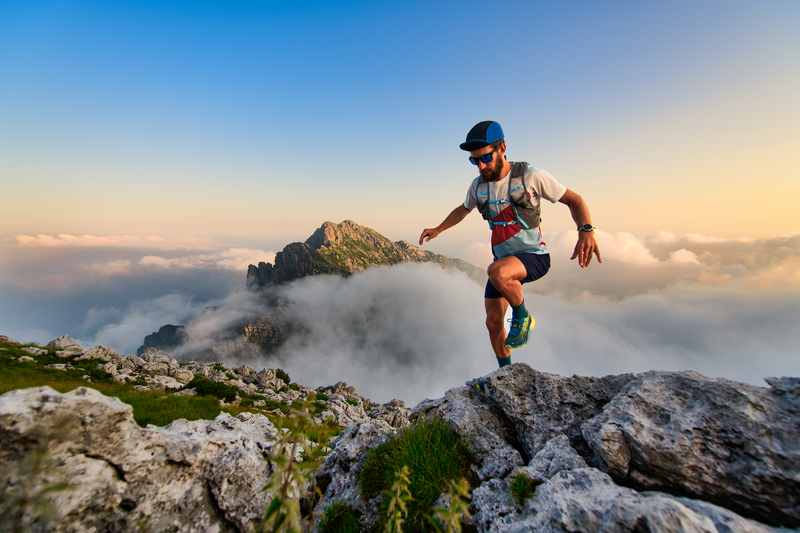
94% of researchers rate our articles as excellent or good
Learn more about the work of our research integrity team to safeguard the quality of each article we publish.
Find out more
REVIEW article
Front. Nucl. Med. , 10 January 2024
Sec. Radionuclide Therapy
Volume 3 - 2023 | https://doi.org/10.3389/fnume.2023.1323514
This article is part of the Research Topic Women in Radionuclide Therapy: 2023 View all 6 articles
Radionuclide-mediated diagnosis and therapy have emerged as effective and low-risk approaches to treating breast cancer. Compared to traditional anatomic imaging techniques, diagnostic radionuclide-based molecular imaging systems exhibit much greater sensitivity and ability to precisely illustrate the biodistribution and metabolic processes from a functional perspective in breast cancer; this transitions diagnosis from an invasive visualization to a noninvasive visualization, potentially ensuring earlier diagnosis and on-time treatment. Radionuclide therapy is a newly developed modality for the treatment of breast cancer in which radionuclides are delivered to tumors and/or tumor-associated targets either directly or using delivery vehicles. Radionuclide therapy has been proven to be eminently effective and to exhibit low toxicity when eliminating both primary tumors and metastases and even undetected tumors. In addition, the specific interaction between the surface modules of the delivery vehicles and the targets on the surface of tumor cells enables radionuclide targeting therapy, and this represents an exceptional potential for this treatment in breast cancer. This article reviews the development of radionuclide molecular imaging techniques that are currently employed for early breast cancer diagnosis and both the progress and challenges of radionuclide therapy employed in breast cancer treatment.
A radionuclide is a nuclide carrying excess nuclear energy that can emit either alpha particles carrying high energy with a short range, beta particles carrying low energy with a longer range, gamma radiation with a long range, or auger electrons with a very short range and low energy (1). Amongst these, alpha particles and beta particles are the most popular choice for radionuclides in breast cancer (BC) diagnosis and treatment (2–4). These two forms of radiation energy can be safely harnessed to break down the physiological processes, most notably the genomic integrity of cancer cells, and eventually lead to cancer cell death and subsequent tumor shrinkage (2). Radionuclide therapy, as a major type of radiopharmaceutical therapy, started in November 1938 when John Lawrence used radioactive phosphorus (32P) to treat leukemia (5). However, radionuclide therapy for use on solid tumors had begun as early as the middle of the 19th century, not long after Dr. Marie Curie discovered radium which was found to damage live tissues and cells following exposure. Subsequently, radium has been employed to treat a variety of diseases besides cancer (6–11). Numerous clinical cases of radionuclide therapy have proved that it is a safe and effective approach for the treatment of many types of cancer (12–14).
Radionuclides are elements, for the medical field this typically means only those radionuclides from the actinide series on the table of elements, that emit energy in the form of radiation (15). The loss of this energy turns the radionuclide from one element to another (daughter isotopes) through a process called decay. In alpha decay, a composite particle composed of two protons and two neutrons is emitted that mimics helium in atomic mass, but it differs from helium in that it is double-ionized. There are two forms of beta particles emitted in beta decay and beta plus decay (also called positron emissions). In beta decay, electrons are expelled from the nucleus at high speeds; these electrons are termed beta particles and are typically used in radionuclide therapies. In beta plus decay, positrons are expelled from the nucleus, also at speeds close to the speed of light, and these positrons are commonly used in the imaging of disease states. Gamma rays are emitted from the nucleus in the form of electromagnetic energy as photons that can be used for either treatment or imaging modalities. Gamma-ray emissions can travel the greatest distance of the radiation types discussed herein. Another form of radioactive decay is electron capture, where orbital electrons fill in a vacancy in the nucleus, resulting in auger emissions. However, the emission of auger electrons requires a vacancy in the nucleus of the radionuclide, which can be induced by other forms of radioactive decay or excitation by an external force, for example by x-rays (16). Regardless of the form of decay, radionuclides express energy from the nucleus (called Emax) that is responsible for cellular DNA damage. The level of energy, however, differs vastly in both the rate of decay and the damage potential.
Radionuclides can damage DNA through either direct or indirect damage. Direct damage can be complex DNA double-strand breaks, single-strand breaks, base damage, and cross-linkage formations (17). Indirect DNA damage typically arises through the generation of reactive species (oxygen or nitrogen) in the treated area that affects the cellular DNA to form mismatches, single-strand breaks, and double-strand breaks (17). Among the three types of radionuclides, alpha is the most ionizing with the greatest potential for direct DNA damage that does not rely on nearby reactive species generation (18, 19). Beta and gamma radionuclides, on the other hand, have less ionizing potential but can damage the DNA through the generation of reactive oxygen species and free radical species. The alpha particles also have a very high linear energy transfer, which contributes to the increased DNA damage potential by causing increasingly complex DNA lesions (20). Additionally, DNA double-strand breaks caused by high linear energy transfer radionuclides are repaired more slowly than are double-strand breaks caused by low linear energy transfer radionuclides (19). Beta particles emitting radionuclides, however, can frequently also generate gamma emissions as a side product of beta decay. Both the alpha and beta particles emitted from radionuclides have direct linear penetration, albeit the particles do not traverse great distances in tissues. However, beta particles can travel farther through the air, and gamma emissions are emitted as photons that travel in a wave that can sometimes co-occur with radionuclides undergoing beta decay. Comparatively, auger emissions have much lower energy and thus a shorter range of penetration in tissues of only a few hundred nanometers. For this reason, auger emitters must be incorporated into the nucleus of a cell to be effective in damaging DNA as a treatment modality.
Recently, targeted radionuclide therapy (TRT) has emerged as a promising strategy to significantly improve radiopharmaceutical efficiency while minimizing toxicity and other side effects (21–28). Unlike traditional radiation therapy in which administration occurs through an external beam, the radionuclides employed in therapy are administered intravenously, intraperitoneally, or orally. During the process of an ideal TRT, internally administered radionuclides will migrate specifically to the tumor region to precisely exert their cytotoxicity without any significant detrimental effects on the surrounding normal tissues (29, 30), as exemplified by a famous case of administration of 131I to patients with thyroid carcinoma (31–35). Specific targeting can be achieved through antigen-antibody recognition (36, 37), ligand-receptor interaction (38), or the interaction between certain biomolecules and unique biomarkers on the surface of tumor cells based on their high affinity (22). As such, the required dosage of radionuclide used in the treatment can be much less, which will significantly minimize the unnecessary exposure of patients to radiation both temporally and spatially. Also, TRT can significantly decrease the background radiation activity which may lead to high drug tolerance (39). In addition, radionuclides can emit either x-rays, gamma-rays, or beta-particles that can be visualized by nuclear medicine imaging systems, such as single-photon emission computed tomography (SPECT) scanning or PET, to directly monitor the efficacy and precision of TRT (40–42).
Accumulated clinical evidence has demonstrated the great treatment potential for TRT targeting both primary tumors and metastases, thus TRT presents a highly effective, safer, and more economical modality when compared to traditional chemotherapy (43). This review will cover the existing TRT in BC and will discuss the clinical development and challenges of TRT.
Breast cancer is the most frequent cancer occurring in women in the United States. According to statistical analyses from the American Cancer Society, about 290 thousand women were diagnosed with BC in 2022, rendering it the most common cancer in U.S. women. Unfortunately, more than 43,000 women died from BC during that year (44). Thus, robust and effective diagnostic and treatment regimens will be critical to improve outcomes for BC patients. As to BC diagnosis, radionuclide molecular imaging has demonstrated indisputable advantages over traditional anatomical imaging strategies that rely on finding the altered anatomical structure of breast tumors, such as mammography, ultrasound, magnetic resonance imaging (MRI), and computed tomography (CT) (45). BC is a highly heterogeneous disease with many subtypes according to its genetic and clinical background (46, 47). The most common classification of BC is based on the expression of estrogen receptor (ER), progesterone receptor (PR), and human epidermal growth factor receptor 2 (HER2). The expression of each of these receptors can be detected by immunohistochemical analyses. The four intrinsic molecular subtypes of BC are luminal A (ER+, PR+, and HER−, the most common), luminal B (ER+, PR+, and HER+), HER2-enriched (ER−, PR−, and HER+), and triple-negative (ER−, PR−, and HER−) (48). Triple-negative BC (TNBC) is the most difficult subtype to treat and the most lethal subtype amongst all BC subtypes (49–51).
Radionuclides employed in BC therapy approaches can be categorized into diagnostic radionuclides and therapeutic radionuclides. Radionuclide-based imaging has been more frequently employed in diagnosing BC in recent years and is particularly important in ascertaining the extent of metastatic disease (52, 53). It offers indisputable advantages to the functional detection of BC through radionuclide-labeled small metabolic compounds for non-invasively illustrating the biological process of BC and radionuclide-labeled ligands/antibodies for specific ligand/receptor interaction-mediated targeting radionuclide molecular imaging of BC (54–56). One well-known clinical technique showing the advantage of radionuclides in cancer therapy is positron emission tomography (PET). As both a research and medical technique, PET is a functional imaging tool employing radiolabeled substances, such as glucose, whose function is to monitor the metabolic processes to detect tumors and search for metastases. The most popular clinical radionuclide used for detecting primary tumors and metastases is 18F-fluoro-deoxy-glucose (FDG) (Figure 1).
Figure 1. Representation of the basic principles and procedures in positron emission tomography (PET) imaging for cancer diagnosis. The targeting molecule is radiolabeled with the positron-emitting isotope. The now radiolabeled targeting complex is then introduced to the patient, typically through injection directly into the bloodstream. The isotope emits positrons within the patients’ system, allowing the PET camera to track the high-density locations of those positron emissions. Finally, an overall picture is generated showing the highest concentration locations of the radiolabeled tumor-targeting complex, thus showing the location of the tumor.
The principle of radionuclide-facilitated diagnosis of BC is illustrated in Figure 2. A common radionuclide cancer therapy is composed of three interconnected parts: A cancer cell-surface specific targeting molecule, a synthetic binding molecule that can specifically bind to the targeting molecule (called a linker), and a radionuclide-labeled chelator that is linked to the binding molecule (Figure 2) (57–59). All these three parts plus the specific surface marker on the cancer cells ensure the radionuclides target BC with high specificity and a high affinity, excluding potential off-target effects.
Figure 2. Schematic overview of receptor-targeting molecular imaging for BC. The molecular radionuclide structure consists of a ligand, linker, chelator, and radionuclide. Ligands that bind to the overexpressed receptors on BC cells can be coupled to chelator often through a linker. Chelators enable the labeling of ligands with radionuclides.
We have listed the commonly used radionuclides and their application in BC in Table 1. Amongst them, 99mTc is the most popular and ideal imaging radionuclide because of its high target/non-target ratio and affordability (71–74).
As to the clinical radionuclide treatment, an optimal radionuclide therapy may provide therapeutic options that were not previously available for BC patients (Table 2). For effective treatment of BC, it is important to maximize tumor cell DNA damage and cytotoxicity while minimizing effects on nearby healthy tissue. The properties of radionuclides that support this therapeutic effect include a short half-life (85), linear energy transfer (86), toxicity (87–89), the range in tissue (2, 90, 91), in vivo stability (92, 93), tissue preference (85), the accumulation in tumors (90), the DNA damage scale (70, 94, 95), and the post-treatment clearance (96, 97). The double-stranded DNA damage caused by the selected particles emitted by a given radionuclide will determine the live or death of the targeted BC cells (69, 94, 98). Theoretically, double-stranded DNA breakage may only require the energy from a single alpha particle or multiple beta particles (99). Although the cytotoxicity of beta particles to cancer cells is much lower than that of alpha particles, alpha particles will generate much less toxicity than beta particles to the surrounding tissues of the tumor because of their short range (less than 100 micrometers), rendering alpha particles a focus for future use in clinical application (100). However, due to the increased penetration of beta particles, beta-emitting radionuclides are currently more frequently used for cancer treatment as they cause more widespread damage that can be somewhat constrained by penetration depth. For example, in 2009, the BC therapeutic agent trastuzumab (Herceptin, a humanized anti-HER-2/neu monoclonal antibody) was cross-linked with succinimidyl 3,6-diaza-5-oxo-3-[2-((triphenylmethyl)thio) ethyl]-8-[(triphenylmethyl)thio] octanoate (SOCTA) followed by labeling with the radionuclide 188Re; using a preclinical orthotopic mouse model, this 188Re-SOCTA-trastuzumab was administered intravenously to mice carrying tumors developed from BT-474 BC cells (HER2+). The results demonstrated that 188Re-SOCTA-trastuzumab accumulated much more in tumors than in normal tissues, suggesting that 188Re-SOCTA-trastuzumab can be a potential agent for targeted therapy (78). A combination treatment was employed using 131I radioactive iodine-conjugated antibodies to target the HER2 antigen to cause cancer cell death. The outcomes from the clinical trials demonstrated the safety and efficacy of this combined therapy for HER2+ BC (101). A recently developed targeted radionuclide theragnostic agent, 131I-GMIB-Anti-Her2-VHH1, has been tested for safety, biodistribution, radiation dosimetry, and tumor-imaging potential in the diagnosis and treatment of HER2+ BC (102). The results indicated that this agent could be a promising drug to image and treat HER2+ BC with much fewer side effects (Figure 3). Recently, Trastuzumab (Herceptin), a monoclonal antibody targeting HER2 receptors, has been covalently bound with 3-phosphonopropionic acid (CEPA) NP and labeled with 225Ac. 225Ac@Fe3O4-CEPA-trastuzumab has shown a high receptor affinity in a preclinical in vivo study in which the significant inhibiting potential of 225Ac@Fe3O4-CEPA-trastuzumab for BC was validated (103, 104).
Figure 3. Administration of radionuclide carriers in the tumor tissue and their further accumulation via active targeting approach of a HER2+ tumor. Representative view of a tumor growing along blood vessels that is HER2+ (purple star receptors). Antibody structures (beige stars) that are radionuclide (radioactive yellow or red symbols attached to antibodies) carriers are introduced. The antibody carriers direct the radionuclides straight to HER2+ tissues such as the tumor, reducing ionizing damage to non-cancerous tissues. On the left of the tumor is a solely β particle emitter (red radioactive symbol), centered above the tumor is both and α and β particle emitter (red and yellow radioactive symbol), and to the right of the tumor is a solely α particle emitter (yellow radioactive symbol). Range and depth of tissue penetration of the respective radionuclides is shown as red clouds centered around the radionuclides.
Radionuclide-labeled antibodies have been tested to improve the therapeutic efficacy and specificity (105–107). The programmed cell death ligand 1 (PD-L1), also named B7-H1 or CD274, is a key element of an immune checkpoint system and is essential for avoiding autoimmunity (108). PD-L1 is expressed on most cancer cells, tumor-associated macrophages (TAM), dendritic cells, activated T cells, as well as cancer-associated fibroblasts within the tumor microenvironment (109–111). PD-L1 can inhibit CD8+ T-cell effector function by interacting with programmed cell death 1 (PD-1) on the surface of T cells (112, 113). Antibodies against PD-L1 have been generated and evaluated in multiple clinical trials against BC, generating exciting outcomes for BC patients (114); perhaps more excitingly, combined anti–PD-L1 therapy with targeted radiotherapy has been shown to yield a higher therapeutic efficacy when compared to the antibody treatment alone (115). Notably, 111In [In]-BnDTPA-trastuzumab-NLS is another radiopharmaceutical agent with theranostic applications for imaging and auger electron radioimmunotherapy of HER2-positive BC (116).
The theranostics of lymph node metastasis is a major barrier to the successful treatment of BC and a key decision-maker in BC patients' prognosis. Notably, a recently designed nano nuclear drug (68Ga-NP-mAb or 177Lu-NP-mAb) displayed exceptional stability, considerable accumulation, and sustained retention in the lymph node metastases post-intratumoral injection. This agent not only significantly reduced the incidence of lymph node metastasis but also shrank the volumes of lymph node metastases as well without apparent toxicity in a mouse model (117). This is an example of how radionuclide-mediated therapy will open new avenues for the diagnosis and treatment of BC metastases. For TNBC, which has no ideal cell surface biomarkers available for targeted therapy, auger emitters have demonstrated an excellent therapeutic effect as long as they can be delivered directly into the nucleus proximal to DNA. The nuclear protein poly (ADP-ribose)-polymerase 1 has been reported as a possible target, but ideal inhibitors (PARPi) are not clinically available for current therapy of BC carrying the BC gene germline mutation (BRCAmut). A recent study employed a theranostic approach in a xenografted mouse TNBC model by radiolabeling a close derivative of the PARPi Olaparib (e.g., PARPi-01) with the auger emitters 125I, or [125I] PARPi-01. The results support the potential role of [125I] PARPi-01 in improving the use of radiation and radionclides to treat TNBC (118, 119).
Radionuclides such as radium-223 (Xofigo) (77, 119–121), strontium-89 (122–124), and samarium-153 EDTMP (125, 126) have been employed to treat BC bone metastases since their radioactive particles (α or β) are most likely to be absorbed in the setting of intensive bone turnover. To improve the efficacy of radionuclide therapy, radionuclides have been frequently combined with nanoparticles (NPs, 1–100 nm in diameter) in BC therapeutics due to their specific advantages for drug delivery. These include biocompatibility, low toxicity, high stability, excellent penetration ability, and tissue retaining efficiency (127–129). NP can be generated from any solid or liquid material, such as dielectrics, semiconductors, inorganic molecules, and organic molecules. To treat epidermal growth factor receptor (EGFR)-positive TNBC, gold NP (AuNP) was modified with polyethylene glycol (PEG) chains derivatized with 1,4,7,10-tetraazacyclododecane-1,4,7,10-tetraacetic acid (DOTA) chelators for conjugating with emitter 177Lu and with PEG chains linked to panitumumab (selectively binds to EGFR) for targeting TNBC cells with surface EGFR expression. Non-targeted 177Lu-NT-AuNP and EGFR-targeted AuNP (177Lu-T-AuNP) were subcutaneously administered into xenograft mouse models bearing EGFR+ MDA-MB-468 human BC tumors. The results demonstrated that 177Lu-T-AuNP is a potent radionuclide therapeutic agent for EGFR-positive TNBC therapy (130–132).
For early-stage cancer, antibodies targeting BC cell surface antigens such as CEA, MUC1, and L6 had been employed (68). Technitium-labeled anti-CEA Fab′ fragments showed 94% more sensitivity in breast tumors in a small clinical trial (133). 90Y-labeled humanized BrE3 antibody against MUC1 displayed a promising anti-tumor effect in a clinical trial (134, 135). The chimeric L6 antibody labeled with 131I also showed a considerable anti-tumor effect in clinical trials (136–138).
Chondroitin sulfate proteoglycan 4 (CSPG4), a highly glycosylated transmembrane protein, has recently been identified as a target for TNBC treatment due to its high expression on the surface of TNBC cells and its limited expression in normal tissues (more than six times lower than tumors) (139, 140). Therefore, we can use monoclonal antibodies recognizing CSPG4 conjugated to a radioactive isotope for radioimmunotherapy, which we achieve by targeting radiation directly and more specifically tumor cells, reducing non-specific exposure of normal cells to the radioactive isotope. Monoclonal antibody 225.28 specifically against CSPG4 was radiolabeled with 212Pb, allowing 212Pb-mAb 225.28 to specifically recognize TNBC cells and cause cell death in vitro and thus tumor reduction in a xenograft-bearing mouse model. These promising outcomes support 212Pb-mAb 225.28 as a potential therapeutic agent against TNBC (82).
Prostate-specific membrane antigen (PSMA) has been recently shown to be highly expressed on the cell surface of TNBC cells and adjacent endothelial cells, suggesting that PSMA can be a promising target for TNBC treatment. [177Lu] Lu-PSMA induced frequent apoptotic events in BT-20 and MDA-MB-231 tumor-associated endothelial cells, significantly limiting the proliferation of TNBC cells in the in vitro co-culture cellular models tested (141). Significant uptake of radiolabeled ligand [68Ga]Ga-PSMA was detected in BC stem cells expressing a high level of PSMA proteins on their cell surface (67). Furthermore, the hypoxic environment significantly promoted the uptake of radiolabeled ligand [177Lu] Lu-PSMA in MDA-MB-231 and MCF-7 cells (142). 177Lu has also been used to label tumor-targeting alkyl phosphocholine (NM600) for TNBC radionuclide therapy. 177Lu-NM600 has been shown to considerably extend the survival rate in syngeneic murine models bearing tumors developed from either 4T07 or 4T1 TNBC cells (143).
Mesothelin is a glycosylphosphatidylinositol-anchored cell-surface glycoprotein that is highly expressed in BC cells with a severely limited expression in normal tissues (144–146). A mesothelin-targeted thorium-227 conjugate, BAY 2287411 was tested for binding activity, radio stability, biodistribution, mode-of-action, and antitumor potency using an in vitro cellular model, an in vivo orthotopic model, and a patient-derived xenograft model. This demonstrated that BAY 2287411 treatment induces double-strand DNA breaks, apoptosis, and oxidative stress. It significantly decreases cell viability and shows a high antitumor potency. Biodistribution studies also suggested a specific uptake and retention of BAY 2287411 in tumors and not in normal tissues (147).
Fulvestrant (an endocrine therapy drug for BC) was labeled with radionuclide 131I to generate 131I-fulvestrant followed by an evaluation of its effect on BC cell viability and attenuation of the development of human BC and its toxicity to major organs in xenograft nude mouse models. 131I-fulvestrant is remarkably stable and shows a strong binding affinity to estrogen receptor-positive (ER+) MCF-7 cells (148). In addition, 131I-fulvestrant exhibited significant cytotoxicity in MCF-7 and MDA-MB-231 cells (ER−) and exerted a more pronounced suppressive effect on tumors derived from MCF-7 cells than from MDA-MB-231 cells. After 131I-fulvestrant was injected into nude mice intravenously, the distribution of radioactivity was tracked to ER expressing locations, and the majority of 131I-fulvestrant was confined to the tumors. 131I-fulvestrant could attenuate the proliferation of MCF-7 BC cells in vitro and inhibited the growth of tumors derived from implanted MCF-7 cells in nude mice whereas the toxicity of 131I-fulvestrant to the major organs of mice was mild and controllable. This renders 131I-fulvestrant a promising drug for BC treatment that combines the advantages of both radiotherapy and endocrine therapy (148).
In a newly-developed target alpha therapy, an anti-androgen receptor (AR)-targeted radiotherapy platform (Hu11B6) labeled with the alpha-particle emitting Ac-225 (225Ac-hu11B6) has been evaluated in murine xenograft AR-positive BC models. The results show a successful site-specific delivery of therapeutic Ac-225 to tumor tissues and effective, long-term, local tumor control (149). 177Lu labeled bombesin-poly (D, L-lactide-co-glycolide) acid (paclitaxel) NP display specific cellular uptake and high treatment efficacy in both in vitro and in vivo BC mouse models since bombesin can specifically recognize the gastrin-releasing peptide receptor, that is overexpressed on more than 75% of BC (75). While 223Ra has been evaluated to treat bone metastases in BC patients, in a mouse model, the administration of 224Ra significantly reduced the bone metastatic incidence from tumors developed from implanted MDA-MB-231(SA)-GFP human BC cells (84).
Growing evidence has suggested that radionuclide therapy holds great potential to robustly improve the treatment efficacy of BC, especially for imaging and therapy. However, one upfront challenge to be solved is the off-target effects and toxicity from radionuclides emitting beta particles, such as 90Sr, 14C, and 210Pb (100, 150). Although radionuclides emitting alpha radiation have a high linear energy transfer and can treat cancer rapidly with high efficiency, their half-life is generally much shorter. There may be a need to combine alpha and beta radiation emitters in a proper ratio to achieve the best therapeutic outcomes. We may also need to find a way to calculate the ratio of the radiation uptake between tumor tissues and surrounding normal healthy tissues. The implementation of radionuclide therapy also has to deal with the deep social fear of radioactivity (151).
Over the last three decades, drug delivery agents and methods have been intensively investigated to discover efficient and specific drug delivery protocols with limited or no off-target effects to improve the diagnostic and therapeutic outcomes of BC; these include peptides, small nanobody molecules, monoclonal antibodies, fragments of monoclonal antibodies, exosomes, and NP (152–156). However, amongst the aforementioned methods, one challenge for radiolabeled antibodies is the intra- and inter-tumor heterogeneity of their uptake by cancer cells, and imaging techniques with high resolution are required to show this heterogeneity. Certain situations may require two types of radionuclides to achieve the best imaging and therapeutic results, one radionuclide for imaging and another one for therapy (157). The antibodies' size limits their capability to penetrate tumors, directing the radiation emitted from radionuclides away from their targeted sites. As such, nanobodies have recently emerged as promising alternatives to robustly increase the capability of tumor penetration (158, 159). Although the resistance mechanisms to alpha particles are not significant, cancer cells may develop mutations to limit the specific delivery of radionuclides to cancer cells, such as the decreased expression of cancer cell surface antigens that are selected for targeting. Therefore, identifying more antigen and epitope candidates on BC cell surfaces for radionuclide-specific targeting will become increasingly indispensable to the elimination of tumors that have already acquired resistance to previous radionuclide therapy.
Thus far, there is not a medical constituency for radionuclide diagnosis and therapy, implying a need for a new specialty to provide the multidisciplinary training (in general oncology, radiation oncology, and nuclear medicine) necessary for safe, efficient, and effective administration of radionuclides to BC patients. Having expertise in both imaging and radionuclide dosimetry becomes extremely important for a medical physicist because the delivery of radionuclides and the distribution of radiation are critical for the successful treatment of BC. In addition, dosimetry calculations for the medical radioisotopes and daughters are still challenging given the many factors that need to be considered (160–164). It is necessary to accurately determine radiochemical purity and dosage as well as to account for both the parent radionuclide and associated decay daughters, as their relocation from the tumor site potentially places healthy tissues at risk. Promisingly, there is a novel method to minimize the release of the radioisotope daughters by encapsulating the radionuclides in exosomes (155) or by conjugating with nanobodies as we have currently designed in our laboratories. These exosomes carry a specific targeting peptide or nanobodies that can recognize the specific cell surface marker on BC cells allowing us to evaluate their anti-oncologic capability in pre-clinical animal models. There are still many additional tests that need to be done before being applied clinically, but novel treatment approaches are clearly needed for this challenging disease.
Despite several radiopharmaceuticals being used for therapeutic targeting that have shown clinical value in many types of cancer and have been or will soon be approved and authorized for clinical use around in the world, radiopharmaceuticals do possess many side effects as well. Therefore, more research is required to establish the efficacy of therapeutic strategies; use in combination with other treatment modalities may result in better efficacy and reduced side effects. Hence incorporation of correlative biomarker studies is imperative to draw meaningful conclusions for individualizing critical therapeutic decisions that can be effectively generalized and implemented beyond the setting of this clinical trial. Additionally, testing the immune priming potential of radiation in combination with chemotherapy and/or immune checkpoint inhibitors will also provide a novel opportunity to induce immune modulation in BCs, which are largely considered to be poorly immunogenic.
YJ: Investigation, Supervision, Writing – original draft. AM: Writing – review & editing. SD: Writing – review & editing. BE: Writing – review & editing. PM: Writing – review & editing. JM: Writing – review & editing.
The author(s) declare that no financial support was received for the research, authorship, and/or publication of this article.
The authors declare that the research was conducted in the absence of any commercial or financial relationships that could be construed as a potential conflict of interest.
All claims expressed in this article are solely those of the authors and do not necessarily represent those of their affiliated organizations, or those of the publisher, the editors and the reviewers. Any product that may be evaluated in this article, or claim that may be made by its manufacturer, is not guaranteed or endorsed by the publisher.
1. Shah HJ, Ruppell E, Bokhari R, Aland P, Lele VR, Ge C, et al. Current and upcoming radionuclide therapies in the direction of precision oncology: a narrative review. Eur J Radiol Open. (2023) 10:100477. doi: 10.1016/j.ejro.2023.100477
2. Sgouros G, Bodei L, McDevitt MR, Nedrow JR. Radiopharmaceutical therapy in cancer: clinical advances and challenges. Nat Rev Drug Discov. (2020) 19(9):589–608. doi: 10.1038/s41573-020-0073-9
3. Bodei L, Herrmann K, Schoder H, Scott AM, Lewis JS. Radiotheranostics in oncology: current challenges and emerging opportunities. Nat Rev Clin Oncol. (2022) 19(8):534–50. doi: 10.1038/s41571-022-00652-y
4. Vermeulen K, Vandamme M, Bormans G, Cleeren F. Design and challenges of radiopharmaceuticals. Semin Nucl Med. (2019) 49(5):339–56. doi: 10.1053/j.semnuclmed.2019.07.001
5. Berlin NI. Treatment of the myeloproliferative disorders with 32P. Eur J Haematol. (2000) 65(1):1–7. doi: 10.1034/j.1600-0609.2000.9r119.x
7. Aly A. Pituitary tumors treated with radium. Acta Unio Int Contra Cancrum. (1952) 8(2):577–8.13007581
8. Barringer BS. Radium therapy of bladder cancer, retrospect and prospect. J Urol. (1952) 68(1):280–2. doi: 10.1016/S0022-5347(17)68194-1
9. Maurer H. Results of chordectomy and radium irradiation in the tumor area in vocal cord cancer. HNO. (1962) 10:8–12.14471548
10. van Dijk B, Lemans JVC, Hoogendoorn RM, Dadachova E, de Klerk JMH, Vogely HC, et al. Treating infections with ionizing radiation: a historical perspective and emerging techniques. Antimicrob Resist Infect Control. (2020) 9(1):121. doi: 10.1186/s13756-020-00775-w
11. Dadachova E, Nakouzi A, Bryan RA, Casadevall A. Ionizing radiation delivered by specific antibody is therapeutic against a fungal infection. Proc Natl Acad Sci U S A. (2003) 100(19):10942–7. doi: 10.1073/pnas.1731272100
12. Yeong CH, Cheng MH, Ng KH. Therapeutic radionuclides in nuclear medicine: current and future prospects. J Zhejiang Univ Sci B. (2014) 15(10):845–63. doi: 10.1631/jzus.B1400131
13. Salih S, Alkatheeri A, Alomaim W, Elliyanti A. Radiopharmaceutical treatments for cancer therapy, radionuclides characteristics, applications, and challenges. Molecules. (2022) 27(16):5231. doi: 10.3390/molecules27165231
14. Gomes Marin JF, Nunes RF, Coutinho AM, Zaniboni EC, Costa LB, Barbosa FG, et al. Theranostics in nuclear medicine: emerging and Re-emerging integrated imaging and therapies in the era of precision oncology. Radiographics. (2020) 40(6):1715–40. doi: 10.1148/rg.2020200021
15. Lepareur N, Ramee B, Mougin-Degraef M, Bourgeois M. Clinical advances and perspectives in targeted radionuclide therapy. Pharmaceutics. (2023) 15(6):1733. doi: 10.3390/pharmaceutics15061733
16. Chirayath VA, Callewaert V, Fairchild AJ, Chrysler MD, Gladen RW, McDonald AD, et al. Auger electron emission initiated by the creation of valence-band holes in graphene by positron annihilation. Nat Commun. (2017) 8:16116. doi: 10.1038/ncomms16116
17. Kratochwil C, Giesel FL, Heussel CP, Kazdal D, Endris V, Nientiedt C, et al. Patients resistant against PSMA-targeting alpha-radiation therapy often harbor mutations in DNA damage-repair-associated genes. J Nucl Med. (2020) 61(5):683–8. doi: 10.2967/jnumed.119.234559
18. Sgouros G, Roeske JC, McDevitt MR, Palm S, Allen BJ, Fisher DR, et al. MIRD Pamphlet No. 22 (abridged): radiobiology and dosimetry of alpha-particle emitters for targeted radionuclide therapy. J Nucl Med. (2010) 51(2):311–28. doi: 10.2967/jnumed.108.058651
19. Thomlinson RH, Gray LH. The histological structure of some human lung cancers and the possible implications for radiotherapy. Br J Cancer. (1955) 9(4):539–49. doi: 10.1038/bjc.1955.55
20. Maliszewska-Olejniczak K, Kaniowski D, Araszkiewicz M, Tyminska K, Korgul A. Molecular mechanisms of specific cellular DNA damage response and repair induced by the mixed radiation field during boron neutron capture therapy. Front Oncol. (2021) 11:676575. doi: 10.3389/fonc.2021.676575
21. Jadvar H. Targeted radionuclide therapy: an evolution toward precision cancer treatment. AJR Am J Roentgenol. (2017) 209(2):277–88. doi: 10.2214/AJR.17.18264
22. Filippi L, Bagni O, Nervi C. Aptamer-based technology for radionuclide targeted imaging and therapy: a promising weapon against cancer. Expert Rev Med Devices. (2020) 17(8):751–8. doi: 10.1080/17434440.2020.1796633
23. Baum RP, Schuchardt C, Singh A, Chantadisai M, Robiller FC, Zhang J, et al. Feasibility, biodistribution, and preliminary dosimetry in peptide-targeted radionuclide therapy of diverse adenocarcinomas using (177)Lu-FAP-2286: first-in-humans results. J Nucl Med. (2022) 63(3):415–23. doi: 10.2967/jnumed.120.259192
24. Czerwinska M, Bilewicz A, Kruszewski M, Wegierek-Ciuk A, Lankoff A. Targeted radionuclide therapy of prostate cancer-from basic research to clinical perspectives. Molecules. (2020) 25(7):1743. doi: 10.3390/molecules25071743
25. Brechbiel MW. Targeted alpha-therapy. Cancer Biother Radiopharm. (2020) 35(6):397. doi: 10.1089/cbr.2020.29008.mbr
26. Gill MR, Falzone N, Du Y, Vallis KA. Targeted radionuclide therapy in combined-modality regimens. Lancet Oncol. (2017) 18(7):e414–23. doi: 10.1016/S1470-2045(17)30379-0
27. Kleinendorst SC, Oosterwijk E, Bussink J, Westdorp H, Konijnenberg MW, Heskamp S. Combining targeted radionuclide therapy and immune checkpoint inhibition for cancer treatment. Clin Cancer Res. (2022) 28(17):3652–7. doi: 10.1158/1078-0432.CCR-21-4332
28. Miao Y, Quinn TP. Peptide-targeted radionuclide therapy for melanoma. Crit Rev Oncol Hematol. (2008) 67(3):213–28. doi: 10.1016/j.critrevonc.2008.02.006
29. Williams LE, DeNardo GL, Meredith RF. Targeted radionuclide therapy. Med Phys. (2008) 35(7):3062–8. doi: 10.1118/1.2938520
30. Artigas C, Mileva M, Flamen P, Karfis I. Targeted radionuclide therapy: an emerging field in solid tumours. Curr Opin Oncol. (2021) 33(5):493–9. doi: 10.1097/CCO.0000000000000762
31. Dargent M, Berger M, Guinet P. Physiopathological data obtained with iodine 131 in the study of thyroid cancer. Tumori. (1951) 25(1):130–52.14835883
32. Henk JM, Kirkman S, Owen GM. Whole-body scanning and 131 I therapy in the management of thyroid carcinoma. Br J Radiol. (1972) 45(533):369–76. doi: 10.1259/0007-1285-45-533-369
33. Closuit M, Lapp R, Lerch P. On the treatment of thyroid cancer and its metastases with radioiodine 131. Helv Med Acta. (1951) 18(4–5):469–74.14873181
34. Leenhardt P, Pourquier H. Value of radioactive iodine I 131 in the diagnosis and treatment of thyroid cancer. Montp Med. (1956) 50(1):68–76.13378396
35. Bricout P, Kibler RS. Experience in the management of thyroid carcinoma by I-131. A report of 39 cases. J Can Assoc Radiol. (1973) 24(4):323–7.4773908
36. Tolmachev V, Bodenko V, Oroujeni M, Deyev S, Konovalova E, Schulga A, et al. Direct in vivo comparison of (99 m)Tc-labeled scaffold proteins, DARPin G3 and ADAPT6, for visualization of HER2 expression and monitoring of early response for trastuzumab therapy. Int J Mol Sci. (2022) 23(23):15181. doi: 10.3390/ijms232315181
37. Zhang Y, Ding Y, Li N, Wang S, Zhou S, Li R, et al. Noninvasive imaging of tumor PD-L1 expression using [(99 m)Tc]Tc-labeled KN035 with SPECT/CT. Mol Pharm. (2023) 20(1):690–700. doi: 10.1021/acs.molpharmaceut.2c00874
38. Kunikowska J, Morgenstern A, Pelka K, Bruchertseifer F, Krolicki L. Targeted alpha therapy for glioblastoma. Front Med. (2022) 9:1085245. doi: 10.3389/fmed.2022.1085245
39. Dash A, Knapp FF, Pillai MR. Targeted radionuclide therapy–an overview. Curr Radiopharm. (2013) 6(3):152–80. doi: 10.2174/18744710113066660023
40. Hamoudeh M, Kamleh MA, Diab R, Fessi H. Radionuclides delivery systems for nuclear imaging and radiotherapy of cancer. Adv Drug Deliv Rev. (2008) 60(12):1329–46. doi: 10.1016/j.addr.2008.04.013
41. Seo Y, Mari C, Hasegawa BH. Technological development and advances in single-photon emission computed tomography/computed tomography. Semin Nucl Med. (2008) 38(3):177–98. doi: 10.1053/j.semnuclmed.2008.01.001
42. Aide N, Heutte N, Rame JP, Rousseau E, Loiseau C, Henry-Amar M, et al. Clinical relevance of single-photon emission computed tomography/computed tomography of the neck and thorax in postablation (131)I scintigraphy for thyroid cancer. J Clin Endocrinol Metab. (2009) 94(6):2075–84. doi: 10.1210/jc.2008-2313
43. Damiana TST, Dalm SU. Combination therapy, a promising approach to enhance the efficacy of radionuclide and targeted radionuclide therapy of prostate and breast cancer. Pharmaceutics. (2021) 13(5):674. doi: 10.3390/pharmaceutics13050674
44. Siegel RL, Miller KD, Wagle NS, Jemal A. Cancer statistics, 2023. CA Cancer J Clin. (2023) 73(1):17–48. doi: 10.3322/caac.21763
45. Tolmachev V, Orlova A, Sorensen J. The emerging role of radionuclide molecular imaging of HER2 expression in breast cancer. Semin Cancer Biol. (2021) 72:185–97. doi: 10.1016/j.semcancer.2020.10.005
46. Polyak K. Heterogeneity in breast cancer. J Clin Invest. (2011) 121(10):3786–8. doi: 10.1172/JCI60534
47. Luond F, Tiede S, Christofori G. Breast cancer as an example of tumour heterogeneity and tumour cell plasticity during malignant progression. Br J Cancer. (2021) 125(2):164–75. doi: 10.1038/s41416-021-01328-7
48. Turner KM, Yeo SK, Holm TM, Shaughnessy E, Guan JL. Heterogeneity within molecular subtypes of breast cancer. Am J Physiol Cell Physiol. (2021) 321(2):C343–54. doi: 10.1152/ajpcell.00109.2021
49. MacDonald I, Nixon NA, Khan OF. Triple-negative breast cancer: a review of current curative intent therapies. Curr Oncol. (2022) 29(7):4768–78. doi: 10.3390/curroncol29070378
50. Yin L, Duan JJ, Bian XW, Yu SC. Triple-negative breast cancer molecular subtyping and treatment progress. Breast Cancer Res. (2020) 22(1):61. doi: 10.1186/s13058-020-01296-5
51. Howard FM, Olopade OI. Epidemiology of triple-negative breast cancer: a review. Cancer J. (2021) 27(1):8–16. doi: 10.1097/PPO.0000000000000500
52. Mankoff DA. Radionuclide breast cancer imaging 2013: state of the art. Semin Nucl Med. (2013) 43(4):268–70. doi: 10.1053/j.semnuclmed.2013.03.002
53. Hsu DF, Freese DL, Levin CS. Breast-dedicated radionuclide imaging systems. J Nucl Med. (2016) 57(1):40S–5S. doi: 10.2967/jnumed.115.157883
54. Josefsson A, Nedrow JR, Park S, Banerjee SR, Rittenbach A, Jammes F, et al. Imaging, biodistribution, and dosimetry of radionuclide-labeled PD-L1 antibody in an immunocompetent mouse model of breast cancer. Cancer Res. (2016) 76(2):472–9. doi: 10.1158/0008-5472.CAN-15-2141
55. Vorobyeva A, Bezverkhniaia E, Konovalova E, Schulga A, Garousi J, Vorontsova O, et al. Radionuclide molecular imaging of EpCAM expression in triple-negative breast cancer using the scaffold protein DARPin Ec1. Molecules. (2020) 25(20):4719. doi: 10.3390/molecules25204719
56. Sgouros G. Radiopharmaceutical therapy. Health Phys. (2019) 116(2):175–8. doi: 10.1097/HP.0000000000001000
57. Fernandes RS, de Aguiar Ferreira C, Soares DCF, Maffione AM, Townsend DM, Rubello D, et al. The role of radionuclide probes for monitoring anti-tumor drugs efficacy: a brief review. Biomed Pharmacother. (2017) 95:469–76. doi: 10.1016/j.biopha.2017.08.079
58. Zhang GX, Liu YL, Yang M, Huang WS, Xu JH. An aptamer-based, fluorescent and radionuclide dual-modality probe. Biochimie. (2020) 171–172:55–62. doi: 10.1016/j.biochi.2020.02.007
59. Lim MS, Jinih M, Ngai CH, Foley NM, Redmond HP. The utility of the radionuclide probe in parathyroidectomy for primary hyperparathyroidism. Ann R Coll Surg Engl. (2017) 99(5):369–72. doi: 10.1308/rcsann.2017.0016
60. D'Huyvetter M, Vos J, Caveliers V, Vaneycken I, Heemskerk J, Duhoux FP, et al. Phase I trial of (131)I-GMIB-anti-HER2-VHH1, a new promising candidate for HER2-targeted radionuclide therapy in breast cancer patients. J Nucl Med. (2021) 62(8):1097–105. doi: 10.2967/jnumed.120.255679
61. Yook S, Cai Z, Lu Y, Winnik MA, Pignol JP, Reilly RM. Intratumorally injected 177Lu-labeled gold nanoparticles: gold nanoseed brachytherapy with application for neoadjuvant treatment of locally advanced breast cancer. J Nucl Med. (2016) 57(6):936–42. doi: 10.2967/jnumed.115.168906
62. Dadachova E, Bouzahzah B, Zuckier LS, Pestell RG. Rhenium-188 as an alternative to iodine-131 for treatment of breast tumors expressing the sodium/iodide symporter (NIS). Nucl Med Biol. (2002) 29(1):13–8. doi: 10.1016/S0969-8051(01)00279-7
63. Kairemo K, Kangasmaki A. Imaging of accidental contamination by fluorine-18 solution: a quick troubleshooting procedure. Asia Ocean J Nucl Med Biol. (2016) 4(1):51–4. doi: 10.7508/aojnmb.2016.04.008
64. Jiang L, Tu Y, Shi H, Cheng Z. PET Probes beyond (18)F-FDG. J Biomed Res. (2014) 28(6):435–46. doi: 10.7555/JBR.28.20130196
65. Chang CY, Hung GU, Hsu B, Yang BH, Chang CW, Hu LH, et al. Simplified quantification of (13)N-ammonia PET myocardial blood flow: a comparative study with the standard compartment model to facilitate clinical use. J Nucl Cardiol. (2020) 27(3):819–28. doi: 10.1007/s12350-018-1450-1
66. Muzik O, Mangner TJ, Leonard WR, Kumar A, Janisse J, Granneman JG. 15O PET measurement of blood flow and oxygen consumption in cold-activated human brown fat. J Nucl Med. (2013) 54(4):523–31. doi: 10.2967/jnumed.112.111336
67. Medina-Ornelas S, Garcia-Perez F, Estrada-Lobato E, Ochoa-Carrillo F. (68)Ga-PSMA PET/CT in the evaluation of locally advanced and metastatic breast cancer, a single center experience. Am J Nucl Med Mol Imaging. (2020) 10(3):135–42.32704404
68. Goldenberg DM. Targeted therapy of cancer with radiolabeled antibodies. J Nucl Med. (2002) 43(5):693–713.11994535
69. Graf F, Fahrer J, Maus S, Morgenstern A, Bruchertseifer F, Venkatachalam S, et al. DNA Double strand breaks as predictor of efficacy of the alpha-particle emitter Ac-225 and the electron emitter Lu-177 for somatostatin receptor targeted radiotherapy. PLoS One. (2014) 9(2):e88239. doi: 10.1371/journal.pone.0088239
70. Grzmil M, Boersema P, Sharma A, Blanc A, Imobersteg S, Pruschy M, et al. Comparative analysis of cancer cell responses to targeted radionuclide therapy (TRT) and external beam radiotherapy (EBRT). J Hematol Oncol. (2022) 15(1):123. doi: 10.1186/s13045-022-01343-y
71. Taillefer R. The role of 99mTc-sestamibi and other conventional radiopharmaceuticals in breast cancer diagnosis. Semin Nucl Med. (1999) 29(1):16–40. doi: 10.1016/S0001-2998(99)80027-0
72. Sharma R, Tripathi M, Panwar P, Chuttani K, Jaimini A, Maitra S, et al. 99mTc-methionine Scintimammography in the evaluation of breast cancer. Nucl Med Commun. (2009) 30(5):338–42. doi: 10.1097/MNM.0b013e32832999dc
73. Mansi L, Rambaldi PF, Cuccurullo V, Pecori B, Quarantelli M, Fallanca F, et al. Diagnostic and prognostic role of 99mTc-tetrofosmin in breast cancer. Q J Nucl Med. (1997) 41(3):239–50.9274132
74. Nawar MF, Turler A. New strategies for a sustainable (99 m)Tc supply to meet increasing medical demands: promising solutions for current problems. Front Chem. (2022) 10:926258. doi: 10.3389/fchem.2022.926258
75. Gibbens-Bandala B, Morales-Avila E, Ferro-Flores G, Santos-Cuevas C, Melendez-Alafort L, Trujillo-Nolasco M, et al. (177)Lu-Bombesin-PLGA (paclitaxel): a targeted controlled-release nanomedicine for bimodal therapy of breast cancer. Mater Sci Eng C Mater Biol Appl. (2019) 105:110043. doi: 10.1016/j.msec.2019.110043
76. Ayati N, Aryana K, Jalilian A, Hoseinnejad T, Samani AB, Ayati Z, et al. Treatment efficacy of (153)Sm-EDTMP for painful bone metastasis. Asia Ocean J Nucl Med Biol. (2013) 1(1):27–31. doi: 10.7508/aojnmb.2013.01.006
77. Rubini G, Nicoletti A, Rubini D, Asabella AN. Radiometabolic treatment of bone-metastasizing cancer: from 186rhenium to 223radium. Cancer Biother Radiopharm. (2014) 29(1):1–11. doi: 10.1089/cbr.2013.1549
78. Luo TY, Tang IC, Wu YL, Hsu KL, Liu SW, Kung HC, et al. Evaluating the potential of 188Re-SOCTA-trastuzumab as a new radioimmunoagent for breast cancer treatment. Nucl Med Biol. (2009) 36(1):81–8. doi: 10.1016/j.nucmedbio.2008.10.014
79. Ahenkorah S, Cassells I, Deroose CM, Cardinaels T, Burgoyne AR, Bormans G, et al. Bismuth-213 for targeted radionuclide therapy: from atom to bedside. Pharmaceutics. (2021) 13(5):599. doi: 10.3390/pharmaceutics13050599
80. Kauffman N, Singh SK, Morrison J, Zinn KR. Effective therapy with Bismuth-212 labeled macroaggregated albumin in orthotopic mouse breast tumor models. Front Chem. (2023) 11:1204872. doi: 10.3389/fchem.2023.1204872
81. Guerard F, Gestin JF, Brechbiel MW. Production of [(211)At]-astatinated radiopharmaceuticals and applications in targeted alpha-particle therapy. Cancer Biother Radiopharm. (2013) 28(1):1–20. doi: 10.1089/cbr.2012.1292
82. Kasten BB, Oliver PG, Kim H, Fan J, Ferrone S, Zinn KR, et al. (212)Pb-Labeled antibody 225.28 targeted to chondroitin sulfate proteoglycan 4 for triple-negative breast cancer therapy in mouse models. Int J Mol Sci. (2018) 19(4):925. doi: 10.3390/ijms19040925
83. Yong K, Brechbiel MW. Towards translation of 212Pb as a clinical therapeutic; getting the lead in!. Dalton Trans. (2011) 40(23):6068–76. doi: 10.1039/c0dt01387k
84. Juzeniene A, Bernoulli J, Suominen M, Halleen J, Larsen RH. Antitumor activity of novel bone-seeking, alpha-emitting (224)Ra-solution in a breast cancer skeletal metastases model. Anticancer Res. (2018) 38(4):1947–55. doi: 10.21873/anticanres.12432
85. Gudkov SV, Shilyagina NY, Vodeneev VA, Zvyagin AV. Targeted radionuclide therapy of human tumors. Int J Mol Sci. (2015) 17(1):33. doi: 10.3390/ijms17010033
86. Hilaris BS, Henschke UK, Holt JG. Clinical experience with long half-life and low-energy encapsulated radioactive sources in cancer radiation theapy. Radiology. (1968) 91(6):1163–7. doi: 10.1148/91.6.1163
87. Wang K, Tepper JE. Radiation therapy-associated toxicity: etiology, management, and prevention. CA Cancer J Clin. (2021) 71(5):437–54. doi: 10.3322/caac.21689
88. Parihar AS, Chopra S, Prasad V. Nephrotoxicity after radionuclide therapies. Transl Oncol. (2022) 15(1):101295. doi: 10.1016/j.tranon.2021.101295
89. Kesavan M, Turner JH. Myeloid toxicity of radionuclide cancer therapy. Cancer Biother Radiopharm. (2022) 37(3):164–72. doi: 10.1089/cbr.2021.0286
90. Sofou S. Radionuclide carriers for targeting of cancer. Int J Nanomedicine. (2008) 3(2):181–99. doi: 10.2147/IJN.S2736
91. Zhu X, Palmer MR, Makrigiorgos GM, Kassis AI. Solid-tumor radionuclide therapy dosimetry: new paradigms in view of tumor microenvironment and angiogenesis. Med Phys. (2010) 37(6):2974–84. doi: 10.1118/1.3431999
92. Lubberink M, Wilking H, Ost A, Ilan E, Sandstrom M, Andersson C, et al. In vivo instability of (177)Lu-DOTATATE during peptide receptor radionuclide therapy. J Nucl Med. (2020) 61(9):1337–40. doi: 10.2967/jnumed.119.237818
93. Tolmachev V, Vorobyeva A. Radionuclides in diagnostics and therapy of malignant tumors: new development. Cancers. (2022) 14(2):297. doi: 10.3390/cancers14020297
94. Tamborino G, Perrot Y, De Saint-Hubert M, Struelens L, Nonnekens J, Jong M, et al. Modeling early radiation DNA damage occurring during (177)Lu-DOTATATE radionuclide therapy. J Nucl Med. (2022) 63(5):761–9. doi: 10.2967/jnumed.121.262610
95. Filippi L, Palumbo B, Bagni O, Frantellizzi V, De Vincentis G, Schillaci O. DNA damage repair defects and targeted radionuclide therapies for prostate cancer: does mutation really matter? A systematic review. Life. (2022) 13(1):55. doi: 10.3390/life13010055
96. Howard BA, James OG, Perkins JM, Pagnanelli RA, Borges-Neto S, Reiman RE. A practical method of I-131 thyroid cancer therapy dose optimization using estimated effective renal clearance. SAGE Open Med Case Rep. (2017) 5:2050313X17745203. doi: 10.1177/2050313X17745203
97. Madsen MT, Menda Y, O'Dorisio TM, O'Dorisio MS. Technical note: single time point dose estimate for exponential clearance. Med Phys. (2018) 45(5):2318–24. doi: 10.1002/mp.12886
98. Sgouros G, Hobbs R, Josefsson A. Dosimetry and radiobiology of alpha-particle emitting radionuclides. Curr Radiopharm. (2018) 11(3):209–14. doi: 10.2174/1874471011666180426130058
99. Cannan WJ, Pederson DS. Mechanisms and consequences of double-strand DNA break formation in chromatin. J Cell Physiol. (2016) 231(1):3–14. doi: 10.1002/jcp.25048
100. Tranel J, Feng FY, James SS, Hope TA. Effect of microdistribution of alpha and beta-emitters in targeted radionuclide therapies on delivered absorbed dose in a GATE model of bone marrow. Phys Med Biol. (2021) 66(3):035016. doi: 10.1088/1361-6560/abd3ef
101. Vinod N, Kim JH, Choi S, Lim I. Combination of (131)I-trastuzumab and lanatoside C enhanced therapeutic efficacy in HER2 positive tumor model. Sci Rep. (2021) 11(1):12871. doi: 10.1038/s41598-021-92460-0
102. Feng Y, Meshaw R, McDougald D, Zhou Z, Zhao XG, Jannetti SA, et al. Evaluation of an (131)I-labeled HER2-specific single domain antibody fragment for the radiopharmaceutical therapy of HER2-expressing cancers. Sci Rep. (2022) 12(1):3020. doi: 10.1038/s41598-022-07006-9
103. Lee J, Park YH. Trastuzumab deruxtecan for HER2+advanced breast cancer. Future Oncol. (2022) 18(1):7–19. doi: 10.2217/fon-2021-0550
104. Cedrowska E, Pruszynski M, Gaweda W, Zuk M, Krysinski P, Bruchertseifer F, et al. Trastuzumab conjugated superparamagnetic iron oxide nanoparticles labeled with (225)Ac as a perspective tool for combined alpha-radioimmunotherapy and magnetic hyperthermia of HER2-positive breast cancer. Molecules. (2020) 25(5):1025. doi: 10.3390/molecules25051025
105. Kawashima H. Radioimmunotherapy: a specific treatment protocol for cancer by cytotoxic radioisotopes conjugated to antibodies. Sci World J. (2014) 2014:492061. doi: 10.1155/2014/492061
106. Larson SM, Carrasquillo JA, Cheung NK, Press OW. Radioimmunotherapy of human tumours. Nat Rev Cancer. (2015) 15(6):347–60. doi: 10.1038/nrc3925
107. Altunay B, Morgenroth A, Beheshti M, Vogg A, Wong NCL, Ting HH, et al. HER2-directed Antibodies, affibodies and nanobodies as drug-delivery vehicles in breast cancer with a specific focus on radioimmunotherapy and radioimmunoimaging. Eur J Nucl Med Mol Imaging. (2021) 48(5):1371–89. doi: 10.1007/s00259-020-05094-1
108. Erber R, Hartmann A. Understanding PD-L1 testing in breast cancer: a practical approach. Breast Care. (2020) 15(5):481–90. doi: 10.1159/000510812
109. Han Y, Liu D, Li L. PD-1/PD-L1 pathway: current researches in cancer. Am J Cancer Res. (2020) 10(3):727–42.32266087
110. Doroshow DB, Bhalla S, Beasley MB, Sholl LM, Kerr KM, Gnjatic S, et al. PD-L1 as a biomarker of response to immune-checkpoint inhibitors. Nat Rev Clin Oncol. (2021) 18(6):345–62. doi: 10.1038/s41571-021-00473-5
111. Ai L, Xu A, Xu J. Roles of PD-1/PD-L1 pathway: signaling, cancer, and beyond. Adv Exp Med Biol. (2020) 1248:33–59. doi: 10.1007/978-981-15-3266-5_3
112. Huang Q, Wu X, Wang Z, Chen X, Wang L, Lu Y, et al. The primordial differentiation of tumor-specific memory CD8(+) T cells as bona fide responders to PD-1/PD-L1 blockade in draining lymph nodes. Cell. (2022) 185(22):4049–66.e25. doi: 10.1016/j.cell.2022.09.020
113. Dolina JS, Van Braeckel-Budimir N, Thomas GD, Salek-Ardakani S. CD8(+) T cell exhaustion in cancer. Front Immunol. (2021) 12:715234. doi: 10.3389/fimmu.2021.715234
114. Brahmer JR, Tykodi SS, Chow LQ, Hwu WJ, Topalian SL, Hwu P, et al. Safety and activity of anti-PD-L1 antibody in patients with advanced cancer. N Engl J Med. (2012) 366(26):2455–65. doi: 10.1056/NEJMoa1200694
115. Guo LM, Ding GF, Xu WC, Ge H, Jiang Y, Lu YF. Anti-PD-L1 antibody enhances T cell immune responses and reduces resistance of breast cancer cells to radiotherapy. Oxid Med Cell Longev. (2022) 2022:5938688. doi: 10.1155/2022/5938688
116. Chan C, Prozzo V, Aghevlian S, Reilly RM. Formulation of a kit under good manufacturing practices (GMP) for preparing [(111)In]In-BnDTPA-trastuzumab-NLS injection: a theranostic agent for imaging and meitner-auger electron (MAE) radioimmunotherapy of HER2-positive breast cancer. EJNMMI Radiopharm Chem. (2022) 7(1):33. doi: 10.1186/s41181-022-00186-9
117. Zhang C, Zhang Y, Liang M, Shi X, Jun Y, Fan L, et al. Near-infrared upconversion multimodal nanoparticles for targeted radionuclide therapy of breast cancer lymphatic metastases. Front Immunol. (2022) 13:1063678. doi: 10.3389/fimmu.2022.1063678
118. Ambur Sankaranarayanan R, Florea A, Allekotte S, Vogg ATJ, Maurer J, Schafer L, et al. PARP targeted auger emitter therapy with [(125)I]PARPi-01 for triple-negative breast cancer. EJNMMI Res. (2022) 12(1):60. doi: 10.1186/s13550-022-00932-9
119. Coleman R, Brown J, Rathbone E, Flanagan L, Reid A, Kendall J, et al. CApecitabine plus Radium-223 (Xofigo) in breast cancer patients with BONe metastases (CARBON): study protocol for a phase IB/IIA randomised controlled trial. Trials. (2020) 21(1):89. doi: 10.1186/s13063-019-3643-6
120. Effect of radium-223 on breast cancer bone metastases in mice. Bonekey Rep. (2013) 2:457. doi: 10.1038/bonekey.2013.191
121. Winter M, Coleman R, Kendall J, Palmieri C, Twelves C, Howell S, et al. A phase IB and randomised phase IIA trial of CApecitabine plus Radium-223 (Xofigo) in breast cancer patients with BONe metastases: CARBON trial results. J Bone Oncol. (2022) 35:100442. doi: 10.1016/j.jbo.2022.100442
122. Robinson RG, Preston DF, Baxter KG, Dusing RW, Spicer JA. Clinical experience with strontium-89 in prostatic and breast cancer patients. Semin Oncol. (1993) 20(3 Suppl 2):44–8.8503027
123. Hansen DV, Holmes ER, Catton G, Thorne DA, Chadwick DH, Schmutz DA. Strontium-89 therapy for painful osseous metastatic prostate and breast cancer. Am Fam Physician. (1993) 47(8):1795–800.7684554
124. Yamada K, Kaise H, Taguchi T, Horiguchi J, Takao S, Suzuki M, et al. Strontium-89 plus zoledronic acid versus zoledronic acid for patients with painful bone metastatic breast cancer. J Bone Miner Metab. (2022) 40(6):998–1006. doi: 10.1007/s00774-022-01366-y
125. Serafini AN. Samarium Sm-153 lexidronam for the palliation of bone pain associated with metastases. Cancer. (2000) 88(12):2934–9. doi: 10.1002/1097-0142(20000615)88:12+<2934::AID-CNCR9>3.0.CO;2-S10898337
126. Gallicchio R, Giacomobono S, Nardelli A, Pellegrino T, Simeon V, Gattozzi D, et al. Palliative treatment of bone metastases with samarium-153 EDTMP at onset of pain. J Bone Miner Metab. (2014) 32(4):434–40. doi: 10.1007/s00774-013-0507-0
127. Shen H, Huang H, Jiang Z. Nanoparticle-based radiosensitization strategies for improving radiation therapy. Front Pharmacol. (2023) 14:1145551. doi: 10.3389/fphar.2023.1145551
128. Llop J, Lammers T. Nanoparticles for cancer diagnosis, radionuclide therapy and theranostics. ACS Nano. (2021) 15(11):16974–81. doi: 10.1021/acsnano.1c09139
129. Tian L, Chen Q, Yi X, Wang G, Chen J, Ning P, et al. Radionuclide I-131 labeled albumin-paclitaxel nanoparticles for synergistic combined chemo-radioisotope therapy of cancer. Theranostics. (2017) 7(3):614–23. doi: 10.7150/thno.17381
130. Facca VJ, Cai Z, Gopal NEK, Reilly RM. Panitumumab-DOTA-(111)In: an epidermal growth factor receptor targeted theranostic for SPECT/CT imaging and meitner-auger electron radioimmunotherapy of triple-negative breast cancer. Mol Pharm. (2022) 19(10):3652–63. doi: 10.1021/acs.molpharmaceut.2c00457
131. Huang Y, Yang Z, Li F, Zhao H, Li C, Yu N, et al. (64)Cu/(177)Lu-DOTA-diZD, a small-molecule-based theranostic pair for triple-negative breast cancer. J Med Chem. (2021) 64(5):2705–13. doi: 10.1021/acs.jmedchem.0c01957
132. Yook S, Cai Z, Lu Y, Winnik MA, Pignol JP, Reilly RM. Radiation nanomedicine for EGFR-positive breast cancer: panitumumab-modified gold nanoparticles complexed to the beta-particle-emitter, (177)Lu. Mol Pharm. (2015) 12(11):3963–72. doi: 10.1021/acs.molpharmaceut.5b00425
133. Zhao L, Liu C, Xing Y, He J, O'Doherty J, Huang W, et al. Development of a (99 m)Tc-labeled single-domain antibody for SPECT/CT assessment of HER2 expression in breast cancer. Mol Pharm. (2021) 18(9):3616–22. doi: 10.1021/acs.molpharmaceut.1c00569
134. Song H, Sgouros G. Radioimmunotherapy of solid tumors: searching for the right target. Curr Drug Deliv. (2011) 8(1):26–44. doi: 10.2174/156720111793663651
135. Boswell CA, Brechbiel MW. Development of radioimmunotherapeutic and diagnostic antibodies: an inside-out view. Nucl Med Biol. (2007) 34(7):757–78. doi: 10.1016/j.nucmedbio.2007.04.001
136. Richman CM, DeNardo SJ, O'Grady LF, DeNardo GL. Radioimmunotherapy for breast cancer using escalating fractionated doses of 131I-labeled chimeric L6 antibody with peripheral blood progenitor cell transfusions. Cancer Res. (1995) 55(23):5916s–20s.7493370
137. DeNardo SJ, O'Grady LF, Richman CM, DeNardo GL. Overview of radioimmunotherapy in advanced breast cancer using I-131 chimeric L6. Adv Exp Med Biol. (1994) 353:203–11. doi: 10.1007/978-1-4615-2443-4_19
138. Denardo SJ, O'Grady LF, Richman CM, Goldstein DS, O'Donnell RT, Denardo DA, et al. Radioimmunotherapy for advanced breast cancer using I-131-ChL6 antibody. Anticancer Res. (1997) 17(3B):1745–51.9179228
139. Wang X, Osada T, Wang Y, Yu L, Sakakura K, Katayama A, et al. CSPG4 Protein as a new target for the antibody-based immunotherapy of triple-negative breast cancer. J Natl Cancer Inst. (2010) 102(19):1496–512. doi: 10.1093/jnci/djq343
140. Ilieva KM, Cheung A, Mele S, Chiaruttini G, Crescioli S, Griffin M, et al. Chondroitin sulfate proteoglycan 4 and its potential as an antibody immunotherapy target across different tumor types. Front Immunol. (2017) 8:1911. doi: 10.3389/fimmu.2017.01911
141. Heesch A, Ortmanns L, Maurer J, Stickeler E, Sahnoun SEM, Mottaghy FM, et al. The potential of PSMA as a vascular target in TNBC. Cells. (2023) 12(4):551. doi: 10.3390/cells12040551
142. Morgenroth A, Tinkir E, Vogg ATJ, Sankaranarayanan RA, Baazaoui F, Mottaghy FM. Targeting of prostate-specific membrane antigen for radio-ligand therapy of triple-negative breast cancer. Breast Cancer Res. (2019) 21(1):116. doi: 10.1186/s13058-019-1205-1
143. Hernandez R, Grudzinski JJ, Aluicio-Sarduy E, Massey CF, Pinchuk AN, Bitton AN, et al. (177)Lu-NM600 targeted radionuclide therapy extends survival in syngeneic murine models of triple-negative breast cancer. J Nucl Med. (2020) 61(8):1187–94. doi: 10.2967/jnumed.119.236265
144. Faust JR, Hamill D, Kolb EA, Gopalakrishnapillai A, Barwe SP. Mesothelin: an immunotherapeutic target beyond solid tumors. Cancers. (2022) 14(6):1550. doi: 10.3390/cancers14061550
145. Wang Y, Wang L, Li D, Wang HB, Chen QF. Mesothelin promotes invasion and metastasis in breast cancer cells. J Int Med Res. (2012) 40(6):2109–16. doi: 10.1177/030006051204000608
146. Hussein NH, Eissa RA, de Bruyn M, El Tayebi HM. NEAT1: culprit lncRNA linking PIG-C, MSLN, and CD80 in triple-negative breast cancer. Life Sci. (2022) 299:120523. doi: 10.1016/j.lfs.2022.120523
147. Hagemann UB, Ellingsen C, Schuhmacher J, Kristian A, Mobergslien A, Cruciani V, et al. Mesothelin-targeted thorium-227 conjugate (MSLN-TTC): preclinical evaluation of a new targeted alpha therapy for mesothelin-positive cancers. Clin Cancer Res. (2019) 25(15):4723–34. doi: 10.1158/1078-0432.CCR-18-3476
148. Yin G, Zeng B, Peng Z, Liu Y, Sun L, Liu C. Synthesis and application of 131I-fulvestrant as a targeted radiation drug for endocrine therapy in human breast cancer. Oncol Rep. (2018) 39(3):1215–26. doi: 10.3892/or.2018.6212
149. Bicak M, Luckerath K, Kalidindi T, Phelps ME, Strand SE, Morris MJ, et al. Genetic signature of prostate cancer mouse models resistant to optimized hK2 targeted alpha-particle therapy. Proc Natl Acad Sci U S A. (2020) 117(26):15172–81. doi: 10.1073/pnas.1918744117
150. Minnix M, Adhikarla V, Caserta E, Poku E, Rockne R, Shively JE, et al. Comparison of CD38-targeted alpha- versus beta-radionuclide therapy of disseminated multiple myeloma in an animal model. J Nucl Med. (2021) 62(6):795–801. doi: 10.2967/jnumed.120.251983
151. Dauer LT, Thornton RH, Hay JL, Balter R, Williamson MJ, St Germain J. Fears, feelings, and facts: interactively communicating benefits and risks of medical radiation with patients. AJR Am J Roentgenol. (2011) 196(4):756–61. doi: 10.2214/AJR.10.5956
152. Higgins MJ, Baselga J. Targeted therapies for breast cancer. J Clin Invest. (2011) 121(10):3797–803. doi: 10.1172/JCI57152
153. Mohamed A, Krajewski K, Cakar B, Ma CX. Targeted therapy for breast cancer. Am J Pathol. (2013) 183(4):1096–112. doi: 10.1016/j.ajpath.2013.07.005
154. Liyanage PY, Hettiarachchi SD, Zhou Y, Ouhtit A, Seven ES, Oztan CY, et al. Nanoparticle-mediated targeted drug delivery for breast cancer treatment. Biochim Biophys Acta Rev Cancer. (2019) 1871(2):419–33. doi: 10.1016/j.bbcan.2019.04.006
155. Weaver JW, Zhang J, Rojas J, Musich PR, Yao Z, Jiang Y. The application of exosomes in the treatment of triple-negative breast cancer. Front Mol Biosci. (2022) 9:1022725. doi: 10.3389/fmolb.2022.1022725
156. Sun X, Liu K, Lu S, He W, Du Z. Targeted therapy and immunotherapy for heterogeneous breast cancer. Cancers. (2022) 14(21):5456. doi: 10.3390/cancers14215456
157. Bockisch A. Matched pairs for radionuclide-based imaging and therapy. Eur J Nucl Med Mol Imaging. (2011) 38(1):S1–3. doi: 10.1007/s00259-011-1780-6
158. Yan Y, Cheng X, Li L, Zhang R, Zhu Y, Wu Z, et al. A novel small molecular antibody, HER2-nanobody, inhibits tumor proliferation in HER2-positive breast cancer cells in vitro and in vivo. Front Oncol. (2021) 11:669393. doi: 10.3389/fonc.2021.669393
159. Yang EY, Shah K. Nanobodies: next generation of cancer diagnostics and therapeutics. Front Oncol. (2020) 10:1182. doi: 10.3389/fonc.2020.01182
160. Loke KS, Padhy AK, Ng DC, Goh AS, Divgi C. Dosimetric considerations in radioimmunotherapy and systemic radionuclide therapies: a review. World J Nucl Med. (2011) 10(2):122–38. doi: 10.4103/1450-1147.89780
161. Ljungberg M, Sjogreen Gleisner K. Personalized dosimetry for radionuclide therapy using molecular imaging tools. Biomedicines. (2016) 4(4):25. doi: 10.3390/biomedicines4040025
162. Capala J, Graves SA, Scott A, Sgouros G, James SS, Zanzonico P, et al. Dosimetry for radiopharmaceutical therapy: current practices and commercial resources. J Nucl Med. (2021) 62(3):3S–11S. doi: 10.2967/jnumed.121.262749
163. Finocchiaro D, Berenato S, Bertolini V, Castellani G, Lanconelli N, Versari A, et al. Comparison of different calculation techniques for absorbed dose assessment in patient specific peptide receptor radionuclide therapy. PLoS One. (2020) 15(8):e0236466. doi: 10.1371/journal.pone.0236466
Keywords: radionuclide, early diagnosis, breast cancer, tumor, therapy
Citation: Musket A, Davern S, Elam BM, Musich PR, Moorman JP and Jiang Y (2024) The application of radionuclide therapy for breast cancer. Front. Nucl. Med. 3:1323514. doi: 10.3389/fnume.2023.1323514
Received: 18 October 2023; Accepted: 27 December 2023;
Published: 10 January 2024.
Edited by:
Egesta Lopci, University of Milan, ItalyReviewed by:
Aiko Yamaguchi, University of Texas MD Anderson Cancer Center, United States© 2024 Musket, Davern, Elam, Musich, and Moorman and Jiang. This is an open-access article distributed under the terms of the Creative Commons Attribution License (CC BY). The use, distribution or reproduction in other forums is permitted, provided the original author(s) and the copyright owner(s) are credited and that the original publication in this journal is cited, in accordance with accepted academic practice. No use, distribution or reproduction is permitted which does not comply with these terms.
*Correspondence: Yong Jiang, amlhbmd5MkBldHN1LmVkdQ==
Disclaimer: All claims expressed in this article are solely those of the authors and do not necessarily represent those of their affiliated organizations, or those of the publisher, the editors and the reviewers. Any product that may be evaluated in this article or claim that may be made by its manufacturer is not guaranteed or endorsed by the publisher.
Research integrity at Frontiers
Learn more about the work of our research integrity team to safeguard the quality of each article we publish.