- 1Australian Nuclear Science and Technology Organization, Sydney, NSW, Australia
- 2Centre for Medical Radiation Physics, University of Wollongong, Wollongong, NSW, Australia
Introduction: The most common current uranium target type for 99Mo production uses plate geometry. This paper investigates the effects of geometry on 99Mo output and target sustainability.
Methods: MCNP6.2 was used to model rectangular, spherical, and cylindrical targets ranging from 12.03 cm3 to 120.34 cm3 to determine the target geometry and volume effects on 99Mo output, sustainability, proliferation concerns, and heating. 4-7 days irradiation was used with a consistent target density of 2 g/cm3 UO2 for all target types.
Results and Discussion: The cylindrical target was found to have the best performance due to having the second highest sustainability, the highest 99Mo output and produce the lowest amounts of 239Pu compared to the other target geometries. The heating comparison showed that there were negligible heating concerns for all target volumes and geometries.
1 Introduction
When devising an operations strategy, 99Mo producers must consider the available irradiation and target configurations, as these variables can affect 99Mo output and wastes generated. Historically, uranium targets have been based on fuel elements for reactors and are therefore made of a mixture of uranium with an aluminum cladding, with target dimensions being limited by the cooling systems of the reactors (Lee et al., 2016). Uranium targets for 99Mo production are irradiated in research reactors that are moderated and cooled with light water. These types of reactors use plate-type fuel to achieve a higher power density (Saliba-Silva et al., 2011). The targets currently used in 99Mo production are manufactured to MTR fuel specification, with a qualified burnup of more than 30%, which is much greater than the actual burnup of the target for 99Mo production purposes. Other types of uranium targets that can be used for 99Mo production include uranium silicide, uranium nitride, uranium metal, uranium oxide, and uranium aluminide alloy (International Atomic Energy Agency, 2013). Despite being based on reactor fuel, the burnup of 235U is 10–20 times less for the targets due to their relatively short irradiation time compared to reactor fuel. Due to the lower burnup, current research is attempting to increase the amount of 235U present in the target by increasing the target density, which, as a side effect, also increases the volume of radioactive wastes produced (Beyer et al., 2016). Radioactive wastes are increased further by producers’ change from low-enriched uranium (LEU) to high-enriched uranium (HEU), as the amount of uranium in the targets increases from approximately 1 gU/cm3 to 2.6 gU/cm3. This increases operational costs for 99Mo manufacturers, as manufacturing processes must be modified to address the higher amount of uranium in the targets and the radioactive wastes produced (Sameh, 2013).
Target geometry can affect 99Mo output due to the self-shielding effects of uranium; a higher amount of self-shielding reduces uranium fission rates. One study found that the neutron self-shielding factors of UO2 pellets weighing 1.2, 12, and 120 g were 0.76, 0.65, and 0.58, respectively, indicating that with a larger pellet mass, larger self-shielding factors and less fission would occur (Yamabayashi, 1979). This indicates that the higher the uranium loading of a target, the more the target will be self-shielding (Davidson et al., 2017). Target geometry can also affect 99Mo extraction if the target is not intended to be dissolved or destroyed in any manner. One proposed method of removing 99Mo from a UO2 target is via fluoride volatility, using nitrogen tetrafluoride to remove Mo in a gaseous state from UO2 without the need to dissolve or destroy the target (McNamara et al., 2020). Another method of gaseous extraction being developed by General Atomics involves a non-destructive process with a target that is cylindrical in nature. It has been demonstrated to be effective at removing 99Mo from the uranium target and has the potential for reuse after further irradiation (Bertch, 2014). These types of processes would appear to be best suited to a cylindrical-type target design, which would act like a column and take full advantage of the neutron flux. Clearly, a plate-type target would perform poorly in such processes due to its geometry, as a flow rate through the target is needed to pass the extraction materials. The heat that a uranium target produces under irradiation conditions is important for reactor safety and target handling, and it is expected that target geometry affects the heat obtained. It is expected that a cylindrical uranium target would hold more heat than a plate-type target after irradiation. Target proliferation concerns also need to be addressed (Raposio et al., 2023); the amounts of 239Pu produced by targets of different geometries need to be considered as well.
2 MCNP6.2 modeling
In the quest for a more sustainable method of fission-based 99Mo production, the concept of a reusable uranium-based target was explored (Raposio et al., 2021), with target efficiency defined as the amount of 99Mo activity produced per gram of 235U present in the target, with the aim of designing a uranium target with a high burnup. This study was followed by research developing the target sustainability index (Raposio et al., 2023). In this study, a theoretical uranium-oxide-based target (UO2) for manufacturing 99Mo gave results indicating that a low target density of 235U leads to high sustainability, with the trade-off of a much lower 99Mo output. To solve the output problem, this study investigates whether maintaining the lower density of the target while increasing the volume and thus the total amount of 235U will lead to an increased 99Mo output, and whether this also influences the sustainability of the target. It proposes that increasing the volume of the target while maintaining a constant low-target density is likely to minimize the effects of target self-shielding because the target has a large percentage of 238U and therefore a high sustainability index and 99Mo output. The 239Pu amounts present in the targets after irradiation are also examined for non-proliferation purposes.
The following simulations were modeled using MCNP6.2 (Werner et al., 2018) and CINDER90, with reactor dimensions and target positioning identical to the model reactor used previously (Raposio et al., 2021; Raposio et al., 2023) and the sustainability index calculated as outlined in Raposio et al. (2023).
In an extension of the previous study (in press), we varied the target geometry, and hence volume, by maintaining a constant target radius and density and increasing the target height. The volume was increased from 12 cm3 to 120 cm3 in intervals of approximately 0.8 cm3, with a corresponding height increase from 3 cm to 30 cm in intervals of 0.2 cm while the radius was kept constant at 1.13 cm. The target density was maintained at 2 g/cm3 for all target volumes. For comparison, spherical and rectangular prism targets were modeled in addition to the cylindrical target to investigate the effects of a large change in geometry on the sustainability index and 99Mo output. The radius was increased in increments that maintained the same volume increases as the cylindrical target. Additionally, the rectangular prism target was also modeled with fixed width, thickness, and density, and with variable height, for further comparison and to determine the effects of target geometry on the sustainability index and 99Mo output. The three target geometries were then modeled for heat obtained during irradiation conditions using the F6 tally in MCNP6.2 (Werner et al., 2018) to give heat in MeV/g. Finally, the amount of 239Pu produced in the three target types was modeled to determine the proliferation risks of using the different target geometries and volumes to give 100,000 GBq runs to produce 1 bare critical mass (BCM) of 239Pu.
The volumes of the targets were calculated by the formulas
where V is the volume, r is the radius, h is the height, w is the width, and t is the thickness of the target. To develop a hypothesis in which target geometry is expected to have the highest sustainability, the target self-shielding factors must be considered. Since all targets in this study have the same volume for each data point, it is expected that the target with the largest ratio of surface area to volume would have the least self-shielding. The surface areas for the three target types are given as follows:
Therefore, the ratios of the target surface areas to volumes are
Example ratios for three different target volumes are given in Table 1.
As shown in Table 1, the rectangular prism has the largest ratio of surface area to volume and the spherical target has the lowest. Therefore, is it expected that the rectangular prism will have the highest sustainability and the spherical target will have the lowest.
Consistent with previous research, irradiation times of 4, 5, 6, and 7 days were used for this modeling, as these were found to be the optimal irradiation times for maximizing the sustainability index of the targets. One percent enriched UO2 targets were used.
The heating of the targets under irradiation conditions was studied using the F6 tally in MCNP6.2 (Werner et al., 2018) for the smallest-, middle-, and largest-volume targets, to ascertain whether heating is affected by target volume or geometry, with a consistent target density of 2 g/cm3 and an initial neutron number of 50,000. MCNP6.2 performs 10 statistical checks on its tallies, as described in Table 2, and the results of the modeling were checked for statistical significance.
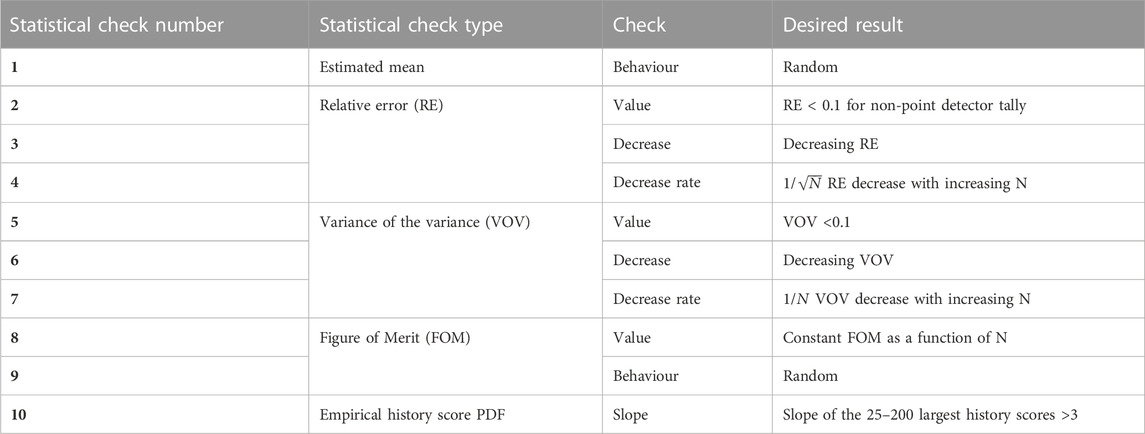
TABLE 2. The 10 statistical tests performed by MCNP. Source: Forster et al. (1994).
3 Results
3.1 Cylindrical target
Firstly, the sustainability index was examined for the target under the above conditions, with the number of initial neutrons set at 5,000, and the results are shown in Figure 1. Next, target output was plotted under the same irradiation conditions, and the results are given in Figure 2. Next, 235U burnup was compared for irradiation times of 4–7 days and increasing target volumes to determine whether a greater target volume resulted in a higher total burnup of 235U due to a larger initial amount of 235U being present in the target. The results are given in Figure 3.
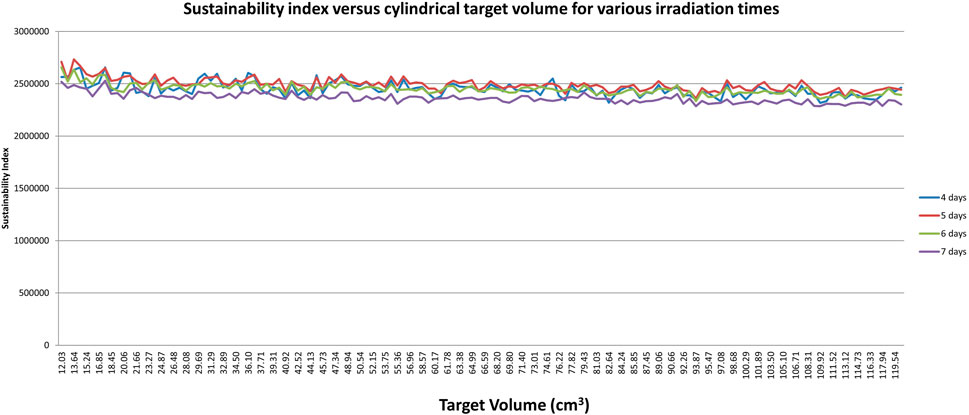
FIGURE 1. How sustainability index varies with increasing target volume for a 1% enriched cylindrical UO2 target.
3.2 Variance in graphs
In Figure 1, the graph of sustainability index versus target volume has a variation in the lines for irradiation times of 4–7 days. This variation also appears in Figure 2 for the lines representing 99Mo output versus target volume. However, in Figure 3—which has data from the same simulations as the data in Figures 1 and 2—there appears to be minimal variation.
MCNP modeling results contain standard deviations that decrease as the number of histories increases, according to the formula
where ES is the standard deviation produced from MCNP and N is the number of histories used in the simulation (García-Herranz et al., 2008). These errors can be produced by uncertainties in the nuclear input data, transport calculation, or normalization factor (García-Herranz et al., 2009). When performing multi-time calculations of fuel burnup using MCNP6.2, the stochastic relative error is produced for each time step but not the total errors in isotopic concentrations. One way to increase the accuracy of MCNP6.2 results is to use the backward Euler numerical method to solve the Bateman equations; this was found to be an effective method for a multi-time step burnup simulation (Chirayath et al., 2021). Another method for decreasing the MCNP6.2 calculation errors is to increase the number of initial neutrons for the simulations and to maintain the same number of cycles to produce a larger data set of neutron histories. For example, if the number of histories used was 5,000, a relative error of magnitude 12% for flux tallies would result, whereas an increase from 5,000 to 50,000 neutron histories for the simulation would reduce the relative error of the flux tallies to approximately 5%, or 2.4 times smaller (García-Herranz et al., 2009). In this study, it was decided to determine the variance by increasing selected target volumes (approximately every 1.5 cm increase in target height data points was used) and repeating the MCNP6.2 simulations with 50,000 incident neutrons instead of the initial 5,000. A sampling of target volumes was used instead of the whole range due to computational time constraints.
Variance was calculated using the following formula:
where V is variance, R5k is the result of the 5,000-initial-neutron simulation and R50k is the result of the 50,000-initial-neutron simulation. This variance calculation was then applied to 235U burnup, 99Mo output, and the sustainability index; the results for 235U burnup were less than ±0.4%, but there was more significant variance (±6%) for 99Mo output and the sustainability index. Figure 4 shows the sustainability index for the same targets but with 50,000 initial neutrons instead of 5,000. Figure 5 shows the original target dimensions but with 50,000 initial neutrons instead of 5,000.
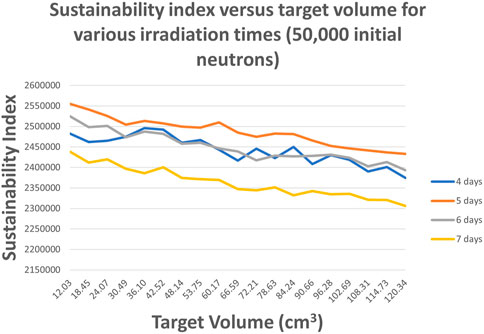
FIGURE 4. How sustainability index varies with increasing target volume for 50,000 initial neutrons.
3.3 Spherical target
Next, the effects of changing the target geometry to a sphere instead of a cylinder were determined using 50,000 initial neutrons, as this value was found to have lower errors (see Figures 6,7).
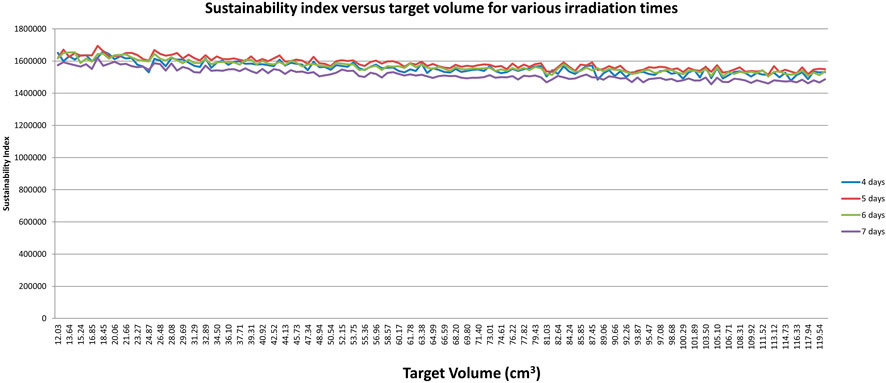
FIGURE 6. How sustainability index varies with increasing target volume for a 1% enriched spherical UO2 target.
3.4 Rectangular prism target
Next, the effects of changing the target geometry to a rectangular prism were determined using 50,000 initial neutrons, as this value was found to have lower errors (see Figures 8,9).
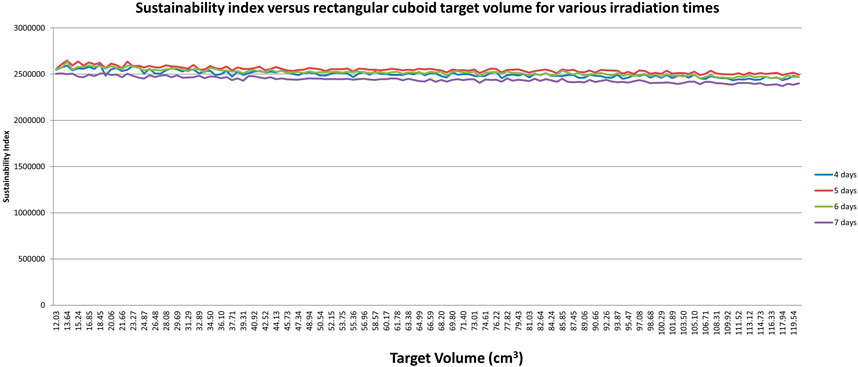
FIGURE 8. How sustainability index varies with increasing target volume for a 1% enriched rectangular prism UO2 target.
3.5 239Pu analysis
239Pu amounts in the targets after irradiation were examined. The number of production runs required to produce 1 BCM of 239Pu (10.4 kg) is given in Figure 10.
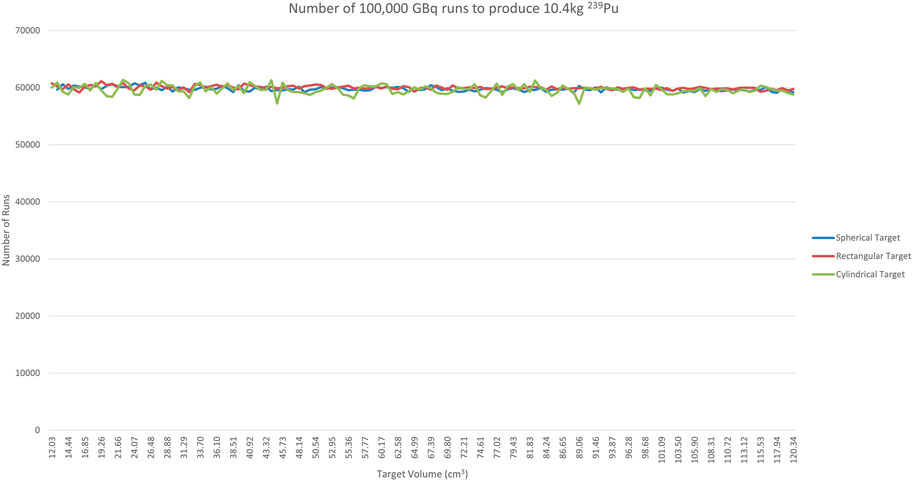
FIGURE 10. Number of 100,000 GBq production runs to produce 1 BCM of 239Pu for various target geometries and volumes.
3.6 Target heating
Table 3 shows the results of the target heating F6 tally output of the simulations.
4 Discussion
In Figure 1, the sustainability index value drops slightly as the target volume is increased, ranging from a high of 2,731,886 Bq2/g2 for a target volume of 13.63 cm3 at 5 days irradiation to a low of 2,286,334 at a target volume of 93.87 cm3 at 7 days irradiation. This is approximately a 19% increase between the highest and lowest sustainability index scores, which can be considered relatively flat as the volume is increased by 689% between these two extreme points. Figure 2 shows that longer irradiation times lead to higher 99Mo output, which is to be expected. Interestingly, Figure 2 clearly demonstrates that 99Mo output increases in a relatively linear manner, with the volume increase one would expect given the higher amount of 235U present in a target with higher volume and constant density. As shown in Table 1, with a roughly 10% increase in target volume, 99Mo output is increased by between 9.20% and 9.65% across all irradiation times (4–7 days). Therefore, as the target volume increases, the sustainability remains almost the same while the yield increases, meaning that targets can be manufactured to be sustainable and produce desired yields. For example, Table 4 shows 12 different configurations that would produce approximately 10,500 GBq. Table 5 suggests that a larger target volume could mean a shorter irradiation time to produce the same yield as a smaller target with a longer irradiation time. Advantages of a short irradiation time include faster turnaround time for target processing and thus higher total output of 99Mo production over a week. As demonstrated by Table 4, the simulations of 50,000 versus 5,000 initial neutrons show relatively small variance between the two results (less than ±0.4%). This indicates that the initial simulations are a close enough result and that there is a slight gain in value from increasing the number of initial neutrons.
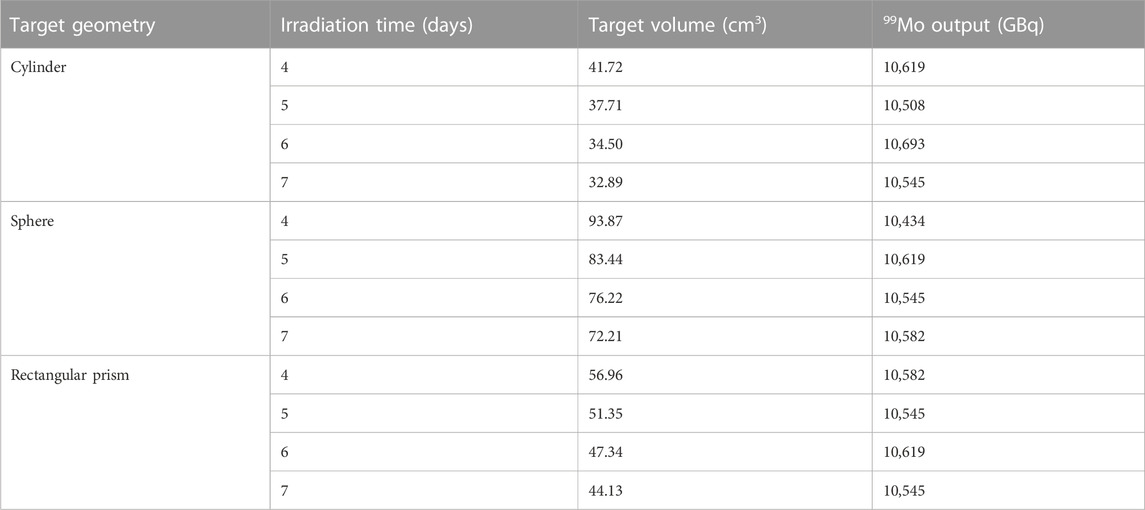
TABLE 4. Comparison of irradiation times with target volumes and geometries to produce desired99Mo outputs.
An unintended artifact was observed throughout the simulations: a variation in the graph lines in Figures 1, 2, which did not seem to make sense from a neutronics point of view. Examination of the variance while repeating some measurements with 50,000 initial neutrons to reduce the standard deviation of the results showed little variance in the results for 235U burnup (less than ±0.4%), but a more significant variance (±6%) for 99Mo output and the sustainability index. Although ±6% is not a large error, target designers and 99Mo producers could determine theoretical outputs more accurately if they used 50,000 initial neutrons for running simulations using MCNP6.2. Figure 5 shows that the difference between 50,000 and 5,000 initial neutrons in the MCNP6.2 simulations leads to a larger but manageable variance in 99Mo output (less than ±5%). However, this variance of ±5% of 99Mo output could be a contributing factor to the variance in lines in Figure 2. The variance in the sustainability index in Figure 1 can also be explained by this variance, as it is approximately ±6% (Table 4). The explanation for this is that the sustainability index is a function of 235U burnup and 99Mo output, and therefore the errors in the 99Mo output would carry over to the sustainability index. The lines in Figure 4 are much smoother for 50,000 than for 5,000 initial neutrons, indicating that the variation in Figure 1 is from the increased statistical errors due to the low initial number of neutrons. Thus, if the sustainability index needs to be predicted with greater than ±6% accuracy, a higher number of initial neutrons can be used in the MCNP6.2 simulation. Figure 8 also shows that when modeling for 99Mo output, the number of initial neutrons can be increased to reduce statistical error, making forecasting 99Mo yields easier.
The first objective of this study was to determine whether the sustainability of a target can be maintained at a constant density. Figure 1 shows that this can be nearly achieved when the sustainability index drops slowly as the volume increases, although it remains relatively flat and is promising for future target development. The drop in sustainability could be attributed to the increased target size, and therefore target thickness, which in turn would lead to increased self-shielding effects. This effect was also observed for the spherical (see Figure 6) and rectangular prism (see Figure 8) targets, although the sustainability index for the spherical target was lower compared to the cylindrical target, which was lower compared to the rectangular prism target, which was to be expected due to the geometry difference. The second objective of this study was to determine if 99Mo output could be increased for a relatively similar sustainability index. Figure 2 shows that this is indeed possible, as 99Mo output increases linearly with cylindrical target volume, which is to be expected as the amount of 235U increases linearly with target volume. Figure 7 shows this to also be the case for the spherical target, as 99Mo output increases with increasing target volume; Figure 9 confirms that this is also the case for the rectangular prism target. Figure 11 compares 99Mo outputs for the different target geometries with a 7-day irradiation, clearly showing that the cylindrical and rectangular geometries have the greatest output and that the spherical target has the least favorable output for the target volume and density modeled. This can be explained by self-shielding, as the cylindrical and rectangular targets had a fixed "thickness" while the height was modified to achieve the desired volume, whereas for the spherical target, only the radius could be increased, which naturally involves increasing the "thickness" of this target type, leading to increased self-shielding.
This opens a new possibility in the target design process for the targeting of specific 99Mo activities, based not only on irradiation time but also on target volume. The results in Table 1 predicted that the rectangular prism would have the highest sustainability and that the spherical target would have the lowest sustainability, which was confirmed by the modeling results (Figures 1,6,8). Another notable finding is that the negative sustainability slopes in Figures 1,6,8 are highest for the spherical target and lowest for the rectangular prism, which shows that geometry differently affects the sustainability over different target volumes. As Table 6 shows, an output of approximately 10,000 GBq can be produced for different target volumes, irradiation times, and geometries. Such options for target design could allow for more flexibility in 99Mo production and thus less overproduction and waste if a fixed target volume is used. This contrasts with current LEU targets, which produce varying yields based on irradiation time since the variables that can be controlled, such as the geometry and volumes, are fixed. The LEU targets hit a maximum output at approximately 10 days of irradiation, which becomes the limitation of the target.
Regarding the non-proliferation and minimization of 239Pu produced in the targets after irradiation, Figure 10 shows that the cylindrical, spherical, and rectangular targets can be used approximately 60,000 times to produce an output of 100,000 GBq of 99Mo and to create 1 BCM of 239Pu. This indicates that target geometry has no effect on 239Pu production.
For target heating, Table 3 shows that the heating remains consistent over target volume changes (target size), with a slight reduction in heating as target volume increases. Although heating is highest for the cylindrical target and lowest for the spherical target, heating across all three target types is very low. The heating tallies passed most of the statistical tests, indicating high accuracy for the results.
5 Conclusion
The possibility of negating the loss of 99Mo output with a high sustainability index was investigated. It was shown that since low-density uranium targets have a high sustainability index, due to the reduced self-shielding effects of 238U, maintaining a low density while increasing the total amount of 235U in the target by increasing the target volume leads to a relatively stable sustainability index for the target while increasing 99Mo output linearly. This was demonstrated for cylindrical, spherical, and rectangular prism type targets, although a rectangular prism geometry produces the highest sustainability index. The cylindrical target was found to have the second-highest sustainability and the highest 99Mo output, and to produce the lowest amounts of 239Pu, compared to the other target geometries. Heating comparison showed that there are negligible heating concerns for all target volumes and geometries. These results show how the sustainability index can be practically used as a way for uranium target designers to develop customized targets to produce a specific 99Mo output to meet market demands, as they can configure targets based on volume and irradiation time parameters to produce the desired output while maintaining a relatively similar amount of sustainability for their targets. The variance in the results was examined to better understand a variance in the predicted linear graphs; it was found to be likely due to a small initial neutron size and the statistical error this caused in MCNP6.2. When the simulations were repeated with 10 times the number of initial neutrons, the variance effects disappeared and the trends in the graphs became more linear. Therefore, uranium target developers would benefit from using at least 50,000 initial neutrons when performing simulations using MCNP6.2 for sustainable target development in order to forecast 99Mo output more accurately.
Data availability statement
The raw data supporting the conclusions of this article will be made available by the authors, without undue reservation.
Author contributions
RR: first authorship; GB: review of MCNP data; AR: reviewer and supervisor; GT: reviewer and supervisor; JW: review of MCNP data.
Conflict of interest
The authors declare that the research was conducted in the absence of any commercial or financial relationships that could be construed as a potential conflict of interest.
Publisher’s note
All claims expressed in this article are solely those of the authors and do not necessarily represent those of their affiliated organizations, or those of the publisher, the editors, and the reviewers. Any product that may be evaluated in this article, or claim that may be made by its manufacturer, is not guaranteed or endorsed by the publisher.
References
Bertch, T. C. (2014). Selective gaseous extraction: Research, development and training for isotope production. Final technical report. Columbia, United States: University of Missouri Research Reactor Center.
Beyer, G. J., Eichler, B., Reetz, T., Muenze, R., and Comor, J. J. (2016). New head process for non-HEU 99Mo production based on the oxidation of irradiated UO2-pellets forming soluble U3O8. Nucl. Technol. Radiat. Prot. 31 (1), 102–108. doi:10.2298/ntrp1601102b
Chirayath, S. S., Schafer, C. R., and Long, G. R. (2021). A new methodology to estimate stochastic uncertainty of MCNP-predicted isotope concentrations in nuclear fuel burnup simulations. Ann. Nucl. Energy 151, 107911. doi:10.1016/j.anucene.2020.107911
Davidson, E. E., Betzler, B. R., Chandler, D., and Ilas, G. (2017). Heat deposition analysis for the High Flux Isotope Reactor’s HEU and LEU core models. Nucl. Eng. Des. 322, 563–576. doi:10.1016/j.nucengdes.2017.06.040
Forster, R. A., Booth, T. E., and Pederson, S. P. (1994). “Ten new checks to assess the statistical quality of Monte Carlo solutions in MCNP,” in 8th International Conference on Radiation Shielding, Arlington, TX (United States), 24-27 Apr 1994.
García-Herranz, N., Cabellos, O., Sanz, J., Juan, J., and Kuijper, J. C. (2008). Propagation of statistical and nuclear data uncertainties in Monte Carlo burn-up calculations. Ann. Nucl. Energy 35 (4), 714–730. doi:10.1016/j.anucene.2007.07.022
García-Herranz, N., Cabellos, O., and Sanz, J. (2009). Assessment of the MCNP-ACAB code system for burnup credit analyses.
International Atomic Energy Agency (2013). Basic principles objectives IAEA nuclear Energy series non-HEU production technologies for molybdenum-99 and technetium-99m. Available at: http://www.iaea.org/Publications/index.html.
Lee, S. K., Beyer, G. J., and Lee, J. S. (2016). Development of industrial-scale fission 99Mo production process using low enriched uranium target. Nucl. Eng. Technol. 48 (3), 613–623. doi:10.1016/j.net.2016.04.006
McNamara, B. K., O’Hara, M. J., Clark, R. A., Morrison, S. S., Soderquist, C. Z., and Scheele, R. D. (2020). Gas-phase molybdenum-99 separation from uranium dioxide by fluoride volatility using nitrogen trifluoride. RSC Adv. 10 (6), 3472–3478. doi:10.1039/c9ra10270a
Raposio, R., Braoudakis, G., Rosenfeld, A., Thorogood, G. J., and Bedwell-Wilson, J. (2023). Investigating an alternative sustainable low enriched uranium target for the manufacture of 99Mo using MCNP6.2 modeling with CINDER90. Front. Nucl. Eng. 1, 978948. doi:10.3389/fnuen.2022.978948
Raposio, R., Braoudakis, G., Rosenfeld, A., and Thorogood, G. J. (2021). Modeling of reusable target materials for the production of fission produced 99Mo using MCNP6.2 and CINDER90. Appl. Radiat. Isotopes 176, 109827. doi:10.1016/j.apradiso.2021.109827
Saliba-Silva, A. M., Urano de Carvalho, E. F., Riella, H. G., and Durazzo, M. (2011). “Research reactor fuel fabrication to produce radioisotopes,” in Radioisotopes – application in physical sciences. Editor N. Singh (London, UK: InTechopen).
Sameh, A. H. A. (2013). Production cycle for large scale fission mo-99 separation by the processing of irradiated leu uranium silicide fuel element targets. Sci. Technol. Nucl. Installations 2013, 1–14. doi:10.1155/2013/704846
Werner, C. J., Bull, J. S., Solomon, C. J., Brown, F. B., Mckinney, G. W., Rising, M. E., et al. (2018). MCNP ® release notes MCNP version 6.2 release notes. Oak Ridge: OSTI.GOV.
Keywords: MCNP modeling, Mo-99 production, uranium target, sustainability, target design
Citation: Raposio R, Braoudakis G, Rosenfeld A, Bedwell-Wilson J and Thorogood G (2023) Investigating 99Mo output changes in high-sustainability uranium targets by modifying target volume and geometry. Front. Nucl. Eng. 2:978990. doi: 10.3389/fnuen.2023.978990
Received: 27 June 2022; Accepted: 13 February 2023;
Published: 02 March 2023.
Edited by:
Daniel Jädernäs, Studsvik , SwedenReviewed by:
Jacob G. Fantidis, International Hellenic University, GreeceLuteng Zhang, Chongqing University, China
Copyright © 2023 Raposio, Braoudakis, Rosenfeld, Bedwell-Wilson and Thorogood. This is an open-access article distributed under the terms of the Creative Commons Attribution License (CC BY). The use, distribution or reproduction in other forums is permitted, provided the original author(s) and the copyright owner(s) are credited and that the original publication in this journal is cited, in accordance with accepted academic practice. No use, distribution or reproduction is permitted which does not comply with these terms.
*Correspondence: Robert Raposio, rra@ansto.gov.au