- 1Instituto de Microbiologia, Universidade Federal do Rio de Janeiro, Rio de Janeiro, Brazil
- 2Laboratório de Glicobiologia, Instituto de Biofísica Carlos Chagas Filho, Universidade Federal do Rio de Janeiro, Rio de Janeiro, Brazil
Glycans are part of the essential components of a cell. These compounds play a fundamental role in several physiopathological processes, including cell differentiation, adhesion, motility, signal transduction, host–pathogen interactions, tumor cell invasion, and metastasis development. Glycans are also able to exert control over the changes in tumor immunogenecity, interfering with tumor editing events and leading to immune-resistant cancer cells. The involvement of glycans in cancer progression is related to glycosylation alterations. Understanding such changes is, therefore, extremely useful to set the stage for their use as biomarkers, improving the diagnostics and therapeutic strategies. Herein, we discuss the basis of how modifications in glycosylation patterns may contribute to cancer genesis and progression as well as their importance in oncology field.
Glycosylation as an Essential Protein Post-Translational Modification
Post-translational modifications (PTMs) exert an important role in controlling protein function in eukaryotes. PTMs comprise processes such as acetylation, carbonylation, methylation, hydroxylation, nitration, palmitoylation, phosphorylation, sulfation, ubiquitination, and glycosylation (1, 2). It is universally accepted that deregulation of such PTMs may lead to the development of a number of diseases. The glycosylation is the most common PTM and occurs in all domains of life. As a result, they impart an additional level of “information content” to underlying protein structures (3, 4). Two basic types of protein glycosylations are N- and O-glycosylations (Figure 1) with significant differences in terms of their biosynthesis and structures, as well as their location within the protein chain (5). In N-linked glycans, the nitrogen atom in the side chain of asparagine is attached to N-acetylglucosamine (GlcNAc). The sequence can be Asn–X–Ser or Asn–X–Thr, where X is any kind of amino acid except proline. In O-linked glycans, the oxygen atom in the side chain of serine or threonine is attached to N-acetylgalactosamine (GalNAc) (5). Furthermore, the glycopeptides Asn-GlcNAc or Ser/Thr-GalNAc may be extended by numerous and specific glycosyltransferase activities (6, 7) (Figure 1).
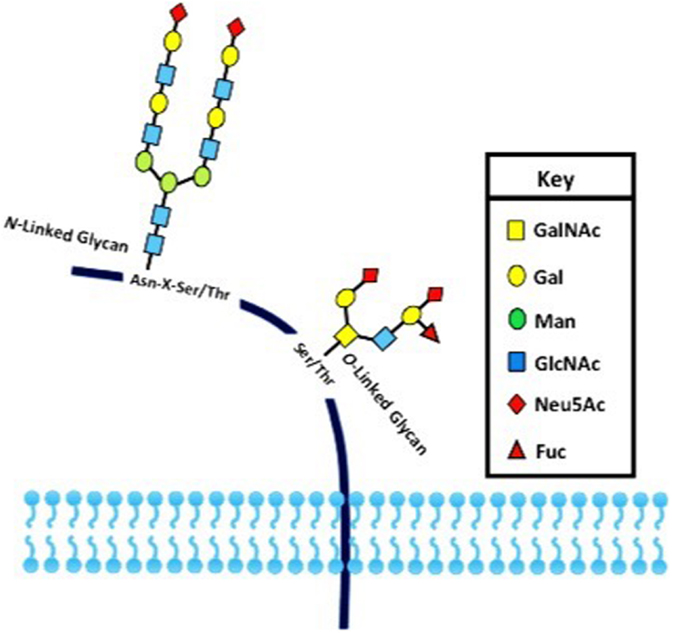
Figure 1. The two major types of protein glycosylation. The attachment of sugar moieties to proteins is a post-translational modification that provides greater proteomic diversity to the proteins. N-linked glycosylation occurs through the asparagine residues of the protein, while O-linked glycosylation occurs through serine or threonine.
Altered Tumor-Cell Glycosylation Promotes Cancer Virulence
During the past few years, we have seen a breakthrough in understanding the molecular and cellular mechanisms of immune cell activation and homeostasis. Several studies have shown that glycosylation orchestrates important features in several pathological processes, mainly in cancer development and/or progression (8–11). Extensive progresses in defining the cellular and molecular networks that regulate the immune responses against different sort of tumors have renewed our enthusiasm to search for potential cancer immunotherapies. However, the successful translation of novel mechanistic insights into effective tumor immunotherapy is hindered by a number of obstacles, including the ability of tumor cells to generate a tolerant microenvironment (12–15). The immune response is crucial not only to elicit protection against pathogens but also to maintain immune surveillance against the development of malignant cells. From this perspective, the development of cancer can be seen as a failure of immune surveillance (16–18). The notion that the immune system can recognize and extinguish developing transformed cells was originally exemplified by the Burnet and Thomas’ hypothesis concerning the mechanisms of cancer immune surveillance (19, 20). This hypothesis supports that the immunoediting of tumor antigens is responsible for sculpting the immunogenic phenotypes of transformed cells that eventually induce immunocompetent hosts. However, most tumor-associated carbohydrate antigens (TACAs) do not elicit strong humoral responses and, in fact, pieces of evidence have shown that the aberrant expression of glycan structures, as well as occurrence of truncated structures, precursors, or novel structures of glycan might prevent effective immune responses against tumor cells (21–23). Some TACAs with immunomodulatory or immunosuppressive properties are presented in Table 1.
Tumor-Associated Glycan Determinants Subvert Key Immunological Defense Mechanisms
The first demonstration that tumor cells express altered glycans came from studies showing that monoclonal antibodies may recognize such abnormal structures (38). Many of these alterations are accompanied by expression of oncofetal antigens on tumor glycoproteins as well as glycosphingolipids (38, 39). Furthermore, it is well known that modifications in glycan structures may contribute to early stages of invasion (40–42), although it is not clear if such alterations may also play a role in the genesis of neoplastic cells (43, 44).
Glycosphingolipids as Immunosuppressive Components in Tumor Cell Progression
Glycosphingolipids might also be involved in tumor cell progression by causing immune silencing. It has been also described that different types of cancer cells occasionally secrete gangliosides into the bloodstream (45, 46). In spite of their immunogenic properties, shaded membrane sialoglycolipids (gangliosides) may cause inhibition of co-stimulatory molecules synthesis, thus, promoting an impairment of dendritic cell (DC) maturation, leading to immune silencing by means of the inability of these cells to arm effective antitumor T cells responses (47, 48). A promising antitumor therapeutic approach using glycosphingolipids relies on therapeutic manipulation of these molecules to generate passive as well as active immunity (49–51).
Role of Endogenous Lectins Blocking the Adaptive Immunity Against Tumor Cells
Alterations in the glycosylation profile in tumor compared to health cells are mainly attributed to the gene expression deregulation of glycosyltransferases, enzymes responsible to transfer sugars from donor to acceptor substrate molecules, leading to the synthesis of immature core glycans (52, 53). Specific glycans may bind to cell surface lectins such as galectins, C-type lectins, and siglecs [sialic acid (Sia)-binding immunoglobulin-type lectins], resulting in the regulation of cancer immune responses due to interference with the tumor immunoediting, characterized by changes in the immunogenicity of target antigens that could favor the dissemination of cancer cells (54–56). Galectins are β-galactoside-binding proteins that share homology in the amino acid sequence of their carbohydrate-recognition domain. Their role in immune responses against tumor cells has been studied over the past years (57–59).
In cancer, galectins secreted by tumor cells exhibit tolerogenic effects over effector T cells, promoting a cytokine imbalance that can either result in T cell anergy or favoring T regulatory (T reg) cell activity (60, 61). This immune modulation leading to tolerogenic responses against tumor cells can be associated with galectin-1 expression (62). The galectin-3, another member of galectin family, is known to induce apoptosis of antitumor CD8+ T cells (CTLs) in murine model of colorectal cancer (63). In addition, galectin-3 was demonstrated to increase the distance between the TCR and CD8 molecule in human CTLs infiltrating the tumors, a matter that turn them to be anergic (64). Besides their role in attenuating adaptive immune responses, galectins are also described to be able to impair the antitumor functions of natural killer (NK) cells (54).
The C-type lectins constitute by far the largest family of animal lectins found as part of membrane proteins and in soluble forms, comprising L-selectin, P-selectin, and E-selectin glycoproteins (65–67). These molecules act promoting the adhesion of leukocytes to the vascular endothelium, recognizing sialyl Lewis X (SLeX), sialyl Lewis A (SLeA), and Sia found in O-linked glycans (68). Such Lewis carbohydrate antigens can be also found on tumor cells as part of mucin glycoproteins and selectins that play a major role in migration processes by binding to endothelium during tissue infiltration along tumor metastasis (69). In murin models, mice displaying deficiency in L- and P-selectins presented a reduction of the metastisation (70). In addition, specific intercellular adhesion molecule-3 grabbing non-integrin 1 expressed on DCs (DC-SIGN), another transmembrane protein belonging to the C-type lectin family, is expressed by DCs and binds to aberrant O-glycosylation structures on cancer cells (71, 72). These abnormal glycoconjugates expressed by tumor cells are also able to interact with other class of receptors on DCs as well as macrophages, known as macrophage galactose-type C-type lectin, which are able to induce cellular cytotoxicity (73).
Role of Sialic Acid Domains in Avoiding Cell-Mediated Immunity Against Tumor Cells
Immune cells continually screen the glycan structures that are expressed on cell surface glycoproteins from pathogen and host cells. The Sias are part of these multiple cell surface carbohydrates (74). The Sia motifs are differently expressed among the species, thus, allowing the immune system to distinguish self from non-self. In this sense, pathogens evolved to express Sia molecules mimicking the one present in host cells, therefore, subverting the host immunity (75, 76). The idea of Sia as self-associated molecular patterns (SAMPs) came from the fact that they may elicit inhibitory signals in order to prevent inadequate immune responses (77). Nonetheless, a growing body of evidence has been pointed out to an extensive contribution of sialoglycan motifs to tumor immune subversion (78, 79).
A multiplicity of ways whereby Sia molecules contribute to immune evasion mechanisms has been described. Complement system has evolved as a first line of defense against non-self or invading pathogens (80, 81). In neoplastic transformation, inhibition of complement activation allows the tumor cells to escape from immune responses (82). In fact, lung cancer cells and glioblastomas, for instance, are able to produce the inhibitory complement factor H, thus avoiding their elimination (83, 84). Tumor cells may evade the complement system activity through binding of Sia motifs present on their surface to polyanionic sites of the complement factor H component, thus activating a complement negative regulatory pathway (85).
Sialoglycans also play a role in tumor immunity mediated by NK cells, which are able to recognize transformed cells due to the lack of MCH class I molecules. However, tumor cells in turn may express inhibitory receptors impairing the cytotoxicity of NK cells (85). The presence of a dense layer of sialoglycans on tumor cell surfaces avoids the normal occurrence of immunological synapses between cancer and NK cells. Such reduced recognition is believed to be enhanced by hypersialylation of tumor ligands for the CD94/NKG2 family of transmembrane C-type lectin-like receptors (NKG2D) expressed by not only NK cells but also NK1.1+ T cells, γδ T cells, and activated CD8+αβ T cells and macrophages (86). The NKG2D receptors specifically recognize self-proteins from MIC and RAET1/ULBP families induced on the surface of stressed, malignant transformed, and infected cells (87). The hypersialylation of tumor ligands is thought to repulse their interaction with NKG2D receptors via highly negative charge (88).
The tumor-derived sialoglycans can also affect antitumor functions of NK cells via Sia/siglecs binding (89, 90). In fact, it has been shown that the immunomodulatory effects of tumor cells in part have influence of interactions between sialoglycans derived from transformed cells and the immune inhibitory siglec receptors (69). In fact, studies have demonstrated that overexpression of siglec ligands in tumor cells leads to impairment of the protective immune responses elicited by NK cells and neutrophils (91, 92). In addition, blockade of siglec-9 improved antitumor neutrophil responses in vitro (92). Siglec receptor triggering with sialylated mucins derived from tumor cells is able to induce inhibitory signals to immune cells, a process that is thought to be associated with tumor progress (41).
Tumor-derived sialoglycans can target different aspects of the immune system to promote evasion responses. It has been shown that tumor-derived Sia inhibits the traffic and subsequent exocytosis of lytic granules from CTLs to the immunological synapse, disabling a key mechanism whereby these lymphocytes eradicate tumor cells (93). Moreover, Sias have also been described to take part in the hypersialylation process of Fas receptor (CD95) on tumor cells, damping its binding to the Fas-ligand (CD95L) expressed by CTLs (94). The blockage of CD95/CD95L interaction impairs the downstream activation of caspases and consequently disarming the apoptosis machinery that would lead to tumor cell elimination (69). In this context, hypersialylation of Fas receptor by upregulation of sialyltransferase ST6Gal-I in tumor cells has also been described (95).
Besides its effects on cytotoxic T cells, tumor-derived sialoglycans are also able to dampen DC functions (96–100). Studies have shown that tumor-derived sialogangliosides inhibit the upregulation of co-stimulatory molecules (CD80/CD86) as well as IL-12 production by DCs, thus impairing T cell effector lymphocyte activation (101). This immunosuppressive effect is thought to be elicited by the interaction of highly sialylated tumor antigens with the siglec receptors expressed by DCs (102, 103). Moreover, the interaction of tumor-derived sialylated antigens with siglec receptors expressed by macrophages has also been described as a mechanism influencing tumor progression (104). In these studies, siglec-9 expressed by macrophages is shown to induce high levels of the immunosuppressive IL-10 cytokine together with reduction of the pro-inflammatory TNF-α cytokine associated with antitumoral responses (105).
Concluding Remarks
Cell surface glycosylation patterns may suffer important changes during pathological conditions, especially in tumor invasion processes. Such alterations are the result of genetics as well as epigenetics changes, conferring to the tumor cells the ability of dissemination by escaping the immunesurveillance mechanisms (10, 106). This immune evasion phenomenon is being clarified, pointing out an important role for the shield created by altered sialylated glycans on the surface of cancer cells on the subversion of the immune system. This evolutionary conserved strategy can also be observed in some pathogens such as trypanosomatids, bacteria, and fungi (57). Understanding how abnormal glycosylation patterns effectively contribute to tumor-induced immune deviation would lead to early detection of potential tissue alterations and ultimately the development of therapeutic approaches against cancer.
Author Contributions
AN, LF-L, CGF-L, and AM wrote the paper. All authors read and approved the final version of the manuscript.
Conflict of Interest Statement
The authors declare that the research was conducted in the absence of any commercial or financial relationships that could be construed as a potential conflict of interest.
Funding
This work was supported by grants from Conselho Nacional de Desenvolvimento Científico e Tecnológico do Brasil (CNPq), Fundação de Amparo à Pesquisa do Estado do Rio de Janeiro (FAPERJ). AM and CGF-L are recipients of fellowships from CNPq.
References
1. Karve TM, Cheema AK. Small changes huge impact: the role of protein post-translational modifications in cellular homeostasis and disease. J Amino Acids (2011) 2011:207691. doi: 10.4061/2011/207691
2. Seo J, Lee KJ. Post-translational modifications and their biological functions: proteomic analysis andsystematic approaches. J Biochem Mol Biol (2004) 37(1):35–44. doi:10.5483/BMBRep.2004.37.1.035
3. Fuster MM, Esko JD. The sweet and sour of cancer: glycans as novel therapeutic targets. Nat Rev Cancer (2005) 5(7):526–42. doi:10.1038/nrc1649
4. Moremen KW, Tiemeyer M, Nairn AV. Vertebrate protein glycosylation: diversity, synthesis and function. Nat Rev Mol Cell Biol (2012) 13(7):448–62. doi:10.1038/nrm3383
5. Spiro RG. Protein glycosylation: nature, distribution, enzymatic formation, and disease implications of glycopeptide bonds. Glycobiology (2002) 12(4):43R–56R. doi:10.1093/glycob/12.4.43R
6. Schwarz F, Aebi M. Mechanisms and principles of N-linked protein glycosylation. Curr Opin Struct Biol (2011) 21(5):576–82. doi:10.1016/j.sbi.2011.08.005
7. Lehle L, Strahl S, Tanner W. Protein glycosylation, conserved from yeast to man: a model organism helps elucidate congenital human diseases. Angew Chem Int Ed Engl (2006) 45(41):6802–18. doi:10.1002/anie.200601645
8. Nicholas NS, Apollonio B, Ramsay AG. Tumor microenvironment (TME)-driven immune suppression in B cell malignancy. Biochim Biophys Act (2016) 1863(3):471–82. doi:10.1016/j.bbamcr.2015.11.003
9. Kölbl AC, Andergassen U, Jeschke U. The role of glycosylation in breast cancer metastasis and cancer control. Front Oncol (2015) 5:219. doi:10.3389/fonc.2015.00219
10. Ohtsubo K, Marth JD. Glycosylation in cellular mechanisms of health and disease. Cell (2006) 126(5):855–67. doi:10.1016/j.cell.2006.08.019
11. Hakomori S. Glycosylation defining cancer malignancy: new wine in an old bottle. Proc Natl Acad Sci U S A (2002) 99(16):10231–3. doi:10.1073/pnas.172380699
12. Eyre TA, Collins GP. Immune checkpoint inhibition in lymphoid disease. Br J Haematol (2015) 170(3):291–304. doi:10.1111/bjh.13397
13. Guo Y, Wang AY. Novel immune check-point regulators in tolerance maintenance. Front Immunol (2015) 18(6):421. doi:10.3389/fimmu.2015.00421
14. Vinay DS, Ryan EP, Pawelec G, Talib WH, Stagg J, Elkord E, et al. Immune evasion in cancer: mechanistic basis and therapeutic strategies. Semin Cancer Biol (2015) 35:S185–98. doi:10.1016/j.semcancer.2015.03.004
15. Liu Y, Cao X. Immunosuppressive cells in tumor immune escape and metastasis. J Mol Med (2015) 93:1–14. doi:10.1007/s00109-015-1376-x
16. Prestwich RJ, Errington F, Hatfield P, Merrick AE, Ilett EJ, Selby PJ, et al. The immune system – is it relevant to cancer development, progression and treatment? Clin Oncol (2008) 20(2):101–12. doi:10.1016/j.clon.2007.10.011
17. Kim R, Emi M, Tanabe K. Cancer immunoediting from immune surveillance to immune escape. Immunology (2007) 121(1):1–14. doi:10.1111/j.1365-2567.2007.02587.x
18. Morvan MG, Lanier LL. NK cells and cancer: you can teach innate cells new tricks. Nat Rev Cancer (2015) 16(1):7–19. doi:10.1038/nrc.2015.5
19. Burnet M. Cancer; a biological approach. I. The processes of control. Br Med J (1957) 1(5022):779–86. doi:10.1136/bmj.1.5022.779
21. Monzavi-Karbassi B, Pashov A, Kieber-Emmons T. Tumor-associated glycans and immune surveillance. Vaccines (Basel) (2013) 1(2):174–203. doi:10.3390/vaccines1020174
22. Pashov A, Monzavi-Karbassi B, Kieber-Emmons T. Immune surveillance and immunotherapy: lessons from carbohydrate mimotopes. Vaccine (2009) 27(25–26):3405–15. doi:10.1016/j.vaccine.2009.01.074
23. Pashov A, Monzavi-Karbassi B, Raghava GP, Kieber-Emmons T. Bridging innate and adaptive antitumor immunity targeting glycans. J Biomed Biotechnol (2010) 2010:354068. doi:10.1155/2010/354068
24. Li D, Li Y, Wu X, Li Q, Yu J, Gen J, et al. Knockdown of Mgat5 inhibits breast cancer cell growth with activation of CD4+ T cells and macrophages. J Immunol (2008) 180(5):3158–65. doi:10.4049/jimmunol.180.5.3158
25. Takamiya R, Ohtsubo K, Takamatsu S, Taniguchi N, Angata T. The interaction between Siglec-15 and tumor-associated sialyl-Tn antigen enhances TGF-beta secretion from monocytes/macrophages through the DAP12-Syk pathway. Glycobiology (2013) 23(2):178–87. doi:10.1093/glycob/cws139
26. Carrascal MA, Severino PF, Guadalupe Cabral M, Silva M, Ferreira JA, Calais F, et al. Sialyl Tn-expressing bladder cancer cells induce a tolerogenic phenotype in innate and adaptive immune cells. Mol Oncol (2014) 8(3):753–65. doi:10.1016/j.molonc.2014.02.0088
27. Kannagi R, Izawa M, Koike T, Miyazaki K, Kimura N. Carbohydrate-mediated cell adhesion in cancer metastasis and angiogenesis. Cancer Sci (2004) 95(5):377–84. doi:10.1111/j.1349-7006.2004.tb03219.x
28. Schultz MJ, Swindall AF, Bellis SL. Regulation of the metastatic cell phenotype by sialylated glycans. Cancer Metastasis Rev (2012) 31(3–4):501–18. doi:10.1007/s10555-012-9359-7
29. Ohyama C, Tsuboi S, Fukuda M. Dual roles of sialyl Lewis X oligosaccharides in tumor metastasis and rejection by natural killer cells. EMBO J (1999) 18(6):1516–25. doi:10.1093/emboj/18.6.1516
30. Tsuboi S, Hatakeyama S, Ohyama C, Fukuda M. Two opposing roles of O-glycans in tumor metastasis. Trends Mol Med (2012) 18(4):224–32. doi:10.1016/j.molmed.2012.02.001
31. Suzuki Y, Sutoh M, Hatakeyama S, Mori K, Yamamoto H, Koie T, et al. MUC1 carrying core 2 O-glycans functions as a molecular shield against NK cell attack, promoting bladder tumor metastasis. Int J Oncol (2012) 40(6):1831–8. doi:10.3892/ijo.2012.1411
32. Tsuboi S. Tumor defense systems using O-glycans. Biol Pharm Bull (2012) 35(10):1633–6. doi:10.1248/bpb.b12-00367
33. Okamoto T, Yoneyama MS, Hatakeyama S, Mori K, Yamamoto H, Koie T, et al. Core2 O-glycan-expressing prostate cancer cells are resistant to NK cell immunity. Mol Med Rep (2013) 7(2):359–64. doi:10.3892/mmr.2012.1189
34. Samraj AN, Pearce OM, Läubli H, Crittenden AN, Bergfeld AK, Banda K, et al. A red meat-derived glycan promotes inflammation and cancer progression. Proc Natl Acad Sci U S A (2015) 112(2):542–7. doi:10.1073/pnas.1417508112
35. Pearce OM, Läubli H, Verhagen A, Secrest P, Zhang J, Varki NM, et al. Inverse hormesis of cancer growth mediated by narrow ranges of tumor-directed antibodies. Proc Natl Acad Sci U S A (2014) 111(16):5998–6003. doi:10.1073/pnas.1209067111
36. Pearce OM, Läubli H. Sialic acids in cancer biology and immunity. Glycobiology (2016) 26(2):111–28. doi:10.1093/glycob/cwv097
37. Samraj AN, Läubli H, Varki N, Varki A. Involvement of a non-human sialic acid in human cancer. Front Oncol (2014) 19(4):33. doi:10.3389/fonc.2014.00033
38. Feizi T. Demonstration by monoclonal antibodies that carbohydrate structures of glycoproteins and glycolipids are onco-developmental antigens. Nature (1985) 314(6006):53–7. doi:10.1038/314053a0
39. Holmes EH, Ostrander GK, Clausen H, Graem N. Oncofetal expression of Lex carbohydrate antigens in human colonic adenocarcinomas. Regulation through type 2 core chain synthesis rather than fucosylation. J Biol Chem (1987) 262(23):11331–8.
40. Reticker-Flynn NE, Bhatia SN. Aberrant glycosylation promotes lung cancer metastasis through adhesion to galectins in the metastatic niche. Cancer Discov (2015) 5(2):168–81. doi:10.1158/2159-8290.CD-13-0760
41. Boligan KF, Mesa C, Fernandez LE, von Gunten S. Cancer intelligence acquired (CIA): tumor glycosylation and sialylation codes dismantling antitumor defense. Cell Mol Life Sci (2015) 72(7):1231–48. doi:10.1007/s00018-014-1799-5
42. Freire-de-Lima L. Sweet and sour: the impact of differential glycosylation in cancer cells undergoing epithelial-mesenchymal transition. Front Oncol (2014) 25(4):59. doi:10.3389/fonc.2014.00059
43. Kakugawa Y, Wada T, Yamaguchi K, Yamanami H, Ouchi K, Sato I, et al. Up-regulation of plasma membrane-associated ganglioside sialidase (Neu3) in human colon cancer and its involvement in apoptosis suppression. Proc Natl Acad Sci U S A (2002) 99(16):10718–23. doi:10.1073/pnas.152597199
44. Handa K, Hakomori SI. Carbohydrate to carbohydrate interaction in development process and cancer progression. Glycoconj J (2012) 29(8–9):627–37. doi:10.1007/s10719-012-9380-7
45. Varki A, Cummings RD, Esko JD, Freeze H, Stanley P, Bertozzi C, et al. Essentials of Glycobiology. New York: Cold Spring Harbor Laboratory Press (2009).
46. Hakomori S. Tumor malignancy defined by aberrant glycosylation and sphingo(glyco)lipid metabolism. Cancer Res (1996) 56(23):5309–18.
47. Wolfl M, Batten WY, Posovszky C, Bernhard H, Berthold F. Gangliosides inhibit the development from monocytes to dendritic cells. Clin Exp Immunol (2002) 130(3):441–8. doi:10.1046/j.1365-2249.2002.02006.x
48. Caldwell S, Heitger A, Shen W, Liu Y, Taylor B, Ladisch S. Mechanisms of ganglioside inhibition of APC function. J Immunol (2003) 171(4):1676–83. doi:10.4049/jimmunol.171.4.1676
49. Bestagno M, Occhino M, Corrias MV, Burrone O, Pistoia V. Recombinant antibodies in the immunotherapy of neuroblastoma: perspectives of new developments. Cancer Lett (2003) 197(1–2):193–8. doi:10.1016/S0304-3835(03)00109-5
50. Carr A, Rodríguez E, Arango Mdel C, Camacho R, Osorio M, Gabri M, et al. Immunotherapy of advanced breast cancerwith a heterophilic ganglioside (NeuGcGM3) cancervaccine. J Clin Oncol (2003) 21(6):1015–21. doi:10.1200/JCO.2003.02.124
51. Ragupathi G, Livingston PO, Hood C, Gathuru J, Krown SE, Chapman PB, et al. Consistent antibody response against ganglioside GD2 induced in patients with melanoma by a GD2 lactone-keyhole limpet hemocyanin conjugate vaccine plus immunological adjuvant QS-21. Clin Cancer Res (2003) 9(14):5214–20.
52. Brooks SA, Carter TM, Royle L, Harvey DJ, Fry SA, Kinch C, et al. Altered glycosylation of proteins in cancer: what is the potential for new anti-tumour strategies. Anticancer Agents Med Chem (2008) 8(1):2–21. doi:10.2174/187152008783330860
53. Amon R, Reuven EM, Leviatan Ben-Arye S, Padler-Karavani V. Glycans in immune recognition and response. Carbohydr Res (2014) 7(389):115–22. doi:10.1016/j.carres.2014.02.004
54. Rabinovich GA, Toscano MA. Turning ‘sweet’ on immunity: galectin-glycan interactions in immune tolerance and inflammation. Nat Rev Immunol (2009) 9(5):338–52. doi:10.1038/nri2536
55. Macauley MS, Crocker PR, Paulson JC. Siglec-mediated regulation of immune cell function in disease. Nat Rev Immunol (2014) 14(10):653–66. doi:10.1038/nri3737
56. Pinho SS, Reis CA. Glycosylation in cancer: mechanisms and clinical implications. Nat Rev Cancer (2015) 15(9):540–55. doi:10.1038/nrc3982
57. Rambaruth ND, Dwek MV. Cell surface glycan-lectin interactions in tumor metastasis. Acta Histochem (2011) 113(6):591–600. doi:10.1016/j.acthis.2011.03.001
58. Perone MJ, Bertera S, Shufesky WJ, Divito SJ, Montecalvo A, Mathers AR, et al. Suppression of autoimmune diabetes by soluble galectin-1. J Immunol (2009) 182(5):2641–53. doi:10.4049/jimmunol.0800839
59. Rabinovich GA, Liu FT, Hirashima M, Anderson A. An emerging role for galectins in tuning the immune response: lessons from experimental models of inflammatory disease, autoimmunity and cancer. Scand J Immunol (2007) 66(2–3):143–58. doi:10.1111/j.1365-3083.2007.01986.x
60. Rabinovich GA, Gabrilovich D, Sotomayor EM. Immunossupressive strategies that are mediated by tumor cells. Annu Rev Immunol (2007) 25:267–96. doi:10.116/annurev.immunol.25.022106.141609
61. Liu FT, Rabinovich GA. Galectins as modulators of tumour progression. Nat Rev Cancer (2005) 5(1):29–41. doi:10.1038/nrc1527
62. Le QT, Shi G, Cao H, Nelson DW, Wang Y, Chen EY, et al. Galectin-1: a link between tumor hypoxia and tumor immune privilege. J Clin Oncol (2005) 23(35):8932–41. doi:10.1200/JCO.2005.02.0206
63. Peng W, Wang HY, Miyahara Y, Peng G, Wang RF. Tumor-associated galectin-3 modulates the function of tumor-reactive T cells. Cancer Res (2008) 68(17):7228–36. doi:10.1158/0008-5472
64. Demotte N, Stroobant V, Courtoy PJ, Van Der Smissen P, Colau D, Luescher IF, et al. Restoring the association of the T cell receptor with CD8 reverses anergy in human tumor-infiltrating lymphocytes. Immunity (2008) 28(3):414–24. doi:10.1016/j.immuni.2008.01.011
65. Glavey SV, Huynh D, Reagan MR, Manier S, Moschetta M, Kawano Y, et al. The cancer glycome: carbohydrates as mediators of metastasis. Blood Rev (2015) 29(4):269–79. doi:10.1016/j.blre.2015.01.003
66. Nakahara S, Raz A. Biological modulation by lectins and their ligands in tumor progression and metastasis. Anticancer Agents Med Chem (2008) 8(1):22–36. doi:10.2174/187152008783330833
67. Fukuda M, Hiraoka N, Yeh JC. C-type lectins and sialyl Lewis X oligosaccharides. Versatile roles in cell-cell interaction. J Cell Biol (1999) 147(3):467–70. doi:10.1083/jcb.147.3.467
68. Varki A. Selectin ligands. Proc Natl Acad Sci U S A (1994) 91(16):7390–7. doi:10.1073/pnas.91.16.7390
69. Häuselmann I, Borsig L. Altered tumor-cell glycosylation promotes metastasis. Front Oncol (2014) 4:28. doi:10.3389/fonc.2014.00028
70. Kohler S, Ullrich S, Richter U, Schumacher U. E-/P-selectins and colon carcinoma metastasis: first in vivo evidence for their crucial role in a clinically relevant model of spontaneous metastasis formation in the lung. Br J Cancer (2010) 102(3):602–9. doi:10.1038/sj.bjc.6605492
71. Guo Y, Feinberg H, Conroy E, Mitchell DA, Alvarez R, Blixt O, et al. Structural basis for distinct ligand-binding and targeting properties of the receptors DC-SIGN and DC-SIGNR. Nat Struct Mol Biol (2004) 11(7):591–8. doi:10.1038/nsmb784
72. Samsen A, Bogoevska V, Klampe B, Bamberger AM, Lucka L, Horst AK, et al. DC–SIGN and SRCL bind glycans of carcinoembryonic antigen (CEA) and CEA-related cell adhesion molecule 1 (CEACAM1): recombinant human glycan-binding receptors as analytical tools. Eur J Cell Biol (2010) 89(1):87–94. doi:10.1016/j.ejcb.2009.11.018
73. Lavrsen K, Madsen CB, Rasch MG, Woetmann A, Ødum N, Mandel U, et al. Aberrantly glycosylated MUC1 is expressed on the surface of breast cancer cells and a target for antibody-dependent cell-mediated cytotoxicity. Glycoconj J (2013) 30(3):227–36. doi:10.1007/s10719-012-9437-7
74. Varki A, Gagneux P. Multifarious roles of sialic acids in immunity. Ann N Y Acad Sci (2012) 1253:16–36. doi:10.1111/j.1749-6632.2012.06517.x
75. Khatua B, Ghoshal A, Bhattacharya K, Mandal C, Saha B, Crocker PR, et al. Sialic acids acquired by Pseudomonas aeruginosa are involved in reduced complement deposition and siglec mediated host-cell recognition. FEBS Lett (2010) 584(3):555–61. doi:10.1016/j.febslet.2009.11.087
76. Carlin AF, Uchiyama S, Chang YC, Lewis AL, Nizet V, Varki A. Molecular mimicry of host sialylated glycans allows a bacterial pathogen to engage neutrophil Siglec-9 and dampen the innate immune resposnse. Blood (2009) 113(14):3333–6. doi:10.1182/blood-2008-11-187302
77. Varki A. Since there are PAMPs and DAMPs, there must be SAMPs? Glycan “self-associatedmolecular patterns” dampen innate immunity, but pathogens can mimic them. Glycobiology (2011) 21(9):1121–4. doi:10.1093/glycob/cwr087
78. Sanford BH. An alteration in tumor histocompatibility induced by neuraminidase. Transplantation (1967) 5(5):1273–9. doi:10.1097/00007890-196709000-00005
79. Bagshawe KD, Currie GA. Immunogenicity of L 1210 murine leukaemia cells after treatment with neuraminidase. Nature (1968) 218(5148):1254–5. doi:10.1038/2181254a0
80. Walport MJ. Complement. First of two parts. N Engl J Med (2001) 344(14):1058–66. doi:10.1056/NEJM200104053441406
81. Ricklin D, Hajishengallis G, Yang K, Lambris JD. Complement: a key system for immune surveillance and homeostasis. Nat Immunol (2010) 11(9):785–97. doi:10.1038/ni.1923
82. Pio R, Corrales L, Lambris JD. The role of complement in tumor growth. Adv Exp Med Biol (2014) 772:229–62. doi:10.1007/978-1-4614-5915-6_11
83. Junnikkala S, Jokiranta TS, Friese MA, Jarva H, Zipfel PF, Meri S. Exceptional resistance of human H2 glioblastoma cells to complement-mediated killing by expression and utilization of factor H and factor H-like protein 1. J Immunol (2000) 164(11):6075–81. doi:10.4049/jimmunol.164.11.6075
84. Ajona D, Hsu YF, Corrales L, Montuenga LM, Pio R. Down-regulation of human complement factor H sensitizes non-small cell lung cancer cells to complement attack and reduces in vivo tumor growth. J Immunol (2007) 178(9):5991–8. doi:10.4049/jimmunol.178.9.5991
85. Büll C, den Brok MH, Adema GJ. Sweet escape: sialic acids in tumor immune evasion. Biochim Biophys Acta (2014) 1846(1):238–46. doi:10.1016/j.bbcan.2014.07.005
86. Lanier LL. NKG2D receptor and its ligands in host defense. Cancer Immunol Res (2015) 3(6):575–82. doi:10.1158/2326-6066
87. Cheng M, Chen Y, Xiao W, Sun R, Tian Z. NK cell-based immunotherapy for malignant diseases. Cell Mol Immunol (2013) 10(3):230–52. doi:10.1038/cmi.2013.10
88. Cohen M, Elkabets M, Perlmutter M, Porgador A, Voronov E, Apte RN, et al. Lichtenstein, sialylation of 3-methylcholanthrene-induced fibrosarcoma determinesantitumor immune responses during immunoediting. J Immunol (2010) 185(10):5869–78. doi:10.4049/jimmunol.1001635
89. Park JE, Wu DY, Prendes M, Lu SX, Ragupathi G, Schrantz N, et al. Fine specificity of natural killer T cells against GD3 ganglioside and identification of GM3 as an inhibitory natural killer T-cell ligand. Immunology (2008) 123(1):145–55. doi:10.1111/j.1365-2567.2007.02760.x
90. Webb TJ, Li X, Giuntoli RL II, Lopez PH, Heuser C, Schnaar RL, et al. Molecular identification of GD3 as a suppressor of the innate immune response in ovarian cancer. Cancer Res (2012) 72(15):3744–52. doi:10.1158/0008-5472.CAN-11-2695
91. Jandus C, Boligan KF, Chijioke O, Liu H, Dahlhaus M, Demoulins T, et al. Interactions between Siglec-7/9 receptors and ligands influence NK cell-dependent tumor immunosurveillance. J Clin Invest (2014) 124(4):1810–20. doi:10.1172/JCI65899
92. Läubli H, Pearce OM, Schwarz F, Siddiqui SS, Deng L, Stanczak MA, et al. Engagement of myelomonocytic Siglecs by tumor-associated ligands modulates the innate immune response to cancer. Proc Natl Acad Sci U S A (2014) 111(39):14211–6. doi:10.1073/pnas.1409580111
93. Lee HC, Wondimu A, Liu Y, Ma JS, Radoja S, Ladisch S. Ganglioside inhibition ofCD8+T cell cytotoxicity: interference with lytic granule trafficking and exocytosis. J Immunol (2012) 189(7):3521–7. doi:10.4049/jimmunol.1201256
94. Lee KH, Feig C, Tchikov V, Schickel R, Hallas C, Schütze S, et al. The role of receptor internalization in CD95 signaling. EMBO J (2006) 25(5):1009–23. doi:10.1038/sj.emboj.7601016
95. Swindall AF, Bellis SL. Sialylation of the Fas death receptor by ST6Gal-I provides protection against Fas-mediated apoptosis in colon carcinoma cells. J Biol Chem (2011) 286(26):22982–90. doi:10.1074/jbc.M110.211375
96. Steinman RM, Adams JC, Cohn ZA. Identification of a novel cell type in peripheral lymphoid organs of mice. IV. Identification and distribution in mouse spleen. J Exp Med (1975) 141:804–20.
97. Steinman RM, Cohn ZA. Identification of a novel cell type in peripheral lymphoid organs of mice. I. Morphology, quantitation, tissue distribution. J Exp Med (1973) 137:1142–62. doi:10.1084/jem.137.5.1142
98. Steinman RM, Cohn ZA. Identification of a novel cell type in peripheral lymphoid organs of mice. II. Functional properties in vitro. J Exp Med (1974) 139:380–97. doi:10.1084/jem.139.2.380
99. Steinman RM, Lustig DS, Cohn ZA. Identification of a novel cell type in peripheral lymphoid organs of mice. III. Functional properties in vivo. J Exp Med (1974) 139:1431–45. doi:10.1084/jem.139.6.1431
100. Steinman RM, Witmer MD. Lymphoid dendritic cells are potent stimulators of the primary mixed leukocyte reaction in mice. Proc Natl Acad Sci U S A (1978) 75:5132–6. doi:10.1073/pnas.75.10.5132
101. Jales A, Falahati R, Mari E, Stemmy EJ, Shen W, Southammakosane C, et al. Ganglioside-exposed dendritic cells inhibit T-cell effectorfunction by promoting regulatory cell activity. Immunology (2011) 132(1):134–43. doi:10.1111/j.1365-2567.2010.03348.x
102. Péguet-Navarro J, Sportouch M, Popa I, Berthier O, Schmitt D, Portoukalian J. Gangliosides from human melanoma tumors impair dendritic cell differentiation from monocytes and induce their apoptosis. J Immunol (2003) 170(7):3488–94. doi:10.4049/jimmunol.170.7.3488
103. Shurin GV, Shurin MR, Bykovskaia S, Shogan J, Lotze MT, Barksdale EM Jr. Neuroblastoma-derived gangliosides inhibit dendritic cell generation and function. Cancer Res (2001) 61(1):363–9.
104. Pillai S, Netravali IA, Cariappa A, Mattoo H. Siglecs and immune regulation. Annu Rev Immunol (2012) 30:357–92. doi:10.1146/annurev-immunol-020711-075018
105. Ando M, Tu W, Nishijima K, Iijima S. Siglec-9 enhances IL-10 production in macrophages via tyrosine-based motifs. Biochem Biophys Res Commun (2008) 369(3):878–83. doi:10.1016/j.bbrc.2008.02.11a
Keywords: glycosylation, immunesurveillance, cancer immunology, immune evasion, tumor virulence
Citation: Nardy AFFR, Freire-de-Lima L, Freire-de-Lima CG and Morrot A (2016) The Sweet Side of Immune Evasion: Role of Glycans in the Mechanisms of Cancer Progression. Front. Oncol. 6:54. doi: 10.3389/fonc.2016.00054
Received: 20 November 2015; Accepted: 24 February 2016;
Published: 09 March 2016
Edited by:
Patrizia Agostinis, University of Leuven, BelgiumReviewed by:
Martin Herrmann, Universitätsklinikum Erlangen, GermanyGiovanna Schiavoni, Istituto Superiore di Sanita’, Italy
Copyright: © 2016 Nardy, Freire-de-Lima, Freire-de-Lima and Morrot. This is an open-access article distributed under the terms of the Creative Commons Attribution License (CC BY). The use, distribution or reproduction in other forums is permitted, provided the original author(s) or licensor are credited and that the original publication in this journal is cited, in accordance with accepted academic practice. No use, distribution or reproduction is permitted which does not comply with these terms.
*Correspondence: Célio Geraldo Freire-de-Lima, Y2VsaW8mI3gwMDA0MDtiaW9mLnVmcmouYnI=;
Alexandre Morrot, bW9ycm90JiN4MDAwNDA7bWljcm8udWZyai5icg==