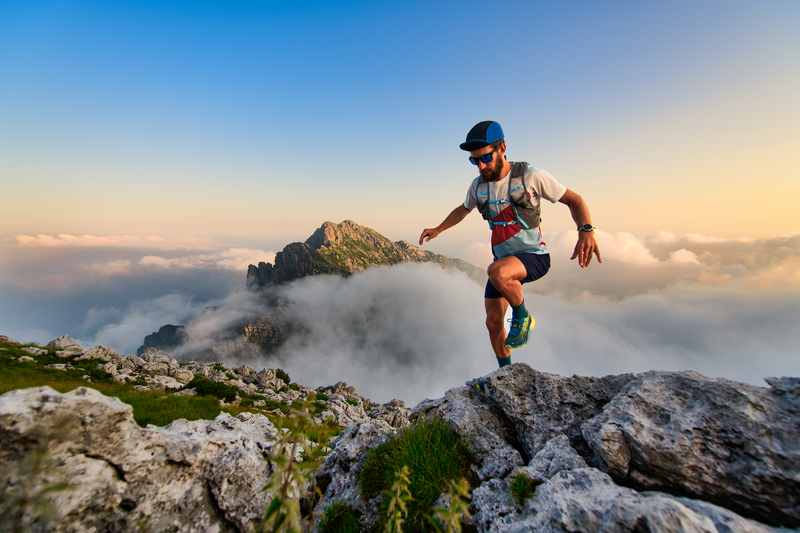
95% of researchers rate our articles as excellent or good
Learn more about the work of our research integrity team to safeguard the quality of each article we publish.
Find out more
PERSPECTIVE article
Front. Nucl. Eng. , 25 March 2025
Sec. Nuclear Materials
Volume 4 - 2025 | https://doi.org/10.3389/fnuen.2025.1569103
This article is part of the Research Topic Accelerating Nuclear Materials Research and Development through Advanced Characterization View all articles
This perspective explores recent advancements in the synthesis and application of uranium fluoride micromaterials, emphasizing their role in the nuclear industry. Uranium micromaterials, including oxides, fluorides, and carbides, are crucial for applications ranging from high-temperature gas-cooled reactors to nuclear forensics and medical isotope production. The perspective highlights a novel chemical transformation process for synthesizing uranium fluoride micromaterials, in which uranium oxides are fluorinated in an autoclave using HF gas (generated from the decomposition of silver bifluoride) or ammonium bifluoride while preserving their original morphologies. This transformation produces various uranium fluoride microstructures, including UF4, UO2F2, and (NH4)3UO2F5, in the form of microrods, microplates, and microspheres. The perspective discusses challenges in maintaining controlled morphologies during fluorination and explores future directions, such as the synthesis of actinide fluoride micromaterials and the development of uranium chloride and other uranium compounds. The continued advancement of these materials holds significant potential for innovations in nuclear fuel cycles, actinide material chemistry, and nuclear forensics.
Uranium materials with well-defined micro-scale morphologies have diverse applications in the nuclear industry. A key example is their use in high-temperature gas-cooled reactors (HTGRs), where tristructural-isotropic (TRISO) fuel—spherical uranium oxide and/or carbide particles with protective coatings—is molded into rods for prismatic-block designs or dispersed as pebbles in pebble-bed reactors (Ueta et al., 2011).
Historically, the United States operated two prismatic-type HTGRs: Peach Bottom (1967–1974) and Fort Saint Vrain (1979–1989) (Beck and Pincock, 2011). More recently, China has developed two Generation IV pebble-bed HTGRs (HTR-PM), scaled up from the earlier HTR-10 prototype, which remains operational (Beck and Pincock, 2011; IAEA, 2011).
Beyond reactor applications, uranium micromaterials play a critical role in environmental and nuclear forensics monitoring. Reference particles with precisely controlled size and shape are used to validate highly sensitive analytical measurements at nuclear facilities. Techniques such as Large Geometry-Secondary Ion Mass Spectrometry, Thermal Ionization Mass Spectrometry, and Multi-Collector Inductively Coupled Plasma Mass Spectrometry enable precise isotopic characterization (Kegler et al., 2021). In 2022, the first uranium oxide microparticle reference materials were officially certified, marking a significant milestone in the field (Richter et al., 2022).
The future applications of uranium microstructured materials are extensive. One emerging area is the fluoride Salt-Cooled High-Temperature Reactor (FHR), a next-generation molten salt reactor (MSR) design that utilizes solid TRISO fuel rather than dissolving fuel into the molten salt coolant (Serp et al., 2014; Qualls et al., 2017). In the United States, Kairos Power is leading FHR development (Fluoride Salt-Cooled Reactor, 2023).
Beyond reactor technology, uranium micromaterials have potential applications in medical isotope production (Henry and Saclay, 1959; Passy and Steiger, 1963; Dorhout et al., 2019), space exploration as energy sources (Carmack et al., 2004), and functional materials for information storage, catalysis, sensors, and luminescent devices (Zhang et al., 2005; Kim et al., 2006; Xu et al., 2007; Pradhan et al., 2011).
A promising approach for synthesizing uranium micromaterials is chemical transformation—a process that selectively modifies the chemical composition, crystal structure, or morphology of micro- and nanoscale materials (Moon et al., 2011; Fayette and Robinson, 2014). This technique is widely used in energy applications, such as MoO3–MoS2 nanowires for hydrogen evolution reactions (Chen et al., 2011), Co–CoO nanoparticles for oxygen reduction reactions (Guo et al., 2012), and Cu–CuxS nanotubes for Li-ion battery anodes (Cai et al., 2012).
A recent chemical transformation process for uranium oxide micromaterials was developed (Jang et al., 2023; Jang and Poineau, 2024a; 2024b). This solid-gas reaction replaces oxygen atoms with fluorine atoms while preserving the material’s original morphology. The transformation is achieved by generating in situ HF gas from bifluoride salts under controlled conditions at mild temperatures (150°C–250°C). Uranium fluorides are essential to the nuclear fuel cycle, particularly as uranium hexafluoride (UF6) in enrichment and uranium tetrafluoride (UF4) as a primary fuel for MSRs. Developing tailored uranium fluoride micromaterials is crucial for advancing next-generation reactor designs. Additionally, different uranium fluoride micromaterials may exhibit distinct nuclear signatures influenced by particle geometry, which is relevant to nuclear forensics—such as the presence of 22Na from potential 19F(α,n)22Na reactions (Croft et al., 2019).
Historically, research on uranium microstructures has focused on binary oxides, nitrides, and carbides, while uranium fluoride micromaterials remained largely unexplored. This perspective highlights recent advancements in the field of uranium fluoride micromaterials and their potential implications.
The experimental setup for preparing uranium micromaterials has been well documented (Jang et al., 2023; Jang and Poineau, 2024a; Jang and Poineau, 2024b). Uranium oxide microparticles were synthesized hydrothermally and fluorinated using Parr model 4749 general-purpose acid digestion vessels (autoclaves). During fluorination, uranium oxide precursors were placed inside a smaller Teflon vial nested within the Teflon liner of the autoclave, which contained either silver bifluoride (AgHF2, SBF) or ammonium bifluoride (NH4HF2, ABF). Upon heating, the gaseous decomposition products of the bifluoride salts—HF gas (HFg) from SBF and HF/NH4F/NH3 from ABF—interacted with the uranium oxide within the inner Teflon vial, enabling controlled fluorination.
Fluorination of uranium oxides with HFg is a straightforward process, primarily dictated by the oxidation state of the starting material. When UO2 is used, the reaction yields UF4, following the standard industrial method. In contrast, fluorination of U3O8 or UO3 produces UO2F2, with potential hydration due to water formation as a reaction byproduct (Equations 1, 2, respectively).
Uranium fluorides containing the ammonium cation (NH4+) can be synthesized using ammonium bifluoride. Fluorination of UO2 yields (NH4)4UF8, whereas U3O8 or UO3 produces (NH4)3UO2F5, both with the possibility of hydration (Equations 3, 4, respectively). In all cases, the presence of water can induce side reactions, necessitating careful control.
Recent studies have reported various microstructures of anhydrous and hydrated UO2F2, including microspheres (ms), microrods (mr), and microplates (mp) (Jang et al., 2023; Jang and Poineau, 2024a; Jang and Poineau, 2024b). These materials were synthesized by reacting hydrothermally precipitated UO3 or U3O8 with in situ generated HF gas. Results indicate that temperature plays a crucial role in hydration: reactions conducted at 200°C–250°C consistently produced anhydrous UO2F2 across all microstructures (Figures 1a–c). In contrast, lower-temperature reactions initially formed hydrated UO2F2, which subsequently converted to its anhydrous form upon heating to 250°C (Figure 1d). The release of bound water at around 250°C is a classical behavior commonly exhibited in oxyhydroxides (Lee et al., 1980; Frolova, 2021).
Figure 1. Uranium fluoride microstructures prepared: (a) UO2F2 ms obtained from the reaction between UO3 ms and SBF at 200°C for 24 h (b) UO2F2 mr obtained from the reaction between U3O8 mr and SBF at 250°C for 24 h (c) UO2F2 mp from the reaction between U3O8 mp and SBF at 250°C for 24 h (d) UO2F2 ms obtained from the treatment of UO2F2·1.5H2O ms at 250°C for 5 days (e) UF4/UF4·2H2O mr obtained from the reaction between UO2 mr and SBF for 24 h at 250°C (f) UF4/UF4·xH2O mr obtained from the reaction between UO2 mr and SBF for 24 h at 250°C prepared under N2 atmosphere (g) UF4·1.5H2O mr obtained from the reaction between UO2 mr and SBF for 24 h at 150°C. (h) UF4 mr obtained from the treatment of UF4 mr (Figure 1e) at 600 °C under argon atmosphere, (i) UF4 mp obtained from the reaction of UO2 mp and SBF at 250 °C.
Microspheres of anhydrous UF4 (∼20 μm) are commercially available from International Bio-Analytical Industries, with several SEM characterizations reported in the literature (Plaue, 2013; Villa-Aleman and Wellons, 2016; Christian et al., 2021; Foley et al., 2022). These UF4 microspheres were reportedly produced via anhydrous hydrofluorination reactions with HF (Foley et al., 2022). Additionally, the room-temperature hydrolysis of UF4 microspheres has been shown to yield UF4(H2O)2.5 microrods (Christian et al., 2021).
Microrods and microplates of both anhydrous and hydrated UF4 have been synthesized via chemical transformation reactions. A study investigating the influence of experimental parameters on morphology found that reactions between UO2 microrods and SBF at 250°C resulted in mixed phases of anhydrous and hydrated UF4 microrods when conducted in air (Figure 1e) and under a nitrogen atmosphere (Figure 1f), with the latter exhibiting a notably rougher surface morphology. In contrast, reactions at 150°C produced UF4‧1.5H2O microrods as a single phase with a smooth morphology (Figure 1g). The anhydrous UF4 microrods shown in Figure 1e were further converted through thermal treatment at 600°C under an argon atmosphere (Figure 1h).
Additionally, reactions between UO2 microplates and SBF at 250 °C directly yielded anhydrous UF4 microplates (Figure 1i). A summary of uranium fluoride microstructures prepared via chemical transformation is presented in Table 1.
Table 1. Reported shape, size, and preparation method of uranium fluorides materials. (ms: microsphere, mr: microrod, mp: microplate).
The chemical transformation of uranium oxide microstructures using ammonium bifluoride as the fluorinating agent has been investigated. In most cases, morphological preservation was not achieved. For instance, reactions between ABF and UO2 microspheres (ms) yielded coalesced particles of (NH4)2UF6 ms, while transformations involving U3O8 microrods (mr) and U3O8 microplates (mp) produced deformed (NH4)3UO2F5 mr and large pebble-like clusters of (NH4)3UO2F5, respectively. In these reactions, the NH3 generated as a decomposition product significantly altered the material’s morphology.
The only successful reaction that maintained the original morphology was observed between U3O8 microspheres and ABF, resulting in (NH4)3UO2F5 ms. This product was further decomposed at 300°C, forming textured UO2F2 ms (Jang and Poineau, 2024b).
One promising direction is the development of actinide tetrafluoride micromaterials, such as AnF4 microspheres (An = Th, Np, Pu) from the fluorination of AnO2 microspheres. Thorium oxide (ThO2) and plutonium oxide (PuO2) microspheres are already used in high-temperature reactors (HTRs) (Lloyd and Haire, 1968; Ganguly and Hegde, 1997; Brandau, 2002), and both react with HFg to produce the tetrafluoride (Dawson et al., 1954; Fisher, 1958; Souček et al., 2017). Additionally, ThO2, NpO2, and PuO2 react with ABF to form ThF4, NpF4, and PuF4/PuF3, respectively (Wani et al., 1989; Silva et al., 2012; Che Zainul Bahri et al., 2019; Tosolin et al., 2021). The controlled morphologies of these actinide oxides have been well studied (Nkou Bouala et al., 2017; Clavier et al., 2019; Clavier et al., 2023; Clavier et al., 2024; Trillaud et al., 2019; Asplanato et al., 2023) as well as for cerium oxides (Wang et al., 2007; Ta et al., 2013) that could be used as surrogate material. While transplutonic oxides may exhibit similar chemistries, it remains uncertain whether their oxide precursors can be morphologically controlled.
Beyond hydrothermal precipitation, template materials have also been used to prepare uranium micromaterials with controlled morphologies. uranium (IV) microspheres (>100 μm) were prepared by sorbing U(VI) ions onto fumarated polystyrene microspheres (Elsalamouny et al., 2017).
The chemical transformation of uranium oxide nanospheres (UOx ns) represents a viable approach. Methods for preparing UOx nanospheres are already known, and reactions such as those for UO2F2 and UF4 could be successfully replicated with these materials, potentially achieving higher reactivity due to their larger surface areas. Preliminary work involving UO2 ns hydrothermally precipitated in acetone/NH4OH (Yan et al., 2019) yielded some success, producing 3UF4·H2O·HF ns. However, UO2 ns precipitated from ionic liquids (Yang et al., 2019) were too small (1–2 nm) to be properly resolved by SEM.
The development of uranium chloride micromaterials is an exciting and promising field. Uranium chloride chemistry is well-established, with several methods to prepare UCl4 and UO2Cl2 using chlorinating agents such as carbon tetrachloride, hexachloropropene, and thionyl chloride (Yoshimura et al., 1971). Additionally, in situ preparation of HCl gas (potentially via MgOHCl) (Ozcan and Dincer, 2016; Asal et al., 2024) is a promising alternative. Due to the high hygroscopicity of uranium chlorides, the retention of morphology is challenging process. Preliminary attempts using similar experimental setups, presented above, with chlorinating agents resulted in coalesced uranium or uranyl chloride materials, with some samples hydrolyzing rapidly prior to SEM analysis.
Uranium micromaterials, including metal, bromides, and sulfides, can be synthesized via chemical transformation. While uranium metal is challenging to produce at the laboratory scale through chemical transformation (Jang et al., 2022), it is a widely used precursor for the preparation of various uranium compounds (Grenthe et al., 2006). Successful transformations could open new pathways for uranium micromaterials. For example, uranium metal can be obtained by treating UO2 with lithium gas (Usami et al., 2002). Uranium disulfide (US2) and monosulfide (US) can be synthesized by reacting uranium metal (Flöter et al., 1980) or uranium tetrachloride (UCl4) (Yoshihara et al., 1967) with hydrogen sulfide (H2S) (Van Lierde and Bressers, 1966). Uranium bromides (UBrx, x = 1–5) can be produced by reacting uranium metal (Morss et al., 2011) or uranium carbide (UC) (Lau and Hildenbrand, 1987) with bromine gas.
Uranium fluoride micromaterials play a crucial role in the nuclear industry, serving as fuel, reference materials, and fission target materials. They also contribute to nuclear forensics and actinide material chemistry. While research on uranium micromaterials has historically focused on uranium microspheres—particularly oxides, nitrides, and carbides—studies on halide-based microstructures remained limited until recently.
In recent years, several studies have expanded this field by developing diverse uranium fluoride micromaterials. Chemical transformation methods using sublimed bifluoride and ammonium bifluoride have opened new pathways for synthesizing various uranium fluoride microstructures, including microspheres, microrods, and microplates. These methods have enabled the preparation of previously unreported micromaterials, such as UO2F2 and UF4 microspheres/microrods/microplates, and (NH4)3UO2F5 microspheres. Continued advancements in the chemical transformation of uranium are expected to yield novel materials with controlled morphologies, driving further progress in this field.
The raw data supporting the conclusions of this article will be made available by the authors, without undue reservation.
HJ: Conceptualization, Investigation, Methodology, Validation, Visualization, Writing–original draft. FP: Conceptualization, Funding acquisition, Project administration, Resources, Supervision, Writing–review and editing.
The author(s) declare that financial support was received for the research, authorship, and/or publication of this article. This material is based upon work performed under the auspices of the Consortium on Nuclear Security Technologies (CONNECT) supported by the Department of Energy/National Nuclear Security Administration under Award Number(s) DENA0003948.
The authors would like to thank Wendee Johns for administrative support and Quinn Summerfield for laboratory support.
The authors declare that the research was conducted in the absence of any commercial or financial relationships that could be construed as a potential conflict of interest.
The authors declare that Gen AI was used in the creation of this manuscript. AI was used for grammatical and spelling correction.
All claims expressed in this article are solely those of the authors and do not necessarily represent those of their affiliated organizations, or those of the publisher, the editors and the reviewers. Any product that may be evaluated in this article, or claim that may be made by its manufacturer, is not guaranteed or endorsed by the publisher.
Asal, S., Acır, A., and Dincer, I. (2024). A study on integrated HTR-PM driven hydrogen production using thermochemical cycles. Energy Convers. Manag. 307, 118336. doi:10.1016/j.enconman.2024.118336
Asplanato, P., Zannouh, W., Fauré, A. L., Imbert, P. H., Lautru, J., Cornaton, M., et al. (2023). Hydrothermal synthesis of homogenous and size-controlled uranium-thorium oxide micro-particles for nuclear safeguards. J. Nucl. Mater. 573, 154142. doi:10.1016/j.jnucmat.2022.154142
Beck, J. M., and Pincock, L. F. (2011). High temperature gas-cooled reactors lessons learned applicable to the next generation nuclear plant.
Brandau, E. (2002). “Microspheres of UO2, ThO2 and PuO2 for the high temperature reactor,” in HTR-2002: proceedings of the conference on high temperature reactors (International Atomic Energy Agency IAEA).
Cai, R., Chen, J., Zhu, J., Xu, C., Zhang, W., Zhang, C., et al. (2012). Synthesis of CuxS/Cu nanotubes and their lithium storage properties. J. Phys. Chem. C 116, 12468–12474. doi:10.1021/jp303120d
Carmack, W. J., Richardson, W. C., Husser, D. L., and Mohr, T. C. (2004). Internal gelation as applied to the production of uranium nitride space nuclear fuel. AIP Conf. Proc. 699, 420–425. doi:10.1063/1.1649601
Chen, Z., Cummins, D., Reinecke, B. N., Clark, E., Sunkara, M. K., and Jaramillo, T. F. (2011). Core-shell MoO3-MoS2 nanowires for hydrogen evolution: a functional design for electrocatalytic materials. Nano Lett. 11, 4168–4175. doi:10.1021/nl2020476
Che Zainul Bahri, C. N. A., Ismail, A. F., Majid, Ab., Bahri, A., Che Zainul Ismail, A. F., and Ab. Majid, A. (2019). Synthesis of thorium tetrafluoride (ThF4) by ammonium hydrogen difluoride (NH4HF2). Nucl. Eng. Technol. 51, 792–799. doi:10.1016/j.net.2018.12.023
Christian, J. H., Klug, C. A., Devore, M., Villa-Aleman, E., Foley, B. J., Groden, N., et al. (2021). Characterizing the solid hydrolysis product, UF4(H2O)2.5, generated from neat water reactions with UF4 at room temperature. Dalton Trans. 50, 2462–2471. doi:10.1039/d0dt03944f
Clavier, N., Asplanato, P., Zannouh, W., Dacheux, N., Fauré, A.-L., and Pointurier, F. (2023). Hydrothermal synthesis of (U,Th)Ox reference materials for nuclear safeguards. Sci. Basis For Nucl. Waste Manag. 47. Available online at: https://hal.science/hal-04704992.
Clavier, N., Depernet, M., Zannouh, W., Tronyo, M., Dacheux, N., Cornaton, M., et al. (2024). “Hydrothermal synthesis of AnOx reference materials for nuclear safeguards,” in 3rd international scientific exchange Meeting on particle reference materials, (richland, United States). Available online at: https://hal.science/hal-04705000.
Clavier, N., Trillaud, V., Bouala, G. I. N., Léchelle, J., Dacheux, N., and Podor, R. (2019). “In situ high temperature electron microscopy observation of sintering first stage of MO2 (M = Ce, Th, U) microspheres,” in PACRIM13, (okinawa, Japan). Available online at: https://hal.science/hal-04702340.
Croft, S., Venkataraman, R., Fugate, G., Gauld, I., McElroy, R., Moore, A., et al. (2019). “Nuclear data – benchmarking 19F,” in Yield data for nuclear safeguards (ORNL/SPR-2019/1128).
Dawson, J. K., Elliott, R. M., Hurst, R., and Truswell, A. E. (1954). The preparation and some properties of plutonium fluorides. J. Chem. Soc. (Resumed) 558, 558. doi:10.1039/jr9540000558
Dorhout, J. M., Wilkerson, M. P., and Czerwinski, K. R. (2019). Irradiation and isolation of fission products from uranium metal–organic frameworks. J. Radioanal. Nucl. Chem. 320, 415–424. doi:10.1007/s10967-019-06478-w
Elsalamouny, A. R., Desouky, O. A., Mohamed, S. A., Galhoum, A. A., and Guibal, E. (2017). Evaluation of adsorption behavior for U(VI) and Nd(III) ions onto fumarated polystyrene microspheres. J. Radioanal. Nucl. Chem. 314, 429–437. doi:10.1007/s10967-017-5389-5
Fayette, M., and Robinson, R. D. (2014). Chemical transformations of nanomaterials for energy applications. J. Mater Chem. A Mater 2, 5965–5978. doi:10.1039/c3ta13982d
Fisher, R. W. (1958). The preparation of thorium oxide and thorium fluoride from thorium nitrate. Prog. Nucl. Energy, Ser. III, Process Chem. 2, 149.
Flöter, W., Hellberg, K.-H., Plöger, F., Schneider, H., Stehle, H., Taumann, L., et al. (1980). in U uran (Berlin, Heidelberg: Springer Berlin Heidelberg). doi:10.1007/978-3-662-10275-6
Fluoride Salt-Cooled Reactor (2023). Ultra safe nuclear corporation. Available online at: https://www.usnc.com/fluoride-salt-cooled-reactor/(Accessed January 28, 2025).
Foley, B. J., Christian, J. H., Klug, C. A., Villa-Aleman, E., Wellons, M. S., DeVore, M., et al. (2022). Probing the hydrolytic degradation of UF4 in humid air. Dalton Trans. 51, 6061–6067. doi:10.1039/d2dt00196a
Frolova, L. (2021). “Studying of iron oxyhydroxide dehydration,” in Springer proceedings in physics (Springer International Publishing), 165–169. doi:10.1007/978-3-030-74800-5_11
Ganguly, C., and Hegde, P. V. (1997). Sol-gel microsphere pelletisation process for fabrication of (U,Pu)O2, (U,Pu)C and (U,Pu)N fuel pellets for the prototype fast breeder reactor in India. J. Solgel Sci. Technol. 9, 285–294. doi:10.1007/BF02437192
Grenthe, I., Drożdżynński, J., Fujino, T., Buck, E. C., Albrecht-Schmitt, T. E., and Wolf, S. F. (2006). “Uranium,” in The chemistry of the actinide and transactinide elements (Netherlands: Springer), 253–698.
Guo, S., Zhang, S., Wu, L., and Sun, S. (2012). Co/CoO nanoparticles assembled on graphene for electrochemical reduction of oxygen. Angew. Chem. - Int. Ed. 51, 11770–11773. doi:10.1002/anie.201206152
Henry, R., and Saclay, H. (1959). The use of recoil for the separation of uranium fission products; Utilisation du recul pour la separation des produits de fission de l’uranium. France.
Jang, H., Louis-Jean, J., Childs, B., Holliday, K., Reilly, D., Athon, M., et al. (2022). Synthetic diversity in the preparation of metallic uranium. R. Soc. Open Sci. 9, 211870. doi:10.1098/rsos.211870
Jang, H., Louis-Jean, J., and Poineau, F. (2023). Synthesis and morphological control of UO2F2 particulates. ACS Omega 8, 21996–22002. doi:10.1021/acsomega.3c01999
Jang, H., and Poineau, F. (2024a). Revealing uranium tetrafluoride microrods. Mater Adv. 1, 8233–8237. doi:10.1039/D4MA00796D
Jang, H., and Poineau, F. (2024b). Tailoring triuranium octoxide into multidimensional uranyl fluoride micromaterials. ACS Omega 9, 26380–26387. doi:10.1021/acsomega.4c02554
Kegler, P., Pointurier, F., Rothe, J., Dardenne, K., Vitova, T., Beck, A., et al. (2021). Chemical and structural investigations on uranium oxide-based microparticles as reference materials for analytical measurements. MRS Adv. 6, 125–130. doi:10.1557/s43580-021-00024-1
Kim, C., Kim, Y. J., Jang, E. S., Yi, G. C., and Kim, H. H. (2006). Whispering-gallery-modelike-enhanced emission from ZnO nanodisk. Appl. Phys. Lett. 88, 1–3. doi:10.1063/1.2174122
Lau, K. H., and Hildenbrand, D. L. (1987). Thermochemistry of the gaseous uranium bromides UBr through UBr5. J. Chem. Phys. 86, 2949–2954. doi:10.1063/1.452046
Lee, J. A., Newnham, C. E., Stone, F. S., and Tye, F. L. (1980). Thermal decomposition of managanese oxyhydroxide. J. Solid State Chem. 31, 81–93. doi:10.1016/0022-4596(80)90010-9
Lloyd, M. H., and Haire, R. G. (1968). A sol-gel process for preparing dense forms of PuO2. Nucl. Appl. 5, 114–122. doi:10.13182/nt68-a28040
Moon, G. D., Ko, S., Min, Y., Zeng, J., Xia, Y., and Jeong, U. (2011). Chemical transformations of nanostructured materials. Nano Today 6, 186–203. doi:10.1016/j.nantod.2011.02.006
L. R. Morss, N. M. Edelstein, and J. Fuger (2011). The chemistry of the actinide and transactinide elements (Dordrecht: Springer Netherlands). doi:10.1007/978-94-007-0211-0
Nkou Bouala, G. I., Clavier, N., Léchelle, J., Monnier, J., Ricolleau, Ch., Dacheux, N., et al. (2017). High-temperature electron microscopy study of ThO2 microspheres sintering. J. Eur. Ceram. Soc. 37, 727–738. doi:10.1016/j.jeurceramsoc.2016.08.029
Ozcan, H., and Dincer, I. (2016). Thermodynamic modeling of a nuclear energy based integrated system for hydrogen production and liquefaction. Comput. Chem. Eng. 90, 234–246. doi:10.1016/j.compchemeng.2016.04.015
Passy, U., and Steiger, N. H. (1963). Some nuclear-chemical and physicochemical problems in fission-product recoil separation. Nucl. Sci. Eng. 15, 366–374. doi:10.13182/NSE63-A26452
Plaue, J. (2013). Forensic signatures of chemical process history in uranium oxides. Las Vegas: University of Nevada. doi:10.34917/4478292
Pradhan, M., Sarkar, S., Sinha, A. K., Basu, M., and Pal, T. (2011). Morphology controlled uranium oxide hydroxide hydrate for catalysis, luminescence and SERS studies. CrystEngComm 13, 2878–2889. doi:10.1039/c0ce00666a
Qualls, A. L., Betzler, B. R., Brown, N. R., Carbajo, J. J., Greenwood, M. S., Hale, R., et al. (2017). Preconceptual design of a fluoride high temperature salt-cooled engineering demonstration reactor: motivation and overview. Ann. Nucl. Energy 107, 144–155. doi:10.1016/j.anucene.2016.11.021
Richter, S., Truyens, J., Venchiarutti, C., Aregbe, Y., Middendorp, R., Neumeier, S., et al. (2022). Certification of the first uranium oxide micro-particle reference materials for nuclear safety and security, IRMM-2329P and IRMM-2331P. J. Radioanal. Nucl. Chem. 332, 2809–2813. doi:10.1007/s10967-022-08255-8
Serp, J., Allibert, M., Beneš, O., Delpech, S., Feynberg, O., Ghetta, V., et al. (2014). The molten salt reactor (MSR) in generation IV: overview and perspectives. Prog. Nucl. Energy 77, 308–319. doi:10.1016/j.pnucene.2014.02.014
Silva, G. W. C., Weck, P. F., Kim, E., Yeamans, C. B., Cerefice, G. S., Sattelberger, A. P., et al. (2012). Crystal and electronic structures of neptunium nitrides synthesized using a fluoride route. J. Am. Chem. Soc. 134, 3111–3119. doi:10.1021/ja209503n
Souček, P., Beneš, O., Claux, B., Capelli, E., Ougier, M., Tyrpekl, V., et al. (2017). Synthesis of UF4 and ThF4 by HF gas fluorination and re-determination of the UF4 melting point. J. Fluor Chem. 200, 33–40. doi:10.1016/j.jfluchem.2017.05.011
Ta, N., Liu, J., and Shen, W. (2013). Tuning the shape of ceria nanomaterials for catalytic applications. Cuihua Xuebao/Chinese J. Catal. 34, 838–850. doi:10.1016/s1872-2067(12)60573-7
Tosolin, A., Leeder, K., and Woods, D. (2021). Plan for synthesis of actinide chlorides and fluorides without using gaseous agents.
Trillaud, V., Bouala, G. N., Clavier, N., Dacheux, N., Ricolleau, C., and Podor, R. (2019). First stage of sintering of ThO2 microspheres: a HT-ESEM and HT-HRTEM study. Microsc. Microanal. 25, 49–50. doi:10.1017/S1431927618015970
Ueta, S., Aihara, J., Sawa, K., Yasuda, A., Honda, M., and Furihata, N. (2011). Development of high temperature gas-cooled reactor (HTGR) fuel in Japan. Prog. Nucl. Energy 53, 788–793. doi:10.1016/j.pnucene.2011.05.005
Usami, T., Kurata, M., Inoue, T., Sims, H. E., Beetham, S. A., and Jenkins, J. A. (2002). Pyrochemical reduction of uranium dioxide and plutonium dioxide by lithium metal. J. Nucl. Mater. 300, 15–26. doi:10.1016/S0022-3115(01)00703-6
Van Lierde, W., and Bressers, J. (1966). Preparation of uranium sulfide single crystals. J. Appl. Phys. 37, 444. doi:10.1063/1.1707867
Villa-Aleman, E., and Wellons, M. S. (2016). Characterization of uranium tetrafluoride (UF4) with Raman spectroscopy. J. Raman Spectrosc. 47, 865–870. doi:10.1002/jrs.4909
Wang, S., Gu, F., Li, C., and Cao, H. (2007). Shape-controlled synthesis of CeOHCO3 and CeO2 microstructures. J. Cryst. Growth 307, 386–394. doi:10.1016/j.jcrysgro.2007.06.025
Wani, B. N., Patwe, S. J., Rao, U. R. K., and Venkateswarlu, K. S. (1989). Fluorination of oxides of uranium and thorium by ammonium hydrogenfluoride. J. Fluor Chem. 44, 177–185. doi:10.1016/S0022-1139(00)83937-8
Xu, C. X., Sun, X. W., Dong, Z. L., Cui, Y. P., and Wang, B. P. (2007). Nanostructured single-crystalline twin disks of zinc oxide. Cryst. Growth Des. 7, 541–544. doi:10.1021/cg060642j
Yan, Q., Mao, Y., Zhou, X., Liang, J., Peng, S., and Ye, M. (2019). Control of the compositions and morphologies of uranium oxide nanocrystals in the solution phase: Multi-monomer growth and self-catalysis. Nanoscale Adv. 1, 1314–1318. doi:10.1039/c8na00392k
Yang, Z., Liu, Y., Liu, Y., Wang, Y., Rao, H., Liu, Y., et al. (2019). Preparation of porous uranium oxide hollow nanospheres with peroxidase mimicking activity: application to the colorimetric determination of tin(II). Microchim. Acta 186, 501–508. doi:10.1007/s00604-019-3624-1
Yoshihara, K., Kanno, M., and Mukaibo, T. (1967). Preparation of uranium disulfide from uranium chloride. J. Nucl. Sci. Technol. 4, 578–581. doi:10.1080/18811248.1967.9732809
Yoshimura, T., Miyake, C., and Imoto, S. (1971). Preparation of anhydrous uranium tetrachloride and measurements on its magnetic susceptibility. J. Nucl. Sci. Technol. 8, 498–502. doi:10.1080/18811248.1971.9732985
Keywords: uranium, fluoride, micromaterials, morphology, chemical transformation
Citation: Jang H and Poineau F (2025) Uranium fluoride micromaterials: a new frontier in nuclear engineering. Front. Nucl. Eng. 4:1569103. doi: 10.3389/fnuen.2025.1569103
Received: 31 January 2025; Accepted: 17 March 2025;
Published: 25 March 2025.
Edited by:
Xing Wang, The Pennsylvania State University (PSU), United StatesReviewed by:
Jacques Lechelle, Commissariat à l'Energie Atomique et aux Energies Alternatives (CEA), FranceCopyright © 2025 Jang and Poineau. This is an open-access article distributed under the terms of the Creative Commons Attribution License (CC BY). The use, distribution or reproduction in other forums is permitted, provided the original author(s) and the copyright owner(s) are credited and that the original publication in this journal is cited, in accordance with accepted academic practice. No use, distribution or reproduction is permitted which does not comply with these terms.
*Correspondence: Frederic Poineau, cG9pbmVhdWZAdW5sdi5uZXZhZGEuZWR1
Disclaimer: All claims expressed in this article are solely those of the authors and do not necessarily represent those of their affiliated organizations, or those of the publisher, the editors and the reviewers. Any product that may be evaluated in this article or claim that may be made by its manufacturer is not guaranteed or endorsed by the publisher.
Research integrity at Frontiers
Learn more about the work of our research integrity team to safeguard the quality of each article we publish.