. (2011) 5:117–24. doi: 10.2147/DDDT.S13029
26. Reed D, Raina K, Agarwal R. Nutraceuticals in prostate cancer therapeutic strategies and their neo-adjuvant use in diverse populations. NPJ Precis Oncol. (2018) 2:15. doi: 10.1038/s41698-018-0058-x
27. Wang Z, Fan J, Liu M, Yeung S, Chang A, Chow MS, et al. Nutraceuticals for prostate cancer chemoprevention: from molecular mechanisms to clinical application. Expert Opin Investig Drugs. (2013) 22:1613–26. doi: 10.1517/13543784.2013.833183
28. Surh YJ. Reverse pharmacology applicable for botanical drug development - inspiration from the legacy of traditional wisdom. J Tradit Complement Med. (2011) 1:5–7. doi: 10.1016/S2225-4110(16)30051-7
29. Diboun I, Wernisch L, Orengo CA, Koltzenburg M. Microarray analysis after RNA amplification can detect pronounced differences in gene expression using limma. BMC Genomics. (2006) 7:252. doi: 10.1186/1471-2164-7-252
30. Su AI, Cooke MP, Ching KA, Hakak Y, Walker JR, Wiltshire T, et al. Large-scale analysis of the human and mouse transcriptomes. Proc Natl Acad Sci USA. (2002) 99:4465–70. doi: 10.1073/pnas.012025199
31. Li J, Xu YH, Lu Y, Ma XP, Chen P, Luo SW, et al. Identifying differentially expressed genes and small molecule drugs for prostate cancer by a bioinformatics strategy. Asian Pac J Cancer Prev. (2013) 14:5281–6. doi: 10.7314/APJCP.2013.14.9.5281
32. Deng M, Bragelmann J, Schultze JL, Perner S. Web-TCGA: an online platform for integrated analysis of molecular cancer data sets. BMC Bioinformatics. (2016) 17:72. doi: 10.1186/s12859-016-0917-9
33. McLaren W, Gil L, Hunt SE, Riat HS, Ritchie GR, Thormann A, et al. The ensembl variant effect predictor. Genome Biol. (2016) 17:122. doi: 10.1186/s13059-016-0974-4
34. Kumar P, Henikoff S, Ng PC. Predicting the effects of coding non-synonymous variants on protein function using the SIFT algorithm. Nat Protoc. (2009) 4:1073–81. doi: 10.1038/nprot.2009.86
35. Adzhubei I, Jordan DM, Sunyaev SR. Predicting functional effect of human missense mutations using PolyPhen-2. Curr Protoc Hum Genet. (2013) Chapter 7:Unit7 20. doi: 10.1002/0471142905.hg0720s76
36. Biasini M, Bienert S, Waterhouse A, Arnold K, Studer G, Schmidt T, et al. SWISS-MODEL: modelling protein tertiary and quaternary structure using evolutionary information. Nucleic Acids Res. (2014) 42(Web Server issue):W252–8. doi: 10.1093/nar/gku340
37. Capriotti E, Calabrese R, Casadio R. Predicting the insurgence of human genetic diseases associated to single point protein mutations with support vector machines and evolutionary information. Bioinformatics. (2006) 22:2729–34. doi: 10.1093/bioinformatics/btl423
38. Mavroconstanti T, Johansson S, Winge I, Knappskog PM, Haavik J. Functional properties of rare missense variants of human CDH13 found in adult attention deficit/hyperactivity disorder (ADHD) patients. PLoS ONE. (2013) 8:e71445. doi: 10.1371/journal.pone.0071445
39. Venselaar H, Te Beek TA, Kuipers RK, Hekkelman ML, Vriend G. Protein structure analysis of mutations causing inheritable diseases. An e-Science approach with life scientist friendly interfaces. BMC Bioinformatics. (2010) 11:548. doi: 10.1186/1471-2105-11-548
40. Li B, Krishnan VG, Mort ME, Xin F, Kamati KK, Cooper DN, et al. Automated inference of molecular mechanisms of disease from amino acid substitutions. Bioinformatics. (2009) 25:2744–50. doi: 10.1093/bioinformatics/btp528
41. Mangal M, Sagar P, Singh H, Raghava GP, Agarwal SM. NPACT: naturally occurring plant-based anti-cancer compound-activity-target database. Nucleic Acids Res. (2013) 41(Database issue):D1124–9. doi: 10.1093/nar/gks1047
42. Dawood S, Zarina S, Bano S. Docking studies of antidepressants against single crystal structure of tryptophan 2, 3-dioxygenase using Molegro Virtual Docker software. Pak J Pharm Sci. (2014) 27(5 Spec no):1529–39.
43. Daina A, Michielin O, Zoete V. SwissADME: a free web tool to evaluate pharmacokinetics, drug-likeness and medicinal chemistry friendliness of small molecules. Sci Rep. (2017) 7:42717. doi: 10.1038/srep42717
44. Gao S, Hsieh CL, Bhansali M, Kannan A, Shemshedini L. A peptide against soluble guanylyl cyclase alpha1: a new approach to treating prostate cancer. PLoS ONE. (2013) 8:e64189. doi: 10.1371/journal.pone.0064189
45. Bellamy TC, Wood J, Garthwaite J. On the activation of soluble guanylyl cyclase by nitric oxide. Proc Natl Acad Sci USA. (2002) 99:507–10. doi: 10.1073/pnas.012368499
46. Cai C, Chen SY, Zheng Z, Omwancha J, Lin MF, Balk SP, et al. Androgen regulation of soluble guanylyl cyclasealpha1 mediates prostate cancer cell proliferation. Oncogene. (2007) 26:1606–15. doi: 10.1038/sj.onc.1209956
47. Krumenacker JS, Hanafy KA, Murad F. Regulation of nitric oxide and soluble guanylyl cyclase. Brain Res Bull. (2004) 62:505–15. doi: 10.1016/S0361-9230(03)00102-3
48. Uhlen M, Zhang C, Lee S, Sjostedt E, Fagerberg L, Bidkhori G, et al. A pathology atlas of the human cancer transcriptome. Science. (2017) 357:eaan2507. doi: 10.1126/science.aan2507
49. Zhang R, Zhou J, Ren J, Sun S, Di Y, Wang H, et al. Transcriptional and splicing dysregulation in the prefrontal cortex in valproic acid rat model of autism. Reprod Toxicol. (2018) 77:53–61. doi: 10.1016/j.reprotox.2018.01.008
50. Lee JE, Kim JH. Valproic acid inhibits the invasion of PC3 prostate cancer cells by upregulating the metastasis suppressor protein NDRG1. Genet Mol Biol. (2015) 38:527–33. doi: 10.1590/S1415-475738420150028
51. Sharma S, Symanowski J, Wong B, Dino P, Manno P, Vogelzang N. A phase II clinical trial of oral valproic acid in patients with castration-resistant prostate cancers using an intensive biomarker sampling strategy. Transl Oncol. (2008) 1:141–7. doi: 10.1593/tlo.08136
52. Zhou J, Gao S, Hsieh CL, Malla M, Shemshedini L. Peptide B targets soluble guanylyl cyclase alpha1 and kills prostate cancer cells. PLoS ONE. (2017) 12:e0184088. doi: 10.1371/journal.pone.0184088
53. Wu D, Gao Y, Qi Y, Chen L, Ma Y, Li Y. Peptide-based cancer therapy: opportunity and challenge. Cancer Lett. (2014) 351:13–22. doi: 10.1016/j.canlet.2014.05.002
54. Masaki E, Kondo I. Methylene blue, a soluble guanylyl cyclase inhibitor, reduces the sevoflurane minimum alveolar anesthetic concentration and decreases the brain cyclic guanosine monophosphate content in rats. Anesth Analg. (1999) 89:484–9. doi: 10.1213/00000539-199908000-00045
55. Moen EL, Godley LA, Zhang W, Dolan ME. Pharmacogenomics of chemotherapeutic susceptibility and toxicity. Genome Med. (2012) 4:90. doi: 10.1186/gm391
56. Wang L, Ellsworth KA, Moon I, Pelleymounter LL, Eckloff BW, Martin YN, et al. Functional genetic polymorphisms in the aromatase gene CYP19 vary the response of breast cancer patients to neoadjuvant therapy with aromatase inhibitors. Cancer Res. (2010) 70:319–28. doi: 10.1158/0008-5472.CAN-09-3224
57. Yu HA, Arcila ME, Harlan Fleischut M, Stadler Z, Ladanyi M, Berger MF, et al. Germline EGFR T790M mutation found in multiple members of a familial cohort. J Thorac Oncol. (2014) 9:554–8. doi: 10.1097/JTO.0000000000000052
58. Asai A, Terasaki M, Nagao A. An epoxide-furanoid rearrangement of spinach neoxanthin occurs in the gastrointestinal tract of mice and in vitro: formation and cytostatic activity of neochrome stereoisomers. J Nutr. (2004) 134:2237–43. doi: 10.1093/jn/134.9.2237
59. Maeda N, Yoshida H, Mizushina Y. Spinach and health: anticancer effect. In: R. R. Watson, V. R. Preedy editors. Bioactive Foods in Promoting Health. London: Elsevier (2010). p. 393–405.
60. Terasaki M, Mutoh M, Fujii G, Takahashi M, Ishigamori R, Masuda S. Potential ability of xanthophylls to prevent obesity-associated cancer. World J Pharmacol. (2014) 3:140–52. doi: 10.5497/wjp.v3.i4.140
61. Kotake-Nara E, Asai A, Nagao A. Neoxanthin and fucoxanthin induce apoptosis in PC-3 human prostate cancer cells. Cancer Lett. (2005) 220:75–84. doi: 10.1016/j.canlet.2004.07.048
62. Liu JS, Chiang TH, Wang JS, Lin LJ, Chao WC, Inbaraj BS, et al. Induction of p53-independent growth inhibition in lung carcinoma cell A549 by gypenosides. J Cell Mol Med. (2015) 19:1697–709. doi: 10.1111/jcmm.12546
63. Aqil F, Munagala R, Jeyabalan J, Vadhanam MV. Bioavailability of phytochemicals and its enhancement by drug delivery systems. Cancer Lett. (2013) 334:133–41. doi: 10.1016/j.canlet.2013.02.032
64. Ughachukwu P, Unekwe P. Efflux pump-mediated resistance in chemotherapy. Ann Med Health Sci Res. (2012) 2:191–8. doi: 10.4103/2141-9248.105671
65. Eberl S, Renner B, Neubert A, Reisig M, Bachmakov I, Konig J, et al. Role of p-glycoprotein inhibition for drug interactions: evidence from in vitro and pharmacoepidemiological studies. Clin Pharmacokinet. (2007) 46:1039–49. doi: 10.2165/00003088-200746120-00004
Keywords: castration-resistant, prostate cancer, phytochemicals, soluble guanylate cyclase, gene expression data
Citation: Rotimi SO, Rotimi OA, Salako AA, Jibrin P, Oyelade J and Iweala EEJ (2019) Gene Expression Profiling Analysis Reveals Putative Phytochemotherapeutic Target for Castration-Resistant Prostate Cancer. Front. Oncol. 9:714. doi: 10.3389/fonc.2019.00714
Received: 02 June 2019; Accepted: 18 July 2019;
Published: 02 August 2019.
Edited by:
Sanjay V. Malhotra, Stanford University, United StatesReviewed by:
Sanja Štifter, University of Rijeka, CroatiaCopyright © 2019 Rotimi, Rotimi, Salako, Jibrin, Oyelade and Iweala. This is an open-access article distributed under the terms of the Creative Commons Attribution License (CC BY). The use, distribution or reproduction in other forums is permitted, provided the original author(s) and the copyright owner(s) are credited and that the original publication in this journal is cited, in accordance with accepted academic practice. No use, distribution or reproduction is permitted which does not comply with these terms.
*Correspondence: Solomon Oladapo Rotimi, b2xhLnJvdGltaUBjb3ZlbmFudHVuaXZlcnNpdHkuZWR1Lm5n
Disclaimer: All claims expressed in this article are solely those of the authors and do not necessarily represent those of their affiliated organizations, or those of the publisher, the editors and the reviewers. Any product that may be evaluated in this article or claim that may be made by its manufacturer is not guaranteed or endorsed by the publisher.
Research integrity at Frontiers
Learn more about the work of our research integrity team to safeguard the quality of each article we publish.