- Mackenzie Cancer Research Group, Department of Pathology, University of Otago, Christchurch, New Zealand
Sufficient uptake and whole body distribution of vitamin C (ascorbate) is essential for many biochemical processes, including some that are vital for tumor growth and spread. Uptake of ascorbate into cancer cells is modulated by availability, tumor blood flow, tissue diffusion parameters, and ascorbate transport proteins. Uptake into cells is mediated by two families of transport proteins, namely, the solute carrier gene family 23, consisting of sodium-dependent vitamin C transporters (SVCTs) 1 and 2, and the SLC2 family of glucose transporters (GLUTs). GLUTs transport the oxidized form of the vitamin, dehydroascorbate (DHA), which is present at negligible to low physiological levels. SVCT1 and 2 are capable of accumulating ascorbate against a concentration gradient from micromolar concentrations outside to millimolar levels inside of cells. Investigating the expression and regulation of SVCTs in cancer has only recently started to be included in studies focused on the role of ascorbate in tumor formation, progression, and response to therapy. This review gives an overview of the current, limited knowledge of ascorbate transport across membranes, as well as tissue distribution, gene expression, and the relevance of SVCTs in cancer. As tumor ascorbate accumulation may play a role in the anticancer activity of high dose ascorbate treatment, further research into ascorbate transport in cancer tissue is vital.
Ascorbate and Cancer
The role of vitamin C (ascorbate) in cancer risk, progression, and therapy is not resolved (1–3), but cancer patients are frequently ascorbate deficient (4, 5). Ascorbate’s role is likely to involve a combination of several of the following proposed functions: (a) by acting as an electron donor it is a potent antioxidant (6), and has thus been proposed to interfere with some chemotherapy regimes (7); (b) ascorbate has prooxidant properties via its ability to reduce redox-active metals (8), and thus is proposed to directly act as a cytotoxin (9); and (c) ascorbate serves as a cofactor for a large family of Fe(II) and 2-oxoglutarate-dependent dioxygenases, which includes the collagen prolyl hydroxylases required for the formation of the tertiary structure of collagen, regulation of hypoxia-inducible transcription (HIF) factors required for tumor angiogenesis, treatment evasion and metastasis, and nucleotide hydroxylases involved in DNA demethylation, which affects global gene expression (10).
In cancer, ascorbate’s function as cofactor for the HIF hydroxylases is currently the most plausible, with several lines of evidence. Studies have shown that (a) in cultured cells, increasing intracellular ascorbate decreased HIF-1 activation (11–13), (b) in ascorbate-dependent mouse models, increasing circulating ascorbate via dietary intervention or ascorbate injections reduced tumor growth and hypoxia, and dampened HIF-1 activity (14–16), and (c) in tumor tissue from cancer patients with endometrial and colorectal cancer, increased ascorbate levels were associated with reduced HIF-1 pathway activity (17, 18). Importantly, tumor levels of ascorbate were associated with improved disease-free survival in colorectal cancer patients (18).
Ascorbate Uptake into Tumors
Uptake of ascorbate into cancer cells is modulated by availability, tumor blood flow, tissue diffusion parameters, and ascorbate transport proteins. Potential limiting factors to ascorbate uptake into solid tumors include (1) tissue characteristics, such as high interstitial fluid pressure and high cell density, (2) suboptimal vascular function, which includes avascular regions, immature and dysfunctional vessels, and varied blood flow, and (3) transporter concentration, location, and function. Data derived from a three-dimensional cellular diffusion model have suggested that ascorbate penetration into a poorly vascularized tumor may require higher than normal plasma concentrations (19). A recent pharmacokinetic study in mice has shown that in response to a single administration of high dose ascorbate, there was a prolonged presence of the vitamin in the tumor compared to plasma and liver, where ascorbate levels reduced rapidly (16), indicating that tumor accumulation may be different from normal tissue.
In patients with colorectal or endometrial cancer, where ascorbate levels of both tumor and uninvolved adjacent normal tissue were measured, no association between ascorbate levels in tumor tissue and matched normal tissue was apparent (Figure 1) (17, 18), indicating differences in tumor uptake. Transporter status of these clinical samples was not reported.
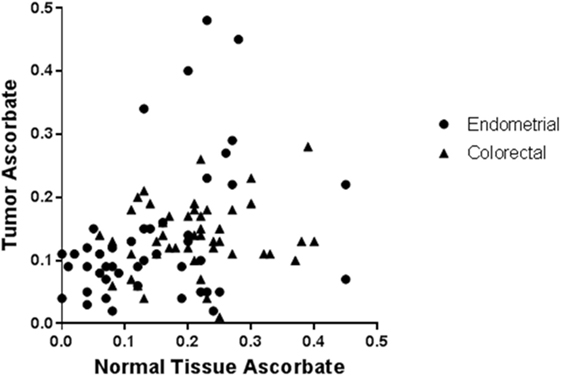
Figure 1. Ascorbate levels in normal tissue are not associated with ascorbate levels in tumors from endometrial and colorectal cancer patients. Tissue samples from patients with endometrial (n = 50) and colorectal cancer (n = 50) were processed, and ascorbate levels (nanomoles per microgram DNA) were measured using HPLC-EC [data from Ref. (17, 18)]. From each patient, samples were obtained from tumor and adjacent normal tissue. There was no association between ascorbate content in tumor vs. normal tissue in individual patients with endometrial or colorectal cancer (Pearson correlation: R2 = 0.001, p = 0.81, and R2 = 0.022, p = 0.30, respectively). Hence, whole body ascorbate status may not predict tumor ascorbate status.
Ascorbate Transport and Elimination
Maintenance of whole body ascorbate levels and distribution to different compartments is mediated by two families of transport proteins (20). While ascorbate is taken up into cells via sodium-dependent vitamin C transporters (SVCTs), its oxidized form, dehydroascorbate (DHA), is accumulated through facilitated diffusion via glucose transporters (GLUTs) (21, 22). A small proportion of ascorbate and DHA can also pass the plasma membrane via passive diffusion (23, 24).
Increased formation of DHA in the tumor microenvironment through oxidative stress (25) or via parenteral administration of high doses of DHA (26) has been proposed to enable the accumulation of ascorbate in cancer cells via GLUTs, but this has not been measured or proven. GLUT1 overexpression is associated with KRAS or BRAF mutations in colorectal tumors, thus potentially increasing DHA uptake in this important subset of patients (25). Here, KRAS-mutant colorectal cancer cells in culture preferentially took up DHA rather than ascorbate and were selectively sensitive to and killed by DHA (25). Yet, in a different study using a range of KRAS-mutant colorectal cell lines, ascorbate transport, and sensitivity was reliant on SVCT2, not GLUTs (27). Due to very low or undetectable physiological DHA concentrations in plasma (<10% of total ascorbate) (28, 29), its uptake is likely to be of minor importance in vivo, and DHA transport is therefore not discussed further in this review.
How ascorbate exits cells is not fully understood. Different mechanisms have been suggested, including volume-sensitive and Ca2+-dependent anion channels, gap-junction hemi-channels, exocytosis of secretory vesicles containing ascorbate, and homo- and hetero-exchange systems at the plasma membrane (30–33). Ascorbate efflux from, as opposed to uptake into cancer cells, and tumors as a whole, has not been studied.
Sodium-dependent vitamin C transporters are members of the solute carrier gene family 23 (SLC23), and each protein is comprised of 12 transmembrane domains (34). Currently, three isoforms have been identified, SVCT1 and 2 transport ascorbate, and the orphan receptor SVCT3 has still unknown function (35). SVCT1 and SVCT2 exert the cotransport of sodium and ascorbate in a ratio of 2:1 down an electrochemical sodium gradient, which is maintained by K/Na+ exchange mechanisms (36). This transport is sensitive to changes in temperature and pH with an optimum at pH 7.5 (37, 38). SVCT2 also relies on Ca2+ and Mg2+ for its activity (36). Expression of the different SVCT transport proteins is tissue and cell type specific and is controlled by transcriptional regulation of SLC23 genes (29, 35) and posttranslational regulation of the transporters (39). However, the exact regulation mechanisms of ascorbate transport proteins are still not fully understood.
The characteristics of SVCT1 and SVCT2, and their known relevance to cancer, are described in the next sections.
Sodium-Dependent Vitamin C Transporter 1
Distribution in Normal and Tumor Tissues
Sodium-dependent vitamin C transporter 1 (SLC23A1) is expressed in the epithelial tissue of kidney, intestine, liver, lung, and skin. In the kidney, SVCT1 is situated in the brush-border membrane of the proximal tubule where it mediates reuptake of ascorbate, thereby playing a major role in maintenance of whole body ascorbate levels (40). SVCT1 transports ascorbate with low affinity with a Km in the range of 65–237 µM, which makes it capable of high capacity uptake of ascorbate from the diet (36, 41). Only one study has measured SVCT1 protein and ascorbate levels in mouse tumors and reported low SVCT1 levels with little variation following ascorbate administration (16). Tissue distribution of SVCT1 in cancer patients has not been described.
SLC23A1 Polymorphisms and Cancer Risk
The gene (SLC23A1) is located on chromosome 5 and contains binding sites for the hepatocyte nuclear factor 1 transcription factor in its promoter region (42). In humans, no loss of SLC23A1 has been described to date. However, different single nucleotide polymorphisms (SNPs) in the coding region have been identified impairing ascorbate transport and reducing plasma levels (29, 41, 43). Studies focusing on the association of SLC23A1 variants and cancer risk gave conflicting results (29, 43–45). SNPs have been linked to increased risk of follicular lymphoma (rs6596473 G>C) (44), with no influence on gastric cancer (43) or advanced colorectal adenoma (45). However, plasma ascorbate levels were not measured in these reports, and further investigations are needed, to evaluate the link of SLC23A1 polymorphisms with disease risk.
Control of Gene Expression
Gene and protein expression studies of SVCT1 in cancer have not been widely reported. Svct1 mRNA levels were upregulated by bile acids in rat hepatoma cells (46), whereas glutathione depletion resulted in decreased Svct1 mRNA, protein levels and ascorbate transport (47). Human hepatoma cells, which unlike rat are incapable of synthesizing ascorbate, were unaffected by glutathione depletion (47). Inconsistent results were reported for colorectal cancer cells. In human colon carcinoma cells, high concentrations of ascorbate downregulated SVCT1 expression in vitro (48), while no difference in SVCT1 protein expression was observed in human colon adenocarcinoma samples compared to normal colon mucosa (49).
Posttranslational Modification
Ascorbate transport is not only regulated at the level of gene expression of SLC23A1 (42) but also via posttranscriptional regulation, and glycosylation and phosphorylation regulate SVCT1 activity (39). Translocation of SVCT1 carriers from the cytosol to the cell membrane was detected in human keratinocytes upon UVB irradiation, without changes in mRNA expression, indicating that cellular localization of SVCTs may be an important determinant of the ascorbate uptake rate (50). Yet, studies in cancer cells have not been conducted.
Sodium-Dependent Vitamin C Transporter 2
Distribution in Normal and Tumor Tissues
Sodium-dependent vitamin C transporter 2 is expressed in almost every tissue and cell in the body (29). It has been characterized as a low capacity, high affinity transporter with a transport Km of ~20 μM and can thus take up lower concentrations of ascorbate than SVCT1 (36). The SVCT2 transporter is highly expressed in the brain where it is essential for maintaining the high ascorbate levels needed for brain function and development (51, 52). In human bronchial epithelium, SVCT2 protein expression inversely correlated with ascorbate concentration in the respiratory tract lining fluid (53). SVCT2 protein was readily detected in Lewis lung tumors grown in ascorbate-dependent mice, and SVCT2 protein levels varied over time following a single high dose ascorbate injection, but their association with tumor ascorbate levels was complex (16). No other studies have measured SVCT2 in tumor tissue.
SLC23A2 Polymorphisms and Cancer Risk
Sodium-dependent vitamin C transporter 2 is encoded by the SLC23A2 gene located on chromosome 20. A short isoform of SVCT2, naturally occurring in humans through alternative splicing, is unable to transport ascorbate (54). It has been shown to negatively regulate the function of the full length transporter by changing its affinity constant via hetero-oligomerization in human embryonic kidney 239T cells and mouse neuronal cells (54). This isoform also had the ability to partially inhibit SVCT1 (54, 55).
Several studies have focused on SLC23A2 gene polymorphisms related to ascorbate levels and disease risks. Two SNPs located in the intron region of SLC23A2 (rs6133175, rs1776948) were associated with risk of chronic lymphocytic leukemia (CLL) in a case–control study (n = 1,691) (56). However, no relationship between CLL risk and dietary ascorbate intake, as determined via questionnaires, was detected, and ascorbate was not measured (56). Another SNP (rs12479919) was inversely correlated with gastric cancer risk in a Polish study cohort (n = 693) (57). Similarly, in a European study (n = 365 cases, 1,284 controls), SLC23A2 SNPs (rs6053005, rs6133175) were predictive of plasma ascorbate levels, and haplotype variants were associated with risk of gastric cancer (43). In Japanese patients with esophageal squamous cell carcinoma (n = 49), two SNPs (rs268116, rs13037458) tended to associate with clinical response and long-term survival after 5-fluorouracil (5-FU)/cisplatin-based chemoradiotherapy, and two SNPs (rs4987219, rs1110277) correlated with chemotherapy-induced toxicity (58). Although several SLC23A family members have been reported to transport nucleobases, such as 5-FU, human SVCT1 and 2 reportedly do not possess this activity (59). Interestingly, SVCT2 mRNA levels also correlated with sensitivity to 5-FU in human esophageal cancer cell lines (60). Analysis of another polymorphism (rs4987219) revealed no association with cancer risk in squamous cell carcinoma of the head and neck in a Brazilian study (n = 165, 230 controls) (61). In neither study, ascorbate was measured.
Control of Gene Expression
Similar to Svct1, mRNA expression of Svct2 was upregulated by bile acids in rat hepatoma cells in vitro (46), while glutathione depleted cells had decreased Svct2 mRNA and protein levels (47). In human hepatoma cells, however, neither bile acids nor glutathione depletion had an effect on SVCT2 expression (46, 47). In human breast cancer cells, SVCT2 mRNA levels differed significantly between cell lines (62).
Subcellular Localization of SVCT2
The localization of SVCT2 within the cell may determine its transport activity, although data are conflicting. SVCT2 protein can be retained within intracellular compartments or transport vesicles and may have different kinetic properties depending on its localization (63), but data in cancer cells are limited. In neurons in culture, SVCT2 translocated to the plasma membrane upon increased extracellular ascorbate concentration (64). In a murine model of Huntington’s disease, impaired membrane translocation of SVCT2 led to insufficient accumulation of ascorbate in neurons (64).
Mitochondrial SVCT2 has been proposed to function as a low-affinity transporter due to differences in intracellular sodium and potassium concentrations (65). Lower sodium and higher potassium concentration inside embryonic kidney cells increased the transport Km from 20 µM to over 600 µM in mitochondria, and this was proposed to make transport more responsive to variations in intracellular ascorbate levels, and enabling transport into intracellular organelles (65). A transporter with a Km of 20 µM or less, as it is the case for SVCT2 in the plasma membrane, would function at maximal velocity at 200 µM ascorbate and not be able to respond to millimolar intracellular concentrations (65). In contrast, in U937 human myeloid leukemia cells, SVCT2 had similar kinetic characteristics at both locations (66). It was thus concluded that the transporter might function with different affinities in different cell types.
SVCT2 Activity
Data in mice showed increased radioactive ascorbate uptake in adrenal glands, compared to adrenocortical or adrenal medulla tumors, and adrenal uptake was sensitive to the ascorbate transport blocking agent sulfinpyrazone (67). In a mouse neuroblastoma cell line, SVCT2 was found to have two different transport kinetics (Km of 13 and 105 µM), and transport could be inhibited with flavonoids (68). Expression of SVCT2 in human neuroblastoma tissue was confirmed by immunofluorescence (68) but activity is unknown. SVCT2 protein levels in breast cancer cells were predictive of ascorbate uptake and cellular sensitivity to ascorbate cytotoxicity, and this was confirmed via overexpression and gene knockdown in vitro (69). Furthermore, in vivo tumor response to ascorbate administration (1 g/kg/day) correlated with increased SVCT2 protein levels in xenografts (69), although tumor levels of ascorbate were not assessed. Western blot (n = 20) and immunohistochemistry (n = 92) in breast cancer patients indicated an inverse relationship between SVCT2 protein levels in tumor tissue and hormone receptor status, with low SVCT2 levels in normal tissue (69), again without ascorbate measurements.
The monoclonal antibody cetuximab, used for the treatment of several types of metastatic cancer, inhibits the human epidermal growth factor receptor, and KRAS-mutant tumors are resistant to cetuximab (70). SVCT2 expression sensitized KRAS-mutant human colon cancer cells to combined administration of ascorbate and cetuximab in vitro (27). Combination treatment also reduced tumor growth in KRAS-mutant xenograft tumors dependent on SVCT2 levels; intracellular or tumor ascorbate levels have again not been measured (27). In a recent study, ascorbate was also shown to act synergistically with the multikinase inhibitor sorafenib through dysregulation of calcium homeostasis, in addition to H2O2 production, in a hepatocellular carcinoma cell line (71). Elucidating the role of vitamin C transporters in this setting might further clarify the mechanism of action of ascorbate in modulating the cytotoxicity of chemotherapeutics.
Concluding Remarks and Future Directions
Ascorbate is critical for many enzymatic reactions in the body and needs to be sufficiently taken up from the diet and distributed into different body compartments. Blood ascorbate levels in cancer patients are measured infrequently, and data are inconsistent in different studies. Importantly, tumor tissue levels in cancer patients have only been analyzed in two studies to date (17, 18). How ascorbate transport proteins are regulated at both the transcriptional and posttranslational level is still not fully understood. There are only three studies that report SVCT protein status in human cancer tissue and none of these report associated ascorbate levels (49, 68, 69). Further research is needed to evaluate if expression and localization of ascorbate transporters in tumors could predict ascorbate uptake, and thus serve as biomarkers for potential therapeutic effect in patients undergoing high dose ascorbate infusion.
Author Contributions
GD conceived the study; CW collected data from the literature and composed the review; and CW, EP, and GD edited, refined, and finalized the manuscript.
Conflict of Interest Statement
The authors declare that the research was conducted in the absence of any commercial or financial relationships that could be construed as a potential conflict of interest.
Funding
CW is supported via a PhD scholarship from the University of Otago; GD received funding from the Genesis Oncology Trust; EP and GD are supported by the Mackenzie Charitable Foundation.
Abbreviations
5-FU, 5-fluorouracil; CLL, chronic lymphocytic leukemia; SLC23, solute carrier gene family 23; SLC2, solute carrier gene family 2; SVCT, sodium-dependent vitamin C transporter; DHA, dehydroascorbate; GLUTs, glucose transporters; Gulo, l-gulonolactone oxidase; SNP, single nucleotide polymorphism.
References
1. Cortés-Jofré M, Rueda J-R, Corsini-Muñoz G, Fonseca-Cortés C, Caraballoso M, Bonfill Cosp X. Drugs for preventing lung cancer in healthy people. Cochrane Database Syst Rev (2012) 10:CD002141. doi: 10.1002/14651858.CD002141.pub2
2. Harris HR, Orsini N, Wolk A. Vitamin C and survival among women with breast cancer: a meta-analysis. Eur J Cancer (2014) 50:1223–31. doi:10.1016/j.ejca.2014.02.013
3. Wilson MK, Baguley BC, Wall C, Jameson MB, Findlay MP. Review of high-dose intravenous vitamin C as an anticancer agent. Asia Pac J Clin Oncol (2014) 10:22–37. doi:10.1111/ajco.12173
4. Anthony HM, Schorah CJ. Severe hypovitaminosis C in lung-cancer patients: the utilization of vitamin C in surgical repair and lymphocyte-related host resistance. Br J Cancer (1982) 46:354–67. doi:10.1038/bjc.1982.211
5. Mayland CR, Bennett MI, Allan K. Vitamin C deficiency in cancer patients. Palliat Med (2005) 19:17–20. doi:10.1191/0269216305pm970oa
6. Padayatty SJ, Levine M. New insights into the physiology and pharmacology of vitamin C. CMAJ (2001) 164:353–5.
7. Heaney ML, Gardner JR, Karasavvas N, Golde DW, Scheinberg DA, Smith EA, et al. Vitamin C antagonizes the cytotoxic effects of antineoplastic drugs. Cancer Res (2008) 68:8031–8. doi:10.1158/0008-5472.CAN-08-1490
8. Buettner GR, Jurkiewicz BA. Catalytic metals, ascorbate and free radicals: combinations to avoid. Radiat Res (1996) 145:532–41. doi:10.2307/3579271
9. Du J, Martin SM, Levine M, Wagner BA, Buettner GR, Wang S, et al. Mechanisms of ascorbate-induced cytotoxicity in pancreatic cancer. Clin Cancer Res (2010) 16:509–20. doi:10.1158/1078-0432.CCR-09-1713
10. Vissers MC, Kuiper C, Dachs GU. Regulation of the 2-oxoglutarate-dependent dioxygenases and implications for cancer. Biochem Soc Trans (2014) 42:945–51. doi:10.1042/BST20140118
11. Vissers MC, Gunningham SP, Morrison MJ, Dachs GU, Currie MJ. Modulation of hypoxia-inducible factor-1 alpha in cultured primary cells by intracellular ascorbate. Free Radic Biol Med (2007) 42:765–72. doi:10.1016/j.freeradbiomed.2006.11.023
12. Knowles HJ, Mole DR, Ratcliffe PJ, Harris AL. Normoxic stabilization of hypoxia-inducible factor-1alpha by modulation of the labile iron pool in differentiating U937 macrophages: effect of natural resistance-associated macrophage protein 1. Cancer Res (2006) 66:2600–7. doi:10.1158/0008-5472.CAN-05-2351
13. Kuiper C, Dachs GU, Currie MJ, Vissers MC. Intracellular ascorbate enhances hypoxia-inducible factor (HIF)-hydroxylase activity and preferentially suppresses the HIF-1 transcriptional response. Free Radic Biol Med (2014) 69:308–17. doi:10.1016/j.freeradbiomed.2014.01.033
14. Campbell EJ, Vissers MC, Bozonet S, Dyer A, Robinson BA, Dachs GU. Restoring physiological levels of ascorbate slows tumor growth and moderates HIF-1 pathway activity in Gulo(-/-) mice. Cancer Med (2015) 4:303–14. doi:10.1002/cam4.349
15. Campbell EJ, Vissers MC, Dachs G. Ascorbate availability affects tumor implantation-take rate and increases tumor rejection in Gulo−/− mice. Hypoxia (Auckl) (2016) 41:41–52. doi:10.2147/HP.S103088
16. Campbell EJ, Vissers MC, Wohlrab C, Hicks KO, Strother RM, Bozonet SM, et al. Pharmacokinetic and anti-cancer properties of high dose ascorbate in solid tumours of ascorbate-dependent mice. Free Radic Biol Med (2016) 99:451–62. doi:10.1016/j.freeradbiomed.2016.08.027
17. Kuiper C, Molenaar IG, Dachs GU, Currie MJ, Sykes PH, Vissers MC. Low ascorbate levels are associated with increased hypoxia-inducible factor-1 activity and an aggressive tumor phenotype in endometrial cancer. Cancer Res (2010) 70:5749–58. doi:10.1158/0008-5472.CAN-10-0263
18. Kuiper C, Dachs GU, Munn D, Currie MJ, Robinson BA, Pearson JF, et al. Increased tumor ascorbate is associated with extended disease-free survival and decreased hypoxia-inducible factor-1 activation in human colorectal cancer. Front Oncol (2014) 4:10. doi:10.3389/fonc.2014.00010
19. Kuiper C, Vissers MC, Hicks KO. Pharmacokinetic modeling of ascorbate diffusion through normal and tumor tissue. Free Radic Biol Med (2014) 77:340–52. doi:10.1016/j.freeradbiomed.2014.09.023
20. Welch RW, Wang Y, Crossman A, Park JB, Kirk KL, Levine M. Accumulation of vitamin C (ascorbate) and its oxidized metabolite dehydroascorbic acid occurs by separate mechanisms. J Biol Chem (1995) 270:12584–92. doi:10.1074/jbc.270.21.12584
21. Tsukaguchi H, Tokui T, Mackenzie B, Berger UV, Chen XZ, Wang Y, et al. A family of mammalian Na+-dependent l-ascorbic acid transporters. Nature (1999) 399:70–5. doi:10.1038/19986
22. Vera JC, Rivas CI, Fischbarg J, Golde DW. Mammalian facilitative hexose transporters mediate the transport of dehydroascorbic acid. Nature (1993) 364:79–82. doi:10.1038/364079a0
23. DiMattio J. A comparative study of ascorbic acid entry into aqueous and vitreous humors of the rat and guinea pig. Invest Ophthalmol Vis Sci (1989) 30:2320–31.
24. Wagner ES, White W, Jennings M, Bennett K. The entrapment of [14C]ascorbic acid in human erythrocytes. Biochim Biophys Acta (1987) 902:133–6. doi:10.1016/0005-2736(87)90143-X
25. Yun J, Mullarky E, Lu C, Bosch KN, Kavalier A, Rivera K, et al. Vitamin C selectively kills KRAS and BRAF mutant colorectal cancer cells by targeting GAPDH. Science (2015) 350:1391–6. doi:10.1126/science.aaa5004
26. McCarty MF. Expression and/or activity of the SVCT2 ascorbate transporter may be decreased in many aggressive cancers, suggesting potential utility for sodium bicarbonate and dehydroascorbic acid in cancer therapy. Med Hypotheses (2013) 81:664–70. doi:10.1016/j.mehy.2013.07.023
27. Jung S-A, Lee D-H, Moon J-H, Hong S-W, Shin J-S, Hwang IY, et al. l-Ascorbic acid can abrogate SVCT2-dependent cetuximab resistance mediated by mutant KRAS in human colon cancer cells. Free Radic Biol Med (2016) 95:200–8. doi:10.1016/j.freeradbiomed.2016.03.009
28. Dhariwal KR, Hartzell WO, Levine M. Ascorbic acid and dehydroascorbic acid measurements in human plasma and serum. Am J Clin Nutr (1991) 54:712–6.
29. Michels AJ, Hagen TM, Frei B. Human genetic variation influences vitamin C homeostasis by altering vitamin C transport and antioxidant enzyme function. Annu Rev Nutr (2013) 33:45–70. doi:10.1146/annurev-nutr-071812-161246
30. Corti A, Casini AF, Pompella A. Cellular pathways for transport and efflux of ascorbate and dehydroascorbate. Arch Biochem Biophys (2010) 500:107–15. doi:10.1016/j.abb.2010.05.014
31. Savini I, Catani MV, Arnone R, Rossi A, Frega G, Del Principe D, et al. Translational control of the ascorbic acid transporter SVCT2 in human platelets. Free Radic Biol Med (2007) 42:608–16. doi:10.1016/j.freeradbiomed.2006.11.028
32. Eck P, Kwon O, Chen S, Mian O, Levine M. The human sodium-dependent ascorbic acid transporters SLC23A1 and SLC23A2 do not mediate ascorbic acid release in the proximal renal epithelial cell. Physiol Rep (2013) 1:e00136. doi:10.1002/phy2.136
33. May JM, Qu Z-C. Ascorbic acid efflux from human brain microvascular pericytes: role of re-uptake. Biofactors (2015) 41:330–8. doi:10.1002/biof.1227
34. Eck P, Erichsen HC, Taylor JG, Yeager M, Hughes AL, Levine M, et al. Comparison of the genomic structure and variation in the two human sodium-dependent vitamin C transporters, SLC23A1 and SLC23A2. Hum Genet (2004) 115:285–94. doi:10.1007/s00439-004-1167-x
35. Bürzle M, Suzuki Y, Ackermann D, Miyazaki H, Maeda N, Clémençon B, et al. The sodium-dependent ascorbic acid transporter family SLC23. Mol Aspects Med (2013) 34:436–54. doi:10.1016/j.mam.2012.12.002
36. Godoy A, Ormazabal V, Moraga-Cid G, Zúñiga FA, Sotomayor P, Barra V, et al. Mechanistic insights and functional determinants of the transport cycle of the ascorbic acid transporter SVCT2. Activation by sodium and absolute dependence on bivalent cations. J Biol Chem (2007) 282:615–24. doi:10.1074/jbc.M608300200
37. Castro M, Caprile T, Astuya A, Millán C, Reinicke K, Vera JC, et al. High-affinity sodium–vitamin C co-transporters (SVCT) expression in embryonic mouse neurons. J Neurochem (2001) 78:815–23. doi:10.1046/j.1471-4159.2001.00461.x
38. Wang Y, Mackenzie B, Tsukaguchi H, Weremowicz S, Morton CC, Hediger MA. Human vitamin C (l-ascorbic acid) transporter SVCT1. Biochem Biophys Res Commun (2000) 267:488–94. doi:10.1006/bbrc.1999.1929
39. Lindblad M, Tveden-Nyborg P, Lykkesfeldt J. Regulation of vitamin C homeostasis during deficiency. Nutrients (2013) 5:2860–79. doi:10.3390/nu5082860
40. Wang H, Dutta B, Huang W, Devoe LD, Leibach FH, Ganapathy V, et al. Human Na+-dependent vitamin C transporter 1 (hSVCT1): primary structure, functional characteristics and evidence for a non-functional splice variant. Biochim Biophys Acta (1999) 1461:1–9. doi:10.1016/S0005-2736(99)00182-0
41. Savini I, Rossi A, Pierro C, Avigliano L, Catani MV. SVCT1 and SVCT2: key proteins for vitamin C uptake. Amino Acids (2008) 34:347–55. doi:10.1007/s00726-007-0555-7
42. Reidling JC, Rubin SA. Promoter analysis of the human ascorbic acid transporters SVCT1 and 2: mechanisms of adaptive regulation in liver epithelial cells. J Nutr Biochem (2011) 22:344–50. doi:10.1016/j.jnutbio.2010.03.001
43. Duell EJ, Lujan-Barroso L, Llivina C, Muñoz X, Jenab M, Boutron-Ruault M-C, et al. Vitamin C transporter gene (SLC23A1 and SLC23A2) polymorphisms, plasma vitamin C levels, and gastric cancer risk in the EPIC cohort. Genes Nutr (2013) 8:549–60. doi:10.1007/s12263-013-0346-6
44. Skibola CF, Bracci PM, Halperin E, Nieters A, Hubbard A, Paynter RA, et al. Polymorphisms in the estrogen receptor 1 and vitamin C and matrix metalloproteinase gene families are associated with susceptibility to lymphoma. PLoS One (2008) 3:e2816. doi:10.1371/journal.pone.0002816
45. Erichsen HC, Peters U, Eck P, Welch R, Schoen RE, Yeager M, et al. Genetic variation in sodium-dependent vitamin C transporters SLC23A1 and SLC23A2 and risk of advanced colorectal adenoma. Nutr Cancer (2008) 60:652–9. doi:10.1080/01635580802033110
46. Hierro C, Monte MJ, Lozano E, Gonzalez-Sanchez E, Marin JJG, Macias RIR. Liver metabolic/oxidative stress induces hepatic and extrahepatic changes in the expression of the vitamin C transporters SVCT1 and SVCT2. Eur J Nutr (2014) 53:401–12. doi:10.1007/s00394-013-0536-4
47. Mardones L, Zúñiga FA, Villagrán M, Sotomayor K, Mendoza P, Escobar D, et al. Essential role of intracellular glutathione in controlling ascorbic acid transporter expression and function in rat hepatocytes and hepatoma cells. Free Radic Biol Med (2012) 52:1874–87. doi:10.1016/j.freeradbiomed.2012.02.017
48. MacDonald L, Thumser AE, Sharp P. Decreased expression of the vitamin C transporter SVCT1 by ascorbic acid in a human intestinal epithelial cell line. Br J Nutr (2002) 87:97–100. doi:10.1079/BJN2001492
49. Aguilera O, Muñoz-Sagastibelza M, Torrejón B, Borrero-Palacios A, Del Puerto-Nevado L, Martínez-Useros J, et al. Vitamin C uncouples the Warburg metabolic switch in KRAS mutant colon cancer. Oncotarget (2016) 7(30):47954–65. doi:10.18632/oncotarget.10087
50. Kang JS, Kim HN, Jung DJ, Kim JE, Mun GH, Kim YS, et al. Regulation of UVB-induced IL-8 and MCP-1 production in skin keratinocytes by increasing vitamin C uptake via the redistribution of SVCT-1 from the cytosol to the membrane. J Invest Dermatol (2006) 127:698–706. doi:10.1038/sj.jid.5700572
51. Harrison FE, May JM. Vitamin C function in the brain: vital role of the ascorbate transporter SVCT2. Free Radic Biol Med (2009) 46:719–30. doi:10.1016/j.freeradbiomed.2008.12.018
52. Meredith ME, Harrison FE, May JM. Differential regulation of the ascorbic acid transporter SVCT2 during development and in response to ascorbic acid depletion. Biochem Biophys Res Commun (2011) 414:737–42. doi:10.1016/j.bbrc.2011.09.146
53. Larsson N, Rankin GD, Bicer EM, Roos-Engstrand E, Pourazar J, Blomberg A, et al. Identification of vitamin C transporters in the human airways: a cross-sectional in vivo study. BMJ Open (2015) 5:e006979. doi:10.1136/bmjopen-2014-006979
54. Lutsenko EA, Carcamo JM, Golde DW. A human sodium-dependent vitamin C transporter 2 isoform acts as a dominant-negative inhibitor of ascorbic acid transport. Mol Cell Biol (2004) 24:3150–6. doi:10.1128/MCB.24.14.6537.2004
55. Salazar K, Cerda G, Martínez F, Sarmiento JM, González C, Rodríguez F, et al. SVCT2 transporter expression is post-natally induced in cortical neurons and its function is regulated by its short isoform. J Neurochem (2014) 130:693–706. doi:10.1111/jnc.12793
56. Casabonne D, Gracia E, Espinosa A, Bustamante M, Benavente Y, Robles C, et al. Fruit and vegetable intake and vitamin C transporter gene (SLC23A2) polymorphisms in chronic lymphocytic leukaemia. Eur J Nutr (2017) 56(3):1123–33. doi:10.1007/s00394-016-1162-8
57. Wright ME, Andreotti G, Lissowska J, Yeager M, Zatonski W, Chanock SJ, et al. Genetic variation in sodium-dependent ascorbic acid transporters and risk of gastric cancer in Poland. Eur J Cancer (2009) 45:1824–30. doi:10.1016/j.ejca.2009.01.027
58. Minegaki T, Kuwahara A, Yamamori M, Nakamura T, Okuno T, Miki I, et al. Genetic polymorphisms in SLC23A2 as predictive biomarkers of severe acute toxicities after treatment with a definitive 5-fluorouracil/cisplatin-based chemoradiotherapy in Japanese patients with esophageal squamous cell carcinoma. Int J Med Sci (2014) 11:321–6. doi:10.7150/ijms.7654
59. Yamamoto S, Inoue K, Murata T, Kamigaso S, Yasujima T, Maeda J, et al. Identification and functional characterization of the first nucleobase transporter in mammals: implication in the species difference in the intestinal absorption mechanism of nucleobases and their analogs between higher primates and other mammals. J Biol Chem (2010) 285:6522–31. doi:10.1074/jbc.M109.032961
60. Minegaki T, Takara K, Hamaguchi R, Tsujimoto M, Nishiguchi K. Factors affecting the sensitivity of human-derived esophageal carcinoma cell lines to 5-fluorouracil and cisplatin. Oncol Lett (2013) 5:427–34. doi:10.3892/ol.2012.1014
61. Santiago MB, DE Lima Marson FA, Secolin R, Ribeiro JD, Lima CSP, Bertuzzo CS. SLC23A2-05 (rs4987219) and KRAS-LCS6 (rs61764370) polymorphisms in patients with squamous cell carcinoma of the head and neck. Oncol Lett (2014) 7:1803–11. doi:10.3892/ol.2014.2029
62. Khurana V, Kwatra D, Pal D, Mitra AK. Molecular expression and functional activity of vitamin C specific transport system (SVCT2) in human breast cancer cells. Int J Pharm (2014) 474:14–24. doi:10.1016/j.ijpharm.2014.07.056
63. Subramanian VS, Marchant JS, Said HM. Molecular determinants dictating cell surface expression of the human sodium-dependent vitamin C transporter-2 in human liver cells. Am J Physiol Gastrointest Liver Physiol (2010) 298:G267–74. doi:10.1152/ajpgi.00435.2009
64. Acuña AI, Esparza M, Kramm C, Beltrán FA, Parra AV, Cepeda C, et al. A failure in energy metabolism and antioxidant uptake precede symptoms of Huntington’s disease in mice. Nat Commun (2013) 4:2917. doi:10.1038/ncomms3917
65. Muñoz-Montesino C, Roa FJ, Peña E, González M, Sotomayor K, Inostroza E, et al. Mitochondrial ascorbic acid transport is mediated by a low-affinity form of the sodium-coupled ascorbic acid transporter-2. Free Radic Biol Med (2014) 70:241–54. doi:10.1016/j.freeradbiomed.2014.02.021
66. Fiorani M, Azzolini C, Cerioni L, Scotti M, Guidarelli A, Ciacci C, et al. The mitochondrial transporter of ascorbic acid functions with high affinity in the presence of low millimolar concentrations of sodium and in the absence of calcium and magnesium. Biochim Biophys Acta (2015) 1848:1393–401. doi:10.1016/j.bbamem.2015.03.009
67. Kim J, Yamamoto F, Gondo S, Yanase T, Mukai T, Maeda M. 6-deoxy-6-[131I]iodo-l-ascorbic acid for the in vivo study of ascorbate: autoradiography, biodistribution in normal and hypolipidemic rats, and in tumor-bearing nude mice. Biol Pharm Bull (2009) 32:1906–11. doi:10.1248/bpb.32.1906
68. Caprile T, Salazar K, Astuya A, Cisternas P, Silva-Alvarez C, Montecinos H, et al. The Na+-dependent l-ascorbic acid transporter SVCT2 expressed in brainstem cells, neurons, and neuroblastoma cells is inhibited by flavonoids. J Neurochem (2009) 108:563–77. doi:10.1111/j.1471-4159.2008.05788.x
69. Hong S-W, Lee S-H, Moon J-H, Hwang JJ, Kim DE, Ko E, et al. SVCT-2 in breast cancer acts as an indicator for l-ascorbate treatment. Oncogene (2013) 32:1508–17. doi:10.1038/onc.2012.176
70. Valtorta E, Misale S, Sartore-Bianchi A, Nagtegaal ID, Paraf F, Lauricella C, et al. KRAS gene amplification in colorectal cancer and impact on response to EGFR-targeted therapy. Int J Cancer (2013) 133:1259–65. doi:10.1002/ijc.28106
Keywords: ascorbate, sodium-dependent vitamin C transporter 1, sodium-dependent vitamin C transporter 2, tumor, expression
Citation: Wohlrab C, Phillips E and Dachs GU (2017) Vitamin C Transporters in Cancer: Current Understanding and Gaps in Knowledge. Front. Oncol. 7:74. doi: 10.3389/fonc.2017.00074
Received: 26 September 2016; Accepted: 06 April 2017;
Published: 24 April 2017
Edited by:
Giuseppe Giaccone, Georgetown University, USAReviewed by:
David Engelberg, Hebrew University of Jerusalem, IsraelVictor C. Kok, Asia University, Taiwan
Copyright: © 2017 Wohlrab, Phillips and Dachs. This is an open-access article distributed under the terms of the Creative Commons Attribution License (CC BY). The use, distribution or reproduction in other forums is permitted, provided the original author(s) or licensor are credited and that the original publication in this journal is cited, in accordance with accepted academic practice. No use, distribution or reproduction is permitted which does not comply with these terms.
*Correspondence: Gabi U. Dachs, Z2FiaS5kYWNoc0BvdGFnby5hYy5ueg==