- School of Nuclear Science and Engineering, Oregon State University, Corvallis, OR, United States
Polymeric materials have multiple potential applications in nuclear power reactors including advanced and small modulus reactors, in addition to their extensive usage in safety electronic cables in the existing light water reactors. Through the qualification programs, knowledge accumulates about the polymer degradation kinetics under gamma-irradiation environment to facilitate the development of service-life predictive models. This paper aims to promote mechanistic-based predictability as a new approach to the qualification of polymeric components. Reviewed in this article are the current qualification standards and procedures, the current understandings of the degradation mechanisms, and kinetic models applicable to accelerated experiments for qualification.
1 Introduction
Modern industrial usage of natural and man-made polymeric materials is extensive and spans various sectors, including the nuclear industry. In nuclear power plants (NPPs), polymers are primarily used as seals and gaskets, valve diaphragms, electrical insulation, hoses, coatings and vibration mountings, as reviewed by Burnay (Burnay, 2001). Following the Fukushima Daiichi accident, the nuclear community recommends rubber bearings for seismic isolation in both new light water and small modular reactors (Kumar et al., 2015; Zhou et al., 2018). Recently, polymer-based soft robots were proposed to replace rigid robots in surveillance, inspection, and decontamination tasks for their advantages in body morphing, high compliance, and lower cost (Yirmibeşoğlu et al., 2019). Additionally, some polymeric materials were proposed as solidifying agents for radioactive waste management (Özdemir and Usanmaz, 2009).
Polymeric materials lose ductility when exposed to gamma irradiation in typical nuclear reactor environments. The embrittlement mechanisms are known to include thermal-radial oxidative degradation, which modify the polymer chain structure and, consequently, the mechanical properties (Bhattacharya, 2000; Assessment and Management of Ageing of Major Nuclear Power Plant Components Important to Safety: In-containment Instrumentation and Control cables. Volume II, 2000; Drobny, 2013). Generally, the chain structures are modified by means of chain scission and cross-linking, both processes involving oxidation. With bond breakage through chain scission, the polymers lose their mechanical strength. On the other hand, the cross-linkage of multiple chains reduces the plastic deformability. As a result of these structural changes, the elongation at failure reduces. This elongation at failure is often used as a performance criterion for polymeric materials in service conditions (Subudhi, 1996).
This review focuses on the degradation of polymeric materials based on extensive studies of NPP safety cables, of which the regulatory considerations and standards will be discussed in Section 2. The service-life qualification requires accelerated testing as the expected service-life of the cables are decades long. Thus, data extrapolation methodologies have been proposed based upon the fundamental understandings of the degradation mechanisms as reviewed in Section 3.
2 Qualification and regulation of safety cables
The most well recognized and studied nuclear application of polymeric materials is as the insulation for safety cables. These cables include instrumentation and control (I&C) cables (∼80%) that are connected to monitoring devices (thermocouples, pressure transducers and dosimeters) and the control circuits (switches and valve controllers), power cables (15%) that energize medium- and high-voltage equipment (e.g., pump motors and switchgear), and communication cables (5%). The cables used in nuclear plants have similar structure, with one or more metallic conductors insulated with polymeric materials as electrical insulation, outer jacket, and sometimes filler to occupy the gaps between insulated conductors (Assessment and Management of Ageing of Major Nuclear Power Plant Components Important to Safety: In-containment Instrumentation and Control cables. Volume II, 2000). In some cables, a metallic screen is sandwiched between two different polymeric materials. Besides shielding the electronics, this metallic screen prevents interdiffusion between two polymeric components by avoiding direct contact with one another. (Assessment and Management of Ageing of Major Nuclear Power Plant Components Important to Safety: In-containment Instrumentation and Control cables. Volume II, 2000). Among the different cable components, of primary degradation concerns are the insulation and jacket materials for their obviously important functionalities.
The U.S. Nuclear Regulatory Commission regulates the qualification of safety-related cables and field splices for nuclear power plants through the Regulatory Guide 1.211, which was issued in 2009 and reviewed in 2015. Despite several minor exceptions and amendments, the Regulatory Guide 1.211 (Qualification of Safety-Related Cables and Field Splices for Nuclear Power Plants, 2009) is in conformance with the IEEE Standard 383-2003 (IEEE Standard for Qualifying Class IE Equipment for Nuclear Power Generating Stations, 2003, 383), which documents the general requirements, direction, and methods for qualifying the safety-related cables, field splices, factory splices, and factory rework for service in nuclear power plants. Based on IEEE Standard 383-2003, IEEE issued the updated Standard 383-2015, incorporating the additions of a normal service test and long-term water test methods, among other updated and clarifications (IEEE Standard for Qualifying Electric Cables and Splices for Nuclear Facilities, 2015, 383).
The principal qualification criterion is that the cables meet or exceed specified performance requirement throughout their installed life, including during and after designed based events (DBEs). This requires cables to be manufactured in accordance with the applicable industry standards, and their qualified life established per the IEEE standards. The service-life qualification utilizes a combination of type testing, operating experience, and analysis as a supplement. The rationale of the qualification program lies in the potential for failures due to the degradation of the cable materials under normal service condition, followed by exposure to DBEs involving the extremes of temperature, pressure, humidity, radiation, mechanical stress, and chemical spray. The type testing methods for qualification and the degradation mechanisms are reviewed in the following section.
IEEE identifies type testing as the preferred method for qualification (IEEE Standard for Qualifying Electric Cables and Splices for Nuclear Facilities, 2015). It is required that the testing samples are representative of assemblies in actual plant applications and the testing conditions are at least as severe as the conditions defined in the qualification plan. The generated data of the sample performance is meant to enable the users to determine if the required safety functions can be achieved for the specific applications. The integrity and properties of both the insulation and the jacket materials are of primary concerns in the qualification process, especially after the exposure to the testing conditions simulating both service and DBE exposures. The post-exposure testing includes, not exclusively, the electrical functionality of the cables, integrity of the jacket materials, and the embrittlement of the cables due to radiation exposure. For DBE testing, samples in both as-fabricated and post-normal radiation and thermal exposure conditions are required. In reality, the normal service exposure could be decades long. Thus, accelerated testing methodology must be applied considering both thermal and radiation degradation mechanisms.
3 Degradation mechanisms and predictability
The aim of accelerated testing is to expedite the degradation process by applying testing conditions under which the degradation kinetics are more rapid. For example, exposure to higher dose rates of gamma irradiation at an elevated temperature is often utilized in the NPP cable community. Such accelerated testing is under the presumptions that an equivalent damage can be achieved through a shortened testing period and that the testing results are extrapolatable to estimate the service life under prototypic conditions.
To bridge the accelerated and prototypical conditions, the empirical “equal dose–equal damage” concept was initially utilized to establish the threshold of radiation damage (Schönbacher and Stolarz-Izycka, 1979; Bruce and Davis, 1981). However, this approach was soon shown to underpredict the embrittlement of a polyethylene insulation on the power control wiring of the Savanah River K-reactor at Aiken, South Carolina (Clough and Gillen, 1982). Concerns arise around the dose-rate effects and the synergism between irradiation and temperature, stimulating further investigations that came up with the concept of dose to equivalent damage (DED), which incorporates the corrections of the dose-rate and irradiation temperature effects (Clough and Gillen, 1981; Machi, 1981; Ohki, 1986; Gillen and Clough, 1989). Also introduced is the concept of time to equivalent damage (TED), which equals to the ratio between DED and dose rate. For certain materials within a given dose rate and temperature window, TED was believed to follow an Arrhenius temperature dependence, allowing the extrapolation from the experimental TED to prototypic service lifetime through an activation energy Ea (Gillen and Clough, 1989; Assessment and Management of Ageing of Major Nuclear Power Plant Components Important to Safety: In-containment Instrumentation and Control cables. Volume II, 2000). On the other hand, the importance of non-Arrhenius behavior was recognized, as the Ea determined under short-term accelerated testing conditions may overestimate the lifetime of materials at prototypic conditions (Gillen and Bernstein, 2010).
Using data from both thermal and radiation ageing, Gillen and Clough proposed a time-temperature-dose rate superposition methodology to obtain Ea at a given dose rate (Gillen and Clough, 1989). This empirical approach has successfully extrapolated accelerated testing data to prototypic conditions when a single dominant ageing mechanisms for both thermal and radiation ageing persist (Assessment and Management of Ageing of Major Nuclear Power Plant Components Important to Safety: In-containment Instrumentation and Control cables. Volume II, 2000). On the other hand, based on fundamental understanding of the degradation mechanisms, radio-thermal oxidation kinetic models have been proposed to describe the time-dependent behavior of the reactants and products, whose quantities can be used to define the end-of-life criterion, based on the qualitative relationships between the embrittlement and the molecular structure and concentrations (Gillen and Clough, 1985; Fuse et al., 2012; 2014; Hettal et al., 2021). Such models are specific to the polymer types and sensitive to the initial boundary conditions (Hettal et al., 2021) and involve multiple kinetic factors that are theoretically dependent on the environmental factors, including temperature, oxygen concentration, and irradiation type and energy. The model predictions have shown satisfactory consistency with experimental results, illustrating plausible degradation mechanisms and their activation processes and providing mechanistic explains to the non-Arrhenius behavior (Fuse et al., 2014). Although most of the kinetic models are too specific and complex to be systematically and directly validated, they provide foundations to the development of mechanistic-based reduced order models that serves as feasible frameworks to meet the practical needs for accelerated testing and lifetime prediction (Gillen and Bernstein, 2010; Celina et al., 2019).
3.1 Degradation processes and environmental dependences
Under NPP environment, common polymeric materials for cables, such as polyethylene (PE), polyvinyl chloride (PVC), and ethylene-propylene-diene monomer (EPDM), undergo oxidative degradation, involving initiation, propagation, and termination processes, as illustrated in Figure 1A (Pilot Study on the Management of Ageing of Instrumentation and Control Cables, 1997). During initiation, free alkyl radicals (R•) are created through the dissociation of the intra-chain chemical bonds by means of both radiolysis and thermal decomposition. Oxidation of the radicals leads to peroxyl radicals (RO2•), which propagate degradation through reactions that form hydroperoxide (ROOH) and/or products plus a free alkyl radical (R•). The propagation is terminated when the radicals (R• and/or RO2•) react with each other or with an antioxidant (AO) to form inactive products. When oxygen and radicals are abundant and the termination mechanisms are limited, the propagation reactions occur spontaneously, leading to the so-called auto-oxidation scenario (Vohlídal, 2021).
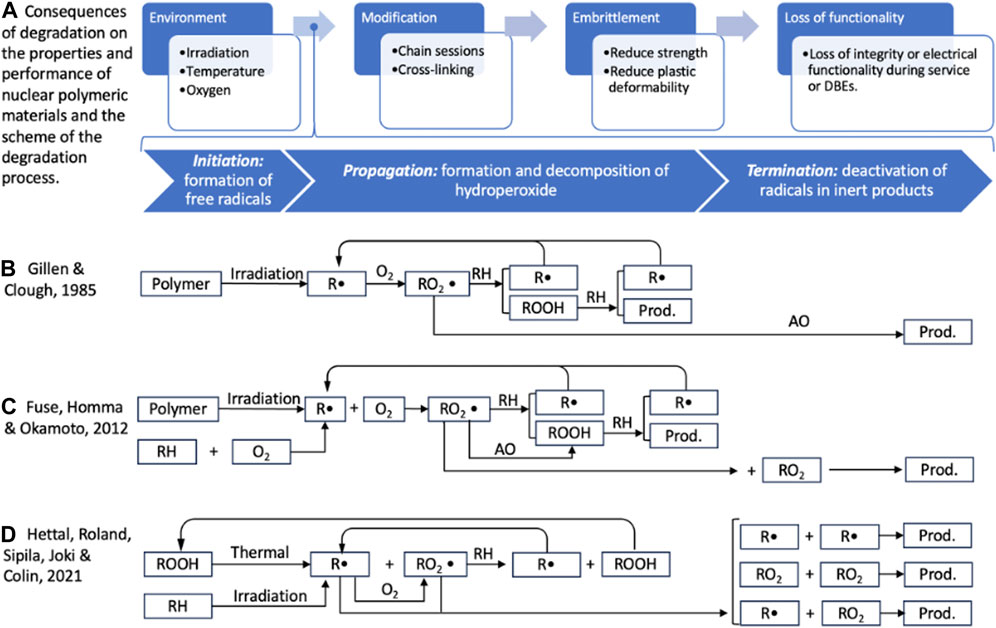
FIGURE 1. Schematic views of the polymeric material degradation and the involved processes (A), and diagrams of three degradation kinetic models to be reviewed in this paper (B–D). R•, RO2•, ROOH, and AO stand for free alkyl radicals, peroxyl radicals, hydroperoxide, and antioxidant, respectively.
The kinetic factors of the radio-thermal oxidative degradation are environmental dependent. Usually, the temperature dependency of thermal-decomposition kinetics can be considered through an Arrhenius rate equation if the governing thermal decomposition mechanism persists. The dose-rate dependency of kinetic model parameters are usually obtained through an optimization procedure by adjusting to experimental data obtained under both “pure thermos-oxidative” and “pure radio-oxidative” conditions (Colin et al., 2007). Additionally, the oxygen condition plays a critical role in controlling the degradation rate. At low oxygen concentration and/or diffusivity, the degradation is diffusion limited. As oxygen can only diffuse to certain depth from the sample surface, this diffusion-limited oxidation is heterogeneous. Under such conditions, cross-linking reaction (the formation of a covalent link of two adjacent macromolecules) may become dominant over scission (the formation of two new chains after the breaking up of one), potentially mitigating the loss of strength and thus the mechanical degradation (Fuse et al., 2014). On the other hand, at high oxygen concentrations, the degradation is more homogeneous, and the rate is controlled by the concentration of radicals that are created via radial and thermal decomposition.
3.2 Mechanistic-based kinetic models
Gillen et al. published the first of its kind kinetic model in 1985 (Gillen and Clough, 1985), summarized in Figure 1B. This model applies to the homogeneous degradation region with an assumption of abundant oxygen, whose diffusion process is then negligible. By considering the thermal decomposition of hydroperoxides (ROOH), a product from the propagation of irradiation-generated radical, the model predicts the auto-acceleration of the degradation as a result of radial-thermal synergism. Furthermore, this model predicts a dose-rate dependency of the DED, illustrated by the solid line in Figure 2, and an analytical and theoretical basis for a time-temperature-dose rate shifting procedure. This procedure provides a practical guidance to the accelerated testing by allowing higher temperature and dose-rate results to be shifted to the lower dose-rate regime (Gillen and Clough, 1989).
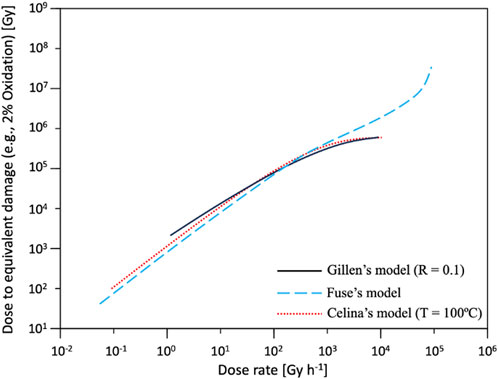
FIGURE 2. Comparison of the dose-rate effect of gamma irradiation on DED predicted by the Gillen’s, Fuse’s and Celina’s models, replotted based on the data published in Refs. (Gillen and Clough, 1985; Fuse et al., 2012; Celina et al., 2019). Note that the curves of Gillen’s and Fuse’s were published in arbitrary units and are shifted here to visually emphasize their consistency. For the model parameters and details, the readers are referred to the original publications (Gillen and Clough, 1985; Fuse et al., 2012; Celina et al., 2019).
Fuse et al. proposed a new model by incorporating the diffusions and concentration evolutions of oxygen and antioxidant (Fuse et al., 2012). As shown in Figure 1C, Fuse et al. further incorporated an oxygen assisted thermal activation (
Fuse’s model extends the predictability in two aspects. First, the dose rate effect is extended from the homogeneous to the heterogeneous degradation region. Shown in Figure 2 as the dashed line, the Fuse model qualitatively agrees with the Gillen’s model in the homogeneous degradation regime when oxygen is abundant and extends to the heterogeneous region as the dose rate further increases. The DED–dose rate curve showed a more rapid slope there as the radical formation due to radiation is so rapid that the diffusion and permeation of oxygen control the heterogeneous degradation behavior. This improvement allows for the simulation of the heterogeneous degradation behavior under the conditions such as in boiling water reactors where oxygen concentration is low and in accelerated testing conditions when the dose rate is so high that the reaction rate is controlled by the diffusion of oxygen. Second, this model quantitatively considers the chemical suppression effect of AO on degradation and predicts a critical AO concentration, below which the rate of degradation transits to extremely high. This critical AO concentration phenomenon has been previously observed and suggested in literature (Gugumus, 1994; Kanegami et al., 2013).
Figure 1D illustrates the experimental-based analytical model developed by Hettal et al. and emphasizes the thermal decomposition of ROOH, which was previously assumed to be thermally stable in both the Fuse and Gillian models. Without considering AO, this model employs the bimolecular termination mechanism in addition to two more, i.e.,
With the aid of the kinetic models, the time-dependent degradation behavior can be simulated and predicted. This is important as a catastrophic failure of cables is expected due to the onset of the auto-acceleration of the degradation. Discussed above, this auto acceleration may be activated through different mechanisms, such as the accumulation of free radicals through radial-thermal decomposition (Gillen and Clough, 1985), the burnup of AO (Fuse et al., 2012), and the accumulation of ROOH through oxidative reactions (Hettal et al., 2021). It can also be informed that a DBE may trigger the failure with the high-dose-rate and high-temperature exposure which boosts the decomposition and reaction kinetics, as well as the permeability and diffusivity of oxygen. This is especially a concern for cables that have been exposed to normal service conditions during which free radicals accumulated.
3.3 From accelerated testing to lifetime prediction
The qualification standards for accelerated testing were originally proposed based on the concept of accelerating the degradation process without changing the underlying mechanisms. Thus, the original purpose of kinetic models was to provide mechanisms to correlate accelerated testing results to the service lifetime, such as through the corrections via DED and TED and the time-temperature-dose rate shifting procedure developed by Gillen et al. (Gillen and Clough, 1989). The advantage of DED method over the conventional superposition of time-dependent data (STD) approach was demonstrated by a comparative analysis based on the NPP cable-aging assessment data published by the Japan Nuclear Energy Safety Organization (Yamamoto and Minakawa, 2009; Gillen and Bernstein, 2010). The DED approach facilitates a two-dimensional data extrapolation considering both temperature and radiation effects and thus is more robust with the presence of a transition from thermal dominated to radiation dominated material response (Gillen and Bernstein, 2010). It provides a tool to verify the Ea as a function of testing conditions to prevent the overestimation of service life when extrapolating accelerated testing data to the low-temperature low-dose-rate conditions.
More recently, the knowledge of fundamental degradation mechanisms has advanced considerably, especially with the developments of low-dimensional modeling and modern characterization techniques (Rivaton et al., 2005; Ullal and Spearot, 2014; Wang et al., 2018; Hettal et al., 2021; Liu et al., 2021). As a result, kinetic models continue to develop, considering different initiation, propagation, and termination mechanisms and leading to different failure mechanisms for different materials and conditions. This knowledge advancement and model development ultimately promote a transition from accelerated testing to physics-based prediction. The latter aims to obtain theoretical understanding through aging model-based experimental design as opposed to the early-stage semi-empirical-based approach.
Celina et al. proposed such a theoretical framework that “combines thermal and radiative activation energies into a general kinetic model” (Celina et al., 2019). Celina’s approach is applicable to the qualification process, as the IEEE standards prescribe an Ea based analytical method for qualified life extrapolation from testing performed in accelerated aging conditions (IEEE Standard for Qualifying Electric Cables and Splices for Nuclear Facilities, 2015). However, unlike the conventional methods where the Ea is empirically found, this new framework employs a secondary Ea whose physical interpretation is based on the radiation-thermal kinetic models. This additional degree of freedom allows for the considerations of radio-thermal synergism and the non-constant degradation rates. As an example shown in Figure 2 with the dotted line, without the complexities of the more specific kinetic models reviewed above, a theoretical-based reduced order model corrected the does-rate dependency of DED from the conventional time-temperature-dose rate shifting model (Gillen and Clough, 1989). Such mechanistic-based corrections will reduce the uncertainty present in conventional data extrapolation where the data form accelerated aging experiments may diverge in the quoted Ea.
4 Conclusion
The qualified life prediction of NPP safety-related cables is discussed from both the regulatory perspective and the underlying scientific considerations of the degradation mechanisms. While this review focuses on nuclear safety cables, the approach is transferable to other polymeric materials under different operational conditions. Discussed in Section 1, the multiple potential applications of polymers in existing and future nuclear reactor designs will lead to a wider range of material selection as well as dose rate, oxidative, and temperature conditions. Thus, qualification through the development of mechanistic-based predictability offers advantages over the conventional accelerated testing approach in both reliability and versatility. Based on the understanding of the degradation kinetics, a reduced order model considering the radiation and thermal activation processes through their activation energies provides a feasible approach to qualify polymers through accelerated experiments. The advancement of fundamental knowledge can facilitate a transition from the conventional accelerated aging approach towards mechanistic-based prediction (Celina et al., 2019). More broadly, such a transition represents the general trend in the qualification and life extension of nuclear materials, from fuels to other NPP components (Eason et al., 2013; Capps et al., 2023). Thus, the concept of mechanistic model-based qualification reviewed here are of cross-cutting benefits to other nuclear material programs.
Author contributions
TC: Conceptualization, Supervision, Visualization, Writing–original draft. T-YC: Formal Analysis, Writing–original draft. BK: Formal Analysis, Writing–original draft. MM: Formal Analysis, Writing–original draft. CP: Funding acquisition, Project administration, Resources, Supervision, Writing–review and editing.
Funding
The author(s) declare financial support was received for the research, authorship, and/or publication of this article. Oregon State University startup funding.
Acknowledgments
TC acknowledged Oregon State University startup funding.
Conflict of interest
The author (TC) declared that they were an editorial board member of Frontiers at the time of submission. This had no impact on the peer review process and the final decision.
The remaining authors declare that the research was conducted in the absence of any commercial or financial relationships that could be construed as a potential conflict of interest.
Publisher’s note
All claims expressed in this article are solely those of the authors and do not necessarily represent those of their affiliated organizations, or those of the publisher, the editors and the reviewers. Any product that may be evaluated in this article, or claim that may be made by its manufacturer, is not guaranteed or endorsed by the publisher.
References
Assessment and Management of Ageing of Major Nuclear Power Plant Components Important to Safety: In-containment Instrumentation and Control cables, (2000). INTERNATIONAL ATOMIC ENERGY AGENCY. https://www.iaea.org/publications/6166/assessment-and-management-of-ageing-of-major-nuclear-power-plant-components-important-to-safety-in-containment-instrumentation-and-control-cables-volume-ii.
Bhattacharya, A. (2000). Radiation and industrial polymers. Prog. Polym. Sci. 25, 371–401. doi:10.1016/S0079-6700(00)00009-5
Bruce, M. B., and Davis, M. V. (1981). Radiation effects on organic materials in nuclear plants Final report. http://inis.iaea.org/search/search.aspx?orig_q=RN:13680024.
Burnay, S. G. (2001). An overview of polymer ageing studies in the nuclear power industry. Nucl. Instrum. Methods Phys. Res. Sect. B Beam Interact. Mater. At. 185, 4–7. doi:10.1016/S0168-583X(01)00757-1
Capps, N., Aagesen, L., Andersson, D., Baldwin, O., Brinkley, W. C., Cooper, M. W. D., et al. (2023). Empirical and mechanistic transient fission gas release model for high-burnup LOCA conditions. J. Nucl. Mater. 584, 154557. doi:10.1016/j.jnucmat.2023.154557
Celina, M., Linde, E., Brunson, D., Quintana, A., and Giron, N. (2019). Overview of accelerated aging and polymer degradation kinetics for combined radiation-thermal environments. Polym. Degrad. Stab. 166, 353–378. doi:10.1016/j.polymdegradstab.2019.06.007
Clough, R. L., and Gillen, K. T. (1982). Investigation of cable deterioration inside reactor containment. Nucl. Technol. 59, 344–354. doi:10.13182/NT82-A33037
Clough, R. L., and Gillen, K. T. (1981). Radiation-thermal degradation of PE and PVC: mechanism of synergism and dose rate effects. Radiat. Phys. Chem. 18, 661–669. doi:10.1016/0146-5724(81)90189-8
Colin, X., Monchy-Leroy, C., Audouin, L., and Verdu, J. (2007). Lifetime prediction of polyethylene in nuclear plants. Nucl. Instrum. Methods Phys. Res. Sect. B Beam Interact. Mater. At. 265, 251–255. doi:10.1016/j.nimb.2007.08.086
Drobny, J. G. (2013). “Radiation-resistant polymers and their applications,” in Ionizing radiation and polymers (Elsevier), Amsterdam, Netherlands, 213–224. doi:10.1016/B978-1-4557-7881-2.00007-9
Eason, E. D., Odette, G. R., Nanstad, R. K., and Yamamoto, T. (2013). A physically based correlation of irradiation-induced transition temperature shifts for RPV steels. J. Nucl. Mater. 433, 240–254. doi:10.1016/j.jnucmat.2012.09.012
Fuse, N., Homma, H., and Okamoto, T. (September 2012). “A new degradation model with critical antioxidant concentration concept for safety cables,” in Proceedings of the 2012 IEEE international conference on condition monitoring and diagnosis Bali, Indonesia(IEEE), 153–156. doi:10.1109/CMD.2012.6416398
Fuse, N., Homma, H., and Okamoto, T. (2014). Remaining issues of the degradation models of polymeric insulation used in nuclear power plant safety cables. IEEE Trans. Dielectr. Electr. Insul. 21, 571–581. doi:10.1109/TDEI.2013.004086
Gillen, K. T., and Clough, R. L. (1989). Time-temperature-dose rate superposition: a methodology for extrapolating accelerated radiation aging data to low dose rate conditions. Polym. Degrad. Stab. 24, 137–168. doi:10.1016/0141-3910(89)90108-0
Gillen, K., and Bernstein, R. (2010). Review of nuclear power plant safety cable aging studies with recommendations for improved approaches and for future work. https://www.osti.gov/servlets/purl/1002106.
Gillen, K. T., and Clough, R. L. (1985). A kinetic model for predicting oxidative degradation rates in combined radiation-thermal environments. J. Polym. Sci. Polym. Chem. Ed. 23, 2683–2707. doi:10.1002/pol.1985.170231011
Gugumus, F. (1994). Critical stabilizer concentrations in oxidizing polymers. Polym. Degrad. Stab. 46, 123–140. doi:10.1016/0141-3910(94)90116-3
Hettal, S., Roland, S., Sipila, K., Joki, H., and Colin, X. (2021). A new analytical model for predicting the radio-thermal oxidation kinetics and the lifetime of electric cable insulation in nuclear power plants. application to silane cross-linked polyethylene. Polym. Degrad. Stab. 185, 109492. doi:10.1016/j.polymdegradstab.2021.109492
IEEE, “IEEE Standard for qualifying class 1E electric cables and field splices for nuclear power generating stations,” in IEEE Std 383-2003 (Revision of IEEE Std 383-1974), 1–21. doi:10.1109/IEEESTD.2004.94567
IEEE, (2015). “IEEE standard for qualifying electric cables and splices for nuclear Facilities,” in IEEE Std 383-2015 (Revision of IEEE Std 383-2003), 1–27. doi:10.1109/IEEESTD.2015.7287711
Kanegami, M., Kurihara, T., Fuse, N., Mizutani, Y., Homma, H., and Okamoto, T. (2013). Relationship between remaining antioxidant content and radiation-thermal degradation in crosslinked polyethylene. IEEJ Trans. Fundam. Mater. 133, 98–104. doi:10.1541/ieejfms.133.98
Kumar, M., Whittaker, A. S., and Constantinou, M. C. (2015). Seismic isolation of nuclear power plants using elastomeric bearings. New York, NY, USA: University at Buffalo, State University of.
Liu, Q., Huang, W., Liu, B., Wang, P.-C., and Chen, H.-B. (2021). Gamma radiation chemistry of polydimethylsiloxane foam in radiation-thermal environments: experiments and simulations. ACS Appl. Mater. Interfaces 13, 41287–41302. doi:10.1021/acsami.1c10765
Machi, S. (1981). Radiation degradation of polymeric materials used in nuclear reactor. Radiat. Phys. Chem. 18, 125–133. doi:10.1016/0146-5724(81)90070-4
Ohki, Y. (1986). Radiation effects on polymers. IEEE Trans. Electr. Insul. EI- 21, 919–922. doi:10.1109/TEI.1986.349002
Özdemir, T., and Usanmaz, A. (2009). Use of poly(methyl methacrylate) in radioactive waste management: I. Radiation stability and degradation. Prog. Nucl. Energy 51, 240–245. doi:10.1016/j.pnucene.2008.04.004
Pilot Study on the Management of Ageing of Instrumentation and Control Cables, (1997). https://www.iaea.org/publications/5584/pilot-study-on-the-management-of-ageing-of-instrumentation-and-control-cables. International atomic energy agency.
Qualification of Safety-Related Cables and Field Splices for Nuclear Power Plants, (2009). Qualification of safety-related cables and field splices for nuclear power plants. https://www.nrc.gov/docs/ML0825/ML082530205.pdf.
Rivaton, A., Cambon, S., and Gardette, J.-L. (2005). Radiochemical ageing of EPDM elastomers. 3. Mechanism of radiooxidation. Nucl. Instrum. Methods Phys. Res. Sect. B Beam Interact. Mater. At. 227, 357–368. doi:10.1016/j.nimb.2004.09.009
Schönbacher, H., and Stolarz-Izycka, A. (1979). Geneva, Switzerland: CERN. Compilation of radiation damage test data.
Subudhi, M. (1996). Literature review of environmental qualification of safety-related electric cables. Brookhaven National Laboratory. New York, NY, USA.
Ullal, V., and Spearot, D. (2014). Molecular dynamics simulation of O2 diffusion in polydimethylsiloxane (PDMS) and end-linked PDMS networks. Mol. Simul. 40, 976–986. doi:10.1080/08927022.2013.830183
Vohlídal, J. (2021). Polymer degradation: a short review. Chem. Teach. Int. 3, 213–220. doi:10.1515/cti-2020-0015
Wang, W., Tanaka, Y., Takada, T., Iwata, S., Uehara, H., and Li, S. (2018). Influence of oxidation on the dynamics in amorphous ethylene-propylene-diene-monomer copolymer: a molecular dynamics simulation. Polym. Degrad. Stab. 147, 187–196. doi:10.1016/j.polymdegradstab.2017.12.001
Yamamoto, T., and Minakawa, T. (2009). The final report of the project of assessment of cable aging for nuclear power plants. Japan Nuclear Energy Safety Organization Nuclear Energy System Safety Division, Chiyoda, Tokyo JNES-SS-0903
Yirmibeşoğlu, O. D., Oshiro, T., Olson, G., Palmer, C., and Mengüç, Y. (2019). Evaluation of 3D printed soft robots in radiation environments and comparison with molded counterparts. Front. Robot. AI 6, 40. doi:10.3389/frobt.2019.00040
Keywords: polymeric materials, nuclear safety cables, degradation mechanisms, dose-rate effect, accelerated testing
Citation: Chen T, Chang T-Y, Konstanczer BA, Mostaan MA and Palmer CJ (2023) Review of qualification and modeling for radiation-induced polymer degradation. Front. Nucl. Eng. 2:1287370. doi: 10.3389/fnuen.2023.1287370
Received: 01 September 2023; Accepted: 29 September 2023;
Published: 19 October 2023.
Edited by:
Peng Wang, University of Michigan, United StatesReviewed by:
Felix Eduardo Pino Andrades, Università degli Studi di Padova, ItalyCopyright © 2023 Chen, Chang, Konstanczer, Mostaan and Palmer. This is an open-access article distributed under the terms of the Creative Commons Attribution License (CC BY). The use, distribution or reproduction in other forums is permitted, provided the original author(s) and the copyright owner(s) are credited and that the original publication in this journal is cited, in accordance with accepted academic practice. No use, distribution or reproduction is permitted which does not comply with these terms.
*Correspondence: Tianyi Chen, dGlhbnlpLmNoZW5Ab3JlZ29uc3RhdGUuZWR1