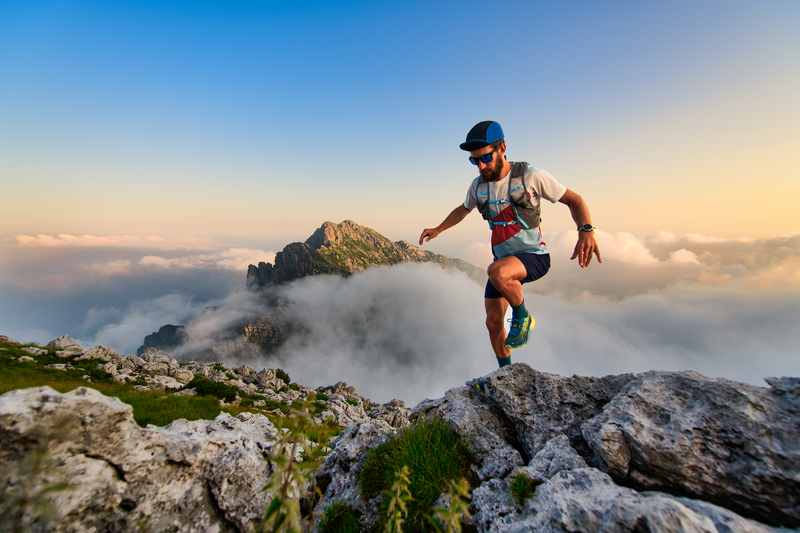
95% of researchers rate our articles as excellent or good
Learn more about the work of our research integrity team to safeguard the quality of each article we publish.
Find out more
PERSPECTIVE article
Front. Oncol. , 02 August 2016
Sec. Cancer Imaging and Image-directed Interventions
Volume 6 - 2016 | https://doi.org/10.3389/fonc.2016.00171
This article is part of the Research Topic Exploring cancer metabolic reprogramming through molecular imaging View all 23 articles
Elucidation of molecular mechanisms underlying the aberrant phosphatidylcholine cycle in cancer cells plays in favor of the use of metabolic imaging in oncology and opens the way for designing new targeted therapies. The anomalous choline metabolic profile detected in cancer by magnetic resonance spectroscopy and spectroscopic imaging provides molecular signatures of tumor progression and response to therapy. The increased level of intracellular phosphocholine (PCho) typically detected in cancer cells is mainly attributed to upregulation of choline kinase, responsible for choline phosphorylation in the biosynthetic Kennedy pathway, but can also be partly produced by activation of phosphatidylcholine-specific phospholipase C (PC-PLC). This hydrolytic enzyme, known for implications in bacterial infection and in plant survival to hostile environmental conditions, is reported to be activated in mitogen- and oncogene-induced phosphatidylcholine cycles in mammalian cells, with effects on cell signaling, cell cycle regulation, and cell proliferation. Recent investigations showed that PC-PLC activation could account for 20–50% of the intracellular PCho production in ovarian and breast cancer cells of different subtypes. Enzyme activation was associated with PC-PLC protein overexpression and subcellular redistribution in these cancer cells compared with non-tumoral counterparts. Moreover, PC-PLC coimmunoprecipitated with the human epidermal growth factor receptor-2 (HER2) and EGFR in HER2-overexpressing breast and ovarian cancer cells, while pharmacological PC-PLC inhibition resulted into long-lasting HER2 downregulation, retarded receptor re-expression on plasma membrane and antiproliferative effects. This body of evidence points to PC-PLC as a potential target for newly designed therapies, whose effects can be preclinically and clinically monitored by metabolic imaging methods.
Phosphatidylcholine-specific phospholipase C (EC 3.1.4.3, here abbreviated as PC-PLC) is responsible for hydrolysis of this glycerophospholipid into phosphocholine (PCho) and 1,2-diacylglycerols (DAG).
Phospholipases of this class are known to be important secreted pathogenicity factors in some bacteria, parasites, and fungi (1, 2) in which they act as lytic agents against eukaryotic cells and interfere with the host immune defense. Some bacterial PC-PLCs are also involved in lipid remodeling in response to phosphate-limiting conditions. Amino acid sequences and encoding genes have been identified for various toxic and non-toxic PC-PLCs produced by Gram-positive and Gram-negative bacteria. Since some PC-PLCs play important roles in the pathogenesis of diseases, they could also form components of vaccines.
Phospholipases C endowed with a broader substrate specificity (collectively called NPC, as an acronym for “non-specific phospholipases C”) were discovered, sequenced, and cloned in plants as a novel family of phospholipid-cleaving enzymes homologous to bacterial PC-PLCs and responsible for lipid conversion under phosphate-limiting conditions (3). Notably, phosphatidylcholine-hydrolyzing members of the NPC family in Arabidopsis were implicated in stress response to phytohormones, root development, and tolerance to adverse environmental conditions (3).
Phosphatidylcholine-specific phospholipase C activity is reported to be an essential source of phospholipid-derived signaling in animal cells (4, 5) in which this phospholipase can be implicated in various intracellular regulatory mechanisms, including long-term cell response to mitogens (6–9); cell cycle regulation and cell proliferation (8, 10, 11); programmed cell death (12, 13); activation of cells of the immune system (14–22); cell transformation (23, 24); oncogene-driven cell signaling and tumor progression (25–28); and cell differentiation of tumoral and non-tumoral cells (29–34).
Phosphatidylcholine-specific phospholipase C isoforms of varying molecular weights have been isolated from mammalian sources (35–37). However, differently from phosphatidylinositol-bis-phosphate specific PLCs (PIP2-PLCs), well-recognized key regulatory enzymes of cell growth, development, and stress responses in living organisms, a slower progress has been so far achieved in the molecular characterization of PC-PLCs in animal cells, in which these phospholipases have not yet been sequenced and cloned. For these reasons, the role of PC-PLCs in mammalian cells has remained elusive until recently. Despite these limitations, the PC-PLC protein expression could be effectively investigated in mammalian cells using cross-reacting polyclonal antibodies raised in rabbits against bacterial PC-PLCs, as first described by Clark et al. (37). Using these antibodies, a 66-kDa PC-PLC isoform has been detected in various mammalian cell systems, such as mouse NIH-3T3 fibroblasts (8, 38), synaptic endings (39, 40), epithelial ovarian cancer (EOC) cells and surgical specimens (26, 27), breast cancer (BC) (28) and hepatoma cells (11, 30, 41). Furthermore, near-infrared probes capable to non-invasively detect PC-PLC in experimental animals have been developed and their utility tested for in vivo cancer imaging (42).
An increasing interest in filling the existing gaps in the molecular and genomic characterization of mammalian PC-PLCs arises from accruing evidence that protein overexpression, subcellular redistribution, and activation of this enzyme in tumor cells represent relevant features of the aberrant choline phospholipid metabolism in cancer (43). In addition, evidence for a physical interaction of PC-PLC with the human epidermal growth factor receptor-2 (HER2) and EGFR is provided by coimmunoprecipitation tests on HER2-overexpressing BC (28) and EOC cells.1 Pharmacological PC-PLC inhibition is associated in these cells with long-lasting HER2 downmodulation and induction of antiproliferative effects, suggesting a role for PC-PLC activity in controlling HER2-driven tumorigenicity. Furthermore, inhibition of PC-PLC is associated with loss of mesenchymal traits in the highly metastatic triple-negative MDA-MB-231 cells and with decreased in vitro cell migration and invasion capabilities, suggesting a pivotal role for PC-PLC in BC cell differentiation (34).
This article provides a brief overview on metabolic and functional features of PC-PLC in BC and EOC cells and outlines some perspectives offered by further elucidation of the impact of this phospholipase on cancer cell biology and therapy targeting.
Phosphatidylcholine, the most abundant phospholipid of eukaryotic cells, plays the double role of basic structural component of cell membranes and precursor of agonist-induced signaling lipids (44) through a network of enzymatic reactions known as the phosphatidylcholine cycle (45) (scheme in Figure 1). The agonist-induced production and utilization within this cycle of signaling lipids, such as DAG, phosphatidate, lysophosphatidylcholine, and arachidonic acid, are associated with changes in the fluxes and steady-state levels of water-soluble phosphatidylcholine metabolites, such as PCho, glycerophosphocholine (GPCho), and free choline (Cho), main components of the so-called total choline (tCho) metabolic profile.
Figure 1. Detection of the 1H-MRS total choline (tCho) metabolic profile in epithelial ovarian cancer (EOC) cells and in MRS/MRSI clinical examinations. The phosphatidylcholine cycle and its links with tyrosine kinase receptors’ stimulation, post-receptor signaling pathways, and cancer cell biological features are sketched in the central scheme. (A–C) High resolution 9.4-T 1H-MRS tCho profile of aqueous extracts of (A) ovarian surface epithelial cells (OSE); (B) SKOV3 cell line and its in vivo-passaged cell variant SKOV3.ip, characterized by higher in vivo tumorigenicity and twofold higher HER2 protein expression level (in the insert, Western blot analysis of HER2, 185 kDa); (C) SKOV3.ip cells exposed for 24 h to the competitive PC-PLC inhibitor D609, compared with control untreated cells. (D) Absolute activity rates (nmol/106 cells × h, mean ± SD) of ChoK and PC-PLC measured in aqueous extracts of different EOC cell lines, compared with those of non-tumoral immortalized hTERT cells (number of independent experiments, n ≥ 3 for all cell lines). (E,F) Examples of in vivo detection of (E)1H-MRS tCho peak (3.2 ppm) in a single voxel and (F) tCho map obtained by MRSI in EOC patient examined at 1.5 T. For further details, see Ref. (25, 27, 49–51). The central scheme was adapted from Figure 1 of Ref. (43). Abbreviations: Enzymes: ChoK, choline kinase (EC 2.7.1.32); CT, cytidylyltransferase (EC 2.7.7.15); LPL, lysophospholipase (EC 3.1.1.5); PCT, phosphocholine transferase (EC 2.7.8.2); PD, glycerophosphocholine phosphodiesterase (EC 3.1.4.2); PLA1, phospholipase A1 (EC 3.1.1.32); PLA2, phospholipase A2 (EC 3.1.1.4); PLC, phosphatidylcholine-specific phospholipase C (EC 3.1.4.3); PLD, phospholipase D (EC 3.1.4.4). Metabolites: AA, arachidonic acid; CDP-Cho, cytidine diphosphate-choline; Cho, free choline; DAG, diacylglycerol; GroP, sn-glycerol-3-phosphate; GPCho, glycerophosphocholine; Ino, myo-inositol; LPA, lysophosphatidate; LPtdCho, lysophosphatidylcholine; PA, phosphatidate; PCho, phosphocholine; PtdCho, phosphatidylcholine; tCho, total choline-containing metabolites (GPCho + PCho + Cho). Transporters: CHT, choline high-affinity transporters; CTL, choline transporter-like proteins; OCT, organic cation transporters.
Magnetic resonance spectroscopy (MRS) and spectroscopic imaging (MRSI) represent powerful means to detect changes induced in the tCho pool by oncogene-driven activation of phosphatidylcholine cycle enzymes in cancer and to evaluate the response of the tCho profile to agents targeted against selected enzymes (43, 46–48). Figure 1 shows examples of (i) the 1H-MRS tCho profile in aqueous extracts of normal ovarian surface epithelial cells (OSE, Figure 1A) compared with the EOC cell line SKOV3 and its in vivo-passaged cell variant SKOV3.ip, characterized by higher HER2 overexpression (Figure 1B) and enhanced in vivo tumorigenicity (25, 27, 49, 50); (ii) the effects induced on the SKOV3.ip tCho profile by cell exposure to D609, a competitive PC-PLC inhibitor (Figure 1C); (iii) the simultaneous activation of ChoK and PC-PLC in different EOC cell lines versus hTERT, a non-tumoral immortalized ovarian cell line (Figure 1D); and (iv) examples of in vivo detection of the 1H-MRS tCho peak (3.2 ppm) in a selected voxel (Figure 1E) and a tCho map obtained by 3D-MRSI in a EOC patient (Figure 1F) (51).
The 1H-MRS PCho signal increased 3.6- to 6.5-fold in the investigated EOC cell lines compared with non-tumoral counterparts (25, 27, 50). Notably, the 2-fold higher HER2 expression of SKOV3.ip versus SKOV3 cells was associated with a 1.7-fold higher PCho content (50). An elevated PCho level was the principal cause of the higher tCho pool detected in these cancer cells, in agreement with the general view that an increased tCho represents a metabolic signature of malignancy (43, 46–48, 52, 53). The enhanced PCho production in cancer cells is currently attributed to the upregulation of choline kinase (43, 46), the enzyme committed to choline phosphorylation in the biosynthetic Kennedy pathway. This enzyme (notably the ChoK-alpha isoform) has been proposed as a new target for cancer therapy (54, 55). A substantial contribution to the production of the intracellular PCho pool in cancer cells may, however, also derive from PC-PLC activation (see scheme in Figure 1). The question therefore arises on the relative contributions possibly given by PC-PLC activation to PCho production in different EOC cells. Measurements in our laboratory (Figure 1D) showed that a mean 12- to 24-fold activation of ChoK was paralleled by a 10- to 30-fold activation of PC-PLC in four EOC cell lines (IGROV1, OVCAR3, SKOV3, and SKOV3.ip) versus the non-tumoral hTERT cell line (in which both enzymes had a basal activity of about 0.5 nmol/106 cells × h). In some EOC cell lines (IGROV1, SKOV3.ip), ChoK and PC-PLC showed very similar activity rates, suggesting about equal contributions of the two enzymes to the PCho production. Accordingly, there was a 40–50% decrease of the PCho signal in SKOV3.ip cells following 24-h exposure to the competitive PC-PLC inhibitor D609 (example in Figure 1C). In another cell line (OVCAR3), the mean activity rate of ChoK was instead fourfold higher than that of PC-PLC. Overall, these data showed that the contribution of PC-PLC to the PCho production could vary, according to the cell line, between 20% and 50%, in substantial agreement with the effects of D609 or si-RNA ChoK silencing on the PCho levels measured in different EOC cells [Figure 1C; (27, 56)].
This body of evidence supports the conclusion that PC-PLC can contribute to a substantial extent to the accumulation of PCho in EOC cells. This evidence may allow a better interpretation of changes occurring in the in vivo MRS- and MRSI-detected tCho peak in preclinical EOC models (57) and in EOC clinical lesions (examples in Figures 1E,F) during tumor progression, response to treatment, or relapse.
Regarding human BC cells, a significant 2- to 5.5-fold ChoK activation was detected by Eliyahu and colleagues in cell lines of different subtypes (58), such as luminal-A MCF-7, HER2-positive SKBr3, and basal-like EGFR-positive MDA-MB-231 (respective activity rates 21 ± 3, 43 ± 6, and 17 ± 2 nmol/mg protein × h), versus mammary epithelial cells (8.0 ± 2.0 nmol/mg protein × h). Enzymatic assays in our laboratory (34) showed threefold to sixfold higher PC-PLC activity rates (ranging between 12 ± 2 and 22 ± 4 nmol/mg protein × h) in these BC cells versus the fibrocystic MCF-10 cell line (Figure 2A). Overall, these data suggest that the contribution of PC-PLC to the intracellular PCho production also varied in these BC cells between 20% and 50%, as reported above for EOC cell lines.
Figure 2. Subcellular localization of PC-PLC in human BC cell lines. (A) PC-PLC activity rates (nmol/mg protein × h, mean ± SD, n ≥ 3) measured in MCF-7, SKBr3, and MDA-MB-231 BC cell lines, possessing different levels of HER2 and EGFR expression, compared with that of the human mammary epithelial cell line of fibrocystic origin MCF-10. (B) Confocal laser scanning microscopy (CLSM) examinations (3D reconstruction images) of the same cell lines as in (A). Cells were either fixed and permeabilized (upper panels, scale bars 20 μm) or maintained unfixed (bottom panels, scale bars 5 μm) and stained for PC-PLC detection with rabbit anti-PC-PLC antibodies (pseudo-color gray); nuclei were stained with DAPI (blue). (C) Colocalization of PC-PLC and HER2 on plasma membrane of SKBr3 cells. CLSM detection of PC-PLC and HER2 was performed on fixed and permeabilized (upper panels) or unfixed cells (bottom panels, 3D reconstruction images) using rabbit polyclonal anti-PC-PLC antibodies (green) and anti-HER2 W6/100 monoclonal antibody (red). Colocalization areas are represented in yellow. Scale bars, 8 μm. (D) HER2 downmodulation in SKBr3 cells exposed for different time intervals to the competitive PC-PLC inhibitor D609. CLSM analyses of unfixed SKBr3 cells cultured in complete medium in absence (t = 0) or presence of D609 (6 and 24 h). After washing, cells were stained with anti-PC-PLC (green) and anti-HER2 antibodies (red). For further details, see Ref. (28, 34).
Western blot analyses showed that the reported threefold to sixfold activation of PC-PLC in BC cell lines was associated with a threefold to sixfold elevated PC-PLC protein expression (34), the highest value being detected in the triple-negative MDA-MB-231 cells. Confocal laser scanning microscopy (CLSM) of fixed and permeabilized cancer cells (Figure 2B, top panels) showed that the enzyme was localized both in nuclear and cytoplasmic compartments. Only a few PC-PLC-positive granules, mainly localized in perinuclear areas and absent in the nuclear matrix, were instead detected in the non-tumoral MCF-10A cells. CLSM of unfixed cells showed that PC-PLC massively accumulated on plasma membrane of the HER2-positive SKBr3 cells, but not on that of cells with basal HER2 expression (Figure 2B, bottom panels). PC-PLC extensively colocalized with HER2 in raft domains of plasma membrane of SKBr3 cells [Figure 2C; (28)], in which coimmunoprecipitation tests showed a physical association of PC-PLC with HER2 and with other members of the ErbB family, such as EGFR and HER3.
Regarding PC-PLC distribution in EOC cells, a massive accumulation of this phospholipase was detected by CLSM on plasma membrane of all investigated cell lines and on that of cancer cells isolated from patient peritoneal exudate, but not on OSE cells (26). The enzyme extensively colocalized with β1-integrin in non-raft domains of EOC cells (26). The extent of colocalization of the two proteins substantially decreased on membrane of serum-deprived cancer cells, but returned to the original level upon cell restimulation by platelet-derived growth factor. These data warrant further investigations on the functional role of the interaction between PC-PLC and β1-integrin, an adhesion protein well-known for its involvement in metastatic spread (59). Furthermore, CLSM and coimmunoprecipitation experiments showed an extensive colocalization and physical association of PC-PLC with HER2 on plasma membrane of SKOV3 and SKOV3.ip cells, confirming the interest of further investigating the role of this phospholipase in regulating HER2 overexpression in ovarian cancer.
Exposure of BC cell lines to D609 led to cell proliferation arrest, changes in cell morphology, and formation of lipid bodies typical of BC cell differentiation (34). Moreover, in the poorly differentiated MDA-MB-231 cells, PC-PLC inhibition was associated with progressive decreases of mesenchymal traits, such as vimentin and N-cadherin expression, reduced galectin-3 and milk fat globule EGF-factor 8 levels, β-casein formation, and decrease of in vitro cell migration and invasion (34). We therefore proposed that the inhibition of this phospholipase can be envisaged as a means to promote differentiation of metastatic BC cells, with potential therapeutic effects.
Besides inducing cell differentiation, exposure of SKBr3 cells to D609 resulted in a progressive downmodulation of both HER2 and PC-PLC on plasma membrane, an effect already evident at 6 h and almost complete at 24 h (Figure 2D). This effect was associated with HER2 internalization and lysosomal degradation, long-lasting retardation of re-expression of this receptor on membrane, and an about 50% decrease in the overall protein expression (28). Notably, no substantial influence on HER2 externalization is known to be exerted in these cells using Trastuzumab (60), the anti-HER2 humanized monoclonal antibody mostly used at the clinical level, whose in vivo therapeutic efficacy is mainly attributed to antibody-mediated cytotoxicity. This body of evidence suggests that PC-PLC inhibition may be envisaged as a potential alternative approach to counteract the tumorigenic effects of HER2, especially in cases of resistance (or contraindication) to current anti-HER2 treatment.
Long-standing decreases of HER2 and phospho-HER2 contents, reduced HER2 mRNA expression, and cell cycle arrest were also detected in the highly tumorigenic SKOV3.ip EOC cells exposed to a non-apoptotic dose of D6091. Furthermore, reduced tumor growth and decrease in HER2 and Ki67 immunostaining were detected in SKOV3.ip xenografts upon in vivo treatment with D609, pointing to the interest of further evaluating the potential role of PC-PLC as a therapy target in preclinical HER2-overexpressing EOC models.
In summary, PC-PLC is overexpressed and activated in BC and EOC cells, and pharmacological PC-PLC inhibition can lead to downregulation of HER2 and induction of antiproliferative effects. Furthermore, cell differentiation and decreases in cell migration and invasion were induced in the highly metastatic MDA-MB-231 BC cell line exposed to the PC-PLC inhibitor. These findings point to PC-PLC as a potential target (or cotarget) for anticancer therapy, especially in cases of resistance or contraindications to currently adopted treatments. In this context, the following issues appear worth of further elucidation:
The 1.7-fold increase in PC-PLC activity in a SKOV3 cell variant (SKOV3.ip) endowed with a 2-fold higher HER2 overexpression (50) and the over 3-fold increase in PC-PLC activity in the HER2-positive SKBr3 cells (34) warrant further investigations on the molecular mechanisms linking PC-PLC activation with HER2 overexpression and oncogenic effects of HER2 and HER2–EGFR heterodimers. Interestingly, the PC-PLC inhibitor D609, but not Trastuzumab, induced decreases of HER2 expression and cell proliferation in the Trastuzumab-resistant SKBr3 cell line. Conversely D609, either alone or in combination with Trastuzumab, induced in the Trastuzumab-sensitive BT-474 cells a decrease in cell proliferation comparable to that induced using Trastuzumab alone (28).
The sixfold activation of PC-PLC in MDA-MB-231 BC cells and its strong nuclear localization (Figures 2A,B) warrant investigations on the relationships between PC-PLC and EGFR overexpression in triple-negative BC cells and their metastatic derivatives.
Overexpression of EGFR and c-Src in BC cells has been reported to synergistically increase ChoK-alpha protein expression and activity levels (61). Further elucidation of EGFR-driven mechanisms responsible for the activation of both ChoK-alpha or PC-PLC in BC cells may lead to novel multi-targeted anticancer therapies, whose effects could be preclinically and clinically monitored by MRS-based and optical metabolic imaging methods.
Integration of MRS with 11C or 18F choline-based positron emission tomography (PET) may allow discrimination of the contributions to PCho production, provided by the biosynthetic and catabolic pathways, respectively. In fact, while both ChoK and PC-PLC can contribute to the MRS-detected PCho signal, the choline-PET standardized uptake value (SUV) mainly reflects choline transport and phosphorylation in the Kennedy pathway, with negligible contributions from products of radiolabeled phosphatidylcholine catabolism in a time window of about 1 h (the duration of a typical choline-PET examination) (43).
The interaction of an activated PC-PLC isoform with HER2, detected by coimmunoprecipitation tests, appears essential to the functional localization of this receptor in BC cells, since PC-PLC inhibition induces a long-lasting HER2 downmodulation (28). The association of these effects with reduced cell proliferation, induction of cell differentiation, and decrease in cell migration and invasion suggests that an activated PC-PLC may act as a sort of chaperone for HER2. A local overproduction of DAG by enhanced PC-PLC-mediated phosphatidylcholine hydrolysis could mediate this action. A local accumulation of DAG can, in fact, perturb the phospholipid bilayer, alter protein-lipid interactions, and influence the formation of microdomains, thus modifying the exposure of surface membrane receptors and affecting their recycling between membrane and inner cell compartments (62, 63). Interestingly, ChoK-alpha has also been proposed to act as a chaperone for the androgen receptor, a ligand-inducible transcription factor of the nuclear hormone receptor superfamily, critically involved in prostate cancer progression (64). The newly suggested role of ChoK and PC-PLC as regulators of expression for oncogenes and growth factor receptors may lead to new targeted or multi-targeted anticancer therapies.
This body of evidence points to the need for overcoming the current limitations deriving from (a) the scarce attention so far devoted to the genomic and structural characterization of mammalian PC-PLC isoforms and (b) the availability until now of only one PC-PLC inhibitor, D609, a synthetic tricyclic compound having a xanthate group, possessing antiviral, anti-tumor, anti-inflammatory, and anti-apoptosis properties and used as a lipid-related enzyme inhibitor for the past three decades (65).
Steps forward in translational oncology may be expected from:
(a) investigating the role of PC-PLC expression and the effects of PC-PLC inhibition in BC and EOC stem cells, to identify potential ways to reinforce therapeutic strategies aimed to eradicate malignancies (66, 67);
(b) exploring in preclinical models the correlation between PC-PLC activity and disease progression or response to therapy, as a ground for evaluating the impact of PC-PLC activation on clinical outcome.
Recent evidence shows protein overexpression and enzymatic activation of PC-PLC in BC and EOC cells compared with non-tumoral counterparts.
The activation of PC-PLC can contribute to 20–50% of the production of intracellular PCho in BC and EOC cells.
Phosphatidylcholine-specific phospholipase C activation is associated in these cancer cells with its protein overexpression and subcellular redistribution, the underlying molecular mechanisms deserving further elucidation.
Phosphatidylcholine-specific phospholipase C interacts with HER2 and EGFR in HER2-overexpressing BC and EOC cells, while its pharmacologic inhibition may play a pivotal role in HER2 downmodulation, reduction of cell proliferation, and cancer cell differentiation.
Overall, this body of evidence supports the interest of evaluating the possible role of this phospholipase as a key metabolic target for anticancer therapy.
The study on patients mentioned in this Perspective article (Figures 1E,F) was approved by the Institutional Review Board of the Research Hospital Policlinico San Donato Milanese, Italy. Written informed consent was obtained from all patients. Between July 2007 and April 2009, the study prospectively enrolled a series of patients with already known ovarian masses, who were consecutively referred to the Radiology Unit of Policlinico San Donato Milanese for presurgical staging. Exclusion criteria were the lack of informed consent and general contraindications to MRI or to the intravenous administration of contrast material. All patients underwent surgery with removal of the mass and histopathological examination. Further details are given in Ref. (51).
The manuscript was written by FP; revised by EI, SC, FSp, RC, FSa, and FP; read and approved by all coauthors.
The authors declare that the research was conducted in the absence of any commercial or financial relationships that could be construed as a potential conflict of interest.
The group at the Istituto Superiore di Sanità (ISS, Rome) acknowledges partial support by the Associazione Italiana per la Ricerca sul Cancro (AIRC) Research Program 2007-2009, IG-4251 (PI: FP) and Ministero della Salute, Integrated Oncology Program RO 065/ISS No. Q09 and Special Research Program Onc. Ord, 37/07/ISS No. 70CF/4 (PI:FP).
2. Ghannoum MA. Potential role of phospholipases in virulence and fungal pathogenesis. Clin Microbiol Rev (2000) 13:122–43. doi:10.1128/CMR.13.1.122-143.2000
3. Pokotylo I, Pejchar P, Potocky M, Kokourková D, Krcková Z, Ruelland E, et al. The plant non-specific phospholipase C gene family. Novel competitors in lipid signaling. Prog Lipid Res (2013) 52:62–79. doi:10.1016/j.plipres.2012.09.001
4. Billah MM, Antes JC. The regulation and cellular functions of phosphatidylcholine hydrolysis (review). Biochem J (1990) 269:281–91. doi:10.1042/bj2690281
5. Exton JH. Phosphatidylcholine breakdown and signal transduction. Biochim Biophys Acta (1994) 1212:26–42. doi:10.1016/0005-2760(94)90186-4
6. Larrodera P, Cornet ME, Diaz-Meco MT, Lopez-Barahona M, Diaz-Laviada I, Guddal PH, et al. Phospholipase C-mediated hydrolysis of phosphatidylcholine is an important step in PDGF-stimulated DNA synthesis. Cell (1990) 61:1113–20. doi:10.1016/0092-8674(90)90074-O
7. Van Dijck MCM, Muriana FJG, De Widt J, Hilkmann H, Van Blitterswijk WJ. Involvement of phosphatidylcholine-specific phospholipase C in platelet-derived growth factor-induced activation of the mitogen-activated protein kinase pathway in rat-1 fibroblasts. J Biol Chem (1997) 272:11011–6. doi:10.1074/jbc.272.17.11011
8. Ramoni C, Spadaro F, Barletta B, Dupuis ML, Podo F. Phosphatidylcholine-specific phospholipase C in mitogen-stimulated fibroblasts. Exp Cell Res (2004) 299:370–82. doi:10.1016/j.yexcr.2004.05.037
9. Li F, Wu N, Su R-B, Zheng JQ, Xu B, Lu XQ, et al. Involvement of phosphatidylcholine-selective phospholipases C in activation of mitogen-activated protein-kinase pathways in imidazoline receptor antisera selected protein. J Cell Biochem (2006) 98:1615–28. doi:10.1002/jcb.20806
10. Plo I, Lautier D, Levade T, Sekouri H, Jaffrézou JP, Laurent G, et al. Phosphatidylcholine-specific phospholipase C and phospholipase D are respectively implicated in mitogen-activated protein kinase and nuclear factor kB activation in tumor-necrosis-factor-α-treated immature acute-myeloid-leukaemia cells. Biochem J (2000) 351:459–67. doi:10.1042/0264-6021:3510459
11. Fu D, Ma Y, Wu W, Zhu X, Jia C, Zao Q, et al. Cell-cycle dependent PC-PLC regulation by APC/CCdc20-mediated ubiquitin-proteasome pathway. J Cell Biochem (2009) 107:686–96. doi:10.1002/jcb.22163
12. Cifone MG, Rocaioli P, De Maria R, Camarda G, Santoni A, Ruberti G, et al. Multiple pathways originated at Fas/Apo-1 (CD95) receptor: sequential involvement of phosphatidylcholine-specific phospholipase C and acidic sphingomyelinase in the propagation of the apoptotic signal. EMBO J (1995) 14:5859–68.
13. Liu X, Zhao Q, Araki S, Zhang S, Miao J. Contrasting effects of phosphatidylinositol- and phosphatidylcholine-specific phospholipase C on apoptosis in cultured endothelial cells. Endothelium (2006) 13:205–11. doi:10.1080/10623320600760423
14. Ramoni C, Spadaro F, Menegon M, Podo F. Cellular localization and functional role of phosphatidylcholine-specific phospholipase C in NK cells. J Immunol (2001) 167:2642–50. doi:10.4049/jimmunol.167.5.2642
15. Andrei C, Margiocco P, Poggi A, Lotti LV, Torrisi MR, Rubartelli A. Phospholipases C and A2 control lysosome-mediated IL-β secretion: implications for inflammatory processes. Proc Natl Acad Sci U S A (2004) 101:9745–50. doi:10.1073/pnas.0308558101
16. Moreno-Garcia ME, Lopez-Bojorguez LN, Zentella A, Humphries LA, Rawlings DJ, Santos-Argumedo L. CD38 signaling regulates B lymphocyte activation via a phospholipase C (PLC)-gamma 2-independent, protein kinase C, phosphatidylcholine-PLC, and phospholipase D-dependent signaling cascade. J Immunol (2005) 174:2687–95. doi:10.4049/jimmunol.174.5.2687
17. von Knethen A, Brune B. PKCα depletion in RAW264.7 macrophages following microbial/IFNγ stimulation is PC-PLC-mediated. Antioxid Redox Signal (2005) 7:1217–22. doi:10.1089/ars.2005.7.1217
18. Luft T, Rodionova E, Maraskovsky E, Kirsch M, Hess M, Buchholtz C, et al. Adaptive functional differentiation of dendritic cells: integrating the network of extra- and intracellular signals. Blood (2006) 107:4763–9. doi:10.1182/blood-2005-04-1501
19. Cuschieri J, Billgren J, Maier RV. Phosphatidylcholine-specific phospholipase C (PC-PLC) is required for LPS-mediated macrophage activation through CD14. J Leukoc Biol (2006) 80:407–14. doi:10.1189/jlb.1105622
20. Spadaro F, Cecchetti S, Sanchez M, Podo F, Ramoni C. Expression and role of phosphatidylcholine-specific phospholipase C in human NK and T-lymphocyte subsets. Eur J Immunol (2006) 36:3277–87. doi:10.1002/eji.200635927
21. Cecchetti S, Spadaro F, Lugini L, Podo F, Ramoni C. Functional role of phosphatidylcholine-specific phospholipase C in regulating CD16 membrane expression in natural killer cells. Eur J Immunol (2007) 37:2912–22. doi:10.1002/eji.200737266
22. Fantuzzi L, Spadaro F, Purificato C, Cecchetti S, Podo F, Belardelli F, et al. Phosphatidylcholine-specific phospholipase C activation is required for CCR5-dependent, NF-kB-driven CCL2 secretion elicited in response to HIV-1 gp120 in human primary macrophages. Blood (2008) 111:3355–63. doi:10.1182/blood-2007-08-104901
23. Johansen T, Biørkøy G, Øvervatn A, Diaz-Meco MT, Traavik T, Moscat J. NIH-3T3 cells stably transfected with the gene encoding phosphatidylcholine-hydrolyzing phospholipase C from Bacillus cereus acquire a transformed phenotype. Mol Cell Biol (1994) 14:646–54. doi:10.1128/MCB.14.1.646
24. Halstead J, Kemp K, Ignotz RA. Evidence for involvement of phosphatidylcholine-phospholipase C and protein kinase C in transforming growth factor-β signaling. J Biol Chem (1995) 270:13600–3. doi:10.1074/jbc.270.23.13600
25. Iorio E, Mezzanzanica D, Alberti P, Spadaro F, Ramoni C, D’Ascenzo S, et al. Alterations of choline phospholipid metabolism in ovarian tumor progression. Cancer Res (2005) 65:9369–76. doi:10.1158/0008-5472.CAN-05-1146
26. Spadaro F, Ramoni C, Mezzanzanica D, Miotti S, Alberti P, Cecchetti S, et al. Phosphatidylcholine-specific phospholipase C activation in epithelial ovarian cancer cells. Cancer Res (2008) 68:6541–9. doi:10.1158/0008-5472.CAN-07-6763
27. Iorio E, Ricci A, Bagnoli M, Pisanu ME, Castellano G, Di Vito M, et al. Activation of phosphatidylcholine cycle enzymes in human epithelial ovarian cancer cells. Cancer Res (2010) 70:2126–35. doi:10.1158/0008-5472.CAN-09-3833
28. Paris L, Cecchetti S, Spadaro F, Abalsamo L, Lugini L, Pisanu ME, et al. Inhibition of phosphatidylcholine-specific phospholipase C downregulates HER2 overexpression on plasma membrane of breast cancer cells. Breast Cancer Res (2010) 12:R27. doi:10.1186/bcr2575
29. Ferretti A, Podo F, Carpinelli GC, Chen LL, Borghi P, Masella R. Detection of neutral active phosphatidylcholine-specific phospholipase C in Friend leukemia cells before and after erythroid differentiation. Anticancer Res (1993) 13:2309–18.
30. Wu XZ, Lu H, Zhou L, Huang Y, Chen H. Changes of phosphatidylcholine-specific phospholipase C in hepatocarcinogenesis and in the proliferation and differentiation of rat liver cancer cells. Cell Biol Int (1997) 21:375–81. doi:10.1006/cbir.1997.0148
31. Kahle PJ, Shooter EM, Johnson RM, Verity AN. Phosphatidylcholine-specific phospholipase inhibitor D609 differentially affects MAP kinases and immediate-early genes in PC12 cells. Cell Signal (1998) 10:321–30. doi:10.1016/S0898-6568(98)00010-2
32. Zhao J, Zhao B, Wang W, Huang B, Zhang S, Miao J. Phosphatidylcholine-specific phospholipases C and ROS were involved in chicken blastodisc differentiation to vascular endothelial cell. J Cell Biochem (2007) 102:421–8. doi:10.1002/jcb.21301
33. Wang N, Sun C, Huo S, Zhang Y, Zhao J, Zhang S, et al. Cooperation of phosphatidylcholine-specific phospholipase C and basic fibroblast growth factor in the neural differentiation of mesenchymal stem cells in vitro. Int J Biochem Cell Biol (2008) 40:294–306. doi:10.1016/j.biocel.2007.08.003
34. Abalsamo L, Spadaro F, Bozzuto G, Paris L, Cecchetti S, Lugini L, et al. Inhibition of phosphatidylcholine-specific phospholipase C results in loss of mesenchymal traits in metastatic breast cancer cells. Breast Cancer Res (2012) 14:R50. doi:10.1186/bcr3151
35. Wolf RA, Gross RW. Identification of neutral active phospholipase C which hydrolyses choline glycerolipids and plasmalogen selective phospholipase A2 in canine myocardium. J Biol Chem (1985) 260:7295–303.
36. Sheikhnejad RG, Srivastava PN. Isolation and properties of a phosphatidylcholine-specific phospholipase C from bull seminal plasma. J Biol Chem (1986) 261:7544–9.
37. Clark MA, Shorr RGL, Bomalaski JS. Antibodies prepared to Bacillus cereus phospholipase C crossreact with a phosphatidylcholine-preferring phospholipase C in mammalian cells. Biochim Biophys Res Commun (1986) 140:114–9. doi:10.1016/0006-291X(86)91065-X
38. Podo F, Ferretti A, Knijn A, Zhang P, Ramoni C, Barletta B, et al. Detection of phosphatidylcholine-specific phospholipase C in NIH-3T3 fibroblasts and their H-ras transformants: NMR and immunochemical studies. Anticancer Res (1996) 16:1399–412.
39. Mateos VM, Uranga RM, Salvador GA, Giusto NM. Activation of phosphatidylcholine signalling during oxidative stress in synaptic endings. Neurochem Int (2008) 53:199–206. doi:10.1016/j.neuint.2008.07.005
40. Mateos VM, Salvador GA, Giusto NM. Selective localization of phosphatidylcholine-derived signaling in detergent-resistant membranes from synaptic endings. Biochim Biophys Acta (2010) 1798:624–36. doi:10.1016/j.bbamem.2009.12.008
41. Wu XZ, Lu H. Correlation of calcium-independent phosphatidylcholine-specific phospholipase C and gamma-GT in hepatoma cells. Acta Biochim Biophys Sin (1998) 30:104–6.
42. Mawn TM, Popov AV, Beardsley NJ, Stefflova K, Milkewitch M, Zheng G, et al. In vivo detection of phospholipase C by enzyme-activated near-infrared probes. Bioconjug Chem (2011) 22:2434–43. doi:10.1021/bc200242v
43. Podo F, Canevari S, Canese R, Pisanu ME, Ricci A, Iorio E. MR evaluation of response to targeted treatment in cancer cells. NMR Biomed (2011) 24:648–72. doi:10.1002/nbm.1658
44. Li Z, Vance DE. Phosphatidylcholine and choline homeostasis. J Lipid Res (2008) 49:1187–94. doi:10.1194/jlr.R700019-JLR200
45. Pelech SL, Vance DE. Signal transduction via phosphatidylcholine cycles. Trends Biochem Sci (1989) 14:28–30. doi:10.1016/0968-0004(89)90086-8
46. Glunde K, Bhujwalla ZM, Ronen SM. Choline metabolism in malignant transformation. Nat Rev Cancer (2011) 11:835–48. doi:10.1038/nrc3162
47. Podo F, Sardanelli F, Iorio E, Canese R, Carpinelli G, Fausto A, et al. Abnormal choline phospholipid metabolism in breast and ovary cancer: molecular bases for noninvasive imaging approaches. Curr Med Imaging Rev (2007) 3:123–37. doi:10.2174/157340507780619160
48. Podo F. Tumour phospholipid metabolism. NMR Biomed (1999) 12:413–39. doi:10.1002/(SICI)1099-1492(199911)12:7<413::AID-NBM587>3.0.CO;2-U
49. Canese R, Pisanu ME, Mezzanzanica D, Ricci A, Paris L, Bagnoli M, et al. Characterization of in vivo ovarian cancer models by quantitative 1H magnetic resonance spectroscopy and diffusion-weighted imaging. NMR Biomed (2012) 25:632–42. doi:10.1002/nbm.1779
50. Pisanu ME, Ricci A, Paris L, Surrentino E, Liliac L, Bagnoli M, et al. Monitoring response to cytostatic cisplatin in a HER2(+) ovary cancer model by MRI and in vitro and in vivo MR spectroscopy. Br J Cancer (2014) 110:625–35. doi:10.1038/bjc.2013.758
51. Esseridou A, Di Leo G, Sconfienza LM, Caldiera V, Raspagliesi F, Grijuela B, et al. In vivo detection of choline in ovarian tumors using 3D magnetic resonance spectroscopy. Invest Radiol (2011) 46:377–82. doi:10.1097/RLI.0b013e31821690ef
52. Katz-Brűll R, Lavin PT, Lenkinsi RE. Clinical utility of proton magnetic resonance spectroscopy in characterizing breast lesions. J Natl Cancer Inst (2002) 94:1197–202. doi:10.1093/jnci/94.16.1197
53. Sardanelli F, Fausto A, Di Leo G, de Nijs R, Vorbuchner M, Podo F. In vivo proton MR spectroscopy of the breast using the total peak integral as a marker of malignancy. AJR Am J Roentgenol (2009) 192:1608–17. doi:10.2214/AJR.07.3521
54. Gadiya M, Mori N, Cao MD, Mironchik Y, Kakkad S, Gribbestad IS, et al. Phospholipase D1 and choline kinase-α are interactive targets in breast cancer. Cancer Biol Ther (2014) 15:593–601. doi:10.4161/cbt.28165
55. Kumar M, Arlauckas SP, Saksena S, Verma G, Ittyerah R, Pickup S, et al. Magnetic resonance spectroscopy for detection of choline kinase inhibition in the treatment of brain tumors. Mol Cancer Ther (2015) 14:899–908. doi:10.1158/1535-7163.MCT-14-0775
56. Granata A, Nicoletti R, Perego P, Iorio E, Krishnamachary B, Benigni F, et al. Global metabolic profile identifies choline kinase alpha as a key regulator of glutathione-dependent antioxidant cell defense in ovarian carcinoma. Oncotarget (2015) 6:11216–30. doi:10.18632/oncotarget.3589
57. Canese R, Mezzanzanica D, Bagnoli M, Indraccolo S, Canevari S, Podo F, et al. In vivo magnetic resonance metabolic and morphofunctional fingerprints in experimental models of human ovarian cancer. Front Oncol (2016) 6:164. doi:10.3389/fonc.2016.00164
58. Eliyahu G, Kreizman T, Degani H. Phosphocholine as a biomarker of breast cancer: molecular and biochemical studies. Int J Cancer (2007) 120:1721–30. doi:10.1002/ijc.22293
59. Chen MB, Lamar JM, Li R, Hynes RO, Kamm RD. Elucidation of the roles of tumor integrin β1 in the extravasation stage of the metastasis cascade. Cancer Res (2016) 76:2513–24. doi:10.1158/0008-5472.CAN-15-1325
60. Longva KE, Pedersen NM, Haslekås C, Stang E, Madshus IH. Herceptin-induced inhibition of ErbB2 signaling involves reduced phosphorylation of Akt but not endocytic down-regulation of ErbB2. Int J Cancer (2005) 116:659–67. doi:10.1002/ijc.21015
61. Miyake T, Parsons SJ. Functional interactions between ChoK-alpha, epidermal growth factor receptor and c-Src in breast cancer cell proliferation. Oncogene (2012) 31:1431–41. doi:10.1038/onc.2011.332
62. Carrasco S, Mérida I. Diacylglycerol, when simplicity becomes complex. Trends Biochem Sci (2007) 32:27–36. doi:10.1016/j.tibs.2006.11.004
63. Haucke V, Di Paolo G. Lipids and lipid modifications in the regulation of membrane traffic. Curr Opin Cell Biol (2007) 19:426–35. doi:10.1016/j.ceb.2007.06.003
64. Asim M, Massie CE, Orafidiya F, Pértega-Gomes N, Warren AY, Esmaeili M, et al. Choline kinase alpha as an androgen receptor chaperone and prostate cancer therapeutic target. J Natl Cancer Inst (2016) 108:djv371. doi:10.1093/jnci/djv371
65. Kato M, Hammam MAS, Taniguchi T, Suga Y, Mond K. What is the true structure of D609, a widely used lipid related enzyme inhibitor? Org Lett (2016) 18:768–71. doi:10.1021/acs.orglett.6b00025
66. Frank NY, Schatton T, Frank MH. The therapeutic promise of the cancer stem cell concept. J Clin Invest (2010) 120:41–50. doi:10.1172/JCI41004
Keywords: choline metabolism, phosphatidylcholine phospholipase C, choline kinase, breast cancer, ovarian cancer, magnetic resonance spectroscopy, targeted therapy
Citation: Podo F, Paris L, Cecchetti S, Spadaro F, Abalsamo L, Ramoni C, Ricci A, Pisanu ME, Sardanelli F, Canese R and Iorio E (2016) Activation of Phosphatidylcholine-Specific Phospholipase C in Breast and Ovarian Cancer: Impact on MRS-Detected Choline Metabolic Profile and Perspectives for Targeted Therapy. Front. Oncol. 6:171. doi: 10.3389/fonc.2016.00171
Received: 16 March 2016; Accepted: 05 July 2016;
Published: 02 August 2016
Edited by:
Cicero Matthew R. Habito, Harvard Medical School, USAReviewed by:
Orazio Schillaci, University of Rome Tor Vergata, ItalyCopyright: © 2016 Podo, Paris, Cecchetti, Spadaro, Abalsamo, Ramoni, Ricci, Pisanu, Sardanelli, Canese and Iorio. This is an open-access article distributed under the terms of the Creative Commons Attribution License (CC BY). The use, distribution or reproduction in other forums is permitted, provided the original author(s) or licensor are credited and that the original publication in this journal is cited, in accordance with accepted academic practice. No use, distribution or reproduction is permitted which does not comply with these terms.
*Correspondence: Franca Podo, ZnJhbmNhLnBvZG9AaXNzLml0, ZnJhbmNhLnBvZG9AYWxpY2UuaXQ=
Disclaimer: All claims expressed in this article are solely those of the authors and do not necessarily represent those of their affiliated organizations, or those of the publisher, the editors and the reviewers. Any product that may be evaluated in this article or claim that may be made by its manufacturer is not guaranteed or endorsed by the publisher.
Research integrity at Frontiers
Learn more about the work of our research integrity team to safeguard the quality of each article we publish.