- 1Collaborative Laboratories for Advanced Decommissioning Science (CLADS), Japan Atomic Energy Agency, Ibaraki, Japan
- 2The Nuclear Damage Compensation and Decommissioning Facilitation Corporation (NDF), Tokyo, Japan
This review is an up-to-date report of the analysis of U-bearing samples from the Fukushima Daiichi Nuclear Power Station (1F). It summarizes the experience gained after previous severe nuclear accidents in the field of fuel debris analysis and the utilization of the results. Current methods of 1F sample analysis and the main results are presented with a discussion on future strategies of fuel debris analysis and the requirements for 1F decommissioning.
1 Introduction
The Great East Japan Earthquake and subsequent tsunami on 11 March 2011 caused a severe accident at the Fukushima Daiichi NPS (further referred to as 1F)—which was the most severe NPP nuclear disaster since the Three Mile Island and Chernobyl accidents. It led to the relocation of tens of thousands of local citizens and significant radioactive contamination (Fukushima Prefectural Government, 2019; Hidaka et al., 2022). During the accident, the reactor cores melted at 1F Units 1, 2, and 3 (International Atomic Energy Agency, 2015). Several months after the accident, the government of Japan declared its intention to completely decommission 1F, with fuel debris removal and site clean-up (Tokyo Electric Power Company, Inc, 2011). One of the most difficult problems during decommissioning is the handling of damaged core materials (“fuel debris”). This challenging goal can be generally divided into two main tasks:
- The removal and packaging of fuel debris in suitable containers.
- The final stage of fuel debris management, such as intermediate or long-term storage, reprocessing or conditioning, and final disposal.
To solve these problems, the “Mid-and-Long-Term Roadmap towards the Decommissioning of TEPCO’s Fukushima Daiichi Nuclear Power Station” (further referred to as “the Roadmap”) was adopted in December 2011 by the Japanese government and the TEPCO Council on Mid-to-Long-Term Response for Decommissioning (Tokyo Electric Power Company, Inc, 2011). The Roadmap is being adjusted as new issues are uncovered during decommissioning. According to the latest revision of the Roadmap issued in 2019 (Tokyo Electric Power Company Holdings, Inc., 2019b), 1F decommissioning is planned to be completed within 30–40 years of the cold shutdown of December 2011. Since 2014, the Nuclear Damage Compensation and Decommissioning Facilitation Corporation (NDF) has coordinated the overall progress of decommissioning, information exchange, and collaboration by the stakeholders (Nuclear Damage Compensation and Decommissioning Facilitation Corporation, 2023). In 2015, NDF issued and kept the regularly updated “Technical Strategic Plan”, which is intended to provide a technical basis for the government’s Roadmap (Nuclear Damage Compensation and Decommissioning Facilitation Corporation, 2022).
Both of these fundamental documents reflect the importance of the debris retrieval process for the 1F decommissioning and define the necessary actions to be taken in the coming decades. However, there is still a gap to be bridged before full-scale fuel debris removal can commence. Fuel debris removal and packaging require data such as an estimation of its quantity, remaining radioactivity and decay heat, chemical and isotope composition, its distribution within the units, its mechanical and chemical properties, the degree of corrosion, and any remaining potential hazards such as hydrogen generation. The analysis of small amounts of debris becomes indispensable to obtaining this information. In turn, such information plays an important role as a background to decisions about retrieval methods and packaging container features. The development and implementation of the retrieval of gram quantities of various debris, some of which will be used for further research, will be one of the first priorities for the next several years. After analysis of the first gram-scale debris from the trial retrieval, TEPCO will gradually scale-up the retrieval process (Tokyo Electric Power Company Holdings, Inc., 2019b).
Safe and reasonable waste management is the inevitable issue for the final stage of decommissioning. Low- and middle-level waste management plans have already been reflected in the Roadmap (Tokyo Electric Power Company Holdings, Inc., 2019b); however, discussions on the waste management of high-level waste, including 1F fuel debris, are still in the preliminary stage. This was highlighted in an independent peer review of the Roadmap by the IAEA Review Team on the detailed characterization of fuel debris not only for the retrieval process but also for developing future strategies for the management of this material, such as initial storage, potential treatment, and conditioning (International Atomic Energy Agency, 2021a). According to the NDF Technical Strategic Plan (Nuclear Damage Compensation and Decommissioning Facilitation Corporation, 2022), fuel debris after retrieval will be kept in a newly built storage facility within the 1F site in specially designed containers (Ono, 2022) although no detailed information is available yet. At the same time, fuel debris management after retrieval plays an important role in the whole decommissioning process (International Atomic Energy Agency, 2021b). It is essential that suitable fuel debris treatment be provided in advance. Reprocessing or conditioning of the fuel debris will be applied before long-term storage or final disposal. Regardless of whether one considers fuel debris a nuclear waste material, it is a non-uniform material with complex chemical and phase composition and does not dissolve easily in nitric acid. It may thus take many efforts to improve reprocessing technologies. The analysis results for 1F fuel debris carry high expectations for progressing the discussion on an optimal solution for the final decommissioning stage.
Besides decommissioning, there is the highly important task of 1F severe accident analysis, such as accident causes and progression investigation (Nuclear Regulation Authority of Japan, 2021). The 1F site is a unique source of information that can be used to better understand severe accident progression in BWRs in general, resulting in improved severe accident models by providing new validation data. However, progression analysis requires investigation of the actual accident site and study of the materials involved. According to the Nuclear Regulation Authority (NRA) annual report, 1F’s current state allows the investigation of facilities and sample collection that are necessary for accident analysis (Nuclear Regulation Authority of Japan, 2021).
It is important to note that although decommissioning and accident analysis activities do not share the same goal, they nevertheless are parallel and continue to use the same initial data from on-site measurements and sample analysis. Thus, the following subjects seem to represent the three main practical goals of R&D activities related to 1F fuel debris:
(1) Fuel debris removal and packaging.
(2) Fuel debris management (temporary storage and preparation for final disposal).
(3) Analysis of 1F accident.
Debris samples analysis is an indispensable and irreplaceable source of information for all three goals. This paper reviews the positive impact of the most helpful previous approaches to sample analysis and the relevant experiences gained during investigations of the damaged Three Mile Island-2 and Chernobyl NPP-4 reactors. The following definitions will be used in the review.
2 Experience of TMI-2 and ChNPP-4 severe accidents: damaged fuel and corium sample analysis
2.1 Three Mile Island NPP Unit 2 accident
The severe nuclear accident at the Three Mile Island NPP Unit 2 (TMI-2) occurred on 28 March 1979. Its cause was a failed valve that was constantly leaking steam out of the primary circuit, which gradually led to the voiding of part of the core and its overheating in the steam-rich atmosphere. As a result of the accident, approximately 62 tons of fuel assemblies were melted and approximately 20 tons were relocated down from the central part of the core and solidified in the reactor pressure vessel (RPV) lower head, which was full of water (Broughton et al., 1989). TMI-2 was not the first nuclear reactor accident with a core meltdown; there had been nuclear accidents with core meltdowns, such as at Chalk River Laboratories, in 1952 (Canada) (Lewis, 1953) and the experimental SL-1 reactor accident in 1961 (United States) (Tardiff, 1962; Mendoza et al., 1981). However, the damaged fuel removal from TMI-2 is one of the most successful decommissioning experiences of a damaged large-scale nuclear reactor. It took a little over 4 years to defuel and clean-up the damaged RPV and transport approximately 130 tons of nuclear waste to the open-air dry storage facility near the Idaho national laboratories (GPU Nuclear, 1990).
At the time of the accident, there were no models and calculations that allowed the prediction of a reactor core failure, and the state of post-accident reactor cores was unknown. From data published in a special issue of Nuclear Technology (Vol. 87, 1989), one can realize that successful decommissioning was achieved through technologies for video observation, radiation measurements, ultrasonic core topography mapping, available industrial drilling technologies, and, in particular, damaged core sample analysis. The first video investigation was performed in 1982 to investigate a damaged core status (Holton, 1990). Visual observations confirmed that a considerable amount of fuel was damaged and that common melting of fuel and core materials had occurred; however, nobody knew the composition of the phases that had formed after the accident. This confirmed the necessity of sampling and investigating damaged material. Sample analysis was performed at all the stages of the decommissioning, starting from 1984—some 5 years after the accident. Analytical methods of fuel debris characterization included chemical and isotope analyses (by gamma and mass spectrometry), optical microscopy to identify the distribution of inclusions, SEM EDS, EPMA, and X-ray and neutron diffraction to identify phase composition.
Before the reactor vessel's upper head was removed during the in situ examination of the core and vessel by video camera, a collection of loose material (grab samples) was performed, and 11 samples (1.37 kg) of loose material were collected. At the time of sampling, the radioactivity of the bulk material was reported as 1 Sv/h. More than 90% of the sample material was related to fragments with dimensions of 1–5 mm. Particles more than 1 mm in diameter were selected for optical metallographic imaging and SEM-EDS analysis, confirming that the upper layers of the solidified corium mostly consisted of molten uranium-zirconium oxide (Akers et al., 1986; McCardell et al., 1990).
The typical analysis flow for grab samples is represented in Figure 1.
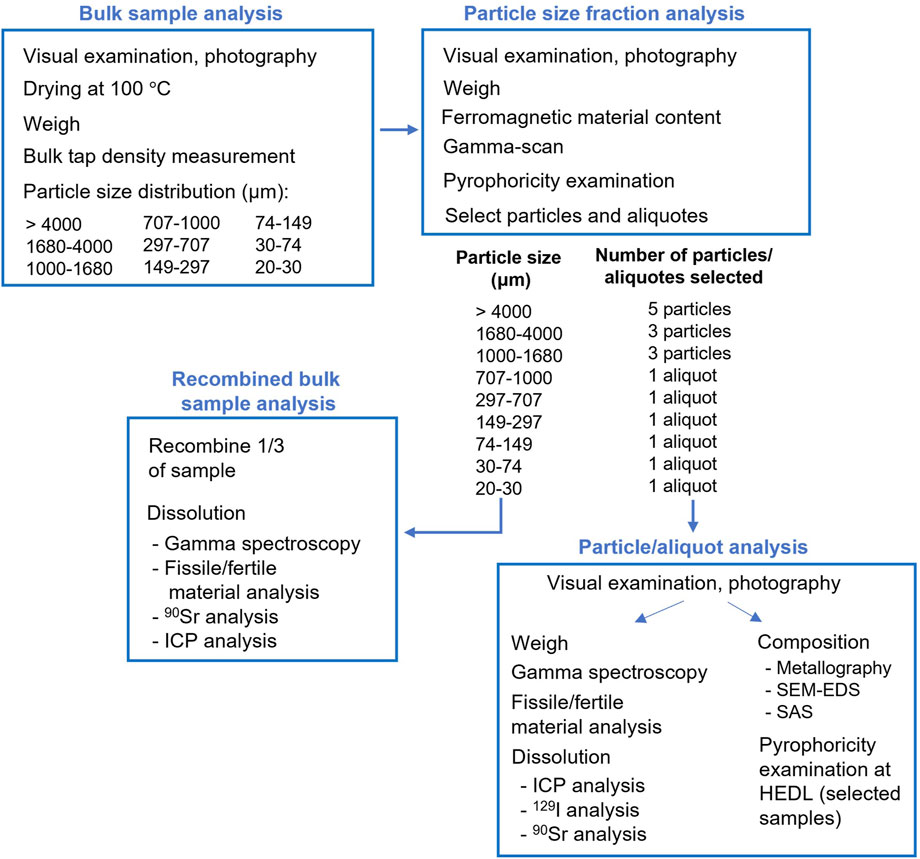
FIGURE 1. Analysis flow for examination of the TMI-2 core debris of grab (loose) samples (simplified by present authors from (Akers et al., 1986)).
After the upper head removal, samples were obtained as a byproduct of the core bore drilling. An examination of cooling-system artifacts was also then performed, including the collection of samples such as debris from the plenum cover, filters from the makeup and letdown system, and control-rod components such as leadscrews and support tubes (Jensen et al., 1987; Tolman et al., 1987). These samples were analyzed using α- and γ-spectroscopy, optical microscopy, and SEM EDS to confirm radionuclide and elemental composition. All collected debris samples were categorized into several groups by the location and method of sampling (such as upper loose debris, solidified debris from the molten corium pool, upper crust and lower crust layers of the molten pool, lower head dense or loose debris, and stump of fuel assembly). Only a combination of systematic sampling and video inspections throughout the decommissioning made it possible to construct a 3D map of the damaged core and clarify the final distribution of the debris.
In parallel with decommissioning activities, plant data analysis was performed in order to investigate the accident progression scenario. It was confirmed that the water level gradually decreased to roughly half of the core level. Phases detected in fuel debris samples allowed conclusions on the maximum temperatures reached by reactor core materials during the accident. The formation of molten uranium-zirconium oxide indicated that parts of the corium pool temperatures had exceeded 2,527 °C (Akers et al., 1986; McCardell et al., 1990). Moreover, microscopic analysis (SEM EDS, EPMA) indicated that temperatures had exceeded 2,827 °C at some points, which was demonstrated by the presence of molten UO2 particles (Bottomley and Coquerelle, 1989). XRD investigations of core bore samples by Brown et al. (1989) allowed for the identification of various oxidic and metallic phases, which provided a basis for reconstructing the accident scenario with the timing of when the materials had melted, relocated, solidified, remelted, or fractured.
Additional separate-effect investigation of the RPV steel microstructure alteration by temperature using specially prepared samples allowed an accurate estimation of the maximum lower head temperature which could have been achieved when the molten corium had relocated. A combination of plant data analysis, video investigation, and separate-effect tests, with the investigation of the corium and the RPV samples and the damaged core mapping efforts, was the most effective for shedding light on the accident progression of the TMI-2 accident and supporting decommissioning and debris management.
2.2 Chernobyl NPP Unit 4 accident
The severe nuclear accident at Unit 4 of the Chernobyl NPP (ChNPP-4) happened on 26 April 1986 (IAEA, 1990). In the report of the USSR State Committee on the Utilization of Atomic Energy and in the IAEA official report, the Chernobyl nuclear accident was classified as a reactivity-induced accident (RIA) caused by a complex of factors: the physical characteristics of the reactor, the specific design features of the control elements (poor design of absorbers and non-optimal channels grid), and the unauthorized state of the reactor (USSR, 1986; IAEA, 1992). The aforementioned reports and publications (Abagyan et al., 1991; Afanas’eva et al., 1994; Fletcher et al., 1988) consider that the accident commenced after activation of the Emergency Protection System button (EPS-5), followed by a fast increase of the core temperature and a dramatic increase of neutron power and reactivity, and culminated with two explosions, the last of which destroyed the reactor (IAEA, 1992). These reports were reticent about the nature of the explosions, which presented the biggest issue of the accident. Evidence on the nature of the explosions was published several years after it. The presence of special gaseous isotopes 133Xe and 133mXe, which never emerge during normal fission of the nuclear fuel, suggests that a supercriticality process took place in at least part of the reactor core and resulted in one of the explosions (Pakhomov et al., 1991).
Right after the accident, severe damage to the reactor and high radiation doses made localization of the damaged fuel difficult. The first map of radioactive sources of the damaged reactor and its neighborhood was created by measuring the γ-dose with a NaI(Tl) detector attached to a helicopter. Fuel debris accumulations were later discovered inside the reactor building in premises below the reactor shaft using radiometers with high-dose-rate limits (up to 30 Sv/h) and heat measurements (Borovoi and Velikhov, 2012). A more detailed distribution of fuel debris was confirmed during borehole drilling investigations (1988–1991), when photograph/video inspections, dose rate, and neutron and heat measurements were performed through horizontal and vertical boreholes. In addition, borehole drilling inside the reactor showed that the reactor shaft was almost empty (Borovoi and Velikhov, 2012). Most samples of fuel-containing material from inside Unit 4 were collected in 1990–1991. At that time, the dose rate of γ-radiation in the premises with fuel agglomerates achieved 4–6 Sv/h; however, on the surface of the Chernobyl “lava”, the dose rate was over 10 Sv/h. Various attempts to implement robotic and remote control techniques for sample collection were unsuccessful because of high background radiation and aggregations of debris on the floor. This is why all corium samples were taken manually using hands and hammers.
Due to the severe destruction of the reactor core, so-called “hot” particles with fuel (UOx) and corium (mainly U-Zr-O solid solutions) composition spread out hundreds of kilometers (Bogatov et al., 1990; Sandalls et al., 1993). Parallel to the sampling inside Unit 4, many samples of fuel-containing particles were collected, mostly within a 30-km zone around ChNPP (Bogatov et al., 1990; Burakov et al., 1994; Shabalev et al., 1997). Analysis of the “hot” particles and fuel-containing materials (corium, products of corium-structural steel material interaction, black and brown “lava”, and pumice), as well as gaseous isotopes released from the core, allowed important conclusions to be drawn about the accident that will be described in the following sections. Despite the relatively small size of “hot” particles (usually 10–100 microns), they represented a broad variety of materials such as UOx, U-Zr-O solid solutions, Fe-Cr-Ni inclusions, and rare particles with silicate or iron oxide matrices (Bogatov et al., 1990; Burakov et al., 1994; Burakov et al., 2003; Shabalev et al., 1997). Some particles had a multiphase structure. Comparison of the chemical and phase composition of the hot particles with experimental results of UO2-Zr interaction (Burakov et al., 1994) and with phase diagrams of Zr(O)-UO2 (Bogatov et al., 1990; Ushakov et al., 1996) showed that the interaction between nuclear fuels and structural zirconium alloys occurred in the core before the explosion. The temperature of this interaction for the majority of “hot” particles with U-Zr-O composition was determined as not less than 1900 °C. However, the composition of some particles (UOx with admixtures of Zr) suggested that temperatures reached at least 2,500–2,600 °C in a part of the core before the explosion. Although many features of the Chernobyl accident such as the materials interaction processes and related temperatures were determined from hot particle analysis, it was difficult to estimate the duration of corium formation and relocation by analyzing particles hundreds of microns in size. In addition, due to the explosion, corium and damaged fuel “hot” particles could not be directly linked to a specific location inside the reactor.
A comprehensive study of “hot” particles with a non-oxidized matrix of U-bearing metallic Zr (Pöml and Burakov, 2017) and the presence of partly molten nuclear fuel (Pöml and Burakov, 2018) confirmed that local UO2-Zr interaction occurred at a very high temperature (>2,850°C) and probably lasted a few microseconds or less. The presence of Zr-U-Fe-O suggested the melting of the spacer grids and was a clue to the relocation of corium melt with fuel, cladding, and steel components (Shiryaev et al., 2018).
Another interesting group of samples represented a class of molten core interaction with concrete. On the tenth day after the explosion (Burakov et al., 1997a), some of the concrete constructions in room #305/2 failed, and “lava” spread throughout the reactor building and solidified. Some lava reached water in the bubbler pond through the steam-discharge pipes and formed a pumice-like material. The collection of such samples inside the destroyed reactor was determined by their accessibility under conditions of extremely high radiation fields (up to 10 Sv/hour). The first analysis of highly radioactive samples collected in premises below the burned-through reactor’s base plate showed that fuel fragments and molten corium reacted with concrete and serpentinite and formed a highly radioactive silicate melt (called silicate-rich corium or “Chernobyl lava”). Three main sources of “lava” were observed during visual inspections of the destroyed reactor and related dose rate measurements (Borovoi et al., 1990; Kiselev et al., 1992; Pazukhin, 1994). EPMA, XRD, Raman spectroscopy, and FIB-assisted TEM of inclusions extracted from silicate matrices of Chernobyl “lava” and “pumice” showed the presence of U-Zr-O solid solutions of various crystalline structures, UOx (relicts of a fuel pellet), metallic inclusions, and uranium-rich artificial zircon (Zr1-xUx)SiO4 (Anderson et al., 1993; Trotabas et al., 1993; Burakov, 2020). Chemical and phase analysis of the “lava” matrix and inclusions combined with the recorded data on radioactive aerosol formation provided insights into the temperature and duration of “lava” formation, then its propagation via the reactor building, and its cooling rate. It was assumed that the main source of “lava” remained in a liquid state at least 1,300°C–1,500°C (in room #305/2) for at least several days (Burakov et al., 1997a). From the presence of dendrite silicate crystals (pyroxene) in samples of the brown “lava” and by comparison with the pyroxene phase diagram, it was assumed that the “lava” temperature reached approximately 1,550°C (Shiryaev et al., 2016). The upper-temperature limit estimation was performed by analysis of zircon crystals from the brown “lava”. It was assumed that the temperature of the silicate melt did not exceed 1,660–1700 °C, or otherwise, the limit of zircon’s thermal stability could be exceeded. By performing a similar laboratory synthesis of zircon crystals, it was concluded that the formation of zircon crystals with several hundreds of microns in size (similar to that found in Chernobyl “lava”) could take approximately 3 days.
Samples from the main source of corium (former underreactor room #305/2) could not be studied for a long time because of their high radioactivity caused by high 106Ru concentration in metallic inclusions (Trotabas et al., 1993; Burakov et al., 2003). Analysis in 2019–2020 showed that samples collected in room 305/2 were formed during molten corium interaction with structural steel material. The study of these samples allowed the specification of the materials involved in corium formation in the early stage of the accident, such as fuel pellets, Zr-based alloy from the channels in the graphite core, and Ni-Cr steel from the bottom of the guide rod (Shiryaev et al., 2022). It also supported previous assumptions that partial cladding degradation and local corium relocation could occur prior to the explosion (Shiryaev et al., 2018).
Over time, the aging and self-destruction of fuel-containing accumulates has become another problem. The partial mechanical and chemical degradation of Chernobyl “lava” with the formation of secondary uranyl phases on surfaces was observed 4 years after the accident (Burakov et al., 1997b). Samples kept in the laboratory under open-air room temperature conditions exhibited the same behavior (Zubekhina and Burakov, 2017). Due to the aforementioned effects, a number of leaching and alteration experiments with Chernobyl corium and “lava” samples at temperatures from room to 150 °C were performed to gain knowledge about fuel debris aging (Rogozin et al., 1991; Zubekhina and Burakov, 2017; Zubekhina et al., 2019; Zubekhina et al., 2021; Shiryaev et al., 2022). This allowed an estimation of the leaching rates of actinides and fission products and of the influence of physical and chemical parameters on sample degradation and established a basis for studying secondary phase formation.
An overview of recent studies (Shiryaev et al., 2016; Pöml and Burakov, 2017; Zubekhina and Burakov, 2017; Pöml and Burakov, 2018; Shiryaev et al., 2018; Zubekhina et al., 2019; Shiryaev et al., 2020; Zubekhina et al., 2021; Shiryaev et al., 2022; Lönartz et al., 2023) shows that, even after more than 30 years of investigation, Chernobyl sample analysis could contribute additional information about accident progression, conditions of corium and “lava” formation, and the processes of their chemical alteration due to aging. Since the damaged fuel was not removed from the destroyed reactor unit, evaluation of the usefulness of sample analysis results for the fuel debris removal process is not possible. However, chemical alteration and change in mechanical properties during the aging of Chernobyl corium over 35 years can also be considered important reference data for the decommissioning of 1F, where fuel debris still remains inside the reactors.
3 Up-to-date situation with 1F post-accident investigation and sample analysis
More than 35 years have passed since the TMI-2 and ChNPP-4 accidents, and advanced materials and techniques have become available for remote work under high radiation fields. At 1F, remote-controlled investigations of the reactor buildings and containments in three severely damaged units were conducted to achieve an overall impression of the damaged status inside the PCV and RPV, including possible debris locations. Numerous types of remotely operated vehicles (ROVs) equipped with high-resolution cameras and gamma-detectors have been successfully tested and utilized in 1F post-accident investigations (International Research Institute for Nuclear Decommissioning, 2022). During these activities, ROVs were contaminated by radioactive materials through contacting various objects and water in the PCV environment near fuel debris. This made it possible to organize indirect sampling by taking smears from contaminated ROVs and extracting U-bearing particles from smears. Such techniques do not allow identification of the exact sampling place but do provide general information on the presence of some particular U-bearing phases in the reactor building. Moreover, a detailed analysis of the U-bearing particles obtained important data for international collaboration on accident progression analysis (Barrachin et al., 2022). In this section, we describe the methods and strategies of 1F sample analysis and briefly summarize the results and the possible utilization of analytical data for the accident progression investigation and decommissioning process.
3.1 Currently available methods and strategies for 1F reactor internal investigation, sample collection, and analysis
As previously discussed on severe accidents, accessibility was an important issue for determining the 1F sample collection progress. High dose rates restricted access to the fuel debris in the reactor buildings, and thus, until 2015, much of the work was performed outside the units. Some data about the situation inside the units were obtained by analyzing instrumentation measurements (e.g., cooling water level, RPV pressure, radiation background) recorded during the accident (fdada.info, 2003). This information was used as a starting point for the first attempts to explain the progression of the 1F severe accident by using already existing models. Numerous samples of contaminated water, soil, and aerosols have also been collected at the 1F site, within the Fukushima area, and in other prefectures to evaluate the level of radioactive contamination. However, the absence of actual samples obtained from the fuel debris made it difficult to confirm any details about the accident progression and, consequently, the damage to PCV and RPV. Information about materials formed inside the units was, for several years after the accident, limited by radioactive microparticles collected outside the 1F site which seem to have originated from the destroyed reactors (Adachi et al., 2013; Abe et al., 2014).
In 2015, the first direct internal PCV video investigation was carried out in Unit 1 (Tokyo Electric Power Company Holdings, Inc, 2015). In 2017, the first samples of sediments from the PCV bottom of Unit 1 were collected. Between 2017 and 2022, samples (smears and small amounts of sediment) from Units 1 to 3 PCVs were regularly taken from different premises, such as the operations floor, PCV walls and bottom, and torus room, for further analysis. Primary gamma-spectroscopy and XRF analysis of some samples were performed immediately on the 1F site. Samples were then transported to Japan Atomic Energy Agency (JAEA) and Nippon Nuclear Fuel Development Co. Ltd. (NFD) for comprehensive analysis of chemical-, phase-, and radio-isotope composition using the scheme depicted in Figure 2 (Tokyo Electric Power Company Holdings, Inc, 2018). Testing of the equipment and techniques for the on-site analysis, such as a portable α-particle imaging detector combined with a Cd-Zn-Te-based γ-spectrometer, also revealed the possibility of measuring Pu and Am isotope content in smear samples (Morishita et al., 2019). These measurements performed on the 1F site agreed well with the results obtained on the same samples in a JAEA laboratory using more precise radiochemical analysis (Sato et al., 2016).
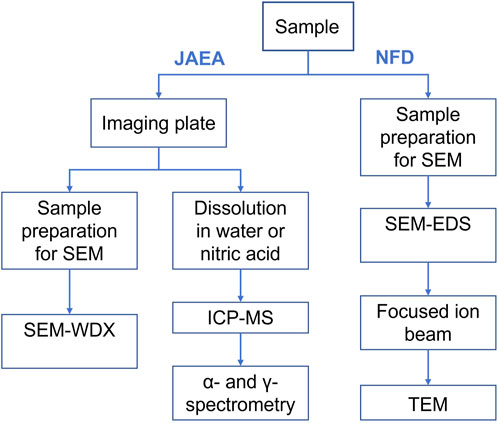
FIGURE 2. Typical scheme of 1F sample analysis in 2017–2022 (Tokyo Electric Power Company Holdings, Inc, 2018).
3.2 Outline of the investigation of damaged units and currently available 1F sample analysis results
According to the current situation, all samples related to the accident at the 1F can be classified by location, nuclide composition, aggregate state, or sample source. Among the variety of materials formed at 1F, fuel-containing samples can be considered a basic source of information for both accident analysis and debris removal. This review summarizes previously published analysis results with a particular focus on the U-containing samples, most of which were published as reports and presentations in Japanese and in the following reviews: Grambow et al. (2021), Barrachin et al. (2022), and Kurata et al. (2022). Based on the data obtained from the various investigations of the three severely damaged units, TEPCO and JAEA collaboratively developed and kept updating a core status map that reflects up-to-date hypothesis on fuel debris distribution (fdada.info, 2023). This and additional information can be found on the information portal website fdada.info, where the data are systematically organized by type of data (document, video, image, etc.), year of publication, 1F Unit, and so forth. In this paper, samples obtained from 1F Units 1 to 3 will be reviewed individually and from samples collected outside the reactor buildings, where their origin cannot be determined.
3.2.1 U-bearing particles collected outside 1F
During the progress of the 1F accident, various kinds of U-bearing particles were widely distributed around the plant—for instance, 50 km west (Kurihara et al., 2020), and several particles were captured 170 km southwest of 1F (Abe et al., 2014). A number of these were collected and analyzed. The particles had different uranium isotope ratios and a variety of phase compositions which may indicate different formation mechanisms (Abe et al., 2014; Furuki et al., 2017; Imoto et al., 2017; Ochiai et al., 2018; Kurihara et al., 2020). One type of particle was represented by Fe-oxide nanoparticles with U content up to 1 wt% incorporated into the SiO2 glass matrix (Furuki et al., 2017; Imoto et al., 2017). Another type contained U as inclusions with fuel (UO2+X nanocrystals of approximately 70 nm size, incorporated into magnetite) and corium matrix ((U,Zr)O2+X nanocrystals of approximately 200 nm size, with U/(U + Zr) molar ratio range of 0.14–0.91) (Ochiai et al., 2018).
The results obtained from the study of U-bearing particles showed that the reactor damage was serious enough that aerosols with nanofragments of fuel pellets and corium were ejected out of PCV. The U/Zr ratio in U-Zr-O-bearing particles varied in a broad range and was comparable with those of TMI-2 and ChNPP-4 corium samples. However, suggestions regarding accident redox conditions based on these studies were rather controversial. It was assumed by Furuki et al. (2017) and Imoto et al. (2017) that at least a part of the fuel experienced oxidizing conditions during melting, whereas Ochiai et al. (2018) claimed that the conditions of fuel melting were reducing rather than oxidizing. It can be assumed that these studies dealt with particles ejected from different units, but once they were ejected, it became difficult to attribute them to a particular unit.
3.2.2 Investigation and sample analysis for Unit 1
The situation with debris distribution inside the RPV of Unit 1 is still unclear. By means of muon tomography measurements of Unit 1, the lower head position appeared transparent on the image; thus, high-density materials (fuel debris) were not identified in the reactor core (International Research Institute for Nuclear Decommissioning, 2015; Yoshizawa, 2017).
Four investigations were conducted on the inside of PCV of Unit 1: in 2015, 2017, 2022, and 2023. The robot successfully accessed the X-100B penetration to the inside of the drywell (D/W) of PCV in 2015 and 2017. By means of a small camera and a dosimeter attached to the ROV, the first-floor metallic grating and a lower area of D/W were mainly investigated. The D/W bottom was covered by water, and the camera detected accumulates at the bottom (at an estimated distance of 30–50 cm above the original D/W floor). The surface of the accumulates was covered by sludge-like material. Further neutron flux and γ-spectrometry measurements confirmed increasing γ-dose and neutron flux near the surface of the deposits. The dose rate rose to almost the same level of 8–10 Sv/h when approaching the bottom of the PCV and a metallic grating surface at different positions (Yoshizawa, 2017).
In the most recent investigation in 2023, the height of accumulates spread through the D/W region was thoroughly measured at various points (Tokyo Electric Power Company Holdings, Inc, 2023a; International Research Institute for Nuclear Decommissioning and Tokyo Electric Power Company Holdings, Inc, 2023). The investigation showed that the layer of sludge-like material on the surface of the accumulates was rather thin (likely a few cm or less). The accumulates lying on the D/W floor ranged from 0.3 to 1.0 m high. The thermal neutron flux measurements performed above the accumulates suggested that those accumulates could contain fuel debris or other U-bearing materials. In this investigation, the inside of the RPV pedestal was first observed by ROVs entering its opening. A shelf-like structure was observed around the opening of the pedestal and the inside of the RPV pedestal, stuck to the concrete side wall. The shelf-like structure was approximately 1.0–1.3 m above the original concrete floor. The thermal neutron flux just above the shelf-like structure was almost at the same level as that above the accumulates in the D/W, suggesting that the shelf-like structure also contained fuel debris. The most surprising result observed in the 2023 investigation was the loss of concrete material from the side wall of the pedestal, although rebar and the so-called “inner skirt” remained (“inner skirt” is a massive round metallic structure holding the hoop stress of the pedestal concrete). Just below the shelf-like structure, approximately a few tens of cm width of the concrete layer was discolored to black, and then, below the discolored layer, the concrete material was absent but the rebar remained. The height of the absent concrete is approximately 1.0 m from the original floor level. The loss of concrete material is observed all around the inside wall of the RPV pedestal and in a part of the outside wall near the pedestal opening. Various kinds of material were accumulated on the bottom floor in the RPV pedestal. Some were identified as a part of the housings of the control rod driving system (CRD) and other degraded steel equipment originally located in the RPV pedestal. A sample of this material was taken in the recent investigation and its analysis was to start in 2023 (Tokyo Electric Power Company Holdings, Inc, 2023b).
The first radioactive samples in the form of suspension were obtained from the surface of these accumulates at the bottom of the D/W by water suction near the sediment surface (Tokyo Electric Power Company Holdings, Inc, 2015; Tokyo Electric Power Company Holdings, Inc, 2018). Gamma-spectrometry and XRF analysis within the 1F site confirmed the presence of U as a sign of degraded fuel, fission products (134Cs, 137Cs, and 125Sb), and 60Co resulting from iron activation. SEM-EDS analysis showed that some U-containing particles were mixed with spinel Fe3O4 and (Fe,Cr)3O4. According to TEM analysis, these particles contained U-rich cubic U-Zr solid solution (U,Zr)O2, and some samples contained Zr-rich tetragonal phase (Zr,U)O2 (Tokyo Electric Power Company Holdings, Inc, 2018). After non-destructive analysis, an attempt was made to dissolve another part of the same sample in two solutions—in deionized water and nitric acid—but significant amounts of non-soluble residue remained even after immersion in nitric acid. Alpha and gamma spectrometry of these solutions detected actinides (Pu, Am, and Cm) at comparable amounts. However, U concentration measured by ICP-MS showed good solubility in nitric acid solution (1.1 μg/sample) but not in water (10–3 μg/sample). In the original treatment, Cs was mainly extracted by water and acidic solution, but some 137Cs also remained in the undissolved portion. Intensive Cs leaching from the fuel debris is supported by analysis of water samples taken from the turbine building in March 2011 and from PCV in October 2012 showing high concentrations of 137Cs, 1.6 × 108 Bq/L (Grambow et al., 2021) and 3.5 × 107 Bq/L (Fukaya et al., 2017), respectively. However, taking Cs volatility into account, it could also be desorbed from structural materials or trapped from the aerosols.
Analysis of samples taken from the outside of the PCV from the operation floor well plug (Tokyo Electric Power Company Holdings, Inc, 2020) also confirmed that some U-containing materials in Unit 1 spread out of the PCV. Various types of U-bearing particles were detected for the operating floor samples. Monoclinic ZrO2, cubic UO2 with some admixtures of Zr, Fe, and Cr, cubic (U,Zr)O2, and unusual cubic (UFe)O2 phase with Fe content up to 20% and without traces of Zr and Cr were found by TEM and SEM-EDS analyses. Both UO2 and (U,Zr)O2 were surrounded by Fe3O4. The ratio of U/Zr in (U,Zr)O2 varied from 6/94 to 93/7 within one particle.
3.2.3 Investigation and sample analysis for Unit 2
Although the image resolution of Unit 2 did not permit precise conclusions, 4 months of muon tomography measurements showed that there might be some dense material at the bottom of the RPV (International Research Institute for Nuclear Decommissioning, 2016). It was thus assumed that much more fuel debris than in Unit 1 may have remained in the RPV (Nuclear Damage Compensation and Decommissioning Facilitation Corporation, 2022). Regarding the internal investigation, since the radiation dose around the X-6 penetration was relatively low, direct access to the inside of the RPV pedestal was planned. At the beginning of 2017, IRID performed the first investigation using a pan-tilt camera attached to the head of a long pole (Kurata et al., 2022). A large hole was observed in the grating of a work platform, which was located in the center of the RPV pedestal. In the second investigation in 2018, observed images showed a few pieces of a broken fuel assembly top tie plate among the scattered rubble on the concrete floor. In addition, the accumulation of pebble-like debris including sediment was widely spread over the concrete floor. The height of the accumulates was less than approximately 1 m (the highest position is just beneath the large hole). These observations suggested that a significant amount of molten corium had passed through the hole in the RPV bottom head. Furthermore, small amounts of deposits were detected adhering to the CRD housing attached to the RPV lower head. This could suggest that a small failure also existed at the RPV lower head (Kurata et al., 2022).
During the Unit 2 investigations, samples were taken directly from two locations inside the PCV (deposits on traversing in-core probes (TIP) tube and materials accumulated in the X6 penetration) (Tokyo Electric Power Company Holdings, Inc, 2015; Tokyo Electric Power Company Holdings, Inc, 2018; Tokyo Electric Power Company Holdings, Inc., 2019a). Smear samples from the investigation camera were also analyzed. Since the camera did not contact the materials inside the RPV pedestal region but was exposed to cooling water continuously dropping from the RPV bottom, the smeared sample could have originated from fuel debris remaining in the RPV. In these samples, U-bearing and U-Zr-bearing particles were found by SEM WDS, ICP-MS, and other microscopic analyses (Tokyo Electric Power Company Holdings, Inc., 2019a). This showed that, in some particles, U coexists with Pu. TEM analysis of the smears confirmed that U-Zr-O particles with a relatively uniform structure and average composition were considered to be a tetragonal phase of (Zr0.64, U0.36)O2. This analytical result is comparable with the chemical composition of some Chernobyl corium “hot” particles, such as Zr0.68–0.71U0.32–0.29)O2.00 or (Zr0.75–0.77U0.25–0.23)O2.00 (Burakov, 2020). This may support the origin of this sample of Unit 2 being molten corium.
Samples collected from the outside of the PCV provided additional information about the composition of U-containing materials in Unit 2. Analysis of smears taken from the operations floor showed U,Zr-bearing particles with a body-centered cubic structure with various Zr contents. Cubic phases of (U,Zr)O2 containing 6–7 at% Fe + Cr and Fe3O4 grains with FeCr2O4 precipitates were also confirmed by TEM EDS (Tokyo Electric Power Company Holdings, Inc, 2020).
Small, 0.5–3.0 μm particles containing Zr, U, and other actinides were found in sediments of stagnant water accumulated in the torus room (Yomogida et al., 2022). Analysis of this water revealed that U was mostly related to particles larger than 10 µm. After filtering through a 0.02-µm filter, the total U concentration in the water became about three orders of magnitude lower. Am and Cm were detected on the surface of Fe-oxide particles. According to SEM EDS analysis, U-Zr-containing particles were attached to larger particles containing Fe (probably iron oxide). The isotope ratio of U in the analyzed material was consistent in the original fuel as the average value, which allowed the sediments from the torus room to be considered fuel-containing materials.
3.2.4 Investigation and sample analysis of Unit 3
Muon tomography measurements did not confirm a massive corium presence in a core region of Unit 3. However, it was suggested that some amount of dense materials still remained at the bottom of RPV (International Research Institute for Nuclear Decommissioning, 2017). Water levels in the PCV of Unit 3 remained higher than for units 1 and 2. For this reason, a submarine type of ROV was utilized to investigate the inside of the RPV pedestal. Investigation showed that a significant amount of fuel debris accumulated in the RPV pedestal. The thickness reached approximately 3 m maximum from the original concrete floor level. The apparent volume of the accumulate was a few times larger than the theoretical volume of the original core materials, suggesting that the fuel debris is likely to be porous or to have many internal vacancies or holes. Several segments of severely damaged structural steel materials were observed to have penetrated into the debris body. In addition, a small mountain-like accumulation was observed in the center-top region of the debris pile, suggesting that the fuel debris may have been rather viscous during relocation (Kurata et al., 2022).
The first samples from inside the RPV pedestal were collected by taking smears from the submarine ROVs (Tokyo Electric Power Company Holdings, Inc, 2018). Several tens of U,Zr-bearing particles with Zr content from 2 to 12 mol% were identified by SEM EDS and SEM WDS analysis of the smears. A TEM analysis of the particles showed a cubic UO2 phase (with admixtures of Fe, Cr, and Zr), a Zr-rich tetragonal (Zr,Fe,U)O2, and an α-Zr and a (Fe,Cr)3O4 phase (Tokyo Electric Power Company Holdings, Inc, 2020; Kurata et al., 2022). The unique multiphase composition of some particles taken from Unit 3 differed from those obtained from units 1 and 2 (Kurata et al., 2022). Particles containing 238Pu and 241Am were found in a smear taken from the floor of the reactor buildings in units 2 and 3 (Morishita et al., 2019).
In the torus room of Unit 3, a sample was taken at the water surface level. The total α-activity was 1.5 × 103 Bq/L: about two orders of magnitude lower than the sample taken near the bottom of the torus room of Unit 2 (2.6 × 105 Bq/L). However, after filtering through a 0.1-μm filter, the difference in total α-activity of filtered solutions from units 3 and 2 became 8.5 times lower (9.5 × 102 vs. 1.2 × 102 Bq/L, respectively) (Tokyo Electric Power Company Holdings, Inc, 2020). Such a result is evidence that a major part of α-activity in the stagnant water of Unit 3 was suspended throughout the water and that the particles were insufficiently large to be settled by gravity. After analyzing the solid phase collected from the filter, U, Pu, and Zr were found to have been adsorbed to the surface of organic-based particles.
A distinct feature of Unit 3 was that 6% of the fuel assemblies contained a mixed uranium-plutonium oxide (MOX) fuel. Additional production of 241Pu from the 239Pu could be the reason for the relatively high 241Pu/239+240Pu activity ratio (108 for 1F and 83 for ChNPP4, respectively) in environmental samples collected outside the 1F site (Zheng et al., 2012). However, it was not possible from Unit 3 sample analysis to estimate the contribution of higher bulk Pu content to the chemical and phase composition of Unit 3 fuel debris.
3.3 1F fuel debris aging
A chemical alteration and mechanical self-destruction of fuel debris under environmental and radiological impacts over time (commonly called “aging”) may have significantly changed the properties of the debris and affected the decommissioning process. In the past, the biggest concern has been changes in debris mechanical properties and the formation of new chemically unstable phases observed in ChNPP-4 (Burakov, 2020; Zubekhina et al., 2021). In the 1F fuel debris, the gradual change of properties could become significant over the years, so analysis will be necessary. However, during such analysis, some important factors should be taken into account. The surrounding environment is a key factor because fuel debris, degraded structure materials, and surrounding water have affected each other. The ionic composition of water may largely define the types of secondary phase formation (Gurzhiy et al., 2023). Samples taken from the surfaces of the accumulated materials that were immersed in water for more than 10 years may have already experienced significant chemical alteration. This could change the chemical and phase composition from the initial state and from the bulk debris composition. On the other hand, strong irradiation from the fuel debris can also affect the interfacing water chemistry. Water radiolysis products locally decrease the pH of the system and create oxidizing conditions in the vicinity of the fuel debris interface with water that speeds up the fuel debris chemical alteration and increases its corrosion rate (Sunder et al., 1997; Sattonnay et al., 2001). For an evaluation of fuel debris alteration and the further evolution of its properties, simultaneous analysis of solid samples and the ionic composition of water from the same location would complement each other.
Information about the 1F fuel debris aging in the damaged units is not yet available. Several attempts to investigate the alteration of the fuel debris were performed indirectly by studying the chemical and radionuclide composition of the debris cooling water after it was pumped out of the PCV and the stagnant water accumulated in the torus room and turbine building (Nishihara et al., 2015; Fukaya et al., 2017; Koma and Murakami, 2019; Grambow et al., 2021). For example, 2 months after the accident, 137Cs concentration in the turbine building water accumulated in Unit 1 increased 30 times, which may be a sign of the dissolution of some 137Cs-containing materials. For comparison, radioactive isotopes that had penetrated Unit 4 from Unit 3 showed similar behavior for Unit 4 (increase of 137Cs concentration by two orders of magnitude) (Nishihara et al., 2015).
However, water analysis data alone do not permit a reasonable suggestion about the current state of fuel debris or making a prognosis decades ahead. Processes of phase degradation and the formation of new phases strongly depend on the conditions in the corium–water interface, such as redox conditions, temperature, and water chemistry. It should be noted that, even after the continuous replacement of cooling water for many years, water composition still largely varies in different sampling locations. For example, Grambow et al. (2021) mentioned that the samples collected from the torus room of Unit 2 in 2020 had a Cl− concentration equivalent to that in the initial sea water when measured near the floor and 1.5 times lower at 1 m from the bottom. These concentrations are 70–100 times higher than for standard tap water (200 ppm of Cl−), meaning that dilution of the initial seawater with fresh water might not have happened in some areas in the reactor buildings.
Selected results of the radiochemical analysis of water samples for Unit 2 are summarized in Table 1, including the water sample from PCV inside the turbine building, and the torus room stagnant water. The pH of stagnant water is close to neutral, so a formation of actinide-bearing colloidal particles should be expected, as in the case of a typical spent fuel–water interaction (Finn et al., 1994; Zhao and Steward, 1997; Romanchuk et al., 2020).
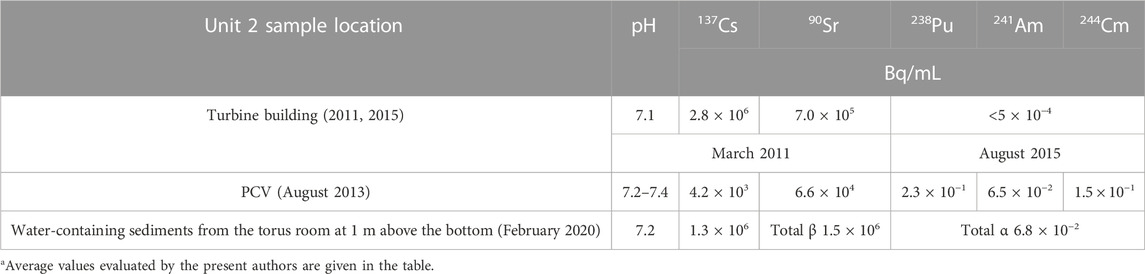
TABLE 1. Selected results of the radiochemical analysis of Unit 2 water samples (Fukaya et al., 2017; Koma and Murakami, 2019; Grambow et al., 2021).
Study of the aging behavior of fuel debris may play a substantial role in the decommissioning. Changing debris properties due to self-irradiation or chemical degradation in water can strongly affect the process of debris recovery. For example, immediately after the accident, the hardness of Chernobyl “lava” and molten steel was so high that it was challenging to collect samples. However, 10 years later, a significant local decrease in hardness (and even mechanical self-destruction) was observed for these materials (Burakov, 2020). This might be caused by processes of local crystallization of the glass-like matrix of the “lava” and irradiation hardening and embrittlement of metal previously reported for nuclear waste glass (Weber W.J. et al., 1997) and structural steels (Xiao, 2019).
3.4 Similarities between fuel-containing samples of TMI-2, ChNPP-4, and 1F; some aspects of the TMI-2 and ChNPP-4 experience important for 1F decommissioning and accident analysis
Despite the different reactor types and accident scenarios (TMI-2, ChNPP-4, and 1F), the basic processes of high-temperature fuel interactions with structural materials in these severe accidents should be similar. Considering the composition of TMI-2, ChNPP-4, and 1F fuel-containing materials, one can divide the fuel debris into damaged UO2 fuel pellets (UO2, UO2+x, molten UO2) and U-Zr-O solid solutions of a different crystalline structure originating from molten corium. Table 2 demonstrates that all main phases of U-Zr-O solid solutions (cubic, tetragonal, and monoclinic) were found in samples of fuel debris in TMI-2 and ChNPP-4, and in 1F U-bearing samples. In some U-bearing microparticles and PCV samples, the UOx and U-Zr-O phases were found to be surrounded by a magnetite phase (Ochiai et al., 2018; Tokyo Electric Power Company Holdings, Inc, 2020) similar to the composition of corium–metal interaction products in ChNPP-4 (Shiryaev et al., 2022) and TMI-2 core bore samples (Bottomley and Coquerelle, 1989).
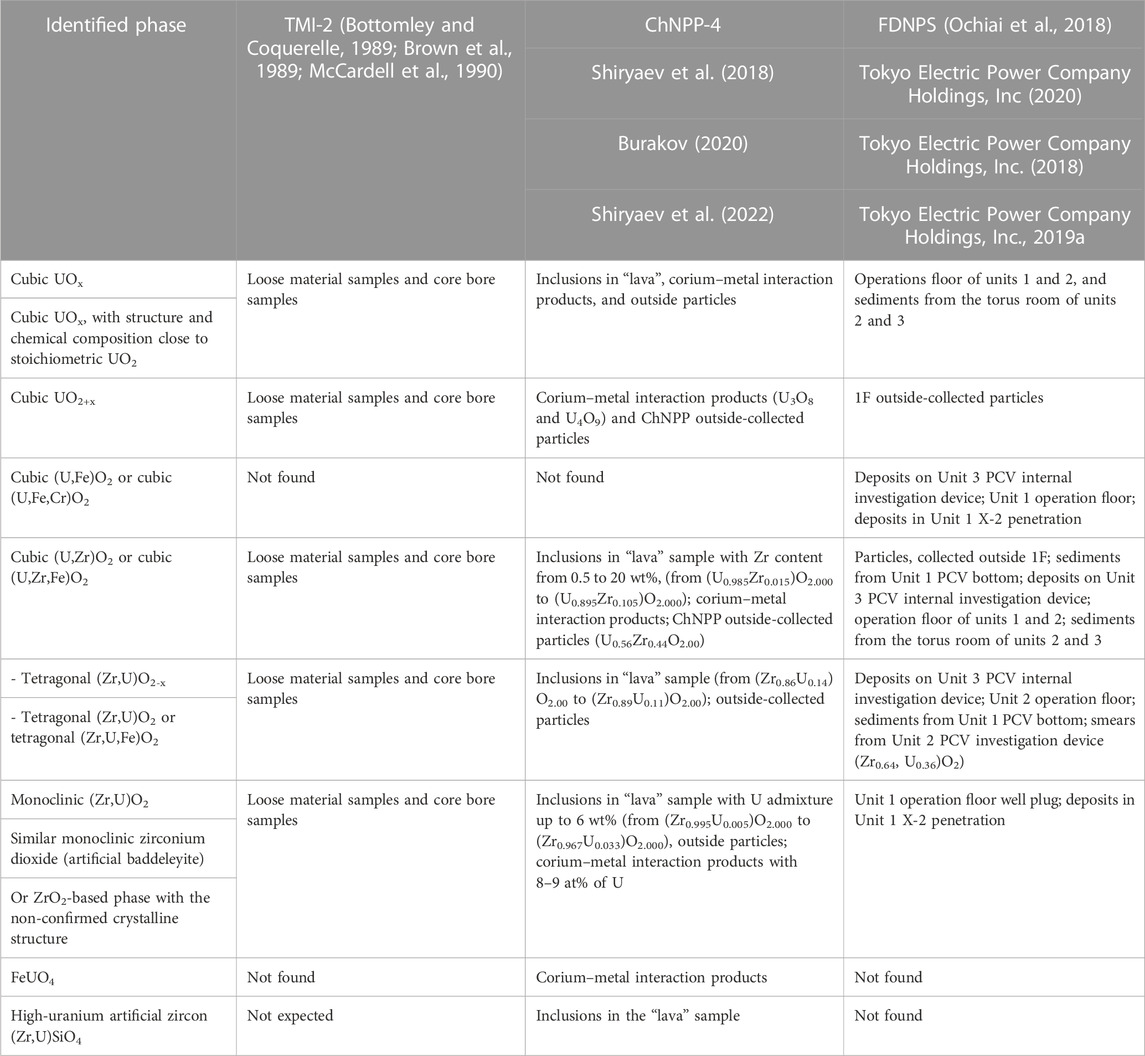
TABLE 2. U-Zr-O-, U-Zr-Fe-O-, and U-Fe-O-containing materials confirmed in TMI-2, ChNPP-4, and 1F samples.
In the case of TMI-2, the mechanical, physical, and chemical analyses of fuel debris provided the initial information for the development of the debris removal equipment, packaging procedures, and the safety case for the debris storage. For ChNPP-4, similar information was also obtained, even though fuel debris removal had not commenced.
In the accident analysis of both TMI-2 and ChNPP-4, the accident progression investigation was based mostly on the results of photograph and video internal reactor inspections and sample analysis. In the case of 1F, the results of sample analysis and on-site investigations are complemented by the results of laboratory tests, numerical modeling, and calculations to better understand the 1F accident progression.
The availability of TMI-2 and ChNPP-4 samples for domestic and international research collaborations (Duco et al., 1989; Akers et al., 1992; Trotabas et al., 1993; Shiryaev et al., 2016; Pöml and Burakov, 2017) was the key factor which allowed the involvement of new competencies from different research groups and increased the quality and impartiality of scientific discussion. Ongoing international collaboration in the OECD/NEA FACE project will be the model for future collaboration on 1F sample analysis (Barrachin et al., 2022). Samples collected at the 1F site will be unique materials for future investigation, and it is essential to keep them available for research and properly manage all related information (sample parameters, location and method of sampling, date, chemical environment, and results of preliminary analysis).
The experience and lessons learned from the TMI-2 and Chernobyl accidents were carefully summarized and included in the IAEA coordinated research project “Management of Severely Damaged Spent Fuel and Corium” and the related TECDOC soon to be published (International Atomic Energy Agency, 2023). Such valuable experience might be helpful during 1F decommissioning.
3.5 Suggestions on methods and strategies of sample analysis and data treatment for upcoming 1F fuel debris
According to the current sample analysis strategy described in the Roadmap, TEPCO is planning to perform gram-scale debris removal from the pedestal region of Unit 2 by using a remotely operated arm. Within several years, approximately ten times more debris will be removed from the pedestal region of Unit 2 by using an improved remote arm (Tokyo Electric Power Company Holdings, Inc., 2019b). The Technical Strategic Plan 2022 for 1F Decommissioning provided by NDF (Nuclear Damage Compensation and Decommissioning Facilitation Corporation, 2022) considers a few possible sampling locations inside the PCV, such as above the grating and from the PCV bottom. The expected form of the first debris is a mixture of particles and sludge.
Analysis of actual debris samples is the basic source of information for planning the decommissioning activities as well as for accident progression investigation. However, methods of sampling and analysis can differ depending on the purpose. In terms of decommissioning, sampling and sample analysis classify the fuel debris by the properties essential for further retrieval, packaging, and storage. Analytical data obtained from the debris samples must satisfy the design requirements from the decommissioning steps summarized in Table 3. Part of the listed properties can be obtained from the first fuel debris analysis. Although the results obtained from particle and sludge samples cannot represent the bulk properties of fuel debris, it is an important step toward the routine analysis of fuel debris samples. The overall concept of future debris analysis was summarized by the Japan Atomic Energy Agency (2020), which listed the available analytical methods and expected analytical results necessary to identify and quantify the various properties of fuel debris. By taking the step-by-step progress of the debris retrieval into consideration, a more detailed strategy for debris analysis should be discussed. A comprehensive understanding of the damage status of the PCV interior by using the analytical results of fuel debris as well as visual data, plant data, and severe accident analysis will be the only way to obtain the knowledge base for determining the industrial-scale debris retrieval method and further packaging.
The preliminary discussion on the strategy for the final stages of fuel debris management has just started in Japan. As an important knowledge base, classification by radionuclide composition in fuel debris and other types of wastes is crucial, especially when contaminated with long-lived actinide (Koma and Murakami, 2019). According to Japan's geological disposal concept (Japan Atomic Energy Agency, 2000), JAEA suggests that 38 isotopes be measured in their final waste form. However, the final waste acceptance criteria for deep geological repository in Japan may include numerous parameters, such as thermal and radiation stability, chemical durability, and heat and gas generation (International Atomic Energy Agency, 1990).
Techniques of sample preparation and analysis may need modification by taking the specific features of the sample into consideration. Figure 3 shows our view of a possible sample preparation sequence and analysis flow for the 1F fuel debris samples.
Proper data management is essential to providing debris analytical data. The following basic principles for analysis should always be taken into consideration for quality identification.
1. Share the definition of technical terms and what exact data are obtained from the debris analysis.
2. Identify the gap between the requirements from the decommissioning process design and the available results from the debris sample analysis, and then consider how one should progress the analysis and sample preparation methods.
3. Devise analytical methods and flows based on the features of the retrieved debris samples.
4. Carefully progress qualitative and quantitative analyses.
5. Further utilize the valuable analytical data on fuel debris samples.
Regarding the first issue, the conceptual implications of “representativeness” vary considerably even among experts. Regarding the second issue, there is a gap between the requirements of decommissioning and the debris analysis data. However, by involving various experts and stakeholders in 1F-decommissioning, analytical data can be utilized more effectively. Regarding the third issue, specific features of the samples (small particles, sludge, and chemically altered samples) may require relevant modification of analytical methods and analysis flow. Regarding the fourth issue, the fuel debris samples could be a considerable multi-element system with a very wide range of chemical composition and concentration. There are no standard samples or standard measurement conditions for such materials. Hence, the availability of each analysis method for unknown materials should be confirmed. Regarding the fifth issue, given the management and systematic treatment of the increasing amount of information, it is useful to continue developing a database, such as Debriwiki (fdada.info, 2003), with all information such as the sampling unit, time, and place of each sample. It is also important to have this data publicly available. In the future, such information would allow the reconstruction of 3D maps of the 1F damaged core. It would be helpful to retain a collection of representative samples of the 1F fuel debris for further research.
Another important issue for the first step of sample analysis is effective collaboration amongst institutions. Several organizations are involved in the 1F sample analysis (TEPCO, NFD, and JAEA), and NDF recognizes the necessity of their close cooperation with each other (NDF, 2022). The project on benchmark analysis of simulated debris is such an example of successful cooperation between Japanese institutions (Koyama et al., 2021). In addition, the importance of international and inter-institutional collaboration was emphasized by the IAEA experts who urged the Fukushima Daiichi Decontamination & Decommissioning Engineering Company (FDEC) to facilitate access to fuel debris samples (IAEA, 2021a).
4 Discussion
While the results of the 1F sample analysis remain limited, they are critically important for both understanding the situation inside the units and future decommissioning. Several general facts emerge from the available data. The presence of U-Zr-O solid solutions and individual actinides (U, Pu, Am, and Cm) in the particles collected from the inside of IF Units 1 to 3 clearly confirms that molten corium formation occurred in the RPV of these units, and then, corium spread from there down to the PCV in these three units. Uranium leaching by nitric acid solution from the samples of PCV sediments is evidence that uranium in this material is related not only to U-Zr-O solid solutions, which are hardly soluble in nitric acid but are also present in less stable chemical forms. Actinides (Pu, Am, and Cm) found in PCV of units 2 and 3 might be in the form of colloids adsorbed into the sediment particles.
Despite their different reactor types and accident scenarios, the experience of the TMI-2 and ChNPP-4 nuclear accidents can be useful for 1F decommissioning. The formation of U-Zr-O and U-Zr-Fe-O solid solutions similar to TMI-2 and ChNPP-4 crystal structure and U/Zr ratio corium was confirmed in 1F samples (Table 2). TMI-2 corium samples and Chernobyl “hot” particles could be seen as prototypes for 1F fuel debris analysis. Chernobyl “lava” remains the only real prototype of the products of corium–concrete interaction.
Regarding the 1F sample analysis process, a few points can be summarized:
1. Preliminary sample analysis directly at the 1F site (without sample transportation) could help fuel debris characterization proceed more effectively. Cases of successfully implementing fast and simple non-destructive methods such as XRF, gamma-spectroscopy, and α-particle imaging clearly demonstrate such a possibility.
2. The representativeness of samples and quality identification are important issues for fuel debris analysis. Providing representative samples could be useful for determining the target materials (combinations of properties) that they are supposed to represent. The analysis of materials from the different locations of the units could help distinguish materials with similar properties. For instance, those materials from ChNPP-4 were mainly corium, “lava”, “pumice”, products of corium–metal interaction, and radioactive molten steel.
For quality identification, five important principles should be applied in the analysis process.
3. It is important to distinguish “sampling” and “retrieval” concepts by their final goals. Sampling and sample analysis process could provide not only support for debris retrieval but also a wide range of information for R&D activities related to decommissioning, fuel debris management, and accident analysis. The sample analysis, going one step ahead of the debris retrieval process, can reduce uncertainties related to debris distribution and properties. Decision making based on analysis results would allow 1F decommissioning to proceed more safely by minimizing additional operations and secondary waste formation.
Author contributions
BZ: visualization, writing–original draft, and writing–review and editing. AP: writing–original draft and writing–review and editing. YN: Supervision. M: writing–original draft and writing–review and editing.
Funding
The author(s) declare that no financial support was received for the research, authorship, and/or publication of this article.
Conflict of interest
MK was employed by the Nuclear Damage Compensation and Decommissioning Facilitation Corporation (NDF).
The remaining authors declare that the research was conducted in the absence of any commercial or financial relationships that could be construed as a potential conflict of interest.
Publisher’s note
All claims expressed in this article are solely those of the authors and do not necessarily represent those of their affiliated organizations, or those of the publisher, the editors, and the reviewers. Any product that may be evaluated in this article, or claim that may be made by its manufacturer, is not guaranteed or endorsed by the publisher.
References
Abagyan, A. A., Arshavskii, I. M., Dmitriev, V. M., Kroshilin, A. E., Krayushkin, A. V., and Khalimonchuk, V. A. (1991). Computational Analysis of the initial stage of the accident at the Chernobyl’ atomic power plant. Sov. At. Energy 71, 785–795. doi:10.1007/bf01123528
Abe, Y., Iizawa, Y., Terada, Y., Adachi, K., Igarashi, Y., and Nakai, I. (2014). Detection of uranium and chemical state analysis of individual radioactive microparticles emitted from the Fukushima nuclear accident using multiple synchrotron radiation X-ray analyses. Anal. Chem. 86, 8521–8525. doi:10.1021/ac501998d
Adachi, K., Kajino, M., Zaizen, Y., and Igarashi, Y. (2013). Emission of spherical cesium-bearing particles from an early stage of the Fukushima nuclear accident. Sci. Rep. 3, 2554. doi:10.1038/srep02554
Afanas’eva, A. A., Fedosov, A. M., Donderer, R., Ehrrenstein, D., Liermann, R., Schumacher, O., et al. (1994). Analysis of the chernobyl accident taking core destruction into account. At. Energy 77, 573–579. doi:10.1007/BF02407429
Akers, D. W., Bart, G., Bottomley, P., and Hofmann, P. (1992). TMI-2 examination results from the OECD/CSNI program. EGG-OECD-9168.
Akers, D. W., Carlson, E. R., Cook, B. A., Ploger, S. A., and Carlson, J. O. (1986). TMI-2 core debris grab samples: examination and analysis: Part 1 (No. GEND-INF-075-Pt.1). Idaho Falls, United States: EG and G Idaho, Inc. Available at: https://tmi2kml.inl.gov/Documents/9a-Data-Recovery/GEND-INF-075-PT-1.
Anderson, E. B., Burakov, B. E., and Pazukhin, E. M. (1993). High-uranium zircon from Chernobyl lavas. Radiochim. Acta 60 (2-3), 149–152. doi:10.1524/ract.1993.60.23.149
Barrachin, M., Nakayoshi, A., Kurata, M., Morreale, A., and Onder, N. (2022). “Preliminary investigation of samples taken from the PCV inside of Fukushima Daiichi Nuclear Power Plant and implications on severe accident analysis,” in Proceedings of the 19th International Meeting on Nuclear Reactor Thermal Hydraulics (NURETH19). Presented at the 19th International meeting on Nuclear Reactor Thermal Hydraulics (NURETH19), Brussels, March 6-11, 2022.
Bogatov, S. A., Borovoi, A. A., Dubasov, Y. V., and Lomonosov, V. V. (1990). Form and parameters of the particles of the fuel ejection in the Chernobyl reactor accident. Sov. At. Energy 69, 595–601. doi:10.1007/BF02086947
Borovoi, A. A., Galkin, B.Ya., Krinitsyn, A. P., et al. (1990). New products formed by reaction of fuel with construction materials in the 4th block of the Chernobyl NPP. Sov. Radiochem. 32, 659–667.
Borovoi, A. A., and Velikhov, E. P. (2012). Experience of Chernobyl (Part 1) (in Russian). Moscow: Russian Federation.
Bottomley, D., and Coquerelle, M. (1989). Metallurgical examination of bore samples from the three mile Island unit 2 reactor core. Nucl. Technol. 87, 120–136. doi:10.13182/NT89-A27642
Broughton, J. M., Tolman, E. L., Kuan, P., and Petti, D. (1989). A scenario of the three mile Island unit 2 accident. Nucl. Technol. 87, 34–53. doi:10.13182/NT89-A27637
Brown, A., McIntyre, G. J., and Gräslund, C. (1989). Analysis of crystalline phases in core bore materials from three mile Island unit 2. Nucl. Technol. 87, 137–145. doi:10.13182/NT89-A27643
Burakov, B., Anderson, E., Galkin, B.Ya., Pazukhin, E. M., and Shabalev, S. I. (1994). Study of chernobyl “hot” particles and fuel containing masses: implications for reconstructing the initial phase of the accident. Radiochim. Acta 65, 199–202. doi:10.1524/ract.1994.65.3.199
Burakov, B. E. (2020). “Lava-like materials formed and solidified during Chernobyl accident,” in Comprehensive nuclear materials Editors R. Konings, and R. E. Stoller 2nd ed. (Amsterdam, Netherlands: Elsevier).
Burakov, B. E., Anderson, E. B., Shabalev, S. I., Strykanova, E. E., Ushakov, S. V., Trotabas, M., et al. (1997a). The behavior of nuclear fuel in first days of the Chernobyl accident. Mat. Res. Soc. Symp. Proc. 465, 1297–1308. doi:10.1557/PROC-465-1297
Burakov, B. E., Shabalev, S. I., and Anderson, E. B. (2003). “Principal features of Chernobyl hot particles: phase, chemical and radionuclide compositions,” in Role of interfaces in environmental protection. Editor S. Barany (Warrendale: Springer), 24. Pennsylvania 145–151.
Burakov, B. E., Strykanova, E. E., and Anderson, E. B. (1997b). Secondary uranium minerals on the surface of Chernobyl “lava.”. Mat. Res. Soc. Symp. Proc. 465, 1309–1311. doi:10.1557/PROC-465-1309
Duco, J., Trotabas, M., Geoffroy, M., Robin, G., and Le Marois, G. (1989). TMI-2 core materials examination at CEA, SEMCI-89-DT-667, december 1989. (No. CEA-DAS--578). fdada.info, 2023.
Ewing, R. C., Angell, C. A., Arnold, G. W., Cormack, A. N., Delaye, J. M., et al. (1997). Radiation effects in glasses used for immobilization of high-level waste and plutonium disposition. J. Mater. Res. 12, 1948–1978. doi:10.1557/jmr.1997.0266
Finn, P. A., Buck, E. C., Gong, M., Hoh, J. C., Emery, J. W., Hafenrichter, L. D., et al. (1994). Colloidal products and actinide species in leachate from spent nuclear fuel. Radiochim. Acta 66 (67), 189–196. doi:10.1524/ract.1994.6667.s1.189
Fletcher, C. D., Chamber, R., Bolander, M. A., and Dallman, R. J. (1988). Simulation of the chernobyl accident. Nucl. Eng. Des. 105, 157–172. doi:10.1016/0029-5493(88)90337-8
Fukaya, Y., Hirasaki, T., Kumagai, K., Tatsuoka, T., Takamor, K., and Suzuki, S. (2017). Corrosion mitigation activities performed after the Fukushima Daiichi accident. Corrosion 74, 577–587. doi:10.5006/2695
Fukushima Prefectural Government (2019. Fukushima Prefectural Government official ). Transition of evacuation designated zones. Available at: https://www.pref.fukushima.lg.jp/site/portal-english/en03-08.html (Accessed April 13, 2023).
Furuki, G., Imoto, J., Ochiai, A., Yamasaki, S., Nanba, K., Ohnuki, T., et al. (2017). Caesium-rich micro-particles: a window into the meltdown events at the Fukushima Daiichi nuclear power plant. Sci. Rep. 7, 42731. doi:10.1038/srep42731
GPU Nuclear (1990). TMI 2 defueling completion report Available at: https://www.nrc.gov/docs/ML1111/ML111100641.pdf .
Grambow, B., Nitta, A., Shibata, A., Koma, Y., Utsunomiya, S., Takami, R., et al. (2021). Ten years after the NPP accident at Fukushima: review on fuel debris behavior in contact with water. J. Nucl. Sci. Technol. 59, 1–24. doi:10.1080/00223131.2021.1966347
Gurzhiy, V. V., Burakov, B. E., Zubekhina, B. Y., and Kasatkin, A. V. (2023). Evolution of chernobyl corium in water: formation of secondary uranyl phases. Materials 14, 4533. doi:10.3390/ma16134533
Hidaka, T., Kasuga, H., Kakamu, T., Endo, S., Masuishi, Y., and Fukushima, T. (2022). Concerns related to returning home to a “difficult-to-return zone” after a long-term evacuation due to Fukushima Nuclear Power Plant Accident: a qualitative study. PLoS ONE 17, e0273684. doi:10.1371/journal.pone.0273684
Holton, W. C., Negin, C. A., and Owrutsky, S. L. (1990). The cleanup of Three Mile Island Unit 2: A technical history, 1979--1990. [Contains bibliography]. Technical report EPRI-NP-6931 (Electric Power Research Inst., Palo Alto, CA (USA): Grove Engineering, Inc., Rockville, United States). Available at: http://svcf.jp/pdf/Three_Mile_Iland2NP-6931.PDF.
Imoto, J., Ochiai, A., Furuki, G., Suetake, M., Ikehara, R., Horie, K., et al. (2017). Isotopic signature and nano-texture of cesium-rich micro-particles: release of uranium and fission products from the Fukushima Daiichi Nuclear Power Plant. Sci. Rep. 7, 5409. doi:10.1038/s41598-017-05910-z
Information Portal for the Fukushima Daiichi Accident Analysis and Decommissioning Activities (2003). Government-TEPCO Council on Mid-to-long term response for decommissioning, sponsored by the Ministry of Economy, Trade and Industry, Japan. https://fdada.info/en/home2/ (Accessed November 28, 2023).
International Atomic Energy Agency (1990). Qualitative acceptance criteria for radioactive wastes to be disposed of in deep geological formations. Vienna: International Atomic Energy Agency. IAEA-TECDOC-560.
International Atomic Energy Agency (1992). The Chernobyl accident: updating of INSAG-1. A report by the international nuclear safety advisory group. Available at: https://www-pub.iaea.org/MTCD/Publications/PDF/Pub913e_web.pdf.
International Atomic Energy Agency (2015). The Fukushima Daiichi accident. Vienna: Report by the Director General. Non-serial Publications. IAEA.
International Atomic Energy Agency (2021a). IAEA international peer review of mid-and-long-term Roadmap towards the decommissioning of TEPCO’s Fukushima Daiichi nuclear power station. Review Report 2021 (Fifth Review). Available at: .https://www.iaea.org/sites/default/files/21/08/review-report-270821.pdf.
International Atomic Energy Agency (2021b). Managing the decommissioning and remediation of damaged nuclear facilities. Available at: https://www-pub.iaea.org/MTCD/Publications/PDF/TE-1989web.pdf.
International Atomic Energy Agency (2023). Management of Severely Damaged Spent Fuel and Corium, International Atomic Energy Agency official website. Available at: https://www.iaea.org/projects/crp/t13015 (Accessed April 13, 2023).
International Research Institute for Nuclear Decommissioning (2015). IRID annual research report 2015. Available at:.https://irid.or.jp/_pdf/pamphleth27_eng.pdf?v=2.
International Research Institute for Nuclear Decommissioning (2016). IRID Annual research report 2016. Available at:.https://irid.or.jp/_pdf/pamphleth28_eng.pdf.
International Research Institute for Nuclear Decommissioning (2017). IRID Annual research report 2017. Available at:.https://irid.or.jp/_pdf/pamphleth29_eng.pdf.
International Research Institute for Nuclear Decommissioning (2020). Development of analysis and estimation Technology for fuel debris characterization. Available at:.https://irid.or.jp/wp-content/uploads/2022/04/IR_for_FY2020_03_Fuel_Debris_Characterization.pdf.
International Research Institute for Nuclear Decommissioning (2022). International research Institute for nuclear decommissioning. Available at:.https://irid.or.jp/wp-content/uploads/2022/04/IRID_ROBOTS_2022_ENG.pdf.
International Research Institute for Nuclear Decommissioning (2023). (IRID) and Tokyo electric power Company holdings, Inc.(TEPCO). Status of the internal investigation of unit 1 PCV. (In Japanese). Available at:.https://www.nra.go.jp/data/000427820.pdf.
Japan Atomic Energy Agency (2000). Technical reliability of geological disposal of high-level radioactive waste in Japan in the second report on geological disposal research and development. (in Japanese).
Japan Atomic Energy Agency (2020). Analysis of debris samples of Tokyo electric power Company holdings Fukushima Daiichi nuclear power station (translated document). JAEA-Review 2020-055. doi:10.11484/jaea-review-2020-055
Jensen, S. M., Akers, D. W., Garner, R. W., and Roybal, G. S. (1987). Examination of the TMI-2 core distinct components (No. GEND-INF-082, september 1987).
Kiselev, A. N., Nenaglyadov, A. Y., Surin, A. I., and Checherov, K. P. (1992). Experimental study of lava-like fuel containing masses (FCM) at 4th unit of ChNPP (based on results obtained in 1986–1991). Moscow: Issue of IAE. (in Russian).
Koma, Y., and Murakami, E. (2019). Contamination of Fukushima Daiichi nuclear power station with actinide elements. Radiochim. Acta 107, 965–977. doi:10.1515/ract-2019-3126
Koyama, (2021). Contaminated Water Management (Development of Analysis and Estimation Technology for Characterization of Fuel Debris. Development of Technologies for Enhanced Analysis Accuracy and Thermal Behavior Estimation of Fuel Debris). Taisaku Jigyo Final report 2020 (in Japanese) Available at: https://dcccprogram.jp/files/202108jaea.pdf
Kurata, M., Okuzumi, N., Nakayoshi, A., Ikeuchi, H., and Koyama, S. (2022). Step-by-step challenge of debris characterization for the decommissioning of fukushima-daiichi nuclear power station (FDNPS). J. Nucl. Sci. Technol. 59, 807–834. doi:10.1080/00223131.2022.2040393
Kurihara, Y., Takahata, N., Yokoyama, T., Miura, H., Kon, Y., Takagi, T., et al. (2020). Isotopic ratios of uranium and caesium in spherical radioactive caesium-bearing microparticles derived from the Fukushima Dai-ichi Nuclear Power Plant. Sci. Rep. 10, 3281. doi:10.1038/s41598-020-59933-0
Lewis, W. B. (1953). The accident to the NRX reactor on December 12, 1952. Available at:.https://www.osti.gov/servlets/purl/4379334.
Lönartz, M. I., Pöml, P., Colle, J., Manara, D., and Burakov, B. E. (2023). Characterization of black and brown Chernobyl “lava” matrices: the formation process reviewed. Prog. Nucl. Energy 163, 104796. doi:10.1016/j.pnucene.2023.104796
McCardell, R. K., Russell, M. L., Akers, D. W., and Olsen, C. S. (1990). Summary of TMI-2 core sample examinations. Nucl. Eng. Des. 118, 441–449. doi:10.1016/0029-5493(90)90045-Y
Mendoza, Z. T., Stevens, C. A., and Ritzman, R. L., 1981. Radiation releases from the SL-1 accident. Nucl. Technol. 53, 155–162. doi:10.13182/NT81-A32620
Morishita, Y., Torii, T., Usami, H., Kikushi, H., Utsugi, W., and Takahira, S. (2019). Detection of alpha particle emitters originating from nuclear fuel inside reactor building of Fukushima Daiichi Nuclear Power Plant. Sci. Rep. 9, 581. doi:10.1038/s41598-018-36962-4
Nishihara, K., Yamaguchi, I., Yasuda, K., Ishimori, K., Tanaka, K., Kuno, T., et al. (2015). Radionuclide release to stagnant water in the Fukushima-1 nuclear power plant. J. Nucl. Sci. Technol. 52, 301–307. doi:10.1080/00223131.2014.946455
Nuclear Damage Compensation and Decommissioning Facilitation Corporation (2022). Technical strategic plan 2022 for decommissioning of the Fukushima Daiichi nuclear power. Station of Tokyo Electric Power Company Holdings, Inc.
Nuclear Damage Compensation and Decommissioning Facilitation Corporation (2023). The nuclear damage compensation and decommissioning facilitation corporation (pamphlet). Available at: https://www.dd.ndf.go.jp/english/about/pamphlet/index.html (Accessed September 10, 2023).
Nuclear Regulation Authority of Japan (2021). Nuclear regulation authority of Japan. Annual Report 2021.
Ochiai, A., Imoto, J., Suetake, M., Komiya, T., Furuki, G., Ikehara, R., et al. (2018). Uranium dioxides and debris fragments released to the environment with cesium-rich microparticles from the Fukushima Daiichi nuclear power plant. Environ. Sci. Technol. 52, 2586–2594. doi:10.1021/acs.est.7b06309
Ono, A. (2022). “Fukushima Daiichi decommissioning current status and challenges,” in 2022 IAEA General Conference, Side Event, Vienna, Austria, 26 September 2022.
Pakhomov, S. A., Krivokhatsky, I. A., and Sokolov, I. A. (1991). Estimation of the instantaneous energy release in the Chernobyl NPP reactor accident based on the ratio of of 133Xe and 133mXe activities in Air. Radiochemistry 33, 685–688.
Pazukhin, E. M. (1994). Fuel-containing lavas of the Chernobyl NPP 4th block – topography, physical chemical properties, formation scenario. Radiochemistry 36, 109–154.
Pöml, P., and Burakov, B. (2017). Study of a “hot” particle with a matrix of U-bearing metallic Zr: clue to supercriticality during the Chernobyl nuclear accident. J. Nucl. Mater. 488, 314–318. doi:10.1016/j.jnucmat.2017.01.041
Pöml, P., and Burakov, B. (2018). Study of the redistribution of U, Zr, Nb, Tc, Mo, Ru, Fe, Cr, and Ni between oxide and metallic phases in the matrix of a multiphase Chernobyl hot-particle extracted from a soil sample of the Western Plume. Radiochim. Acta 106, 985–990. doi:10.1515/ract-2018-2957
Rogozin, Yu.M., Smirnova, E. A., Savonenkov, V. G., Krivokhatsky, A. S., Andreev, V. A., and Sagaidachenko, E.Yu. (1991). Leaching of radionuclides from some newly formed products, extracted from reactor zone of 4th unit of Chernobyl NPP. Radiochemistry 33, 160–167.
Romanchuk, A., Vlasova, I., and Kalmykov, S. (2020). Speciation of uranium and plutonium from nuclear legacy sites to the environment: a mini review. Front. Chem. 8, 630. doi:10.3389/fchem.2020.00630
Sandalls, F. J., Segal, M. G., and Victorova, N. (1993). Hot particles from chernobyl: a review. J. Environ. Radioact. 18, 5–22. doi:10.1016/0265-931X(93)90063-D
Sato, Y., Kiwamu, T., Ueno, T., Ishimori, K., and Kameo, Y. (2016). Radiochemical analysis of rubble collected from Fukushima Daiichi nuclear power station. Hoken Butsuri 51, 209–217. doi:10.5453/jhps.51.209
Sattonnay, G., Ardois, C., Corbel, C., Lucchini, J., Barthe, M. F., Garrido, F., et al. (2001). Alpha-radiolysis effects on UO2 alteration in water. J. Nucl. Mater. 288, 11–19. doi:10.1016/S0022-3115(00)00714-5
Shabalev, S. I., Burakov, B. E., and Anderson, E. B. (1997). General classification of “hot” particles from the nearest chernobyl contaminated areas. MRS Proc. 465 465, 1343–1350. doi:10.1557/proc-465-1343
Shiryaev, A., Burakov, B., Yapaskurt, V., Zubekhina, B., Averin, A., Petrov, Y., et al. (2022). Products of molten corium-metal interaction in chernobyl accident: composition and leaching of radionuclides. Prog. Nucl. Energy 152, 104373. doi:10.1016/j.pnucene.2022.104373
Shiryaev, A. A., Burakov, B. E., Vlasova, I. E., Nickolsky, M. S., Averin, A. A., and Pakhnevich, A. V. (2020). Study of mineral grains extracted from the Chernobyl “lava.”. Mineralogy Petrology 114, 489–499. doi:10.1007/s00710-020-00718-8
Shiryaev, A. A., Vlasova, I. E., Burakov, B. E., Averin, A. A., and Elantyev, I. (2018). Forensic study of early stages of the Chernobyl accident: story of three hot particles. J. Nucl. Mater. 511, 83–90. doi:10.1016/j.jnucmat.2018.09.003
Shiryaev, A. A., Vlasova, I. E., Burakov, B. E., Ogorodnikov, B. I., Yapaskurt, V. O., Averin, A. A., et al. (2016). Physico-chemical properties of Chernobyl lava and their destruction products. Prog. Nucl. Energy 92, 104–118. doi:10.1016/j.pnucene.2016.07.001
Sunder, S., Shoesmith, D. W., and Miller, N. H. (1997). Oxidation and dissolution of nuclear fuel (UO2) by the products of the alpha radiolysis of water. J. Nucl. Mater. 244, 66–74. doi:10.1016/S0022-3115(96)00709-X
Tardiff, N. (1962). Some aspects of the WTR and SL-1 accidents. Prepared for presentation at International Atomic Energy Agency Symposium on Reactor Safety. doi:10.2172/4828615
Tokyo Electric Power Company Holdings, Inc. (2015). Development of Technology for the reactor containment vessel internal investigating. Available at: .https://www.tepco.co.jp/decommission/common/images/progress/retrieval/unit1_meeting_20150430.pdf.
Tokyo Electric Power Company Holdings, Inc. (2018. Materials of 62nd Specified Nuclear Facilities Monitoring and Evaluation Study Group meeting). 2018 Analysis results of the samples related to PCV internal investigation of Units 1-3 (in Japanese)Available at: https://www.tepco.co.jp/nu/fukushima-np/handouts/2018/images2/handouts_180810_04-j.pdf (Accessed August 10, 2018), 1–3.
Tokyo Electric Power Company Holdings, Inc. (2019a. Mid-and-Long-Term Roadmap towards the Decommissioning of TEPCO’s Fukushima Daiichi Nuclear Power Station). The Inter-Ministerial Council for Contaminated Water and Decommissioning Issues. Available at: https://www.meti.go.jp/english/earthquake/nuclear/decommissioning/pdf/20191227_3.pdf (Accessed December 27, 2019).
Tokyo Electric Power Company Holdings, Inc. (2019b). Mid-and-Long-Term Roadmap towards the decommissioning of TEPCO’s Fukushima Daiichi nuclear power station units, 1–4.
Tokyo Electric Power Company Holdings, Inc. (2020). 2020 Analysis results of the samples related to PCV internal investigation of Units 1-3. Available at: https://www.meti.go.jp/earthquake/nuclear/decommissioning/committee/osensuitaisakuteam/2020/11/3-3-4.pdf.
Tokyo Electric Power Company Holdings, Inc. (2020). Fukushima Daiichi samples analysis (in Japanese). Available at: .https://www.tepco.co.jp/nu/fukushima-np/handouts/2017/images2/handouts_171030_07-j.pdf.
Tokyo Electric Power Company Holdings, Inc. (2023a). Unit 1 PCV internal investigation results. (in Japanese).
Tokyo Electric Power Company, Inc. (2011). Mid-and-long-Term Roadmap towards the decommissioning of Fukushima Daiichi nuclear power station units. Available at: .https://www.tepco.co.jp/en/press/corp-com/release/betu11_e/images/111221e14.pdf.
Tolman, E. L., Smith, R. P., Martin, M. R., McCardell, R. K., and Broughton, J. M. (1987). TMI-2 Core Bore Acquisition Summary Report, Technical Report No. EGG-TMI-7385, February 1987 (EG and G Idaho, Inc., Idaho Falls, ID (United States). Available at: https://www.osti.gov/servlets/purl/285489-P22qE7/webviewable/.
Trotabas, M., Blanc, J.-Y., Burakov, B., Anderson, E., and Duco, J. (1993). Impact on the accident scenario understanding (No. DMT/92/309, SETIC/LECR-92/36, Report IPSN/93/02, Report RI-1-63/92. Saclay, France: CEA. ).Examination of chernobyl samples
Ushakov, S. V., Burakov, B. E., Shabalev, S. I., and Anderson, E. B. (1996). Interaction of UO2 and zircaloy during the Chernobyl accident. MRS Proc. 465, 1313–1318. doi:10.1557/PROC-465-1313
USSR State Committee on the Utilization of Atomic Energy (1986). Information on the accident at the Chernobyl nuclear power plant and its consequences prepared for IAEA. Sov. At. Energy 61, 845–868.
Xiao, X. (2019). Fundamental mechanisms for irradiation-hardening and embrittlement: a review. Metals 9, 1132. doi:10.3390/met9101132
Yomogida, T., Ouchi, K., Oka, T., Kitatsuji, Y., Koma, Y., and Konno, K. (2022). Analysis of particles containing alpha-emitters in stagnant water at torus room of Fukushima Dai-ichi Nuclear Power Station’s Unit 2 reactor. Sci. Rep. 12, 7191. doi:10.1038/s41598-022-11334-1
Yoshizawa, A. (2017). Overview of IRID R&D. Available at: https://www.irid.or.jp/_pdf/20170428.pdf.
Zhao, P., and Steward, S. A. (1997). Literature review of intrinsic actinide colloids related to spent fuel waste package release rates. (UCRL-ID-126039). doi:10.2172/461376
Zheng, J., Tagami, K., Watanabe, Y., Uchida, S., Aono, T., Ishii, N., et al. (2012). Isotopic evidence of plutonium release into the environment from the Fukushima DNPP accident. Sci. Rep. 2, 304. doi:10.1038/srep00304
Zubekhina, B., and Burakov, B. (2017). Leaching of actinides and other radionuclides from matrices of Chernobyl “lava” as analogues of vitrified HLW. J. Chem. Thermodyn. 114, 25–29. doi:10.1016/j.jct.2016.08.029
Zubekhina, B., Burakov, B., Bogdanova, O., and Petrov, Y. (2019). Leaching of 137Cs from Chernobyl fuel debris: corium and “lava.”. Radiochim. Acta 107, 1155–1160. doi:10.1515/ract-2019-0009
Keywords: Fuel debris, Corium, Fukushima Dai-Ichi (1F), 1F decommissioning, 1F samples analysis
Citation: Zubekhina B, Pshenichnikov A, Nagae Y and Kurata M (2023) The key role of sample analysis in Fukushima Dai-Ichi decommissioning, debris management, and accident progression investigation. Front. Nucl. Eng. 2:1324221. doi: 10.3389/fnuen.2023.1324221
Received: 19 October 2023; Accepted: 09 November 2023;
Published: 05 December 2023.
Edited by:
Walter Villanueva, Bangor University, United KingdomReviewed by:
Akitoshi Hotta, Nuclear Regulation Authority, JapanJuri Stuckert, Karlsruhe Institute of Technology (KIT), Germany
Copyright © 2023 Zubekhina, Pshenichnikov, Nagae and Kurata. This is an open-access article distributed under the terms of the Creative Commons Attribution License (CC BY). The use, distribution or reproduction in other forums is permitted, provided the original author(s) and the copyright owner(s) are credited and that the original publication in this journal is cited, in accordance with accepted academic practice. No use, distribution or reproduction is permitted which does not comply with these terms.
*Correspondence: Bella Zubekhina, enViZWtoaW5hLmJlbGxhQGphZWEuZ28uanA=