- 1SOTIO a.s, Prague, Czechia
- 2Department of Immunology, 2nd Faculty of Medicine and University Hospital Motol, Charles University, Prague, Czechia
Radiotherapy (RT) plays an important role in the management of cancer patients. RT is used in more than 50% of patients during the course of their disease in a curative or palliative setting. In the past decades it became apparent that the abscopal effect induced by RT might be dependent on the activation of immune system, and that the induction of immunogenic cancer cell death and production of danger-associated molecular patterns from dying cells play a major role in the radiotherapy-mediated anti-tumor efficacy. Therefore, the combination of RT and immunotherapy is of a particular interest that is reflected in designing clinical trials to treat patients with various malignancies. The use of cytokines as immunoadjuvants in combination with RT has been explored over the last decades as one of the immunotherapeutic combinations to enhance the clinical response to anti-cancer treatment. Here we review mainly the data on the efficacy of IFN-α, IL-2, IL-2-based immunocytokines, GM-CSF, and TNF-α used in combinations with various radiotherapeutic techniques in clinical trials. Moreover, we discuss the potential of IL-15 and its analogs and IL-12 cytokines in combination with RT based on the efficacy in preclinical mouse tumor models.
Introduction
The radiation therapy or radiotherapy (RT) started to be used as a cancer treatment modality soon after the discovery of X-rays in 1895 by Wilhelm Röntgen and Marie Curie's discovery of the radioactive elements polonium and radium in 1898. More than 100 years later, RT plays an important role in the therapy of cancer patients and represents a part of the management of more than 50% of patients during the course of their disease. RT is generally used as a primary therapy of localized tumors and regional lymph nodes in a curative setting but also as a palliative treatment to alleviate symptoms or for local control of metastasis. Ionizing radiation is frequently administered in combination with other treatment modalities such as surgery, chemotherapy, hyperthermia, hormone therapy, or immunotherapy (1, 2). RT can be administered as a neoadjuvant intervention to decrease the tumor size, intra-operatively to gain access to neoplastic lesions in a particularly complicated anatomic location or as an adjuvant treatment to prevent disease relapse (3). The most common cancer indications for RT include tumors of breast, lung, cervix uteri, endometrium, stomach, prostate, leukemia, lymphomas, skin, brain, or head and neck (4).
RT utilizes ionizing radiation that delivers its energy via photons, protons, and electrons. High doses of ionizing radiation are employed to kill tumor cells or slow their growth by inducing DNA damage and block the cell division. This process may take days and weeks of treatment before DNA is damaged enough for cancer cells to die, and the cancer cells keep dying over weeks after the termination of radiation treatment. The amount of absorbed radiation in photon RT is measured as joules per kilogram, expressed in the unit gray (Gy) and applied doses vary depending on the cancer type and stage of cancer being treated. The curative use of local ionizing radiation aims at achieving the cancer cells elimination while causing the least toxicity to normal adjacent tissues. The total dose of ionizing radiation is applied in fractions which refers to the delivery of the prescribed dose during separate radiation sessions, usually once per day (5). This provides time to normal healthy cells to recover, while tumor cells are generally less efficient in repair between fractions. Similarly, fractionation can sensitize tumor cells to RT by inducing reoxygenation or shifting the tumor cells to a radiation-sensitive phase of the cell cycle. Fractionation regimens are individualized for different clinical applications. Nevertheless, the standard fractionation schedule (“normofractionation”), which is based on extensive clinical empirical evidence, involves doses of 1.8–2 Gy per day, 5 days a week. The total cumulative dose can differ based on the tumor radiosensitivity and can range from 20 to 40 Gy for lyphomas, from 45 to 60 Gy for most tumor types to control microscopic disease after surgical resection or preoperatively in a neoadjuvant approach and from 60 to 80 Gy for curative purposes in some types of solid epithelial tumors (5). Modified fractionation schedules such as hyperfractionation or hypofractionation are also used. Hyperfractionation involves increasing the number of fractions per day while the dose per fraction becomes lower. This was shown to be beneficial in fast growing tumors such as head and neck squamous cell carcinoma (HNSCC) (6). Hypofractionation means lowering the number of fractions per weak while increasing the dose. Hypofractionation schedules are used in palliative treatments of i.e., bone metastasis (7). Similarly, radiation schedules applying single high radiation doses are employed in stereotactic radiosurgery (SRS) for brain metastasis (8).
RT comprises a wide range of various techniques which are used in dependence on the type of cancer, size of the tumor, anatomic location of the tumor, proximity of the tumor to normal tissue sensitive to radiation, or how radiation is applied to target. The use of RT techniques also depends on the patient's medical history and general health. RT can be broadly divided into 2 groups in dependence on how the radioactivity is applied to target malignant lesions: external-beam radiotherapy (EBRT) and internal radiotherapy (1). EBRT is the most common RT, which is applied on malignant lesions through the intact skin. The internal radiotherapy can be further divided into brachytherapy and systemic RT. In brachytherapy, the radiation source is placed directly at the site of the tumor. Tumors can be treated with very high doses of localized radiation with low probability of damage to the surrounding healthy tissues. This might provide an advantage over EBRT in certain clinical settings. Systemic radioisotope therapy is based on the distribution of a radionuclide or on radioisotopes attached to a tumor-targeting antibody or another tumor-targeting molecule (1). RT has several side effects which are, except for fatigue, associated with the anatomical location of irradiated volumes of the RT fields (4). RT-induced side effects can be broadly divided into early toxicities, occurring during or shortly after the end of RT treatment and late toxicities. Late toxicities occur at least 6 month after the end of RT treatment and are often irreversible (4). Over the last two decades, new RT techniques in the field have been developed and made accessible to cancer patients in routine clinical practice to improve the therapeutic efficacy of RT and to lessen the RT-related toxicities. This involves the introduction of intensity-modulated radiotherapy (IMRT), image-guided radiotherapy (IGRT), stereotactic radiotherapy (SRT), or proton or carbon beam therapy (4). These techniques can greatly increase the therapeutic efficiency by localizing the radiation effect to the target volume, steeper dose gradient, and utilization of imaging methods which leads to lower toxicity to normal tissue and shorter treatment duration (4, 9).
Radiation-Induced Effects on Tumors and Immune System
Historically, it was thought that RT exerts immunosuppressive effects. However, in the light of recent research it has been shown that the interaction with immune system is much more complex (10). Unfortunately, the immune response against tumor cells elicited by local RT alone is mostly insufficient to eliminate all tumor cells. In successful RT treatments, beside the direct effect on the irradiated cells, there has been also observed tumor regression in sites distant to the irradiated field called abscopal effect (from the Latin ab scopus—away from the target) (11). The abscopal response following radiation is rare in the clinic. Despite millions of patients treated worldwide between 1969 and 2014, the abscopal effect of RT was reported only in 46 cases (11). Recently, more frequent abscopal responses were observed in patients refractory to immunotherapy with checkpoint inhibitors (ICIs) alone, who then received RT in combination with ICIs, e.g., with ipilimumab as reported by Postow et al. (12). The abscopal effect was also observed in patients undergoing RT in combination with other immunotherapeutic approaches such as cytokine therapy, Toll-like receptor (TLR) agonists or adoptive cell transfer therapy (2, 13–15).
On the molecular level, radiation causes DNA damage directly and indirectly by means of induced free radicals. Cytoplasmic double-stranded DNA (dsDNA) is detected by a cytosolic dsDNA sensor cyclic GMP-AMP synthase (cGAS). cGAS is a pattern recognition receptor that triggers IFN-I production via the downstream adaptor stimulator of interferon genes (STING) and is critical for activation of immune response to viruses (16). The RT-induced damage to the cells leads to the exposure and/or release of several damage-associated molecular pattern (DAMP) molecules such as plasma membrane-exposed calreticulin, HMGB1, and ATP during the radiation-induced immunogenic cell death (17). These molecules attract and activate dendritic cells to phagocytose dying tumor cells, to process and present released tumor antigens to T cells (17). Particularly, BATF3-tumor-infiltrating dendritic cells are stimulated by autocrine production of interferon β (IFN-β) upon detecting cell-derived dsDNA via the cGAS-STING pathway (18). Activated BATF-3-dendritic cells then migrate to tumor draining lymph nodes where they can prime CD8+ T cells to initiate cytotoxic T cell response. Cytotoxic CD8+ T cells migrate to the irradiated tumor and eliminate the residual cancer cells as well as to distant metastatic sites which can lead to a systemic tumor regression, the abscopal effect (10). Besides increasing immunogenicity of tumor cells by inducing immunogenic cell death, RT improves also the access of chemotherapeutic agents and leukocytes into the tumor sites. RT can change the immunosuppressive tumor environment by triggering expression of MHC class I, NKG2D ligands, or FAS/CD95 on tumor cells. RT can stimulate secretion of various proinflammatory cytokines or release of biologically active molecules such as reactive oxygen species and nitrogen species that can act locally to promote cell death of bystander cells (10, 19, 20). On the other hand, RT can hinder the development of anti-tumor immunity by promoting the immunosuppressive tumor microenvironment. Several mechanisms have been documented. Some cytokines, chemokines or growth factors such as tumor growth factor-β (TGF-β), the chemokine C-C motif ligand 2 (CCL2), colony-stimulating factor 1 (CSF-1), C-X-C motif chemokine ligand 12 (CXCL12), or insulin-like growth factor 1 (IRF1) are induced by RT in the tumor environment. These molecules can attract and drive differentiation of immunosuppressive populations such as M2 macrophages, myeloid-derived suppressor cells (MDSC) or directly inhibit the function of immune cells (10, 21). RT can also activate the hypoxia-inducible factor 1α (HIF-1α) which upregulates genes that control angiogenesis, metabolism and metastasis (22). Similarly, some RT regimens lead to upregulation of DNA exonuclease three-prime repair exonuclease 1 (TREX1) which cleaves RT-induced dsDNA and limits the cGAS/STING/IFN-β pathway induction (23). Interestingly, the upregulation of TREX1 was dependent on the RT regimen used. Whereas, 6 Gy and 8 Gy doses enhanced dsDNA without increasing TREX1, 20 Gy induced a prominent upregulation of TREX1 (24). This suggests that the upregulation of TREX1 is determined by the dose of a single RT fraction and not by the total dose applied. The more detailed mechanisms of RT-induced immune activation or suppression are summarized in Table 1. Radiation, specifically in combination with immunotherapy, alters the balance between immune-activating and immune-suppressive signals in the tumor microenvironment, however it seems that the success of RT is determined by the intrinsic immunogenicity of the tumor, the type of radiation dose and fractionation regimen and the type of immunotherapy agent used (10).
In 2016, there were 95 clinical trials reported which examined the combinatorial effect of RT and immunotherapy. These trials included mainly ICIs, but also immunostimulatory antibodies, anti-cancer vaccines, oncolytic viruses, TLR agonists, indoleamine 2,3-dioxygenase 1 (IDO1) inhibitors, recombinant cytokines, adoptively transferred cells, and several small molecules with immunostimulatory effects (2, 46). So far, the clinical data of the effective synergy of RT and immunotherapy and reported abscopal effects are largely limited mainly to the combination of anti-CTLA-4 and RT in melanoma (2, 20, 47). Beside the ICIs, clinically significant abscopal effects were observed in a study where the combination of RT and GM-CSF in various tumor types resulted in overall response rate of 26% (11 patients out of 41) (14). The interest in cytokine therapy has been recently renewed mainly by the development of improved IL-2 analogs and IL-15 agonist being currently tested in clinical trials showing lower toxicity and improved therapeutic window (48, 49). Therefore, the main focus of this review is to summarize the data on the anti-tumor effects of combining RT and recombinant cytokines obtained in preclinical testing, but mainly in clinical trials. We discuss the effectivity of RT and cytokine treatment, and potential pitfalls and benefits together with future directions for research on RT and cytokine combinatorial treatment.
Cytokines in Cancer Immunotherapy
Cytokines belong to a large diverse family of small glycoproteins that regulate a plethora of physiological functions in a paracrine, autocrine and endocrine manner. They play a crucial role in regulation of the innate and adaptive immunity. The development of recombinant protein technology allowed their use in modulating various pathophysiological conditions including their use in cancer treatment. Despite efforts to develop systemic anti-cancer treatment with cytokines as a standalone therapy, there are several limitations in the form of severe dose-limiting toxicities and generally low objective response rates (durable responses are approximately 10% for a systemic high dose IL-2 therapy). To circumvent this, cytokines are being investigated clinically using novel engineered cytokine mutants (superkines) or chimeric antibody-cytokine fusion proteins (immunocytokines) (50, 51).
To date only few cytokines have been licensed for clinical use in a limited number of oncologic indications. Namely, recombinant IFN-α2a, IFN-α2b, interleukin (IL)-2, granulocyte colony-stimulating factor (G-SCF), granulocyte monocyte colony-stimulating factor (GM-CSF), and tumor necrosis factor α (TNF-α) (50). IFN-α2a is approved for the treatment of hairy cell leukemia and chronic myelogenous leukemia, IFN-α2b for follicular lymphoma, multiple myeloma, AIDS-related Kaposi's sarcoma, melanoma, cervical intraepithelial neoplasms, and hairy cell leukemia, and IL-2 for the treatment of metastatic melanoma and renal cell carcinoma. G-CSF and GM-CSF are approved as immunoreconstituting agents and TNF-α as an oncotoxic factor rather than to augment the anti-tumor immune responses (50). Besides the approved cytokines, there are other cytokines such as IL-12, IL-21, IL-7, IL-15, IFN-γ, IL-8, and IL-18 tested in anti-cancer treatment in clinical trials. These cytokines were tested either as monotherapy but mostly to use their immunoadjuvant potential to boost the effectivity of other therapeutic agents (50, 51). Data on the combination of RT and cytokine treatment are available only for IFN-α, IL-2, IL-15, GM-CSF, TNF-α, and IL-12, out of which the potential effects of IL-15 and IL-12 with RT have not yet been explored in patients. The summary of clinical trials combining cytokine treatment with some form of RT is shown in Table 2. The simplified immune cell-enhancing mode of action of these cytokines in combination with RT treatment is depicted in Figure 1.
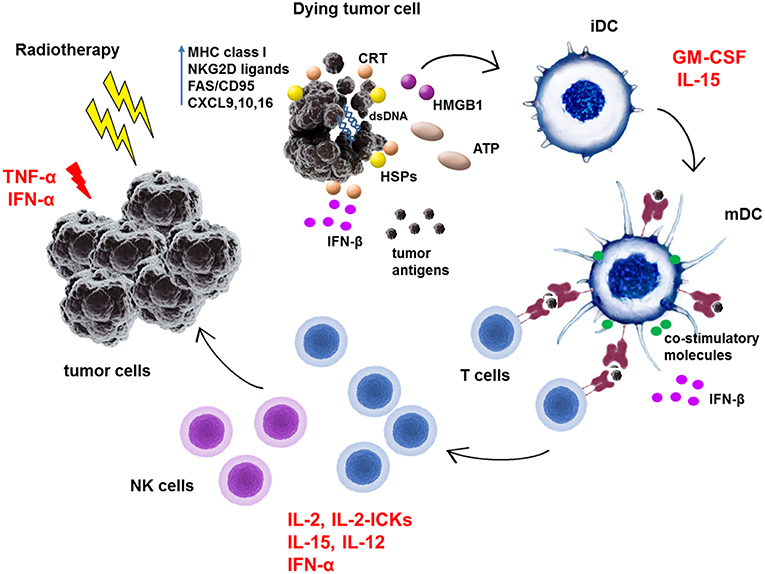
Figure 1. Schematic representation of immunoadjuvant effects of cytokines in combination with radiotherapy treatment of tumors. Radiotherapy was shown to stimulate anti-tumor immunity by increasing expression of MHC class I molecules, NKG2D ligands or FAS/CD95 in tumor cells. RT induces production of chemokines such as CXCL9, 10, and 16 in tumor cells which attract effector T cells to tumor site. RT induces immunogenic cell death and exposure and release of danger-associated molecular patterns (DAMPs) such as calreticulin, HMGB1, ATP, or heat-shock proteins (HSPs). Moreover, RT-generated dsDNA activates via cGAS/STING pathway the IFN-β production in tumor cells as well as in dendritic cells (DC). All of these molecules facilitate the phagocytosis of dead tumor cells and uptake of released tumor antigens by DC. They activate immature dendritic cells (iDC) to process antigens, enhance the expression of MHC class I and II molecules and co-stimulatory molecules such as CD80, CD86, CD83 on its surface to become mature dendritic cells (mDC). In lymph nodes, mDC prime T cells to become effector cells which then exert direct cytotoxic effects on tumor cells or generate the proinflammatory milieu in tumors. TNF-α and IFN-α can directly exert apoptotic effects on tumor cells. GM-CSF mainly activates dendritic cells. IL-2, IL-2 immunocytokines (IL-2-ICKs), IFN-α, and IL-15 activate directly T cells and NK cells to become cytotoxic effector cells. Moreover, IL-15 can bind to its high affinity receptor IL-15Rα on DC and as a part of immunological synapse can enhance T cell functions.
Interleukin 2 (IL-2)
IL-2 is a 15.5 kDa glycoprotein composed of four amphipathic α-helixes. IL-2 mediates signaling via three subunits of its IL-2 receptor which include the γ chain (γc) (CD132) shared with IL-4, IL-7, IL-9, IL-15, and IL-21, the IL-2Rβ (CD122) shared with IL-15 and the IL-2Rα (CD25) chain. IL-2 signals via its high affinity receptors IL-2Rαβγ and via its intermediate affinity receptors, IL-2Rγβ. IL-2 promotes expansion of antigen-activated CD8+ T cells, acts as an important CD4+ T cell and NK cell growth factor and boosts antibody production in B cells. It activates NK cells and promotes differentiation and proliferation of memory CD8+ T cells. On the other hand, IL-2 plays a crucial role in negative regulation of T cell responses by maintaining and activating regulatory T cells and by inducing Fas-mediated activation-induced cell death (AICD) of T cells (51, 71). At low doses, IL-2 activates mainly regulatory T cells via its high affinity receptors and this might be convenient for treatment of autoimmune diseases (72). At high doses IL-2 induces cytolytic activity of T cells and NK cells involving also signaling via its intermediate affinity receptors on target cells (73). IL-2 was approved by FDA for immunotherapy of metastatic renal cell carcinoma in 1992 and metastatic melanoma in 1998 (48).
Several studies mainly in metastatic melanoma or renal cell carcinoma have been conducted over the last two decades combining IL-2 and various doses and techniques of RT, however, generally with a low efficacy or partial responses.
The combination of rapid fractionation radiation up to 20 Gy followed within 24 h by IL-2 treatment in 28 metastatic patients showed a good tolerability. Four patients showed a significant shrinkage of the tumor at the irradiated site and 2 patients showed an abscopal effect outside the irradiation field (54). Low-dose total body irradiation exhibited a synergistic immune-mediated anti-tumor effect when used in combination with IL-2 in a murine metastatic malignant melanoma model (74–76). Based on this preclinical data a phase II clinical trial combining IL-2 with RT was conducted in metastatic melanoma (52). Forty-five patients received a maximum of 2 cycles of high dose subcutaneous IL-2 and low-dose total body irradiation (single radiation fraction of 0.1 Gy on days 1, 8, 22, and 30). Of note, 0.1 Gy total body irradiation is not comparable to clinical RT. The treatment was well-tolerated but the clinical efficacy was low. In this study, an increase in percentage of cells expressing IL-2Rβ (CD122), an increase in NK cells and a decrease of B cells and monocytes was observed (52). A pilot study assessing the safety and response rate combination of SBRT followed by a high-dose IL-2 regimen in patients with metastatic melanoma and renal cell carcinoma showed complete response or partial response in 8 out of 12 patients (53). Currently, there are two ongoing phase II studies to assess the treatment with SABR in combination with a high dose IL-2 regimen for patients with metastatic renal carcinoma (NCT01896271, NCT02306954), one phase II trial for patients with metastatic melanoma (NCT01416831) and one phase II study for both patients with metastatic melanoma and renal cell carcinoma (55) (NCT01884961). Several additional clinical studies examining SABR in combination with IL-2 in metastatic melanoma and renal cell carcinoma are underway (Table 2).
Triple combinations of IL-2 administration, RT and immune checkpoint blockade is currently designed in two clinical trials as well as IL-2 and RT combined with autologous DC vaccine (NCT03226236) (Table 2). Nivolumab and Ipilimumab with IL-2 and RT will be tested in pilot phase I trial for patients with metastatic NSCLC (NCT03224871) and pembrolizumab in phase I/II trial for patients with various metastatic tumors (NCT03474497).
In recent years, new derivatives of IL-2 such as PEGylated IL-2 (NKTR-214) or IL-2 conjugated with tumor-targeting antibodies (IL-2-based immunocytokines) have found their way to the clinical testing (77, 78). More preclinical data is needed to evaluate the potential of NKTR-214 and RT combination. Currently there are no clinical trials in progress to combine NKTR-214 with RT, but some data have been already collected for IL-2 based immunocytokines.
IL-2-based Immunocytokines
IL-2-based immunocytokines tested with RT involve L19-IL2 and NHS-IL2. Both immunocytokines showed promising results in preclinical models (79, 80). L19-IL-2 (Darleukin) is a conjugate of IL-2 and L19 an antibody fragment targeting extracellular domain B of fibronectin (ED-B). L19-IL-2 in combination with RT showed efficacy in preclinical mouse models (81–83). A long-lasting synergistic effect was observed in C51 colon tumor model with 75% of tumors cured (82). The induction of an abscopal effect was observed as well as an increase in memory CD44+CD127+ T cells. These preclinical findings set base for the initiation of phase I clinical trial in patients with metastatic solid tumors (NCT02086721), and phase II trial for stage IV NSCLC patients (currently withdrawn) (NCT02735850) (Table 2).
NHS-IL2 (selectikine) is an IL-2-based conjugate of a human antibody (NHS76) targeting necrotic tissue (non-membrane-enclosed DNA/histone complexes) fused to genetically modified human IL-2 which selectively targets the high affinity IL-2 receptor (84). Similarly to L19-IL-2, NHS-IL2 was tested in LLC lung carcinoma animal model to examine the efficacy when combined with RT and cisplatin. Mice were treated with NHS-IL2 alone (5 mg/kg; days 7–9), fractionated radiation (3.6 Gy; days 0–4) plus cisplatin (4 mg/kg; day 0), or the triple combination. Tumor regression was observed in 80% of mice when treated with RT and NHS-IL2 and in almost 100% mice when treated with the triple combination (84). Based on these results, a phase I clinical trial in patients with stage IV NSCLC (NCT00879866) was conducted. Patients received local irradiation (5 × 4 Gy) of a single pulmonary nodule. Dose-escalated NHS-IL2 was administered as 1 h intravenous infusion on three consecutive days every 3 weeks. The treatment was well-tolerated and in 2 out of 13 patients it achieved long term survival (84).
Interleukin 15 (IL-15)
Interleukin I5 (IL-15) is a 15 kDa cytokine structurally similar to IL-2. It belongs to the four-α-helix bundle family of cytokines. The IL-15 receptor involves the γc subunit, IL-15Rβ shared with IL-2 and IL-15 specific subunit IL-15Rα. Mainly monocytes, macrophages and dendritic cells produce IL-15. This cytokine induces proliferation of various effector cells including NK cells and CD8+ T cells via mechanism called trans-presentation (85, 86). Soluble IL-15 binds to its IL-15Rα subunit located on the surface of antigen presenting cells, mainly dendritic cells, and then it is ligated to IL-15Rβγ receptors on target cells. IL-15 also supports the IgG production from B cells and activation and maintenance of memory CD8+ T cells. Even though IL-15 displays a similar effect on immune cells as IL-2, there are major differences. Unlike IL-2, IL-15 exerts anti-apoptotic effects on cells and does not expand regulatory T cells (51). IL-15 is the only cytokine found to correlate with the progression-free survival in colorectal cancer patients and with immune cell density within tumors (87). The NCI review listed IL-15 as the most promising cytokine among 12 other immunotherapeutic agents that could potentially cure cancer (88).
Recombinant human IL-15 has been tested in clinical trials as a monotherapy (89, 90), but no patient data are available on its combination with RT. In preclinical research it has been shown that IL-15 can potentiate immune activation induced by RT (91). Poorly immunogenic TSA breast cancer tumors were treated with RT (locally in 8 Gy fractions on days 13, 14, and 15), IL-15 (2 μg/mouse daily for 10 days starting on day 12), or a combination of RT and IL-15. The highest survival was observed in the RT and IL-15 combination group (median 102 days) with 1 of 6 mice showing complete tumor rejection and a development of a long-lasting immunity. Moreover, a significant infiltration of T cells was detected (91).
Similarly to IL-2, there have been various analogs of IL-15 developed to increase the anti-tumor efficacy and lower the toxicity (49). ALT-803 is a mutated IL-15 (N72D) to enhance its biological activity bound to an IL-15RαSu/Fc fusion protein (92, 93). ALT-803 has been evaluated for safety and efficacy in a few clinical trials (94, 95) including combinatorial clinical trials with ICIs. However, the data on the combination of ALT-803 and RT are available only from one preclinical study (96). Here ALT-803 was combined with a SRS in murine glioblastoma model. However, no synergistic effect was observed. More data is necessary to evaluate the effectivity of IL-15 or IL-15 analogs in cancer treatment in combination with RT.
Granulocyte Macrophage Colony-Stimulating Factor (GM-CSF)
Granulocyte macrophage colony-stimulating factor (GM-CSF) is a 23 kDa glycoprotein that binds to a heterodimeric receptor which consists of subunits belonging to the type 1 cytokine receptor family (51). GM-CSF stimulates production of monocytes, neutrophils, and eosinophils. It is produced by various types of cells including T and B lymphocytes, neutrophils, eosinophils, epithelial cells, fibroblasts, and other cells (97). GM-CSF stimulates antigen presentation to the immune system by directly acting on dendritic cells and macrophages (98). GM-CSF was also shown to stimulate the capacity of neutrophils, macrophages and monocytes to mediate antibody-dependent cytotoxicity (51). In contrast to data from preclinical mouse models, the adjuvant effects of GM-CSF in human trials were inconsistent. This may be explained by the capacity of GM-CSF on one hand to stimulate dendritic cells, and on the other hand also to induce myeloid suppressor cells (99).
However, preclinical data show that GM-CSF in combination with RT can help boost the abscopal effect (100). Similarly, the abscopal effect of GM-CSF and RT in a patient with metastatic pancreatic cancer has been documented (101). Based on the preclinical data there had been several clinical trials conducted investigating combination of RT and GM-CSF. A proof-of-principle trial showed that 11 out of 41 patients with various metastatic diseases, developed abscopal responses (14) (NCT02474186). This trial set base for an ongoing phase II trial combining carbon ion RT and GM-CSF for patients with hepatocellular carcinoma (NCT02946138). GM-CSF in combination with RT is also combined or planned to be combined with additional agents (Table 2). GM-CSF is planned to be combined with pembrolizumab and RT (1 × 8 Gy) in follicular lymphoma (NCT02677155) or with pembrolizumab, GVAX (GM-CSF gene-transduced tumor cell vaccine) and SBRT in patients with locally advanced pancreatic cancer (NCT02648282). There is a phase II study investigating combination of GM-CSF with hypofractionated IMRT and temozolomide for patients with glioblastoma multiforme (NCT02663440). Another immune enhancer, thymosine-α, is investigated with this combination of GM-CSF and SABR in phase II trial for patients with stage IV NSCLC (NCT02976740). Similarly, in glioblastoma there is a study planning the intratumoral addition of polyI:C together with GM-CSF and RT (NCT03392545). A phase I/II study combining chemoradiotherapy with a herpes simplex type 1 oncolytic virus expressing GM-CSF in patients with HNSCC was conducted (57). The treatment was well-tolerated, 14 out of 17 patients showed response to the treatment and pathologic complete remission was confirmed in 93% of patients at neck dissection. GM-CSF treatment was also used in combination with RT and PSA tumor antigen-encoding poxviral vaccines (58) or with multipeptide vaccines (59) where an increase of antigen-specific T cells was detected in comparison to control arms.
Interferon Alpha (IFN-α)
Interferon alpha (IFN-α) is member of the type I interferon family and acts as immune modulator with antiviral and anti-proliferative properties (102, 103). Twenty IFNs have been identified in humans, out of which the most subtypes belong to the IFN-α group. Type I IFNs signal via a common pair of receptors, IFNAR1 and IFNAR2. IFN-α is produced mainly by plasmacytoid dendritic cells but also by most of other cell types in response to encounter with DAMPs by pattern recognition receptors (PRRs) which can be produced by virus-infected or cancer cells (104, 105). IFN-α induces MHC class I expression on tumor cells, induces apoptosis of tumor cells, mediates maturation of dendritic cells and activation of B and T cells, and displays antiangiogenic properties (51).
IFN-α has been approved by FDA for treatment of several malignancies including hairy cell leukemia, chronic myelogenous leukemia, follicular lymphomas, malignant melanoma, multiple myeloma, or renal cell carcinoma (106, 107) but displays also significant toxicity and side effects such as flu-like symptoms, anorexia, fatigue, depression. It has been shown in in vitro models that IFN-α has a synergistic cytotoxic effect with chemotherapy and RT (108, 109). This synergistic effect as well as radiosensitizing effect of 5-fluorouracil was also observed in patients with small cell lung cancer and anal cancer (110, 111). A phase I/II study combining 5-fluorouracil, cisplatin, IFN-α and concurrent EBRT before resection in patients with advanced esophageal cancer resulted in 80% of the patients responding to the therapy but the authors claimed that the contribution of IFN-α to the treatment was uncertain (62). In a preliminary phase II study, patients with pancreatic cancer underwent similar adjuvant therapy of 5-fluorouracil, cisplatin, IFN-α and RT after pancreaticoduodenectomy (63). The study showed better survival in the group of patients receiving IFN-α in comparison with patients with similar adjuvant therapy without IFN-α. In a similar multicenter phase II study (NCT00059826), patients with pancreatic cancer undergoing this adjuvant therapy had better overall survival results but the study had to be terminated prematurely due to the high toxicity of treatment (61). Acceptable toxicity was observed in phase II clinical trial (NCT01276730) where patients with stage III cervical cancer were treated with RT in combination with IFN-α and retinoic acid (60). Unfortunately, there was no survival advantage in comparison with the group receiving RT and cisplatin. In melanoma, there have been several trials examining treatment with IFN-α and various forms of RT with mixed outcomes involving high toxicity of the combinatorial treatment as well as generally low efficacy. These studies are summarized extensively in the review of Barker and Postow (112).
A phase II study investigating the combination of RT and IFN-α with a dendritic cell-based vaccine for patients with metastatic melanoma is currently ongoing (NCT01973322). Two other clinical studies combining chemotherapy and/or cellular therapy and IFN-α have been completed (NCT 00003195 and NCT00003263) (Table 2).
Tumor Necrosis Factor α (TNF-α)
Tumor necrosis factor alpha (TNF-α) is a strong proinflammatory cytokine with anti-tumor activity both in vitro and in vivo (113, 114). TNF-α is produced mainly by macrophages, granulocytes and epithelial cells but also by other types of cells and its anti-tumor properties are due to direct cytotoxic and antiangiogenic effects (70). Also it has been shown that TNF-α acts as a radiosentitizer and enhances cytotoxic effect of radiation (115). Despite of its anti-tumor properties, the systemic administration of TNF-α in a sufficient dose is associated with a high toxicity (116, 117). Thereby the use of TNF-α in cancer therapy is limited to isolated limb perfusion (ILP) of advanced melanoma and soft tissue sarcoma (118, 119). The only clinical testing of TNF-α and RT involves gene therapy delivering TNF-α gene to cancer cells—TNFerade™ (120, 121). TNFerade is an adenovector containing TNF-α gene with early growth response gene (Egr-1) radiation activated promoter that is injected intratumorally. In preclinical models this combination showed remarkable anti-tumor effects with minimal toxicity (122). Several trials combining TNFerade and RT or chemoradiotherapy were conducted in patients with various types of tumors including breast, lung, pancreatic, head and neck, rectal cancer, melanoma, esophageal cancer, or soft tissue sarcoma (64–67, 69, 70, 123, 124). These studies showed that the treatment is well tolerated with complete or partial tumor responses and complete tumor regressions in some patients (Table 2). Despite these favorable results a phase III clinical trial randomizing patients with locally advanced pancreatic cancer to 2:1 groups standard of care (SOC) plus TNFerade vs. SOC alone showed no survival benefit of the patients in SOC plus TNFerade group (68). This dampened the enthusiasm of using this approach and there are currently no open clinical trials (Table 2).
Interleukin 12 (IL-12)
IL-12 is a four-bundle α-helix heterodimeric cytokine encoded by two genes: IL-12A (p35 subunit) and IL-12B (p40 subunit). The active IL-12 forms a heterodimer of p35 and p40 subunits referred as p70. The receptor for IL-12 consists of IL-12Rβ1 and IL-12Rβ2. IL-12 is produced by macrophages, dendritic cells and B cells. IL-12 induces proliferation of T cells and NK cells as well as their IFN-γ production. IL-12 polarizes Th1 immune response and displays antiangiogenic properties (51). The anti-tumor efficacy of IL-12 was shown in several animal models (125–127). IL-12 has shown promising results in preclinical studies but the clinical trials did not result in satisfactory outcome. As IL-12 displays a high systemic toxicity, the local treatment in the form of gene or viral therapy was tested in combination with RT in preclinical models (128, 129). A non-viral murine IL-2 and IL-12 gene therapy and external beam radiation (2 × 1 Gy) was tested in HNSCC in an orthotopic murine model (130). A significant increase in anti-tumor effects and T lymphocyte infiltration was detected in comparison to single therapies and the control. Furthermore, the anti-tumor and anti-metastatic activity of the oncolytic adenovirus expressing IL-12 and GM-CSF injected intratumorally in combination with RT was investigated in a murine hepatic cancer (HCa-I) model (131). This combinatorial therapy was effective in suppressing primary tumor growth and an increased immune cell infiltration was observed. The therapeutic effect of the naked IL-12 cytokine combined with fractionated RT was investigated in Lewis lung carcinoma mouse model (132). The treatment was effective against primary tumor and the number of lung metastasis decreased. A pronounced tumor growth delay was observed when GM-CSF was added together with IL-12 and fractionated RT. IL-12, similarly to IL-2, has been fused to an antibody tumor-necrosis targeting IgG1 (NHS76) to create a novel immunocytokine NHS-IL12 (133). NHS-IL12 immunocytokine exhibited a longer half-life and a selective tumor targeting in vivo. NHS-IL12 showed a superior anti-tumor effect when combined with RT in MC38 mouse colorectal cancer model (133). Currently there are no clinical trials combining IL-12 cytokine therapy or NHS-IL12 immunocytokine with RT.
Conclusions and Future Perspectives
Cancer immunology has made a remarkable progress, which led to the development of various immunotherapies that can be combined with ionizing radiation. The combination of RT and immunotherapy represents a growing field of clinical investigation with an increasing number and various types of clinical trials (2). Despite partial therapeutic success of the combination of RT and immunotherapy including the rare abscopal effect, most patients do not respond to RT and immunotherapy, and the same goes for using cytokine adjuvant treatment and RT. For RT itself, there are important challenges to overcome and there is a need to conduct rigorous research. These include the selection of appropriate radiation dose, fractionation, appropriate technique for RT, sequencing of therapies and selection of meaningful endpoints in clinical trials (134). Radiation dose and regimen is likely to be a critical determinant in successful generation of an anti-tumor response. Radiation dose and regimen largely affect both the immunomodulatory and cytotoxic effects of RT. These might attenuate the immunosuppressive environment but might not induce the immunogenic cell death of cancer cells to elicit strong anti-tumor responses. In the same line, although the cytokine therapy as documented with IL-2 can induce significant durable responses in patients, there are strong limitations, which lie mainly in the toxicity after the systemic administration. This can be mitigated by intratumoral administration of cytokines, which on the other hand, might represent technical challenges for the clinicians, or targeted versions of cytokines (e.g., immunocytokines). Out of the clinically tested cytokines IL-2, IFN-α, GM-CSF, and TNF-α, overall only IL-2 and GM-CSF combinatorial treatment with RT showed some even clinically relevant efficacy with acceptable toxicity. Novel analogs of IL-2 engineered to mitigate the toxic effects and to reduce the induction of immunosuppressive T regulatory cells might increase the efficacy of treatment with radiation. Similarly, analogs of IL-15 showing promising results in clinical trials when combined with ICIs hold the potential to boost the immunogenic effect of RT. From this summary, it becomes clear that multiple combinations using cytokines and RT together with some other immunotherapeutic approaches might hold the promise to increase the clinical benefit of cancer patients. As we are only beginning to explore the possibilities of multiple immunotherapeutic combinations, the understanding how to best integrate the scientific rationale, mode of actions and the most effective therapeutic regimens remains of urgent need.
Author Contributions
All authors listed have made a substantial, direct and intellectual contribution to the work, and approved it for publication.
Conflict of Interest Statement
The authors are employees of Sotio a.s. None of the authors has any potential financial conflict of interest related to this manuscript.
References
1. Vacchelli E, Vitale I, Tartour E, Eggermont A, Sautes-Fridman C, Galon J, et al. Trial watch: anticancer radioimmunotherapy. Oncoimmunology. (2013) 2:e25595. doi: 10.4161/onci.25595
2. Kang J, Demaria S, Formenti S. Current clinical trials testing the combination of immunotherapy with radiotherapy. J Immunother Cancer. (2016) 4:51. doi: 10.1186/s40425-016-0156-7
3. Bloy N, Pol J, Manic G, Vitale I, Eggermont A, Galon J, et al. Trial watch: radioimmunotherapy for oncological indications. Oncoimmunology. (2014) 3:e954929. doi: 10.4161/21624011.2014.954929
4. Ahmad SS, Duke S, Jena R, Williams MV, Burnet NG. Advances in radiotherapy. BMJ. (2012) 345:e7765. doi: 10.1136/bmj.e7765
5. Demaria S, Formenti SC. Radiation as an immunological adjuvant: current evidence on dose and fractionation. Front Oncol. (2012) 2:153. doi: 10.3389/fonc.2012.00153
6. Beckmann GK, Hoppe F, Pfreundner L, Flentje MP. Hyperfractionated accelerated radiotherapy in combination with weekly cisplatin for locally advanced head and neck cancer. Head Neck. (2005) 27:36–43. doi: 10.1002/hed.20111
7. Chow E, Zeng L, Salvo N, Dennis K, Tsao M, Lutz S. Update on the systematic review of palliative radiotherapy trials for bone metastases. Clin Oncol. (2012) 24:112–24. doi: 10.1016/j.clon.2011.11.004
8. Frazier JL, Batra S, Kapor S, Vellimana A, Gandhi R, Carson KA, et al. Stereotactic radiosurgery in the management of brain metastases: an institutional retrospective analysis of survival. Int J Radiat Oncol Biol Phys. (2010) 76:1486–92. doi: 10.1016/j.ijrobp.2009.03.028
9. Agency IAE, Zubizarreta E. Radiotherapy in Cancer Care: Facing the Global Challenge. International Atomic Energy Agency Vienna (2017).
10. Rodríguez-Ruiz ME, Vanpouille-Box C, Melero I, Formenti SC, Demaria S. Immunological mechanisms responsible for radiation-induced abscopal effect. Trends Immunol. (2018) 39:644–55. doi: 10.1016/j.it.2018.06.001
11. Abuodeh Y, Venkat P, Kim S. Systematic review of case reports on the abscopal effect. Curr Probl Cancer. (2016) 40:25–37. doi: 10.1016/j.currproblcancer.2015.10.001
12. Postow MA, Callahan MK, Barker CA, Yamada Y, Yuan J, Kitano S, et al. Immunologic correlates of the abscopal effect in a patient with melanoma. N Engl J Med. (2012) 366:925–31. doi: 10.1056/NEJMoa1112824
13. Brody JD, Ai WZ, Czerwinski DK, Torchia JA, Levy M, Advani RH, et al. In situ vaccination with a TLR9 agonist induces systemic lymphoma regression: a phase I/II study. J Clin Oncol. (2010) 28:4324–32. doi: 10.1200/JCO.2010.28.9793
14. Golden EB, Chhabra A, Chachoua A, Adams S, Donach M, Fenton-Kerimian M, et al. Local radiotherapy and granulocyte-macrophage colony-stimulating factor to generate abscopal responses in patients with metastatic solid tumours: a proof-of-principle trial. Lancet Oncol. (2015) 16:795–803. doi: 10.1016/S1470-2045(15)00054-6
15. Reynders K, Illidge T, Siva S, Chang JY, De Ruysscher D. The abscopal effect of local radiotherapy: using immunotherapy to make a rare event clinically relevant. Cancer Treat Rev. (2015) 41:503–10. doi: 10.1016/j.ctrv.2015.03.011
16. Cai X, Chiu YH, Chen ZJ. The cGAS-cGAMP-STING pathway of cytosolic DNA sensing and signaling. Mol Cell. (2014) 54:289–96. doi: 10.1016/j.molcel.2014.03.040
17. Krysko DV, Garg AD, Kaczmarek A, Krysko O, Agostinis P, Vandenabeele P. Immunogenic cell death and DAMPs in cancer therapy. Nat Rev Cancer. (2012) 12:860–75. doi: 10.1038/nrc3380
18. Deng L, Liang H, Xu M, Yang X, Burnette B, Arina A, et al. STING-dependent cytosolic DNA sensing promotes radiation-induced type I interferon-dependent antitumor immunity in immunogenic tumors. Immunity. (2014) 41:843–52. doi: 10.1016/j.immuni.2014.10.019
19. Mothersill C, Seymour C. Radiation-induced bystander effects: past history and future directions. Radiat Res. (2001) 155:759–67. doi: 10.1667/0033-7587(2001)155[0759:RIBEPH]2.0.CO;2
20. Walle T, Martinez Monge R, Cerwenka A, Ajona D, Melero I, Lecanda F. Radiation effects on antitumor immune responses: current perspectives and challenges. Ther Adv Med Oncol. (2018) 10:1758834017742575. doi: 10.1177/1758834017742575
21. Wennerberg E, Lhuillier C, Vanpouille-Box C, Pilones KA, Garcia-Martinez E, Rudqvist NP, et al. Barriers to radiation-induced in situ tumor vaccination. Front Immunol. (2017) 8:229. doi: 10.3389/fimmu.2017.00229
22. Dewhirst MW, Cao Y, Moeller B. Cycling hypoxia and free radicals regulate angiogenesis and radiotherapy response. Nat Rev Cancer. (2008) 8:425–37. doi: 10.1038/nrc2397
23. Stetson DB, Ko JS, Heidmann T, Medzhitov R. Trex1 prevents cell-intrinsic initiation of autoimmunity. Cell. (2008) 134:587–98. doi: 10.1016/j.cell.2008.06.032
24. Vanpouille-Box C, Alard A, Aryankalayil MJ, Sarfraz Y, Diamond JM, Schneider RJ, et al. DNA exonuclease Trex1 regulates radiotherapy-induced tumour immunogenicity. Nat Commun. (2017) 8:15618. doi: 10.1038/ncomms15618
25. Barsoum IB, Smallwood CA, Siemens DR, Graham CH. A mechanism of hypoxia-mediated escape from adaptive immunity in cancer cells. Cancer Res. (2014) 74:665–74. doi: 10.1158/0008-5472.CAN-13-0992
26. Noman MZ, Desantis G, Janji B, Hasmim M, Karray S, Dessen P, et al. PD-L1 is a novel direct target of HIF-1alpha, and its blockade under hypoxia enhanced MDSC-mediated T cell activation. J Exp Med. (2014) 211:781–90. doi: 10.1084/jem.20131916
27. Voron T, Marcheteau E, Pernot S, Colussi O, Tartour E, Taieb J, et al. Control of the immune response by pro-angiogenic factors. Front Oncol. (2014) 4:70. doi: 10.3389/fonc.2014.00070
28. Barsoum IB, Hamilton TK, Li X, Cotechini T, Miles EA, Siemens DR, et al. Hypoxia induces escape from innate immunity in cancer cells via increased expression of ADAM10: role of nitric oxide. Cancer Res. (2011) 71:7433–41. doi: 10.1158/0008-5472.CAN-11-2104
29. Matsumura S, Wang B, Kawashima N, Braunstein S, Badura M, Cameron TO, et al. Radiation-induced CXCL16 release by breast cancer cells attracts effector T cells. J Immunol. (2008) 181:3099–107. doi: 10.4049/jimmunol.181.5.3099
30. Burnette BC, Liang H, Lee Y, Chlewicki L, Khodarev NN, Weichselbaum RR, et al. The efficacy of radiotherapy relies upon induction of type i interferon-dependent innate and adaptive immunity. Cancer Res. (2011) 71:2488–96. doi: 10.1158/0008-5472.CAN-10-2820
31. Lugade AA, Moran JP, Gerber SA, Rose RC, Frelinger JG, Lord EM. Local radiation therapy of B16 melanoma tumors increases the generation of tumor antigen-specific effector cells that traffic to the tumor. J Immunol. (2005) 174:7516–23. doi: 10.4049/jimmunol.174.12.7516
32. Kalbasi A, Komar C, Tooker GM, Liu M, Lee JW, Gladney WL, et al. Tumor-derived CCL2 mediates resistance to radiotherapy in pancreatic ductal adenocarcinoma. Clin Cancer Res. (2017) 23:137–48. doi: 10.1158/1078-0432.CCR-16-0870
33. Xu J, Escamilla J, Mok S, David J, Priceman S, West B, et al. CSF1R signaling blockade stanches tumor-infiltrating myeloid cells and improves the efficacy of radiotherapy in prostate cancer. Cancer Res. (2013) 73:2782–94. doi: 10.1158/0008-5472.CAN-12-3981
34. Kioi M, Vogel H, Schultz G, Hoffman RM, Harsh GR, Brown JM. Inhibition of vasculogenesis, but not angiogenesis, prevents the recurrence of glioblastoma after irradiation in mice. J Clin Invest. (2010) 120:694–705. doi: 10.1172/JCI40283
35. Gasser S, Orsulic S, Brown EJ, Raulet DH. The DNA damage pathway regulates innate immune system ligands of the NKG2D receptor. Nature. (2005) 436:1186–90. doi: 10.1038/nature03884
36. Reits EA, Hodge JW, Herberts CA, Groothuis TA, Chakraborty M, Wansley EK, et al. Radiation modulates the peptide repertoire, enhances MHC class I expression, and induces successful antitumor immunotherapy. J Exp Med. (2006) 203:1259–71. doi: 10.1084/jem.20052494
37. Allard B, Beavis PA, Darcy PK, Stagg J. Immunosuppressive activities of adenosine in cancer. Curr Opin Pharmacol. (2016) 29:7–16. doi: 10.1016/j.coph.2016.04.001
38. Zhang B, Bowerman NA, Salama JK, Schmidt H, Spiotto MT, Schietinger A, et al. Induced sensitization of tumor stroma leads to eradication of established cancer by T cells. J Exp Med. (2007) 204:49–55. doi: 10.1084/jem.20062056
39. Hellevik T, Martinez-Zubiaurre I. Radiotherapy and the tumor stroma: the importance of dose and fractionation. Front Oncol. (2014) 4:1. doi: 10.3389/fonc.2014.00001
40. Tommelein J, De Vlieghere E, Verset L, Melsens E, Leenders J, Descamps B, et al. Radiotherapy-activated cancer-associated fibroblasts promote tumor progression through paracrine IGF1R activation. Cancer Res. (2018) 78:659–70. doi: 10.1158/0008-5472.CAN-17-0524
41. Spadaro O, Camell CD, Bosurgi L, Nguyen KY, Youm YH, Rothlin CV, et al. IGF1 shapes macrophage activation in response to immunometabolic challenge. Cell Rep. (2017) 19:225–34. doi: 10.1016/j.celrep.2017.03.046
42. Chakraborty M, Abrams SI, Camphausen K, Liu K, Scott T, Coleman CN, et al. Irradiation of tumor cells up-regulates Fas and enhances CTL lytic activity and CTL adoptive immunotherapy. J Immunol. (2003) 170:6338–47. doi: 10.4049/jimmunol.170.12.6338
43. Jobling MF, Mott JD, Finnegan MT, Jurukovski V, Erickson AC, Walian PJ, et al. Isoform-specific activation of latent transforming growth factor beta (LTGF-beta) by reactive oxygen species. Radiat Res. (2006) 166:839–48. doi: 10.1667/RR0695.1
44. Barcellos-Hoff MH, Cucinotta FA. New tricks for an old fox: impact of TGFbeta on the DNA damage response and genomic stability. Sci Signal. (2014) 7:re5. doi: 10.1126/scisignal.2005474
45. Vanpouille-Box C, Diamond JM, Pilones KA, Zavadil J, Babb JS, Formenti SC, et al. TGFbeta is a master regulator of radiation therapy-induced antitumor immunity. Cancer Res. (2015) 75:2232–42. doi: 10.1158/0008-5472.CAN-14-3511
46. Vacchelli E, Bloy N, Aranda F, Buque A, Cremer I, Demaria S, et al. Trial watch: immunotherapy plus radiation therapy for oncological indications. Oncoimmunology. (2016) 5:e1214790. doi: 10.1080/2162402X.2016.1214790
47. Tang C, Welsh JW, De Groot P, Massarelli E, Chang JY, Hess KR, et al. Ipilimumab with stereotactic ablative radiation therapy: phase I results and immunologic correlates from peripheral T cells. Clin Cancer Res. (2017) 23:1388–96. doi: 10.1158/1078-0432.CCR-16-1432
48. Jiang T, Zhou C, Ren S. Role of IL-2 in cancer immunotherapy. Oncoimmunology. (2016) 5:e1163462. doi: 10.1080/2162402X.2016.1163462
49. Robinson TO, Schluns KS. The potential and promise of IL-15 in immuno-oncogenic therapies. Immunol Lett. (2017) 190:159–68. doi: 10.1016/j.imlet.2017.08.010
50. Vacchelli E, Aranda F, Bloy N, Buque A, Cremer I, Eggermont A, et al. Trial watch-immunostimulation with cytokines in cancer therapy. Oncoimmunology. (2016) 5:e1115942. doi: 10.1080/2162402X.2015.1115942
51. Waldmann TA. Cytokines in cancer immunotherapy. Cold Spring Harb Perspect Biol. (2017) 10:a028472. doi: 10.1101/cshperspect.a028472
52. Safwat A, Schmidt H, Bastholt L, Fode K, Larsen S, Aggerholm N, et al. A phase II trial of low-dose total body irradiation and subcutaneous Interleukin-2 in metastatic melanoma. Radiother Oncol. (2005) 77:143–7. doi: 10.1016/j.radonc.2005.09.008
53. Seung SK, Curti BD, Crittenden M, Walker E, Coffey T, Siebert JC, et al. Phase 1 study of stereotactic body radiotherapy and interleukin-2–tumor and immunological responses. Sci Transl Med. (2012) 4:137ra174. doi: 10.1126/scitranslmed.3003649
54. Lange JR, Raubitschek AA, Pockaj BA, Spencer WF, Lotze MT, Topalian SL, et al. A pilot study of the combination of interleukin-2-based immunotherapy and radiation therapy. J Immunother. (1992) 12:265–71. doi: 10.1097/00002371-199211000-00007
55. Ridolfi L, De Rosa F, Ridolfi R, Gentili G, Valmorri L, Scarpi E, et al. Radiotherapy as an immunological booster in patients with metastatic melanoma or renal cell carcinoma treated with high-dose Interleukin-2: evaluation of biomarkers of immunologic and therapeutic response. J Transl Med. (2014) 12:262. doi: 10.1186/s12967-014-0262-6
56. Vacchelli E, Eggermont A, Fridman WH, Galon J, Zitvogel L, Kroemer G, et al. Trial watch: immunostimulatory cytokines. Oncoimmunology. (2013) 2:e24850. doi: 10.4161/onci.24850
57. Harrington KJ, Hingorani M, Tanay MA, Hickey J, Bhide SA, Clarke PM, et al. Phase I/II study of oncolytic HSVGM-CSF in combination with radiotherapy and cisplatin in untreated stage III/IV squamous cell cancer of the head and neck. Clin Cancer Res. (2010) 16:4005–15. doi: 10.1158/1078-0432.CCR-10-0196
58. Gulley JL, Arlen PM, Bastian A, Morin S, Marte J, Beetham P, et al. Combining a recombinant cancer vaccine with standard definitive radiotherapy in patients with localized prostate cancer. Clin Cancer Res. (2005) 11:3353–62. doi: 10.1158/1078-0432.CCR-04-2062
59. Rampling R, Peoples S, Mulholland PJ, James A, Al-Salihi O, Twelves CJ, et al. A cancer research UK first time in human phase I trial of IMA950 (Novel Multipeptide Therapeutic Vaccine) in patients with newly diagnosed glioblastoma. Clin Cancer Res. (2016) 22:4776–85. doi: 10.1158/1078-0432.CCR-16-0506
60. Basu P, Jenson AB, Majhi T, Choudhury P, Mandal R, Banerjee D, et al. Phase 2 randomized controlled trial of radiation therapy plus concurrent interferon-alpha and retinoic acid versus cisplatin for stage III cervical carcinoma. Int J Radiat Oncol Biol Phys. (2016) 94:102–10. doi: 10.1016/j.ijrobp.2015.09.040
61. Picozzi VJ, Abrams RA, Decker PA, Traverso W, O'reilly EM, Greeno E, et al. Multicenter phase II trial of adjuvant therapy for resected pancreatic cancer using cisplatin, 5-fluorouracil, and interferon-alfa-2b-based chemoradiation: ACOSOG Trial Z05031. Ann Oncol. (2011) 22:348–54. doi: 10.1093/annonc/mdq384
62. Posner MC, Gooding WE, Landreneau RJ, Rosenstein MM, Clarke MR, Peterson MS, et al. Preoperative chemoradiotherapy for carcinoma of the esophagus and gastroesophageal junction. Cancer J Sci Am. (1998) 4:237–46.
63. Nukui Y, Picozzi VJ, Traverso LW. Interferon-based adjuvant chemoradiation therapy improves survival after pancreaticoduodenectomy for pancreatic adenocarcinoma. Am J Surg. (2000) 179:367–71. doi: 10.1016/S0002-9610(00)00369-X
64. Mundt AJ, Vijayakumar S, Nemunaitis J, Sandler A, Schwartz H, Hanna N, et al. A Phase I trial of TNFerade biologic in patients with soft tissue sarcoma in the extremities. Clin Cancer Res. (2004) 10:5747–53. doi: 10.1158/1078-0432.CCR-04-0296
65. Senzer N, Mani S, Rosemurgy A, Nemunaitis J, Cunningham C, Guha C, et al. TNFerade biologic, an adenovector with a radiation-inducible promoter, carrying the human tumor necrosis factor alpha gene: a phase I study in patients with solid tumors. J Clin Oncol. (2004) 22:592–601. doi: 10.1200/JCO.2004.01.227
66. Seiwert TY, Darga T, Haraf D, Blair EA, Stenson K, Cohen EE, et al. A phase I dose escalation study of Ad GV.EGR.TNF.11D (TNFerade Biologic) with concurrent chemoradiotherapy in patients with recurrent head and neck cancer undergoing reirradiation. Ann Oncol. (2013) 24:769–76. doi: 10.1093/annonc/mds523
67. Chang KJ, Reid T, Senzer N, Swisher S, Pinto H, Hanna N, et al. Phase I evaluation of TNFerade biologic plus chemoradiotherapy before esophagectomy for locally advanced resectable esophageal cancer. Gastrointest Endosc. (2012) 75:1139–1146 e1132. doi: 10.1016/j.gie.2012.01.042
68. Herman JM, Wild AT, Wang H, Tran PT, Chang KJ, Taylor GE, et al. Randomized phase III multi-institutional study of TNFerade biologic with fluorouracil and radiotherapy for locally advanced pancreatic cancer: final results. J Clin Oncol. (2013) 31:886–94. doi: 10.1200/JCO.2012.44.7516
69. Mcloughlin JM, Mccarty TM, Cunningham C, Clark V, Senzer N, Nemunaitis J, et al. TNFerade, an adenovector carrying the transgene for human tumor necrosis factor alpha, for patients with advanced solid tumors: surgical experience and long-term follow-up. Ann Surg Oncol. (2005) 12:825–30. doi: 10.1245/ASO.2005.03.023
70. Hecht JR, Farrell JJ, Senzer N, Nemunaitis J, Rosemurgy A, Chung T, et al. EUS or percutaneously guided intratumoral TNFerade biologic with 5-fluorouracil and radiotherapy for first-line treatment of locally advanced pancreatic cancer: a phase I/II study. Gastrointest Endosc. (2012) 75:332–8. doi: 10.1016/j.gie.2011.10.007
71. Sim GC, Radvanyi L. The IL-2 cytokine family in cancer immunotherapy. Cytokine Growth Factor Rev. (2014) 25:377–90. doi: 10.1016/j.cytogfr.2014.07.018
72. Rosenzwajg M, Lorenzon R, Cacoub P, Pham HP, Pitoiset F, El Soufi K, et al. Immunological and clinical effects of low-dose interleukin-2 across 11 autoimmune diseases in a single, open clinical trial. Ann Rheum Dis. (2019) 78:209–17. doi: 10.1136/annrheumdis-2018-214229
73. Arenas-Ramirez N, Woytschak J, Boyman O. Interleukin-2: biology, design and application. Trends Immunol. (2015) 36:763–77. doi: 10.1016/j.it.2015.10.003
74. Cameron RB, Spiess PJ, Rosenberg SA. Synergistic antitumor activity of tumor-infiltrating lymphocytes, interleukin 2, and local tumor irradiation. Studies on the mechanism of action. J Exp Med. (1990) 171:249–63. doi: 10.1084/jem.171.1.249
75. Safwat A, Aggerholm N, Roitt I, Overgaard J, Hokland M. Low-dose total body irradiation augments the therapeutic effect of interleukin-2 in a mouse model for metastatic malignant melanoma. J Exp Ther Oncol. (2003) 3:161–8. doi: 10.1046/j.1359-4117.2003.01093.x
76. Safwat A, Aggerholm N, Roitt I, Overgaard J, Hokland M. Tumour burden and interleukin-2 dose affect the interaction between low-dose total body irradiation and interleukin 2. Eur J Cancer. (2004) 40:1412–7. doi: 10.1016/j.ejca.2004.01.037
77. List T, Neri D. Immunocytokines: a review of molecules in clinical development for cancer therapy. Clin Pharmacol Adv Appl. (2013) 5:29–45. doi: 10.2147/CPAA.S49231
78. Charych DH, Hoch U, Langowski JL, Lee SR, Addepalli MK, Kirk PB, et al. NKTR-214, an engineered cytokine with biased IL2 receptor binding, increased tumor exposure, and marked efficacy in mouse tumor models. Clin Cancer Res. (2016) 22:680–90. doi: 10.1158/1078-0432.CCR-15-1631
79. Carnemolla B, Borsi L, Balza E, Castellani P, Meazza R, Berndt A, et al. Enhancement of the antitumor properties of interleukin-2 by its targeted delivery to the tumor blood vessel extracellular matrix. Blood. (2002) 99:1659–65. doi: 10.1182/blood.V99.5.1659
80. Gillies SD, Lan Y, Hettmann T, Brunkhorst B, Sun Y, Mueller SO, et al. A low-toxicity IL-2-based immunocytokine retains antitumor activity despite its high degree of IL-2 receptor selectivity. Clin Cancer Res. (2011) 17:3673–85. doi: 10.1158/1078-0432.CCR-10-2921
81. Rekers NH, Zegers CM, Germeraad WT, Dubois L, Lambin P. Long-lasting antitumor effects provided by radiotherapy combined with the immunocytokine L19-IL2. Oncoimmunology. (2015) 4:e1021541. doi: 10.1080/2162402X.2015.1021541
82. Zegers CML, Rekers NH, Quaden DHF, Lieuwes NG, Yaromina A, Germeraad WTV, et al. Radiotherapy combined with the immunocytokine L19-IL2 provides long-lasting antitumor effects. Clin Cancer Res. (2015) 21:1151–60. doi: 10.1158/1078-0432.CCR-14-2676
83. Rekers NH, Olivo Pimentel V, Yaromina A, Lieuwes NG, Biemans R, Zegers CML, et al. The immunocytokine L19-IL2: an interplay between radiotherapy and long-lasting systemic anti-tumour immune responses. Oncoimmunology. (2018) 7:e1414119. doi: 10.1080/2162402X.2017.1414119
84. Van Den Heuvel MM, Verheij M, Boshuizen R, Belderbos J, Dingemans A-MC, De Ruysscher D, et al. NHS-IL2 combined with radiotherapy: preclinical rationale and phase Ib trial results in metastatic non-small cell lung cancer following first-line chemotherapy. J Transl Med. (2015) 13:32. doi: 10.1186/s12967-015-0397-0
85. Stonier SW, Schluns KS. Trans-presentation: a novel mechanism regulating IL-15 delivery and responses. Immunol Lett. (2010) 127:85–92. doi: 10.1016/j.imlet.2009.09.009
86. Waldmann TA. The biology of IL-15: implications for cancer therapy and the treatment of autoimmune disorders. J Invest Dermatol Symp Proc. (2013) 16:S28–S30. doi: 10.1038/jidsymp.2013.8
87. Mlecnik B, Bindea G, Angell HK, Sasso MS, Obenauf AC, Fredriksen T, et al. Functional network pipeline reveals genetic determinants associated with in situ lymphocyte proliferation and survival of cancer patients. Sci Transl Med. (2014) 6: 228ra237. doi: 10.1126/scitranslmed.3007240
88. Steel JC, Waldmann TA, Morris JC. Interleukin-15 biology and its therapeutic implications in cancer. Trends Pharmacol Sci. (2012) 33:35–41. doi: 10.1016/j.tips.2011.09.004
89. Conlon KC, Lugli E, Welles HC, Rosenberg SA, Fojo AT, Morris JC, et al. Redistribution, hyperproliferation, activation of natural killer cells and CD8 T cells, and cytokine production during first-in-human clinical trial of recombinant human interleukin-15 in patients with cancer. J Clin Oncol. (2015) 33:74–82. doi: 10.1200/JCO.2014.57.3329
90. Miller JS, Morishima C, Mcneel DG, Patel MR, Kohrt HEK, Thompson JA, et al. A first-in-human phase I study of subcutaneous outpatient recombinant human IL15 (rhIL15) in adults with advanced solid tumors. Clin Cancer Res. (2018) 24:1525–35. doi: 10.1158/1078-0432.CCR-17-2451
91. Pilones K, Aryankalayil J, Formenti S, Demaria S. Intratumoral IL-15 potentiates radiation-induced anti-tumor immunity. J Immunother Cancer. (2015) 3:P239. doi: 10.1186/2051-1426-3-S2-P239
92. Kim PS, Kwilas AR, Xu W, Alter S, Jeng EK, Wong HC, et al. IL-15 superagonist/IL-15RalphaSushi-Fc fusion complex (IL-15SA/IL-15RalphaSu-Fc; ALT-803) markedly enhances specific subpopulations of NK and memory CD8+ T cells, and mediates potent anti-tumor activity against murine breast and colon carcinomas. Oncotarget. (2016) 7:16130–45. doi: 10.18632/oncotarget.7470
93. Rhode PR, Egan JO, Xu W, Hong H, Webb GM, Chen X, et al. Comparison of the superagonist complex, ALT-803, to IL15 as cancer immunotherapeutics in animal models. Cancer Immunol Res. (2016) 4:49–60. doi: 10.1158/2326-6066.CIR-15-0093-T
94. Margolin K, Morishima C, Velcheti V, Miller JS, Lee SM, Silk AW, et al. Phase I trial of ALT-803, a novel recombinant IL15 complex, in patients with advanced solid tumors. Clin Cancer Res. (2018) 24:5552–61. doi: 10.1158/1078-0432.CCR-18-0945
95. Wrangle JM, Velcheti V, Patel MR, Garrett-Mayer E, Hill EG, Ravenel JG, et al. ALT-803, an IL-15 superagonist, in combination with nivolumab in patients with metastatic non-small cell lung cancer: a non-randomised, open-label, phase 1b trial. Lancet Oncol. (2018) 19:694–704. doi: 10.1016/S1470-2045(18)30148-7
96. Mathios D, Park C-K, Marcus WD, Alter S, Rhode PR, Jeng EK, et al. Therapeutic administration of IL-15 superagonist complex ALT-803 leads to long-term survival and durable antitumor immune response in a murine glioblastoma model. Int J Cancer. (2016) 138:187–94. doi: 10.1002/ijc.29686
97. Barreda DR, Hanington PC, Belosevic M. Regulation of myeloid development and function by colony stimulating factors. Dev Comp Immunol. (2004) 28:509–54. doi: 10.1016/j.dci.2003.09.010
98. Choi K-J, Kim J-H, Lee Y-S, Kim J, Suh B-S, Kim H, et al. Concurrent delivery of GM-CSF and B7-1 using an oncolytic adenovirus elicits potent antitumor effect. Gene Ther. (2006) 13:1010–20. doi: 10.1038/sj.gt.3302759
99. Kaufman HL, Ruby CE, Hughes T, Slingluff CLJr. Current status of granulocyte-macrophage colony-stimulating factor in the immunotherapy of melanoma. J Immunother Cancer. (2014) 2:11. doi: 10.1186/2051-1426-2-11
100. Demaria S, Ng B, Devitt ML, Babb JS, Kawashima N, Liebes L, et al. Ionizing radiation inhibition of distant untreated tumors (abscopal effect) is immune mediated. Int J Radiat Oncol Biol Phys. (2004) 58:862–70. doi: 10.1016/j.ijrobp.2003.09.012
101. Shi F, Wang X, Teng F, Kong L, Yu J. Abscopal effect of metastatic pancreatic cancer after local radiotherapy and granulocyte-macrophage colony-stimulating factor therapy. Cancer Biol Ther. (2017) 18:137–41. doi: 10.1080/15384047.2016.1276133
102. Pfeffer LM, Dinarello CA, Herberman RB, Williams BR, Borden EC, Bordens R, et al. Biological properties of recombinant alpha-interferons: 40th anniversary of the discovery of interferons. Cancer Res. (1998) 58:2489–99.
103. Parker BS, Rautela J, Hertzog PJ. Antitumour actions of interferons: implications for cancer therapy. Nat Rev Cancer. (2016) 16:131–44. doi: 10.1038/nrc.2016.14
104. Swiecki M, Colonna M. The multifaceted biology of plasmacytoid dendritic cells. Nat Rev Immunol. (2015) 15:471–85. doi: 10.1038/nri3865
105. Musella M, Manic G, De Maria R, Vitale I, Sistigu A. Type-I-interferons in infection and cancer: unanticipated dynamics with therapeutic implications. Oncoimmunology. (2017) 6:e1314424. doi: 10.1080/2162402X.2017.1314424
106. Borden EC. Gene regulation and clinical roles for interferons in neoplastic diseases. Oncologist. (1998) 3:198–203.
107. Platanias LC. Mechanisms of type-I- and type-II-interferon-mediated signalling. Nat Rev Immunol. (2005) 5:375–86. doi: 10.1038/nri1604
108. Wadler S, Wersto R, Weinberg V, Thompson D, Schwartz EL. Interaction of fluorouracil and interferon in human colon cancer cell lines: cytotoxic and cytokinetic effects. Cancer Res. (1990) 50:5735–9.
109. Ismail A, Van Groeningen CJ, Hardcastle A, Ren Q, Aherne GW, Geoffroy F, et al. Modulation of fluorouracil cytotoxicity by interferon-alpha and -gamma. Mol Pharmacol. (1998) 53:252–61. doi: 10.1124/mol.53.2.252
110. Holsti LR, Mattson K, Niiranen A, Standertskiold-Nordenstam CG, Stenman S, Sovijarvi A, et al. Enhancement of radiation effects by alpha interferon in the treatment of small cell carcinoma of the lung. Int J Radiat Oncol Biol Phys. (1987) 13:1161–6. doi: 10.1016/0360-3016(87)90189-1
111. Sischy B, Doggett RL, Krall JM, Taylor DG, Sause WT, Lipsett JA, et al. Definitive irradiation and chemotherapy for radiosensitization in management of anal carcinoma: interim report on Radiation Therapy Oncology Group study no. 8314. J Natl Cancer Inst. (1989) 81:850–6. doi: 10.1093/jnci/81.11.850
112. Barker CA, Postow MA. Combinations of radiation therapy and immunotherapy for melanoma: a review of clinical outcomes. Int J Radiat Oncol Biol Phys. (2014) 88:986–97. doi: 10.1016/j.ijrobp.2013.08.035
113. Carswell EA, Old LJ, Kassel RL, Green S, Fiore N, Williamson B. An endotoxin-induced serum factor that causes necrosis of tumors. Proc Natl Acad Sci USA. (1975) 72:3666–70. doi: 10.1073/pnas.72.9.3666
114. Gaur U, Aggarwal BB. Regulation of proliferation, survival and apoptosis by members of the TNF superfamily. Biochem Pharmacol. (2003) 66:1403–8. doi: 10.1016/S0006-2952(03)00490-8
115. Hallahan DE, Beckett MA, Kufe D, Weichselbaum RR. The interaction between recombinant human tumor necrosis factor and radiation in 13 human tumor cell lines. Int J Radiat Oncol Biol Phys. (1990) 19:69–74. doi: 10.1016/0360-3016(90)90136-8
116. Spriggs DR, Sherman ML, Michie H, Arthur KA, Imamura K, Wilmore D, et al. Recombinant human tumor necrosis factor administered as a 24-hour intravenous infusion. A phase I and pharmacologic study. J Natl Cancer Inst. (1988) 80:1039–44. doi: 10.1093/jnci/80.13.1039
117. Kemeny N, Childs B, Larchian W, Rosado K, Kelsen D. A phase II trial of recombinant tumor necrosis factor in patients with advanced colorectal carcinoma. Cancer. (1990) 66:659–63. doi: 10.1002/1097-0142(19900815)66:4<659::AID-CNCR2820660410>3.0.CO;2-2
118. Lejeune F, Lienard D, Eggermont A, Schraffordt Koops H, Kroon B, Gerain J, et al. Clinical experience with high-dose tumor necrosis factor alpha in regional therapy of advanced melanoma. Circ Shock. (1994) 43:191–7.
119. Eggermont AM, Schraffordt Koops H, Klausner JM, Schlag PM, Kroon BB, Ben-Ari G, et al. Isolated limb perfusion with high-dose tumor necrosis factor-alpha for locally advanced extremity soft tissue sarcomas. Cancer Treat Res. (1997) 91:189–203. doi: 10.1007/978-1-4615-6121-7_13
120. Kircheis R, Wagner E. Technology evaluation: TNFerade, GenVec. Curr Opin Mol Ther. (2003) 5:437–47.
121. Kali A. TNFerade, an innovative cancer immunotherapeutic. Indian J Pharmacol. (2015) 47:479–83. doi: 10.4103/0253-7613.165190
122. Rasmussen H, Rasmussen C, Lempicki M, Durham R, Brough D, King CR, et al. TNFerade Biologic: preclinical toxicology of a novel adenovector with a radiation-inducible promoter, carrying the human tumor necrosis factor alpha gene. Cancer Gene Ther. (2002) 9:951–7. doi: 10.1038/sj.cgt.7700518
123. Sharma A, Mani S, Hanna N, Guha C, Vikram B, Weichselbaum RR, et al. Clinical protocol. An open-label, phase I, dose-escalation study of tumor necrosis factor-alpha (TNFerade Biologic) gene transfer with radiation therapy for locally advanced, recurrent, or metastatic solid tumors. Hum Gene Ther. (2001) 12:1109–31. doi: 10.1089/104303401750214320
124. Citrin D, Camphausen K, Wood BJ, Quezado M, Denobile J, Pingpank JF, et al. A pilot feasibility study of TNFerade biologic with capecitabine and radiation therapy followed by surgical resection for the treatment of rectal cancer. Oncology. (2010) 79:382–8. doi: 10.1159/000323488
125. Sgadari C, Angiolillo AL, Tosato G. Inhibition of angiogenesis by interleukin-12 is mediated by the interferon-inducible protein 10. Blood. (1996) 87:3877–82.
126. Smyth MJ, Taniguchi M, Street SE. The anti-tumor activity of IL-12: mechanisms of innate immunity that are model and dose dependent. J Immunol. (2000) 165:2665–70. doi: 10.4049/jimmunol.165.5.2665
127. Lu X. Impact of IL-12 in Cancer. Curr Cancer Drug Targets. (2017) 17:682–97. doi: 10.2174/1568009617666170427102729
128. Lasek W, Zagozdzon R, Jakobisiak M. Interleukin 12: still a promising candidate for tumor immunotherapy? Cancer Immunol Immunother. (2014) 63:419–35. doi: 10.1007/s00262-014-1523-1
129. Berraondo P, Etxeberria I, Ponz-Sarvise M, Melero I. Revisiting interleukin-12 as a cancer immunotherapy agent. Clin Cancer Res. (2018) 24:2716–8. doi: 10.1158/1078-0432.CCR-18-0381
130. Xian J, Yang H, Lin Y, Liu S. Combination nonviral murine interleukin 2 and interleukin 12 gene therapy and radiotherapy for head and neck squamous cell carcinoma. Arch Otolaryngol Head Neck Surg. (2005) 131:1079–85. doi: 10.1001/archotol.131.12.1079
131. Kim W, Seong J, Oh HJ, Koom WS, Choi K-J, Yun C-O. A novel combination treatment of armed oncolytic adenovirus expressing IL-12 and GM-CSF with radiotherapy in murine hepatocarcinoma. J Radiat Res. (2011) 52:646–54. doi: 10.1269/jrr.10185
132. Teicher BA, Ara G, Buxton D, Leonard J, Schaub RG. Optimal scheduling of interleukin-12 and fractionated radiation therapy in the murine Lewis lung carcinoma. Radiat Oncol Investig. (1998) 6:71–80. doi: 10.1002/(SICI)1520-6823(1998)6:2<71::AID-ROI2>3.0.CO;2-E
133. Fallon J, Tighe R, Kradjian G, Guzman W, Bernhardt A, Neuteboom B, et al. The immunocytokine NHS-IL12 as a potential cancer therapeutic. Oncotarget. (2014) 5:1869–84. doi: 10.18632/oncotarget.1853
Keywords: radiotherapy, cytokine, immunocytokine, immunotherapy, immunogenic cell death
Citation: Palata O, Hradilova Podzimkova N, Nedvedova E, Umprecht A, Sadilkova L, Palova Jelinkova L, Spisek R and Adkins I (2019) Radiotherapy in Combination With Cytokine Treatment. Front. Oncol. 9:367. doi: 10.3389/fonc.2019.00367
Received: 29 October 2018; Accepted: 23 April 2019;
Published: 22 May 2019.
Edited by:
Udo S. Gaipl, University Hospital Erlangen, GermanyReviewed by:
Claire Isabelle Vanpouille-Box, Cornell University, United StatesFranziska Eckert, Tübingen University Hospital, Germany
Copyright © 2019 Palata, Hradilova Podzimkova, Nedvedova, Umprecht, Sadilkova, Palova Jelinkova, Spisek and Adkins. This is an open-access article distributed under the terms of the Creative Commons Attribution License (CC BY). The use, distribution or reproduction in other forums is permitted, provided the original author(s) and the copyright owner(s) are credited and that the original publication in this journal is cited, in accordance with accepted academic practice. No use, distribution or reproduction is permitted which does not comply with these terms.
*Correspondence: Irena Adkins, YWRraW5zQHNvdGlvLmNvbQ==