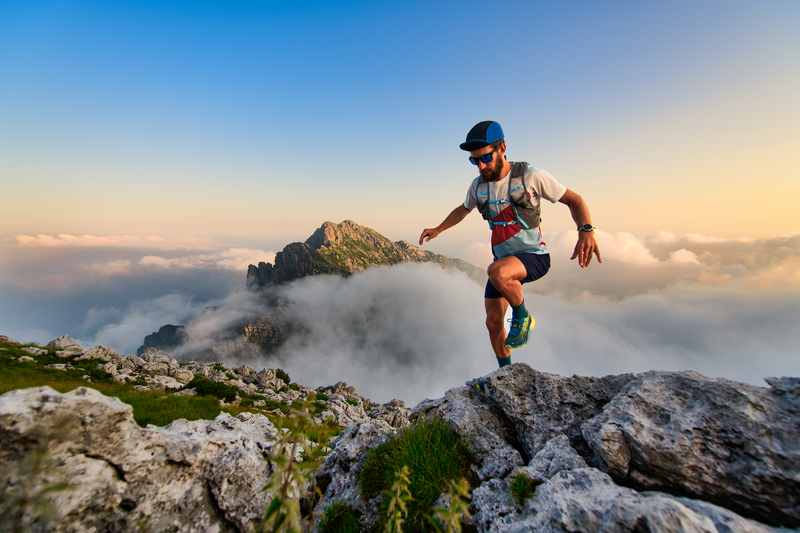
95% of researchers rate our articles as excellent or good
Learn more about the work of our research integrity team to safeguard the quality of each article we publish.
Find out more
MINI REVIEW article
Front. Oncol. , 15 December 2017
Sec. Cancer Molecular Targets and Therapeutics
Volume 7 - 2017 | https://doi.org/10.3389/fonc.2017.00312
Phosphatidylinositol-4,5-bisphosphate 3-kinase (PI3K) plays a critical role in the pathogenesis of cancer including glioblastoma, the most common and aggressive form of brain cancer. Targeting the PI3K pathway to treat glioblastoma has been tested in the clinic with modest effect. In light of the recent finding that PI3K catalytic subunits (PIK3CA/p110α, PIK3CB/p110β, PIK3CD/p110δ, and PIK3CG/p110γ) are not functionally redundant, it is imperative to determine whether these subunits play divergent roles in glioblastoma and whether selectively targeting PI3K catalytic subunits represents a novel and effective strategy to tackle PI3K signaling. This article summarizes recent advances in understanding the role of PI3K catalytic subunits in glioblastoma and discusses the possibility of selective blockade of one PI3K catalytic subunit as a treatment option for glioblastoma.
Glioblastoma is a grade IV glioma accounting for approximately 55% of all cases of malignant brain tumors. The 5-year overall survival for glioblastoma is only about 5% even after aggressive treatments including maximal surgical removal of the tumor, ionizing radiation, and chemotherapy (1–6). This poor prognosis is, at least in part, owing to the high incidence of tumor recurrence; nearly 100% of glioblastoma patients will succumb to tumor recurrence if they live more than 2 years. The average survival for patients with recurrent glioblastoma is about 7.5 months (7), because these patients often cannot undergo another major resection, and the tumors are resistant to radiation and chemotherapies (7–10). With no standard of care treatment for recurrent glioblastoma, new strategies for therapeutic intervention are urgently needed. Therapies targeting essential survival pathways in glioblastoma [e.g., inhibitors of receptor tyrosine kinases (RTKs) or signaling molecules] have achieved modest, yet encouraging, therapeutic benefits in recurrent glioblastoma (11–22). The difficulty of designing targeted therapies in the clinic is often attributed to the lack of biomarkers for patient selection, the high toxicity of these drugs, the difficulties in delivering drugs into the brain, and the unclear mechanisms of therapeutic targets in glioblastoma.
The PI3K pathway plays an essential role in signal transduction and modulates various biological processes including cell proliferation, survival, motility, death, and metabolism (23, 24). Aberrations in these processes are pivotal in the pathogenesis of cancer, including glioblastoma (25, 26). Upon activation of RTKs or G protein-coupled receptors (GPCRs), PI3K phosphorylates phosphatidylinositol-4,5-bisphosphate (PIP2 or PtdIns4,5P2) on the plasma membrane, yielding phosphatidylinositol-3,4,5-triphosphate (PIP3 or PtdIns3,4,5P3), which subsequently activates V-Akt murine thymoma viral oncogene homolog (AKT) to inhibit cell death and thereby sustain cell survival. The PI3K pathway is negatively regulated by the tumor suppressor phosphatase and tensin homolog (PTEN), which dephosphorylates PIP3 [reviewed in Ref. (27, 28)]. Based upon genome sequencing data from The Cancer Genome Atlas (TCGA), more than 88% of glioblastoma tumors harbor mutations in the signaling pathways driven by RTKs, oncogenic RAS gene family, and/or PI3K (29). PTEN is altered in approximately 40% of glioblastoma patients and is considered as a biomarker of glioblastoma prognosis (30, 31). Frequent mutations in PTEN and certain PI3K genes have been reported in primary and recurrent glioblastomas (29, 32–40). The oncogenic role of these genes has been validated in genetically engineered mouse models of glioblastoma [summarized in Ref. (41–43)]. A recent study analyzing the spatiotemporal genomic architecture of glioblastoma found that mutations of a PI3K gene were commonly clonal, early events of tumorigenesis, and affected the tumor response to therapies (44).
Because of the critical role of hyper-activation of PI3K in cancer and therapeutic resistance, considerable efforts have been directed to develop chemical inhibitors targeting the PI3K/AKT signaling pathway. To date, there are more than 50 chemical compounds specifically blocking the activity of PI3K/AKT and showed promising effects on tumor inhibition in preclinical studies, but only some drugs have successfully entered clinical trials for glioblastoma treatment (Table 1). PI3K or PI3K/mechanistic target of rapamycin (mTOR) dual inhibitors (e.g., XL765, wortmannin, PI-103, PX-866, and LY294002) alone or in combination with radiotherapy or chemotherapy substantially inhibited glioblastoma cell growth in preclinical studies (45–49). A pan PI3K inhibitor PX-866 showed modest effect on the prognosis of 33 recurrent glioblastoma patients in a phase II clinical trial (12). While this is encouraging, the high toxicity and severe side effects associated with non-selective PI3K inhibitors has limited their clinical applications (50–53). An in-depth understanding of PI3K signaling will help improve the therapeutic efficacy of PI3K-based glioblastoma therapies.
Table 1. Phosphatidylinositol-4,5-bisphosphate 3-kinase (PI3K) inhibitors in clinical trials for glioblastoma in the United States.
Based on the structural differences, specificities to their substrates, and differences in modes of regulation, PI3K genes are grouped into three classes (I, II, and III) (26). Class I PI3K genes control the activity of PI3K/AKT signaling and are often genetically altered in glioblastoma (29). Class II PI3K genes, while not well studied, are implicated in regulating angiogenesis and cilium function (26). Class III PI3K genes are primarily involved in the regulation of autophagy (54). In this review, we will focus on class I PI3K genes owing to their essential roles in PI3K/AKT signaling pathway and glioblastoma. The differential regulation of class I PI3K genes further divides this class into two subclasses: IA and IB. The class IA PI3K gene family consists of three highly homologous catalytic subunits PIK3CA, PIK3CB, and PIK3CD (PI3K catalytic subunit α, β, and δ) that encode p110α, p110β, and p110δ, respectively. These subunits form a complex with any of five regulatory subunits p85α, p55α (a splicing variant of p85α), p50α (a splicing variant of p85α), p85β, and p55γ, encoded by PIK3R1, PIK3R2, and PIK3R3 (PI3K regulatory subunit 1, 2, and 3), respectively. Class IB PI3K is composed of one catalytic subunit p110γ encoded by PIK3CG (PI3K catalytic subunit γ) and two regulatory subunits: p101 encoded by PIK3R5 (PI3K regulatory subunit 5) and p87 (also known as p84 or p87PIKAP) encoded by PIK3R6 (PI3K regulatory subunit 6) (26, 55). Our recent work has revealed that class IB catalytic subunit p110γ is expressed at an undetected level in glioblastoma cells and blocking this specific subunit exhibits no cytotoxicity (56). As such, we will herein only discuss the role of class IA PI3K catalytic subunits in glioblastoma.
PIK3CA, frequently mutated in cancer (57), is often oncogenic (58–61); hence, much attention has been drawn on this particular PI3K subunit in many different cancers. The frequency of PIK3CA mutations in glioblastoma ranges from 4.3 to 26.7% due to diverse detection approaches and different sample sizes (29, 39, 40, 57, 62–65). For example, Broaderick et al. reported that 5 out of 105 glioblastoma patients harbored PIK3CA mutations (4.8%) (66), whereas PIK3CA mutations were detected in 4 samples when Sameul et al. analyzed 15 glioblastoma specimens (26.7%) (57). Genome-wide sequencing of 91 glioblastomas revealed a 6.6% mutation rate in the PIK3CA gene (29). However, frequencies of PIK3CA mutations detected by PCR amplification followed by DNA sequencing varied significantly as stated above. Based on the report from Kita et al. (62), PIK3CA mutations in primary (directly diagnosed as glioblastoma) or secondary (originated from low-grade gliomas) glioblastoma were 4.7% (5 out of 107) or 3.1% (1 out of 32), respectively. To date, there is no evidence showing that PIK3CA mutations alone are able to transform glia cells to induce the formation of glioblastoma. Additional studies investigating the role of PIK3CA mutants in glioblastoma are therefore needed.
Our laboratory recently analyzed the gene expression profile and clinical data from 99 recurrent glioblastomas retrieved from the TCGA database. We found that PIK3CA mutations had no correlation with recurrence rate. In addition, levels of PIK3CA mRNAs had no significant association with recurrence risk and recurrence-associated patient survival (56). In the same study, we knocked down PIK3CA/p110α in a panel of glioblastoma cell lines and found that loss of PIK3CA/p110α failed to both inactivate AKT and block the survival of A172, U87MG, SF295, and U251 glioblastoma cells. Our results together suggest that PIK3CA/p110α is dispensable for PI3K/AKT signaling in glioblastoma, and perhaps the progression of this deadly disease. Consistent to our results, depletion of PIK3CA using short hairpin RNAs (shRNAs) did not decrease levels of active AKT in U251 and U87MG cells and failed to inhibit the viability of U251 cells or the growth of U87MG xenograft tumors (67–69). However, inconsistent or contradictory results have been shown in some other studies. For example, Weber et al. reported that knockdown of PIK3CA/p110α blocked the survival and migration of SKMG26, D54, and primary glioblastoma cells (70). In combination with temozolomide or carmustine, small interfering RNAs (siRNAs) of PIK3CA and AKT3 substantially reduced the viability of T98G glioblastoma cells (71). Future studies should focus on clarifying the role of PIK3CA/p110α in glioblastoma using patient-derived primary glioblastoma cells in conjunction with orthotopic glioblastoma models or genetically engineered mouse glioblastoma models.
In our recent work, we tested a panel of p110α-specific inhibitors (PIK75, BYL719, MLN1117, and HS173) in glioblastoma. PIK75 and HS173 significantly inhibited the viability of glioblastoma cell lines and primary tumor cells, whereas MLN1117 and BYL719 only showed modest toxicity (56). Congruently, other studies showed that PIK75 at 100 nM blocked the growth of U87MG cells (72) or T98G cells in culture or in animals (73), whereas BYL-719 alone did not induce a remarkable growth inhibition in LN229 and U87MG cells (74). As stated earlier, p110α inhibitors often induce hyperglycemia in patients (50, 75, 76). This is consistent with our observation that p110α inhibitors are significantly toxic to astrocytes (IC50 ranging from 0.1 to 8 µM) (56). Hence, it is perhaps difficult to utilize p110α-specific inhibitors as cancer drugs due to their limited therapeutic window.
Compared to PIK3CA, oncogenic PIK3CB mutations are rare. Several studies have recently revealed that mutations at the residue D1067 (PIK3CBD1067Y, D1067A, or D1067V) display oncogenic potential, and confer resistance to erlotinib [an inhibitor of epidermal growth factor receptor (EGFR)] in non-small-cell lung cancer or decrease the sensitivity of breast cancer cells to pictilisib (also known as GDC-0941, an inhibitor of p110α/δ) (77, 78). Similarly, PIK3CBA1048V induces tumor formation with a short latency after introduced into human embryonic kidney HA1E-M cells (79). Intriguingly, PIK3CBD1067A and PIK3CBD1067V have been found in two glioblastoma patients based on the TCGA genomic sequencing data of 599 glioblastoma tissues, indicative of an approximately 0.3% mutation rate. Other than oncogenic mutants described above, wild-type PIK3CB/p110β protein is able to induce tumor formation. Biochemical studies have revealed that the amino acid K342 in wild type p110β exhibits structural changes (i.e., disrupted interactions between p110β and its regulatory partner p85), which endows p110β with an unusually high transformation potential similar to the oncogenic p110α mutant p110α-N345K (80). In fact, p110β is proven to be essential for the formation of prostate and breast cancer in mouse models (81, 82) and for tumors driven by the loss of PTEN (68, 81) or GPCRs (26, 83–85).
In search of novel survival factors for glioblastoma, we recently identified 20 kinase genes important for the survival of U87MG cells (86). Through exploring the relationship of these candidates with glioblastoma recurrence using the TCGA patient data, we found that high levels of PIK3CB mRNAs correlated with high incidence and poor survival of glioblastoma recurrence (86). Compared to other class IA PI3K isoforms, PIK3CB was the only PI3K catalytic subunit that showed a strong association with recurrence rate, risk, and prognosis (56). In line with the observation that PIK3CA mutations and levels of PTEN exhibited no relationship with glioblastoma disease progression (56), the above results have demonstrated that PIK3CB is more important than other PI3K catalytic subunits and PTEN in the diagnosis and prognosis of glioblastoma recurrence.
Our recent work also determined the role of PI3K catalytic subunits in AKT signaling and survival of glioblastoma cells (56). First, we found that levels of p110β, but not other p110 isoforms or PTEN, strongly correlated with levels of active AKT in nine glioblastoma cell lines, eight primary glioblastoma cells, and six lines of glioblastoma stem cells. In U87MG cells that express high levels of p110β (p110βhigh), depletion of p110β inactivated AKT, whereas knockdown of other p110 proteins had no effect. In contrast, p110β knockdown failed to mitigate AKT activity in A172 cells expressing a low level of p110β (p110βlow). These results were further substantiated by the observation that p110β-selective inhibitors are more specific in deactivating AKT than other PI3K isoform-selective inhibitors. These results demonstrate that p110β dictates AKT activation and promotes cell survival in p110βhigh glioblastoma cells. This conclusion is further supported by the results from other studies. Zhao et al. showed that the p110β-specific inhibitor TGX-221 significantly decreased the levels of phosphorylated AKT at both S473 and T308 (pAKTS473 and pAKTT308) in U87MG cells, whereas the p110α inhibitor PIK75 and p110δ inhibitor CAL101 only induced a reduction of pAKTT308, but not pAKTS473 (72). This difference may explain why SP600125 (an inhibitor of c-Jun N-terminal kinases) only synergizes with TGX-221, but not PIK75 or CAL101, to inhibit cell viability and tumor growth (72). Chen et al. (67) and Pu et al. (87) also reported that shRNAs of PIK3CB, but not PIK3CA, inactivated AKT and decreased cell viability of U251 cells. More importantly, depletion of PIK3CB substantially blocked the growth of U251 xenografts in mice. Similarly, Wee et al. (68) and Millan-Ucles et al. (88) showed that siRNAs or shRNAs of PIK3CB, but not shRNAs of PIK3CA, inhibited the growth of U87MG cells.
We exploited a panel of PI3K isoform-selective inhibitors and tested their activities on glioblastoma cell viability (56). The p110β inhibitor TGX-221 and GSK2636771 mitigated the proliferation of p110βhigh U87MG and SF295 cells while having no effect on p110βlow A172 and LN229 cells. The p110α inhibitor PIK75 showed a strong cytotoxicity to all glioblastoma cell lines tested, whereas another p110α inhibitor BYL719 failed to display selective growth inhibition in p110αhigh cells. To provide a possible explanation for the unusually high toxicity of PIK-75, we monitored activity of AKT and extracellular signal-regulated kinase (ERK) and found that PIK-75 inhibited both AKT and ERK, suggesting that this particular p110α inhibitor is not a selective inhibitor of PI3K/AKT. By contrast, the p110β inhibitor TGX-221 only attenuated the activity of AKT, but not ERK. Hence, p110β inhibitors are more selective in affecting PI3K activities in glioblastoma. Relevant to clinical applications, IC50s of p110β inhibitors in human astrocytes are relatively high (100.4 and 291 µM, respectively). In stark contrast, other PI3K inhibitors robustly block astrocyte growth with IC50s ranging from 0.1 to 21.2 µM. Given this low cytotoxicity to normal astrocytes, selectively targeting PIK3CB/p110β is expected to have minimal side effects, thereby representing an appealing approach in treating glioblastoma.
A PIK3CD/p110δ inhibitor idelalisib had achieved a plausible clinical outcome in treating chronic lymphocytic leukemia (CLL) (89). While PIK3CD/p110δ has been well studied in blood cancers, little is known about the role of this subunit in glioblastoma. We have probed different glioblastoma cells with a PIK3CD/p110δ antibody (56). We found that this subunit was differentially expressed in glioblastoma cells and its expression levels did not correlate with AKT activation. Moreover, knockdown or inhibitors of PIK3CD/p110δ failed to inhibit AKT and cell viability. Our results suggest that PIK3CD/p110δ plays a dispensable role in the disease progression of glioblastoma. This conclusion is also supported by studies from Jones et al. (90), Holland et al. (73), and Zhao et al. (72). In these studies, treatment of U87MG, T98G, or LN18 cells with PIK3CD/p110δ inhibitors CAL101 or IC87114 displayed no or negligible cytotoxicity.
However, Schulte et al. have shown that expression of PIK3CD/p110δ, but not other PI3K catalytic subunits, is significantly enhanced in BS153 glioblastoma cells with acquired resistance to the EGFR inhibitor erlotinib (91). siRNA of p110δ restores erlotinib sensitivity in BS153 cells. In another study, siRNA-mediated knockdown of p110δ mitigates the migration of U87MG cells, whereas siRNAs of p110α and p110β exhibit no activity (92). Together, these results suggest that PIK3CD/p110δ regulates the migration, but not the survival, of glioblastoma cells. Nonetheless, the role of this subunit in glioblastoma and whether targeting PIK3CD/p110δ is an effective treatment for glioblastoma require further research.
A growing body of information strongly suggests that the four PI3K catalytic subunits are not functionally redundant in normal and malignant tissues. For example, insulin signaling often requires p110α, but not p110β, suggesting that blockade of p110α may be more harmful to normal tissue than inhibiting p110β (81, 93–96). Indeed, p110α inhibitors often induce hyperglycemia in patients (50, 75, 76). Two other PI3K catalytic subunits, p110γ and p110δ, are highly expressed in immune cells and have been shown to be more involved in maintaining homeostasis of the immune system and in the development of blood malignancies (97–100). The p110δ inhibitor, idelalisib (CAL-101), has been recently approved by the Food and Drug Administration to treat relapsed CLL, and this drug has achieved an overall response in more than 70% of patients in a phase I clinical trial (89). Furthermore, a combination of idelalisib and rituximab (cytotoxic anti-CD20 antibody) resulted in a 95% overall response, including a 19% complete response of relapsed CLL patients (101).
As we discussed above, PIK3CB/p110β is more important in cell survival than other PI3K catalytic subunits in glioblastoma. Similar results have also been shown in colorectal and breast cancer (68, 81, 100). One possible explanation is that PI3K catalytic subunits are differentially expressed in these cancers. Another possible reason is that upstream activators of PI3K catalytic subunits differ in these cancers. In fact, RTKs often signal through p110α, p110β, or p110γ, whereas GPCRs selectively activate p110β (26, 83, 102, 103). Herein, we speculate that GPCRs preferentially activate PIK3CB/p110β, but not other PI3K catalytic subunits, in glioblastoma expressing high levels of PIK3CB/p110β. This preferential activation of PIK3CB/p110β results in sustained cell survival, which promotes glioblastoma progression by inducing drug resistance and leading to tumor recurrence (Figure 1). Hence, PI3K catalytic subunits have different assignments in normal and malignant tissues, highlighting the importance of selectively targeting one PI3K catalytic subunit to treat cancer.
Figure 1. PIK3CB/p110β in glioblastoma. This figure illustrates a PIK3CB/p110β-dictated survival pathway in glioblastoma. Receptor tyrosine kinases (RTKs) or G protein-coupled receptors (GPCRs) selectively activates PIK3CB/p110β (but not PIK3CA/p110α or PIK3CD/p110δ), leading to production of phosphatidylinositol-3,4,5-triphosphate (PIP3) and subsequent phosphorylation of AKT. The activation of this signaling pathway promotes cancer cell survival while inhibiting cell death, the consequences of which are drug resistance and tumor recurrence. Hence, PIK3CB/p110β promotes glioblastoma disease progression and targeting PIK3CB/p110β using selective inhibitors represents a novel and effective approach to treating glioblastoma.
Research described above has demonstrated that PI3K catalytic subunits are not functionally redundant in glioblastoma. In particular, research from our group strongly suggests that PIK3CB/p110β is a dominant PI3K catalytic subunit that dictates PI3K/AKT signaling, thereby becoming a selective survival factor for glioblastoma. Future research will focus on elucidating the molecular mechanisms underlying the selective activation of p110β/AKT signaling in p110βhigh glioblastoma. These details at the molecular level will shed light on the design and production of PIK3CB/p110β-selective inhibitors with stronger inhibition of glioblastoma and less toxicity to normal brain tissues. Taken together, PI3K catalytic subunits play divergent roles in glioblastoma, underscoring the importance and necessity of selectively targeting one PI3K subunit to treat glioblastoma.
ZS: conception of this review article, preparation of figures, and manuscript writing. KP: preparation of chapter I and manuscript editing. RV: preparation of chapter I and Table 1 and manuscript editing.
The authors declare that the research was conducted in the absence of any commercial or financial relationships that could be construed as a potential conflict of interest.
This work was supported by the Start-up funds from Virginia Tech Carilion Research Institute provided to ZS.
1. Siegel RL, Miller KD, Jemal A. Cancer statistics, 2016. CA Cancer J Clin (2016) 66:7–30. doi:10.3322/caac.21332
2. Miller KD, Siegel RL, Lin CC, Mariotto AB, Kramer JL, Rowland JH, et al. Cancer treatment and survivorship statistics, 2016. CA Cancer J Clin (2016) 66:271–89. doi:10.3322/caac.21349
3. Ostrom QT, Gittleman H, Fulop J, Liu M, Blanda R, Kromer C, et al. Primary brain and central nervous system tumors diagnosed in the United States in 2008–2012. Neuro Oncol (2015) 17(Suppl 4):iv1–62. doi:10.1093/neuonc/nov189
4. Ostrom QT, Gittleman H, Liao P, Rouse C, Chen Y, Dowling J, et al. CBTRUS statistical report: primary brain and central nervous system tumors diagnosed in the United States in 2007–2011. Neuro Oncol (2014) 16(Suppl 4):iv1–63. doi:10.1093/neuonc/nou223
5. Ostrom QT, Gittleman H, Farah P, Ondracek A, Chen Y, Wolinsky Y, et al. CBTRUS statistical report: primary brain and central nervous system tumors diagnosed in the United States in 2006–2010. Neuro Oncol (2013) 15(Suppl 2):ii1–56. doi:10.1093/neuonc/not151
6. Dolecek TA, Propp JM, Stroup NE, Kruchko C. CBTRUS statistical report: primary brain and central nervous system tumors diagnosed in the United States in 2005–2009. Neuro Oncol (2012) 14(Suppl 5):v1–49. doi:10.1093/neuonc/nos218
7. Weller M, Cloughesy T, Perry JR, Wick W. Standards of care for treatment of recurrent glioblastoma – are we there yet? Neuro Oncol (2013) 15:4–27. doi:10.1093/neuonc/nos273
8. Kim HR, Kim KH, Kong DS, Seol HJ, Nam DH, Lim DH, et al. Outcome of salvage treatment for recurrent glioblastoma. J Clin Neurosci (2015) 22:468–73. doi:10.1016/j.jocn.2014.09.018
9. Hasan S, Chen E, Lanciano R, Yang J, Hanlon A, Lamond J, et al. Salvage fractionated stereotactic radiotherapy with or without chemotherapy and immunotherapy for recurrent glioblastoma multiforme: a single institution experience. Front Oncol (2015) 5:106. doi:10.3389/fonc.2015.00106
10. Gallego O. Nonsurgical treatment of recurrent glioblastoma. Curr Oncol (2015) 22:e273–81. doi:10.3747/co.22.2436
11. Taylor JW, Dietrich J, Gerstner ER, Norden AD, Rinne ML, Cahill DP, et al. Phase 2 study of bosutinib, a Src inhibitor, in adults with recurrent glioblastoma. J Neurooncol (2015) 121:557–63. doi:10.1007/s11060-014-1667-z
12. Pitz MW, Eisenhauer EA, MacNeil MV, Thiessen B, Easaw JC, Macdonald DR, et al. Phase II study of PX-866 in recurrent glioblastoma. Neuro Oncol (2015) 17:1270–4. doi:10.1093/neuonc/nou365
13. Lassman AB, Pugh SL, Gilbert MR, Aldape KD, Geinoz S, Beumer JH, et al. Phase 2 trial of dasatinib in target-selected patients with recurrent glioblastoma (RTOG 0627). Neuro Oncol (2015) 17:992–8. doi:10.1093/neuonc/nov011
14. Hacibekiroglu I, Kodaz H, Erdogan B, Turkmen E, Ozcelik M, Esenkaya A, et al. Single-agent bevacizumab is an effective treatment in recurrent glioblastoma. Med Oncol (2015) 32:460. doi:10.1007/s12032-014-0460-3
15. Hassler MR, Vedadinejad M, Flechl B, Haberler C, Preusser M, Hainfellner JA, et al. Response to imatinib as a function of target kinase expression in recurrent glioblastoma. Springerplus (2014) 3:111. doi:10.1186/2193-1801-3-111
16. Muhic A, Poulsen HS, Sorensen M, Grunnet K, Lassen U. Phase II open-label study of nintedanib in patients with recurrent glioblastoma multiforme. J Neurooncol (2013) 111:205–12. doi:10.1007/s11060-012-1009-y
17. Gilbert MR, Kuhn J, Lamborn KR, Lieberman F, Wen PY, Mehta M, et al. Cilengitide in patients with recurrent glioblastoma: the results of NABTC 03-02, a phase II trial with measures of treatment delivery. J Neurooncol (2012) 106:147–53. doi:10.1007/s11060-011-0650-1
18. Wen PY, Schiff D, Cloughesy TF, Raizer JJ, Laterra J, Smitt M, et al. A phase II study evaluating the efficacy and safety of AMG 102 (rilotumumab) in patients with recurrent glioblastoma. Neuro Oncol (2011) 13:437–46. doi:10.1093/neuonc/noq198
19. Iwamoto FM, Lamborn KR, Kuhn JG, Wen PY, Yung WK, Gilbert MR, et al. A phase I/II trial of the histone deacetylase inhibitor romidepsin for adults with recurrent malignant glioma: North American Brain Tumor Consortium Study 03-03. Neuro Oncol (2011) 13:509–16. doi:10.1093/neuonc/nor017
20. Yung WK, Vredenburgh JJ, Cloughesy TF, Nghiemphu P, Klencke B, Gilbert MR, et al. Safety and efficacy of erlotinib in first-relapse glioblastoma: a phase II open-label study. Neuro Oncol (2010) 12:1061–70. doi:10.1093/neuonc/noq072
21. Wick W, Puduvalli VK, Chamberlain MC, van den Bent MJ, Carpentier AF, Cher LM, et al. Phase III study of enzastaurin compared with lomustine in the treatment of recurrent intracranial glioblastoma. J Clin Oncol (2010) 28:1168–74. doi:10.1200/JCO.2009.23.2595
22. Thiessen B, Stewart C, Tsao M, Kamel-Reid S, Schaiquevich P, Mason W, et al. A phase I/II trial of GW572016 (lapatinib) in recurrent glioblastoma multiforme: clinical outcomes, pharmacokinetics and molecular correlation. Cancer Chemother Pharmacol (2010) 65:353–61. doi:10.1007/s00280-009-1041-6
23. Engelman JA, Luo J, Cantley LC. The evolution of phosphatidylinositol 3-kinases as regulators of growth and metabolism. Nat Rev Genet (2006) 7:606–19. doi:10.1038/nrg1879
24. Cantley LC. The phosphoinositide 3-kinase pathway. Science (2002) 296:1655–7. doi:10.1126/science.296.5573.1655
25. Li X, Wu C, Chen N, Gu H, Yen A, Cao L, et al. PI3K/Akt/mTOR signaling pathway and targeted therapy for glioblastoma. Oncotarget (2016) 7:33440–50. doi:10.18632/oncotarget.7961
26. Thorpe LM, Yuzugullu H, Zhao JJ. PI3K in cancer: divergent roles of isoforms, modes of activation and therapeutic targeting. Nat Rev Cancer (2015) 15:7–24. doi:10.1038/nrc3860
27. Viaud J, Mansour R, Antkowiak A, Mujalli A, Valet C, Chicanne G, et al. Phosphoinositides: important lipids in the coordination of cell dynamics. Biochimie (2016) 125:250–8. doi:10.1016/j.biochi.2015.09.005
28. Wymann MP, Schultz C. The chemical biology of phosphoinositide 3-kinases. Chembiochem (2012) 13:2022–35. doi:10.1002/cbic.201200089
29. N. Cancer Genome Atlas Research. Comprehensive genomic characterization defines human glioblastoma genes and core pathways. Nature (2008) 455:1061–8. doi:10.1038/nature07385
30. Masica DL, Karchin R. Correlation of somatic mutation and expression identifies genes important in human glioblastoma progression and survival. Cancer Res (2011) 71:4550–61. doi:10.1158/0008-5472.CAN-11-0180
31. Koul D. PTEN signaling pathways in glioblastoma. Cancer Biol Ther (2008) 7:1321–5. doi:10.4161/cbt.7.9.6954
32. Nickel GC, Barnholtz-Sloan J, Gould MP, McMahon S, Cohen A, Adams MD, et al. Characterizing mutational heterogeneity in a glioblastoma patient with double recurrence. PLoS One (2012) 7:e35262. doi:10.1371/journal.pone.0035262
33. Martinez R, Schackert HK, von Kannen S, Lichter P, Joos S, Schackert G. Independent molecular development of metachronous glioblastomas with extended intervening recurrence-free interval. Brain Pathol (2003) 13:598–607. doi:10.1111/j.1750-3639.2003.tb00488.x
34. Hulsebos TJ, Troost D, Leenstra S. Molecular-genetic characterisation of gliomas that recur as same grade or higher grade tumours. J Neurol Neurosurg Psychiatry (2004) 75:723–6. doi:10.1136/jnnp.2003.025031
35. Ohgaki H, Kleihues P. Population-based studies on incidence, survival rates, and genetic alterations in astrocytic and oligodendroglial gliomas. J Neuropathol Exp Neurol (2005) 64:479–89. doi:10.1093/jnen/64.6.479
36. Toth J, Egervari K, Klekner A, Bognar L, Szanto J, Nemes Z, et al. Analysis of EGFR gene amplification, protein over-expression and tyrosine kinase domain mutation in recurrent glioblastoma. Pathol Oncol Res (2009) 15:225–9. doi:10.1007/s12253-008-9082-4
37. Martinez R, Rohde V, Schackert G. Different molecular patterns in glioblastoma multiforme subtypes upon recurrence. J Neurooncol (2010) 96:321–9. doi:10.1007/s11060-009-9967-4
38. Parsons DW, Jones S, Zhang X, Lin JC, Leary RJ, Angenendt P, et al. An integrated genomic analysis of human glioblastoma multiforme. Science (2008) 321:1807–12. doi:10.1126/science.1164382
39. Hartmann C, Bartels G, Gehlhaar C, Holtkamp N, von Deimling A. PIK3CA mutations in glioblastoma multiforme. Acta Neuropathol (2005) 109:639–42. doi:10.1007/s00401-005-1000-1
40. Knobbe CB, Trampe-Kieslich A, Reifenberger G. Genetic alteration and expression of the phosphoinositol-3-kinase/Akt pathway genes PIK3CA and PIKE in human glioblastomas. Neuropathol Appl Neurobiol (2005) 31:486–90. doi:10.1111/j.1365-2990.2005.00660.x
41. Lino MM, Merlo A. PI3Kinase signaling in glioblastoma. J Neurooncol (2011) 103:417–27. doi:10.1007/s11060-010-0442-z
42. Cheng CK, Fan QW, Weiss WA. PI3K signaling in glioma – animal models and therapeutic challenges. Brain Pathol (2009) 19:112–20. doi:10.1111/j.1750-3639.2008.00233.x
43. Vanhaesebroeck B, Ali K, Bilancio A, Geering B, Foukas LC. Signalling by PI3K isoforms: insights from gene-targeted mice. Trends Biochem Sci (2005) 30:194–204. doi:10.1016/j.tibs.2005.02.008
44. Lee JK, Wang J, Sa JK, Ladewig E, Lee HO, Lee IH, et al. Spatiotemporal genomic architecture informs precision oncology in glioblastoma. Nat Genet (2017) 49:594–9. doi:10.1038/ng.3806
45. Kubota N, Okada S, Inada T, Ohnishi K, Ohnishi T. Wortmannin sensitizes human glioblastoma cell lines carrying mutant and wild type TP53 gene to radiation. Cancer Lett (2000) 161:141–7. doi:10.1016/S0304-3835(00)00614-5
46. Westhoff MA, Kandenwein JA, Karl S, Vellanki SH, Braun V, Eramo A, et al. The pyridinylfuranopyrimidine inhibitor, PI-103, chemosensitizes glioblastoma cells for apoptosis by inhibiting DNA repair. Oncogene (2009) 28:3586–96. doi:10.1038/onc.2009.215
47. Koul D, Shen R, Kim YW, Kondo Y, Lu Y, Bankson J, et al. Cellular and in vivo activity of a novel PI3K inhibitor, PX-866, against human glioblastoma. Neuro Oncol (2010) 12:559–69. doi:10.1093/neuonc/nop058
48. Prasad G, Sottero T, Yang X, Mueller S, James CD, Weiss WA, et al. Inhibition of PI3K/mTOR pathways in glioblastoma and implications for combination therapy with temozolomide. Neuro Oncol (2011) 13:384–92. doi:10.1093/neuonc/noq193
49. Carminati PO, Donaires FS, Marques MM, Donadi EA, Passos GA, Sakamoto-Hojo ET. Cisplatin associated with LY294002 increases cytotoxicity and induces changes in transcript profiles of glioblastoma cells. Mol Biol Rep (2014) 41:165–77. doi:10.1007/s11033-013-2849-z
50. Yap TA, Bjerke L, Clarke PA, Workman P. Drugging PI3K in cancer: refining targets and therapeutic strategies. Curr Opin Pharmacol (2015) 23:98–107. doi:10.1016/j.coph.2015.05.016
51. Fruman DA, Rommel C. PI3K and cancer: lessons, challenges and opportunities. Nat Rev Drug Discov (2014) 13:140–56. doi:10.1038/nrd4204
52. Rodon J, Dienstmann R, Serra V, Tabernero J. Development of PI3K inhibitors: lessons learned from early clinical trials. Nat Rev Clin Oncol (2013) 10:143–53. doi:10.1038/nrclinonc.2013.10
53. Akinleye A, Avvaru P, Furqan M, Song Y, Liu D. Phosphatidylinositol 3-kinase (PI3K) inhibitors as cancer therapeutics. J Hematol Oncol (2013) 6:88. doi:10.1186/1756-8722-6-88
54. Pasquier B. Autophagy inhibitors. Cell Mol Life Sci (2016) 73:985–1001. doi:10.1007/s00018-015-2104-y
55. Martini M, De Santis MC, Braccini L, Gulluni F, Hirsch E. PI3K/AKT signaling pathway and cancer: an updated review. Ann Med (2014) 46:372–83. doi:10.3109/07853890.2014.912836
56. Pridham KJ, Le L, Guo S, Varghese RT, Algino S, Liang Y, et al. PIK3CB/p110beta is a selective survival factor for glioblastoma. Neuro Oncol (2017). doi:10.1093/neuonc/nox181
57. Samuels Y, Wang Z, Bardelli A, Silliman N, Ptak J, Szabo S, et al. High frequency of mutations of the PIK3CA gene in human cancers. Science (2004) 304:554. doi:10.1126/science.1096502
58. Shibata T, Kokubu A, Tsuta K, Hirohashi S. Oncogenic mutation of PIK3CA in small cell lung carcinoma: a potential therapeutic target pathway for chemotherapy-resistant lung cancer. Cancer Lett (2009) 283:203–11. doi:10.1016/j.canlet.2009.03.038
59. Chaussade C, Cho K, Mawson C, Rewcastle GW, Shepherd PR. Functional differences between two classes of oncogenic mutation in the PIK3CA gene. Biochem Biophys Res Commun (2009) 381:577–81. doi:10.1016/j.bbrc.2009.02.081
60. Hafner C, Lopez-Knowles E, Luis NM, Toll A, Baselga E, Fernandez-Casado A, et al. Oncogenic PIK3CA mutations occur in epidermal nevi and seborrheic keratoses with a characteristic mutation pattern. Proc Natl Acad Sci U S A (2007) 104:13450–4. doi:10.1073/pnas.0705218104
61. Kozaki K, Imoto I, Pimkhaokham A, Hasegawa S, Tsuda H, Omura K, et al. PIK3CA mutation is an oncogenic aberration at advanced stages of oral squamous cell carcinoma. Cancer Sci (2006) 97:1351–8. doi:10.1111/j.1349-7006.2006.00343.x
62. Kita D, Yonekawa Y, Weller M, Ohgaki H. PIK3CA alterations in primary (de novo) and secondary glioblastomas. Acta Neuropathol (2007) 113:295–302. doi:10.1007/s00401-006-0186-1
63. Gallia GL, Rand V, Siu IM, Eberhart CG, James CD, Marie SK, et al. PIK3CA gene mutations in pediatric and adult glioblastoma multiforme. Mol Cancer Res (2006) 4:709–14. doi:10.1158/1541-7786.MCR-06-0172
64. Bleeker FE, Lamba S, Zanon C, Molenaar RJ, Hulsebos TJ, Troost D, et al. Mutational profiling of kinases in glioblastoma. BMC Cancer (2014) 14:718. doi:10.1186/1471-2407-14-718
65. Tabone T, Abuhusain HJ, Nowak AK, Australian G, Australian Genomics and Clinical Outcome of Glioma (AGOG) NetworkErber WN, et al. Multigene profiling to identify alternative treatment options for glioblastoma: a pilot study. J Clin Pathol (2014) 67:550–5. doi:10.1136/jclinpath-2014-202173
66. Broderick DK, Di C, Parrett TJ, Samuels YR, Cummins JM, McLendon RE, et al. Mutations of PIK3CA in anaplastic oligodendrogliomas, high-grade astrocytomas, and medulloblastomas. Cancer Res (2004) 64:5048–50. doi:10.1158/0008-5472.CAN-04-1170
67. Chen H, Mei L, Zhou L, Shen X, Guo C, Zheng Y, et al. PTEN restoration and PIK3CB knockdown synergistically suppress glioblastoma growth in vitro and in xenografts. J Neurooncol (2011) 104:155–67. doi:10.1007/s11060-010-0492-2
68. Wee S, Wiederschain D, Maira SM, Loo A, Miller C, deBeaumont R, et al. PTEN-deficient cancers depend on PIK3CB. Proc Natl Acad Sci U S A (2008) 105:13057–62. doi:10.1073/pnas.0802655105
69. Opel D, Westhoff MA, Bender A, Braun V, Debatin KM, Fulda S. Phosphatidylinositol 3-kinase inhibition broadly sensitizes glioblastoma cells to death receptor- and drug-induced apoptosis. Cancer Res (2008) 68:6271–80. doi:10.1158/0008-5472.CAN-07-6769
70. Weber GL, Parat MO, Binder ZA, Gallia GL, Riggins GJ. Abrogation of PIK3CA or PIK3R1 reduces proliferation, migration, and invasion in glioblastoma multiforme cells. Oncotarget (2011) 2:833–49. doi:10.18632/oncotarget.346
71. Paul-Samojedny M, Pudelko A, Kowalczyk M, Fila-Danilow A, Suchanek-Raif R, Borkowska P, et al. Combination therapy with AKT3 and PI3KCA siRNA enhances the antitumor effect of temozolomide and carmustine in T98G glioblastoma multiforme cells. BioDrugs (2016) 30:129–44. doi:10.1007/s40259-016-0160-y
72. Zhao HF, Wang J, Jiang HR, Chen ZP, To SS. PI3K p110beta isoform synergizes with JNK in the regulation of glioblastoma cell proliferation and migration through Akt and FAK inhibition. J Exp Clin Cancer Res (2016) 35:78. doi:10.1186/s13046-016-0356-5
73. Holand K, Boller D, Hagel C, Dolski S, Treszl A, Pardo OE, et al. Targeting class IA PI3K isoforms selectively impairs cell growth, survival, and migration in glioblastoma. PLoS One (2014) 9:e94132. doi:10.1371/journal.pone.0094132
74. Iqbal A, Eckerdt F, Bell J, Nakano I, Giles FJ, Cheng SY, et al. Targeting of glioblastoma cell lines and glioma stem cells by combined PIM kinase and PI3K-p110alpha inhibition. Oncotarget (2016) 7:33192–201. doi:10.18632/oncotarget.8899
75. Yang Q, Modi P, Newcomb T, Queva C, Gandhi V. Idelalisib: first-in-class PI3K delta inhibitor for the treatment of chronic lymphocytic leukemia, small lymphocytic leukemia, and follicular lymphoma. Clin Cancer Res (2015) 21:1537–42. doi:10.1158/1078-0432.CCR-14-2034
76. Furman RR, Sharman JP, Coutre SE, Cheson BD, Pagel JM, Hillmen P, et al. Idelalisib and rituximab in relapsed chronic lymphocytic leukemia. N Engl J Med (2014) 370:997–1007. doi:10.1056/NEJMc1402716
77. Nakanishi Y, Walter K, Spoerke JM, O’Brien C, Huw LY, Hampton GM, et al. Activating mutations in PIK3CB confer resistance to PI3K inhibition and define a novel oncogenic role for p110beta. Cancer Res (2016) 76:1193–203. doi:10.1158/0008-5472.CAN-15-2201
78. Pazarentzos E, Giannikopoulos P, Hrustanovic G, St John J, Olivas VR, Gubens MA, et al. Oncogenic activation of the PI3-kinase p110beta isoform via the tumor-derived PIK3Cbeta(D1067V) kinase domain mutation. Oncogene (2016) 35:1198–205. doi:10.1038/onc.2015.173
79. Kim E, Ilic N, Shrestha Y, Zou L, Kamburov A, Zhu C, et al. Systematic functional interrogation of rare cancer variants identifies oncogenic alleles. Cancer Discov (2016) 6:714–26. doi:10.1158/2159-8290.CD-16-0160
80. Dbouk HA, Pang H, Fiser A, Backer JM. A biochemical mechanism for the oncogenic potential of the p110beta catalytic subunit of phosphoinositide 3-kinase. Proc Natl Acad Sci U S A (2010) 107:19897–902. doi:10.1073/pnas.1008739107
81. Jia S, Liu Z, Zhang S, Liu P, Zhang L, Lee SH, et al. Essential roles of PI(3)K-p110beta in cell growth, metabolism and tumorigenesis. Nature (2008) 454:776–9. doi:10.1038/nature07091
82. Zhao JJ, Liu Z, Wang L, Shin E, Loda MF, Roberts TM. The oncogenic properties of mutant p110alpha and p110beta phosphatidylinositol 3-kinases in human mammary epithelial cells. Proc Natl Acad Sci U S A (2005) 102:18443–8. doi:10.1073/pnas.0508988102
83. Dbouk HA, Vadas O, Shymanets A, Burke JE, Salamon RS, Khalil BD, et al. G protein-coupled receptor-mediated activation of p110beta by Gbetagamma is required for cellular transformation and invasiveness. Sci Signal (2012) 5:ra89. doi:10.1126/scisignal.2003264
84. Dbouk HA, Backer JM. A beta version of life: p110beta takes center stage. Oncotarget (2010) 1:729–33. doi:10.18632/oncotarget.207
85. Wang Q, Von T, Bronson R, Ruan M, Mu W, Huang A, et al. Spatially distinct roles of class Ia PI3K isoforms in the development and maintenance of PTEN hamartoma tumor syndrome. Genes Dev (2013) 27:1568–80. doi:10.1101/gad.216069.113
86. Varghese RT, Liang Y, Guan T, Franck CT, Kelly DF, Sheng Z. Survival kinase genes present prognostic significance in glioblastoma. Oncotarget (2016) 7:20140–51. doi:10.18632/oncotarget.7917
87. Pu P, Kang C, Zhang Z, Liu X, Jiang H. Downregulation of PIK3CB by siRNA suppresses malignant glioma cell growth in vitro and in vivo. Technol Cancer Res Treat (2006) 5:271–80. doi:10.1177/153303460600500308
88. Millan-Ucles A, Zuluaga S, Marques M, Vallejo-Diaz J, Sanz L, Cariaga-Martinez AE, et al. E-cadherin downregulation sensitizes PTEN-mutant tumors to PI3Kbeta silencing. Oncotarget (2016) 7:84054–71. doi:10.18632/oncotarget.13414
89. Brown JR, Byrd JC, Coutre SE, Benson DM, Flinn IW, Wagner-Johnston ND, et al. Idelalisib, an inhibitor of phosphatidylinositol 3-kinase p110delta, for relapsed/refractory chronic lymphocytic leukemia. Blood (2014) 123:3390–7. doi:10.1182/blood-2013-11-535047
90. Jones NM, Rowe MR, Shepherd PR, McConnell MJ. Targeted inhibition of dominant PI3-kinase catalytic isoforms increase expression of stem cell genes in glioblastoma cancer stem cell models. Int J Oncol (2016) 49:207–16. doi:10.3892/ijo.2016.3510
91. Schulte A, Liffers K, Kathagen A, Riethdorf S, Zapf S, Merlo A, et al. Erlotinib resistance in EGFR-amplified glioblastoma cells is associated with upregulation of EGFRvIII and PI3Kp110delta. Neuro Oncol (2013) 15:1289–301. doi:10.1093/neuonc/not093
92. Luk SK, Piekorz RP, Nurnberg B, Tony To SS. The catalytic phosphoinositol 3-kinase isoform p110delta is required for glioma cell migration and invasion. Eur J Cancer (2012) 48:149–57. doi:10.1016/j.ejca.2011.09.006
93. Foukas LC, Claret M, Pearce W, Okkenhaug K, Meek S, Peskett E, et al. Critical role for the p110alpha phosphoinositide-3-OH kinase in growth and metabolic regulation. Nature (2006) 441:366–70. doi:10.1038/nature04694
94. Graupera M, Guillermet-Guibert J, Foukas LC, Phng LK, Cain RJ, Salpekar A, et al. Angiogenesis selectively requires the p110alpha isoform of PI3K to control endothelial cell migration. Nature (2008) 453:662–6. doi:10.1038/nature06892
95. Sopasakis VR, Liu P, Suzuki R, Kondo T, Winnay J, Tran TT, et al. Specific roles of the p110alpha isoform of phosphatidylinsositol 3-kinase in hepatic insulin signaling and metabolic regulation. Cell Metab (2010) 11:220–30. doi:10.1016/j.cmet.2010.02.002
96. Guillermet-Guibert J, Bjorklof K, Salpekar A, Gonella C, Ramadani F, Bilancio A, et al. The p110beta isoform of phosphoinositide 3-kinase signals downstream of G protein-coupled receptors and is functionally redundant with p110gamma. Proc Natl Acad Sci U S A (2008) 105:8292–7. doi:10.1073/pnas.0707761105
97. Sasaki T, Irie-Sasaki J, Jones RG, Oliveira-dos-Santos AJ, Stanford WL, Bolon B, et al. Function of PI3Kgamma in thymocyte development, T cell activation, and neutrophil migration. Science (2000) 287:1040–6. doi:10.1126/science.287.5455.1040
98. Bi L, Okabe I, Bernard DJ, Nussbaum RL. Early embryonic lethality in mice deficient in the p110beta catalytic subunit of PI 3-kinase. Mamm Genome (2002) 13:169–72. doi:10.1007/BF02684023
99. Clayton E, Bardi G, Bell SE, Chantry D, Downes CP, Gray A, et al. A crucial role for the p110delta subunit of phosphatidylinositol 3-kinase in B cell development and activation. J Exp Med (2002) 196:753–63. doi:10.1084/jem.20020805
100. Ciraolo E, Iezzi M, Marone R, Marengo S, Curcio C, Costa C, et al. Phosphoinositide 3-kinase p110beta activity: key role in metabolism and mammary gland cancer but not development. Sci Signal (2008) 1:ra3. doi:10.1126/scisignal.1161577
101. O’Brien SM, Lamanna N, Kipps TJ, Flinn I, Zelenetz AD, Burger JA, et al. A phase 2 study of idelalisib plus rituximab in treatment-naive older patients with chronic lymphocytic leukemia. Blood (2015) 126:2686–94. doi:10.1182/blood-2015-03-630947
102. Khalil BD, Hsueh C, Cao Y, Abi Saab WF, Wang Y, Condeelis JS, et al. Tumor metastasis via PI3Kbeta. Cancer Res (2016) 76:2944–53. doi:10.1158/0008-5472.CAN-15-1675
Keywords: phosphatidylinositol-4,5-bisphosphate 3-kinase, glioblastoma, class IA phosphatidylinositol-4,5-bisphosphate 3-kinase catalytic subunits, PIK3CA, PIK3CB, PIK3CD
Citation: Pridham KJ, Varghese RT and Sheng Z (2017) The Role of Class IA Phosphatidylinositol-4,5-Bisphosphate 3-Kinase Catalytic Subunits in Glioblastoma. Front. Oncol. 7:312. doi: 10.3389/fonc.2017.00312
Received: 27 October 2017; Accepted: 04 December 2017;
Published: 15 December 2017
Edited by:
Justin Lathia, Cleveland Clinic Lerner College of Medicine, United StatesReviewed by:
Monica Venere, The Ohio State University, United StatesCopyright: © 2017 Pridham, Varghese and Sheng. This is an open-access article distributed under the terms of the Creative Commons Attribution License (CC BY). The use, distribution or reproduction in other forums is permitted, provided the original author(s) or licensor are credited and that the original publication in this journal is cited, in accordance with accepted academic practice. No use, distribution or reproduction is permitted which does not comply with these terms.
*Correspondence: Zhi Sheng, emhpc2hlbmdAdnRjLnZ0LmVkdQ==
Disclaimer: All claims expressed in this article are solely those of the authors and do not necessarily represent those of their affiliated organizations, or those of the publisher, the editors and the reviewers. Any product that may be evaluated in this article or claim that may be made by its manufacturer is not guaranteed or endorsed by the publisher.
Research integrity at Frontiers
Learn more about the work of our research integrity team to safeguard the quality of each article we publish.