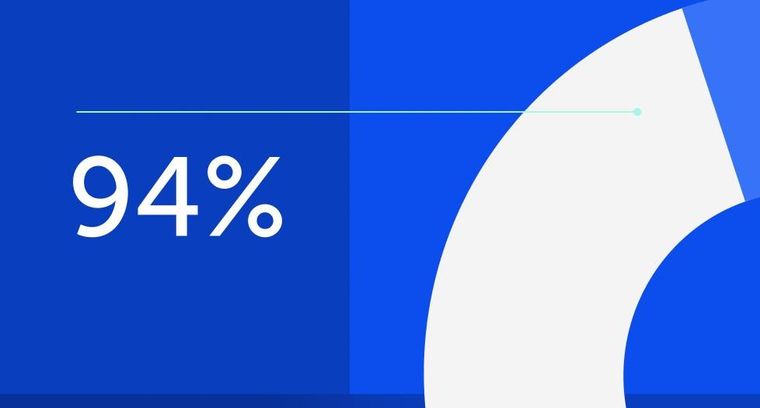
94% of researchers rate our articles as excellent or good
Learn more about the work of our research integrity team to safeguard the quality of each article we publish.
Find out more
REVIEW article
Front. Nucl. Med., 21 September 2023
Sec. Dosimetry and Radiation Safety
Volume 3 - 2023 | https://doi.org/10.3389/fnume.2023.1225034
This article is part of the Research TopicInsights in Dosimetry and Radiation SafetyView all 4 articles
Ionizing radiation and microgravity are two considerable health risks encountered during deep space exploration. Both have deleterious effects on the human body. On one hand, weightlessness is known to induce a weakening of the immune system, delayed wound healing and musculoskeletal, cardiovascular, and sensorimotor deconditioning. On the other hand, radiation exposure can lead to long-term health effects such as cancer and cataracts as well as have an adverse effect on the central nervous and cardiovascular systems. Ionizing radiation originates from three main sources in space: galactic cosmic radiation, solar particle events and solar winds. Furthermore, inside the spacecraft and inside certain space habitats on Lunar and Martian surfaces, the crew is exposed to intravehicular radiation, which arises from nuclear reactions between space radiation and matter. Besides the approaches already in use, such as radiation shielding materials (such as aluminium, water or polyethylene), alternative shielding materials (including boron nanotubes, complex hybrids, composite hybrid materials, and regolith) and active shielding (using fields to deflect radiation particles) are being investigated for their abilities to mitigate the effects of ionizing radiation. From a biological point of view, it can be predicted that exposure to ionizing radiation during missions beyond Low Earth Orbit (LEO) will affect the human body in undesirable ways, e.g., increasing the risks of cataracts, cardiovascular and central nervous system diseases, carcinogenesis, as well as accelerated ageing. Therefore, it is necessary to assess the risks related to deep space exploration and to develop mitigation strategies to reduce these risks to a tolerable level. By using biomarkers for radiation sensitivity, space agencies are developing extensive personalised medical examination programmes to determine an astronaut's vulnerability to radiation. Moreover, researchers are developing pharmacological solutions (e.g., radioprotectors and radiomitigators) to proactively or reactively protect astronauts during deep space exploration. Finally, research is necessary to develop more effective countermeasures for use in future human space missions, which can also lead to improvements to medical care on Earth. This review will discuss the risks space travel beyond LEO poses to astronauts, methods to monitor astronauts' health, and possible approaches to mitigate these risks.
With the current desire of space-faring nations to travel back to the Moon, the world will soon turn its attention to Mars once a lunar base has been established. However, with a mission profile unlike anything astronauts have flown before, such a mission will be extremely challenging. It involves traveling 50 million kilometres to reach Mars. The distance between the planets is so large that there will be latency of up to 20 min in voice and data transmissions between mission control on Earth and a base on Mars. As a result, neither the surface habitat nor the systems on board the transit spacecraft will be under the real-time control of the ground support team. The onboard inventory of equipment and supplies needs to be strategically arranged in advance because cargo resupply from Earth will not be possible. The size, quantity, and functionality of onboard equipment will also be restricted by volume, mass, and power parameters. All vehicle systems must be powerful and reliable because there will be less ground supervision. The systems and astronauts must also work independently of ground support. In order to handle the inevitable critical crises that may occur, astronauts will be dependent on their own capabilities and onboard supplies.
Today, we have numerous technological obstacles that prevent us from sending a human expedition to Mars. We will need to devise procedures for utilizing in situ resources before launching the first crew. Future astronauts will need to create some of these consumables from local space-based resources instead of bringing enormous quantities of oxygen, water, and propellant with them from Earth (1). We will require systems to land heavier payloads (up to 40 tonnes of equipment and supplies for a human trip) on planetary surfaces, and we will need ion propulsion systems to shorten journey durations to interplanetary destinations. Before humanity travels into outer space, these and other advancements will be required.
Maintaining the health of the astronauts is considered to be one of the biggest barriers (2) for deep space exploration. It will no longer be possible for ground-based medical professionals to monitor astronaut health as they have in the past, especially in an emergency. A deep space mission cannot be aborted in order to return an injured or unwell crew member to Earth for treatment. Future crews need to be fully trained and capable of managing their own health. Imaging, surgery, emergency treatment, and laboratory examinations of blood, urine, and other biospecimens must all be available as onboard medical resources. There should be at least one physician on the crew who has experience practicing remote medicine.
The environment in outer space is dangerous. As ionizing radiation and microgravity/weightlessness are the two main risks going into space, it is of importance to review the consequences and possible health effects and ways of mitigating those space stresses (3). However, little data are currently available on radiation and microgravity effects. The data gathered pertaining to space radiation are obtained from atomic bomb survivors or those exposed to chronic radiation for medical purposes, whereas the data pertaining to microgravity exposure are based on a combination of data obtained from prior space missions and experiments conducted in simulated microgravity (4, 5).
This article will first focus on a detailed definition and description of cosmic radiation and microgravity. Thereafter, the effects of these risks on health are described. Lastly, several types of protection and countermeasures are reviewed and discussed.
Ionizing radiation is one of the main dangers astronauts face during their missions. Before traveling into deep space, numerous concerns related to radiation must be resolved. Can radiation protection strategies already employed in LEO be modified for use in outer space? Can low atomic weight materials be used in the design of spacecraft destined for deep space in order to protect crews? Could a safe haven compartment offer the crew protection against severe radiation exposure during a significant solar particle event whilst in transit to a planet? Could Martian regolith serve as a lining for underground habitats to provide protection? Will shielding be enough to reduce exposure on its own, or will biological and pharmaceutical countermeasures also be required? This section intends to describe the different types of space radiation and their effects on astronauts.
Radiation is a form of energy that is emitted or transmitted in the form of electromagnetic waves and/or energised particles (6, 7). There are two kinds of radiation, ionizing and non-ionizing radiation. Ionizing radiation has particles that have enough energy to remove electrons from (ionise) atoms or molecules. Non-ionizing radiation may also be dangerous; however, it can be more easily shielded, e.g., by using sunglasses (6, 7). Missions going beyond Earth's magnetosphere will encounter different forms of ionizing radiation. Solar winds or flares are the main source of energetic particles, which are emitted constantly by the Sun. Levels of these solar flares will vary depending on the 11-year cycle of sun activity. These solar particle events (SPE) produce large plasma clouds, which carry highly energetic protons and heavy ions. Moreover, Galactic Cosmic Radiation (GCR), with its origin being from different solar systems, is another type of radiation. GCR contains high energetic ions that move at relativistic speeds (8). Exposure to both GCR and SPE could have a major effect on the health of astronaut crews (3). Different types of space radiations are shown in Figure 1.
Figure 1. Types of ionizing radiation in space: illustration depicting the various types of ionizing radiation present in the space environment. These include solar energetic particles, galactic cosmic rays, ultraviolet radiation, intravehicular radiation and magnetic field. The figure highlights the distinct characteristics of each type of radiation, such as their composition and energy levels, which contribute to their unique effects on biological systems and materials. Created with BioRender.com.
GCR ions, originating from outside our solar system, produce high linear energy transfer (LET) radiation. GCR is composed primarily of protons (87%), helium (12%) and heavier nuclei (1%) (9–11). Those particles that come into contact with biological tissue or other (in)organic materials have sufficient energy to penetrate several centimetres of material (12). When transiting outside of LEO, it has been estimated that a hydrogen ion will pass through every cell nucleus of an astronaut crew member every day, and heavier High (H) atomic number (Z) and high energy (E) (HZE) nuclei every few months (13). These HZE ions pose an enormous threat to human health because of their very high LET values. Shielding is currently used to reduce the radiation penetrating the spacecraft, but this means of protection is limited by the practical capabilities of current launch systems (3).
Solar energetic particles, which are emitted by solar flares and Coronal Mass Ejections (14), produce large quantities of energetic protons (9–11). Their energies are proportional to the sun's peak activity when equatorial sunspot activity is at its largest stage. The phase (11-year cycle) of the sun during an SPE has no effect on its intensity. Furthermore, the largest measured SPEs have occurred during off-peak periods of the 11-year cycle (3). Therefore, spacecraft designers must still consider this issue and ensure that these energies are shielded by the spacecrafts' hulls during LEO missions. Nevertheless, there is a possibility that astronauts are exposed to this form of radiation during extravehicular activities (EVA) and in poorly shielded environments (13).
The health of astronauts is affected not only by SPE and GCR particles, but also by the interaction of these energetic particles with the spacecraft structure. When these particles pass through the spacecraft shielding, a nuclear fission reaction occurs in most cases, generating a plethora of secondary radiation composed of beta particles, x-rays, gamma rays, neutrons, protons, alpha particles, and heavy-charged particles (3).
Ionizing radiation will be present in higher fluences beyond the Earth's protective magnetosphere. Astronauts who are exposed to galactic cosmic radiation for an extended period may develop cataracts as well as delayed and impaired wound healing and degenerative tissue disorders. Cancer could also develop with a higher incidence at a later stage in life, and genetic alterations are another possibility. Finally, a significant SPE could cause acute radiation sickness, possibly death. The risks encountered by astronauts exposed to cosmic radiation are outlined in Figure 2 and are discussed below (15, 16).
Figure 2. Health effects of space radiation: this illustrative figure offers a comprehensive visualization of the intricate and multifaceted health effects induced by space radiation exposure. The diagram indicates the major physiological risks that can occur in response to ionizing radiation encountered in space environments. Ionizing radiation can cause single-strand breaks, double-strand breaks, and oxidative damage to DNA. Starting from the top, the figure emphasizes the potential for genetic mutations and disruptions to DNA repair mechanisms, contributing to long-term health risks such as cancer. Transitioning to the tissue and organ level, the figure illustrates how cellular damage and responses can lead to tissue dysfunction. Emphasis is placed the potential for acute effects, such as radiation syndromes, as well as chronic effects such as degenerative tissue effects and impaired organ function. Finally, the figure highlights the link between these immediate and systemic effects and their long-term health consequences. The illustration emphasizes the increased risk of cancer, cardiovascular diseases, neurodegenerative disorders, and other chronic conditions that astronauts might face over their lifetime. Created with BioRender.com.
The high-LET radiation of GCR particles can damage biomolecules, organelles, and other cellular structures. Studies have shown that increased exposure to ionizing radiation altered tissues in a manner similar to aging (3). In contrast to high-LET radiation, studies have demonstrated a relationship between high doses of low-LET radiation and heart diseases, cataracts, and premature aging, amongst others (17, 18). However, the results of studies on low doses of low-LET radiation have not always been consistent. At the basis of these degenerative diseases lies the induction of DNA damage. When a DNA molecule is damaged by ionizing radiation, e.g., by losing a base or base modification such as oxidation, cells will try to repair it by using repair systems in order to maintain integrity and functionality. When DNA replication occurs before repair ends, or when repair was unsuccessful at restoring the DNA, this can result in apoptosis, a form of programmed cell death, but also in mutations that can possibly lead to genome instability and ultimately carcinogenesis (19, 20). Studies relating higher doses of radiation in space to carcinogenesis are still in their infancy. The majority of these studies used animals, cell models or atomic bomb survivors as their sample population to investigate the effects of high doses of radiation. However, the effects of space radiation on carcinogenesis remain unclear due to the small sample size of astronauts in space and wide range of time and dose intervals (21).
Radiation can interfere with cognitive functions (22–25). Studies at the Space Radiation Laboratory at NASA demonstrated cognitive health risks during Beyond Low Earth Orbit (BLEO) missions. Other research has demonstrated that low doses of GCR radiation can affect learning and memory, and in drastic cases can even kill neuronal cells (26). Therefore, executive functions in astronauts may deteriorate over time (27).
A study by Kennedy et al. (28) investigated the effects of high doses of SPE on blood cells, immune system parameters and skin, using different animal models (29, 30). The damage to the DNA of larger species was irreversible and adverse effects on the skin, deeper organs, and tissues were observed. Furthermore, lower doses of SPE particles also induced a decline in the immune system's performance. Fatigue, emesis, and retching are some well-known consequences of exposure to high doses of radiation (3).
Due to intravehicular radiation, susceptibility to infections is also increased, together with delayed wound healing and even a decreased survival chance. However, studies revealed that the susceptibility to diseases after exposure to radiation depends on the individual. Hence, further research needs to take this inter-individual variation into account and is discussed later in this paper (31, 32).
In space, astronauts are subjected to a variety of gravity conditions that have an impact on fundamental biological functions. Most human spaceflights have taken place in LEO, which is between 160 and 2,000 kilometres above the surface of the planet. This region is still shielded from charged particle space radiation (33). On Earth, gravity pulls objects towards the centre of the planet. This is known as normal terrestrial gravity (1 g). Earth's gravity's pulling nature is resisted by the Earth's surface, and it is this combination of force that determines the structure of our musculoskeletal system and how it supports our body. Therefore, contact forces that provide a variety of mechanical stimulation that is necessary for the operation of numerous physiological systems are constantly applied to organisms on Earth (33).
The effects of physical exercise stresses on the weight-bearing skeleton are a clear example of how these mechanical contact forces affect the human body. For the body to be able to resist these greater stresses, an increased load during weight-bearing exercise induces increased musculoskeletal growth.
Furthermore, the Earth's gravitational pull is also felt by spacecraft near to Earth. This enables spacecraft to orbit around the planet. Astronauts and the spacecraft's cargo are in a state of free fall while in LEO orbit around the Earth (33), and experience microgravity with a gravitational force of about 1 × 10–6 g. Many of the physiological problems that astronauts experience in space, such as motion sickness and otolith dysfunction as well as cardiovascular, bone, and muscle deterioration, are caused by the absence of normal terrestrial gravity (1 g) and the ensuing lack of mechanical stimulation of cells and tissues. The environment of spaceflight during astronaut missions has revealed numerous rapid tissue degenerative effects. These include bone and muscle loss, cardiovascular deconditioning, delayed wound healing and bone fracture healing, and impaired immune function (34).
It is quasi-impossible, on Earth, to study effects in the absence of gravity, also referred to as weightlessness. On Earth, therefore, simulation models are used to replicate microgravity. By using such simulation models, it is possible to investigate the effects of microgravity on different cellular systems. The primary device used to simulate microgravity is the rotating-wall vessel also known as the slow rotating clinostat or rotating wall bioreactor (RWV; Figure 3).
Figure 3. The rotary cell culture system containing the rotating-wall vessel bioreactor: schematic diagram illustrating the rotary cell culture system incorporating the rotating-wall vessel (RWV) bioreactor. The figure provides an overview of the bioreactor's design and its role in simulating microgravity conditions. It showcases how the RWV facilitates three-dimensional cell growth and enables studies on cellular responses under simulated weightlessness. Created with BioRender.com.
In a controlled setting where gravitational forces are either reduced to a minimum or completely eliminated by continuous rotation, this laboratory apparatus is intended to imitate microgravity conditions for biological experiments. The apparatus consists of a cylindrical container that accommodates biological materials or cell cultures. An oxygen- and nutrient-rich growth medium that is inside the vessel provides the cells with what they require to live and develop. The rotating wall vessel's essential characteristic is its steady, continuous revolution. The biological samples inside the vessel undergo a constant shift in gravitational orientation as the RWV rotates. The samples enter a state of “free fall” as a result of this rotation, simulating near-weightlessness as the force of gravity is evenly dispersed throughout the cells (35–37). Another device is the Random Positioning Machine, which rotates biological samples along two distinct axes in a random pattern that removes any particular orientation (of the samples) in space (38). On Earth, this can be used to counteract gravity (39).
The revolving wall vessel is used by researchers to examine a variety of biological processes, including gene expression, immunological response, cell signaling, tissue development, and cell proliferation. It aids in the understanding of how microgravity affects cellular function and has possible implications for space travel, medicine, and regenerative medicine. It also sheds light on how organisms adapt to space conditions.
A lack of gravity can have negative effects on human physiology, which are listed in Figure 4. The impact of microgravity can be observed on the musculoskeletal, cardiovascular, sensory-motor, and immune systems as well as on a cellular level. The following section details the impact on these systems.
Figure 4. Effects of microgravity on health: comprehensive overview of the effects of microgravity on human health. The figure delves into physiological changes. By visualizing the impact on different bodily systems, the figure highlights the challenges posed by prolonged space missions on astronauts’ well-being. Created with BioRender.com.
Studies have shown that microgravity can change bone tissue (40). This alteration in bone tissue can lead to a decrease in bone mass, an increase in bone resorption, and, accelerate osteoporosis (41–45). The function of the skeleton is three-fold: delivering mechanical integrity for movements and protection as well as controlling mineral homeostasis for metabolic paths (46). Under normal terrestrial conditions, mechanical stresses are exerted during bone formation. These stresses do not exist in microgravity, and their absence results in uncoupling of the bone formation and resorption mechanisms, leading to an increase in bone resorption (47, 48).
Changes in the muscle tissue, such as muscle atrophy, alterations in protein metabolism and stiffness, have also been observed (49, 50–53). The effects on antigravity muscles were deemed the most profound, as these muscles play a crucial role under standard terrestrial conditions. For example, the quadriceps help the body to remain upright. Moreover, amongst these antigravity muscles, the extensor muscles show a greater impact due to microgravity than the flexors. However, when considering long duration flights in space, all skeletal muscles are affected. Kalb & Solomon categorized the factors that cause a decrease in muscle functions into three groups (54). Firstly, the removal of antigravity load in the antigravity muscles leads to a reduction in muscle gene transcription and protein translation. The second factor is a decrease in neural drive. Microgravity changes the control and coordination resulting in a decreased muscle power. Thirdly, systematic factors such as hormone alterations and changes in metabolism contribute to a loss of muscle mass. Finally, upon returning to Earth, astronauts experience a “reloading process”. They encounter weakness and delayed onset muscle soreness due to the returning gravity, which results in muscle damage. This could be problematic when performing interplanetary missions (47). Therefore, astronauts must exercise in space to ensure muscle integrity.
Research has indicated a reduction in astronauts' heart rates when in space, related to the lower vascular resistance in space. Furthermore, blood and plasma volumes also change during spaceflight. Facial oedema and thinning of legs are observed because of the redistribution of some body fluids to the head. Moreover, plasma and blood volumes are decreased. This can be traced back to several factors. Firstly, a depressed urge to drink reduces fluid intake, leading to a reduction in urinary output. The diminished blood volume experienced in space arises from three factors. Firstly, a negative equilibrium caused by a decreased fluid intake coupled with a comparatively modest reduction in urine output. Secondly, fluid redistributions from the intravascular to the interstitial space are a consequence of diminished transmural pressure. The reduced transmural pressure is caused by a decrease in compression of all tissues, notably the thorax cage, from the reduced gravitational forces. Lastly, fluid transfers from the intravascular to the muscle interstitial space occur due to reduced muscular tension required for sustaining body posture (55). When astronauts return to Earth, post-flight orthostatic intolerance often occurs. Orthostatic intolerances are signs of the body's inability to compensate for the fast translocation of blood from the upper body to the lower body. Reduction in vasoconstrictor responses and other alterations during spaceflights, such as cardiovascular de-conditioning and hypovolemia (low circulation of blood volumes), can also be the cause of this intolerance. It is expected that similar disorders will occur during interplanetary missions. However, no studies exist that show whether reduced gravitational fields, such as that on Mars, could worsen or ameliorate the effects observed in microgravity. Further research is needed to guarantee the safety of astronauts during interplanetary missions (47).
Primarily due to microgravity, the anatomy of the human brain is known to change during spaceflight, according to several studies (56–60). Spaceflight causes the brain to shift upward within the skull (61, 62). Grey matter volume increases in the top of the brain and reduces near the base of the brain as a result (57, 63).
The displacement of cerebral fluid, including extracellular free water such as cerebrospinal fluid, occurs in conjunction with post-flight grey matter shifts. Following a spaceflight, there is an increase in cerebral fluid volume near the base of the brain and a decrease in cerebral fluid volume towards the top of the brain. Additionally, during spaceflight, the ventricles expand, with reported average volume increases ranging from 11% to 25% (56–60).
However, the spaceflight experience of current and past astronauts varies greatly. The normal duration of a space mission is between 2 weeks and 1 year, and there are both experienced and inexperienced astronauts (with various numbers of past flights and inter-mission gaps). It is not well understood if or how these individual variations in past flying experiences are related to the structural brain changes and intracranial fluid shifts brought on by spaceflight.
When people are exposed to a new gravity environment, their brains centrally reinterpret information from various sensory sources to create a sensorimotor state that is suitable for their motor activities and spatial orientation perceptions in the new environment (64, 65). The completion of this fundamental adaptation can take weeks of constant exposure to novel situations, yet the temporal evolution of this adaptation is not instantaneous (66–68).
Astronauts who are newly exposed to microgravity may experience perceptual and functional deficiencies, such as errors in perception of spatial orientation and changes in locomotor and postural control (69). The capacity of crew members to carry out mission-critical operational duties, including controlling vehicles and using other complex systems, may be impacted by this sensorimotor impairment (70). Furthermore, it is believed that space motion sickness (SMS) is caused primarily by an adaptive central nervous system (CNS) that mistakenly interprets vestibular signals of self-motion as sensory information about self-orientation (71, 72).
The sensory-motor system consists of sensory and motor neurons. Of all systems within the human body, it is one of the most strongly affected by microgravity (73, 74). To adapt to microgravity, a reorganization of the central nervous system including the visual, vestibular, and somatosensory sub-systems must occur in order to process spatial information. When no gravitational fields are present, visual references are of utmost importance to determine one's orientation and position. An unaltered central vestibular system could send a signal that is misleading, leading to disorientation. Space adaptation syndrome (SAS) is caused by these conflicting signals from the visual and tactile senses, and inputs received by the vestibular organs, which leads to space motion sickness (SMS). The central nervous system has the potential to adapt to these conflicting signals. If the input received by the brain is transformed due to the change in gravitation, the central nervous system is obliged to develop new interpretations. If these new interpretations do not match with the patterns in our brains, SAS and consequently SMS could arise (47).
The immune system is incredibly complex, comprising a plethora of different cell types, each with a specific role. While adaptive immune cells mount a delayed, antigen-specific response that results in long-term memory, innate immunity cells respond promptly and in a non-antigen-specific manner (75).
Immunity exhibits a notably translational nature, operating throughout the body, exchanging information, interacting with most systems, and being significantly affected by the likes of stress, diet, and exercise (76).
The immune system also reacts and is therefore sensitive to various types of stressors—psychological, physical, and local environmental (e.g., oxidative and radiation exposure). Negative effects on the immune system can occur when environmental extremes are prolonged or of high intensity, or disturb circadian cycles, alter diet or other factors that affect physiological and psychological stress (77, 78, 79).
Furthermore, immune system overactivity disorders that can lead to increased susceptibility to infectious diseases, such as allergy, asthma, eczema, and autoimmunity, have also been reported (80). As a result, the immune system is a special network of organs that is keenly sensitive to and responsive to changes occurring throughout the body (80).
Immune cells are also affected by microgravity, which could lead to immunodeficiency. The body would have great difficulties protecting itself from infections and tumours, as well as repairing itself. This decrease in performance of immune cells can stir up allergies and autoimmune pathologies, putting the mission at risk. Recent research has shown that during a 6-month orbital spaceflight, the human immune system is disregulated and latent herpes viruses can be reactivated (80).
Furthermore, during flight, some crew members report ongoing hypersensitive reactions. This phenomenon may elevate some crew clinical hazards during long space exploration missions when combined with galactic radiation exposure.
Part of this research field investigates T-lymphocytes in space. These cells are sub-divided into CD4+ T helper cells and CD8+ T cytotoxic cells (81). The T helper cells secrete cytokines to regulate the immune system's reaction to infections whilst the cytotoxic T cells induce cell death. A study on mice, performed in space, showed that the number of T cells diminished under microgravity conditions. The study indicated that spaceflights have a noticeable effect on the expression of cancer-related genes, and can therefore also increase carcinogenesis (81). This could result in astronauts developing cancer during long-duration missions. The development of carcinogenesis is increased further by the effects of microgravity on the natural killer cells (CD8+ T cytotoxic cells).
In conclusion, the frequent occurrence of immune abnormalities amongst crew members during low-earth orbit flights and the inability to predict which specific crew members will suffer such alterations provide clinical challenges for future missions to Mars.
Due to the impact of microgravity on immune cells and cytokines, the ability of the body to repair itself is also impaired in space. Furthermore, spaceflights negatively affect tissue repair and wound healing (82). The skin is the largest organ in the human body, comprising up to 16 percent of total body weight (83). Its purpose is to shield the body from environmental dangers, and, as a result, it serves as a barrier protecting the underlying tissues. Furthermore, the skin consists of various layers, each of which performs a specific role. During spaceflights, itches, rashes, and dry skin are the skin-related issues that astronauts most frequently report (84, 85). The International Space Station (ISS) environment, where the only options for skin hygiene are wet tissue wipes and non-rinse shampoos and soaps, is regarded to be a contributing factor to these problems. Atopic dermatitis, skin infections, and dryness and itching of the skin appear with increased incidence, in part because of other factors such as temperature, air circulation, and low humidity levels (86). Furthermore, changes in the skin's microbiota have been linked to higher skin infections and hypersensitivity in a cohort of nine astronauts, as well as a decrease in Proteobacteria (87). Finally, contact dermatitis, which is typically brought on by irritants like biosensors, tape, or electrode patches, is frequently reported. Delayed healing of wounds and minor cutaneous damage are also observed during spaceflight. On Earth, the wound healing process occurs in different stages. First, blood reaches the wound, together with platelets that form a fibrin clot. This clot prevents further bleeding at the wound site and ensures that wound healing can continue. However, in space, proteins and cells in the blood do not function normally, resulting in reduced wound healing efficiency (88).
One example is the creation of collagen, by fibroblasts, which is integral to the wound healing process. The production of this protein, however, is reduced in space, leading to a less efficient wound healing process. Due to the various effects of microgravity on the components of the wound healing process, the probability of successful wound healing is decreased. During long duration spaceflights or interplanetary missions, minor lacerations are unavoidable. Therefore, it is critical that further studies are performed to improve wound healing efficiency in microgravity (47).
It is widely known that the mechanical cues cells receive from their surroundings influence cell behaviour (89, 90). Cells can detect changes in gravity, as well as changes in the mechanical properties of their surroundings, which stimulate biological reactions that can affect processes occurring at the cellular and tissue levels. Therefore, mechanical and gravitational forces can alter a number of processes, including gene expression, signalling, adhesion/migration properties, proliferation (91), differentiation (92) and apoptosis (93–95), as well as the way that cells organize themselves into three-dimensional (3-D) structures (constructs, tissues, and organs) (96).
Cells require gravitation for normal cellular processes. Therefore, in microgravity conditions, mechanical, biomechanical, and even physiological processes could be altered (46).
Figure 5 describes the general risk assessment and mitigation strategies used in space and are described below.
Figure 5. Risk assessment and mitigation: A flowchart-style depiction of the risk assessment and mitigation strategies for space missions. The figure outlines the systematic approach for identifying potential hazards, evaluating the risks of potential hazards, and implementing effective strategies to reduce or eliminate these risks. It highlights the importance of comprehensive risk management strategies to ensure mission success. Created with BioRender.com.
A range of medical tests is performed to ensure that astronauts are ready to go into space. These tests are conducted throughout the astronaut's working career, mostly on an annual basis. Most of these tests are conducted by all space agencies and are part of a long-term health follow-up programme for all astronauts to monitor potential occupational related injury or disease occurring during or after their space missions. These health programmes examine the incidence of various diseases, as well as acute and chronic morbidity and mortality of astronauts and define the risks associated with occupational exposures (80, 97–101).
By proactively monitoring and screening astronauts for occupational hazards, scientists can detect potential acute and chronic health problems at an earlier stage and possibly prevent progression of these health problems upon re-entry from space and over subsequent years.
A comprehensive review explaining all the various tests performed on astronauts upon their return from space can be found in (102). These include various blood, biochemical, musculoskeletal, dermatological, ophthalmological, audiological as well as cardio-pulmonary tests (102).
To protect the health of astronauts, the various space agencies, such as NASA and ESA, estimate risks, set rules, and apply regulations, which may vary depending on the mission. A list of references that describes the risk methodology applied for astronaut protection in space can be found in (103–106).
These specific regulations must also be applied to touristic spaceflights. Furthermore, keeping these regulations up to date is essential as new hazards are continuously discovered, and their application could lead to planetary conflict if they were not relatively practical and universally applicable. The rules should be applied when there is no other alternative for reducing the exposure to a particular threat.
Another crucial element in the health legislation is the so-called permissible exposure standards (PESs) for radiation and for other risks (107). These standards are important for protecting astronauts from harmful exposures. If those standards were to be exceeded, health risks increase substantially, and heavy penalties or even imprisonment could be imposed. Unfortunately, these standards could also limit the work of astronauts. Hence, if the task is necessary for survival, exceptions can be made (108).
Astronauts require medical intervention training to attend possible injuries that could occur during a mission (109, 110). This training gives the astronauts the necessary tools to recognize various diseases and possible treatments. Moreover, astronauts must be kept up to date with new scientific and technological developments in the form of regular training. As space exploration continues, new potential risks might be discovered (108).
Since the start of the Covid-19 pandemic, billions of people have been subjected to quarantine restrictions. However, astronauts have been familiar with quarantine since the dawn of the space age. There is a potential risk that astronauts may carry bacteria or viruses from earth into the spacecraft. To reduce this risk, astronauts are placed in quarantine for several days before the mission launch. Furthermore, pre-flight quarantine is not the only period where astronauts are quarantined. In space, the change in bacteria (more virulent) can increase the risks of contracting infections.
Space modules are small, sealed environments that are home to many different microbial ecosystems. Such contained communities are a substantial source of viruses, some of which can be harmful to humans and pose a risk to both personal and crew health (111, 112). Due to high radiation doses, microgravity, and compact spaces, space modules provide extraordinary conditions for Earth’s bacteria to proliferate and flourish (113).
If an astronaut does develop an illness, microbiologists and astronautical hygienists will need to characterize the hazard and act accordingly. This could be achieved by placing the infected crew member in quarantine. However, imposing a quarantine restriction in a spacecraft is not always possible due to its limited size.
Microorganisms, such as archaea that can be found in the extreme environments on Earth, are also present in space. These microorganisms are brought by the spacecraft into space and possess the ability to mutate. This can lead to astronauts contracting new diseases after exposure and potentially spreading them in the craft. Therefore, vaccination programs are implemented to limit the spread of infection and to protect individuals against these possible infections.
It is advised to implement a robust vaccination program that includes tetanus/diphtheria/acellular pertussis (Tdap), measles/mumps/rubella (MMR), influenza, pneumococcal, meningococcal, and hepatitis A and B vaccines (114). Varicella zoster virus vaccine should be administered due to the increased reactivation of herpes viruses, which has been observed in previous space missions (115).
Air- and water-quality must be monitored to ensure that no harmful substances are present during the mission. NASA developed specific tracers to follow up the presence of various pollutants such as carbon dioxide, because these substances could cluster in isolated spaces or create propellants or formaldehyde. Trace volatile organic analysers are used to monitor air quality. When potable water is recovered from urine, total organic carbon (TOC) analysers are used to ensure a satisfactory water quality. Many materials used in spacecraft expel different toxic chemicals. Toxicologists try to calculate and predict potential risks by analysing the route of exposure, duration of exposure, the amount of chemicals present during exposure—mixed and singular—as well as potential by-products of these chemicals. To follow up these different parameters, a variety of environmental monitoring sensors are present on the ISS (116). The monitoring of radiation is a special type of monitoring and will be described in the last section of this paper.
For more than 50 years, cytogenetic biodosimetry has helped to provide dose estimates for accidental radiation overexposures (117). Cytogenetics is the branch of genetics and cytology related to the study of chromosomes. Since the early 1990s, Cytogenetics has also been used on astronauts (118–120) by taking lymphocyte (a type of white blood cells) samples prior and after space mission to give an in vivo evaluation of radiation-induced damage induced during space travel (120, 121).
Biodosimetry provides a direct measurement of space radiation damage in vivo, taking into account the combined individual radiosensitivity of astronauts, the attenuation by the body, the shielding of the spacecraft and the combined influence of microgravity and other stress conditions on the body, all of which cannot be captured as accurately by physical dosimetry (122).
Biodosimetry of astronaut blood samples is incorporated into the astronaut medical records of European and Canadian astronauts and is a medical requirement as specified in the International Space Station (ISS) Medical Evaluation Document (123, 124). Maintaining reliable, standardized biodosimetry techniques are crucial as longer-duration space travel is being considered.
The exposome is the collection of all exposures to environmental factors on our body from inception to death (125). Exposure to environmental factors can be exogenous or endogenous in nature, including diet, chemical exposure, stress, and use of alcohol. Exposome science is interested in non-genetic explanations to disease development. Therefore, the use of exposome monitoring could aid in ascertaining whether particular astronauts are more prone to developing diseases after exposure to certain space-related risks such as radiation. Astronauts could be screened on both terrestrial and extra-terrestrial factors to identify exposomes related to certain exposures. This could allow astronauts who are less likely to develop diseases following the exposure to specific hazards to be selected for missions that encounter such hazards. However, the ethics of restricting certain astronauts from participating in missions makes the use of exposomes not as straightforward as it might seem. European legislation does not permit ESA to restrict an astronaut based on genetic analysis. Doing so is akin to discrimination, which the European Union is against. Who will make the decision to restrict an astronaut from going into space?
Will the insurance fee for an astronaut more prone to developing illnesses be higher? Will the astronaut face restrictions and penalties during the mission, such as limited Extravehicular activities due to his/her higher risk susceptibility? If, after several studies, the use of exposomes indicates that certain races or sexes are more prone to developing diseases after exposure to specific hazards, is it ethically correct to restrict this population of astronauts from going on certain missions? Who can get access to this data? The use of exposomes seems at first a promising avenue for investigation, but once the questions about how the data will be used arise, it becomes complicated to justify (108).
Since radiation risk is directly related to dose, a mitigation strategy must be developed to reduce the dose absorbed to an acceptable level. The radiation dose depends on the mission scenario, i.e., the type and intensity of radiation to which the astronauts are exposed, as well as the duration of the mission, the spacecraft's attitude, and solar activity. Therefore, these different mission parameters can have a drastic impact on the radiation dose absorbed by astronauts. For example, the average dose received by astronauts inside the ISS is approximately 0.5–1 mSv/day (126). For a mission to Mars, the radiation dose will differ from those on board the ISS. In deep space, the Radiation Assessment Detector (RAD) device [on board the unmanned Mars Science Laboratory (MSL) “Curiosity”] measured a mean GCR dose-equivalent rate of 1.84 mSv/day (13), with an estimated dose equivalent for the Martian surface of 0.64 mSv/day (127).
Two types of countermeasures exist to protect astronauts from space radiation, the first being to reduce the radiation exposure to an as low as reasonably practical (ALARP) dose by using space shielding (section 5.1.1). The second involves biological countermeasures through the use of radioprotectors and radiomitigators, genetic approaches and hibernation (section 5.1.2). Figure 6 describes the various possible risk assessment and mitigation strategies.
Figure 6. Radiation risk assessment and mitigation: detailed illustration outlining the radiation risk assessment and mitigation strategies used during space exploration. The figure highlights countermeasures such as shielding and safe exposure duration, pharmacological protection and hibernation, emphasizing the multidisciplinary approach to managing radiation hazards. It also portrays space radiation exposure assessment and monitoring strategies, space environment information system as well as biosensors for space radiation. Created with BioRender.com.
Shielding is a logical approach to enduring the brunt of space radiation. Unfortunately, it is more complex than it may appear.
Nowadays, the majority of spacecraft are sufficiently protected from space radiation within LEO. However, outside LEO, these forms of shielding will not provide an adequate barrier to protect astronauts. Protection against SPE particles is possible with spacecraft shielding, but the heavy elements produced by GCR ions have such large amounts of energy that it is nearly impossible to prevent them from entering the vehicle (128).
Physical based mitigation methods aim to reduce the radiation dose by acting on the incoming radiation field in two different ways: (i) active shielding, in which electromagnetic fields deflect or trap cosmic rays, and (ii) passive shielding, which exploits nuclear and electromagnetic interactions between incident radiation and materials (129).
Studies have shown that active shielding could be the solution, several types of active shielding have been proposed. Electrostatic shielding operates by creating an electric field around the spacecraft to slow down negatively charged particles (130–135). However, the effects of living in a giant electrostatic field are not fully known. Therefore, studies investigating this technology must be performed. Another type of active shielding is magnetic shielding (136–139), in which solenoids generate a magnetic field around the spacecraft to either deflect or trap charged particles. Lastly, plasma-shielding uses ionized particles to form a magnetic field around the spacecraft (140–145). These particles could entrap or deflect charged radiation particles. This protection works threefold since it combines both electrostatic and magnetic shielding, together with the ionized particles. Advantages and disadvantages of these methods have been discussed in (146–148). Although active shielding remains an area of ongoing research, it is not clear whether an active shielding solution can provide adequate protection against the high-energy GCR components of the space radiation field (149) whilst still being practically feasible for space travel.
Significant research has been performed in the field of passive shielding to optimize material selection and shielding in the various space radiation environment scenario (150–153).
Previous studies have shown that conventional shielding methods (such as polyethylene or aluminium) of specific thickness cannot provide sufficient capacity of shielding against the ion component HZE, which is the biggest threat to astronauts when embarking on long-term voyages (154, 155).
The increased shielding thickness beyond standard spacecraft values (average thickness of about 10 g cm−2) is required to reduce the risk of radiation below the Space Permitted Exposure Limit (SPEL) (156). However, with the current NASA launch capabilities, the increased shielding exceeds mass limits for launches set by NASA (16, 157). This limit refers to a predetermined threshold of mass established by NASA and indicates the maximum allowable mass that must not be exceeded during the launch. It is a critical criterion that ensures the safety and well-being of astronauts and spacecraft equipment throughout their journey. A solution is therefore needed to reduce radiation exposure below regulatory limits, without compromising launch restrictions. For this reason, the current approach is to reduce the dose by modifying the radiation field composition via nuclear fragmentation, namely by breaking ions into particles with lower charges and similar velocities, while avoiding the dose increase of the mixed field induced by the secondary particles (158).
Passive shielding methods could provide sufficient shielding on the lunar and Martian surfaces. This could be achieved by building the surface habitats from regolith (159, 160). Another shielding approach to reduce radiation exposure during a stay on the surface relies on analysing the lunar geography to take advantage of particular geographical features when selecting the construction site.
Despite these major discoveries, further studies are needed to implement them in current and future spacecraft designs (16). ESA has been supporting theoretical and experimental studies on space radiation shielding (ROSSINI) (152), aiming at dose reduction through improvement of structure configuration and development of new materials. Lithium hydride (LiH) compounds were identified as possible alternatives to polyethylene (currently used on the ISS) for radiation shielding (161).
Boron nitride nanotubes (BNNTs) spun into yarn, making it very flexible, have also been studied (162). Research has been performed by sampling regolith and studying the lunar geography to identify suitable materials for radiation protection (163). Results showed that lava tubes and lunar regolith could be used as shielding materials against radiation particles, as well as for controlling the thermal environment (164, 165).
Finally, hybrid active-passive shielding approaches, where active shielding (comprising an electrostatic shielding configuration consisting of multiple charged planes and charged rods) was placed outside passive shielding have been studied to investigate the efficacy of combined passive and active shielding for protection against extreme solar particle events (SPEs) and free-space GCR spectra under solar minimum and solar maximum conditions (166).
In parallel to shielding, scientists are also considering alternative methods of radiation protection. Pharmacological protection could ensure that radiation does not damage the astronauts’ bodies or that any such damage is repaired with a high degree of fidelity. McLaughlin et al. divided this form of protection into three categories: radioprotectors (preventing the harmful effects of radiation exposure on biological tissue); radio modulators (increasing the body's resistance to radiation by giving a substance prior to actual radiation exposure) and radiomitigators (limiting and preventing tissue degeneration after radiation exposure). Drugs and antioxidants could ensure that the pharmacological protections described above would work in the human body (167).
Radioprotectors and radiomitigators are potentially interesting compounds for future deep space missions (167). Radioprotectors and radiomitigators increase the resistance of the body to radiation exposure by increasing the effectiveness of antioxidative protection and by neutralising radiation effects (radioprotectors) or by accelerating the restoration of tissue following exposure (radiomitigators) (168). Radioprotectors are molecules that protect tissues from direct and indirect damage induced by ionizing radiation whilst radiomitigators can reduce radiation-induced damage or promote repair, depending on whether they are administered pre or post radiation exposure (168).
Most radioprotectors (and radiomitigators) are antioxidants, and thus act as free-radical scavengers. They can therefore increase the levels of antioxidant defences, such as reduced glutathione and/or of antioxidant enzymes such as superoxide dismutase, glutathione peroxidase and thioreductase. They can also stimulate one or more DNA repair pathways, prevent apoptotic cell death, modulate redox sensitive genes, and display an anti- inflammatory response as well as promoting tissue regeneration (168).
For radioprotectors to be suitable for spaceflight usage, they should reduce or prevent direct and indirect effects of ionizing radiation on cells and healthy tissues in animals and humans (whilst not protecting tumour cells). Furthermore, they should not be toxic, nor cause any adverse effects when administered alone or in combination with other drugs. They should have a sufficiently broad window of effectiveness once administered, have a long and stable shelf life, and be easily accessible without complex handling or transport arrangements. In recent decades, various natural as well as synthetic compounds have been brought forward and investigated for their potential as radioprotectors (169).
There exists a variety of currently approved agents that show preclinical and clinical efficacy in preventing or reducing acute and chronic sequelae of radiation exposure (167). Many of these agents could be combined with vitamins, nutraceuticals, or other endogenous substances to obtain synergistic effects (170, 171).
Amifostine, for example, as well as other thiol compounds and their derivatives have already been used clinically on cancer patients on Earth to prevent complications from radiotherapy treatment. However, the practical applications of most of these synthetic compounds remain restricted due to their limited administration routes, their narrow administration window for efficacy and their toxicity at high doses or during recurrent usage, making them far from ideal candidates for use by astronauts (172).
To conclude, antioxidants appear to offer benefits to human health in space. Although the future for antioxidants seems promising, insufficient data are available to reach a positive conclusion about their use in space.
Other strategies to increase human radiation resistance to withstand long exposures to cosmic radiation are being investigated (173). The first such approach entails inducing an overexpression of endogenous and exogenous antioxidants via integration and expression of their cognate transgenes (174). Various studies using this approach have reported a substantial reduction in early and late-stage irradiation damage following the delivery of magnesium superoxide dismutase (MnSOD) transgenes systemically or in a tissue-specific manner by using plasmid/liposomal vectors (175, 176).
A second strategy is to induce an overexpression of endogenous and exogenous DNA repair genes (such as overexpression of O6-alkylguanine DNA alkyltransferase and yeast AP endonuclease) to enhance the efficiency of endogenous DNA repair and to reduce mutagenesis and associated carcinogenesis (177, 178).
A third option aims to increase the expression of endogenous and exogenous radioprotective transgenes, such as the promising nuclear protein Damage suppressor, (Dsup), discovered in the radiation resistant tardigrades (173, 179, 180).
The fourth strategy consists of characterising the genetic determinants of high human radioresistance in conjunction with clinical translation via multiplex genetic engineering. For example, single-nucleotide polymorphisms (SNPs) are genomic variants at a single base position in the DNA. SNPs occur at specific positions within the genome and are present in at least 1% of the human population. Part of the individual differences in radiosensitivity within the human population can be attributed to differences in SNP profiles. The set of candidate SNPs that yield high radioresistance could be identified, making the use of targeted gene editing technologies (e.g., CRISPR/Cas9) to replicate those SNP variants in individual humans possible (181, 182).
Finally, an additional option is the generation and characterisation of enhanced radioresistance via experimental evolution in conjunction with clinical translation via multiplex genetic engineering (183). This strategy would take advantage of experimental evolution to generate populations of model organisms or human cells with enhanced radioresistance through exposure to high levels of radiation. The surviving cells or organisms are then bred, followed by the characterisation of the genetic determinants of the resulting enhanced radioresistance phenotype and the subsequent clinical translation of such genetic determinants to humans via multiplex genetic engineering. This technology was successfully implemented in the bacteria E. coli (184).
A more recent approach to radiation mitigation is found in the form of hibernation. This state can be observed in mammals as a means of surviving periods of reduced resource availability or adverse meteorological conditions. Hibernation has long been known to reduce the impact of radiation damage, in addition to its protection against famine and starvation periods (185). Hibernation induces a remarkable resistance to radiation damage, which could help mitigate the effects of radiation exposure experienced during spaceflights (186, 187).
Torpor is an animal's metabolic condition during hibernation. During torpor, the metabolism is drastically reduced over a period of a few hours to several weeks. Scientists are investigating whether the state of torpor could be induced during long manned space trips to Mars and beyond (188). Natural torpor's decreased metabolic rate is associated with deep biochemical changes, such as the switch from glycolysis to lipolysis and ketone consumption, extensive but reversible changes in organs including the brain and kidney, and Ca2+-mediated heart rate control (188).
The lowering of astronauts' physiological needs through intermittent or constant torpor states (that mimic those experienced naturally during hibernation) could potentially be groundbreaking for deep space missions (189). If astronauts could endure this physiologically natural state, it would significantly reduce the number of supplies and the size of the habitat needed, but would require constant monitoring and artificial intelligence (AI) assisted monitoring of the crew (189). It would also mitigate radiation and low-gravity side effects, potentially reducing neurobehavioural effects such as boredom, loneliness, and aggression. Furthermore, up to 75% less water and food could be consumed, which would reduce both the spacecraft's cargo requirements and excreta production (189).
Recently, the comprehensive Mission Concept and Requirements Assessment (MicRA) study was carried out by the European Space Agency (ESA) to evaluate the potential and the research needs for the use of torpor as a tool for human spaceflight (189).
All hibernators, studied so far, have shown increased radiation resistance when in their torpid state (185). Recent studies have examined the fundamental mechanisms and their applicability to space missions (186, 190). Therefore, under prolonged physical stress, starvation, low metabolic rate, and low body temperature, torpor and hibernation have been shown to offer a robust state of tolerance and tissue preservation, as well as radiation resistance.
Scientists are currently developing artificial methods of torpor, where GABA-A receptor agonist—“muscimol” is injected into the brainstem region. This induced torpor would increase radioprotection (191). Currently, research has been performed successfully on zebra fish (192) and rats. Torpor decreases cell death (apoptosis) (193) by up-regulation of anti-apoptotic proteins such as Bcl-XL in an organ-specific manner (194, 195), and the necrotic tissue injury seems to be minimal (196).
However, more studies are needed to appreciate the complexity of the radiation resistance mechanism before applying it fully to astronauts (188).
During all stages of flight and even post flight, astronauts are affected by space radiation which can be considered an imperceptible risk (197).
In addition, the nature of space radiation is unique compared to terrestrial radiation, and data on the effects of space radiation on humans are extremely sparse. Exposure data are only available from 24 astronauts on 12-day missions outside LEO (3). This lack of data requires space radiation risk models to rely on terrestrial radiation exposure data, which may have different physiological effects than those encountered in space (16, 198).
Furthermore, a key duty for medical practitioners is to understand, foresee, and minimize potential health risks during space flight (199). To that end, guidelines have been put in place for radiation doses that humans can absorb during a given period.
The amount of radiation absorbed by human tissues is measured using the unit Sievert (Sv) (200). The yearly average dose equivalent for humans is 3.6 mSv resulting from natural exposure on Earth. However, this is a relatively small dose compared to the doses encountered when working in a radiation rich environment. The radiation protection regulations from the International Commission for Radiological Protection (ICRP) set the limit for occupational exposure to 20 mSv per year of effective dose, averaged over a defined period of 5 years (100 mSv in 5 year), with no yearly dose exceeding 50 mSv (200). However, astronauts are exposed to significantly higher amounts of radiation than the average worker on Earth. Furthermore, astronauts encounter different types of radiation when in space. Therefore, these guidelines are not sufficient to protect astronauts for all types of space travel. To maintain crew doses below set limits, or as low as reasonably achievable, the ionizing radiation environment must be monitored throughout a space flight, meaning crewmembers' critical organ and tissue doses are monitored and assessed. The data collected are recorded for each mission, as well as for cumulative career records (124). This enables immediate countermeasures to be implemented in the event of transient radiation exposure events (such as during EVA: solar particle events or electron belt enhancements).
To keep astronauts safe but delve deeper into space, the ICRP has defined three fundamental guiding concepts that should be adhered to during human spaceflight. These concepts complement the ICRP's effective dose regulation, mentioned previously. The first of these three guiding concepts is justification. Report 60 of the International Commission on Radiological Protection (ICRP) states that “no practice involving exposures to radiation should be adopted unless it produces sufficient benefit to the exposed individual or to society to offset the detriment it causes” (201).
The second principle, Optimization, is defined as “…a process or method used to make a system of protection as effective as possible within the given criteria and constraints”. Thirdly, the principle of Limitation states: “individual dose limits are applied to ensure that the principles of justification and ALARA (as low as reasonably achievable) are not applied in a manner that would result in individuals or groups of individuals exceeding levels of acceptable risks” (201).
The effective dose regulation and the three guiding concepts provide a basic framework to manage radiation exposures related to human spaceflight. However, to fully comprehend and communicate detailed information about potential radiation related risks to a specific astronaut or to a space mission, more metrics and uncertainty evaluations are needed. Future missions will travel to distant locations, well beyond LEO. These will present new design and radiation protection challenges (202). A list of current operational radiation limitations utilized by the ISS partners can be found in (199) and updates can be found in (203, 204).
An astronaut's annual and mission-related medical examinations, medical certification, and re-certification procedures all address radiation in space and its associated possible health risks (199). During these extensive medical examinations, the general organ functions, the blood-forming organs, the central nervous system, and the endocrine system in particular, are observed, analysed and assessed. In addition, pathogenetic factors, as well as potential new risk factors, are monitored (199). For an astronaut's mission assignment, pre-flight crew radiation exposure histories are reviewed and analysed.
Recently, NASA has modified the previous risk-based radiation limits for long-term deep space flights to a new limit of 600 mSv total effective dose over an astronaut's career (205), based on the recommendation of the National Academies of Sciences, Engineering, and Medicine (206). Previously, a risk-based limit was imposed by NASA that ensured a career-integrated 3% risk of exposure-induced death (REID) at the upper 95% confidence interval (156). The new radiation limit removes the dependence on astronaut age and biological sex, making future space travel more inclusive and removing the inherent complexity in computing REID. In addition to the total effective dose for a career, short-term limits exist to restrict doses to prevent specific tissue reactions (also known as non-carcinogenic or deterministic effects) to organs, such as skin, lens, blood forming organs and the circulatory and central nervous systems (205).
To ensure that astronauts and electronics can tolerate the radiation environment found in space, even whilst being shielded by the spacecraft/habitat, the radiation environment must first be determined during the mission planning and design stages. This requires instrumentation that can provide the necessary data, as well as analysis of existing data from current detection systems, such as those on the lunar surface (207) or in other locations (208).
Accurate space radiation models are crucial for lowering the risks astronauts encounter and for building high-performance, low-cost space equipment. The AP-8 (209) and AE-8 (210) models of the Earth's radiation belts are commonly used models.
The AP-8 models of trapped protons, which are appropriate for solar maximum and minimum, respectively, include AP-8 MAX and AP-8 MIN. Both AE-8 MAX and AE-8 MIN are included in the AE-8 models for trapped electrons. These common models are praised for their thorough spatial coverage and user-friendliness, although they have drawbacks and suffer from some inaccuracies (211–214).
However, both models, AP-8, and AE-8, were constructed using data that were gathered between 1958 and 1979. The models might no longer accurately represent the environment that today's space systems experience due to the dynamic nature of the space environment. Notably, it is known that the Earth's inner radiation belt is tainted by the high-altitude nuclear device detonations that occurred in the late 1950s and early 1960s (211).
New standard radiation belt environment models are thus required because modern applications require more precision, functionality, and energy coverage that AP-8 and AE-8 do not deliver. Therefore, newer systems were implemented. For example, the SPENVIS is an ESA's space environment information system used to simulate and model the space environment (https://www.spenvis.oma.be/). It includes models used for the analysis and prediction of radiation environments from galactic cosmic rays, solar energetic particles, natural radiation belts, plasmas, gases, meteoroids, and debris. SPENVIS uses the Jensen and Cain 1960 internal field model for AE-8 MIN and MAX, as recommended in (212). It also models the space impact on the spacecraft faces and systems during flight into orbit by using the Jenson and Cain model (212).
Thereafter, the computer code SHIELDOSE program (215) can help to calculate the radiation doses based on the shielding, the damage to solar cells, LET spectra of cosmic rays and energetic particles and make estimations of single-event disturbances for micro-electronics and equipment.
Other publicly available models can also be found. For proton models, PSB97 (216), Low Altitude Trapped Radiation Model (LATRM) (217), Trapped Proton Model (TPM-1) (218), and the Combined Release and Radiation Effects Satellite Proton model (CRRESPRO) (219) have been developed since AP-8.
Electron models that were developed since AE-8 include the Combined Release and Radiation Effects Satellite Electron model (CRRESELE) (220), Flux Model for Internal Charging (FLUMIC) (221), and the Particle ONERA2-LANL3 Electron (POLE) model (222).
Each astronaut on board the ISS has their own passive dosimeter. The radiation levels are monitored regularly by active and passive detectors throughout the entire station. A gas radiation numerator, called Tissue Equivalent Proportional Counter (TEPC), estimates the radiation dose absorbed by crew members. Furthermore, the detector is designed to function like a human body. The detector has a plastic casing that mimics human tissue whilst the internal gas simulates human cells.
However, to obtain more reliable radiation measurements linked to biological response, various biosensors are being developed. One example of research on radiation is the nanosatellite BioSentinel, launched from Artemis-I, that will perform in situ biological tests on living organisms (yeast) in the radiation environment of deep space (223). On-board active dosimetry will take measurements of the radiation field found within the spacecraft after having passed the shielding and internal components found within the BioSentinel nanosatellite (224). The primary objective will be to assess the damage and reaction in cells exposed to radiation during spaceflight. This enables scientists to measure the effects of space radiation on living organisms over long periods of time beyond LEO (224, 225). In view of future deep space missions, other biosensor devices are being tested within the Artemis programme (226, 227).
In this review, the investigation of ionizing radiation's causes, effects, and health hazards highlights the importance of effective radiation safety regulations and mitigation strategies for use during extended deep space missions. To achieve safety standards for future deep space missions, innovative shielding techniques will be required because solar particle events and galactic cosmic radiation pose a serious health risk to astronauts. Furthermore, the necessity for specialized medicinal countermeasures reviewed in the biological section of this review is further highlighted by the wide range of adverse health impacts, such as tissue degradation, carcinogenesis, and central nervous system damage. The thorough analysis of the effects of microgravity on many physiological systems reveals how crucial it is to devise mitigation strategies to counteract these effects. The in-depth analysis of risk assessment techniques, including the rules, and guidelines for medical examinations, emphasizes how healthcare and safety protocols must continuously change to maintain astronaut safety. In order to reduce health risks during space missions, quarantine, immunization, and monitoring become essential processes as discussed in this review. Exposomes and biodosimetry illustrate the multidisciplinary approach necessary to thoroughly assess astronaut health. Additionally, research into radiation protection techniques including shielding, biological protection, radioprotectors, as well as cutting-edge methods such as gene therapy and hibernation offer additional solutions to radiation related health hazards. The use of these techniques, as highlighted in this review, could have significant potential for facilitating deep space exploration whilst ensuring astronaut safety.
In conclusion, the complex interactions between ionizing radiation, microgravity, and astronaut health during space travel are highlighted in this review. The urgency to establish effective risk assessment and mitigation strategies is driven by the impact these stressors have on different physiological systems. This review highlights potential avenues that could be stepping stones in astronaut safety for upcoming space exploration missions. It is important to mention that although methods to protect the health of astronauts already exist, innovative technologies are being studied and developed to push the boundaries of where Humans can travel to. However, there is still a need for extensive research in this field. As we continue to collect data on the vital signs of astronauts' bodies, we can broaden our understanding of the risks encountered during space exploration. Moreover, we must continue to produce new risk assessment methods appropriate for both current and future risks.
WD and LM: literature research, main writing of the manuscript. DM: figure design. KT, BB, and SB: financial support, original concept of the manuscript, management of the writing, interpretation of literature, manuscript reviewing & editing. All authors contributed to the article and approved the submitted version.
Authors acknowledge the support of ESA/BELSPO/Prodex, IMPULSE contract CO-90-11-2801-04. The authors are grateful to Dr Andrew Dobney for the reviewing and to Tine Slegers for the manuscript formatting. The manuscript has been presented as a proceeding paper during the International Astronautical Conference, Paris October 2022 (https://iafastro.directory/iac/paper/id/72952/abstract-pdf/IAC-22,A1,5,11,x72952.brief.pdf?2022-03-29.12:37:07).
The authors declare that the research was conducted in the absence of any commercial or financial relationships that could be construed as a potential conflict of interest.
The authors SB, BB, and KT declared that they were an editorial board member of Frontiers, at the time of submission. This had no impact on the peer review process and the final decision.
All claims expressed in this article are solely those of the authors and do not necessarily represent those of their affiliated organizations, or those of the publisher, the editors and the reviewers. Any product that may be evaluated in this article, or claim that may be made by its manufacturer, is not guaranteed or endorsed by the publisher.
1. Amini K, Moradi M, Vossoughi B, Janabadi ED. Space-technological and architectural methodology and process towards design of long-term habitats for scientific human missions on Mars. MethodsX. (2023) 11:102270. doi: 10.1016/j.mex.2023.102270
2. Thirsk RB. Health care for deep space explorers. Ann ICRP. (2020) 49(1):182–4. doi: 10.1177/0146645320935288
3. Chancellor JC, Scott GB, Sutton JP. Space radiation: the number one risk to astronaut health beyond low earth orbit. Life (Basel). (2014) 4(3):491–510. doi: 10.3390/life4030491
4. Krittanawong C, Isath A, Kaplin S, Virk HUH, Fogg S, Wang Z, et al. Cardiovascular disease in space: a systematic review. Prog Cardiovasc Dis. (2023):S0033-0620(23)00075-0. doi: 10.1016/j.pcad.2023.07.009. [Epub ahead of print]37531984
5. Baran R, Marchal S, Garcia Campos S, Rehnberg E, Tabury K, Baselet B, et al. The cardiovascular system in space: focus on in vivo and in vitro studies. Biomedicines. (2021) 10(1):59. doi: 10.3390/biomedicines10010059
6. Kellerer AM, Rossi HH. The theory of dual radiation action. In: Elbert M, Howard A, editors. Current topics in radiation research quarterly. vol 8. New York, NY: American Elsevier Publishing Company (1974). p. 85–158.
7. Hall EJ. Radiobiology for the radiologist. 5th edn. Philadelphia: Lippincott Williams & Wilkins (2000).
8. Simpson JA. Elemental and isotopic composition of the galactic cosmic rays. Ann Rev Nucl Part Sci. (1983) 33:323–81. doi: 10.1029/RG021i002p00295
9. Zhang S, Wimmer-Schweingruber RF, Yu J, Wang C, Fu Q, Zou Y, et al. First measurements of the radiation dose on the lunar surface. Sci Adv. (2020) 6(39):eaaz1334. doi: 10.1126/sciadv.aaz1334
10. Cucinotta FA, Kim M-HY, Chappell LJ. Space Radiation Cancer Risk Projections and Uncertainties—2012. National Aeronautics and Space Administration NASA/TP-2013-217375 (2013).
11. Cucinotta FA, Alp M, Rowedder B, Kim M-HY. Safe days in space with acceptable uncertainty from space radiation exposure. Life Sci Space Res. (2015) 5:31–8. doi: 10.1016/j.lssr.2015.04.002
12. Cucinotta FA, Kim MH, Ren L. Evaluating shielding effectiveness for reducing space radiation cancer risks. Radiat Meas. (2006) 41(9–10):1173–85. doi: 10.1016/j.radmeas.2006.03.011
13. Zeitlin C, Hassler DM, Cucinotta FA, Ehresmann B, Wimmer-Schweigruber RF, Brinza DE, et al. Measurements of energetic particle radiation in transit to Mars on the Mars science laboratory. Science. (2013) 340(6136):1080–4. doi: 10.1126/science.1235989
14. Benton ER, Benton EV. Space radiation dosimetry in low-earth orbit and beyond. Nucl Instr Meth Phy Res B. (2001) 184(1–2):255–94. doi: 10.1016/S0168-583X(01)00748-0
15. Cucinotta FA, Durante M. Cancer risk from exposure to galactic cosmic rays: implications for space exploration by human beings. Lancet Oncol. (2006) 7(5):431–5. doi: 10.1016/S1470-2045(06)70695-7
16. Durante M, Cucinotta FA. Physical basis of radiation protection in space travel. Rev Mod Phys. (2011) 83(4):1245–81. doi: 10.1103/RevModPhys.83.1245
17. Darby SC, McGale P, Taylor CW, Peto R. Long-term mortality from heart disease and lung cancer after radiotherapy for early breast cancer: prospective cohort study of about 300,000 women in US seer cancer registries. Lancet Oncol. (2005) 6(8):557–65. doi: 10.1016/S1470-2045(05)70251-5
18. Preston DL, Shimizu Y, Pierce DA, Suyama A, Mabuchi K. Studies of mortality of atomic bomb survivors. Report 13: solid cancer and noncancer disease mortality: 1950–1997. Radiat Res (2003) 160(4):381–407. doi: 10.1667/RR3049
19. Hoeijmakers JHJ. DNA damage, aging, and cancer. N Engl J Med. (2009) 361(15):1475–85. doi: 10.1056/NEJMra0804615
20. Dizdaroglu M. Oxidatively induced DNA damage: mechanisms, repair and disease. Cancer Lett. (2012) 327(1–2):26–47. doi: 10.1016/j.canlet.2012.01.016
21. Institute of Medicine (US) Committee on Longitudinal Study of Astronaut Health. In: Longnecker DE, Manning FJ, Worth MH, editors. Review of NASA’s longitudinal study of astronaut health. Washington, DC, USA: National Research Council (2004). doi: 10.17226/10903
22. Raber J, Rola R, LeFevour A, Morhardt D, Curley J, Mizumatsu S, et al. Radiation-induced cognitive impairments are associated with changes in indicators of hippocampal neurogenesis. Radiat Res. (2004) 162(1):39–47. doi: 10.1667/rr3206
23. Monje ML, Mizumatsu S, Fike JR, Palmer TD. Irradiation induces neural precursor-cell dysfunction. Nat Med. (2002) 8(9):955–62. doi: 10.1038/nm749
24. Zegers CML, Offermann C, Dijkstra J, Compter I, Hoebers FJP, de Ruysscher D, et al. Clinical implementation of standardized neurocognitive assessment before and after radiation to the brain. Clin Transl Radiat Oncol. (2023) 42:100664. doi: 10.1016/j.ctro.2023.100664
25. Shi L, Adams MM, Long A, Carter CC, Bennett C, Sonntag WE, et al. Spatial learning and memory deficits after whole-brain irradiation are associated with changes in NMDA receptor subunits in the hippocampus. Radiat Res. (2006) 166(6):892–9. doi: 10.1667/RR0588.1
26. Britten RA, Davis LK, Johnson AM, Keeney S, Siegel A, Sanford LD, et al. Low (20 cGy) doses of 1 GeV/u 56Fe-particle radiation led to a persistent reduction in the spatial learning ability of rats. Radiat Res. (2012) 177(2):146–51. doi: 10.1667/rr2637.1
27. Lonart G, Parris B, Johnson AM, Miles S, Sanford LD, Singletary SJ, et al. Executive function in rats is impaired by low (20 cGy) doses of 1 GeV/u 56Fe particles. Radiat Res. (2012) 178(4):289–94. doi: 10.1667/rr2862.1
28. Kennedy AR. Biological effects of space radiation and development of effective countermeasures. Life Sci Sp Res. (2014) 1:10–43. doi: 10.1016/j.lssr.2014.02.004
29. Wilson JM, Sanzari JK, Diffenderfer ES, Yee SS, Seykora JT, Maks C, et al. Acute biological effects of simulating the whole-body radiation dose distribution from a solar particle event using a porcine model. Radiat Res. (2011) 176(5):649–59. doi: 10.1667/rr2541.1
30. Sanzari JK, Wan XS, Wroe AJ, Rightnar S, Cengel KA, Diffenderfer ES, et al. Acute hematological effects of solar particle event proton radiation in the porcine model. Radiat Res. (2013) 180(1):7–16. doi: 10.1667/RR3027.1
31. Sanzari JK, Wambi C, Lewis-Wambi JS, Kennedy AR. Antioxidant dietary supplementation in mice exposed to proton radiation attenuates expression of programmed cell death-associated genes. Radiat Res. (2011) 175(5):650–6. doi: 10.1667/RR2330.1
32. Wilson JM, Krigsfeld GS, Sanzari JK, Wagner ES, Mick R, Kennedy AR. Comparison of hindlimb unloading and partial 634 weight suspension models for spaceflight-type condition induced effects on white blood cells. Adv Space Res. (2012) 49(2):237–635 248. doi: 10.1016/j.asr.2011.09.019
33. Lackner JR, DiZio P. Human orientation and movement control in weightless and artificial gravity environments. Exp Brain Res. (2000) 130(1):2–26. doi: 10.1007/s002210050002
34. Blaber E, Sato K, Almeida EAC. Stem cell health and tissue regeneration in microgravity. Stem Cells and Dev. (2014) 23(1):73–8. doi: 10.1089/scd.2014.0408
35. Goodwin TJ, Prewett TL, Wolf DA, Spaulding GF. Reduced shear stress: a major component in the ability of mammalian tissues to form three-dimensional assemblies in simulated microgravity. J Cell Biochem. (1993) 51(3):301–11. doi: 10.1002/jcb.240510309
36. Gardner JK, Herbst-Kralovetz MM. Three-dimensional rotating wall vessel-derived cell culture models for studying virus-host interactions. Viruses. (2016) 8(11):304. doi: 10.3390/v8110304
37. Infanger M, Kossmehl P, Shakibaei M, Baatout S, Witzing A, Grosse J, et al. Induction of three-dimensional assembly and increase in apoptosis of human endothelial cells by simulated microgravity: impact of vascular endothelial growth factor. Apoptosis. (2006) 11(5):749–64. doi: 10.1007/s10495-006-5697-7
38. Calvaruso M, Militello C, Minafra L, La Regina V, Torrisi F, Pucci G, et al. Biological and mechanical characterization of the random positioning machine (RPM) for microgravity simulations. Life (Basel). (2021) 11(11):1190. doi: 10.3390/life11111190
39. Grimm D, Wise P, Lebert M, Richter P, Baatout S. How and why does the proteome respond to microgravity? Exp Rev Proteom. (2011) 8(1):13–27. doi: 10.1586/epr.10.105
40. Nabavi N, Khandani A, Camirand A, Harrison RE. Effects of microgravity on osteoclast bone resorption and osteoblast cytoskeletal organization and adhesion. Bone. (2011) 49(5):965–74. doi: 10.1016/j.bone.2011.07.036
41. Hughes-Fulford M. Function of the cytoskeleton in gravisensing during spaceflight. Adv Space Res. (2003) 32(8):1585–93. doi: 10.1016/S0273-1177(03)90399-1
42. Ohshima H. Bone loss and bone metabolism in astronauts during long—duration space flight. Clin Calcium. (2006) 16(1):81–5. clica0601818516397355
43. Smith SM, Heer M. Calcium and bone metabolism during space flight. Nutrition. (2002) 18(10):849–52. doi: 10.1016/S0899-9007(02)00895-X
44. Zayzafoon M, Meyers VE, McDonald JM. Microgravity: the immune response and bone. Immunol Rev. (2005) 208:267–80. doi: 10.1111/j.0105-2896.2005.00330.x
45. Bikle DD, Sakata T, Halloran BP. The impact of skeletal unloading on bone formation. Gravit Space Biol Bull. (2003) 16(2):45–54. doi: 10.1016/0094-5765(94)90116-3
46. Zerath E. Effects of microgravity on bone and calcium homeostasis. Adv Space Res. (1998) 21(8–9):1049–58. doi: 10.1016/S0273-1177(98)00026-X
47. Blaber E, Marçal H, Burns BP. Bioastronautics: the influence of microgravity on astronaut health. Astrobiology. (2010) 10(5):463–73. doi: 10.1089/ast.2009.0415
48. Tamma R, Colaianni G, Camerino C, Di Benedetto A, Greco G, Strippoli M, et al. Microgravity during spaceflight directly affects in vitro osteoclastogenesis and bone resorption. FASEB J. (2009) 23(8):2549–54. doi: 10.1096/fj.08-127951
49. Bajotto G, Shimomura Y. Determinants of disuse—induced skeletal muscle atrophy: exercise and nutrition countermeasures to prevent protein loss. J Nutr Sci Vitaminol. (2006) 52(4):233–47. doi: 10.3177/jnsv.52.233
50. Ferrando AA, Paddon-Jones D, Wolfe RR. Alterations in protein metabolism during space flight and inactivity. Nutrition. (2002) 18(10):837–41. doi: 10.1016/S0899-9007(02)00930-9
51. Fitts RH, Riley DR, Widrick JJ. Functional and structural adaptations of skeletal muscle to microgravity. J Exp Biol. (2001) 204(Pt 18):3201–8. doi: 10.1242/jeb.204.18.3201
52. Ogneva IV. Transversal stiffness of fibers and desmin content in leg muscles of rats under gravitational unloading of various durations. J Appl Physiol. (2010) 109(6):1702–9. doi: 10.1152/japplphysiol.00793.2010
53. Fitts RH, Trappe SW, Costill DL, Gallagher PM, Creer AC, Colloton PA, et al. Prolonged space flight—induced alterations in the structure and function of human skeletal muscle fibres. J Physiol. (2010) 588(Pt 18):3567–92. doi: 10.1113/jphysiol.2010.188508
54. Kalb R, Solomon D. Space exploration, Mars, and the nervous system. Arch Neurol (2007) 64(4):485–90. doi: 10.1001/arch-neur.64.4.485
55. Diedrich A, Paranjape SY, Robertson D. Plasma and blood volume in space. Am J Med Sci. (2007) 334(1):80–5. doi: 10.1097/MAJ.0b013e318065b89b
56. Van Ombergen A, Jillings S, Jeurissen B, Tomilovskaya E, Rumshiskaya A, Litvinova L, et al. Brain ventricular volume changes induced by long-duration spaceflight. Proc Natl Acad Sci. (2019) 116(21):10531–6. doi: 10.1073/pnas.1820354116
57. Van Ombergen A, Jillings S, Jeurissen B, Tomilovskaya E, Rühl RM, Rumshiskaya A, et al. Brain tissue-volume changes in cosmonauts. N Engl J Med. (2018) 379(17):1678–80. doi: 10.1056/NEJMc1809011
58. Roberts DR, Asemani D, Nietert PJ, Eckert MA, Inglesby DC, Bloomberg JJ, et al. Prolonged microgravity affects human brain structure and function. AJNR Am J Neuroradiol. (2019) 40(11):1878–85. doi: 10.3174/ajnr.A6249
59. Roberts DR, Inglesby DC, Brown TR, Collins HR, Eckert MA, Asemani D. Longitudinal change in ventricular volume is accelerated in astronauts undergoing long-duration spaceflight. Aging Brain. (2021) 1:100017. doi: 10.1016/j.nbas.2021.100017
60. Riascos RF, Kamali A, Hakimelahi R, Mwangi B, Rabiei P, Seidler RD, et al. Longitudinal analysis of quantitative brain MRI in astronauts following microgravity exposure. J Neuroimaging. (2019) 29(3):323–30. doi: 10.1111/jon.12609
61. Lee JK, Koppelmans V, Riascos RF, Hasan KM, Pasternak O, Mulavara AP, et al. Spaceflight-associated brain white matter microstructural changes and intracranial fluid redistribution. JAMA Neurol. (2019) 76(4):412–9. doi: 10.1001/jamaneurol.2018.4882
62. Roberts DR, Albrecht MH, Collins HR, Asemani D, Chatterjee AR, Spampinato MV, et al. Effects of spaceflight on astronaut brain structure as indicated on MRI. N Engl J Med. (2017) 377(18):1746–53. doi: 10.1056/NEJMoa1705129
63. Hupfeld KE, McGregor HR, Lee JK, Beltran NE, Kofman IS, De Dios YE, et al. The impact of 6 and 12 months in space on human brain structure and intracranial fluid shifts. Cereb Cortex Commun. (2020) 1(1):tgaa023. doi: 10.1093/texcom/tgaa023
64. Clark TK. Effects of spaceflight on the vestibular system. In: Pathak Y, Araújo dos Santos M, Zea L, editors. Handbook of space pharmaceuticals. Berlin: Springer International Publishing (2019). p. 1–39.
65. Allred AR, Kravets VG, Ahmed N, Clark TK. Modeling orientation perception adaptation to altered gravity environments with memory of past sensorimotor states. Front Neural Circuits. (2023) 17:1190582. doi: 10.3389/fncir.2023.1190582
66. Roll JP, Popov K, Gurfinkel V, Lipshits M, André-Deshays C, Gilhodes JC, et al. Sensorimotor and perceptual function of muscle proprioception in microgravity. J Vestib Res. (1993) 3(3):259–73. doi: 10.3233/VES-1993-3307
67. Mulavara AP, Feiveson AH, Fiedler J, Cohen H, Peters BT, Miller C, et al. Locomotor function after long-duration space flight: effects and motor learning during recovery. Exp Brain Res. (2010) 202(3):649–59. doi: 10.1007/s00221-010-2171-0
68. Wood SJ, Paloski WH, Clark JB. Assessing sensorimotor function following ISS with computerized dynamic posturography. Aerosp Med Hum Perform. (2015) 86(12 Suppl):45–53. doi: 10.3357/AMHP.EC07.2015
70. Paloski WH, Oman CM, Bloomberg JJ, Reschke MF, Wood SJ, Harm DL, et al. Risk of sensory-motor performance failures affecting vehicle control during space missions: a review of the evidence. J Gravitat Physiol. (2008) 15(2):1–29.
71. Oman CM. A heuristic mathematical model for the dynamics of sensory conflict and motion sickness. Acta Otolaryngol Suppl. (1982) 392:1–44. doi: 10.3109/00016488209108197
72. Lackner JR, DiZio P. Space motion sickness. Exp Brain Res (2006) 175(3):377–99. doi: 10.1007/s00221-006-0697-y
73. Williams D, Kuipers A, Mukai C, Thirsk R. Acclimation during space flight: effects on human physiology. CMAJ. (2009) 180(13):1317–23. doi: 10.1503/cmaj.090628
74. Demontis GC, Germani MM, Caiani EG, Barravecchia I, Passino C, Angeloni D. Human pathophysiological adaptations to the space environment. Front Physiol. (2017) 8:547. doi: 10.3389/fphys.2017.00547
75. Jacob P, Oertlin C, Baselet B, Westerberg LS, Frippiat JP, Baatout S. Next generation of astronauts or ESA astronaut 2.0 concept and spotlight on immunity. NPJ Microgravity. (2023) 9(1):51. doi: 10.1038/s41526-023-00294-z
76. Gleeson M, Nieman DC, Pedersen BK. Exercise, nutrition and immune function. J Sports Sci. (2004) 22(1):115–25. doi: 10.1080/0264041031000140590
77. Marcos A, Nova E, Montero A. Changes in the immune system are conditioned by nutrition. Eur J Clin Nutr. (2003) 57(Suppl 1):S66–69. doi: 10.1038/sj.ejcn.1601819
78. Sephton S, Spiegel D. Circadian disruption in cancer: a neuroendocrine-im-mune pathway from stress to disease? Brain Behav Immun. (2003) 17(5):321–8. doi: 10.1016/S0889-1591(03)00078-3
79. Gruenberg DA, Wright RJ, Visness CM, Jaffee KF, Bloomberg GR, Cruikshank WW, et al. Relation between stress and cytokine responses in inner-city mothers. Ann Allergy Asthma Immunol. (2015) 115(5):439–45.e3. doi: 10.1016/j.anai.2015.07.021
80. Crucian BE, Choukèr A, Simpson RJ, Mehta S, Marshall G, Smith SM, et al. Immune system dysregulation during spaceflight: potential countermeasures for deep space exploration missions. Front Immunol. (2018) 9. doi: 10.3389/fimmu.2018.01437
81. Gridley DS, Slater JM, Luo-Owen X, Rizvi A, Chapes SK, Stodieck LS, et al. spaceflight effects on T lymphocyte distribution, function and gene expression. J Appl Physiol. (2009) 106:194–202. doi: 10.1152/japplphys-iol.91126.2008
82. Radstake WE, Baselet B, Baatout S, Verslegers M. Spaceflight stressors and skin health. Biomedicines. (2022) 10(2):364. doi: 10.3390/biomedicines10020364
83. Burge S, Matin R, Wallis D. Structure and function of the skin. In: Oxford Handbook of medical dermatology. Oxford, UK: Oxford University Press (2016). p. 1–16.
84. Gontcharov IB, Kovachevich IV, Pool SL, Navinkov OL, Barratt MR, Bogomolov VV, et al. In-flight medical incidents in the NASA-mir program. Aviat Space Environ Med. (2005) 76(7):692–6.16018356
85. Crucian B, Babiak-Vazquez A, Johnston S, Pierson DL, Ott CM, Sams C, et al. Incidence of clinical symptoms during long-duration orbital spaceflight. Int J Gen Med. (2016) 9:383–91. doi: 10.2147/IJGM.S114188
86. Dunn C, Boyd M, Orengo I. Dermatologic manifestations in spaceflight: a review. Dermatol Online J. (2018) 24(11):13030/qt9dw087tt. doi: 10.5070/D32411042001
87. Voorhies AA, Mark Ott C, Mehta S, Pierson DL, Crucian BE, Feiveson A, et al. Study of the impact of long-duration space missions at the international space station on the astronaut microbiome. Sci Rep. (2019) 9(1):9911. doi: 10.1038/s41598-019-46303-8
88. Baum CL, Arpey CJ. Normal cutaneous wound healing: clinical correlation with cellular and molecular events. Dermatol Surg. (2005) 31(6):674–86. doi: 10.1111/j.1524-4725.2005.31612
89. Bradbury P, Wu H, Choi JU, Rowan AE, Zhang H, Poole K, et al. Modeling the impact of microgravity at the cellular level: implications for human disease. Front Cell Dev Biol. (2020) 8:96. doi: 10.3389/fcell.2020.00096
90. Vandenburgh HH. Mechanical forces and their second messengers in stimulating cell growth in vitro. Am J Physiol. (1992) 262(3 Pt 2):R350–355. doi: 10.1152/ajpregu.1992.262.3.r350
91. Unsworth BR, Lelkes PI. Growing tissues in microgravity. Nat Med. (1998) 4(8):901–7. doi: 10.1038/nm0898-901
92. Imura T, Otsuka T, Kawahara Y, Yuge L. “Microgravity” as a unique and useful stem cell culture environment for cell-based therapy. Regen Ther. (2019) 12:2–5. doi: 10.1016/j.reth.2019.03.001
93. Morbidelli L, Monici M, Marziliano N, Cogoli A, Fusi F, Waltenberger J, et al. Simulated hypogravity impairs the angiogenic response of endothelium by up-regulating apoptotic signals. Biochem Biophys Res Commun. (2005) 334(2):491–9. doi: 10.1016/j.bbrc.2005.06.124
94. Monici M, Fusi F, Paglierani M, Marziliano N, Cogoli A, Pratesi R, et al. Modeled gravitational unloading triggers differentiation and apoptosis in preosteoclastic cells. J Cell Biochem. (2006) 98(1):65–80. doi: 10.1002/jcb.20747
95. Riwaldt S, Corydon TJ, Pantalone D, Sahana J, Wise P, Wehland M, et al. Role of apoptosis in wound healing and apoptosis alterations in microgravity. Front Bioeng Biotechnol. (2021) 9:679650. doi: 10.3389/fbioe.2021.679650
96. Zhang C, Li L, Chen J, Wang J. Behavior of stem cells under outer-space microgravity and ground-based microgravity simulation. Cell Biol Int. (2015) 39(6):647–56. doi: 10.1002/cbin.10452
97. Wostyn P, Mader TH, Gibson CR, Nedergaard M. The effect of long-duration spaceflight on perivascular spaces within the brain. Proc Natl Acad Sci U S A. (2022) 119(32):e2207724119. doi: 10.1073/pnas.2207724119
98. Delp MD, Charvat JM, Limoli CL, Globus RK, Ghosh P. Apollo lunar astronauts show higher cardiovascular disease mortality: possible deep space radiation effects on the vascular endothelium. Sci Rep. (2016) 6:29901. doi: 10.1038/srep29901
99. Afshinnekoo E, Scott RT, MacKay MJ, Pariset E, Cekanaviciute E, Barker R, et al. Fundamental biological features of spaceflight: advancing the field to enable deep-space exploration. Cell. (2020) 183(5):1162–84. doi: 10.1016/j.cell.2020.10.050
100. Tanaka K, Nishimura N, Kawai Y. Adaptation to the microgravity, deconditioning, and countermeasures. J Physiol Sci. (2017) 67(2):271–81. doi: 10.1007/s12576-016-0514-8
101. Liu T, Melkus G, Ramsay T, Sheikh A, Laneuville O, Trudel G. Bone marrow adiposity modulation after long duration spaceflight in astronauts. Nat Commun. (2023) 14(1):4799. doi: 10.1038/s41467-023-40572-8
102. Lewis R. Medical Examination Requirements (MER) for Former Astronauts. NASA (2019). Available at: https://www.NASA.gov/hhp/medical-examination-requirements/ (Accessed November 8, 2021).
103. Walsh L, Hafner L, Straube U, Ulanowski A, Fogtman A, Durante M, et al. A bespoke health risk assessment methodology for the radiation protection of astronauts. Radiat Environ Biophys. (2021) 60(2):213–32. doi: 10.1007/s00411-021-00910-0
104. Straube U. Operational radiation protection for human space flight: the flight surgeon’s perspective. Ann ICRP. (2020) 49(Suppl 1):193. doi: 10.1177/0146645320966570
105. Antonson EL, Myers JG, Boley L, Arellano J, Kerstman E, Kadwa B, et al. Estimating medical risk in human spaceflight. NPJ Microgravity. (2022) 8(1):8. doi: 10.1038/s41526-022-00193-9
106. Gray GW, Sargsyan AE, Davis JR. Clinical risk management approach for long duration space missions. Aviat Space Environ Med. (2010) 81(12):1128–32. doi: 10.3357/ASEM.2829.2010
107. Simonsen LC, Slaba TC. Improving astronaut cancer risk assessment from space radiation with an ensemble model framework. Life Sci Space Res. (2021) 31:14–28. doi: 10.1016/j.lssr.2021.07.002
108. Cockell CS. The meaning of liberty beyond earth. Cham, Switzerland: Springer Publishing (2014). doi: 10.1007/978-3-319-09567-71
109. Lewis R. Medical Examination Requirements (MER) for Former Astronauts. NASA (2017). Available at: https://www.nasa.gov/hhp/medical-examination-requirements (Accessed August 1, 2023).
110. Sgobba T, Landon LB, Marciacq J-B, Groen E, Tikhonov N, Torchia F. Chapter 16—selection and training. In: Sgobba T, Kanki B, Clervoy J, Sandal GM, editors. Space safety and human performance. Oxford, UK: Butterworth-Heinemann (2018). p. 721–93. doi: 10.1016/B978-0-08-101869-9.00016-9
111. Hjelmsø MH, Mollerup S, Jensen RH, Pietroni C, Lukjancenko O, Schultz AC, et al. Metagenomic analysis of viruses in toilet waste from long distance flights—a new procedure for global infectious disease surveillance. PLoS One. (2019) 14(1):e0210368. doi: 10.1371/journal.pone.0210368
112. Mora M, Wink L, Kögler I, Mahnert A, Rettberg P, Schwendner P, et al. Space station condition sare selective but do not alter microbial characteristics relevant to human health. Nat Commun. (2019) 10(1):3990. doi: 10.1038/s41467-019-11682-z
113. McKernan LT, Wallingford KM, Hein MJ, Burge H, Rogers CA, Herrick R. Monitoring microbial populations on wide-body commercial passenger aircraft. Ann Occup Hyg. (2008) 52(2):139–49. doi: 10.1093/annhyg/mem068
114. Mermel LA. Infection prevention and control during prolonged human space travel. Clin Infect Dis. (2013) 56(1):123–30. doi: 10.1093/cid/cis861
115. Cohrs RJ, Mehta SK, Schmid DS, Gilden DH, Pierson DL. Asymptomatic reactivation and shed of infectious varicella zoster virus in astronauts. J Med Virol. (2008) 80(6):1116–22. doi: 10.1002/jmv.21173
116. Khan-Mayberry N, James JT, Tyl R, Lam CW. Space toxicology. Int J Toxicol. (2011) 30(1):3–18. doi: 10.1177/1091581810386389
117. International Atomic Energy Agency. Cytogenetic dosimetry applications in preparedness for and response to radiation emergencies. Vienna: IAEA (2011).
118. Obe G, Johannes I, Johannes C, Hallman K, Reitz G, Facius R. Chromosomal aberrations in blood lymphocytes of astronauts after long-term space flights. Int J Radiat Biol. (1997) 72(6):727–34. doi: 10.1080/095530097142889
119. Testard I, Ricoul M, Hoffschir F, Flury-Herard A, Dutrillaux B, Fedorenko B, et al. Radiation-induced chromosome damage in astronauts’ lymphocytes. Int J Radiat Biol. (1996) 70(4):403–11. doi: 10.1080/095530096144879
120. Durante M. Biomarkers of space radiation risk. Radiat Res. (2005) 164(4 Pt 2):467–73. doi: 10.1667/RR3359.1
121. Horstmann M, Durante M, Johannes C, Pieper R, Obe G. Space radiation does not induce a significant increase of intrachromosomal exchanges in astronauts’ lymphocytes. Radiat Environ Biophys. (2005) 44(3):219–24. doi: 10.1007/s00411-005-0017-0
122. George K, Durante M, Wu H, Willingham V, Badhwar G, Cucinotta FA. Chromosome aberrations in the blood lymphocytes of astronauts after space flight. Radiat Res. (2001) 156(6):731–8. doi: 10.1667/0033-7587(2001)156[0731:CAITBL]2.0.CO;2
123. Beaton-Green LA, Lachapelle S, Straube U, Wilkins RC. Evolution of the health Canada astronaut biodosimetry program with a view toward international harmonization. Mutat Res Genet Toxicol Environ Mutagen. (2015) 793:101–6. doi: 10.1016/j.mrgentox.2015.07.013
124. Lavin KM, Coen PM, Baptista LC, Bell MB, Drummer D, Harper SA, et al. State of knowledge on molecular adaptations to exercise in humans: historical perspectives and future directions. Compr Physiol. (2022) 12(2):3193–279. doi: 10.1002/cphy.c200033
125. DeBord DG, Carreón T, Lentz TJ, Middendorf PJ, Hoover MD, Schulte PA. Use of the “exposome” in the practice of epidemiology: a primer on -omic technologies. Am J Epidemiol. (2016) 184(4):302–14. doi: 10.1093/aje/kwv325
126. Kodaira S, Naito M, Uchihori Y, Hashimoto H, Yano H, Yamagishi A. Space radiation dosimetry at the exposure facility of the international space station for the tanpopo mission. Astrobiol. (2021) 21(12):1473–8. doi: 10.1089/ast.2020.2427
127. Boscolo D, Durante M. Dose limits and countermeasures for mitigating radiation risk in moon and Mars exploration. Physics (College Park Md). (2022) 4(1):172–84. doi: 10.3390/physics4010013
128. Hughson RL, Helm A, Durante M. Heart in space: effect of the extraterrestrial environment on the cardiovascular system. Nat Rev Cardiol. (2018) 15(3):167–80. doi: 10.1038/nrcardio.2017.157
129. Spillantini P, Casolino M, Durante M, Mueller-Mellin R, Reitz G, Rossi L, et al. Shielding from cosmic radiation for interplanetary missions: active and passive methods. Radiat Meas. (2007) 42(1):14–23. doi: 10.1016/j.radmeas.2006.04.028
130. Vogler FH. Analysis of an electrostatic shield for space vehicles. AIAA J. (1964) 2(5):872–8. doi: 10.2514/3.2451
131. Frisina W. Optimizing electrostatic radiation shielding for manned space vehicles. Acta Astronaut. (1985) 12(12):995–1003. doi: 10.1016/0094-5765(85)90028-1
132. Metzger PT, Youngquist RC, Lane JE. Asymmetric electrostatic radiation shielding for spacecraft. 2004 IEEE Aerospace Conference Proceedings (IEEE Cat. No.04TH8720); Vol. 1 (2004). p. 637
133. Tripathi RK, Wilson JW, Youngquist RC. Electrostatic active radiation shielding—revisited. Aerospace Conference; IEEE (2006). doi: 10.1109/AERO.2006.1655760
134. Tripathi RK, Wilson JW, Youngquist RC. Electrostatic space radiation shielding. Adv Space Res. (2008) 42(6):1043–9. doi: 10.1016/j.asr.2007.09.015
135. Joshi RP, Qiu H, Tripathi RK. Configuration studies for active electrostatic space radiation shielding. Acta Astronaut. (2013) 88:138–45. doi: 10.1016/j.actaastro.2013.03.011
136. Levy RH. “Radiation shielding of space vehicles by means of superconducting coils,” design and reliability, and invited addresses. Cambridge, MA: Elsevier (1961). p. 119–55. doi: 10.1016/B978-1-4832-2899-0.50011-1
137. Cougnet C, Crosby NB, Eckersley S, Foullon C, Guarneiri V, Guatelli S, et al. Radiation exposure and mission strategies for interplanetary manned missions (REMSIM). Earth Moon Planets. (2005) 94(3–4):279–85. doi: 10.1007/s11038-005-9014-1
138. Bruce R, Baudouy B. Cryogenic design of a large superconducting magnet for astro-particle shielding on deep space travel missions. Phys Procedia. (2015) 67:264–9. doi: 10.1016/j.phpro.2015.06.085
139. Ambroglini F, Battiston R, Burger WJ. Evaluation of superconducting magnet shield configurations for long duration manned space missions. Front Oncol. (2016) 6:97. doi: 10.3389/fonc.2016.00097
140. Levy RH, Janes GS. Plasma radiation shielding. AIAA J. (1964) 2(10):1835–8. doi: 10.2514/3.2683
141. Levy RH, French FW. Plasma radiation shield—concept and applications to space vehicles. J Spacecr Rockets. (1968) 5(5):570–7. doi: 10.2514/3.29306
142. Winglee RM, Slough J, Ziemba T, Goodson A. Mini-magnetospheric plasma propulsion: tapping the energy of the solar wind for spacecraft propulsion. J Geophys Res Space Phys. (2000) 105(A9):21067–77. doi: 10.1029/1999JA000334
143. Bamford R, Gibson KJ, Thornton AJ, Bradford J, Bingham R, Gargate L, et al. The interaction of a flowing plasma with a dipole magnetic field: measurements and modelling of a diamagnetic cavity relevant to spacecraft protection. Plasma Phys Control Fusion. (2008) 50(12):124025. doi: 10.1088/0741-3335/50/12/124025
144. Gargaté L, Bingham R, Fonseca RA, Bamford R, Thornton A, Gibson K, et al. Hybrid simulations of mini-magnetospheres in the laboratory. Plasma Phys Control Fusion. (2008) 50(7):074017. doi: 10.1088/0741-3335/50/7/074017
145. Bamford RA, Kellet B, Bradford J, Todd TN, Benton MG Sr, Stafford-Allen R, et al. An exploration of the effectiveness of artificial mini-magnetospheres as a potential solar storm shelter for long term human space missions. Acta Astronaut. (2014) 105(2):385–94. doi: 10.1016/j.actaastro.2014.10.012
146. Sussingham JC, Watkins SA, Cocks FH. Forty years of development of active systems for radiation protection of spacecraft. J Astronaut Sci. (1999) 47(3–4):165–75. doi: 10.1007/BF03546197
147. Townsend LW. Overview of active methods for shielding spacecraft from energetic space radiation. Phys Med. (2001) 17:84–5.11770543
148. Spillantini P. Active shielding for long duration interplanetary manned missions. Adv Space Res. (2010) 45(7):900–16. doi: 10.1016/j.asr.2010.01.025
149. Vuolo M, Giraudo M, Musenich R, Calvelli V, Ambroglini F, Burger WJ, et al. Monte carlo simulations for the space radiation superconducting shield project (SR2S). Life Sci Space Res. (2016) 8:22–9. doi: 10.1016/j.lssr.2015.12.003
150. Zeitlin C, Guetersloh S, Heilbronn L, Miller J, Elkhayari N, Empl A, et al. Shielding experiments with high-energy heavy ions for spaceflight applications. New J Phys. (2008) 10(7):075007. doi: 10.1088/1367-2630/10/7/075007
151. Durante M. Space radiation protection: destination Mars. Life Sci Space Res. (2014) 1:2–9. doi: 10.1016/j.lssr.2014.01.002
152. Giraudo M, Schuy C, Weber U, Rovituso M, Santin G, Norbury JW, et al. Accelerator-based tests of shielding effectiveness of different materials and multilayers using high-energy light and heavy ions. Radiat Res. (2018) 190(5):526–37. doi: 10.1667/RR15111.1
153. Horst F, Boscolo D, Durante M, Luoni F, Schuy C, Weber U. Thick shielding against galactic cosmic radiation: a monte carlo study with focus on the role of secondary neutrons. Life Sci Space Res. (2022) 33:58–68. doi: 10.1016/j.lssr.2022.03.003
154. Cucinotta FA. Space radiation risks for astronauts on multiple international space station missions. PLoS One. (2014) 9(4):1–14. doi: 10.1371/journal.pone.0096099
155. Washburn SA, Blattnig SR, Singleterry RC, Westover SC. Active magnetic radiation shielding system analysis and key technologies. Life Sci Space Res. (2015) 4:22–34. doi: 10.1016/j.lssr.2014.12.004
156. NASA. NASA Space flight human-system standard volume 1, revision a: Crew health. Washington, DC: National Aeronautics and Space Administration (2015).
157. Singleterry RC. Radiation engineering analysis of shielding materials to assess their ability to protect astronauts in deep space from energetic particle radiation. Acta Astronaut. (2013) 91:49–54. doi: 10.1016/j.actaastro.2013.04.013
158. Fogtman A, Baatout S, Baselet B, Berger T, Hellweg CE, Jiggens P, et al. Towards sustainable human space exploration—priorities for radiation research to quantify and mitigate radiation risks. NPJ Microgravity. (2023) 9(1):8. doi: 10.1038/s41526-023-00262-7
159. Miller J, Taylor L, Zeitlin C, Heilbronn L, Guetersloh S, DiGiuseppe M, et al. Lunar soil as shielding against space radiation. Radiat Meas. (2009) 44(2):163–7. doi: 10.1016/j.radmeas.2009.01.010
160. Ferrone KL, Taylor AB, Helvajian H. In situ resource utilization of structural material from planetary regolith. Adv Space Res. (2022) 69(5):2268–82. doi: 10.1016/j.asr.2021.12.025
161. Sangwan N, Kumar A. An extensive study of depth dose distribution and projectile fragmentation cross-section for shielding materials using Geant4. Appl Radiat Isot. (2022) 180:110068. doi: 10.1016/j.apradiso.2021.110068
162. Wang J, Li Q, Liu D, Chen C, Chen Z, Hao J, et al. High temperature thermally conductive nanocomposite textile by “green” electrospinning. Nanoscale. (2018) 10(35):16868–72. doi: 10.1039/c8nr05167d
163. Sauro F, Pozzobon R, Massironi M, De Berardinis P, Santagata T, De Waele J. Lava tubes on earth, moon and Mars: a review on their size and morphology revealed by comparative planetology. Earth-Sci Rev. (2020) 209:103288. doi: 10.1016/j.earscirev.2020.103288
164. Boston PJ, Frederick RD, Welch SM, Werker J, Meyer TR, Sprungman B, et al. Human utilization of subsurface extraterrestrial environments. Gravit Space Biol Bull. (2023) 16(2):121–31.
165. Wynne JJ, Titus TN, Agha-Mohammadi AA, Azua-Bustos A, Boston PJ, de León P, et al. Fundamental science and engineering questions in planetary cave exploration. J Geophys Res. (2022) 127(11):e2022JE007194. doi: 10.1029/2022JE007194
166. Pal Chowdhury R, Stegeman LA, Lund ML, Fry D, Madzunkov S, Bahadori AA. Hybrid methods of radiation shielding against deep-space radiation. Life Sci Space Res. (2023) 38:67–78. doi: 10.1016/j.lssr.2023.04.004
167. McLaughlin MF, Donoviel DB, Jones JA. Novel indications for commonly used medications as radiation protectants in space flight. Aerosp Med Hum Perform. (2017) 88(7):665–76. doi: 10.3357/AMHP.4735.2017
168. Montoro A, Obrador E, Mistry D, Forte GI, Bravatà V, Minafra L, et al. Chapter 11: radioprotectors, radiomitigators and radiosensitizers. In: Baatout S, editor. Radiobiology textbook. Cham, Switzerland: Springer (2023). In Press.
169. Mun GI, Kim S, Choi E, Kim CS, Lee YS. Pharmacology of natural radioprotectors. Arch Pharm Res. (2018) 41(11):1033–50. doi: 10.1007/s12272-018-1083-6
170. Jones JA, Epperly M, Law J, Scheuring R, Montesinos C, Popov D, et al. Space radiation hazards and strategies for astronaut/cosmonaut protection. Medical Radiology and Radiation Safety. (2013) 58(3):5–23.
171. Jones JA, Karouia F. Radiation disorders. In: Barratt M, Pool SL, editors. Principles of clinical medicine for space flight. 1st ed. New York, NY: Springer, (2008). p. 475–519.
172. Tokatli F, Uzal C, Doganay L, Kocak Z, Kaya M, Ture M, et al. The potential cardioprotective effects of amifostine in irradiated rats. Int J Radiat Oncol Biol Phys. (2004) 58(4):1228–34. doi: 10.1016/j.ijrobp.2003.09.071
173. Cortese F, Klokov D, Osipov A, Stefaniak J, Moskalev A, Schastnaya J, et al. Vive la radiorésistance!: converging research in radiobiology and biogerontology to enhance human radioresistance for deep space exploration and colonization. Ocontarget. (2018) 9(18):14692–722. doi: 10.18632/oncotarget.24461
174. Greenberger JS. Gene therapy for radiation protection. Gene Ther. (1999) 6:1495–6. doi: 10.1038/sj.gt.3301005
175. Zhang X, Epperly MW, Kay MA, Smith T, Franicola D, Greenberger B, et al. Radioprotection in vitro and in vivo by mini circle plasmid containing the human manganese superoxide dismutase (MnSOD) transgene. Hum Gene Ther. (2008) 19(8):820–6. doi: 10.1089/hum.2007.141
176. Epperly MW, Bray JA, Krager S, Berry LM, Gooding W, Engelhardt JF, et al. Intratracheal injection of adenovirus containing the human MnSOD transgene protects athymic nude mice from irradiation-induced organizing alveolitis. Int J Radiat Oncol Biol Phys. (1999) 43(1):169–81. doi: 10.1016/S0360-3016(98)00355-1
177. Frosina G. Overexpression of enzymes that repair endogenous damage to DNA. Eur J Biochem. (2000) 267(8):2135–49. doi: 10.1046/j.1432-1327.2000.01266.x
178. Frosina G. Counteracting spontaneous transformation via overexpression of rate-limiting DNA base excision repair enzymes. Carcinogenesis. (2001) 22(9):1335–41. doi: 10.1093/carcin/22.9.1335
179. Jönsson KI, Rabbow E, Schill RO, Harms-Ringdahl M, Rettberg P. Tardigrades survive exposure to space in low earth orbit. Curr Biol. (2008) 18(17):R729–31. doi: 10.1016/j.cub.2008.06.048
180. Hashimoto T, Horikawa DD, Saito Y, Kuwahara H, Kozuka-Hata H, Shin-I T, et al. Extremotolerant tardigrade genome and improved radiotolerance of human cultured cells by tardigrade-unique protein. Nat Commun. (2016) 7:12808. doi: 10.1038/ncomms12808
181. Andreassen CN, Rosenstein BS, Kerns SL, Ostrer H, De Ruysscher D, Cesaretti JA, et al. Individual patient data meta-analysis shows a significant association between the ATM rs1801516 SNP and toxicity after radiotherapy in 5456 breast and prostate cancer patients. Radiother Oncol. (2016) 121(3):431–9. doi: 10.1016/j.radonc.2016.06.017
182. Kato TA, Wilson PF, Nagasaw H, Peng Y, Weil MM, Little JB, et al. Variations in radiosensitivity among individuals: a potential impact on risk assessment? Health Phys. (2009) 97(5):470–80. doi: 10.1097/HP.0b013e3181b08eee
183. Kawecki TJ, Lenski RE, Ebert D, Hollis B, Olivieri I, Whitlock MC. Experimental evolution. Trends Ecol Evol. (2012) 27(10):547–60. doi: 10.1016/j.tree.2012.06.001
184. Byrne RT, Klingele AJ, Cabot EL, Schackwitz WS, Martin JA, Martin J, et al. Evolution of extreme resistance to ionizing radiation via genetic adaptation of DNA repair. eLife. (2014) 3:e01322. doi: 10.7554/eLife.01322
185. Musacchia XJ, Barr RE. Survival of whole-body-irradiated hibernating and active ground squirrels; citellus tridecemlineatus. Radiat Res. (1968) 33(2):348–56. doi: 10.2307/3572485
186. Cerri M, Tinganelli W, Negrini M, Helm A, Scifoni E, Tommasino F, et al. Hibernation for space travel: impact on radioprotection. Life Sci Space Res. (2016) 11:1–9. doi: 10.1016/j.lssr.2016.09.001
187. Wilson WR, Hay MP. Targeting hypoxia in cancer therapy. Nat Rev Cancer. (2011) 11(6):393–410. doi: 10.1038/nrc3064
188. Choukèr A, Bereiter-Hagn J, Singer D, Heldmaier G. Hibernating astronauts—science or fiction?,” pflugers arch—eur. J Physiol. (2019) 471(6):819–28. doi: 10.1007/s00424-018-2244-7
189. Choukèr A, Jennifer Ngo-Anh T, Briesbroek R, Heldmaier G, Heppener M, Bereiter-Hahn J. European space agency’s hibernation (torpor) strategy for deep space missions: linking biology to engineering. Neurosci Biobehav Rev. (2021) 131:618–26. doi: 10.1016/j.neubiorev.2021.09.054
190. Tinganelli W, Hitrec T, Romani F, Simoniello P, Squarcio F, Stanzani A, et al. Hibernation and radioprotection: gene expression in the liver and testicle of rats irradiated under synthetic torpor. Int J Mol Sci. (2019) 20(2):352. doi: 10.3390/ijms20020352
191. Cerri M, Mastrotto M, Tupone D, Martelli D, Luppi M, Perez E, et al. The inhibition of neurons in the central nervous pathways for the thermoregulatory cold defense induced a suspended animation state in the rat. J Neurosci. (2013) 33(7):2984–93. doi: 10.1523/JNEUROSCI.3596-12.2013
192. Cahill T, da Silveira WA, Renaud L, Williamson T, Wang H, Chung D, et al. Induced torpor as a countermeasure for low dose radiation exposure in a zebrafish model. Cells. (2021) 10(14):906. doi: 10.3390/cells10040906
193. Logan SM, Luu BE, Storey KB. Turn down genes for WAT? Activation of anti-apoptosis pathways protects white adipose tissue in metabolically depressed thirteen-lined ground squirrels. Mol Cell Biochem. (2016) 416(1–2):47–62. doi: 10.1007/s11010-016-2695-0
194. Rouble AN, Hefler J, Mamady H, Storey KB, Tessier SN. Anti-apoptotic signalling as a cytoprotective mechanism in mammalian hibernation. Peer J. (2013) 1:e29. doi: 10.7717/peerj.29
195. Fleck CC, Carey HV. Modulation of apoptotic pathways in intestinal mucosa during hibernation. Am J Physiol Regul Integr Comp Physiol. (2005) 289(2):R586–95. doi: 10.1152/ajpregu.00100.2005
196. Bouma HR, Verhaag EM, Otis JP, Heldmaier G, Swoap SJ, Strijkstra AM, et al. Induction of torpor: mimicking natural metabolic suppression for biomedical applications. J Cell Physiol. (2011) 227(4):1285–90. doi: 10.1002/jcp.22850
197. National Research Council. Space radiation hazards and the vision for space exploration: Report of a workshop. Washington, DC: The National Academies Press (2006).
198. Norbury JW, Schimmerling W, Slaba TC, Azzam EI, Badavi FF, Baiocco G, et al. Galactic cosmic ray simulation at the NASA space radiation laboratory. Life Sci Space Res. (2016) 8:38–51. doi: 10.1016/j.lssr.2016.02.001
199. Straube U, Berger T, Reitz G, Facius R, Fuglesang C, Reiter T, et al. Operational radiation protection for astronauts and cosmonauts and correlated activities of ESA medical operations. Acta Astronaut. (2010) 66(7):963–73. doi: 10.1016/j.actaastro.2009.10.004
200. ICRP. The 2007 recommendations of the international commission on radiological protection. ICRP publication 103. Ann ICRP. (2007) 37(2–4):1–332.
201. International Commission on Radiological Protection, ICRP. 1990 Recommendations of the international commission on radiological protection. ICRP publication 60. Ann ICRP. (1990) 21 (1–3):1–201.
202. Shavers M, Semones E, Tomi L, Chen J, Straube U, Komiyama T, et al. Space agency-specific standards for crew dose and risk assessment of ionising radiation exposures for the international space station. J Med Phy. (2023). S0939-3889(23)00082-X. doi: 10.1016/j.zemedi.2023.06.005. [Epub ahead of print]
203. International Space Station Program. Medical evaluations document, volume A, (MEDA), medical standards for ISS crewmembers, SSP 50667. Houston, TX: NASA-JSC (2004).
204. International Space Station Programme. “International space station medical operations requirements documents (ISS MORD),” SSP 50260, revision B. Houston, TX: National Aeronautics and Space Administration (2003).
205. NASA. NASA Space flight human-system standard: Volume 1: Crew health. San Francisco: National Aeronautics and Space Administration (2007).
206. National Academies of Sciences, Engineering, and Medicine (2021). Space radiation and astronaut health: Managing and communicating cancer risks. Washington DC: The National Academies Press (2021).
207. Zhang S, Wimmer-Schweingruber RF, Yu J, Wang C, Fu Q, Zou Y, et al. First measurements of the radiation dose on the lunar surface. Sci Adv. (2020) 6(39):eaaz1334. doi: 10.1126/sciadv.aaz1334
208. Narici L, Berger T, Matthiä D, Reitz G. Radiation measurements performed with active detectors relevant for human space exploration. Front Oncol. (2015) 5(273):273. doi: 10.3389/fonc.2015.00273
209. Sawyer DM, Vette JI. “AP-8 trapped proton environment for solar maximum and solar minimum,” NASA TM-X-72605 (1976).
211. Abel B, Thorne M, Vampola AL. Solar cyclic behavior of trapped energetic electrons in earth’s inner radiation belt. J Geophys Res. (1994) 99(A10):19,427–31. doi: 10.1029/94JA01626
212. Heynderickx D. Comparison between methods to compensate for the secular motion of the south atlantic anomaly. Rad Meas. (1996) 26(3):369–73. doi: 10.1016/1350-4487(96)00056-X
213. Daly EJ, Evans HDR. Problems in radiation environment models at low altitudes. Rad Meas. (1996) 26(3):363–8. doi: 10.1016/1350-4487(96)00058-3
214. Armstrong TW, Colburn BL. “Evaluation of trapped radiation model uncertainties for spacecraft design,” NASA/CR-2000-210072 (2000).
215. Seltzer SM. “SHIELDOSE, A Computer Code for Space-Shielding Radiation Dose Calculations,” National Bureau of Standards, NBS Technical Note 1116, U.S. Government Printing Office, Washington, D.C. (1980).
216. Heynderickx D, Kruglanski M, Pierrard V, Lemaire J, Looper MD, Blake JB. A low altitude trapped proton model for solar minimum conditions based on SAMPEX/PET data. IEEE Trans Nucl Sci. (1999) 46(6):1475–80. doi: 10.1109/23.819110
217. Huston SL, Kauffman W. “Space Environments and Effects: Trapped Proton Model,” NASA/CR-2002-211784 (2002).
218. Boscher DM, Bourdarie SA, Friedel RHW, Belian RD. Model for the geostationary electron environment: POLE. IEEE Trans Nucl Sci. (2003) 50(6):2278–83. doi: 10.1109/TNS.2003.821609
219. Huston SL, Pfitzer KA. “Space Environment Effects: Low-Altitude Trapped Radiation Model,” NASA/CR-1998-208593 (1998).
220. Gussenhoven MS, Mullen EG, Violet MD, Hein C, Bass J, Madden D. CRRES high energy proton flux maps. IEEE Trans Nucl Sci. (1993) 40(6):1450–7. doi: 10.1109/23.273519
221. Wrenn GL, Rodgers DJ, Buehler P. Modeling the outer belt enhancements of penetrating electrons. J Spacecraft Rockets. (2000) 37(3):408–15. doi: 10.2514/2.3575
222. Brautigam DH, Gussenhoven MS, Mullen EG. Quasi-static model of outer zone electrons. IEEE Trans Nucl Sci. (1992) 39(6):1797–803. doi: 10.1109/23.211369
223. Liddell LC, Gentry DM, Gilbert R, Marina D, Massaro Tieze S, Padgen MR, et al. Biosentinal: validating sensitivity of yeast biosensors to deep space relevant radiation. Astrobiology. (2023) 23(6):648–56. doi: 10.1089/ast.2022.0124
224. Rahmanian S, Slaba TC, Braby LA, Santa Maria SR, Bhattacharya S, Straume T. Galactic cosmic ray environment predictions for the NASA BioSentinal mission. Life Sci Space Res. (2023) 38:19–28. doi: 10.1016/j.lssr.2023.05.001
225. Roda A, Mirasoli M, Guardigli M, Zangheri M, Caliceti C, Calabria D, et al. Advanced biosensors for monitoring astronauts’ health during long-duration space missions. Biosens Bioelectron. (2018) 111:18–26. doi: 10.1016/j.bios.2018.03.062
226. Calabria D, Trozzi I, Lazzarini E, Pace A, Zangheri M, Iannascoli L, et al. AstroBio-CubeSat: a lab-in-space for chemiluminescence-based astrobiology experiments. Biosens Bioelectron. (2023) 226:115110. doi: 10.1016/j.bios.2023.115110
Keywords: space radiation, microgravity, risk assessments, mitigations, radiation protection
Citation: Dobney W, Mols L, Mistry D, Tabury K, Baselet B and Baatout S (2023) Evaluation of deep space exploration risks and mitigations against radiation and microgravity. Front. Nucl. Med. 3:1225034. doi: 10.3389/fnume.2023.1225034
Received: 18 May 2023; Accepted: 4 September 2023;
Published: 21 September 2023.
Edited by:
Alexandros G. Georgakilas, National Technical University of Athens, GreeceReviewed by:
Emilio Mezzenga, Scientific Institute of Romagna for the Study and Treatment of Tumors (IRCCS), Italy© 2023 Dobney, Mols, Mistry, Tabury, Baselet and Baatout. This is an open-access article distributed under the terms of the Creative Commons Attribution License (CC BY). The use, distribution or reproduction in other forums is permitted, provided the original author(s) and the copyright owner(s) are credited and that the original publication in this journal is cited, in accordance with accepted academic practice. No use, distribution or reproduction is permitted which does not comply with these terms.
*Correspondence: Sarah Baatout, c2FyYWguYmFhdG91dEBzY2tjZW4uYmU=
†These authors have contributed equally to this work and share first authorship
Disclaimer: All claims expressed in this article are solely those of the authors and do not necessarily represent those of their affiliated organizations, or those of the publisher, the editors and the reviewers. Any product that may be evaluated in this article or claim that may be made by its manufacturer is not guaranteed or endorsed by the publisher.
Research integrity at Frontiers
Learn more about the work of our research integrity team to safeguard the quality of each article we publish.