- Massachusetts Institute of Technology, Cambridge, MA, United States
In fusion, the validation of turbulent transport models is undertaken with the goals of making basic physics discoveries as well as for development of new predictive models to improve the operation and enhance the performance of existing and future fusion reactors. A fusion industry is just beginning to emerge globally. Like fission, validation in fusion energy research is a vibrant research area, but unlike fusion, a fission industry exists. The fission power industry motivates validation efforts, often performed at universities with small-scale experiments and advanced models and simulations developed in-house. Because fission research spans basic physics and applications, and addresses near-term and long-term industry interests, validation is thriving. This perspective article describes the validation of turbulent transport models in both fusion research and fission research, draws parallels between the validation methods and techniques used in two areas of the fields, and presents an outlook for thriving university fusion and fission research programs underpinned by a virtual cycle of basic and applied research that supports industry needs as well as tackling intellectual grand challenges.
1 Introduction
The field of fusion and plasma physics is changing rapidly, with a new global fusion energy ecosystem set to emerge over the next decades. In the United States, recent federal advisory board reports and community activities have built consensus for a new energy mission within the US Department of Energy (DOE) Office of Fusion Energy Sciences. The report Bringing Fusion to the U.S. Grid (2021) from the US National Academies of Science, Engineering and Medicine (NASEM) noted three key recommendations; that, DOE and the private sector should demonstrate net electricity in a fusion pilot plant in the 2035–2040 timeframe; DOE should move forward now via public-private partnerships to develop and bring fusion to commercial viability; and Urgent investments by DOE and private industry are needed to resolve the remaining science and technology issues to realize a fusion pilot plant (Nasem 2021). As of early 2024, there were over 35 private fusion companies who were members of the Fusion Industry Association, which is a US-based non-profit organization composed of private companies and active in advocacy and education.
The path to fusion commercialization accelerated further, when the White House put forth a “Bold Decadal” vision for fusion in Spring 2022, announcing a new $55 Million Milestone Program for funding promising fusion pilot plant concepts. The funding opportunity called for private companies who were pursuing fusion energy systems to submit proposals for one of two tiers for funding. Tier one activities support moving a Fusion Pilot Plant to a stage called a “Preliminary Design Review”. This means that an awarded Tier one company would deliver a report to the DoE that documents the design of a fusion pilot plant just 5 years after the start of the award, and that the design is mature enough such that the next two steps on the path to a pilot plant are final design and construction. In May 2023, the DoE announced eight private companies who had been awarded funding under the milestone program; with the Tier one companies aiming toward fusion pilot plants that could operate by the early 2030s. Fusion is most certainly closer to commercialization that at any point in its over 60-year history.
As academic researchers, we are often asked by colleagues what does the future hold for plasma physics and fusion research at universities once fusion is on the grid? Given the science and technology gaps identified in the NASEM report (Nasem 2021), we anticipate growth in the research and development on systems and subsystems of a fusion power plant. This will include materials that maintain performance in the extreme environment of fusion energy systems; as well as in fusion technology, such as subsystems that convert fusion neutron energy to electricity, or on superconducting magnets and cost-efficient, high-power lasers that underpin magnetic and inertial fusion confinement concepts, respectively. This will certainly bring more scientists and engineers from non-traditional science engineering academic backgrounds into the field of fusion and into fusion faculty positions at universities, as has been noted by other authors (Whyte et al., 2023).
While fusion materials and technology have always been part of research on fusion energy, most students who have pursued fusion over the years and the majority of faculty in the field have focused on plasma physics as their core traditional discipline. This can be seen by examining the fusion graduate curricula at many top universities. The emergence of energy producing fusion systems on the grid raises questions about the future of the role for plasma physics in fusion research at universities. Our answer to this question is that over time there will be an expansion of the breadth of academic fields connected to fusion, while also a deepening of fundamental plasma physics research.
In the authors’ areas of research, three of us are focused on the science of fusion energy systems, through the study of plasma turbulence and the resulting transport in magnetically confined fusion plasmas. Two of us are focused on the science of fission energy systems, through the study of boiling heat transfer and turbulence in neutral fluids. This perspective article will provide an overview of turbulence and turbulent-transport model validation research in tokamaks and examples of validation research on boiling heat transfer and turbulence in neutral fluids with applications to fission power systems. We also comment on the future roles of mid-scale fusion energy research facilities for validation by drawing parallels with the fission field.
2 Validation of turbulence transport models for fusion energy
Nuclear Fusion is a physical process that combines light nuclei to make heavier nuclei. It is the process that powers every star. Fusion energy research is a branch of science and engineering dedicated to harnessing fusion reactions for the benefit of humankind. The goal of fusion energy research is to build a fusion power station to make clean electricity (or heat) from energy released in fusion reactions. Fusion energy has many advantages, including an abundant, high energy density fuel that can provide energy with no greenhouse gases, and features manageable residual radioactive waste (Freidberg 2007) and minimal proliferation risks (Glaser and Goldston, 2012).
Developing a net-energy fusion system is very challenging because of the physics and engineering involved in confining a very hot 100-million-degree Celsius plasma in steady state with high fusion performance. For the most widely studied fusion fuel, a plasma of deuterium (D) and tritium (T), high-performance would mean a plasma with large amounts of self-heating from the alpha (α) particles that are on product of the D-T fusion reaction.
Before becoming ignited, the plasma will first enter a burning plasma state, where the self-heating from alpha-particles will exceed the external heating of the fuel. Once the self-heating overcomes the energy losses, due to radiation, for example, the plasma becomes self-sustaining and considered to be ‘ignited’ (Lawson 1957). We note there are important differences in definitions when quantifying ignition for different fusion energy confinement systems, and there are different definitions of ignition even for the same system in some cases (Wurzel 2022). For example, when the National Ignition Facility was undertaking their most recent efforts to achieve net energy, the facility first conducted experiments that conclusively reached a burning plasma state (Zylstra et al., 2022) before achieving ignition (and proved they had achieved ignition according to several definitions) (Abu-Shawareb, 2022). A few years later at the National Ignition Facility (NIF), net energy gain in the target was achieved. In the experiment described in Abu-Shawareb (2022) the target gain was 0.72, or 1.37 MJ of fusion for 1.92 MJ of laser energy. On 5 December 2022, the NIF achieved 3.15 MJ (MJ) of fusion energy output from 2.05 MJ of laser energy delivered to the target, reaching target gain above unity, and further fusion gain records were set during the year 2023 (NIF 2023).
Decades prior, the JET tokamak set plasma gain records for magnetically confined fusion, achieving a record plasma gain of Q = 0.62. It is important to emphasize that the net fusion energy gain (or target gain) in the plasma fuel, Q, is different from net energy gain for the system, often described as “Q-Engineering”, QENG. Values of QENG >1 are needed to produce electricity from the fusion system. Two simple definitions that differentiate between the two are Q = (net thermal power out)/(heating power in) and QENG = (net electric power out)/(electric power in) (Freidberg 2007). There are engineering and technological challenges to achieving QENG >1, such as efficiently converting the neutrons produced from D-T reactions within a Q > 1 plasma into useable electric power and recovering and recycling the tritium for further use in the fuel.
The success of JET and other magnetic confinement devices has led to the tokamak being one of the most widely studied and most developed fusion energy systems studied. A D-T tokamak is also considered to be closest to a net electricity system, given the combination of fusion parameter performance (high density and high temperature) and pulse duration relative to that needed for a commercial power plant (NASEM 2021). The fusion parameter performance target can be summarized as achieving the so-called Lawson criterion. This is often described as achieving a high triple product of density, temperature, and energy confinement time, or nTτE = 8.3 atm-s at a temperature of T = 15 keV (note that the product of density and energy confinement time nτE is known as the Lawson parameter).
The fundamental physics concept behind magnetic confinement relies on motion of charged particles in a magnetic field. Charged particles are “confined” perpendicular to B field lines. The trajectory is a helix with characteristic radius (gyroradius)
At a magnetic field of 5T and plasma temperature of 10 keV, the electron gyroradius is ρe ∼ 0.06 mm and ion gyroradius is ρi ∼ 3 mm. Fuel ions are moving ∼1,000 km/sec. The characteristic scale length along the field line is millions of times the gyroradius, since parallel transport is determined largely by particle coulomb collisions and the parallel mean free path several times larger than the system size. This means there is no confinement parallel to B as there is perpendicular to B. Closing the magnetic field lines or avoiding end losses is key in any magnetic confinement system. Tokamaks do this by using closed field lines and nested toroidal flux surfaces, and to date, tokamak experiments have featured some of the highest performing fusion plasmas (Wurzel 2022).
Many tokamak experiments have demonstrated high density and temperature operation but achieving high pressures simultaneously with high energy confinement time, τE, is challenging. This is because turbulence in the plasma very efficiently mixes the hot and cold plasma, limiting high pressures in the core plasma. Turbulent transport therefore limits the confinement time and therefore limits achievement of net-energy in tokamaks (and other magnetic confinement systems as well). For some time, this was not fully understood. Originally, when the observed transport levels in tokamaks exceeded what would be expected from collisional transport theory, it was called “anomalous” transport in the literature. As experiments and modeling evolved, the high transport levels were determined to be caused by turbulence. The turbulence is unavoidable, because it is driven by pressure gradients always present in the plasma, which are sources of free energy. These gradients give rise to “universal” drift-wave instabilities, which nonlinearly evolve into saturated turbulence (Horton 1999). The turbulence drives large amounts of heat and particles from the hot core to the colder edge. The turbulence is often referred to as micro-turbulence, due to the small length scale of the turbulent structures or “eddies” in the direction perpendicular to the confining magnetic field compared to the size of plasma, which is a ≈ 1 m. In contrast, the size of the turbulent structures will scale with the gyro-radii, ρe ≈ 0.06 mm, ρi ≈ 3.6 mm at 10 keV and 5.4 T, as shown in Figure 1. The broadband turbulence has frequencies ranging from 10 kHz to over 1 MHz and fluctuation amplitudes, of 0.1%–10% of equilibrium values of plasma pressure or magnetic field.
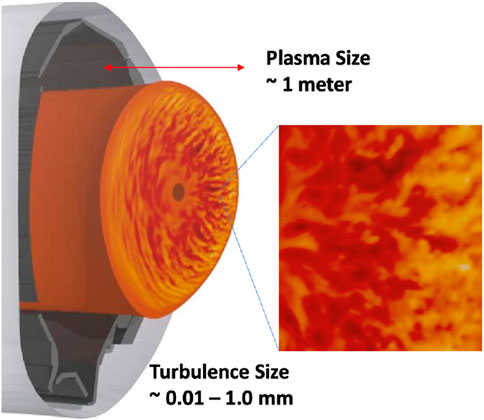
Figure 1. The output of a gyrokinetic simulation of micro-turbulence in a tokamak performed with the code GENE (GENE 2023) is adapted to illustrate the small length scale of the turbulent structures or “eddies” compared to the size of the plasma.
Over the past decades a standard model describing the turbulence and transport in tokamaks and other plasmas of interest for magnetic confinement fusion has merged. The turbulence in fusion plasmas is well-described by nonlinear gyrokinetics (Brizard and Hahm, 2007) and there is a large area of research in the field focused on carefully comparing experimental measurements of turbulence to simulation by a process known as validation (White 2019).
Validating gyrokinetic codes using experimental data from operating tokamaks is key for being able to use these codes to then make reliable predictions for future performance in tokamaks yet to be built and operated, such as ITER (Howard et al., 2021) and SPARC (Fernandez et al., 2020). Simply speaking, validation of a turbulent transport model proceeds as follows, nonlinear gyrokinetic codes take experimental profile data as input, and the input files must be carefully prepared. The code outputs are the heat fluxes and turbulence characteristics. Typically, only the latter are directly measured. Modern comparisons between experiment and simulation then involve sensitivity scans, error analysis, uncertainty quantification and the use of metrics. Over the years, the comparisons have led to better and better models that the codes can run. Validation has revealed importance of including realistic plasma geometry, kinetic electrons, collisions, the flow shear and turbulence-generated zonal flows, electromagnetic effects, and multi-scale effects caused by coupling between electron and ion scale turbulence.
Turbulence diagnostics have played key role in validation studies (Rhodes et al., 2011; White 2019). And of course, gyrokinetic theory itself is continuously being developed, such as recent work on the details of fast ion–turbulence interactions (Citrin and Mantica, 2023) or the effects of a non-axisymmetric 3-D equilibrium magnetic field (Wilcox et al., 2017). Numerical approaches are also evolving, for example, new gyrokinetic codes are being developed specifically to perform electromagnetic simulations of a helical model for the scrape-off-layer plasma (Hakim et al., 2020), revealing intermittent blob-like structures ejected from a source region, see Figure 2. While reminiscent of observations of the structure and motion of edge turbulence and “blobs” in experiments (Zweben et al., 2022), direct comparisons in this edge region remain as exciting future work.
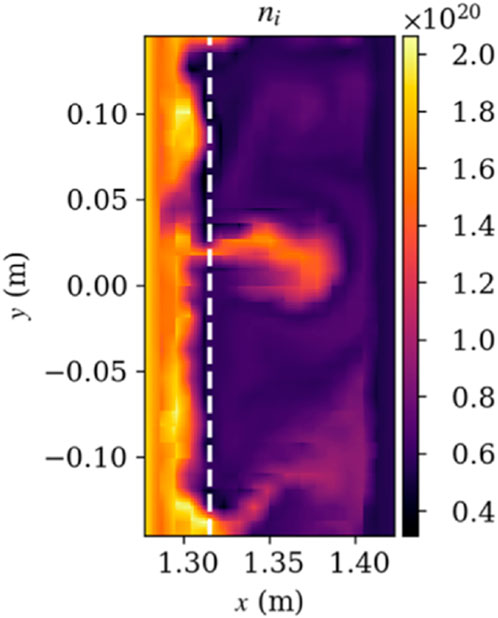
Figure 2. Output of an electromagnetic simulation of a helical model for the scrape-off-layer plasma in a tokamak, performed with the GEYKLL code revealing an intermittent structure with features that qualitatively resemble experimentally observed “blobs” being ejected from the source region in the simulation (left side of vertical dashed line) (Hakim et al., 2020).
One goal of validation in fusion is to develop reliable models that can be used to predict performance in future devices. In one example, a reduced model that is routinely used to predict profiles was improved based on multiscale turbulence studies that combined simulation with comparisons to experiments. A model called TGLF is a reduced model for the core plasma turbulent-driven transport (Staebler et al., 2007) originally developed by scientists at General Atomics. TGLF was derived via comparisons with higher fidelity gyrokinetic simulations, which had been validated against experiment. Ten years after the original TGFL model was created, a new “TGLF-SAT1” turbulent transport model was introduced (Staebler et al., 2017). This new SAT1 model included the effects of cross-scale coupling between ion-scale and electron turbulence, which were not included in the original TGLF models. This SAT1 model was developed using the nonlinear gyrokinetic simulations of multi-scale turbulence that were used for validation studies performed at the MIT tokamak, Alcator C-Mod by the MIT group (Howard et al., 2016).
Recent work used this newly developed TGLF SAT1 model to make predictions for the SPARC tokamak. This work shows important differences in physics-based model predictions for Q, the plasma gain metric, and predictions made with empirical scaling laws (Fernandez 2020). Using empirical scaling laws with conservative assumptions (H98, y2 = 1.0 and density profile peaking factors as empirically predicted) for the reference discharge in SPARC leads to a predicted Q ≈ 11. The profiles predicted for this case are shown in Figure 3. Using a new reduced model for the transport caused by the turbulence within a code called TGLF, the modeling work indicated that the current version of the SPARC design with nominal parameters will generate Pfus ≈100 MW of fusion power, with a gain of Q ≈ 9. As mentioned above, this new reduced model used in the work was itself developed from high fidelity multi-scale simulations that were validated against experiments performed at the MIT tokamak, Alcator C-Mod (Howard et al., 2014; Howard, 2016). Choosing what boundary conditions to use in the modeling also relied on another reduced model, called EPED, that has been extensively validated against experiments, including new extension to high densities relevant for SPARC using comparisons to experiments at the MIT Alcator C-Mod tokamak (Hughes et al., 2018). More recently, integrated modeling and predictive simulations indicates the tremendous value of using experimentally validated physics-based models for predicting performance of future fusion energy systems, including the use of surrogate models developed from high-fidelity codes (Fernandez et al., 2022). This is important, since the use of empirical scaling laws to predict performance in future devices can introduce significant uncertainties if used alone.
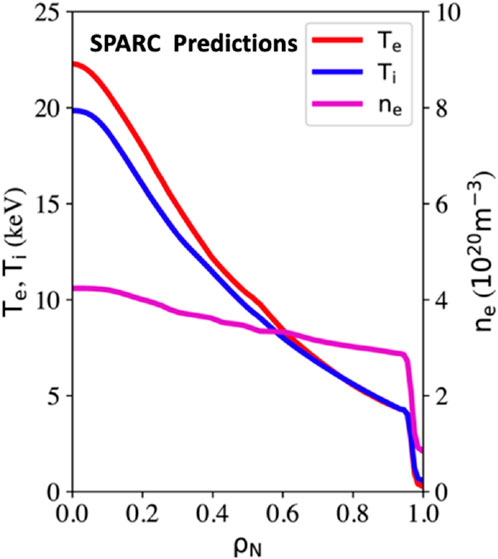
Figure 3. Predictions from a turbulent transport model for the temperature and density profile expected in the tokamak SPARC (Fernandez et al., 2020).
Another goal of validation in fusion is to make new, fundamental physics discoveries. For example, theory suggested a transition to subcritical turbulence occurs via an intermediate state dominated by low number of coherent long-lived structures, close to threshold. Then, more conventional turbulence emerges after structures increase in number to fill domain as system moves away from threshold, as shown in Figure 4. There was a theory prediction that properties of turbulence are functions of the distance to threshold, and this could be quantified by the ion heat flux. Validation work helped corroborate this new subcritical turbulence physics result, as described in (Wyk, 2017).
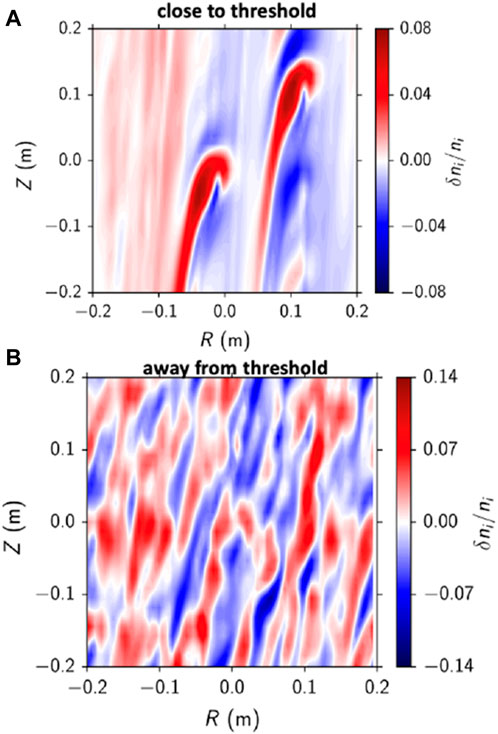
Figure 4. A gyrokinetic simulation output showing a low number of coherent long-lived structures (A) that evolve into more conventional turbulence (B) after the number of structures increases to fill the domain as system moves away from threshold. From Wyk (2017).
3 University-based validation research in fission
Just as with fusion, validation of turbulent transport models in fission energy systems is undertaken with the goals of making basic physics discoveries as well as for development of new predictive models for operation and performance of future fission reactors.
Fission energy systems are of course dramatically different from fusion energy systems. Unlike a fusion energy system, where the fuel is a hot plasma, the fuel for operating fission power plants is a solid. Conventional fission fuel consists of uranium pellets within a fuel rod that has an outer layer of cladding, which is zirconium-based. Coolant water is used to manage the temperature of fuel rods and other parts of light water reactor (LWR) fission energy system. The study of heat transfer in the coolant water is very important for industry to support current fleet. Other coolants for advanced reactor are important to future expansion of fission energy to meet zero-carbon goals.
Boiling water is one of the most effective heat transfer mechanisms at commercial scale. Prof. Varanasi from MIT, a world-leader in field of heat transfer and surface engineering has explained, “Roughly 85 percent of the worldwide installed base of electricity relies on steam power generators, and in the U.S. it’s 90 percent,” Varanasi says. “If you’re able to improve the boiling process that produces this steam, you can improve the overall power plant efficiency.” (MIT News 2015). This underlines the importance of specific academic research (boiling fluid dynamics) to improve an ongoing, mature industry.
If nucleate boiling provides an excellent mechanism for heat removal from a heated surface, then a departure from nucleate boiling can be disastrous for the system to be cooled down (Zhang et al., 2023). When a surface is cooled by a liquid, at high heat transfer rates, the surface temperature may exceed the fluid boiling point. The liquid in contact with the surface vaporizes. Vapor bubbles nucleate and grow on top of the surface and move away. Both the process of bubble nucleation and the convective flows created by the growth and detachment of bubbles remove energy from the surface. However, at higher heat transfer rates, more and more area will be covered with bubbles. At the so-called critical heat flux (CHF), a “boiling crisis” may occur. When that happens, the surface gets suddenly covered by a stable expanding vapor patch (instead of small detaching bubbles). This instability, called departure from nucleate boiling, is very detrimental. Vapor has poor thermal conductivity. Thus, the surface is not effectively cooled down, and its temperature may even exceed its melting point.
To avoid exceeding the critical heat flux (CHF) limit, power plants are operated at a thermal power lower than they otherwise could, which limits their electric power output and cost-competitiveness.
A major validation effort in fission was led by the DOE Consortium for Advanced Simulation of Light Water Reactors (CASL), which ended in 2020 (CASL 2020). CASL was a decade-long DOE funded program for research and development, technology deployment, education, and workforce development. It was organized around several “Challenge Problems” for LWRs, across a variety of physical phenomena and processes. The CASL THM (Thermal-Hydraulics Methods) group focused on the development of predictive models for Departure from Nucleate Boiling (DNB) and Flow Regimes. To tackle this problem, the researchers realized that Multilevel Validation was needed, but there was a paucity of measurements.
Nuclear fission reactors have been historically designed based on empirical and semi-empirical correlation obtained by low-resolution diagnostics, e.g., thermocouples. These types of measurements can elucidate the physics of the phenomena only superficially. For instance, thermocouples measurements allow detecting a boiling crisis by measuring a spike in the average boiling surface temperature, but do not allow to understand the mechanisms that trigger such boiling crisis. Thus, the design of two-phase system often requires ad hoc experiments run in prototypical conditions. However, it is practically impossible to run prototypical experiment for any geometry and operating and boundary conditions. Non-prototypical data (and the attached correlations) can only be used with large margin of uncertainties, which ultimately hinder fission reactors operation and profitability.
Unlike in fusion where a large suite of turbulence diagnostics has been developed over the past 20 years, turbulence diagnostics to study water boiling systems that operate at high temperature and high pressure had not been developed until more recently. Major advances in this field have been possible thanks to the development of infrared thermometry and phase detection techniques, mostly in the last decade. These techniques rely on special heaters, consisting of a substrate, ideally transparent to both infrared and visible light, coated with a visible light transparent, but IR opaque, electrically conductive coating. This electrically conductive coating is in contact with water and releases by Joule effect the heat necessary to boil the fluid. Its thermal capacity and thermal resistance are negligible. Thus, its temperature practically coincides with the temperature at the interface between the solid and the fluid. The infrared radiation emitted by this coating can be used to measure the time-dependent temperature distribution on the boiling surface.
However, this process is complicated by the fact that the substrate is never perfectly transparent, and it tends to act as black body, it will partially absorb the radiation emitted by the ITO and re-emit radiation at a slightly different temperature (and frequency). The imperfect transparency could be used to quantify the radiation transport through the imaging surface. By solving for the various components of the IR transmission, reflections, absorption and remission, a team at MIT came up with a full radiation transport model that he could use to predict what the signal would look like. Solving this inverse problem allowed for extraction the time-dependent temperature and the heat flux distributions absolutely from the high-speed IR camera images, like the one shown in Figure 5. This new diagnostic development has unlocked the measurement of boiling parameters, such as nucleation site density, bubble growth and wait time, bubble departure diameter, and bubble size distributions, and, importantly, a parameter known as the Heat Flux Partitioning (Richenderfer et al., 2018), which was a key missing piece for the validation of two-phase modeling tools for fission energy systems (Baglietto et al., 2019).
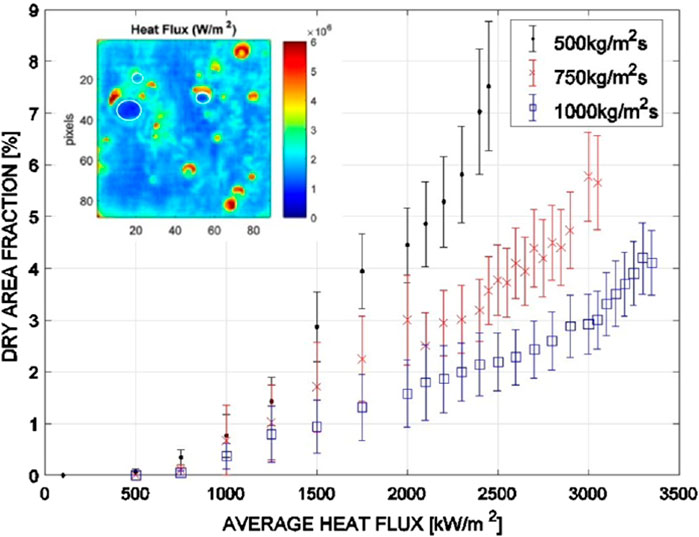
Figure 5. IR camera image data of nucleate boiling is used to calculate the dry area fraction as a function of applied heat flux. The inset image shows the heat flux distribution from a single IR camera frame, with darkest blue colors outlined in white indicate the dry regions (Richenderfer et al., 2018).
Accessing direct measurements of the heat flux partitioning is akin to measuring turbulence directly in the tokamak case. Predictions of these parameters from a simulation are shown in Figure 6. Heat flux partitioning describes the importance of heat transfer mechanisms during bubble life cycle, including evaporation, and different modes of convection, conduction. Heat flux partitioning models use nucleation site density, bubble growth, and wait time, and bubble departure diameter as input. Given a surface temperature, the models are used to predict the total heat flux removed from the surface by predicting the heat flux removed by each of the removal mechanisms.
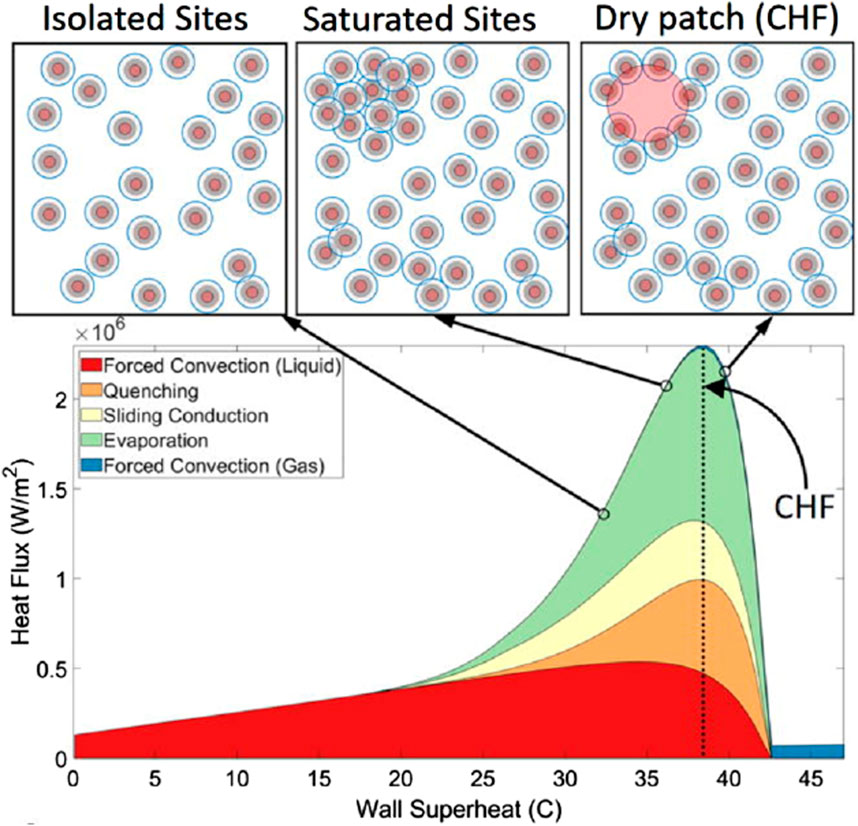
Figure 6. Adapted from Baglietto et al. (2019): “Illustration of CHF predictions: as the wall superheat increases, the bubbles start saturating the boiling surface, driving interactions and accelerated growth of the dry area with a sudden decrease in the total heat flux, which is the signature of CHF. The images on the top illustrate the local dry patch formation as CHF is reached.”
It is also worth noting that fission is arguably much further ahead of fusion in the approach to multimachine validation. There is a predictive simulation tools developed by CASL used to simulate many different reactors in the US fleet called Virtual Environment for Reactor Applications (VERA). VERA is being deployed as an integrated, high performance computing platform for performing multi-physics simulation for advanced Light Water Reactors ref [CASL 2020)]. As of 2020, CASL had simulated 170 operating fuel cycles across 28 reactors representing the full spectrum of designs within the US nuclear fission fleet.
4 Roles of small and medium-scale facilities and research reactors in fusion and fission
In a fusion power plant, like a fission power plant, there will be very limited diagnostics, so the development of better models to optimize reactors will come from small-scale and medium-scale experimental facilities at universities and labs. Research reactors, which can be sited both at universities and national labs, will also have an important role to play. Deciding what kinds of facilities are needed is driven both by fundamental physics questions as well as industry needs.
Looking at fission first, the industry needs include efforts to develop more efficient, cheaper, safer reactors. This drives academic research in small-scale platforms for the study of materials and radiation effects, as well as thermal-hydraulics and fluid-dynamics for fission applications.
Specialized small-scale facilities can address and overcome measurement challenges in detail.
Consider the new experimental platform being designed and built by Bucci and team at MIT. This first of a kind small scale facility will be capable of matching fission reactor conditions (350°C, 150 bar) to study boiling without needing a fission reactor. Several important diagnostics are deployed on this platform, such as an innovative Phase Detection technique to track phase (liquid or vapor) in contact with boiling surface using inexpensive color LEDs instead of more expensive lasers. For example, in a recent study, the CHF for a nanoengineered surface was compared at 1 bar and 4 bar conditions (Wang et al., 2024).
Fission research reactors may be considered medium and large-scale devices. There are 25 research reactors sited at universities in the US. Including a high-performance 6 MW thermal fission research reactor (the MITR) at MIT. It is the second largest university research reactor in the U.S. and the only one located on the campus of a major research university. It is also the only university research facility in the U.S. where students can be directly involved in the development and implementation of nuclear engineering experimental programs with neutron flux levels comparable to power reactors. Undergraduates can learn to operate the reactor (some go on to work in industry as operators and team leaders, others go on to PhD programs).
Such medium scale and large-scale fission reactor experiments are best augmented with extensive auxiliary measurement capabilities, both in core and outside the core. For example, the MIT CRISP is a new University-Based Collaboration with a National Laboratory. CRISP stands for the Center for Reactor Instrumentation and Sensor Physics and is a joint undertaking with MIT and INL. The CRISP Vision to advance the current state of automation in nuclear systems by developing foundational technologies, as well as existing technology for monitoring and controls of future nuclear systems. The Focus Areas include Sensing Physics and Instrumentation; Signal Processing and AI/ML-based Data Analysis; and Advanced Controls and Decision Sciences.
The fission field has placed a renewed emphasis placed in recent years on research reactors that explore the utility of advanced reactors and microreactors. For example, the U.S. DOE is building a new research reactor at Idaho National Lab (INL) for the first time in over 40 years to help researchers understand how microreactors can integrate with other technologies. This is called the Microreactor Applications Research Validation and EvaLuation or MARVEL project (Marvel 2023). The MARVEL design is a liquid-metal cooled microreactor with Stirling engines that will produce 100 kW of energy using small amounts of high-assay, low-enriched uranium (HALEU) from available research materials. Its design is primarily based on existing technology and will be built using off-the-shelf components allowing for faster construction. The reactor is being built inside the Transient Reactor Test facility (TREAT) at INL and is expected to be completed in 2025, and will be connected to a microgrid to examine decarbonization pathways. Notably, universities will play key role in validation using codes and measurements on MARVEL, as there is recognition in the field of university leadership in this area. The INL approach to engagement broadly with universities is worth examining to see how this might translate to the fusion field.
Turning now to fusion, over the past 20 years, the US has made relatively few new investments in university based fusion confinement experiments. In fact, in 2016, the Alcator C-Mod tokamak was shuttered by the DOE, as were others such as the Electric Tokamak at UCLA a decade prior. These facilities, and others were important for creating workforce training and student research opportunities. Their closure occurred during a difficult time for the US Fusion Energy Science program.
Thankfully the new FESAC Long Range Plan from 2021 Calls Out the need for Synergy Between University Based Experiments and Large Facilities. That report explains, “SPARC will be parallel and complementary to international fusion efforts, including ITER, and to other ongoing private-sector fusion endeavors. The existing DIII-D and NSTX-U national tokamak facilities are key to preparation for the study of burning plasmas in ITER and in other planned and future private devices. Continuing support of existing university tokamak programs, and utilization of US expertise in theory and simulation, is needed to find solutions to remaining technical gaps. These gaps include disruption prediction, avoidance, and mitigation; plasma-facing component integration; and FPP- relevant scenario development.”
In fusion, there is also a desire to make power plants more compact, which will make them more economically competitive. Specifically, “It is highly desirable to reduce the costs of the research path for fusion energy development, as well as making the end-product - fusion reactors–sufficiently economically attractive. Of course, the economic attractiveness of power plants is determined by many variables. Studies of nuclear fission reactor costs have shown that factors such as utility structure, reactor size, regulatory regime, and international collaboration have the largest impact”, as noted in a recent community report (FESAC 2018). It is indeed notable that most of commercial fusion companies now active, including those that have raised the most capital from investors, promise compact fusion power plant concepts.
Based on lessons learned from fission, we believe that support for a thriving fusion energy industry would also require a variety of large-scale, medium-scale and small-scale experimental platforms at universities and national labs that can bridge between the wonderful foundational research of plasma physics and the applied need for fusion energy systems on the grid. We anticipate that soon, medium-scale tokamaks, stellarators, and other fusion research devices will be desirable at universities for the training of operators at fusion power plants.
5 Outlook and discussion
There are several frontier areas to emphasize in validation in fusion, involving “Multilevel, Multichannel, and Multimachine Comparisons”. Large uncertainties and model sensitivity can lead to fortuitous agreement and difficulty discriminating between models. One approach to overcome this challenge is to make use of a “Primacy Hierarchy” (Multilevel), whereby fluctuation amplitudes, correlation lengths, cross-phase angles, and inferred heat fluxes from power balance, are all compared simultaneously with models, often using quantitative metrics (Holland 2016). It is also important to probe both electron and ion heat transport simultaneously with particle and impurity transport (Multichannel) (Sciortino et al., 2020). And finally, while Multilevel and Multichannel validation are becoming more common, applying the same turbulence models and same measurements to different tokamaks, spherical tokamaks, stellarators, and other magnetic confinement configurations (Multimachine) has yet to be done extensively.
Frontier areas in fission validation include both the development of the new measurements and the development of new high-fidelity, physics based, fast running single and multiphase computational fluid dynamics (CFD) models. Such new models can be used to make predictions of performance in existing and next-generation fission reactors to optimize operation and improve safety and cost-competitiveness. For example, new high-fidelity CFD simulations are used to predict the evolution of flow driven component failure mechanisms, with thermal fatigue accumulation being one of the most challenging to address. Since turbulent mixing causes temperature oscillations in structural components that can lead to accelerated growth of cracks and failure over time. Simulations of the fatigue evolution for primary piping locations in the reactor, as well as other internal systems performed with CFD can be combined with AI enabled digital twins to allow for innovative predictive maintenance approaches for future advanced fission reactors.
As discussed in this perspective paper, validation of turbulent transport models in fusion is an exciting and active area of research, with relevance both for advancing fusion energy through prediction of new device design, as well as fundamental plasma physics research. Similarly, validation of turbulent (and boiling) transport models in fission is an exciting and active area of research, with relevance for fission energy research and fundamental physics research.
Consider this concise statement about boiling heat transfer in fission systems (Kommajosyula 2020): “Boiling is an efficient mode of heat transfer and is heat removal mechanism in power systems, including fission reactors. Physics-based models that describe boiling heat transfer can be an invaluable tool to increase the performance of fission reactors. New experiments with detailed data of boiling dynamics, including fundamental bubble parameters, are essential for validating such physics-based models.”
One could make a nearly identically structured statement about turbulent heat transfer in fusion systems: Turbulence is an extremely important mode of heat transfer and is a primary transport mechanism in fusion plasmas, like tokamaks. Physics-based models that describe turbulence-driven transport can be an invaluable tool to increase the performance of future fusion power plants. New experiments with detailed data of transport dynamics, including fundamental turbulence parameters, are essential for validating physics-based, predictive models.
These parallel statements for fusion turbulence and fission boiling studies help to summarize the deep connection between these two fields at a deeper level than perhaps previously explored in the literature, and considering these two statements were a helpful motivation for this perspective article.
Undoubtedly, to address climate change with fission energy and fusion energy systems, there must be a virtuous circle for university-based fission and fusion research, that attracts the most talented students from all backgrounds. The best students and faculty, researchers and scholars are always driven by grand challenges. The grand challenges can be intellectual (how does boiling work? How does plasma turbulence work?) or can be societal (how can turbulence be tamed to produce efficient, clean energy systems? How can physics understanding be used for optimization of net energy systems?).
To answer the questions posed about the future for university turbulent transport research in fusion or plasma physics research more broadly, we would argue that a fusion industry opens doors for university plasma physics research. The emergence of a new fusion energy industry will create more opportunities for fundamental research, especially in plasma turbulence and transport, at universities in the United States, not fewer. Fission power produces 20% of electricity in the USA; power plants have been reliably and safely operating over 60 years. Research on turbulence in neutral fluids is thriving at universities and has important applications for the fission industry. The fuel for fusion is a plasma, and that plasma is always turbulent.
There is a virtuous cycle of basic research motivated by grand challenges, which in turn, leads into applied science research with the goal of near-term industry impact, which is often driven by validation efforts that combine advances in experiment and simulation, Figure 7. Fusion-relevant grand intellectual challenges in plasma physics will remain long after fusion is on the grid. University researchers will engage with sponsors and collaborators including governments, national labs and private companies, and utilities around the world. Development of turbulence and transport measurements, and predictive simulation and modeling, in support of fusion and fission tell an evergreen story of innovation. The future of plasma physics research at universities is bright as we move through and beyond the era of burning plasmas to the era of ignited plasmas and fusion energy on the grid.
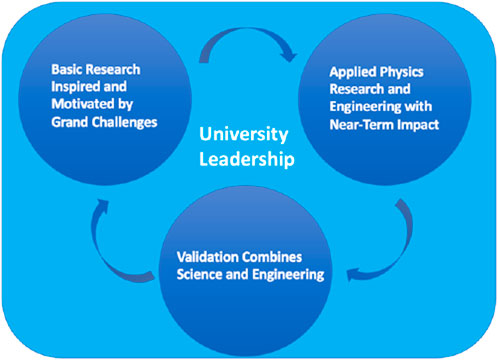
Figure 7. A virtuous cycle of basic research motivated by grand challenges, which in turn leads into applied science research with the goal of near-term industry impact, which is often driven by validation efforts that combine advances in experiment and simulation.
Data availability statement
The original contributions presented in the study are included in the article/Supplementary Material, further inquiries can be directed to the corresponding author.
Author contributions
AW: Writing–original draft. EB: Writing–review and editing. MB: Writing–review and editing. NH: Writing–review and editing. PR-F: Writing–review and editing.
Funding
The author(s) declare that no financial support was received for the research, authorship, and/or publication of this article.
Acknowledgments
The author AW is grateful to several colleagues in the fusion field for encouraging a perspective article on this topic.
Conflict of interest
The authors declare that the research was conducted in the absence of any commercial or financial relationships that could be construed as a potential conflict of interest.
Publisher’s note
All claims expressed in this article are solely those of the authors and do not necessarily represent those of their affiliated organizations, or those of the publisher, the editors and the reviewers. Any product that may be evaluated in this article, or claim that may be made by its manufacturer, is not guaranteed or endorsed by the publisher.
References
Abu-Shawareb, H., Acree, R., Adams, P., Adams, J., Addis, B., Aden, R., et al. (2022). Indirect drive ICF collaboration. Phys. Rev. Lett. 129, 075001. Published 8 August 2022. doi:10.1103/PhysRevLett.129.075001
Baglietto, (2021). Presented at annual nuclear review meeting ARPA-E. Available at: https://arpa-e.energy.gov/2021-annual-nuclear-review-meeting.
Baglietto, E., Demarly, E., and Kommajosyula, R. (2019). Boiling crisis as the stability limit to wall heat partitioning. Appl. Phys. Lett. 114, 103701. doi:10.1063/1.5080724
Brizard, A. J., and Hahm, T. S. (2007). Foundations of nonlinear gyrokinetic theory. Rev. Mod. Phys. 79, 421–468. doi:10.1103/revmodphys.79.421
CASL (2020). Consortium for advanced simulation of light water reactors. Oak Ridge, TN, USA: CASL Phase II Summary Report.
Citrin, , and Mantica, P. (2023). Overview of tokamak turbulence stabilization by fast ions. Plasma Phys. control. Fusion 65, 033001. doi:10.1088/1361-6587/acab2b
Fernandez, P. R., Howard, N., and Candy, J. (2022). Nonlinear gyrokinetic predictions of SPARC burning plasma profiles enabled by surrogate modeling. Nucl. Fusion 62, 076036. doi:10.1088/1741-4326/ac64b2
Fernandez, P. R., Howard, N. T., Greenwald, M. J., Creely, A. J., Hughes, J. W., Wright, J. C., et al. (2020). Predictions of core plasma performance for the SPARC tokamak. J. Plasma Phys. 86 (5), 865860503. doi:10.1017/s0022377820001075
FESAC (2018). Transformative enabling capabilities for efficient advance toward fusion energy. Available at: https://science.osti.gov/-/media/fes/fesac/pdf/2018/TEC_Report_1Feb20181.pdf.
FESAC (2021). Fusion energy sciences advisory committee long Range plan report. Available at: https://usfusionandplasmas.org/.
Freidberg, J. (2007). Plasma physics and fusion energy. Cambridge, United Kingdom: Cambridge University Press.
GENE (2023). Gene. Available at: https://genecode.org/(Accessed online January 30, 2024).
Glaser, A., and Goldston, R. J. (2012). Proliferation risks of magnetic fusion energy: clandestine production, covert production and breakout. Nucl. Fusion 52, 043004. doi:10.1088/0029-5515/52/4/043004
Hakim, , Mandell, N. R., Bernard, T. N., Francisquez, M., Hammett, G. W., and Shi, E. L. (2020). Continuum electromagnetic gyrokinetic simulations of turbulence in the tokamak scrape-off layer and laboratory devices. Phys. Plasmas 27, 042304. doi:10.1063/1.5141157
Holland, C. (2016). Validation metrics for turbulent plasma transport. Phys. Plasmas 23, 060901. doi:10.1063/1.4954151
Horton, W. (1999). Drift waves and transport. Rev. Mod. Phys. 71, 735–778. doi:10.1103/revmodphys.71.735
Howard, N. T., Holland, C., Rhodes, T., Candy, J., Rodriguez-Fernandez, P., Greenwald, M., et al. (2021). The role of ion and electron-scale turbulence in setting heat and particle transport in the DIII-D ITER baseline scenario. Nucl. Fusion 61, 106002. doi:10.1088/1741-4326/ac1bc2
Howard, N. T., Holland, C., White, A., Greenwald, M., and Candy, J. (2016). Multi-scale gyrokinetic simulation of tokamak plasmas: enhanced heat loss due to cross-scale coupling of plasma turbulence. Nucl. Fusion 56, 014004. doi:10.1088/0029-5515/56/1/014004
Howard, N. T., Holland, C., White, A. E., Greenwald, M., and Candy, J. (2014). Synergistic cross-scale coupling of turbulence in a tokamak plasma. Phys. Plasmas 21. doi:10.1063/1.4902366
Hughes, J. W., Snyder, P., Reinke, M., LaBombard, B., Mordijck, S., Scott, S., et al. (2018). Access to pedestal pressure relevant to burning plasmas on the high magnetic field tokamak Alcator C-Mod. Nucl. Fusion 58, 112003. doi:10.1088/1741-4326/aabc8a
Keilhacker, M., Gibson, A., Gormezano, C., and Rebut, P. (2001). The scientific success of JET. Nucl. Fusion 41, 1925–1966. doi:10.1088/0029-5515/41/12/217
Kommajosyula, R. (2020). PhD thesis in mechanical engineering and computation. Cambridge, MA, United States: Massachusetts Institute of Technology, Department of Mechanical Engineering. Available at: https://hdl.handle.net/1721.1/129051.
Lawson, J. D. (1957). Some criteria for a power producing thermonuclear reactor. Proc. Phys. Soc. B 70, 6–10. doi:10.1088/0370-1301/70/1/303
Marvel (2023). Marvel-microreactor-reaches-final-design-step. Available at: https://www.energy.gov/ne/articles/marvel-microreactor-reaches-final-design-step (Accessed January 30, 2024).
MIT News (2015). Boiling-more-efficient-less-dangerous-power-plants-0908. Available at: https://news.mit.edu/2015/boiling-more-efficient-less-dangerous-power-plants-0908 (Accessed January 30, 2024).
National Academies of Sciences, Engineering, and Medicine (2021). Bringing fusion to the U.S. Grid. Washington, DC: The National Academies Press. doi:10.17226/25991
NIF (2023). Lawrence livermore national laboratory website. Available at: https://lasers.llnl.gov/science/pursuit-of-ignition (Accessed January 30, 2023).
Rhodes, T. L., Holland, C., Smith, S., White, A., Burrell, K., Candy, J., et al. (2011). L-mode validation studies of gyrokinetic turbulence simulations via multiscale and multifield turbulence measurements on the DIII-D tokamak. Nucl. Fusion 51, 063022. doi:10.1088/0029-5515/51/6/063022
Richenderfer, A., Kossolapov, A., Seong, J. H., Saccone, G., Demarly, E., Kommajosyula, R., et al. (2018). Investigation of subcooled flow boiling and CHF using high-resolution diagnostics. Exp. Therm. Fluid Sci. 99, 35–58. doi:10.1016/j.expthermflusci.2018.07.017
Sciortino, F., Howard, N., Marmar, E., Odstrcil, T., Cao, N., Dux, R., et al. (2020). Inference of experimental radial impurity transport on Alcator C-Mod: bayesian parameter estimation and model selection. Nucl. Fusion 60, 126014. doi:10.1088/1741-4326/abae85
Staebler, G. M., Howard, N., Candy, J., and Holland, C. (2017). A model of the saturation of coupled electron and ion scale gyrokinetic turbulence. Nucl. Fusion 57, 066046. doi:10.1088/1741-4326/aa6bee
Staebler, G. M., Kinsey, J. E., and Waltz, R. E. (2007). A theory-based transport model with comprehensive physics. Phys. Plasmas 14 (5), 055909. doi:10.1063/1.2436852
Wang, C., Su, G., Akinsulire, O., Zhang, L., Rahman, Md M., and Bucci, M. (2024). Investigation of critical heat flux enhancement on nanoengineered surfaces in pressurized subcooled flow boiling using infrared thermometry. Heat. Transf. Eng. 45 (4-5), 417–432. doi:10.1080/01457632.2023.2191441
White, A. E. (2019). Validation of nonlinear gyrokinetic transport models using turbulence measurements. J. Plasma Phys. 85 (1), 925850102. doi:10.1017/S0022377818001253
Whyte, D. G., Paz-Soldan, C., and Wirth, B. (2023). The academic research ecosystem required to support the development of fusion energy. Phys. Plasmas 30. doi:10.1063/5.0167369
Wilcox, R. S., Wingen, A., Cianciosa, M., Ferraro, N., Hirshman, S., Paz-Soldan, C., et al. (2017). Modeling of 3D magnetic equilibrium effects on edge turbulence stability during RMP ELM suppression in tokamaks. Nucl. Fusion 57, 116003. doi:10.1088/1741-4326/aa7bad
Wurzel, , and Hsu, S. C. (2022). Progress toward fusion energy breakeven and gain as measured against the Lawson criterion. Phys. Plasmas 29, 062103. doi:10.1063/5.0083990
Wyk, F. van, Highcock, E. G., Field, A. R., Roach, C. M., Schekochihin, A. A., Parra, F. I., et al. (2017). Ion-scale turbulence in MAST: anomalous transport, subcritical transitions, and comparison to BES measurements. Plasma Phys. control. Fusion 59, 114003. doi:10.1088/1361-6587/aa8484
Zhang, L., Wang, C., Su, G., Kossolapov, A., Matana Aguiar, G., Seong, J. H., et al. (2023). A unifying criterion of the boiling crisis. Nat. Commun. 14, 2321. doi:10.1038/s41467-023-37899-7
Zweben, S., Lampert, M., and Myra, J. R. (2022). Temporal structure of blobs in NSTX. Phys. Plasmas 29, 072504. doi:10.1063/5.0097282
Keywords: fusion, tokamak, validation, turbulence, fission
Citation: White AE, Baglietto E, Bucci M, Howard NT and Rodriguez-Fernandez P (2024) Fusion plasma turbulence research beyond the burning plasma era: perspectives on transport model validation in fusion and fission. Front. Nucl. Eng. 3:1380108. doi: 10.3389/fnuen.2024.1380108
Received: 01 February 2024; Accepted: 02 April 2024;
Published: 07 May 2024.
Edited by:
Anne Campbell, Oak Ridge National Laboratory (DOE), United StatesReviewed by:
Rinkle Juneja, Oak Ridge National Laboratory (DOE), United StatesCopyright © 2024 White, Baglietto, Bucci, Howard and Rodriguez-Fernandez. This is an open-access article distributed under the terms of the Creative Commons Attribution License (CC BY). The use, distribution or reproduction in other forums is permitted, provided the original author(s) and the copyright owner(s) are credited and that the original publication in this journal is cited, in accordance with accepted academic practice. No use, distribution or reproduction is permitted which does not comply with these terms.
*Correspondence: A. E. White, d2hpdGVhQG1pdC5lZHU=