- 1Department of Optometry and Vision Sciences, The University of Melbourne, Parkville, VIC, Australia
- 2Institute for Information Transmission Problems (Kharkevich Institute), Russian Academy of Sciences, Moscow, Russia
- 3Department of Biomedical Engineering, The University of Melbourne, Parkville, VIC, Australia
- 4Graeme Clark Institute, The University of Melbourne, Parkville, VIC, Australia
- 5Florey Institute of Neuroscience & Mental Health, University of Melbourne, Parkville, VIC, Australia
- 6Department of Medicine, University of Melbourne, Parkville, VIC, Australia
- 7Department of Neuroscience, St. Vincent’s Hospital, University of Melbourne, Melbourne, VIC, Australia
- 8Florey Department of Neuroscience & Mental Health, University of Melbourne, Parkville, VIC, Australia
The development of a seizure relies on two factors. One is the existence of an overexcitable neuronal network and the other is a trigger that switches normal activity of that network into a paroxysmal state. While mechanisms of local overexcitation have been the focus of many studies, the process of triggering remains poorly understood. We suggest that, apart from the known exteroceptive sources of reflex epilepsy such as visual, auditory or olfactory signals, there is a range of interoceptive triggers, which are relevant for seizure development in Temporal Lobe Epilepsy (TLE). The hypothesis proposed here aims to explain the prevalence of epileptic activity in sleep and in drowsiness states and to provide a detailed mechanism of seizures triggered by interoceptive signals.
Introduction
Temporal Lobe Epilepsy (TLE) is the most frequent form of focal epilepsy (60–70%) and constitutes around 24% of all cases of epilepsy (Semah et al., 1998, reviewed by Téllez-Zenteno and Hernández-Ronquillo, 2012). Among TLEs, mesial temporal lobe epilepsy (mTLE) is the most common form. It is a type of focal epilepsy originating from the medial part of the hippocampus, amygdala or the entorhinal cortex and relies on oversynchronization of activity of neuronal circuits in these areas (Diehl and Duncan, 2015). However, mTLE is likely a system disorder with network alterations due to structural and/or functional abnormalities in neocortical areas, especially the limbic, lateral temporal and frontal cortices and the thalamus (Bernhardt et al., 2013). TLE has also the highest rate of pharmaco-resistance (75–89%), only a moderate rate of successful surgical treatment at 65–70% (Sperling et al., 2005; Fanselow and Dong, 2010; Téllez-Zenteno and Hernández-Ronquillo, 2012) and an estimated Standard Mortality ratio of treatment-resistant epilepsy at 2.54 (Mohanraj et al., 2006). Although local neuronal changes associated with TLE have been extensively studied, much less is known about the triggers of seizure activity and mechanisms related to that triggering. Since frequency, severity, prodromal symptoms and patterns of occurrence across sleep–wake cycle vary between patients (Karoly et al., 2017, 2018; Lunardi et al., 2016; Niu et al., 2025), understanding the mechanisms that trigger seizures in TLE can provide substantial advantage for their prediction and treatment. Here we propose a scheme that describes a nexus between circadian rhythm, visceral inputs to the brain and the hippocampus that provides a fundamental insight into triggering of seizures in TLE and the role that stimulation of the vagus nerve can potentially fill in the management of TLE.
Vagus nerve stimulation: an unorthodox treatment for TLE
Vagus Nerve Stimulation (VNS) is a treatment option that is being increasingly adopted for pharmaco-resistant cases of epilepsy (for recent reviews, Austelle et al., 2024; Clifford et al., 2024; Salama et al., 2024). The vagus nerve carries signals between the internal (visceral) organs, such as the lungs, heart and intra-abdominal structures and the brain. The information carried by vagal afferents is necessary for ‘interoception’, which helps the brain to process the internal signals that are related to our physiological state in both subconscious and conscious states (Craig, 2002; Quadt et al., 2018; Khalsa et al., 2018; Berntson and Khalsa, 2021; Quigley et al., 2021; Feldman et al., 2024). On average, about two thirds of patients have fewer seizures following VNS, though one third do not benefit from it (Yap et al., 2020; Elliott, 2011; Englot et al., 2011). The side effects are generally mild and often decrease over time or after optimizing the stimulation parameters individually for each patient (Sackeim et al., 2001).
The antiepileptic effects of VNS were initially attributed to widespread cortical desynchronization (Zanchetti et al., 1952), but both synchronization and desynchronization have been observed with VNS in animal studies (Chase et al., 1966, 1967). EEG studies in human patients have also reported variable outcomes (Vonck and Larsen, 2018). A hypothesis involving modulation of noradrenaline pathways (Vonck and Larsen, 2018) has also been met with criticism. The slow speed of the development of noradrenaline modulation cannot explain the immediate effects of VNS (Dorr and Debonnel, 2006), although such modulation can potentially contribute to the increase of the effectiveness of VNS over time. Another confound is the lack of VNS-associated sleep disturbances usually caused by excessive noradrenaline levels. Although some VNS parameters can provoke obstructive sleep apnoea, it has been reported to generally improve sleep architecture to the extent that VNS has been proposed as an insomnia treatment (Wu et al., 2022). The slow effects of VNS in TLE might also be related to normalization of hippocampal activity from neuronal development and growth as well as changes in sensitivity of hippocampal neurons to GABA. The latter may be because GABAA receptor density in the hippocampus increases in patients responsive to VNS (Groves and Brown, 2005; Marrosu et al., 2003). VNS can also affect cytokine production, potentially controlling inflammation during long-term application (Majoie et al., 2011).
In summary, an explanation for the fast-acting component of vagal stimulation is lacking and the slow effects of VNS are also poorly understood. Thus, the method is currently used largely on an empirical basis (Yap et al., 2020; Groves and Brown, 2005). Such uncertainty means the outcome of the intervention cannot be predicted. This is an important issue, since not all patients benefit from VNS and so its effectiveness can only be established after the implantation and not prognostically (Yap et al., 2020).
Novel model for action of VNS in TLE
We have recently proposed a new framework describing how the antiepileptic effect of VNS may arise from interruption of the resonant paroxysmal activity triggered by rhythmical interoceptive signaling (Pigarev et al., 2020). In the present paper, we discuss specifically the applicability of that hypothesis to TLE and TLE-comorbid conditions, with an emphasis on hippocampal connectivity, including signals arising from sensory systems.
Since approximately 80% of vagal fibers are afferent, VNS is expected to activate multiple brain areas due to the broad representation of the vagal input in subcortical and cortical structures; e.g., nucleus of the solitary tract (NTS), hippocampus, hypothalamus, thalamus and amygdala, as well as multiple cortical areas such as the insular, orbitofrontal, medial prefrontal and cingulate cortices and some somatosensory and motor cortical areas (Saper, 2002; Cechetto and Saper, 1987; Neafsey, 1990; Ongür et al., 1998; Ongür and Price, 2000; Nieuwenhuys, 2012; Azzalini et al., 2019). The most important brain regions involved in visceral sensation and regulation of visceral functions are shown in Figure 1.
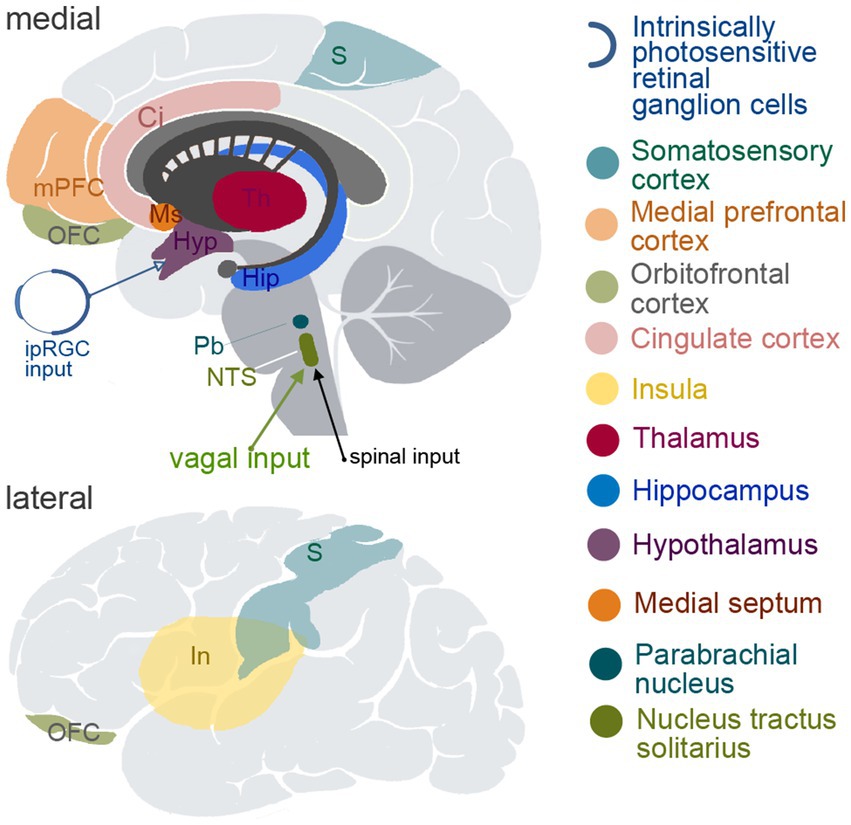
Figure 1. Schematic depiction of the brain structures known to be involved in viscerosensory and/or visceromotoric functions. The upper panel demonstrates medial view of the brain, and the lower panel shows lateral view. ipIGC, intrinsically photosensitive retinal ganglion cells; S, somatosensory cortical areas; mPFC, medial prefrontal cortex; OFC, orbitofrontal cortex; Ci, cingulate cortex; In, insula; Th, thalamus; Hip, hippocampus; Hyp, hypothalamus; Ms, medial septum; Pb, parabrachial nucleus; NTS, nucleus tractus solitarius.
Hippocampal functional connectivity and epilepsy—in sleep and when awake
Of the many brain areas potentially influenced by VNS, the hippocampus is the most seizure-susceptible structure in the brain (Green and Scheetz, 1964) and so it warrants a close examination of its afferent and efferent projections. However, the susceptibility for seizures is not uniformly distributed across the hippocampus but increases along its dorso-ventral axis in animals (corresponding to posterior-to-anterior axis in humans), as consistently reported in kindling studies in vitro and in vivo (Bragdon et al., 1986; Elul, 1964, Strange et al., 2014; Isaeva et al., 2015; Akaike et al., 2001). There is also a matching profile of GABAA receptor distribution along the same axis (Sotiriou et al., 2005). Human patients suffering from TLE also have greater structural atrophy in the anterior parts of the hippocampus in comparison to the posterior parts (Barnett et al., 2019). Furthermore, dorsal and ventral parts of the hippocampus have distinctly different connectivity with subcortical and cortical areas (Strange et al., 2014; Fanselow and Dong, 2010).
There is a specific smoothly changing pattern of connections along the above axis, which supports different goal-oriented behaviors. Dorsal hippocampus is engaged in cognitive functions including memory and spatial navigation, while ventral hippocampus is involved in emotional and affective behavior, stress-related responses and autonomic regulation. For example, lesions of dorsal hippocampus in rodents impair spatial memory (Moser et al., 1995), while ventral hippocampus lesions produce changes in emotional behavior and in reactions to stress (Kishi et al., 1990). In both rodents and primates, the dorsal hippocampus is connected with cortical and subcortical structures that form a circuit organizing exploratory and foraging activities (Swanson, 2000).
Ventral hippocampus connects extensively with structures of visceral and emotional control: amygdala, insular cortex, infralimbic and prelimbic cortices and the areas of hypothalamus that control autonomic, endocrine and somatomotor activities supporting behaviors with strong emotional components, such as feeding, reproduction and defense (Dong and Swanson, 2006; Herman et al., 2005; Kishi et al., 2000; Petrovich et al., 2001; Fanselow and Dong, 2010; Castle et al., 2005). The outermost ventral portions of hippocampal CA1 and subiculum project to hypothalamic neuroendocrine motor neurons via lateral septum and the bed nucleus of the stria terminalis, which is an important relay for hypothalamic–pituitary–adrenal axis of stress response (Dong and Swanson, 2006; Fanselow and Dong, 2010; Herman et al., 2016). Ventral hippocampus also projects to the shell of nucleus accumbens and shows responses related to expectation of food or to receiving food reward (Vidyasagar et al., 1991; Salzman et al., 1993). This emotion-related connectivity pattern of the ventral hippocampus may correspond to the high comorbidity of TLE and depression (Hermann et al., 2000). A recent study has highlighted both the high prevalence of depression in epilepsy and its persistence despite therapy with antidepressants (Ongchuan Martin et al., 2022). Human data regarding hippocampal dysfunction and volume loss also demonstrate association with other psychiatric conditions having an affective component, such as anxiety, bipolar and posttraumatic stress disorders (Frey et al., 2007; Bonne et al., 2008). It is notable that VNS was confirmed to be effective in treating depression and approved for use (reviewed by Nemeroff et al., 2006). This suggests the possible commonality of the VNS mechanism in epilepsy and depression.
Ventral hippocampus also possesses connections with the regions of hypothalamus that regulate the sleep–wake cycle, namely the suprachiasmatic and dorsomedial hypothalamic nuclei (Cenquizca and Swanson, 2007; Kishi et al., 2000; Krout et al., 2002; Saper et al., 2005). Interestingly, there are several factors related to sleep that could potentially play a direct role in TLE. The intrinsically photosensitive retinal ganglion cells (ipRGCs), a class of RGCs containing the photosensitive pigment, melanopsin, have a fundamental function in circadian rhythm in causing the release of melatonin in the dark through a separate pathway that does not contribute to image formation (Mure, 2021). This is via their projection to the suprachiasmatic nucleus (SCN), which in turn can send these signals on to the hippocampus through the known connections between the SCN and the medial septum (Ruby et al., 2008, 2013; Snider et al., 2018) and between the medial septum and hippocampus (Castle et al., 2005).
Intrinsically photosensitive retinal ganglion cells (ipRGCs) have their peak sensitivity in the blue part of the light spectrum, which is consistent with the seizure-preventing effects of blue light in photosensitive epilepsy (reviewed by Fisher et al., 2022). The role of the SCN was recently highlighted by Liang et al. (2024) who demonstrated that either SCN lesions or knocking out a clock gene Bmal1 in the SCN led to an increase of seizure frequency in a mice model, as well as leading to morphological damage and alterations of GABAergic signaling in hippocampus. Core clock genes such as CLOCK, Bmal1, PER, and CRY regulate circadian rhythms, which influence the timing and occurrence of seizures in many types of epilepsy (Li et al., 2017; Castro et al., 2018; Jin et al., 2020; Kreitlow et al., 2022; Niu et al., 2025). In kainic acid-induced temporal lobe seizures, CRY1 and CLOCK were found to be dysregulated in the hippocampus (Casillas-Espinosa et al., 2023; Matos et al., 2020). The SCN, as the central circadian pacemaker, coordinates the expression of clock genes throughout the body, including in brain regions prone to seizures. This regulation modifies neuronal excitability in a time-dependent manner, potentially affecting seizure susceptibility (Gerstner et al., 2012; Chan and Liu, 2021).
Although the hippocampus has its own mechanisms of circadian regulation that influence memory functioning over a sleep–wake cycle, the suprachiasmatic nucleus is known to function as a “master clock,” providing phase setting of circadian rhythms of many structures throughout the brain (Mohawk et al., 2012), including in the hippocampus (Snider et al., 2018). This is conducted via both endocrinal and neuronal pathways. The main endocrine mechanism is the regulation of corticosterone production of the adrenal gland by the SCN and the dependence of hippocampal circadian phase on corticosterone levels. Cortisol levels have been linked to both circadian and ultradian (i.e., recurrent periods within a day, as during sleep) rhythmicities (Niu et al., 2025). The neural pathway regulating circadian phase of the hippocampus connects the SCN with hippocampus via the medial septum, as described earlier. This ultimately modulates the balance of excitation and inhibition in the hippocampus, potentially altering neural activity and synaptic plasticity (Cirelli, 2013; Wu et al., 2022). Medial septum receives inhibitory GABA-ergic signals from the SCN during subjective night phase (Ruby et al., 2008, 2013; Snider et al., 2018). In the absence of cholinergic support from the inhibited septal areas, the hippocampus switches from tonic to phasic type from its own local GABA-dependent inhibition (Wu et al., 2022). Such reduction of tonic GABA-ergic inhibition and increase of phasic inhibition is known to support an oscillatory type of neuronal activity (Farrant and Nusser, 2005), which is typical during slow wave sleep.
Notably, vagal input into the hippocampus is also transmitted via the medial septum (Castle et al., 2005; Suarez et al., 2018). This pathway thus links the major brainstem input area receiving vagal afferents, namely the nucleus of the solitary tract (NTS), to the hippocampus. We hypothesize that a confluence of signals during sleep can contribute to increased visceral oscillatory input to the hippocampus during sleep. We suggest that medial septum is a structure that provides gating of the vagal inputs based on the information coming from the SCN, increasing the strength of vagal influence during the night when it is dark and attenuating it during daytime.
Another brain area involved in regulation of hippocampal activity and in interoception is the locus coeruleus (LC), the main noradrenergic center of the brain. LC receives input from the vagus nerve via the NTS and in turn sends projections to various brain regions, including the hippocampus, amygdala, thalamus and neocortical areas and is known to be involved in processing and modulating interoceptive information (Tsetsenis et al., 2023; Fornai et al., 2011).
LC activation has been shown to modulate hippocampal synaptic plasticity. Stimulation of the LC facilitates long-term depression (LTD) in the dentate gyrus of the hippocampus, which is dependent on β-adrenergic receptors (Lemon et al., 2009). This LC-induced LTD may play a role in selecting salient information for subsequent synaptic processing in the hippocampus (Lemon et al., 2009). Since many interoceptive signals are of importance to the animal’s survival, they are likely to be salient on many occasions. Furthermore, VNS activates the NTS, which in turn activates LC neurons, promoting the release of noradrenaline throughout the brain including the hippocampus and the potential induction of long-term plastic changes such as those observed after prolonged VNS application (Fornai et al., 2011). LC can contribute to neuroplastic changes in the hippocampus and the control of arousal in it (Berridge and Foote, 1991). With the emerging view that epileptogenesis may involve the disturbance of normal NREM sleep-related homeostatic plasticity (Halász et al., 2019), neuroplasticity has become an important contemporary target for anti-seizure treatments (Asim et al., 2024a, b; Ma et al., 2024; Waris et al., 2024),
We have described previously increased propagation of visceral information to various cortical areas during sleep (Pigarev, 1994; Pigarev et al., 2013; Pigarev and Pigareva, 2013) and more recently we have also reported a striking increase in the responsiveness of cells in the insula to visceral stimulation during sleep (Levichkina et al., 2021) as well as a dependency of hippocampal synchronization to visceral signals on the state of vigilance (Vidyasagar et al., 2022). Gastric activity, which is communicated to the brain by vagal afferents, is also partly synchronized with the activity during resting state in many areas of the brain including the limbic system (Rebollo et al., 2018; Cao et al., 2022). It has also been recently demonstrated that shorter sleep duration in humans is associated with increased sympathetic activity, thus contributing to increased risk of hypertension and cardiovascular disease in people with shortened sleep (Tai et al., 2023). Rembado et al. (2021) demonstrated substantial increase of VNS responses during slow wave sleep (SWS) in multiple cortical areas in primates. This indicates that there are more pronounced vagal inputs during sleep in comparison to waking hours. The disturbance of such inputs caused by poor sleep might be the source of comorbidities between shortened sleep and cardiovascular diseases (Forshaw et al., 2022; Tai et al., 2023).
One cause for the increased visceral afferent signals during sleep may itself be due to increased parasympathetic stimulation of the internal organs from the vagal complex, which, in turn, receives a significant modulation from the suprachiasmatic nucleus (Ferini-Strambi and Smirne, 1997; Mutoh et al., 2003; Buijs et al., 2001; Cabiddu et al., 2012; De Zambotti et al., 2018; Karemaker, 2022). The light related changes in autonomic regulation are known to be pronounced and are mediated through the SCN, since SCN ablation aborts the autonomic responses to light (Mutoh et al., 2003). Thus, the changes in ipRGC signals from the eye in the sleep/wake cycle may be modulating the circadian signals to the vagal complex via the SCN.
As described above, there may be a number of ways in which the likelihood of seizures happening during sleep increases. If the modulation of visual signals between day and night has a significant influence on triggering TLE, it has significant implications for preventing seizures. The above-described link between visual system and epileptogenesis may also be a critical area for future investigations.
Paroxysmal activity during sleep and TLE
The association between slow wave activity and epileptic discharges and the possibility that they may share the same mechanisms have been highlighted by Beenhakker and Huguenard (2009). There is a strong prevalence of paroxysmal activity during SWS and in drowsy states in contrast to wakefulness and REM. Roughly half of all seizures occur during SWS or drowsy states despite these states occupying less than a third of the whole sleep–wake cycle of 24 hours (e.g., Shouse et al., 1996; Herman et al., 2001; Dinner, 2002; Combi et al., 2004; Pavlova et al., 2004; Hofstra and de Weerd, 2009; Chokroverty and Nobili, 2017). Temporal lobe seizures occur more frequently during drowsiness and early stages of sleep, becoming less frequent during REM sleep (Herman et al., 2001). Furthermore, although seizures in TLE occur at equal frequency in wakefulness and sleep, as many as 75% of drug-resistant patients suffer from daytime sleepiness, reporting more frequent naps during daytime (Zanzmera et al., 2012). Thus, correct estimation of the seizure frequencies as per state of vigilance is problematic in TLE patients. Focal epilepsy seems to be provoked by sleep and sleep deprivation leads to hyperexcitability of hippocampal networks. SWS predisposes to the development of interictal epileptiform discharges in TLE and to generalization of seizure activity (for a systematic review see Garg et al., 2022). The burst-firing mode of SWS, which normally produces sleep spindles, can turn into an epileptic working mode in mTLE (Halász and Szűcs, 2020). In mTLE, there is evidence for local wake slow waves (LoWS) that share key features with slow waves in sleep (Sheybani et al., 2023). The hypersynchrony and interictal spikes present during SWS may facilitate the onset or spread of partial seizures in mTLE (Sammaritano et al., 1991; Malow et al., 1998).
TLE often shows high amplitude spikes lasting for 50–100 ms, followed by a slow wave lasting for 200–500 ms. Such events are common in SWS, when they propagate to other areas more easily due to the increased slow wave synchronization (Mendes et al., 2019). Another stereotypical activity is a “sharp wave-ripple” complex consisting of a ~ 100 ms wave followed by a high frequency ripple, initiated by synchronized activity of CA3 pyramidal neurons. The sharp wave-ripples (SPW-Rs) in the hippocampus occurring during SWS are known to be essential for memory processing and synaptic plasticity. However, an increase of the ripple frequency potentially transform the SPW-Rs into epileptic spikes, thus causing the characteristic “fast ripples” in epilepsy (Staba et al., 2007; Maier and Kempter, 2017). Recent studies have demonstrated the mechanism of the natural ripple control during sleep. It involves cholecystokinin-expressing basket cells in the hippocampus (Karaba et al., 2024; Amin, 2024). Cholecystokinin (CCK) is known to increase neuronal activity in the hippocampus (Asim et al., 2024a). Furthermore, in the hippocampus, CCK immunoreactivity has been found to coexist with GABA (Somogyi et al., 1984) and CCK-induced hypersensitivity in the auditory system has been linked to sound-triggered seizures (Fedotova et al., 2021). CCK receptors have extensive presence in the brain, including hippocampus, amygdala and the cortex. They modulate both GABA and glutamatergic systems and affect paroxysmal activity (Asim et al., 2024a, b). CCK enhances the inhibitory tone provided by hippocampal GABAergic neurons by increasing GABA release (Miller et al., 1997). Such modulation of GABAergic activity by CCK may contribute to sleep regulation and hippocampal slow wave activity, as GABAergic neurons play a critical role in promoting SWS (Saper and Fuller, 2017) and in regulation of hippocampal oscillatory behavior (Földy et al., 2007; Whissell et al., 2015; Klausberger et al., 2004).
Synchronous activity of hippocampal CA3 cells is also inhibited by adenosine, which builds up during waking hours and whose levels drop by at least 20% during SWS in comparison to wakefulness (Basheer et al., 2004). This increases the probability of CA3 neurons to ovesynchronize during SWS, since both ripples and fast ripples occur mostly during sleep.
Visceral triggers of hippocampal paroxysmal activity
The question now arises as to what may facilitate the slow wave oversynchronization that is expected to drive susceptible ventral hippocampal circuits into paroxysmal activity. A highly plausible candidate that we propose is the afferent rhythmic signaling coming from visceral systems. Breathing, heart rate, gastric and intestinal activities are all rhythmic. Hippocampal breathing rhythm is a particularly well-known phenomenon and the hippocampal sharp wave-ripple complex can be entrained by respiration (Liu et al., 2017; Lockmann et al., 2016; Radna and MacLean, 1981; Bordoni et al., 2018). This breathing-associated rhythmic activity is also present in prefrontal cortex, especially in areas connected with the olfactory system. This provides a ground for synchronization of large brain networks by breathing due to their extensive connections to the hippocampus. Frequency of the hippocampal breathing rhythm is different in different species, with the one reported in human epileptic patients being around 0.16–0.33 Hz (Zelano et al., 2016) while, in smaller animals, this rhythm is faster and can be mistaken for delta or theta activity (Lockmann et al., 2016). In a study on the relationship between visceral events such as heart rate and breathing with hippocampal cell responses in human patients, a large proportion of neurons in hippocampus and amygdala were found to either synchronize with the heart rate itself (20%) or respond to changes in heart rate (23%), in addition to 15% of cells being synchronized to the respiratory period (Frysinger and Harper, 1989). Neuronal responses associated with cardiac rhythm were studied in human subjects by Kim et al. (2019), who confirmed their presence during the resting state in hippocampus, parahippocampal cortical areas, amygdala, and the cingulate cortex.
Our framework is consistent with the many studies that have reported comorbidities between epilepsy and cardiac and respiratory illnesses (e.g., Doherty et al., 2022; Gaitatzis et al., 2004). Cardiovascular disorders which can potentially cause abnormal rhythms such as atrial fibrillation and myocardial infarction show significant comorbidity with epilepsy (Doherty et al., 2022). Respiratory rhythm is of particular interest due to its connection to Sudden Unexpected Death in Epilepsy (SUDEP), which predominantly occurs in sleep (Purnell et al., 2018; Niu et al., 2025). There is a strong association between epilepsy and sleep-related respiratory conditions such as obstructive and central sleep apnoeae. Obstructive sleep apnoea (OSA) occurs in 10% of adult epilepsy patients, 20% of children with epilepsy and up to 30% of drug-resistant epilepsy cases (Manni and Terzaghi, 2010; Zanzmera et al., 2012; Sivathamboo et al., 2019), while treatment with continuous positive airway pressure (CPAP) has been shown to improve seizure control in some cases (Malow et al., 2008). Patients with TLE have been reported to have a higher risk of obstructive sleep apnoea as well (Yildiz et al., 2015). However, the most severe comorbidity exists between TLE and central sleep apnoea, particularly ictal central apnoea (ICA). ICA has a higher prevalence in TLE compared to extratemporal epilepsy. It was observed in 36.9% of seizures and 43.2% of patients with focal epilepsy, all of whom had temporal lobe involvement (Lacuey et al., 2018; Lacuey et al., 2019; Vilella et al., 2019). In a study of patients with mTLE, the incidence of ICA was reported to be as high as 68.7% (Vilella et al., 2019). Thus, the association between TLE and central apnoea appears to be often related to the involvement of temporal lobe structures in respiratory control. There is also comorbidity between bronchial asthma and epilepsy. However, it varies across studies, ranging between 9 and 13.4% (Gaitatzis et al., 2004; Téllez-Zenteno et al., 2005; Chiang et al., 2018; Doherty et al., 2022). It is also interesting that, at 22%, the condition having the highest comorbidity with epilepsy is the group of anxiety disorders (Doherty et al., 2022). A typical and common symptom in anxiety disorders is hyperventilation. It is notable that hyperventilation, used as an activation method to provoke interictal discharges and seizures during video-EEG monitoring of epilepsy patients is more effective in temporal lobe patients (Guaranha et al., 2005).
When the activity in those with TLE falls roughly into the delta frequency range and seems to be correlated with cardiac activity, it is possible that oversynchronisation may lead to epileptic seizures. However, this raises a few issues: (i) In smaller animals, which usually tend to have faster heart rates, higher frequency ranges would be expected to become oversynchronised, which may not be the case. (ii) In the over 50% of the cases where no correlation with cardiac or breathing rhythm is seen, is there another rhythmic activity that could provide the trigger for seizures?
The above leads to the possibility that rhythmic signals from abdominal viscera may set off an over-synchronization. Hippocampus is not only known to receive vagal inputs as described earlier, but it is also engaged in organization of feeding behavior and has been shown to receive signals from the gastrointestinal tract, with neurons in the macaque hippocampus and parahippocampus showing responses related to food reward (Vidyasagar et al., 1991; Tamura et al., 1991; Salzman et al., 1993). Positron Emission Tomography (PET) studies in humans have also revealed hippocampal responses to gastric stimulation (Wang et al., 2006). We have recently reported a coupling between gastrointestinal myoelectric activity and the spiking of hippocampal cells (Vidyasagar et al., 2022). Furthermore, it was shown that vagal afferents that transmit information from the stomach to the brain may act as a peripheral clock themselves and that mechanosensitivity of these vagal afferents changes over a 24-hour period, indicating that their responses to mechanical stimuli are not static but exhibit circadian variation (Kentish et al., 2013).
Serotonin and gut peptides such as CCK, peptide-YY and leptin released in response to gastric distention provoke satiety and somnolence via activation of the vagus nerve (Kim and Lee, 2009). Thus, though CCK does not cross the blood–brain barrier, it affects neural activity in the brain via the vagus nerve (Cao et al., 2012). Furthermore, capillary endothelial cells of the blood–brain barrier expressing CCK-1 receptors change their permeability to other molecules including leptin in response to CCK (Cano et al., 2008). Leptin plays a significant role in hippocampal neuroplasticity, affecting multiple molecular pathways involved in synaptic changes (Li et al., 2002; Moult and Harvey, 2011; Dhar et al., 2014; McGregor et al., 2018). It facilitates LTP in the CA1 region and the dentate gyrus, which are believed to underlie learning and memory formation (Shanley et al., 2001; Oomura et al., 2006). It also influences excitatory synaptic transmission in the hippocampus. In adult tissue, it induces a persistent increase in excitatory synaptic transmission, termed leptin-induced LTP (Li et al., 2002). Interestingly, intraperitoneal CCK injections led to increase of EEG slow wave activity and sleep (Kapás et al., 1988). The above studies indicate a strong association between increased plasma CCK levels and TLE (Gao and Bao, 2020).
Thus, there are multiple ways in which gastrointestinal events can potentially modulate hippocampal activity and there is reason to believe that rhythmic gastrointestinal events have different patterns in different states of vigilance. Some of these patterns could also lead to rhythmic activity in the hippocampus, potentially triggering seizures. We propose that all the variants of TLE, including those where correlation or modulation with easily recordable visceral events such as breathing or heart rate are not observed, share essentially the same mechanism of being susceptible to be triggered by interoceptive slow waves. We suggest that networks susceptible to paroxysmal activity that include the ventral hippocampus may be triggered by any of the afferent visceral signals, including those arising from the gastrointestinal tract.
Autonomic disturbances accompany TLE seizures in up to 75% of cases and are frequent during the aura period (van Buren and Ajmone-Marsan, 1960). Ictal autonomic changes include cardiorespiratory, gastrointestinal, vascular, urogenital and pupillary symptoms (Dütsch et al., 2006). The most commonly reported seizure triggers relate to particular visceral states such as menstruation, sleep deprivation, fatigue, eating, fever, et cetera or to stress and anxiety (Lunardi et al., 2011, 2016), indicating that such changes do not just accompany, but may also induce paroxysmal activity.
External modulation of visceral triggers of hippocampal paroxysmal activity
Externally driven seizure events are not uncommon and reflex epilepsy is a well-known example of the epileptiform activity triggered by sensory stimuli. Seizures in TLE can be provoked by rhythmic olfactory stimulation (Lunardi et al., 2016), mediated by strong projections from the olfactory system to hippocampus. Olfactory auras also occur in drowsy/dreaming states in 6% of TLE patients. TLE-associated, ‘eating epilepsy’ has been described as well (Nagaraja and Chand, 1984). Here, sight, smell or thought of food did not cause seizures and they only rarely ever occurred during eating itself. In most cases, the seizures occurred at the end of a heavy meal, suggestive of the higher gastrointestinal activity associated with digestion as the trigger and partial control of seizures was achieved simply by changing the eating habits. Another example of epileptic activity associated with gastrointestinal function is the seizure resulting from gastroenteritis in children (Cusmai et al., 2010). Irritable bowel syndrome also increases the risk to develop epilepsy (Chen et al., 2015). Our proposal provides a neural framework for these phenomena.
Figure 2 summarizes our model of changes of functional connectivity that predispose to facilitation of seizures originating in the ventral hippocampus during the dark (night) phase of the sleep–wake cycle (right panel) in comparison to their relative suppression during the light (day) phase (left panel). During the light phase, signals from the ipRGC stimulate neurons in the SCN, which leads to reduction of the SCN suppression of the medial septum, which is known to project to the hippocampus (Ruby et al., 2008; Snider et al., 2018). The medial septum supplies cholinergic support to hippocampus and also causes hippocampal cells to receive inhibition in a tonic mode (Kalsbeek et al., 2006; Wu et al., 2022). However, during the night, in the absence of light, ipRGCs stop sending their signals to SCN, which suppresses medial septum via GABA-ergic connections. Thus, during night, there is a decrease in the cholinergic input from the medial septum to hippocampus and as a result, hippocampal cells are likely to switch to the phasic inhibition mode (Wu et al., 2022). We hypothesize that the vagal input which comes to the medial septum from NTS is also disinhibited during the night. In addition, vagal and general parasympathetic activity are more pronounced during slow wave, non-rapid eye movement (NREM) sleep (Trinder et al., 2001; Cabiddu et al., 2012; De Zambotti et al., 2018). Thus, during slow wave sleep, enhanced and potentially disinhibited vagal signals have higher chances of influencing ventral hippocampus. The other structures receiving vagal input can also be vulnerable to seizures triggered by interoceptive signals, especially the insular, anterior cingulate, orbitofrontal, somatosensory and motor cortices. One particularly striking example is frontal lobe epileptic seizures that consistently show predisposition to occur during sleep (Herman et al., 2001). Furthermore, a strong association exists between frontal lobe seizures and NREM sleep. Nobili et al. (2012) reported that in patients with nocturnal frontal lobe epilepsy (NFLE), 98% of all seizures occurred in NREM sleep. Of these NREM seizures, 72% emerged from slow wave sleep (SWS). Many frontal lobe seizures also originate from the primary motor cortex or the orbitofrontal cortex (Wang et al., 2019; Herman et al., 2001), which are areas that receive vagal inputs.
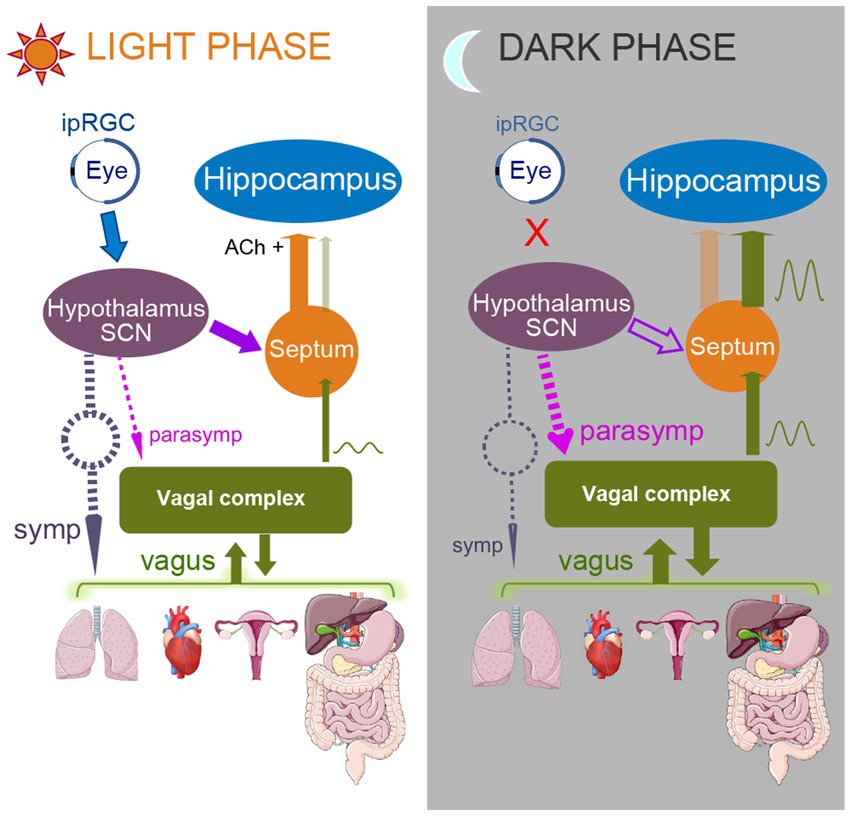
Figure 2. Model of functional connectivity changes occurring upon transition from light to dark phase of a sleep–wake cycle. Left panel represents subjective light phase, and right panel represents subjective dark phase. During the light phase, ipRGCs of the retina are activated by light and send their signals to SCN. SCN stops producing melatonin, thus enhancing sympathetic activity (dashed gray arrow). As a result, predominantly parasympathetic vagal activity (dashed purple and green arrows) is attenuated. SCN also does not inhibit medial septum during the light phase; that allows disinhibited septal areas to send cholinergic signals to the hippocampus (orange arrow, Ach+), promoting arousal and supporting tonic type of hippocampal inhibition. Medial septum also “closes the gate” for the vagal signals, reducing chances for hippocampal triggering by the vagal input. In contrast, during the dark phase, when SCN does not receive ipRGC input, it increases melatonin production, supporting parasympathetic activity (dashed purple arrows) and so the vagal activity is enhanced. SCN also inhibits medial septum (open purple arrow), which decreases the medial septum’s cholinergic input to the hippocampus, causing switching of the hippocampus to a phasic inhibition mode with increased susceptibility to low frequency entrainment. Medial septum also “opens the gate” for the vagal signals, thus increasing the influence of the visceral activity on hippocampus and allowing the triggering of paroxysmal responses to occur.
However, the hippocampal network is especially vulnerable to seizures due to multiple factors such as heightened risk of sclerosis, high density of glutamate receptors that increases chances of excitotoxicity and neuronal hyperexcitability, enhanced blood–brain barrier permeability compared to other brain regions, which makes it more susceptible to circulating toxins and pathogens causing inflammation, increased oxygen demand leading to higher sensitivity to hypoperfusion and low oxygen saturation (Sloviter, 1987; Olney et al., 1986; Marchi et al., 2007; Wei et al., 2014; Montagne et al., 2015; Mitra et al., 2016).
Mechanism of visceral trigger of TLE seizures
There is a case for arguing that slow waves such as from the viscera (heart and lungs and the gastrointestinal tract) can induce seizure activity which is usually at higher frequencies. Slowing of EEG activity with irregular delta waves occurs in over 60% of TLE patients (Jan et al., 2010). Stimulation frequencies in a low range, such as 1 Hz, are used for pre-operative testing aimed to provoke epileptic seizures to define their source. This stimulation induces seizures when applied to the hippocampus and related structures of the hippocampal gyrus and propagate in ways that match seizure propagation (Corcoran and Cain, 1980; Munari et al., 1993). It seems likely that natural visceral activity occurring at comparable low frequencies, such as cardiac rhythms, can also initiate the same processes through resonance.
The frequency range of oscillatory activities, both normal and ictal, is determined by the biophysical properties of the morphological cell types of the oscillating cell assembly and the balance of excitation and inhibition on the cells of the assembly (Hutcheon and Yarom, 2000; Economo and White, 2012; Markram et al., 2004). These cell assemblies, by virtue of the circuitry they are embedded in, would also have a resonant frequency to which they are most susceptible (Hutcheon and Yarom, 2000; Herrmann, 2001). This has been pointed out in the general context of communication through coherence between brain regions (Vidyasagar, 2013; Esghaei et al., 2022) and the concept is extended more specifically in its potential to trigger ictal activity (Pigarev et al., 2020; Sohanian Haghighi and Markazi, 2019). Thus, when there is sufficient overlap between the frequency ranges of the interoceptive wave and the local hippocampal oscillatory activity to trigger the resonant frequency in the hippocampal circuit, a typical ictal over-synchronization can potentially occur. Such overlap and over-synchronization at the resonant frequency of the hippocampal circuit may be the result of a widening of the frequency bandwidth of one or both circuits, thanks to an abnormal hippocampal circuit from a lesion and/or a faster than normal visceral oscillation. Consistent with this idea, dependence of stimulation treatment outcomes on its frequency, with low frequencies being pro-convulsive while high frequencies being anti-convulsive, has been demonstrated for both deep brain stimulation and spinal stimulation approaches to epilepsy (Waris et al., 2024).
However, it still remains to be explained how the slow interoceptive waves (mostly <1 Hz, e.g., breathing or gastrointestinal activity) lead to the ictal over-synchronization that occur at higher frequencies and the spread of the seizure from the hippocampus to various cortical areas. For example, patients with TLE often have “sinusoidal” patches of ictal activity at frequencies of 5–10 Hz (Jan et al., 2010), and fast ripples occurring at very high frequencies (>150 Hz) are characteristic of hippocampal epileptiform activity (Foffani et al., 2007; Ogren et al., 2009).
One possibility is the occurrence of resonance at harmonics of the fundamental frequency, as was pointed out earlier (Pigarev et al., 2020). Thus, in a study of photosensitive epilepsy when photic stimulation was applied at 10–20 Hz, synchronized activity at harmonically related frequencies occurred within 30–120 Hz (Parra et al., 2003). The other option has been recently described by Stark et al. (2022). In their experiment, hippocampal pyramidal cells in CA1 were optically stimulated at 5 Hz, which resulted in slow depolarization and oscillatory response at frequencies between 60 and 80 Hz. This was followed by application of linear chirp stimulation (sinusoidal waveform stimulation with frequency changing over time linearly from 0 to 100 Hz), which revealed a resonant peak of a cell to occur at 40 Hz. Thus, high frequency oscillations can result from stimulation well outside of the cell’s resonant peak, and a neuronal oscillator does not have to be a resonator to show evoked oscillations.
Yet another and likely a more common mechanism is cross-frequency coupling, which has been suggested as an efficient means of communication between brain areas (Canolty and Knight, 2010; Lisman and Jensen, 2013; Vidyasagar and Levichkina, 2019; Esghaei et al., 2022). CFC can be either amplitude-amplitude coupling, when the two frequencies are similar and oscillation in area A directly adds to the oscillation in its target area B, or phase-amplitude coupling, when the phase of lower frequency oscillation in area A modulates the amplitude of a local higher frequency oscillation in area B. Normal hippocampal ripples are known to be entrained by breathing (Liu et al., 2017; Lockmann et al., 2016; Radna and MacLean, 1981; Bordoni et al., 2018; Nokia and Penttonen, 2022). As pathological fast ripples in the hippocampus have similar origin as the normal ripples (Foffani et al., 2007), it seems safe to assume that CFC-based entrainment of respiration or other low frequency visceral events can cause fast ripples as well. Figure 3 schematically compares the influence of two potential mechanisms of seizure-triggering on the ongoing hippocampal oscillations.
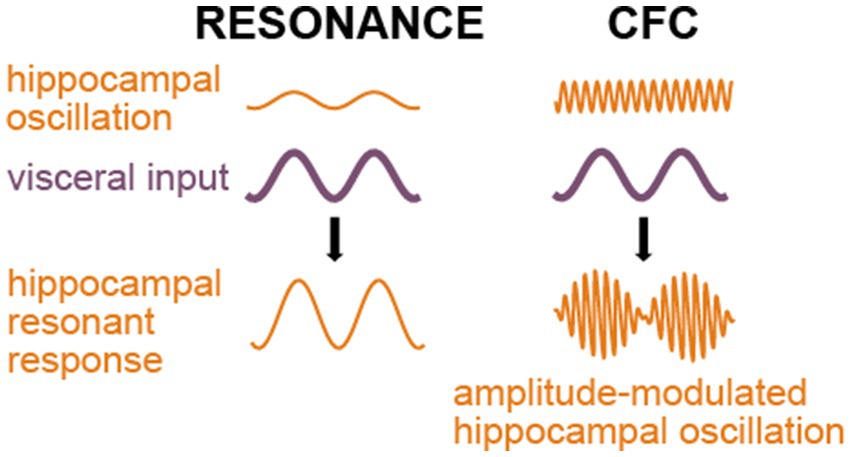
Figure 3. Outcomes of the resonant and phase-amplitude CFC mechanisms of seizure triggering. Both mechanisms require visceral input (purple); however, the resonance mechanism (left side of the picture) implies similarity between frequencies of the hippocampal oscillation and the visceral input or a harmonic relationship between them, while phase-amplitude CFC (right side) leads to an amplitude modulation of any prevailing higher-frequency hippocampal activity.
That the visceral waves usually have a frequency range that barely overlaps with the theta, beta and gamma frequencies typically seen in most of the brain regions, including the hippocampus, explains why seizures are not easily triggered, at least during waking hours when the vagal afferent activity from gastrointestinal tract is less (Pigarev, 1994; Pigarev et al., 2013; Rembado et al., 2021; Levichkina et al., 2021; Levichkina et al., 2022). However, we suggest that, with an altered abnormal hippocampal circuit, this protection is lost, especially during sleep, when the visceral oscillations are stronger.
The next question to address is the spread of activity from the hippocampus to other brain regions, such as the frontal cortex, which receives a strong projection from the hippocampus (Barbas and Blatt, 1995; Thierry et al., 2000; Catenoix et al., 2011), sometimes leading to generalized convulsions. CFC-mediated spread of hippocampal seizure may be further augmented by the claustrum, consistent with the proposed function of the claustrum with a unique morphology and connectivity that enables it to enhance neural synchrony between brain areas (Vidyasagar and Levichkina, 2019; Madden et al., 2022). The claustral involvement is also consistent with the finding that during sleep, the slow wave activity is enhanced through claustral projections to widespread neocortical areas (Narikiyo et al., 2020). It has been pointed out that the claustral projections are uniquely organized to rapidly abort the enhanced neural synchrony between cortical areas (Vidyasagar and Levichkina, 2019). This could potentially explain why such augmentation of synchrony does not usually cause seizures and why claustral damage, on the other hand, can sometimes cause seizures (Meletti et al., 2017).
Implications for therapy
The above account of the role of the vagus in transmitting the visceral signals to the brain, including the hippocampus, provides a basis for the efficacy of vagal nerve stimulation (VNS) in controlling seizures in many cases of pharmacoresistant epilepsy. VNS stimulation can potentially desynchronise the hippocampal network that is causing, or about to cause, a seizure (Pigarev et al., 2020). In that case, there is no need for VNS to desynchronize the whole cortex to be effective. In fact, consistent with our framework, studies show that patients who respond favorably to VNS in comparison to non-responders exhibit more heterogeneity in the brain areas where desynchronisation in the theta band EEG is most pronounced (Vespa et al., 2021; Clifford et al., 2024). Furthermore, stronger desynchronisation in the theta band during sleep compared to wakefulness was found to distinguish VNS responders from non-responders (Vespa et al., 2021).
From the above point of view, we suggest both VNS and ventral hippocampus stimulation may be effective in seizure prevention. However, VNS implantation has less risk and is, therefore, may be preferable in many cases.
In conclusion, we propose that TLE is largely a reflex type of epilepsy with visceral and external events serving as triggers. These triggers are expected to be more effective in provoking resonant activity and over-synchronization in the hippocampus in sleep and during darker hours. From that point of view, effectiveness of VNS relies on its ability to stop such over-synchronization of the networks involved in the analysis of visceral information.
Data availability statement
The original contributions presented in the study are included in the article. Further inquiries can be directed to the corresponding authors.
Author contributions
EL: Conceptualization, Project administration, Writing – original draft, Writing – review & editing, Visualization. DG: Conceptualization, Funding acquisition, Writing – review & editing. SP: Conceptualization, Funding acquisition, Writing – review & editing. MC: Funding acquisition, Writing – review & editing, Conceptualization. TV: Funding acquisition, Writing – review & editing, Conceptualization, Project administration, Resources, Supervision, Writing – original draft.
Funding
The author(s) declare that financial support was received for the research and/or publication of this article. This project was supported by the Clifford Trust in Neural Engineering and the National Health and Medical Research Council (ID 2027651). Salary support for EL was provided by both sources and the publications charges by the Clifford Trust in Neural Engineering.
Acknowledgments
Ivan Pigarev is gratefully acknowledged by all the authors for his original contribution of the basic idea and for drafting the first version of the manuscript prior to his unfortunate death on 15 July 2021.
Conflict of interest
The authors declare that the research was conducted in the absence of any commercial or financial relationships that could be construed as a potential conflict of interest.
The author(s) declared that they were an editorial board member of Frontiers, at the time of submission. This had no impact on the peer review process and the final decision.
Generative AI statement
The authors declare that no Gen AI was used in the creation of this manuscript.
Publisher’s note
All claims expressed in this article are solely those of the authors and do not necessarily represent those of their affiliated organizations, or those of the publisher, the editors and the reviewers. Any product that may be evaluated in this article, or claim that may be made by its manufacturer, is not guaranteed or endorsed by the publisher.
References
Akaike, K., Tanaka, S., Tojo, H., Fukumoto, S., Imamura, S., and Takigawa, M. (2001). Kainic acid-induced dorsal and ventral hippocampal seizures in rats. Brain Res. 900, 65–71. doi: 10.1016/s0006-8993(01)02252-1
Amin, H. (2024). Balancing memory in sleep: firing barrages as a circuit breaker for reactivation. Signal Transduct. Target. Ther. 9:328. doi: 10.1038/s41392-024-02057-y
Asim, M., Qianqian, G., Waris, A., Wang, H., Lai, Y., and Chen, X. (2024a). Unraveling the role of cholecystokinin in epilepsy: mechanistic insight into neuroplasticity. Neurochem. Int. 180:105870. doi: 10.1016/j.neuint.2024.105870
Asim, M., Wang, H., and Chen, X. (2024b). Shedding light on cholecystokinin's role in hippocampal neuroplasticity and memory formation. Neurosci. Biobehav. Rev. 159, 105615. doi: 10.1016/j.neubiorev.2024.105615
Austelle, C. W., Cox, S. S., Wills, K. E., and Badran, B. W. (2024). Vagus nerve stimulation: recent advances and future directions. Clin. Auton. Res. 34, 529–547. doi: 10.1007/s10286-024-01065-w
Azzalini, D., Rebollo, I., and Tallon-Baudry, C. (2019). Visceral signals shape brain dynamics and cognition. Trends Cogn. Sci. 23, 488–509. doi: 10.1016/j.tics.2019.03.007
Barbas, H., and Blatt, G. J. (1995). Topographically specific hippocampal projections target functionally distinct prefrontal areas in the rhesus monkey. Hippocampus 5, 511–533. doi: 10.1002/hipo.450050604
Barnett, A. J., Man, V., and McAndrews, M. P. (2019). Parcellation of the hippocampus using resting functional connectivity in temporal lobe epilepsy. Front. Neurol. 10:920. doi: 10.3389/fneur.2019.00920
Basheer, R., Strecker, R. E., Thakkar, M. M., and McCarley, R. W. (2004). Adenosine and sleep-wake regulation. Prog. Neurobiol. 73, 379–396. doi: 10.1016/j.pneurobio.2004.06.004
Beenhakker, M. P., and Huguenard, J. R. (2009). Neurons that fire together also conspire together: is normal sleep circuitry hijacked to generate epilepsy? Neuron 62, 612–632. doi: 10.1016/j.neuron.2009.05.015
Bernhardt, B. C., Hong, S., Bernasconi, A., and Bernasconi, N. (2013). Imaging structural and functional brain networks in temporal lobe epilepsy. Front. Hum. Neurosci. 7:624. doi: 10.3389/fnhum.2013.00624
Berntson, G. G., and Khalsa, S. S. (2021). Neural circuits of Interoception. Trends Neurosci. 44, 17–28. doi: 10.1016/j.tins.2020.09.011
Berridge, C. W., and Foote, S. L. (1991). Effects of locus coeruleus activation on electroencephalographic activity in neocortex and hippocampus. J. Neurosci. 11, 3135–3145. doi: 10.1523/JNEUROSCI.11-10-03135.1991
Bonne, O., Vythilingam, M., Inagaki, M., Wood, S., Neumeister, A., Nugent, A. C., et al. (2008). Reduced posterior hippocampal volume in posttraumatic stress disorder. J. Clin. Psychiatry 69, 1087–1091. doi: 10.4088/JCP.v69n0707
Bordoni, B., Purgol, S., Bizzarri, A., Modica, M., and Morabito, B. (2018). The influence of breathing on the central nervous system. Cureus. 10:e2724. doi: 10.7759/cureus.2724
Bragdon, A. C., Taylor, D. M., and Wilson, W. A. (1986). Potassium-induced epileptiform activity in area CA3 varies markedly along the septotemporal axis of the rat hippocampus. Brain Res. 378, 169–173. doi: 10.1016/0006-8993(86)90300-8
Buijs, R. M., Chun, S. J., Niijima, A., Romijn, H. J., and Nagai, K. (2001). Parasympathetic and sympathetic control of the pancreas: a role for the suprachiasmatic nucleus and other hypothalamic centers that are involved in the regulation of food intake. J. Comp. Neurol. 431, 405–423. doi: 10.1002/1096-9861(20010319)431:4<405::aid-cne1079>3.0.co;2-d
Cabiddu, R., Cerutti, S., Viardot, G., Werner, S., and Bianchi, A. M. (2012). Modulation of the sympatho-vagal balance during sleep: frequency domain study of heart rate variability and respiration. Front. Physiol. 3:45. doi: 10.3389/fphys.2012.00045
Canolty, R. T., and Knight, R. T. (2010). The functional role of cross-frequency coupling. Trends Cogn. Sci. 14, 506–515. doi: 10.1016/j.tics.2010.09.001
Cano, V., Merino, B., Ezquerra, L., Somoza, B., and Ruiz-Gayo, M. (2008). A cholecystokinin-1 receptor agonist (CCK-8) mediates increased permeability of brain barriers to leptin. Br. J. Pharmacol. 154, 1009–1015. doi: 10.1038/bjp.2008.149
Cao, B., Zhang, X., Yan, N., Chen, S., and Li, Y. (2012). Cholecystokinin enhances visceral pain-related affective memory via vagal afferent pathway in rats. Mol. Brain 5:19. doi: 10.1186/1756-6606-5-19
Cao, J., Wang, X., Chen, J., Zhang, N., and Liu, Z. (2022). The vagus nerve mediates the stomach-brain coherence in rats. NeuroImage 263:119628. doi: 10.1016/j.neuroimage.2022.119628
Casillas-Espinosa, P., Yamakawa, G., Lin, R., Mychasiuk, R., and O'Brien, T. (2023). Dysfunction of central and peripheral circadian clock genes in models of absence and temporal lobe epilepsy. AES Abstr. 3:005.
Castle, M., Comoli, E., and Loewy, A. D. (2005). Autonomic brainstem nuclei are linked to the hippocampus. Neuroscience 134, 657–669. doi: 10.1016/j.neuroscience.2005.04.031
Castro, O. W., Duzzioni, M., Batista, T. H., Souza, L. C., Covolan, L., Mello, L. E., et al. (2018). Rhythms of core clock genes and spontaneous locomotor activity in post-status epilepticus model of mesial temporal lobe epilepsy. Front. Neurol. 9:632. doi: 10.3389/fneur.2018.00632
Catenoix, H., Magnin, M., Mauguière, F., and Ryvlin, P. (2011). Evoked potential study of hippocampal efferent projections in the human brain. Clin. Neurophysiol. 122, 2488–2497. doi: 10.1016/j.clinph.2011.05.007
Cechetto, D. F., and Saper, C. B. (1987). Evidence for a viscerotopic sensory representation in the cortex and thalamus in the rat. J. Comp. Neurol. 262, 27–45. doi: 10.1002/cne.902620104
Cenquizca, L. A., and Swanson, L. W. (2007). Spatial organization of direct hippocampal field CA1 axonal projections to the rest of the cerebral cortex. Brain Res. Rev. 56, 1–26. doi: 10.1016/j.brainresrev.2007.05.002
Chan, F., and Liu, J. (2021). Molecular regulation of brain metabolism underlying circadian epilepsy. Epilepsia 62, S32–S48. doi: 10.1111/epi.16796
Chase, M. H., Nakamura, Y., Clemente, C. D., and Sterman, M. B. (1967). Afferent vagal stimulation: neurographic correlates of induced EEG synchronization and desynchronization. Brain Res. 5, 236–249. doi: 10.1016/0006-8993(67)90089-3
Chase, M. H., Sterman, M. B., and Clemente, C. D. (1966). Cortical and subcortical patterns of response to afferent vagal stimulation. Exp. Neurol. 16, 36–49. doi: 10.1016/0014-4886(66)90084-7
Chen, C. H., Lin, C. L., and Kao, C. H. (2015). Irritable bowel syndrome increases the risk of epilepsy: a population-based study. Medicine (Baltimore) 94:e1497. doi: 10.1097/MD.0000000000001497
Chiang, K.-L., Kuo, F.-C., Lee, J.-Y., and Huang, C.-Y. (2018). Association of epilepsy and asthma: a population-based retrospective cohort study. PeerJ 6:e4792. doi: 10.7717/peerj.4792
Chokroverty, S., and Nobili, L. (2017). “Sleep and epilepsy” in Sleep Disorders Medicine. ed. S. Chokroverty (New York, NY: Springer), 1–6.
Cirelli, C. (2013). Sleep and synaptic changes. Curr. Opin. Neurobiol. 23, 841–846. doi: 10.1016/j.conb.2013.04.001
Clifford, H. J., Paranathala, M. P., Wang, Y., Thomas, R. H., da Silva Costa, T., Duncan, J. S., et al. (2024). Vagus nerve stimulation for epilepsy: a narrative review of factors predictive of response. Epilepsia 65, 3441–3456. doi: 10.1111/epi.18153
Combi, R., Dalprà, L., Tenchini, M. L., and Ferini-Strambi, L. (2004). Autosomal dominant nocturnal frontal lobe epilepsy—a critical overview. J. Neurol. 251, 923–934. doi: 10.1007/s00415-004-0541-x
Corcoran, M. E., and Cain, D. P. (1980). Kindling of seizures with low-frequency electrical stimulation. Brain Res. 196, 262–265. doi: 10.1016/0006-8993(80)90735-0
Craig, A. D. (2002). How do you feel? Interoception: the sense of the physiological condition of the body. Nat. Rev. Neurosci. 3, 655–666. doi: 10.1038/nrn894
Cusmai, R., Jocic-Jakubi, B., Cantonetti, L., Japaridze, N., and Vigevano, F. (2010). Convulsions associated with gastroenteritis in the spectrum of benign focal epilepsies in infancy: 30 cases including four cases with ictal EEG recording. Epileptic Disord. 12, 255–261. doi: 10.1684/epd.2010.0340
De Zambotti, M., Trinder, J., Silvani, A., Colrain, I. M., and Baker, F. C. (2018). Dynamic coupling between the central and autonomic nervous systems during sleep: a review. Neurosci. Biobehav. Rev. 90, 84–103. doi: 10.1016/j.neubiorev.2018.03.027
Dhar, M., Wayman, G. A., Zhu, M., Lambert, T. J., Davare, M. A., and Appleyard, S. M. (2014). Leptin-induced spine formation requires TrpC channels and the CaM kinase cascade in the hippocampus. J. Neurosci. 34, 10022–10033. doi: 10.1523/JNEUROSCI.2868-13.2014
Diehl, B., and Duncan, J. S. (2015). “Temporal lobe epilepsy” in Epilepsy society teaching weekend. eds. B. Diehl and J. S. Duncan (London: Epilepsy Society), 1–20.
Dinner, D. S. (2002). Effect of sleep on epilepsy. J. Clin. Neurophysiol. 19, 504–513. doi: 10.1097/00004691-200212000-00003
Doherty, A. J., Harrison, J., Christian, D. L., Boland, P., Harris, C., Hill, J. E., et al. (2022). The prevalence of comorbidities in epilepsy: a systematic review. Br. J. Neurosci. Nurs. 18, 98–106. doi: 10.12968/bjnn.2022.18.2.98
Dong, H. W., and Swanson, L. W. (2006). Projections from bed nuclei of the stria terminalis, anteromedial area: cerebral hemisphere integration of neuroendocrine, autonomic, and behavioral aspects of energy balance. J. Comp. Neurol. 494, 142–178. doi: 10.1002/cne.20788
Dorr, A. E., and Debonnel, G. (2006). Effect of vagus nerve stimulation on serotonergic and noradrenergic transmission. J. Pharmacol. Exp. Ther. 318, 890–898. doi: 10.1124/jpet.106.104166
Dütsch, M., Hilz, M. J., and Devinsky, O. (2006). Impaired baroreflex function in temporal lobe epilepsy. J. Neurol. 253, 1300–1308. doi: 10.1007/s00415-006-0210-3
Economo, M. N., and White, J. A. (2012). Membrane properties and the balance between excitation and inhibition control gamma-frequency oscillations arising from feedback inhibition. PLoS Comput. Biol. 8:e1002354. doi: 10.1371/journal.pcbi.1002354
Elliott, R. E. (2011). Efficacy of vagus nerve stimulation over time: review of 65 consecutive patients with treatment-resistant epilepsy treated with VNS > 10 years. Epilepsy Behav. 20, 478–483. doi: 10.1016/j.yebeh.2010.12.042
Elul, R. (1964). Regional differences in the hippocampus of the cat. Electroencephalogr. Clin. Neurophysiol. 16, 470–488. doi: 10.1016/0013-4694(64)90089-6
Englot, D. J., Chang, E. F., and Auguste, K. I. (2011). Vagus nerve stimulation for epilepsy: a meta-analysis of efficacy and predictors of response. J. Neurosurg. 115, 1248–1255. doi: 10.3171/2011.7.jns11977
Esghaei, M., Treue, S., and Vidyasagar, T. R. (2022). Dynamic coupling of oscillatory neural activity and its roles in visual attention. Trends Neurosci. 45, 323–335. doi: 10.1016/j.tins.2022.01.003
Fanselow, M. S., and Dong, H. W. (2010). Are the dorsal and ventral hippocampus functionally distinct structures? Neuron 65, 7–19. doi: 10.1016/j.neuron.2009.11.031
Farrant, M., and Nusser, Z. (2005). Variations on an inhibitory theme: phasic and tonic activation of GABAA receptors. Nat. Rev. Neurosci. 6, 215–229. doi: 10.1038/nrn1625
Fedotova, I. B., Surina, N. M., Nikolaev, G. M., Revishchin, A. V., and Poletaeva, I. I. (2021). Rodent brain pathology, audiogenic epilepsy. Biomedicine 9:1745. doi: 10.3390/biomedicines9111641
Feldman, M. J., Bliss-Moreau, E., and Lindquist, K. A. (2024). The neurobiology of interoception and affect. Trends Cogn. Sci. 28, 643–661. doi: 10.1016/j.tics.2024.01.009
Ferini-Strambi, L., and Smirne, S. (1997). Cardiac autonomic function during sleep in several neuropsychiatric disorders. J. Neurol. 244, S29–S36. doi: 10.1007/bf03160569
Fisher, R. S., Acharya, J. N., Baumer, F. M., French, J. A., Parisi, P., Solodar, J. H., et al. (2022). Visually sensitive seizures: An updated review by the Epilepsy Foundation. Epilepsia 63, 739–768. doi: 10.1111/epi.17175
Foffani, G., Uzcategui, Y. G., Gal, B., and Menendez de la Prida, L. (2007). Reduced spike-timing reliability correlates with the emergence of fast ripples in the rat epileptic hippocampus. Neuron 55, 930–941. doi: 10.1016/j.neuron.2007.07.040
Földy, C., Lee, S. Y., Szabadics, J., Neu, A., and Soltesz, I. (2007). Cell type-specific gating of perisomatic inhibition by cholecystokinin. Nat. Neurosci. 10, 1128–1130. doi: 10.1038/nn1952
Fornai, F., Ruffoli, R., Giorgi, F. S., and Paparelli, A. (2011). The role of locus coeruleus in the antiepileptic activity induced by vagus nerve stimulation. Eur. J. Neurosci. 33, 2169–2178. doi: 10.1111/j.1460-9568.2011.07707.x
Forshaw, P. E., Correia, A. T. L., Roden, L. C., Lambert, E. V., and Rae, D. E. (2022). Sleep characteristics associated with nocturnal blood pressure nondipping in healthy individuals: a systematic review. Blood Press. Monit. 27, 357–370. doi: 10.1097/MBP.0000000000000619
Frey, B. N., Andreazza, A. C., Nery, F. G., Martins, M. R., Quevedo, J., Soares, J. C., et al. (2007). The role of hippocampus in the pathophysiology of bipolar disorder. Behav. Pharmacol. 18, 419–430. doi: 10.1097/FBP.0b013e3282df3cde
Frysinger, R. C., and Harper, R. M. (1989). Cardiac and respiratory correlations with unit discharge in human amygdala and hippocampus. Electroencephalogr. Clin. Neurophysiol. 72, 463–470. doi: 10.1016/0013-4694(89)90222-8
Gaitatzis, A., Carroll, K., Majeed, A., and Sander, J. W. (2004). The epidemiology of the comorbidity of epilepsy in the general population. Epilepsia 45, 1613–1622. doi: 10.1111/j.0013-9580.2004.17504.x
Gao, J.-B., and Bao, M. (2020). Plasma neuropeptide as a prognostic marker of vagus nerve stimulation in the treatment of epilepsy. Brain Stimul. 13, 959–960. doi: 10.1016/j.brs.2020.03.021
Garg, D., Charlesworth, L., and Shukla, G. (2022). Sleep and temporal lobe epilepsy - associations, mechanisms and treatment implications. Front. Hum. Neurosci. 16:849899. doi: 10.3389/fnhum.2022.849899
Gerstner, J. R., Smith, G. G., Lenz, O., Perron, I. J., Buono, R. J., and Ferraro, T. N. (2012). BMAL1 controls the diurnal rhythm and set point for electrical seizure threshold in mice. Front. Syst. Neurosci. 6:18. doi: 10.3389/fnsys.2012.00018
Green, J. R., and Scheetz, D. G. (1964). Surgery of epileptogenic lesions of the temporal lobe. Arch. Neurol. 10, 135–148. doi: 10.1001/archneur.1964.00460140021004
Groves, D. A., and Brown, V. J. (2005). Vagal nerve stimulation: a review of its applications and potential mechanisms that mediate its clinical effects. Neurosci. Biobehav. Rev. 29, 493–500. doi: 10.1016/j.neubiorev.2005.01.004
Guaranha, M. S. B., Garzon, E., Buchpiguel, C. A., Tazima, S., Yacuban, E. M. T., and Sakamoto, A. C. (2005). Hyperventilation revisited: physiological effects and efficacy on focal seizure activation in the era of video-EEG monitoring. Epilepsia 46, 69–75. doi: 10.1111/j.0013-9580.2005.11104.x
Halász, P., Bodizs, R., Ujma, P. P., Fabo, D., and Szucs, A. (2019). Strong relationship between NREM sleep, epilepsy and plastic functions - a conceptual review on the neurophysiology background. Epilepsy Res. 150, 95–105. doi: 10.1016/j.eplepsyres.2018.11.008
Halász, P., and Szűcs, A. (2020). Sleep and epilepsy link by plasticity. Front. Neurol. 11:911. doi: 10.3389/fneur.2020.00911
Herman, J. P., McKlveen, J. M., Ghosal, S., Kopp, B., Wulsin, A., Makinson, R., et al. (2016). Regulation of the hypothalamic-pituitary-adrenocortical stress response. Compr. Physiol. 6, 603–621. doi: 10.1002/cphy.c150015
Herman, J. P., Ostrander, M. M., Mueller, N. K., and Figueiredo, H. (2005). Limbic system mechanisms of stress regulation: hypothalamo-pituitary-adrenocortical axis. Prog. Neuro-Psychopharmacol. Biol. Psychiatry 29, 1201–1213. doi: 10.1016/j.pnpbp.2005.08.006
Hermann, B. P., Seidenberg, M., and Bell, B. (2000). Psychiatric comorbidity in chronic epilepsy: identification, consequences, and treatment of major depression. Epilepsia. 41, S31–S41. doi: 10.1111/j.1528-1157.2000.tb01522.x
Herman, S. T., Walczak, T. S., and Bazil, C. W. (2001). Distribution of partial seizures during the sleep-wake cycle: differences by seizure onset site. Neurology 56, 1453–1459. doi: 10.1212/WNL.56.11.1453
Herrmann, C. S. (2001). Human EEG responses to 1-100 Hz flicker: resonance phenomena in visual cortex and their potential correlation to cognitive phenomena. Exp. Brain Res. 137, 346–353. doi: 10.1007/s002210100682
Hofstra, W. A., and de Weerd, A. W. (2009). The circadian rhythm and its interaction with human epilepsy: a review of literature. Sleep Med. Rev. 13, 413–420. doi: 10.1016/j.smrv.2009.01.002
Hutcheon, B., and Yarom, Y. (2000). Resonance, oscillation and the intrinsic frequency preferences of neurons. Trends Neurosci. 23, 216–222. doi: 10.1016/S0166-2236(00)01547-2
Isaeva, E., Romanov, A., Holmes, G. L., and Isaev, D. (2015). Status epilepticus results in region-specific alterations in seizure susceptibility along the hippocampal longitudinal axis. Epilepsy Res. 110, 166–170. doi: 10.1016/j.eplepsyres.2014.12.009
Jan, M. M., Sadler, M., and Rahey, S. R. (2010). Electroencephalographic features of temporal lobe epilepsy. Can. J. Neurol. Sci. 37, 439–448. doi: 10.1017/S0317167100010441
Jin, B., Aung, T., Geng, Y., and Wang, S. (2020). Epilepsy and its interaction with sleep and circadian rhythm. Front. Neurol. 11:327. doi: 10.3389/fneur.2020.00327
Kalsbeek, A., Palm, I. F., La Fleur, S. E., Scheer, F. A., Perreau-Lenz, S., Ruiter, M., et al. (2006). SCN outputs and the hypothalamic balance of life. J. Biol. Rhythm. 21, 458–469. doi: 10.1177/0748730406293854
Kapás, L., Obál, F. Jr., Alföldi, P., Rubicsek, G., Penke, B., and Obál, F. (1988). Effects of nocturnal intraperitoneal administration of cholecystokinin in rats: simultaneous increase in sleep, increase in EEG slow-wave activity, reduction of motor activity, suppression of eating, and decrease in brain temperature. Brain Res. 438, 155–164. doi: 10.1016/0006-8993(88)91334-0
Karaba, L. A., Boyce, R., Meira, T., Bender, F., Grienberger, C., Tonegawa, S., et al. (2024). A hippocampal circuit mechanism to balance memory reactivation during sleep. Science 385, 738–743. doi: 10.1126/science.ado5708
Karemaker, J. M. (2022). The multibranched nerve: vagal function beyond heart rate variability. Biol. Psychol. 172:108378. doi: 10.1016/j.biopsycho.2022.108378
Karoly, P. J., Goldenholz, D. M., Freestone, D. R., Moss, R. E., Grayden, D. B., Theodore, W. H., et al. (2018). Circadian and circaseptan rhythms in human epilepsy: a retrospective cohort study. Lancet Neurol. 17, 977–985. doi: 10.1016/S1474-4422(18)30274-6
Karoly, P. J., Ung, H., Grayden, D. B., Kuhlmann, L., Leyde, K., Cook, M. J., et al. (2017). The circadian profile of epilepsy improves seizure forecasting. Brain 140, 2169–2182. doi: 10.1093/brain/awx173
Kentish, S. J., Frisby, C. L., Kennaway, D. J., Wittert, G. A., and Page, A. J. (2013). Circadian variation in gastric vagal afferent mechanosensitivity. J. Neurosci. 33, 19238–19242. doi: 10.1523/JNEUROSCI.3846-13.2013
Khalsa, S. S., Adolphs, R., Cameron, O. G., Critchley, H. D., Davenport, P. W., Feinstein, J. S., et al. (2018). Interoception and mental health: a roadmap. Biol. Psychiatry Cogn. Neurosci. Neuroimag. 3, 501–513. doi: 10.1016/j.bpsc.2017.12.004
Kim, K., Ladenbauer, J., Babo-Rebelo, M., Buot, A., Lehongre, K., Adam, C., et al. (2019). Resting-state neural firing rate is linked to cardiac-cycle duration in the human cingulate and parahippocampal cortices. J. Neurosci. 39, 3676–3686. doi: 10.1523/JNEUROSCI.2291-18.2019
Kim, S. W., and Lee, B. I. (2009). Metabolic state, neurohormones, and vagal stimulation, not increased serotonin, orchestrate postprandial drowsiness. Biosci. Hypotheses 2, 422–427. doi: 10.1016/j.bihy.2009.07.008
Kishi, T., Tsumori, T., Ono, K., Yokota, S., Ishino, H., and Yasui, Y. (2000). Topographical organization of projections from the subiculum to the hypothalamus in the rat. J. Comp. Neurol. 419, 205–222. doi: 10.1002/(SICI)1096-9861(20000403)419:2<205::AID-CNE5>3.0.CO;2-0
Kishi, T., Tsumori, T., Ono, K., Yokota, S., Ishino, H., Yasui, Y., et al. (1990). Hippocampal pathway to the amygdala and stress ulcer development. Brain Res. Bull. 25, 691–695. doi: 10.1016/0361-9230(90)90111-K
Klausberger, T., Marton, L. F., Baude, A., Roberts, J. D., Magill, P. J., and Somogyi, P. (2004). Spike timing of dendrite-targeting bistratified cells during hippocampal network oscillations in vivo. Nat. Neurosci. 7, 41–47. doi: 10.1038/nn1159
Kreitlow, B. L., Li, W., and Buchanan, G. F. (2022). Chronobiology of epilepsy and sudden unexpected death in epilepsy. Front. Neurosci. 16:936104. doi: 10.3389/fnins.2022.936104
Krout, K. E., Kawano, J., Mettenleiter, T. C., and Loewy, A. D. (2002). CNS inputs to the suprachiasmatic nucleus of the rat. Neuroscience 110, 73–92. doi: 10.1016/s0306-4522(01)00551-6
Lacuey, N., Hampson, J. P., Harper, R. M., Miller, J. P., and Lhatoo, S. (2019). Limbic and paralimbic structures driving ictal central apnea. Neurology 92, e655–e669. doi: 10.1212/WNL.0000000000006920
Lacuey, N., Zonjy, B., Hampson, J. P., Rani, M. R. S., Zaremba, A., Sainju, R. K., et al. (2018). The incidence and significance of periictal apnea in epileptic seizures. Epilepsia 59, 573–582. doi: 10.1111/epi.14006
Lemon, N., Aydin-Abidin, S., Funke, K., and Manahan-Vaughan, D. (2009). Locus coeruleus activation facilitates memory encoding and induces hippocampal LTD that depends on β-adrenergic receptor activation. Cereb. Cortex 19, 2827–2837. doi: 10.1093/cercor/bhp065
Levichkina, E., Pigareva, M. L., Limanskaya, A., and Pigarev, I. N. (2022). Somatovisceral convergence in sleep-wake cycle: transmitting different types of information via the same pathway. Front. Netw. Physiol. 2:840565. doi: 10.3389/fnetp.2022.840565
Levichkina, E. V., Busygina, I. I., Pigareva, M. L., and Pigarev, I. N. (2021). The mysterious island: insula and its dual function in sleep and wakefulness. Front. Syst. Neurosci. 14:592660. doi: 10.3389/fnsys.2020.592660
Liang, X., Liang, X., Zhao, Y., Ding, Y., Zhu, X., Zhou, J., et al. (2024). Dysregulation of the suprachiasmatic nucleus disturbs the circadian rhythm and aggravates epileptic seizures by inducing hippocampal GABAergic dysfunction in C57BL/6 mice. J. Pineal Res. 76:e12993. doi: 10.1111/jpi.12993
Li, P., Fu, X., Smith, N. A., Ziobro, J., Curiel, J., Tenga, M. J., et al. (2017). Loss of CLOCK results in dysfunction of brain circuits underlying focal epilepsy. Neuron 96, 387–401.e6. doi: 10.1016/j.neuron.2017.09.044
Lisman, J. E., and Jensen, O. (2013). The θ-γ neural code. Neuron 77, 1002–1016. doi: 10.1016/j.neuron.2013.03.007
Liu, Y., McAfee, S. S., and Heck, D. H. (2017). Hippocampal sharp-wave ripples in awake mice are entrained by respiration. Sci. Rep. 7:8950. doi: 10.1038/s41598-017-09511-8
Li, X. L., Aou, S., Oomura, Y., Hori, N., Fukunaga, K., and Hori, T. (2002). Impairment of long-term potentiation and spatial memory in leptin receptor-deficient rodents. Neuroscience 113, 607–615. doi: 10.1016/S0306-4522(02)00162-8
Lockmann, A. L. V., Laplagne, D. A., Leão, R. N., and Tort, A. B. L. (2016). A respiration-coupled rhythm in the rat hippocampus independent of theta and slow oscillations. J. Neurosci. 36, 5338–5352. doi: 10.1523/JNEUROSCI.3452-15.2016
Lunardi, M. D. S., Sukys-Claudino, L., Guarnieri, R., Walz, R., and Lin, K. (2011). Seizure precipitants and inhibiting factors in mesial temporal lobe epilepsy. J. Neurol. Sci. 308, 21–24. doi: 10.1016/j.jns.2011.06.041
Lunardi, M. S., Lin, K., Mameniškienė, R., Beniczky, S., Bogacz, A., Braga, P., et al. (2016). Olfactory stimulation induces delayed responses in epilepsy. Epilepsy Behav. 61, 90–96. doi: 10.1016/j.yebeh.2016.05.022
Madden, M. B., Stewart, B. W., White, M. G., Krimmel, S. R., Qadir, H., Barrett, F. S., et al. (2022). A role for the claustrum in cognitive control. Trends Cogn. Sci. 26, 1133–1152. doi: 10.1016/j.tics.2022.09.006
Maier, N., and Kempter, R. (2017). "hippocampal sharp wave/ripple complexes—physiology and mechanisms," in studies in neuroscience. Psychol. Behav. Econ. 227-249. doi: 10.1007/978-3-319-45066-7_14
Majoie, H. J., Rijkers, K., Berfelo, M. W., Hulsman, J. A., Myint, A., Schwarz, M., et al. (2011). Vagus nerve stimulation in refractory epilepsy: effects on pro- and anti-inflammatory cytokines in peripheral blood. Neuroimmunomodulation 18, 52–56. doi: 10.1159/000315530
Malow, B. A., Foldvary-Schaefer, N., Vaughn, B. V., Selwa, L. M., Chervin, R. D., Weatherwax, K. J., et al. (2008). Treating obstructive sleep apnea in adults with epilepsy: a randomized pilot trial. Neurology 71, 572–577. doi: 10.1212/01.wnl.0000323927.13250.54
Malow, B. A., Lin, X., Kushwaha, R., and Aldrich, M. S. (1998). Interictal spiking increases with sleep depth in temporal lobe epilepsy. Epilepsia 39, 1309–1316. doi: 10.1111/j.1528-1157.1998.tb01329.x
Ma, M., Hua, X., Jia, C., Xiao, N., Zhang, L., Wei, L., et al. (2024). Tanshinone IIA regulates synaptic plasticity in Mg2+-free-induced epileptic hippocampal neurons via the PI3K/Akt signaling pathway. J. Integr. Neurosci. 23:61. doi: 10.31083/j.jin2303061
Manni, R., and Terzaghi, M. (2010). Comorbidity between epilepsy and sleep disorders. Epilepsy Res. 90, 171–177. doi: 10.1016/j.eplepsyres.2010.05.006
Marchi, N., Angelov, L., Masaryk, T., Fazio, V., Granata, T., Hernandez, N., et al. (2007). Seizure-promoting effect of blood-brain barrier disruption. Epilepsia 48, 732–742. doi: 10.1111/j.1528-1167.2007.00988.x
Markram, H., Toledo-Rodriguez, M., Wang, Y., Gupta, A., Silberberg, G., and Wu, C. (2004). Interneurons of the neocortical inhibitory system. Nat. Rev. Neurosci. 5, 793–807. doi: 10.1038/nrn1519
Marrosu, F., Serra, A., Maleci, A., Puligheddu, M., Biggio, G., and Piga, M. (2003). Correlation between GABA(a) receptor density and vagus nerve stimulation in individuals with drug-resistant partial epilepsy. Epilepsy Res. 55, 59–70. doi: 10.1016/s0920-1211(03)00107-4
Matos, H. C., Koike, B. D. V., Pereira, W. D. S., de Andrade, T. G., Castro, O. W., Duzzioni, M., et al. (2020). Rhythms of core clock genes and spontaneous locomotor activity in post-status epilepticus model of temporal lobe epilepsy. Front. Neurol. 11:327. doi: 10.3389/fneur.2018.0063
McGregor, G., Clements, L., Farah, A., Irving, A. J., and Harvey, J. (2018). Age-dependent regulation of excitatory synaptic transmission at hippocampal temporoammonic-CA1 synapses by leptin. Neurobiol. Aging 69, 76–93. doi: 10.1016/j.neurobiolaging.2018.05.007
Meletti, S., Giovannini, G., d'Orsi, G., Toran, L., Monti, G., Guha, R., et al. (2017). New-onset refractory status epilepticus with claustrum damage: definition of the clinical and neuroimaging features. Front. Neurol. 8:111. doi: 10.3389/fneur.2017.00111
Mendes, R. A. V., Zacharias, L. R., Ruggiero, R. N., Leite, J. P., Moraes, M. F. D., and Lopes-Aguiar, C. (2019). Hijacking of hippocampal–cortical oscillatory coupling during sleep in temporal lobe epilepsy. Epilepsy Behav. 121:106608. doi: 10.1016/j.yebeh.2019.106608
Miller, K. K., Hoffer, A., Svoboda, K. R., and Lupica, C. R. (1997). Cholecystokinin increases GABA release by inhibiting a resting K+ conductance in hippocampal interneurons. J. Neurosci. 17, 4994–5003. doi: 10.1523/JNEUROSCI.17-13-04994.1997
Mitra, A., Snyder, A. Z., Hacker, C. D., Pahwa, M., Tagliazucchi, E., and Laufs, H. (2016). Human cortical–hippocampal dialogue in wake and slow-wave sleep. Proc. Natl. Acad. Sci. 113, E6868–E6876
Mohanraj, R., Norrie, J., Stephen, L. J., Kelly, K., Hitiris, N., and Brodie, M. J. (2006). Mortality in adults with newly diagnosed and chronic epilepsy: a retrospective comparative study. Lancet Neurol. 5, 481–487. doi: 10.1016/S1474-4422(06)70448-3
Mohawk, J. A., Green, C. B., and Takahashi, J. S. (2012). Central and peripheral circadian clocks in mammals. Annu. Rev. Neurosci. 35, 445–462. doi: 10.1146/annurev-neuro-060909-153128
Montagne, A., Barnes, S. R., Sweeney, M. D., Halliday, M. R., Sagare, A. P., Zhao, Z., et al. (2015). Blood-brain barrier breakdown in the aging human hippocampus. Neuron. 85:296–302. doi: 10.1016/j.neuron.2014.12.032
Moser, M. B., Moser, E. I., Forrest, E., Andersen, P., and Morris, R. G. (1995). Spatial learning with a minislab in the dorsal hippocampus. Proc. Natl. Acad. Sci. USA 92, 9697–9701. doi: 10.1073/pnas.92.21.9697
Moult, P. R., and Harvey, J. (2011). NMDA receptor subunit composition determines the polarity of leptin-induced synaptic plasticity. Neuropharmacology 61, 924–936. doi: 10.1016/j.neuropharm.2011.06.021
Munari, C., Kahane, P., Tassi, L., Francione, S., Hoffmann, D., Lo Russo, G., et al. (1993). Intracerebral low frequency electrical stimulation: a new tool for the definition of the "epileptogenic area"? Acta Neurochir. 58, 181–185. doi: 10.1007/978-3-7091-9297-9_42
Mure, L. S. (2021). Intrinsically photosensitive retinal ganglion cells of the human retina. Front. Neurol. 12:636330. doi: 10.3389/fneur.2021.636330
Mutoh, T., Shibata, S., Korf, H. W., and Okamura, H. (2003). Melatonin modulates the light-induced sympathoexcitation and vagal suppression with participation of the suprachiasmatic nucleus in mice. J. Physiol. 547, 317–332. doi: 10.1113/jphysiol.2002.028001
Nagaraja, D., and Chand, R. P. (1984). Eating epilepsy. Clin. Neurol. Neurosurg. 86, 95–99. doi: 10.1016/0303-8467(84)90072-6
Narikiyo, K., Mizuguchi, R., Ajima, A., Shiozaki, M., Hamanaka, H., Johansen, J. P., et al. (2020). The claustrum coordinates cortical slow-wave activity. Nat. Neurosci. 23, 741–753. doi: 10.1038/s41593-020-0625-7
Neafsey, E. J. (1990). Prefrontal cortical control of the autonomic nervous system: anatomical and physiological observations. Prog. Brain Res. 85, 147–165. doi: 10.1016/S0079-6123(08)61575-2
Nemeroff, C. B., Mayberg, H. S., Krahl, S. E., McNamara, J., Frazer, A., Henry, T. R., et al. (2006). VNS therapy in treatment-resistant depression: clinical evidence and putative neurobiological mechanisms. Neuropsychopharmacology 31, 1345–1355. doi: 10.1038/sj.npp.1301082
Nieuwenhuys, R. (2012). The insular cortex: a review. Prog. Brain Res. 195, 123–163. doi: 10.1016/B978-0-444-53860-4.00007-6
Niu, R., Guo, X., Wang, J., Li, Y., Zhang, Q., Chen, S., et al. (2025). The hidden rhythms of epilepsy: exploring biological clocks and epileptic seizure dynamics. Acta Epileptologica 7:1. doi: 10.1186/s42494-024-00197-w
Nobili, L., Proserpio, P., Combi, R., Provini, F., Plazzi, G., Bisulli, F., et al. (2012). Distinctive polysomnographic traits in nocturnal frontal lobe epilepsy. Epilepsia 53, 1178–1184. doi: 10.1111/j.1528-1167.2012.03502.x
Nokia, M. S., and Penttonen, M. (2022). Rhythmic memory consolidation in the hippocampus. Front. Neural Circuits 16:885684. doi: 10.3389/fncir.2022.885684
Ogren, J. A., Wilson, C. L., Bragin, A., Lin, J. J., Salamon, N., Dutton, R. A., et al. (2009). Three-dimensional surface maps link local atrophy and fast ripples in human epileptic hippocampus. Ann. Neurol. 66, 783–791. doi: 10.1002/ana.21703
Olney, J. W., Collins, R. C., and Sloviter, R. S. (1986). Excitotoxic mechanisms of epileptic brain damage. Adv. Neurol. 44, 857–877.
Ongchuan Martin, S., Sadeghifar, F., Snively, B. M., Alexander, H., Kimball, J., Conner, K., et al. (2022). Positive anxiety or depression screen despite ongoing antidepressant prescription in people with epilepsy: a large cross-sectional analysis. Epilepsy Behav. Rep. 20:100572. doi: 10.1016/j.ebr.2022.100572
Ongür, D., An, X., and Price, J. L. (1998). Prefrontal cortical projections to the hypothalamus in macaque monkeys. J. Comp. Neurol. 401, 480–505. doi: 10.1002/(SICI)1096-9861(19981130)401:4<480::AID-CNE4>3.0.CO;2-F
Ongür, D., and Price, J. L. (2000). The organization of networks within the orbital and medial prefrontal cortex of rats, monkeys, and humans. Cereb. Cortex 10, 206–219. doi: 10.1093/cercor/10.3.206
Oomura, Y., Hori, N., Shiraishi, T., Fukunaga, K., Takeda, H., Tsuji, M., et al. (2006). Leptin facilitates learning and memory performance and enhances hippocampal CA1 long-term potentiation and CaMK II phosphorylation in rats. Peptides 27, 2738–2749. doi: 10.1016/j.peptides.2006.07.001
Parra, J., Kalitzin, S. N., Iriarte, J., Velazquez, J. L., Falloure, J. M., Dubeau, F., et al. (2003). Gamma-band phase clustering and photosensitivity: is there an underlying mechanism common to photosensitive epilepsy and visual perception? Brain 126, 1164–1172. doi: 10.1093/brain/awg109
Pavlova, M. K., Shea, S. A., and Bromfield, E. B. (2004). Day/night patterns of focal seizures. Epilepsy Behav. 5, 44–49. doi: 10.1016/j.yebeh.2003.10.013
Petrovich, G. D., Canteras, N. S., and Swanson, L. W. (2001). Combinatorial amygdalar inputs to hippocampal domains and hypothalamic behavior systems. Brain Res. Brain Res. Rev. 38, 247–289. doi: 10.1016/S0165-0173(01)00080-7
Pigarev, I. N. (1994). Neurons of visual cortex respond to visceral stimulation during slow wave sleep. Neuroscience 62, 1237–1243. doi: 10.1016/0306-4522(94)90355-7
Pigarev, I. N., Bagaev, V. A., Levichkina, E. V., Fedorov, G. O., and Busigina, I. I. (2013). Cortical visual areas process intestinal information during slow-wave sleep. Neurogastroenterol. Motil. 25, 268–275. doi: 10.1111/nmo.12052
Pigarev, I. N., and Pigareva, M. L. (2013). Sleep, emotions, and visceral control. Hum. Physiol. 39, 590–601. doi: 10.1134/S036211971306008X
Pigarev, I. N., Pigareva, M. L., and Levichkina, E. (2020). Probable mechanism of antiepileptic effect of the vagus nerve stimulation in the context of the recent results in sleep research. Front. Neurosci. 14:160. doi: 10.3389/fnins.2020.00160
Purnell, B. S., Thijs, R. D., and Buchanan, G. F. (2018). Dead in the Night: Sleep-Wake and Time-Of-Day Influences on Sudden Unexpected Death in Epilepsy. Front. Neurol. 9:1079. doi: 10.3389/fneur.2018.01079
Quadt, L., Critchley, H. D., and Garfinkel, S. N. (2018). The neurobiology of interoception in health and disease. Ann. N. Y. Acad. Sci. 1428, 112–128. doi: 10.1111/nyas.13915
Quigley, K. S., Kanoski, S., Grill, W. M., Barrett, L. F., and Tsakiris, M. (2021). Functions of Interoception: from energy regulation to experience of the self. Trends Neurosci. 44, 29–38. doi: 10.1016/j.tins.2020.09.008
Radna, R. J., and MacLean, P. D. (1981). Vagal elicitation of respiratory-type and other unit responses in basal limbic structures of squirrel monkeys. Brain Res. 213, 45–61. doi: 10.1016/0006-8993(81)91247-6
Rebollo, I., Devauchelle, A. D., Béranger, B., and Tallon-Baudry, C. (2018). Stomach-brain synchrony reveals a novel, delayed-connectivity resting-state network in humans. eLife 7:e33321. doi: 10.7554/eLife.33321
Rembado, I., Song, W., Su, D. K., Mayhew, M. K., Aslan, A. T., Baker, K. B., et al. (2021). Cortical responses to vagus nerve stimulation are modulated by brain state in nonhuman primates. Cereb. Cortex 31, 5289–5307. doi: 10.1093/cercor/bhab158
Ruby, N. F., Fernandez, F., Garrett, A., and Franken, P. (2013). Spatial memory and long-term object recognition are impaired by circadian arrhythmia and restored by the GABAA antagonist pentylenetetrazole. PLoS One 8:e72433. doi: 10.1371/journal.pone.0072433
Ruby, N. F., Hwang, C. E., Wessells, C., Bolaños, C. A., and Heller, H. C. (2008). Hippocampal-dependent learning requires a functional circadian system. Proc. Natl. Acad. Sci. USA 105, 15593–15598. doi: 10.1073/pnas.0808259105
Sackeim, H. A., Rush, A. J., George, M. S., Marangell, L. B., Husain, M. M., Nahas, Z., et al. (2001). Vagus nerve stimulation (VNS) for treatment-resistant depression: efficacy, side effects, and predictors of outcome. Neuropsychopharmacology 25, 713–728. doi: 10.1016/S0893-133X(01)00271-8
Salama, H., Salama, A., Oscher, L., Jallo, G. I., and Shimony, N. (2024). The role of neuromodulation in the management of drug-resistant epilepsy. Neurol. Sci. 45, 4243–4268. doi: 10.1007/s10072-024-07513-9
Salzman, E., Vidyasagar, T. R., and Creutzfeldt, O. D. (1993). Functional comparison of neuronal properties in the primate posterior hippocampus and parahippocampus (area TF/TH) during different behavioral paradigms involving memory and selective attention. Behav. Brain Res. 53, 133–149. doi: 10.1016/S0166-4328(05)80273-6
Sammaritano, M., Gigli, G. L., and Gotman, J. (1991). Interictal spiking during wakefulness and sleep and the localization of foci in temporal lobe epilepsy. Neurology 41, 290–297. doi: 10.1212/wnl.41.2_part_1.290
Saper, C. B. (2002). The central autonomic nervous system: conscious visceral perception and autonomic pattern generation. Annu. Rev. Neurosci. 25, 433–469. doi: 10.1146/annurev.neuro.25.032502.111311
Saper, C. B., and Fuller, P. M. (2017). Wake-sleep circuitry: an overview. Curr. Opin. Neurobiol. 44, 186–192. doi: 10.1016/j.conb.2017.03.021
Saper, C. B., Scammell, T. E., and Lu, J. (2005). Hypothalamic regulation of sleep and circadian rhythms. Nature 437, 1257–1263. doi: 10.1038/nature04284
Semah, F., Picot, M. C., Adam, C., Broglin, D., Arzimanoglou, A., Vignal, J. P., et al. (1998). Is the underlying cause of epilepsy a major prognostic factor for recurrence? Neurology 51, 1256–1262. doi: 10.1212/wnl.51.5.1256
Shanley, L. J., Irving, A. J., and Harvey, J. (2001). Leptin enhances NMDA receptor function and modulates hippocampal synaptic plasticity. J. Neurosci. 21:RC186. doi: 10.1523/JNEUROSCI.21-24-j0001.2001
Sheybani, L., Vivekananda, U., Rodionov, R., Diehl, B., Chowdhury, F. A., McEvoy, A. W., et al. (2023). Wake slow waves in focal human epilepsy impact network activity and cognition. Nat. Commun. 14:7397. doi: 10.1038/s41467-023-42971-3
Shouse, M. N., da Silva, A. M., and Sammaritano, M. (1996). Circadian rhythm, sleep, and epilepsy. J. Clin. Neurophysiol. 13, 32–50. doi: 10.1097/00004691-199601000-00004
Sivathamboo, S., Perucca, P., Velakoulis, D., Jones, N. C., Goldin, J., Kwan, P., et al. (2019). Sleep-disordered breathing in epilepsy: epidemiology, mechanisms, and treatment. Sleep 42:zsz015. doi: 10.1093/sleep/zsz015
Sloviter, R. S. (1987). Decreased hippocampal inhibition and a selective loss of interneurons in experimental epilepsy. Science 235, 73–76. doi: 10.1126/science.2879352
Snider, K. H., Sullivan, K. A., and Obrietan, K. (2018). Circadian regulation of hippocampal-dependent memory: circuits, synapses, and molecular mechanisms. Neural Plast. 2018:7292540. doi: 10.1155/2018/7292540
Sohanian Haghighi, H., and Markazi, A. H. D. (2019). Dynamic origin of spike and wave discharges in the brain. NeuroImage 203:116108. doi: 10.1016/j.neuroimage.2019.116108
Somogyi, P., Hodgson, A., Smith, A., Nunzi, M. G., Gorio, A., and Wu, J. (1984). Different populations of GABAergic neurons in the visual cortex and hippocampus of cat contain somatostatin-or cholecystokinin-immunoreactive material. J. Neurosci. 4, 2590–2603. doi: 10.1523/JNEUROSCI.04-10-02590.1984
Sotiriou, E., Papatheodoropoulos, C., and Angelatou, F. (2005). Differential expression of γ-aminobutyric acid-a receptor subunits in rat dorsal and ventral hippocampus. J. Neurosci. Res. 82, 690–700. doi: 10.1002/jnr.20670
Sperling, M. R., Harris, A., Nei, M., English, W., and Weintraub, D. (2005). Mortality after epilepsy surgery. Epilepsia 46, 49–53. doi: 10.1111/j.1528-1167.2005.00410.x
Staba, R. J., Frighetto, L., Behnke, E. J., Soda, T., Wilson, C. L., Bragin, A., et al. (2007). Increased fast ripple to ripple ratios correlate with reduced hippocampal volumes and neuron loss in temporal lobe epilepsy patients. Epilepsia 48, 2130–2138. doi: 10.1111/j.1528-1167.2007.01225.x
Stark, E., Levi, A., and Rotstein, H. G. (2022). Network resonance can be generated independently at distinct levels of neuronal organization. PLoS Comput. Biol. 18:e1010364. doi: 10.1371/journal.pcbi.1010364
Strange, B. A., Witter, M. P., Lein, E. S., and Moser, E. I. (2014). Functional organization of the hippocampal longitudinal axis. Nat. Rev. Neurosci. 15, 655–669. doi: 10.1038/nrn3785
Suarez, A. N., Hsu, T. M., Liu, C. M., Kanoski, S. E., and Knight, R. (2018). Gut vagal sensory signaling regulates hippocampus function through multi-order pathways. Nat. Commun. 9:2181. doi: 10.1038/s41467-018-04639-1
Swanson, L. W. (2000). Cerebral hemisphere regulation of motivated behavior. Brain Res. 886, 113–164. doi: 10.1016/S0006-8993(00)02905-X
Tai, B. W. S., Dawood, T., Macefield, V. G., and Yiallourou, S. R. (2023). The association between sleep duration and muscle sympathetic nerve activity. Clin. Auton. Res. 33, 647–657. doi: 10.1007/s10286-023-00965-7
Tamura, R., Ono, T., Fukuda, M., and Nishijo, H. (1991). Role of monkey hippocampus in recognition of food and nonfood. Brain Res. Bull. 27, 457–461. doi: 10.1016/0361-9230(91)90142-7
Téllez-Zenteno, J. F., and Hernández-Ronquillo, L. (2012). A review of the epidemiology of temporal lobe epilepsy. Epilepsy Res. Treat. 2012:630853. doi: 10.1155/2012/630853
Téllez-Zenteno, J. F., Matijevic, S., and Wiebe, S. (2005). Somatic comorbidity of epilepsy in the general population in Canada. Epilepsia 46, 1955–1962. doi: 10.1111/j.1528-1167.2005.00344.x
Thierry, A. M., Gioanni, Y., Dégenètais, E., and Glowinski, J. (2000). Hippocampo-prefrontal cortex pathway: anatomical and electrophysiological characteristics. Hippocampus 10, 411–419. doi: 10.1002/1098-1063(2000)10:4<411::AID-HIPO7>3.0.CO;2-A
Trinder, J., Kleiman, J., Carrington, M., Smith, S., Breen, S., Tan, N., et al. (2001). Autonomic activity during human sleep as a function of time and sleep stage. J. Sleep Res. 10, 253–264. doi: 10.1046/j.1365-2869.2001.00263.x
Tsetsenis, T., Broussard, J. I., and Dani, J. A. (2023). Dopaminergic regulation of hippocampal plasticity, learning, and memory. Front. Behav. Neurosci. 16:1092420. doi: 10.3389/fnbeh.2022.1092420
van Buren, J. M., and Ajmone-Marsan, C. (1960). A correlation of autonomic and EEG components in temporal lobe epilepsy. Arch. Neurol. 3, 683–703. doi: 10.1001/archneur.1960.00450060071009
Vespa, S., Heyse, J., Stumpp, L., Liberati, G., Ferrao Santos, S., Rooijakkers, H., et al. (2021). Vagus nerve stimulation elicits sleep EEG desynchronization and network changes in responder patients in epilepsy. Neurotherapeutics 18, 2623–2638. doi: 10.1007/s13311-021-01124-4
Vidyasagar, T. R. (2013). Reading into neuronal oscillations in the visual system: implications for developmental dyslexia. Front. Hum. Neurosci. 7:811. doi: 10.3389/fnhum.2013.00811
Vidyasagar, T. R., and Levichkina, E. (2019). An integrated neuronal model of claustral function in timing the synchrony between cortical areas. Front. Neural Circuits 13:3. doi: 10.3389/fncir.2019.00003
Vidyasagar, T. R., Levichkina, E., and Pigarev, I. N. (2022). “Hippocampal cells synchronize to visceral activity: implications for seizures.” In: Australasian Neuroscience Society Conference, Melbourne, Australia, 5–7 December 2022.
Vidyasagar, T. R., Salzmann, E., and Creutzfeldt, O. D. (1991). Unit activity in the hippocampus and the parahippocampal temporobasal association cortex related to memory and complex behaviour in the awake monkey. Brain Res. 544, 269–278. doi: 10.1016/0006-8993(91)90064-3
Vilella, L., Lacuey, N., Hampson, J. P., Rani, M. R. S., Sainju, R. K., Friedman, D., et al. (2019). Incidence, recurrence, and risk factors for peri-ictal central apnea and sudden unexpected death in epilepsy. Front. Neurol. 10:166. doi: 10.3389/fneur.2019.00166
Vonck, E. J., and Larsen, L. E. (2018). “Vagus nerve stimulation: mechanism of action” in Neuromodulation: Comprehensive textbook of principles, technologies, and therapies. 2nd edn. Eds. E. Krames, P. H. Peckham, A. R. Rezai (Amsterdam, Netherlands: Elsevier), 211–220.
Wang, G. J., Yang, J., Volkow, N. D., Telang, F., Ma, Y., Zhu, W., et al. (2006). Gastric stimulation in obese subjects activates the hippocampus and other regions involved in brain reward circuitry. Proc. Natl. Acad. Sci. USA 103, 15641–15645. doi: 10.1073/pnas.0601977103
Wang, Y., Wang, X., Mo, J., Sang, L., Zhao, B., Zhang, C., et al. (2019). Symptomatogenic zone and network of oroalimentary automatisms in mesial temporal lobe epilepsy. Epilepsia 60, 1150–1159. doi: 10.1111/epi.15457
Waris, A., Siraj, M., Khan, A., Lin, J., Asim, M., and Alhumaydh, F. A. (2024). A comprehensive overview of the current status and advancements in various treatment strategies against epilepsy. ACS Pharmacol. Transl. Sci. 7, 3729–3757. doi: 10.1021/acsptsci.4c00494
Wei, Y., Ullah, G., and Schiff, S. J. (2014). Oxygen and seizure dynamics: II. Computational modeling. J. Neurophysiol. 112, 213–223. doi: 10.1152/jn.00541.2013
Whissell, P. D., Cajanding, J. D., Fogel, N., and Kim, J. C. (2015). Comparative density of CCK- and PV-GABA cells within the cortex and hippocampus. Front. Neuroanat. 9:124. doi: 10.3389/fnana.2015.00124
Wu, K., Han, W., and Lu, W. (2022). Sleep and wake cycles dynamically modulate hippocampal inhibitory synaptic plasticity. PLoS Biol. 20:e3001812. doi: 10.1371/journal.pbio.3001812
Wu, Y., Song, L., Wang, X., Li, N., Zhan, S., Rong, P., et al. (2022). Transcutaneous vagus nerve stimulation could improve the effective rate on the quality of sleep in the treatment of primary insomnia: a randomized control trial. Brain Sci. 12:1296. doi: 10.3390/brainsci12101296
Yap, J. Y. Y., Keatch, C., Lambert, E., Woods, W., Stoddart, P. R., and Kameneva, T. (2020). Critical review of transcutaneous vagus nerve stimulation: challenges for translation to clinical practice. Front. Neurosci. 14:284. doi: 10.3389/fnins.2020.00284
Yildiz, F. G., Tezer, F. I., Saygi, S., and Oguz, K. K. (2015). Temporal lobe epilepsy is a predisposing factor for sleep apnea: a questionnaire study in video-EEG monitoring unit. Epilepsy Behav. 48, 1–3. doi: 10.1016/j.yebeh.2015.05.019
Zanchetti, A., Wang, S. C., and Moruzzi, G. (1952). The effect of vagal afferent stimulation on the EEG pattern of the cat. Electroencephalogr. Clin. Neurophysiol. 4, 357–361. doi: 10.1016/0013-4694(52)90064-3
Zanzmera, P., Shukla, G., Gupta, A., Singh, H., Goyal, V., Srivastava, A., et al. (2012). Markedly disturbed sleep in medically refractory compared to controlled epilepsy: a clinical and polysomnography study. Seizure 21, 487–490. doi: 10.1016/j.seizure.2012.04.005
Keywords: vagus, epilepsy, circadian rhythm, Hippocampus, sleep, ipRGC (intrinsically photosensitive retinal ganglion cells)
Citation: Levichkina E, Grayden DB, Petrou S, Cook MJ and Vidyasagar TR (2025) Sleep links hippocampal propensity for epileptiform activity to its viscerosensory inputs. Front. Neurosci. 19:1559529. doi: 10.3389/fnins.2025.1559529
Edited by:
Vitor Engracia Valenti, São Paulo State University, BrazilReviewed by:
Muhammad Asim, Stanford University, United StatesRohan Kandasamy, University College London, United Kingdom
Copyright © 2025 Levichkina, Grayden, Petrou, Cook and Vidyasagar. This is an open-access article distributed under the terms of the Creative Commons Attribution License (CC BY). The use, distribution or reproduction in other forums is permitted, provided the original author(s) and the copyright owner(s) are credited and that the original publication in this journal is cited, in accordance with accepted academic practice. No use, distribution or reproduction is permitted which does not comply with these terms.
*Correspondence: Trichur R. Vidyasagar, dHJ2QHVuaW1lbGIuZWR1LmF1; Ekaterina Levichkina, ZWxlQHVuaW1lbGIuZWR1LmF1