- 1Center for Radiopharmaceutical Sciences, PSI Center for Life Sciences, Villigen PSI, Switzerland
- 2Radiopharmacy and Cyclotron Department, IRCCS Sacro Cuore Don Calabria, Negrar di Valpolicella, Italy
- 3PSI Center for Nuclear Engineering and Sciences, Villigen PSI, Switzerland
The interest in terbium radionuclides, which can be used in nuclear medicine, has increased tremendously over the last decade. Several research studies have shown the potential of four terbium radionuclides 149,152,155,161Tb both for cancer diagnosis as well as therapy. The comparison of 161Tb and 177Lu showed 161Tb as the preferred candidate not only for standard radiotherapy, but also for the treatment of minimal residual disease. Nevertheless, among the terbium sisters, currently, only 161Tb has an established production protocol where its no-carrier-added form is obtained via neutron irradiation of enriched 160Gd targets. The other terbium radioisotopes face challenges related to production capacity and production yield, which currently restricts their use in nuclear medicine. The purpose of this review is to report on recent research on the production and separation of terbium sisters and to assess the prospects for upscaling their production for nuclear medicine applications.
1 Introduction
According to World Health Organization, about one in five people develop cancer in their lifetime, while approximately one in nine men and one in 12 women die from the disease (1). The share of nuclear medicine in cancer treatment has seen significant growth in the last few decades. This progress is largely due to advancements in radiopharmaceutical delivery to cancer cells, enhancing both diagnosis and therapy. Contemporary radiopharmaceuticals with radiometals usually consist of the vector–chelator–radionuclide framework to offer flexibility in the choice of radionuclides. Currently, there are only a few radiometal-based radiopharmaceuticals that have been approved by medical authorities, namely, Lutathera [(177Lu)Lu-DOTA-TATE], Pluvicto [(177Lu)Lu-PSMA-617], Octreoscan [(111In)In-DTPA-pentetreotide], [67Ga]Ga-citrate, Netspot [(68Ga)Ga-DOTA-TATE], [68Ga]Ga-DOTA-TOC, Locametz/Illuccix [(68Ga)Ga-PSMA-11], Detectnet [(64Cu)Cu-DOTA-TATE], and Zevalin ([90Y]Y-ibritumomab-tiuxetan (2). However, there can be a much broader choice of radiometals, suitable for cancer treatment or diagnosis, according to their nuclear properties. Furthermore, radiometals’ application in nuclear medicine fosters innovative strategies (such as imaging, treatment with α- or Auger-electron emitters, and theragnostics) that could enhance the role of radiopharmaceuticals in cancer treatment and diagnosis.
The current trend in Radioligand Therapy (RLT) revolves around the use of 177Lu (T1/2 = 6.65 d), as a therapeutic β−-emitter, e.g., in radiopharmaceuticals such as Pluvicto and Lutathera (2). The use of this radiolanthanide in the clinical setting has encouraged research into other radiolanthanides/actinides that may have therapeutic capabilities. While 177Lu is currently seen as the most prominent radiometal in RLT, it does have its shortcomings, as its curative effect has not been as significant as expected (3). Particularly, the wide range of β−-particles in biological tissue—up to 12 mm—implies a low linear energy transfer (LET, ∼0.2 keV/µm), which might not effectively damage targeted cells (Figure 1). However, based on theoretical data, it was calculated that α-emitters are about two orders of magnitude more effective in killing cells (4). 149Tb, a potential therapeutic radionuclide due to an emission of α-particles, has a LET of 142 keV/µm, while the penetration range itself is only 28 µm (5). This indicates that the treatment can be more effective and that healthy cells around the tumour should suffer less. Thus, more attention is being focused on radionuclides with lower ranges of emitted particles and greater LET. Conversion/Auger electron (LET ∼20 keV/µm for 161Tb) and α-emitters are coming to the fore in production and nuclear medicine application possibilities (6).
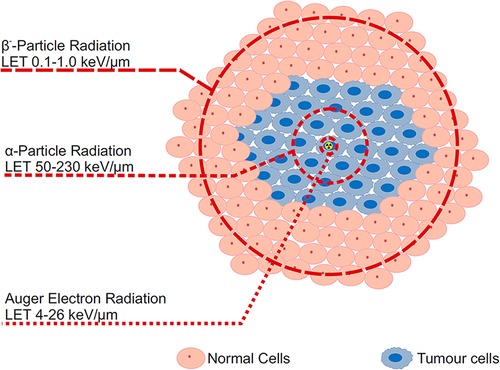
Figure 1. Illustration demonstrating linear energy transfer (LET) range of α-particle, β-particle, and Auger election radiation within tumour cells from a specific radioactive source (indicated by the radioactive symbol).
Delivering such short-range radiation to the cell nucleus seems to be essential, presenting several challenges in radiopharmaceutical delivery. However, it appears that the membrane is a more sensitive target than the cytoplasm for the dense ionization produced by Auger electrons (7). This indicates that, even in the absence of nuclear localization, the proximity of an Auger-electron emitter to the cancer cell membrane may still significantly enhance the therapeutic efficacy.
Another important aspect of radiometals is the synergistic properties of the radioisotopes, which can be effectively utilized in the theragnostic concept. It is particularly promising where diagnostic imaging and therapeutic interventions apply the same radioligand, emitting particles suitable both for therapy and diagnostic [= theragnostic (8)] purposes (9). For example, 149Tb can be applied in targeted α-therapy (TAT) and could potentially provide visualization by positron emission tomography/computed tomography (PET/CT), thus, enabling efficient dosimetry (10). In alternative scenarios, sets of radioisotopes are employed wherein one radionuclide fulfills a diagnostic function, while the other serves a therapeutic purpose. According to this principle, a triplet of radioisotopes, namely, 123I (EC), 124I (β+), and 131I (β−) is already actively used (11), and other sets, such as 43Sc (β+), 44Sc (β+), 47Sc (β−) and 61Cu(β+), 64Cu (β+ 61.5%, β− 38.5%), 67Cu (β−), are being actively studied. Another example would be diagnostic 152Tb for PET or 155Tb (T1/2 = 5.32 d, ε) for single photon emission computed tomography (SPECT) combined with 161Tb (T1/2 = 6.95 d, β− 100%, conversion and Auger-electrons) for therapy.
Terbium radioisotopes meet all the requirements of modern nuclear medicine, mentioned above. 149Tb exhibits theragnostic properties according to its nuclear data (with the possibility to use it for PET, as well as TAT). 152Tb has performed well in PET/CT imaging and has been applied in preclinical testing, as well as first-in-human applications (12–14). 155Tb has soft γ-lines similar to 99mTc, which allows it to be used in SPECT (15). 161Tb is the closest analogue of the very popular therapeutic β−-emitter 177Lu in terms of its nuclear and chemical properties; however, it is more effective due to the additional effect of conversion and Auger-electron emission, should it be applied with the correct targeting agent (16, 17). 161Tb and 155Tb can be obtained with simply achievable (n,γ) (18) and (p,n)/(p,2n)/(d,2n) nuclear reactions (Figure 2) (19, 20), respectively. 149Tb and 152Tb, however, are located further away from the stable nuclides in the nuclide chart; therefore, it is more challenging to produce them. Alternative methods may be used for their production, such as α-induced irradiations of europium targets (21, 22).
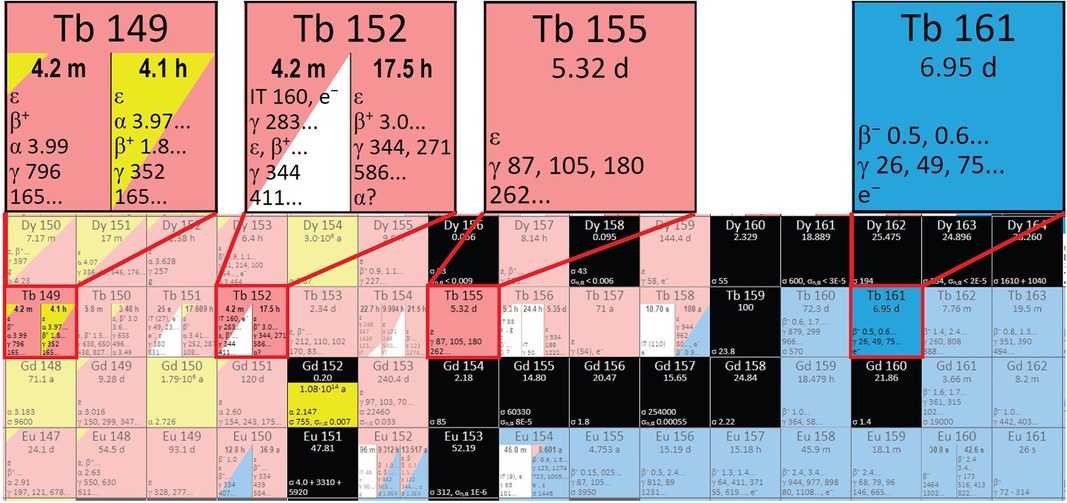
Figure 2. A section of the Karlsruhe nuclide chart showing the region containing the terbium sisters (adapted from www.nucleonica.com).
Despite the proven effectiveness of the terbium sisters (23) in animal experiments, their production is associated with serious limitations, thereby, preventing clinical studies. To date, only 161Tb can be produced in quantities and purity for clinical application, where its no-carrier-added (n.c.a.) form is obtained via neutron irradiation of enriched 160Gd targets (24). The process has recently been commercialized, indicating its potential for future application (25). The other terbium radioisotopes have significant challenges related to production capacity and production yield, requiring either ultra-enriched target materials (for example, >99% enriched 152Gd) or middle- and high-energy particle accelerators paired with Isotope Separation On-Line (ISOL) facilities, whose availability is limited. Only a few institutions worldwide have worked on new methods for terbium sisters’ production (Tables 1–4). Of those, 22 have published articles about terbium production in the last decade. The main results will be further discussed in this review.
2 Production of terbium radioisotopes at large research facilities around the world
2.1 Neutron irradiation
The most effective route to produce neutron-rich nuclides is the (n,γ) nuclear reaction thanks to their relatively high cross-sections and potential of utilizing a high neutron flux. However, to produce n.c.a. radionuclides, the use of an indirect production route, should one exist, is advantageous. An example of this type of method was explored and implemented in 161Tb production. The indirect route 160Gd(n,γ)161Gd → 161Tb is attractive due to its simplicity and product quality (30). The enriched 160Gd target is irradiated with neutrons, which induce 161Gd production (T1/2 = 3.66 min, β−) with a cross section of 1.4 b. 161Gd quickly decays into 161Tb and, upon completion of the separation of Tb from the gadolinium target, a high-quality product with radionuclidic purity (RNP) > 99.99% is obtained. The main impurity is 160Tb (T1/2 = 72.3 d, β−), which is produced by the activation of 159Tb, present as impurity in the target material. Initially, a collective of authors from the Technical University of Munich (TUM), the Paul Scherrer Institute (PSI), Institut Laue-Langevin (ILL), and University of Bern presented a possibility to produce up to 15 GBq (up to 80% of the available activity) after 14-day irradiation at BER II/FRM II of up to 40 mg 160Gd2O3 (98.2% 160Gd) and subsequent separation (30). 161Tb–DOTA-peptide could be prepared with >99% reaction yield by incubating 161Tb and DOTA-TATE, corresponding to a 161Tb-to-DOTA-TATE molar ratio of 1:12 (30). To date, several facilities have been producing 161Tb via this route (Table 1). Tens of GBq 161Tb were produced in the collaboration between ILL, the South African Nuclear Energy Corporation (NECSA), and PSI (24). The product was obtained at an activity concentration of 11–21 MBq/μl with ≥99% radionuclidic and radiochemical purity (24). Radiolabelling of PSMA-617 and other DOTA-based targeting compounds with 161Tb was achieved at molar activities up to 100 MBq/nmol at a radiochemical purity of ≥98%, demonstrating the high quality of the radionuclide (76). It has been stipulated that 7-day irradiation of 10 mg of 160Gd2O3 (98.2% 160Gd) targets at SCK.CEN's BR2 reactor typically produces 7–10 GBq of 161Tb (33). Radiolabelling of crown-αMSH with 161Tb was achieved at molar activities up to 144.9 MBq/nmol at a radiochemical purity ≥99% (33). Lately, 161Tb production has been presented by other research groups, such as the Center for Applied Nuclear Science and Technology—National Nuclear Energy Agency of Indonesia (BATAN), and Czech Technical University in Prague, FNSPE. The Indonesian group stated that a quartz ampoule with 5 mg of 160Gd2O3 target was irradiated with thermal neutron flux ∼1013 n·cm−2·s−1 during ∼3-day irradiation at the Bandung Triga 2,000 Reactor (37). Unfortunately, the 161Tb yield was not reported. The Czech group did not report it either after irradiations in the nuclear reactor LVR 15 (CV Řež) (36).
The 161Tb production process can be scaled up by increasing the target mass and aiming for higher neutron fluxes. However, the availability of high flux reactors is a critical point due to the shutdown of older reactors (for example, High Flux Beam Reactor and Brookhaven Medical Research Reactor at BNL), thus, requiring the construction of new ones to meet the growing demand for neutron-rich radionuclides. At least nine organizations produced 161Tb using the 160Gd(n,γ)161Gd → 161Tb production route (Table 1). There are likely more working on preclinical activities, however. It is expected to increase drastically over the next decade, due to the growing frequency of clinical studies involving 161Tb. According to ClinicalTrials.gov, there are three approved clinical trials at the time of writing (77–79).
2.2 Light-ion and photon-induced nuclear reactions
The most common proton and deuteron-induced reactions on gadolinium (natural and enriched) targets were explored (19, 26, 27, 39–41, 45–47, 50, 62–64). Generally, 152,155Tb production routes were studied, however, the 152Gd(p,4n)149Tb nuclear reaction was also investigated (45).
The most optimal reactions for 152Tb production are 152Gd(p,n) [42.8 kBq after 2 h irradiation with 12 MeV protons, at an average current of 0.9 μA (63)] and 155Gd(p,4n) [cross-section peak 900 mb at an energy of about 39 MeV (45)]. Despite promising results, they are complicated to realize. For example, the target material enriched in 152Gd is only commercially available as 30% enrichment due to its low natural abundance (0.20%). Nuclear reactions on the other gadolinium nuclides drastically decrease the RNP. When irradiating 155Gd with protons, there are two disadvantages. Firstly, the enrichment of available 155Gd target material does not exceed 93%. So, as with the 152Gd target, parallel reactions on the other gadolinium isotopes negatively affect the RNP. Secondly, the (p,4n) reaction is accompanied by (p,3n) reaction in the lower energies and (p,5n) reaction in the higher energies of protons. The contribution of these reactions can be varied, but in conjunction with the first disadvantage, it may require an electromagnetic isotope separation for nuclear medicine application of 152Tb (45).
Two proton-induced nuclear reactions for 155Tb production were studied at the Paul Scherrer Institute (Switzerland) and the Bern medical cyclotron (Inselspital University Hospital Bern), namely, 155Gd(p,n) and 156Gd(p,2n). In the case of 155Gd irradiation, the cross section presents a peak at around 11.5 MeV, corresponding to 447 mb, while in the case of 156Gd, the cross-section peak is about 1 b at 18 MeV (39). Production yields of 155Tb were calculated as well. The yield using the reaction 155Gd(p,n)155Tb, a target mass of ∼40 mg and beam entry energy ∼10.3 MeV equals 0.42 ± 0.26 MBq/µAh. Irradiations of 156Gd gave more promising results. The yield of the reaction 156Gd(p,2n)155Tb with target mass ∼40 mg and beam entry energy ∼22.8 MeV equals 3.28 ± 0.65 MBq/µAh (19). Unfortunately, 156Tb was produced as a radionuclidic impurity in both scenarios (6% for 155Gd irradiation and 8% for 156Gd irradiation). It is an inevitable impurity due to its comparable half-life (5.35 d) and target material enrichment. Supposedly, it will noticeably increase the dose to the patient due to its characteristic gamma-rays (1,065.1 keV 10.8%, 1,154.1 keV 10.4%, 1,222.4 keV 31%, 1,421.7 keV 12.2%, etc.). However, dosimetry calculations showed that the use of 155Gd enriched targets, containing 156Gd as impurity ≤2%, keeps the dose increase below the 10% threshold and ensures high-quality images (80).
A deuteron-induced reaction on 155Gd, enriched to 92.8%, for 155Tb production was recently performed at GIP ARRONAX cyclotron facility (20). The target material also contained 5.7% 156Gd. The cross-section peak for the reaction *Gd(d,x)155Tb is almost 800 mb at the deuteron energy ∼14.2 MeV. However, the cross-section of 156Tb production at the same point was 83 mb, and 154g/m1/m2Tb radionuclides were detected. As a result, maximum purity of 155Tb even after 14 days of cooling was not more than 89%. The production yield at the End of Bombardment (EoB) was determined to be 10.2 MBq/µAh at deuteron beam energy of 15.1 MeV, which may be enough for preclinical studies. It may have great potential if the 156Gd content of the enriched 155Gd target does not exceed 2% (80).
Alternatively, 155Tb can be produced using Tb4O7 targets via an indirect production route [natTb(p,5n)155Dy → 155Tb] (45, 81, 82). In this way, as a decay product of 155Dy, radionuclidically pure 155Tb can be produced (42, 43). 155Dy has a compatible half-life (9.9 h) and decays into 155Tb (Figure 3). Furthermore, neighbouring dysprosium nuclides do not decrease the purity of the product in the case of 155Tb separation from the Dy fraction.
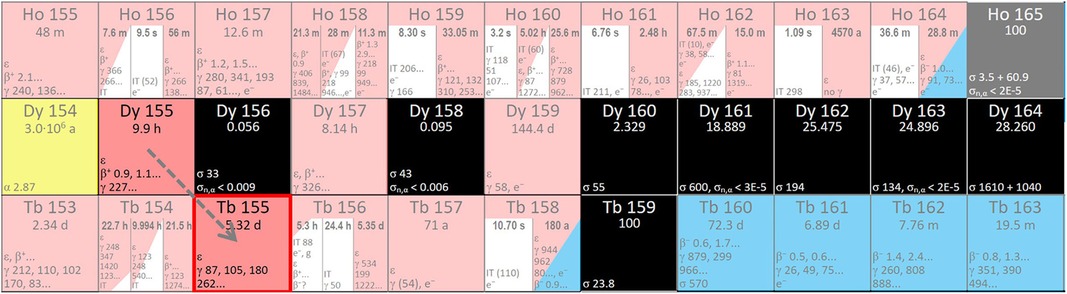
Figure 3. Region of the Karlsruhe nuclide chart displaying possible Dy to Tb nuclear reactions (www.nucleonica.com).
Several other indirect methods were researched, e.g., photon irradiation of 156Dy targets would lead to 155Tb production (58). However, the abundance of 156Dy in the natural material is only 0.056%, which means that the production of a monoisotopic 156Dy target is likely to be unaffordable. Also, the yield of the photonuclear reaction is 3.0 µCi/(µAh·g) e.g., 111 kBq/(µAh·g), so it is not enough for medical purposes.
Finally, promising results were presented by the Kurchatov Institute with irradiation of gadolinium targets with α-particles (55, 57). natGd and enriched 155Gd targets were irradiated with 60 MeV and 55 MeV α-particles, respectively, to reach the natGd(α,xn)155Dy → 155Tb and 155Gd(α,4n)155Dy → 155Tb nuclear reaction. In the case of the natural target, the thick target yield (TTY) of 155Tb was 3 MBq/μAh, and the terbium fraction had 94.6% RNP after two separation processes. The only impurity was 153Tb (5.4%), due to the parallel nuclear reactions on 152,154Gd, leading to the formation of 153Dy (T1/2 6.4 h, EC) and, as a result, 153Tb in the product (55, 56). Irradiation of enriched material allowed the production of 155Tb with RNP of >99.5% and the only radionuclidic impurity was 156Tb. However, the result can be improved to >99.99% through radiochemical separation, as the α,4n reaction on 156Gd yields stable 156Dy and no protons for the direct nuclear reaction are available to produce 156Tb. Any terbium impurities can be separated radiochemically in the initial separation step from 155Dy (57). The TTY of the reaction 155Gd(α,4n)155Dy → 155Tb (11.7 MBq/μAh) is comparable to the 156Gd(p,2n)155Tb reaction (12.7 MBq/µAh). However, an α-particle accelerator capable of reaching 40 MeV or higher is required.
Nuclear reactions induced by α-particles and 3He-particles may also be used for 149Tb and 152Tb production (21, 22). 149,152Tb were produced using both types of irradiations, but the reaction with 3He-particles resulted in a low TTY of 152Tb, and the cross section of the reaction 151Eu(α,6n)149Tb was not more than 10 mb in the investigated energy range. However, the excitation function of 151Eu(3He,5n)149Tb reaction reaches 70 mb at 47 MeV (21). Thus, for 8 h irradiation with a current of 20 μA, 3.4 GBq (EoB) of 149Tb would be produced. Radionuclidic impurities such as 150,151Tb would also be formed simultaneously. The optimal energy range was calculated according to an optimal ratio TTY/RNP to increase RNP, and irradiation of a 670 µm 151Eu2O3 target was proposed as a reasonable compromise. In this case, the output energy of 3He-particles would be ∼40 MeV, TTY of 149Tb 150 MBq/μA, and RNP ∼43%. The main radionuclidic impurity (∼100%) would be 150Tb (T1/2 = 3.48 h, EC β+ 100%), which may have no critical negative biological effects for the dose load due to its half-life, decay type, and γ-ray emission. The second radionuclidic impurity (28%), 151Tb (T1/2 = 17.609 h, EC β+ 100%), is undesirable due to its hard γ-ray emission. Nevertheless, the method can be useful due to the high 149Tb production yield, suitable at least for preclinical studies. Should one wish to pursue clinical application using this route, mass-separation techniques may need to be used.
In addition, 151Eu irradiation with α-particles can be an interesting route to produce 152Tb. Indeed, the cross-section peak of the 151Eu(α,3n)152Tb reaction reaches 800 mb, would make it possible to produce up to 8 GBq with an 8 h irradiation at a current of 20 μA (22). 151,153Tb radionuclides are also formed during the irradiation, but it is impossible to reduce their activity when irradiating in the maximum cross-section energy range. Thus, the optimal energy range was proposed as 42 → 34 MeV, with the TTY of 152Tb equal to 222 MBq/μA for 8 h irradiation. In this case, the total content of impurities would be less than 20%: ∼9% of 151Tb and ∼9% of 153Tb. However, 151Tb is an undesirable impurity and can be eliminated by decreasing the energy of incident α-particles. Due to the Q-value for the reaction 151Eu(α,4n)151Tb, equal to −32.89 MeV, it is possible to avoid 151Tb production with α-particles with energy <35 MeV. As a result, the only radionuclidic impurity would be 153Tb (T1/2 = 2.34 d, EC β+ 100%) with only one high-intensity γ-ray line at 212.00 keV (28%). However, its influence on biological studies requires further research. In addition, using the abovementioned conditions, the TTY of 152Tb would decrease from 65.7 MBq/μAh to ∼30 MBq/μAh.
2.3 Heavy-ion nuclear reactions
Heavy-ion (7Li, 10,11B, 12C, 14,15N, 16,18O, 19F, 20,22Ne) reactions provide means to access radionuclides that are located far from the stability line. Thus, this method can be suitable for 149Tb and, to a lesser extent, for 152Tb production.
Over the years, five institutes explored 149,152Tb production from target irradiation with heavy ions (67, 68, 73, 75, 83–88). However, the interest in such research faded in the 2000s. The main reason was due to the low yield and purity of the product, as well as the complex radiochemical separation process necessary. The last publication covering this type of nuclear reaction was produced more than a decade ago (88), while other attempts to isolate Tb from targets irradiated with heavy ions (published more than 20 years ago) were not fully successful (68, 73).
The initial goal of the earlier studies was only to investigate physical aspects of the formation of the ground and metastable states, since 149mTb does not decay into 149Tb. Thus, the ground state 149gTb can only be formed as a result of a direct nuclear reaction, and individual cross section thereof can be calculated and compared with theoretical data. Numerous reaction cross sections were presented for 10,11B, 12C, 14,15N, 16,18O, 19F, 20,22Ne beams in the energy range from 150 MeV to 35 MeV on target materials ranging from Ba to Nd (75, 83, 84). According to the results, all the reactions M(HI,xn)149,152Tb studied, where M is the target material, HI are heavy ions, yield low activities of 149gTb. The largest value of cross section for the formation of 149gTb turned out to be in the 142Nd(11B,4n)149gTb nuclear reaction, which resulted in ∼58 mb at an average energy of 55.2 MeV 11B ions (75), much lower than that theoretically calculated. In contrast, the excitation function of 149mTb (T1/2 = 4.16 min) seems to be much larger than that of the ground state for the same nuclear reaction on Nd. It was also noted that the ratio of the probability of formation of 149mTb to 149gTb increases very quickly with increasing energy. Therefore, it can be concluded that 149mTb has a higher spin than 149gTb, and an isomeric transition is unlikely to happen (75). Although direct reactions of 149gTb production with heavy ions have a low yield, an indirect route via 149Dy is of interest. 149Dy (T1/2 = 4.20 min) decays into 149Tb, and cross sections for the formation of 149Dy with the participation of heavy ions are much higher than for 149Tb (85). The reaction cross section for the formation of 149Tb from 142Nd reaches 446 mb at an incident particle energy of 97 MeV (67). It would allow the production of 15-30 GBq 149Tb from enriched targets of 142Nd (∼60 mg/cm2 thick) after 8–10 h bombardment with 12C ions at an energy of 120 MeV and an intensity of 50–100 μA (73, 86, 87).
On the other hand, regarding 152Tb, an irradiation of a thick natNd target with 12C nuclei at an energy of 85 MeV for 15.3 h would allow production of 100 MBq 152Dy at EoB (68). The RNP of the daughter radionuclide 152Tb should be high enough for biological studies, however, that was not measured. 144Sm targets have also been irradiated with 7Li ions to produce 152Tb, but the cross-section peak was only 45 mb (88).
2.4 Spallation reaction
The spallation reaction by itself cannot produce a pure mononuclidic product. However, when used in combination with ISOL techniques, 149Tb and its parent radionuclide 149Dy (T1/2 4.20 min) can be produced by spallation of suitable target with high cross-section (70). The proton-induced spallation reaction occurs with high-energy protons on the desired target material. The spallation products produced (a vast quantity of nuclides) are released and extracted at high temperature (over 2,000°C). The resultant ion beam is accelerated and mass-separated online in a magnetic sector field and the desired mass number collected by implantation into a foil (the ISOL technique). The desired radionuclide is subsequently chemically separated from its isobars and impurities. In addition, diagnostic 152,155Tb can be produced with this method as well. For this purpose, cyclotrons accelerating protons to hundreds of MeV energies are required.
The first attempts to produce 149Tb using a spallation reaction were carried out in the 1960s (72, 89–91) and continued for the next five decades (71, 92). Tantalum, gold, and bismuth targets were irradiated with high-energy protons (up to 30 GeV). The production of 149Tb was used as a monitor reaction to estimate the proton beam current due to easily identified α-particles of 149Tb. A rapid increase in the cross section of this reaction on a gold target in the energy range of 0.2–0.5 GeV was shown (90). It peaks at 1.3–2 GeV (70) and then up to 30 GeV, the cross section of this reaction decreases slightly (72, 89). The cross-section peak (∼19 mb) of the spallation reaction on a tantalum target is reached at a proton energy of 1–1.7 GeV, and on a bismuth target—2.0–3.2 GeV (∼10.5 mb) (72). Thus, by the time nuclear medicine became interested in terbium radionuclides (5, 31), the production of 149,152,155Tb had already been demonstrated at ISOLDE/CERN, Switzerland, using spallation reactions on tantalum target (12, 15, 93). The spallation method is very effective, but so far, there are few large facilities built for this purpose. However, recently, successful 155Tb production at the ISAC facility (TRIUMF, Canada) was published (94), while new facilities in Belgium (ISOL@MYRRHA), Switzerland (IMPACT-TATTOOS), and Japan (J-PARC ISOL) have been announced for launch over the next decade (10).
3 Separation of terbium sisters from irradiated target
Due to the numerous options for producing terbium radioisotopes, methods to isolate them from various lanthanides, such as lanthanum, cerium, praseodymium, and neodymium, as well as europium, gadolinium, and dysprosium have been reported.
In the past, liquid-liquid extraction was proposed to separate terbium isotopes from a lanthanum or cerium target irradiated with 16O nuclei, as well as from a neodymium target irradiated with 12C nuclei (66, 95, 96). A solution, containing the target dissolved in HNO3 + H2SO4, was evaporated and redissolved in HCl. A hydrochloric acid solution was equilibrated with a solution of di(2-ethylhexyl)phosphoric acid (HDEHP) in cyclohexane. Separation factors with different concentrations of HDEHP and HCl were studied. The optimal concentrations for the three systems are shown in Table 5.
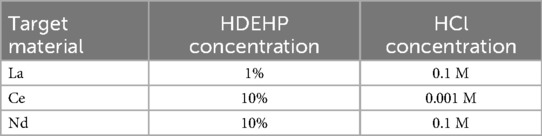
Table 5. Conditions for liquid-liquid extraction of terbium from La, Ce, and Nd targets (66, 95, 96).
About 70% of the terbium fraction transitioned into the organic phase in one cycle, completely retaining the macro amount of target material in the aqueous phase. A high separation factor (∼800) was achieved for the lanthanum/terbium system. However, solvent extraction is less effective for the separation of neighbouring lanthanides, as it typically requires multiple stages to achieve separation. As a result, cerium/terbium and neodymium/terbium separation were complicated by the presence of dysprosium isotopes (lanthanide adjacent to terbium), thus, requiring additional separation. Multiple extraction and back-extraction of dysprosium into the aqueous phase in a 10% HDEHP/1.5 M HCl system allowed the terbium fraction to be isolated in the organic phase (with 10% loss of terbium re-extracted into the aqueous phase along with dysprosium). A complete re-extraction of terbium fraction from the organic phase was possible with an aqueous solution of 1 M HCl. Reverse recovery of lanthanum radioisotopes has been studied using DTPA and EDTA solutions so that they could be directly applied in vivo without further processing (95). The need for repeated back and forth extractions to separate neighbouring lanthanides by liquid-liquid extraction make this approach inefficient. Subsequently, extraction resins were developed and have been used for this process to improve on separation efficiency.
Nowadays, the most useful method for lanthanide separation is by cation exchange chromatography, as strong cation exchange resins are specifically helpful for separating neighboring lanthanide pairs. Cation exchange resins operate by exchanging positively charged lanthanide ions with cations on the resin, where smaller, heavier lanthanides with higher charge densities are exchanged and eluted earlier due to stronger electrostatic interactions. For example, a separation of terbium fraction from irradiated neodymium target was provided using a chromatographic column filled with KU-2 or DOWEX 50 cation exchange resin (a copolymer of sulfonated divinylbenzene and styrene) (73). Before the elution of terbium, the column was saturated with 1.2 M NH4Cl. Terbium was eluted from the column with α-hydroxyisobutyric acid (α-HIBA), with yield >90%.
A similar method was proposed for a separation of 149Tb from the mix of isobars collected after mass separation of a tantalum target irradiated with protons (87). The solution containing 149 isobars included 149Eu, 149Gd, 149Tb—as well as pseudo-isobars 133La and 133Ce collected as 133LaO+ and 133CeO+. All lanthanides in chloride form were separated on a column, filled with AMINEX A5 cation exchange resin (KU-2 analogue) and afterwards eluted with α-HIBA at pH 5.0 using a concentration gradient, resulting in a terbium elution yield exceeding 90%.
Unfortunately, the AMINEX A5 and A6 resins are not produced anymore, but Bio-Rad has replaced them with alternatives in limited supply. Other strong cation exchange resins may be used to separate lanthanides (14, 24). An extraction chromatographic separation method on LN resin was proposed for the production of 161Tb as an alternative to cation exchange resins (37, 38, 97, 98). Commercially available LN Series Extractants (Triskem, Eichrom) based on HDEHP were deemed suitable for lanthanide separation. Unlike cation exchange resins, elements with smaller atomic numbers are washed off from LN resin earlier than those with greater atomic numbers. This is due to the extraction mechanism of the LN resin, where heavier lanthanides, possessing smaller ionic radii and higher charge densities, form more stable complexes with the extractant ligands on the resin, resulting in delayed elution. Therefore, in the gadolinium/terbium system, the gadolinium fraction is washed off earlier than the terbium fraction. This can affect the purity of terbium fraction, as the long “tail” of the gadolinium elution profile may overlap with that of terbium, especially when considering upscaling the method to handle larger gadolinium targets. Nevertheless, the terbium/gadolinium separation factor was demonstrated to be the highest for lanthanide pairs and the method can be implemented for terbium production. LN resin was also used in a two-step separation of terbium from gadolinium targets irradiated with α-particles (55, 57), where a dysprosium/gadolinium separation was conducted, followed by a dysprosium/terbium separation (after decay of 155Dy into 155Tb). In particular, the 155Dy produced by the irradiation was separated from the gadolinium target, followed by the isolation of 155Tb obtained from 155Dy decay 40 h later. When irradiating enriched (90.4%) 155Gd, a less efficient dysprosium/terbium separation was observed, which led to <0.3% 156Tb in the 155Tb fraction. In another work, the separation yield of terbium after isolation from a 20 mg dysprosium target with LN resin yielded only 39% separation efficiency (29). These discrepancies showcase that optimal parameters for terbium/dysprosium separation still need to be devised. In addition, LN3 resin was proposed for post-purification and concentration of terbium fraction in 0.05 M HCl (19).
Later, a study on 161Tb radiochemical separation with the more recently developed TK211 and TK212 resins was published (33). These resins are similar to LN resins but use mixed organophosphoric, organophosphonic and organophosphinic acid extractants that may work in synergy to improve selectivity (99). Using these types of resins, it was possible to separate 161Tb and Dy from the gadolinium fraction on TK212 resin and then isolate the Tb fraction on TK211 resin. Moreover, a semi-automated module for the proposed separation system was built. Quality controls on the terbium fractions showed nanogram levels of gadolinium, however, that did not affect terbium labelling capabilities. This could be used for the reprocessing of aged/partly decayed 161Tb by separating it from its stable daughter (161Dy), which interferes with apparent molar activity and, as a result, chemical purity.
In conclusion, there are currently three methods for the selective isolation of terbium from irradiated lanthanide targets and their decay products (Table 6).
The authors believe that the main problems for terbium sisters’ production nowadays include the poor availability of separation components (especially chromatographic resins with suitable specifications), as well as the limited supply of enriched target material. HDEHP-based resins are expensive and are being produced by few manufacturers. Moreover, extraction resins also have a limited loading capacity. The physical impregnation of the organic layer onto the solid support limits the lifetime of using these resins for neighbouring lanthanide separations. Therefore, given the potential of the terbium sisters in the field, it is of paramount importance to increase the availability of resins and separation method possibilities for terbium radioisotope production.
4 Opportunities and potential challenges of using terbium sisters
The current production situation for terbium sisters is summarized in Figure 4.
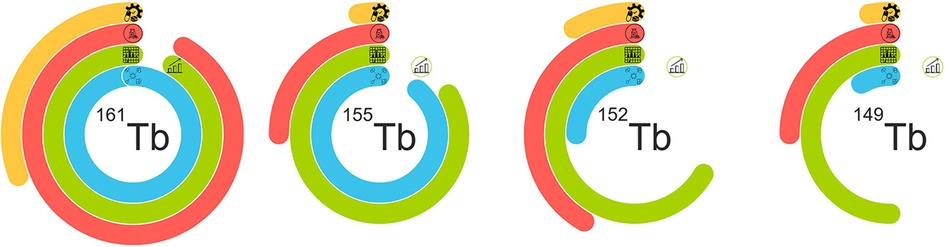
Figure 4. Representation of the current situation of terbium sisters’ production. Four rings symbolize the four major phases of radiopharmaceuticals implementation: the blue line shows cyclotron/reactor production situation, the green line represent separation process readiness and includes problems with the scale-up process at its last bend, the red line shows preclinical studies, and the yellow line–GMP development and clinical implementation.
4.1 Terbium-161
A lot of work has been done towards 161Tb production since its initial publication (30) over a decade ago (Figure 4). Nevertheless, there are still considerable tasks remaining to be addressed. The most optimal way to produce 161Tb is via indirect method 160Gd(n,γ)161Gd→161Tb. The main challenge of this method is the chemical purity of the target material, as it needs to contain less than 5 ppm of 159Tb (30) in order to decrease 160Tb production via the 159Tb(n,γ)160Tb nuclear reaction and ensure that the radionuclidic and radiochemical purity of the resulting 161Tb exceeds 99% (24, 37). Another challenge to be faced in the long term is the availability of highly enriched target material, since the chains of nuclear reactions 158Gd(n,γ)159Gd → 159Tb(n,γ)160Tb and 157Gd(n,γ)158Gd(n,γ)159Gd → 159Tb(n,γ)160Tb are also contributing to the final content 160Tb in the final product. Three successful separation methods were developed: using macroporous cation exchange resin [up to 140 mg of 160Gd2O3 (24)], using LN resin [up to 5 mg of 160Gd2O3 (38)], and using TK212/TK211 resins [up to 10 mg of Gd2O3 (33)]. Currently, the primary focus for 161Tb revolves around its medical applications. Clinical trials should be conducted to benchmark it against 177Lu. In the meantime, 161Tb production must be upscaled to provide enough activity for clinical studies. For that, a few changes in the current separation process should be implemented (for example, a lengthy evaporation process should be avoided to optimize production time). To date, the first studies in humans with radiopharmaceuticals based on 161Tb were started at the Universitätsspital Basel, Switzerland (79, 100), Saarland University, Medical Centre, Germany (101), King Hussein Cancer Centre, Jordan (102), and Peter MacCallum Cancer Centre, Australia (78).
4.2 Terbium-155
Terbium-155 has several promising methods for its production and separation, but they have yet to be improved. However, it is assumed that the medical implementation of other terbium sisters might occur much faster than 161Tb due to the identical chemical properties of all terbium radionuclides (31, 34). Nowadays, two methods are mainly being used for 155Tb production. The first is the spallation reaction on a tantalum target followed by ISOL separation. This method was realized by CERN and TRIUMF at the scale of hundreds of MBq (31, 34). Furthermore, radiochemical separation of 155Tb from isobars is required. Despite the consumption of a lot of energy and materials this process could provide the activity only for preclinical studies and cannot be implemented in routine medical cycles. Another method is proton irradiation of gadolinium targets enriched in 155Gd or 156Gd (19, 39). The 155Gd(p,n) reaction gives a purer product (RNP up to 94%), but the yield of the reaction is twice lower than the 156Gd(p,2n) reaction (RNP up to 92%). The recently studied 155Gd(d,2n)155Tb nuclear reaction may become the third method for 155Tb production (RNP up to 89%) (20). In all these scenarios, however, the produced impurity is 156Tb—an undesirable radionuclide for nuclear medicine which has a comparable half-life to 155Tb (5.35 days) and could noticeably increase the dose to patient due to abundant hard γ-lines. To prevent the presence of 156Tb in the 155Tb solution, an ultra-pure 155Gd or 156Gd target may be used, which drastically affects the material cost.
Otherwise, two indirect methods via 155Dy production were proposed (45, 55). The idea of indirect production of 155Tb is being actively studied at the PSI and the Kurchatov Institute (42, 55, 57). These two institutes have different large facilities, so the production methods will differ. However, both are based on the production of 155Dy, followed by its quick separation from the target material and final isolation of 155Tb after 155Dy decay. The radiochemical separation technique proposed by the Kurchatov Institute (separation of 155Dy from 155Gd targets irradiated with α-particles, and then 155Tb from the 155Dy fraction) should be simpler than separation of 155Dy from natTb, but in this case, an α-accelerator is required. Proton accelerators have better distribution, the yield of 155Tb should be ten times higher in the case of proton irradiation, and the use of natural material more convenient. Unfortunately, the separation of terbium and dysprosium appears to present greater challenges. As a result, the optimal production and separation methods for 155Tb are still under development.
In conclusion, increased investment in large-scale facilities with the necessary capabilities to conduct these reactions is crucial for advancing the study of promising indirect production routes. Such infrastructure would enable higher yields and more efficient production processes. Additionally, the radiochemical separation between terbium and dysprosium should be deeply investigated, as this separation is more challenging compared to the well-documented terbium/gadolinium separation. Addressing this challenge is essential for improving the production purity of 155Tb. Furthermore, the successful clinical application of ¹⁵⁵Tb will rely not only on optimized production and separation techniques but also on a comprehensive understanding of radiation effects and precise dosimetry for both patients and healthcare personnel. These factors must be rigorously evaluated to ensure the safe and effective use of ¹⁵⁵Tb in medical applications.
4.3 Terbium-152
Medical implementation of 152Tb was studied better than 155Tb, due to the urgent need for additional β+-emitters (13). 152Tb can be produced via the spallation reaction and the ISOL collection method (31), or the 152Gd(p,n) reaction (63). Unfortunately, the available enrichment of 152Gd (30% maximum) is too low to ensure a radionuclidically pure product. Therefore, 152Tb's success will depend on ISOL facilities being constructed in the future as nowadays, this production method is very limited. In this context it is expected that 152Tb production will see significant advances at the PSI with the TATTOOS development (Targeted Alpha Tumour Therapy and Other Oncological Solutions, https://www.psi.ch/en/impact/tattoos) as part of the upgrade of the High-Intensity Proton Accelerator complex HIPA which plays a pivotal role in Swiss large-scale infrastructure. HIPA's Ring Cyclotron is the most powerful proton cyclotron worldwide and among the most energy-efficient accelerators. It provides a world-leading 1.4 MW high-intensity proton beam of 590 MeV energy and up to 2.4 mA of beam current to target stations. According to the calculated data, 12 h irradiation of a tantalum target on TATTOOS (100 μA, 590 MeV proton beam) gives 625 GBq of 152Tb (103). Due to the 50-fold higher proton beam intensity foreseen at TATTOOS (100 μA) compared to ISOLDE/CERN (2 μA), this corresponds to an at least 50-fold increase compared to ISOLDE's production capacity, under consideration of all extraction and adsorption losses as well as losses during ionization and ion transport. A similar setup is planned at ISOL@MYRRA (Belgium, https://myrrha.be/myrrha-applications/nuclear-science/isolmyrrha), which will allow for extended preclinical and clinical studies. The ISOL method will allow to use of the same radiochemical separation system, that already was proposed for 152Tb isolation from isobars (12, 13). As for other terbium radionuclides collected with isobars, cation exchange chromatography is a rapid and effective way to isolate them.
4.4 Terbium-149
Finally, great interest in 149Tb has been shown by nuclear medicine physicians, due to its alpha emission (104). Its production and separation are provided with the same methods, as for 152Tb. Unfortunately, the production of 149Tb is the most challenging, as there are no known nuclear reactions capable of yielding a radionuclidically pure product. As a result, offline mass-separation or ISOL techniques are inevitable, but the production yield should be improved, which, as mentioned above, should be feasible in facilities with higher currents such as TATTOOS or ISOL@MYRRHA. The calculated production yield of 149Tb as obtained from TATTOOS using a tantalum (12 h irradiation) target gives 2,390 GBq of 149Tb. The radiochemical separation method from isobars was also previously reported and based on cation exchange resins (31, 70). Although the therapeutic activity has yet to be established for 149Tb-based preparations, one can estimate it from other alpha emitters. For 213Bi this value is 10–50 MBq/kg; for 225Ac—20–150 kBq/kg, for 223Ra–55 kBq/kg, and for 212Pb, a parent of 212Bi,—200–500 kBq/kg (105). It can be assumed that the therapeutic activity of 149Tb can be 10–55 MBq/kg, according to its decay characteristics (α 16.7%, T1/2 = 4.118 h). Therefore, the activity produced with the abovementioned facilities would be more than enough for clinical application and even adequate distribution.
5 Conclusions & outlook
Discussions regarding the theragnostic potential of terbium sisters have persisted for over a decade. Nonetheless, significant progress has been achieved only with one terbium radionuclide, 161Tb. This is due mainly to difficulties associated with producing other terbium sisters with high RNP. 155Tb appears to be the most prospective diagnostic pair for 161Tb for the next decade due to more realistic production methods. Production of 152Tb and 149Tb requires the implementation of large facilities such as TATTOOS or ISOL@MYRRHA to ensure sufficient activity and RNP for medical purposes. Thus, large investments in such facilities are required, which are highly dependent on governmental funds and subsidies. Improved commercial availability of separation resins would expedite the development process and facilitate wider access to terbium worldwide. Therefore, in conclusion, significant investments should be directed towards large-scale facilities capable of producing such radionuclides in quantities suitable for clinical use and distribution. In this regard, collaborations of research centers, which has been initiated by The European medical radionuclides program (https://www.prismap.eu/), could be another valuable option to increase the availability of the terbium sisters for preclinical and clinical studies.
Author contributions
AM: Data curation, Investigation, Visualization, Writing – original draft, Writing – review & editing. CF: Formal Analysis, Validation, Writing – review & editing. ZT: Conceptualization, Methodology, Writing – review & editing. PG: Data curation, Software, Writing – review & editing. NvdM: Project administration, Resources, Supervision, Writing – original draft, Writing – review & editing.
Funding
The author(s) declare financial support was received for the research, authorship, and/or publication of this article. Open access funding by PSI - Paul Scherrer Institute.
Conflict of interest
Author AM, CF, PG, and NvdM were employed by PSI has an agreement with a commercial entity with regard to upscale of Tb-161 production, however, this is not relevant for this review.
The remaining author declares that the research was conducted in the absence of any commercial or financial relationships that could be construed as a potential conflict of interest.
The reviewer MVDV declared a past co-authorship with the authors ZT and NvdM to the handling editor.
Publisher's note
All claims expressed in this article are solely those of the authors and do not necessarily represent those of their affiliated organizations, or those of the publisher, the editors and the reviewers. Any product that may be evaluated in this article, or claim that may be made by its manufacturer, is not guaranteed or endorsed by the publisher.
References
1. World Health Organization. Global Cancer Burden Growing, Amidst Mounting Need for Services. (2024). Available online at: https://www.who.int/news/item/01-02-2024-global-cancer-burden-growing–amidst-mounting-need-for-services (Accessed September 13, 2024).
2. Cardinal Health. Medication Management FDA-Approved Radiopharmaceuticals. (2023). Available online at: https://www.cardinalhealth.com/content/dam/corp/web/documents/fact-sheet/cardinal-health-fda-approved-radiopharmaceuticals.pdf (Accessed September 13, 2024).
3. McDevitt MR, Sgouros G, Finn RD, Humm JL, Jurcic JG, Larson SM, et al. Radioimmunotherapy with alpha-emitting nuclides. Eur J Nucl Med. (1998) 25:1341–51. doi: 10.1007/s002590050306
4. Allen B. Targeted alpha therapy: evidence for potential efficacy of alpha-immunoconjugates in the management of micrometastatic cancer. Australas Radiol. (1999) 43:480–6. doi: 10.1046/j.1440-1673.1999.00717.x
5. Allen BJ, Blagojevic N. Alpha- and beta-emitting radiolanthanides in targeted cancer therapy. Nucl Med Commun. (1996) 17:40–7. doi: 10.1097/00006231-199601000-00008
6. Filosofov D, Kurakina E, Radchenko V. Potent candidates for targeted auger therapy: production and radiochemical considerations. Nucl Med Biol. (2021) 94–95:1–19. doi: 10.1016/j.nucmedbio.2020.12.001
7. Pouget JP, Santoro L, Raymond L, Chouin N, Bardiès M, Bascoul-Mollevi C, et al. Cell membrane is a more sensitive target than cytoplasm to dense ionization produced by auger electrons. Radiat Res. (2008) 170:192–200. doi: 10.1667/RR1359.1
8. Frangos S, Buscombe JR. Why should we be concerned about a “g”? Eur J Nucl Med Mol Imaging. (2019) 46:519. doi: 10.1007/s00259-018-4204-z
9. Bentzen SM. Theragnostic imaging for radiation oncology: dose-painting by numbers. Lancet Oncol. (2005) 6:112–7. doi: 10.1016/S1470-2045(05)01737-7
10. Müller C, Vermeulen C, Köster U, Johnston K, Türler A, Schibli R, et al. Alpha-PET with terbium-149: evidence and perspectives for radiotheragnostics. EJNMMI Radiopharm Chem. (2016) 1:2–6. doi: 10.1186/s41181-016-0008-2
11. Silberstein EB. Radioiodine: the classic theranostic agent. Semin Nucl Med. (2012) 42:164–70. doi: 10.1053/j.semnuclmed.2011.12.002
12. Müller C, Vermeulen C, Johnston K, Köster U, Schmid R, Türler A, et al. Preclinical in vivo application of 152Tb-DOTANOC: a radiolanthanide for PET imaging. EJNMMI Res. (2016) 6:35. doi: 10.1186/s13550-016-0189-4
13. Baum RP, Singh A, Benešová M, Vermeulen C, Gnesin S, Köster U, et al. Clinical evaluation of the radiolanthanide terbium-152: first-in-human PET/CT with 152Tb-DOTATOC. Dalt Trans. (2017) 46:14638–46. doi: 10.1039/c7dt01936j
14. Müller C, Singh A, Umbricht CA, Kulkarni HR, Johnston K, Benešová M, et al. Preclinical investigations and first-in-human application of 152Tb-PSMA-617 for PET/CT imaging of prostate cancer. EJNMMI Res. (2019) 9:68. doi: 10.1186/s13550-019-0538-1
15. Müller C, Fischer E, Behe M, Köster U, Dorrer H, Reber J, et al. Future prospects for SPECT imaging using the radiolanthanide terbium-155—production and preclinical evaluation in tumor-bearing mice. Nucl Med Biol. (2014) 41:58–65. doi: 10.1016/j.nucmedbio.2013.11.002
16. Müller C, Reber J, Haller S, Dorrer H, Bernhardt P, Zhernosekov K, et al. Direct in vitro and in vivo comparison of 161Tb and 177Lu using a tumour-targeting folate conjugate. Eur J Nucl Med Mol Imaging. (2014) 41:476–85. doi: 10.1007/s00259-013-2563-z
17. Borgna F, Barritt P, Grundler P V, Talip Z, Cohrs S, Zeevaart JR, et al. Simultaneous visualization of 161Tb-and 177Lu-labeled somatostatin analogues using dual-isotope SPECT imaging. Pharmaceutics. (2021) 13:1–13. doi: 10.3390/pharmaceutics13040536
18. Gracheva N, Müller C, Talip Z, Heinitz S, Köster U, Zeevaart JR, et al. Production and characterization of no-carrier-added 161Tb as an alternative to the clinically-applied 177Lu for radionuclide therapy. EJNMMI Radiopharm Chem. (2019) 4:1–16. doi: 10.1186/s41181-019-0063-6
19. Favaretto C, Talip Z, Borgna F, Grundler P V, Dellepiane G, Sommerhalder A, et al. Cyclotron production and radiochemical purification of terbium-155 for SPECT imaging. EJNMMI Radiopharm Chem. (2021) 6:37. doi: 10.1186/s41181-021-00153-w
20. Wang Y, Sounalet T, Guertin A, Nigron E, Michel N, Haddad F. Study of terbium production from enriched Gd targets via the reaction 155Gd(d,2n)155Tb. Appl Radiat Isot. (2023) 201:110996. doi: 10.1016/j.apradiso.2023.110996
21. Moiseeva AN, Aliev RA, Unezhev VN, Zagryadskiy VA, Latushkin ST, Aksenov N V, et al. Cross section measurements of 151Eu(3He,5n) reaction: new opportunities for medical alpha emitter 149Tb production. Sci Rep. (2020) 10:1–7. doi: 10.1038/s41598-020-57436-6
22. Moiseeva AN, Aliev RA, Unezhev VN, Gustova NS, Madumarov AS, Aksenov NV, et al. Alpha particle induced reactions on 151Eu: possibility of production of 152Tb radioisotope for PET imaging. Nucl Instruments Methods Phys Res Sect B Beam Interact with Mater Atoms. (2021) 497:59–64. doi: 10.1016/j.nimb.2021.04.007
23. Müller C, van der Meulen NP. Terbium “sisters”: more than just a ‘Swiss army knife. In: Prasad V, editor. Beyond Becquerel and Biology to Precision Radiomolecular Oncology: Festschrift in Honor of Richard P. Baum. Cham: Springer International Publishing (2024). p. 225–36. doi: 10.1007/978-3-031-33533-4_23.
24. Gracheva N, Müller C, Talip Z, Heinitz S, Köster U, Zeevaart JR, et al. Production and characterization of no-carrier-added 161Tb as an alternative to the clinically-applied 177Lu for radionuclide therapy. EJNMMI Radiopharm Chem. (2019) 4:12. doi: 10.1186/s41181-019-0063-6
25. Müller C, van der Meulen NP, Schibli R. Opportunities and potential challenges of using terbium-161 for targeted radionuclide therapy in clinics. Eur J Nucl Med Mol Imaging. (2023) 50:3181–4. doi: 10.1007/s00259-023-06316-y
26. Tárkányi F, Takács S, Ditrói F, Csikai J, Hermanne A, Ignatyuk AV. Activation cross-sections of deuteron induced reactions on natGd up to 50 MeV. Appl Radiat Isot. (2014) 83:25–35. doi: 10.1016/j.apradiso.2013.10.010
27. Szelecsényi F, Kovács Z, Nagatsu K, Zhang M-R, Suzuki K. Investigation of deuteron-induced reactions on natGd up to 30 MeV: possibility of production of medically relevant 155Tb and 161Tb radioisotopes. J Radioanal Nucl Chem. (2016) 307:1877–81. doi: 10.1007/s10967-015-4528-0
28. Tárkányi F, Hermanne A, Takács S, Ditrói F, Csikai J, Ignatyuk AV. Cross-section measurement of some deuteron induced reactions on 160Gd for possible production of the therapeutic radionuclide 161Tb. J Radioanal Nucl Chem. (2013) 298:1385–92. doi: 10.1007/s10967-013-2507-x
29. Fedotova AR, Egorova BV, Kormazeva S, Konevega L, Belyshev SS, Khankin VV, et al. Photonuclear production of medical radioisotopes 161Tb and 155Tb. Appl Radiat Isot. (2023) 198:110840. doi: 10.1016/j.apradiso.2023.110840
30. Lehenberger S, Barkhausen C, Cohrs S, Fischer E, Grünberg J, Hohn A, et al. The low-energy β—and electron emitter 161Tb as an alternative to 177Lu for targeted radionuclide therapy. Nucl Med Biol. (2011) 38:917–24. doi: 10.1016/j.nucmedbio.2011.02.007
31. Muller C, Zhernosekov K, Koster U, Johnston K, Dorrer H, Hohn A, et al. A unique matched quadruplet of terbium radioisotopes for PET and SPECT and for α- and β−-radionuclide therapy: an in vivo proof-of-concept study with a new receptor-targeted folate derivative. J Nucl Med. (2012) 53:1951–9. doi: 10.2967/jnumed.112.107540
32. Favaretto C, Grundler PV, Talip Z, Landolt S, Sepini L, Köster U, et al. 161Tb-DOTATOC production using a fully automated disposable cassette system: a first step toward the introduction of 161Tb into the clinic. J Nucl Med. (2023) 64:1138–44. doi: 10.2967/jnumed.122.265268
33. McNeil SW, Van de Voorde M, Zhang C, Ooms M, Bénard F, Radchenko V, et al. A simple and automated method for 161Tb purification and ICP-MS analysis of 161Tb. EJNMMI Radiopharm Chem. (2022) 7:31. doi: 10.1186/s41181-022-00183-y
34. Wharton L, McNeil SW, Merkens H, Yuan Z, Van de Voorde M, Engudar G, et al. Preclinical evaluation of [155/161Tb]Tb-Crown-TATE—a novel SPECT imaging theranostic agent targeting neuroendocrine tumours. Molecules. (2023) 28:3155. doi: 10.3390/molecules28073155
35. Cassells I, Ahenkorah S, Burgoyne AR, Van de Voorde M, Deroose CM, Cardinaels T, et al. Radiolabeling of human serum albumin with terbium-161 using mild conditions and evaluation of in vivo stability. Front Med. (2021) 8:1–12. doi: 10.3389/fmed.2021.675122
36. Skálová M, Palušák M, Suchánková P, Vlk M, Šoltés J, Vinš M, et al. Separation of terbium-161 from gadolinium target irradiated in nuclear reactor. 19th Radiochemical Conference (2022). p. 136–7
37. Aziz A. Physico-chemical characterization of the terbium-161 radioisotope through separation based on cartridge LN resin column from irradiated of enriched Gd2O3 target. J Phys Conf Ser. (2020) 1436:012097. doi: 10.1088/1742-6596/1436/1/012097
38. Aziz A, Artha WT. Radiochemical separation of 161Tb from Gd/Tb matrix using Ln resin column. Indones J Chem. (2016) 16:283–8. doi: 10.22146/ijc.21143
39. Dellepiane G, Casolaro P, Favaretto C, Grundler PV, Mateu I, Scampoli P, et al. Cross section measurement of terbium radioisotopes for an optimized 155Tb production with an 18 MeV medical PET cyclotron. Appl Radiat Isot. (2022) 184:110175. doi: 10.1016/j.apradiso.2022.110175
40. Formento-Cavaier R, Haddad F, Alliot C, Sounalet T, Zahi I. New excitation functions for proton induced reactions on natural gadolinium up to 70 MeV with focus on 149Tb production. Nucl Instruments Methods Phys Res Sect B Beam Interact with Mater Atoms. (2020) 478:174–81. doi: 10.1016/j.nimb.2020.06.029
41. Vermeulen C, Steyn GF, Szelecsényi F, Kovács Z, Suzuki K, Nagatsu K, et al. Cross sections of proton-induced reactions on natGd with special emphasis on the production possibilities of 152Tb and 155Tb. Nucl Instruments Methods Phys Res Sect B Beam Interact with Mater Atoms. (2012) 275:24–32. doi: 10.1016/j.nimb.2011.12.064
42. Favaretto C. Development of terbium radioisotopes towards clinical theragnostics applications in nucelar medicine (Doctoral thesis). ETH Zurich (2023). doi: 10.3929/ethz-b-000602441.
43. Favaretto C, Talip Z, Zhang H, Schibli R, Eichler R, van der Meulen NP. LRC Annual Report. (2021). 41 p.
44. Engle JW, Bach H, Couture A, Gritzo R, Smith DM, Bitteker LJ, et al. Cross sections for proton induced reactions on terbium at 200 MeV. AIP Conf Proc. (2012) 1509:152–6. doi: 10.1063/1.4773958
45. Steyn GF, Vermeulen C, Szelecsényi F, Kovács Z, Hohn A, Van Der Meulen NP, et al. Cross sections of proton-induced reactions on 152Gd, 155Gd and 159Tb with emphasis on the production of selected Tb radionuclides. Nucl Instruments Methods Phys Res Sect B Beam Interact with Mater Atoms. (2014) 319:128–40. doi: 10.1016/j.nimb.2013.11.013
46. Tárkányi F, Ditrói F, Takács S, Hermanne A, Ignatyuk AV. Extension of the energy range of the experimental activation cross-sections data of longer-lived products of proton induced nuclear reactions on dysprosium up to 65 MeV. Appl Radiat Isot. (2015) 98:87–95. doi: 10.1016/j.apradiso.2015.01.015
47. Tárkányi F, Ditrói F, Takács S, Hermanne A, Ignatyuk AV. Activation cross-sections of longer-lived products of proton induced nuclear reactions on dysprosium up to 36 MeV. Ann Nucl Energy. (2013) 62:375–81. doi: 10.1016/j.anucene.2013.06.038
48. Červenák J, Lebeda O. Measurement of the natDy(p,x) nuclear reactions cross-sections. Nucl Instruments Methods Phys Res Sect B Beam Interact with Mater Atoms. (2022) 522:1–13. doi: 10.1016/j.nimb.2022.04.007
49. Shahid M, Kim K, Naik H, Kim G. Measurement of activation cross-sections of natDy(p,x) reactions up to 45 MeV. Nucl Instruments Methods Phys Res Sect B Beam Interact with Mater Atoms. (2020) 464:74–83. doi: 10.1016/j.nimb.2019.12.009
50. Duchemin C, Guertin A, Haddad F, Michel N, Métivier V. Deuteron induced Tb-155 production, a theranostic isotope for SPECT imaging and auger therapy. Appl Radiat Isot. (2016) 118:281–9. doi: 10.1016/j.apradiso.2016.09.030
51. Wang Y, Sounalet T, Guertin A, Haddad F, Michel N, Nigron E. Electrochemical co-deposition of Ni–Gd2O3 for composite thin targets preparation: production of 155Tb as a case study. Appl Radiat Isot. (2022) 186:2–10. doi: 10.1016/j.apradiso.2022.110287
52. Ichinkhorloo D, Aikawa M, Tsoodol Z, Komori Y, Haba H. Production cross sections of terbium and gadolinium radioisotopes from the deuteron-induced reactions on natural gadolinium up to 24 MeV. Nucl Instruments Methods Phys Res Sect B Beam Interact with Mater Atoms. (2023) 536:30–7. doi: 10.1016/j.nimb.2023.01.001
53. Levin VI, Tronova IN, Dmitriev PP, Tikhomirova EA, Gromova NP, Guskov AF. Terbium-155 production without a carrier. Radiokhimia. (1977) 3:388–93.
54. Gayoso RE, Sonzogni AA, Nassiff SJ. (Α,pxn) reactions on natural gadolinium. Radiochim Acta. (1996) 72:55–60. doi: 10.1524/ract.1996.72.2.55
55. Moiseeva AN, Aliev RA, Furkina EB, Novikov VI, Unezhev VN. New method for production of 155Tb via 155Dy by irradiation of natGd by medium energy alpha particles. Nucl Med Biol. (2022) 106–107:52–61. doi: 10.1016/j.nucmedbio.2021.12.004
56. Ichinkhorloo D, Aikawa M, Tsoodol Z, Murata T, Sakaguchi M, Komori Y, et al. Production cross sections of dysprosium, terbium and gadolinium radioisotopes from the alpha-particle-induced reactions on natural gadolinium up to 50 MeV. Nucl Instruments Methods Phys Res Sect B Beam Interact with Mater Atoms. (2021) 499:46–52. doi: 10.1016/j.nimb.2021.04.018
57. Moiseeva AN, Makoveeva KA, Furkina EB, Artyushova EV, German MN, Khomenko IA, et al. Co-production of 155Tb and 152Tb irradiating 155Gd/151Eu tandem target with a medium energy α-particle beam. Nucl Med Biol. (2023) 126–127:108389. doi: 10.1016/j.nucmedbio.2023.108389
58. Levin VI, Malinin AB, Tronova IN. Production of radionuclides by photonuclear reactions. I. Production of terbium-155 and thulium-167 using the electron accelerator EA-25. Radiochem Lett. (1981) 49:111–7.
59. Jakobsson U, Mäkilä E, Rahikkala A, Imlimthan S, Lampuoti J, Ranjan S, et al. Preparation and in vivo evaluation of red blood cell membrane coated porous silicon nanoparticles implanted with 155Tb. Nucl Med Biol. (2020) 84–85:102–10. doi: 10.1016/j.nucmedbio.2020.04.001
60. Webster B, Ivanov P, Russell B, Collins S, Stora T, Ramos JP, et al. Chemical purification of terbium-155 from pseudo-isobaric impurities in a mass separated source produced at CERN. Sci Rep. (2019) 9:1–9. doi: 10.1038/s41598-019-47463-3
61. Michel R, Gloris M, Protoschill J, Herpers U, Kuhnhenn J, Sudbrock F, et al. Cross sections for the production of radionuclides by proton-induced reactions on W, Ta, Pb and Bi from thresholds up to 2.6 GeV. J Nucl Sci Technol. (2002) 39:242–5. doi: 10.1080/00223131.2002.10875084
62. Challan MB, Moawad GS, Abou-Zeid MA, Comsan MNH. Excitation functions of radionuclides produced by proton induced reactions on gadolinium targets. In: Comsan MNH, editor. Proc. 6th Conf. of Nuclear and Particle Physics, Luxor (2007). p. 159–68.
63. Köster U, Assmann W, Bacri CO, Faestermann T, Garrett P, Gernhäuser R, et al. Electromagnetic isotope separation of gadolinium isotopes for the production of 152,155Tb for radiopharmaceutical applications. Nucl Instruments Methods Phys Res Sect B Beam Interact with Mater Atoms. (2020) 463:111–4. doi: 10.1016/j.nimb.2019.07.017
64. Güray RT, Özkan N, Yalçln C, Rauscher T, Gyürky G, Farkas J, et al. Measurements of 152Gd(p,γ)153Tb and 152Gd(p,n)152Tb reaction cross sections for the astrophysical γ process. Phys Rev C—Nucl Phys. (2015) 91:1–8. doi: 10.1103/PhysRevC.91.055809
65. Cicone F, Gnesin S, Denoël T, Stora T, van der Meulen NP, Müller C, et al. Internal radiation dosimetry of a 152Tb-labeled antibody in tumor-bearing mice. EJNMMI Res. (2019) 9:53. doi: 10.1186/s13550-019-0524-7
66. Lahiri S, Nayak D, Das SK, Ramaswami A, Manohor SB, Das NR. Separation of carrier free dysprosium and terbium isotopes from 12C6+ irradiated Nd2O3. Appl Radiat Isot. (1999) 51:27–32. doi: 10.1016/S0969-8043(98)00189-4
67. Sarkar S, Allen BJ, Imam S, Goozee G, Leigh J, Meriaty H, et al. Production and separation of terbium-149,152 for targeted cancer therapy. Second Int Conf Isot. (1997) 104:206–11.
68. Allen BJ, Goozee G, Sarkar S, Beyer G, Morel C, Byrne AP. Production of terbium-152 by heavy ion reactions and proton induced spallation. Appl Radiat Isot. (2001) 54:53–8. doi: 10.1016/S0969-8043(00)00164-0
69. Abbas RS, Sarkar S, Goozee G, Allen BJ. Radioimmunoconjugates for targeted alpha therapy of malignant melanoma. Melanoma Res. (2000) 10:281–9. doi: 10.1097/00008390-200010030-00011
70. Favaretto C, Grundler PV, Talip Z, Köster U, Johnston K, Busslinger SD, et al. Terbium-149 production : a focus on yield and quality improvement towards preclinical application. Sci Rep. (2024) 14:3284. doi: 10.1038/s41598-024-53610-2
71. Verhoeven H, Cocolios TE, Dockx K, Farooq-Smith GJ, Felden O, Formento-Cavaier R, et al. Measurement of spallation cross sections for the production of terbium radioisotopes for medical applications from tantalum targets. Nucl Instruments Methods Phys Res Sect B Beam Interact with Mater Atoms. (2020) 463:327–9. doi: 10.1016/j.nimb.2019.04.071
72. Winsberg L. Recoil studies of nuclear reactions induced by high-energy particles. I. Production of Tb-149. Phys Rev. (1964) 135:B1105. doi: 10.1103/PhysRev.135.B1105
73. Zaitseva NG, Dmitriev SN, Maslov OD, Molokanova LG, Starodub GY, Shishkin SV, et al. Terbium-149 for nuclear medicine. The production of 149Tb via heavy ions induced nuclear reactions. Czechoslov J Phys. (2003) 53:A455–8. doi: 10.1007/s10582-003-0058-z
74. Imam S, Allen B, Goozee G, Sarkar S, Hennier A. 149Terbium, A novel a-emitter for radioimmunotherapy. International Conference on Isotopes (1997). p. 200–5
75. Alexander JM, Simonoff GN. Excitation functions for Tb-149 g from reactions between complex nuclei. Phys Rev. (1963) 130:2383–7. doi: 10.1103/PhysRev.130.2383
76. Müller C, Umbricht CA, Gracheva N, Tschan VJ, Pellegrini G, Bernhardt P, et al. Terbium-161 for PSMA-targeted radionuclide therapy of prostate cancer. Eur J Nucl Med Mol Imaging. (2019) 46:1919–30. doi: 10.1007/s00259-019-04345-0
77. National Library of Medicine. Combined Beta- Plus Auger Electron Therapy Using a Novel Somatostatin Receptor Subtype 2 Antagonist Labelled With Terbium-161 (161Tb-DOTA-LM3) (Beta plus). Identifier NCT05359146 (2022) Available online at: https://clinicaltrials.gov/study/NCT05359146?term=161Tb&rank=1(Accessed September 13, 2024).
78. National Library of Medicine. Evaluation of Radioligand Treatment in Men with Metastatic Castration-resistant Prostate Cancer with [161Tb]Tb-PSMA-I&T: Phase I/II Study. Identifier NCT05521412 (2022) Available online at: https://clinicaltrials.gov/study/NCT05521412?term=161Tb&rank=3 (Accessed September 13, 2024).
79. National Library of Medicine. Targeted Radionuclide Therapy in Metastatic Prostate Cancer Using a New PSMA Ligand Radiolabelled With Terbium-161 (161Tb-SibuDAB)—Dose Identification/Escalation Phase Ia/b Study (PROGNOSTICS). Identifier NCT06343038. (2024). Available online at: https://clinicaltrials.gov/study/NCT06343038?term=161Tb&rank=2 (Accessed September 13, 2024).
80. Barbaro F, Canton L, Uzunov N, De Nardo L, Melendez-Alafort L. 155Tb Production by cyclotrons: what level of 155Gd enrichment allows clinical applications? EJNMMI Phys. (2024) 11:26. doi: 10.1186/s40658-024-00630-6
81. Colombi A, Fontana A. Comparative study of 155Tb production via 155Dy precursor with p, d and α beams on natural targets for medical applications. Appl Radiat Isot. (2024) 212:111443. doi: 10.1016/j.apradiso.2024.111443
82. Colucci M, Bolchini FC, Confalonieri L, Haddad F, Nigron E, Groppi F, et al. Experimental cross-section measurement of the nuclear reactions induced by protons on 159Tb: evaluation of the 155Dy/155Tb precursor system. Radiat Phys Chem. (2024) 224:112069. doi: 10.1016/j.radphyschem.2024.112069
83. Kossakowski R, Jastrzbski J, Rymuza P, Skulski W, Gizon A, André S, et al. Heavy residues following 5–10 MeV/nucleon−12 and−14 induced reactions on Sm and Pr targets. Phys Rev C. (1985) 32:1612–30. doi: 10.1103/PhysRevC.32.1612
84. Maiti M, Lahiri S, Tomar BS. Investigation on the production and isolation of 149,150,151Tb from 12C irradiated natural praseodymium target. Radiochim Acta. (2011) 99:527–34. doi: 10.1524/ract.2011.1839
85. Alexander JM, Simonoff GN. Average energy and angular momentum removed from Dy compound nuclei by neutrons and photons. Phys Rev. (1964) 133:B93. doi: 10.1103/PhysRev.133.B93
86. Dmitriev SN, Beyer GJ, Zaitseva NG, Maslov OD, Molokanova LG, Starodub GY, et al. Lanthanides in nuclear medicine: preparation of 149Tb by irradiation with heavy ions. Radiochemistry. (2002) 44:171–3. doi: 10.1023/A:1019627514277
87. Beyer GJ, Čomor JJ, Daković M, Soloviev D, Tamburella C, Hagebø E, et al. Production routes of the alpha emitting 149Tb for medical application. Radiochim Acta. (2002) 90:247–52. doi: 10.1524/ract.2002.90.5_2002.247
88. Rath PK, Santra S, Singh NL, Nayak BK, Mahata K, Palit R, et al. Complete fusion in 7Li+144,152Sm reactions. Phys Rev C—Nucl Phys. (2013) 88:1–10. doi: 10.1103/PhysRevC.88.044617
89. Bruninx E. The production of Tb-149 from gold in the GeV region. Nucl Phys. (1965) 64:481–5. doi: 10.1016/0029-5582(65)90572-9
90. Franz EM, Friedlander G. Cross sections for production of 149Tb from Au by high-energy protons. Nucl Phys. (1966) 76:123–8. doi: 10.1016/0029-5582(66)90963-1
91. Heydegger HR, Van Ginneken A. Production of 149Tb from gold by 0.2 to 0.5 GeV protons. Nucl Physics, Sect A. (1972) 196:156–60. doi: 10.1016/0375-9474(72)90957-8
92. Titarenko YE, Batyaev VF, Titarenko AY, Butko MA, Pavlov KV, Florya SN, et al. Measurement and simulation of the cross sections for nuclide production in natW and 181Ta targets irradiated with 0.04- to 2.6-GeV protons. Phys At Nucl. (2011) 74:551–72. doi: 10.1134/S1063778811040181
93. Müller C, Reber J, Haller S, Dorrer H, Köster U, Johnston K, et al. Folate receptor targeted alpha-therapy using terbium-149. Pharmaceuticals. (2014) 7:353–65. doi: 10.3390/ph7030353
94. Fiaccabrino DE, Kunz P, Radchenko V. Potential for production of medical radionuclides with on-line isotope separation at the ISAC facility at TRIUMF and particular discussion of the examples of 165Er and 155Tb. Nucl Med Biol. (2021) 94–95:81–91. doi: 10.1016/j.nucmedbio.2021.01.003
95. Nayak D, Lahiri S, Ramaswami A, Manohar SB, Das NR. Separation of carrier free 151,152Tb produced in 16O irradiated lanthanum oxide matrix. Appl Radiat Isot. (1999) 51:631–6. doi: 10.1016/S0969-8043(99)00106-2
96. Lahiri S, Nayak D, Das SK, Ramaswami A, Manohor SB, Das NR. Separation of carrier free 152,153Dy and 151–153Tb from16O7+ irradiated CeO2 by liquid-liquid extraction. J Radioanal Nucl Chem. (1999) 241:201–6. doi: 10.1007/BF02347313
97. Monroy-Guzman F, Salinas EJ. Separation of micro-macrocomponent systems : 149Pm – Nd, 161Tb-Gd, Ho-Dy and 177Lu-Yb by extraction chromatography. J Mex Chem Soc. (2015) 59:143–50. doi: 10.29356/jmcs.v59i2.28
98. Kazakov AG, Aliev RA, Bodrov AY, Priselkova AB, Kalmykov SN. Separation of radioisotopes of terbium from a europium target irradiated by 27 MeV α-particles. Radiochim Acta. (2018) 106:135–40. doi: 10.1515/ract-2017-2777
99. Triskem. Product Sheet LN/LN2/LN3 Resins. (2020). Available online at: https://www.triskem-international.com/scripts/files/5f46343a7c97d945835022/PS_LN-Resin_EN_200603.pdf (Accessed September 13, 2024).
100. Fricke J, Westerbergh F, McDougall L, Favaretto C, Christ E, Nicolas GP, et al. First-in-human administration of terbium-161-labelled somatostatin receptor subtype 2 antagonist [(161Tb)Tb-DOTA-LM3] in a patient with a metastatic neuroendocrine tumour of the ileum. Eur J Nucl Med Mol Imaging. (2024) 51:2517–9. doi: 10.1007/s00259-024-06641-w
101. Schaefer-Schuler A, Burgard C, Blickle A, Maus S, Petrescu C, Petto S, et al. [161tb]Tb-PSMA-617 radioligand therapy in patients with mCRPC: preliminary dosimetry results and intra-individual head-to-head comparison to [177Lu]Lu-PSMA-617. Theranostics. (2024) 14:1829–40. doi: 10.7150/thno.92273
102. Al-Ibraheem A, Doudeen RM, Juaidi D, Abufara A, Maus S. 161Tb-PSMA radioligand therapy: first-in-humans SPECT/CT imaging. J Nucl Med. (2023) 64:1322–3. doi: 10.2967/jnumed.122.265291
103. Eichler R, Kneht A, Kiselev D, van der Meulen N, Koshik A, Scheibl R. IMPACT Conceptual Design Report. (2022). 304 p.
104. Beyer GJ, Miederer M, Vranješ-Durić S, Čomor JJ, Künzi G, Hartley O, et al. Targeted alpha therapy in vivo: direct evidence for single cancer cell kill using 149Tb-rituximab. Eur J Nucl Med Mol Imaging. (2004) 31:547–54. doi: 10.1007/s00259-003-1413-9
Keywords: terbium, theragnostics, radiolanthanides, production capabilities, nuclear reactions
Citation: Moiseeva AN, Favaretto C, Talip Z, Grundler PV and van der Meulen NP (2024) Terbium sisters: current development status and upscaling opportunities. Front. Nucl. Med. 4:1472500. doi: 10.3389/fnume.2024.1472500
Received: 29 July 2024; Accepted: 26 September 2024;
Published: 11 October 2024.
Edited by:
Francesco Cicone, Magna Græcia University, ItalyReviewed by:
Petra Martini, University of Ferrara, ItalyMichiel Van de Voorde, Belgian Nuclear Research Center (SCK CEN), Belgium
Copyright: © 2024 Moiseeva, Favaretto, Talip, Grundler and van der Meulen. This is an open-access article distributed under the terms of the Creative Commons Attribution License (CC BY). The use, distribution or reproduction in other forums is permitted, provided the original author(s) and the copyright owner(s) are credited and that the original publication in this journal is cited, in accordance with accepted academic practice. No use, distribution or reproduction is permitted which does not comply with these terms.
*Correspondence: Nicholas P. van der Meulen, bmljay52YW5kZXJtZXVsZW5AcHNpLmNo